- 1Plant and Microbial Biology Department, University of California, Berkeley, Berkeley, CA, United States
- 2Instituto de Ciências Biomédicas de Abel Salazar, Universidade do Porto, Porto, Portugal
- 3I3S - Instituto de Investigação e Inovação em Saúde, Porto, Portugal
Cell death occurs in all domains of life. While some cells die in an uncontrolled way due to exposure to external cues, other cells die in a regulated manner as part of a genetically encoded developmental program. Like other eukaryotic species, fungi undergo programmed cell death (PCD) in response to various triggers. For example, exposure to external stress conditions can activate PCD pathways in fungi. Calcium redistribution between the extracellular space, the cytoplasm and intracellular storage organelles appears to be pivotal for this kind of cell death. PCD is also part of the fungal life cycle, in which it occurs during sexual and asexual reproduction, aging, and as part of development associated with infection in phytopathogenic fungi. Additionally, a fungal non-self-recognition mechanism termed heterokaryon incompatibility (HI) also involves PCD. Some of the molecular players mediating PCD during HI show remarkable similarities to major constituents involved in innate immunity in metazoans and plants. In this review we discuss recent research on fungal PCD mechanisms in comparison to more characterized mechanisms in metazoans. We highlight the role of PCD in fungi in response to exogenic compounds, fungal development and non-self-recognition processes and discuss identified intracellular signaling pathways and molecules that regulate fungal PCD.
Introduction
We have all heard the phrase “death is a part of life,” which most often is referred to in connection with the human lifespan. However, this statement can be applied to all organisms when we think of death in a broader sense, i.e., cell death. Many cells die in a regulated manner controlled by an intracellular process termed programmed cell death (PCD). In general, some form of PCD occurs in all domains of life, from bacteria to higher eukaryotes (Engelberg-Kulka et al., 2006; Fuchs and Steller, 2011; van Doorn, 2011). In animals, the term PCD is mostly associated with developmental processes such as modeling of organs, regulation of cell number or deletion of certain macrostructures and removal of defective and potentially harmful cells (Fuchs and Steller, 2011). At the cellular level PCD is often mediated by an apoptotic mechanism associated with the externalization of phosphatidylserine to the outer leaflet of the plasma membrane, DNA fragmentation, cytochrome C release from mitochondria and caspase activation (Fuchs and Steller, 2015). In this review we highlight the role of PCD in fungi and summarize the knowledge on molecular mediators of regulated death in these organisms, with a special emphasis on filamentous species (Figure 1).
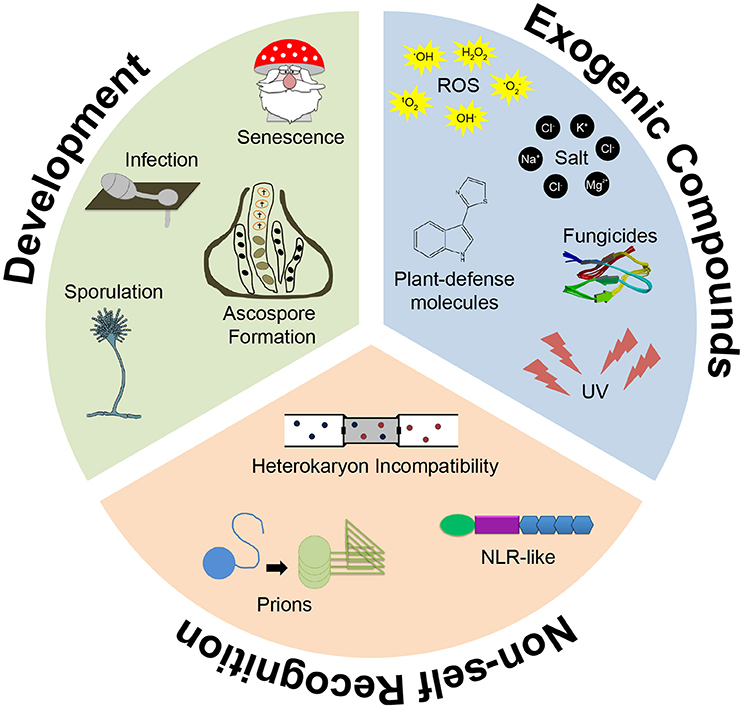
Figure 1. Programmed Cell Death (PCD) in filamentous fungi. PCD has been reported to occur in filamentous fungi in response to various exogenic compounds, such as plant defense molecules (e.g., camalexin), toxins and fungicides (e.g., Penicillium AntiFungal protein), during non-self-recognition and heterokaryon incompatibility and during developmental programs throughout the fungal life cycle, including morphogenesis associated plant infection.
General Considerations on Cell Death
There are many ways for a cell to die. Death can occur in response to physicochemical and mechanical triggers or it can occur in a controlled way by a genetically encoded apparatus. Classically, there have been three main categories of cell death: autophagy, apoptosis, and necrosis. Apoptosis and autophagy are genetically regulated PCD reactions that allow the orderly degradation and recycling of cellular components whereas necrosis has been classically defined as a more abrupt type of death driven by physical or chemical injuries. Advances in research on cell death have revealed an immense complexity of these processes, which has blurred the lines between what was traditionally defined as uncontrolled vs. controlled death. Additional forms of programmed cell death have been defined including the finding that cells can execute necrosis in a programmed fashion (necroptosis) or die in a regulated manner in response to an infection caused by intracellular pathogens (pyroptosis). To help researchers to discriminate and define different aspects of cell death the Nomenclature Committee on Cell Death (NCCD) has released recommendations for the classification of cell death to bring consensus in the field (Kroemer et al., 2005, 2009; Galluzzi et al., 2012, 2015).
The concept of cell death as a programmed event was initially suggested in the 19th century, when Carl Vogt described cell death during amphibian metamorphosis (Vogt, 1842; Clarke and Clarke, 1996). Ever since, PCD in multicellular metazoans was generally referred to as a developmental strategy to remove unwanted cells and make way for cellular remodeling and differentiation without causing an inflammatory response. The first description of apoptosis dates back to the early 1970s when it was defined as a “mechanism of controlled cell deletion, which appears to play a complementary but opposite role to mitosis in the regulation of animal cell populations” (Kerr et al., 1972). Subsequent genetic studies in the nematode Caenorhabditis elegans identified genes dedicated to the developmental death program and its control (Ellis and Horvitz, 1986). Some of these genes are homologous to mammalian genes (Yuan et al., 1993; Hengartner and Horvitz, 1994) and their roles in cell death are largely conserved in metazoans. The importance of PCD correlates with the complexity of the organism. For example, PCD-deficient nematodes have a normal lifespan in the laboratory, even though they have about 15% more cells and function less well than wild-type worms (Ellis et al., 1991). However, PCD-deficient flies die early in development (White et al., 1994), and mice with inhibited apoptosis die perinatally with a vast excess of cells in their central nervous system (Kuida et al., 1996). Over the past two decades, substantial progress has been made in the understanding of the intracellular molecular mechanisms of PCD and its control in metazoans (reviewed in Oberst et al., 2008). Key enzymes involved are specific proteases (caspases) that cleave target proteins at specific aspartic acid residues. Caspases exist as inactive precursors within cells and their activation occurs by conserved mechanisms (oligomerization, cleavage of regulatory pro-domains, and/or dissociation from endogenous inhibitors) and are subject to strict cellular regulation. Limited proteolysis of certain substrates by caspases activate specific downstream signaling pathways ensuring that the cellular components are degraded in a controlled manner to avoid damage of surrounding tissue (Lamkanfi et al., 2007; Pop and Salvesen, 2009; Julien and Wells, 2017; Man et al., 2017). Soon after caspases were identified as major regulators of cell death in metazoans, a hunt for homologous peptidases in other kingdoms was initiated, with distantly related caspase-like proteins (metacaspases) identified in plants, fungi, and protozoa (Uren et al., 2000). However, although metacaspases seem to play some role during cell death in non-metazoans, it is still unclear if they function in comparable fashion as caspases to regulate the transition to cell death (Tsiatsiani et al., 2011).
The Multiple Manifestations of PCD in Fungi
In the unicellular yeast, Saccharomyces cerevisiae, PCD was reported in the 1990s in a temperature-sensitive mutant of cdc48 (cdc48S565G), which showed an endoplasmic reticulum-localized ATPase, externalization of phosphatidylserine to the outer leaflet of the plasma membrane, and DNA fragmentation and condensation under non-permissive conditions (Madeo et al., 1997). PCD has also been reported in the fission yeast Schizosaccharomyces pombe, where it has been associated with perturbation of lipid metabolism, defective DNA replication/mitotic entry, and chronological/replicative aging (Low and Yang, 2008). Despite many additional observations of PCD-like phenotypes in unicellular fungi (Falcone and Mazzoni, 2016), the degree of similarity between fungal and metazoan cell death is still controversial. Coined as phenoptosis by Skulachev (1999), cell death of unicellular organisms means organismal death and therefore lacks a developmental aspect. However, the terms apoptotic-like cell death or programmed cell death have still been employed when dying fungal cells exhibit morphological and biochemical features of PCD including DNA fragmentation and condensation, cell shrinkage, phosphatidylserine exposure, reactive oxygen species (ROS) production, mitochondrial membrane depolarization, reduced glutathione (GSH) efflux and caspase-like activity (Almeida et al., 2008; Low and Yang, 2008; Sharon et al., 2009; Carmona-Gutierrez et al., 2010a; Ramsdale, 2012). Furthermore, cells of yeast colonies form social communities (Palkova and Vachova, 2006) that undergo a differentiation-like program involving regulated cell death (Vopalenska et al., 2005). For example, if a dead zone at the colony center is removed, growth reduction occurs in cells at the periphery of the colony, suggesting that cell death occurring at the center of the colony supports growth of the whole colony (Longo et al., 2005; Vopalenska et al., 2005; Sukhanova et al., 2012). It has been hypothesized that cell density is the threshold factor that determines whether DNA mutational load and the nutritional environment are at a critical value, leading to cell death of parts of the population (Longo et al., 2005; Severin et al., 2008). In line with these findings, both chronological and replicative aging result in the appearance of apoptotic-like markers in S. cerevisiae and S. pombe (Laun et al., 2001; Herker et al., 2004; Low and Yang, 2008). For example, in chronological aging, a small subpopulation of S. cerevisiae cells are able to regrow after more than 90% of the population has died (Fabrizio et al., 2004), possibly due to the release of substances from dying cells that alter the composition of the growth medium (Longo et al., 2005). A recent report also showed the involvement of programmed cell death in yeast gametogenesis. In a process called programmed nuclear destruction cells develop aborted meiotic products to control the spore number, which apparently benefits sibling cells (Eastwood et al., 2012). PCD has also been implicated as a key evolutionary event during the transition from uni- to multicellularity in yeast cells. For example, when the ability to form primitive multicellular structures was selected for in yeast cells (through a sedimentation strategy), the development of cell clusters leading to a snowflake-type phenotype was associated with high rates of cell death. Apoptotic cells in the clusters may generate “weak links” that allow small branches to separate from large clusters which can increase a cluster's fecundity (Ratcliff et al., 2012).
PCD during Reproduction
Due to their multicellular nature and higher complexity, PCD plays a more diverse role in filamentous fungi as compared to unicellular yeast (Shlezinger et al., 2012; Figure 1 and Table 1). In Aspergillus nidulans, asexual sporulation was associated with apoptotic-like cell death that included caspase activity (Thrane et al., 2004). In Podospora anserina, disruption of the two metacaspases (highly similar to caspases; Uren et al., 2000, see section Metacaspases) led to defects in sexual spore (ascospore) formation (Hamann et al., 2007). During ascus/ascospore maturation in Coniochaete tetrasperma, the number of ascospores per asci is reduced from 8 to 4 by means of a regulated cell death process (Raju and Perkins, 2000), which also occurs in Neurospora species and is controlled by the selfish genetic element known as Spore killer (Sk) (Raju, 1979; Turner and Perkins, 1979; Hammond et al., 2012). In Coprinopsis cinereus, cytological indicators of cell death were observed in immature basidiospores of meiotic mutants whose nuclei arrested after meiotic metaphase I (Lu et al., 2003) as well as prior to basidial differentiation in Agaricus bisporus (Umar and Griensven Van, 1997).
PCD during Aging
Podospora anserina undergoes a senescence-type form of cell death characterized by a reorganization of the mitochondrial DNA, decrease in mycelium growth rate and increase in pigmentation and death of peripheral hyphae (Osiewacz, 2011; Bernhardt et al., 2014). Podospora anserina therefore has been used as a model for studying aging (Rizet, 1953). Senescence is associated with cytochrome c oxidase (complex IV) activity of the mitochondrial respiratory chain (Dufour et al., 2000; Osiewacz and Borghouts, 2000; Lorin et al., 2006). Although for many years the mitochondrial accumulation of ROS has been considered the key event underlying biological aging and lifespan in P. anserina, recent data indicates that other signals, including the mitochondrial quality control are also implicated in this process (Osiewacz and Bernhardt, 2013).
PCD and Non-self-recognition
PCD has also been associated with non-self-recognition in a number of filamentous fungi (Figure 1). Non-self-recognition in filamentous fungi is termed vegetative or heterokaryon incompatibility (HI) and has been described in both ascomycete and basidiomycete species (Rayner, 1996; Worrall, 1997; Saupe, 2000; Glass and Kaneko, 2003; Glass and Dementhon, 2006; Van der Nest et al., 2014). Non-self-recognition can occur at distance resulting in hyphal inhibition or as a consequence of hyphal or cell fusion between genetically different colonies, thus forming a heterokaryon where nuclei with different genetic backgrounds are present in the same cell. If nuclei within the heterokaryon are genetically dissimilar at the so called het loci, heterokaryotic parts of the colony are compartmentalized and cell death reactions are triggered, leading to a rejection of stable heterokaryon formation (Glass and Kaneko, 2003; Glass and Dementhon, 2006). HI has been shown to restrict viral transfer via hyphal fusion between genetically different fungal colonies and to prevent resource appropriation (Debets et al., 1994; Van Diepeningen et al., 1997; Debets and Griffiths, 1998; Biella et al., 2002).
When progeny or transformants have a duplication of incompatible het alleles or when incompatible heterokaryons are forced using auxotrophic markers, the heterokaryotic colony shows a macroscopic phenotype of slow growth and diminished conidiation, together with hyphal compartmentalization, lipid droplet accumulation, vacuolization and cell death (Perkins, 1975; Jacobson et al., 1998; Glass and Dementhon, 2006; Hutchison et al., 2009). HI is associated with the production of reactive oxygen species (ROS) and activation of phosphatidylinositol- and calcium (Ca2+)-related genes (Hutchison et al., 2009). Furthermore, nuclear DNA condensation and fragmentation, plasma membrane shrinkage, vesicle formation and internalization of vital dyes occur, drawing parallels between HI reactions and apoptosis in metazoans (Marek et al., 2003; Glass and Dementhon, 2006; Hutchison et al., 2009).
In spite of the genetic identification and characterization of various genes inducing HI in filamentous fungi, the molecular basis of the death reaction are still not well understood. A common feature among proteins inducing HI in filamentous fungi is the presence of a ~150 aa region termed the HET domain (PF06985, named after HETerokaryon incompatibility), suggesting that HI reactions induced by different HET domain proteins have similar downstream PCD pathways. This assumption is corroborated by the fact that the deletion of a single transcription factor, vib-1 (vegetative incompatibility blocked-1), suppresses the HI reaction mediated by genetic differences at all molecularly characterized het loci in Neurospora crassa (Dementhon et al., 2006). Additionally, in P. anserina, autophagy was also associated with HI (Pinan-Lucarre et al., 2003, 2005). In support of this hypothesis, treatment with rapamycin, an inhibitor of TOR kinase, mimics the typical alterations of HI, namely the induction of idi (induced during incompatibility) genes and cytological alterations such as increased septation, vacuolization and coalescence of lipid droplets, suggesting that autophagic cell death is part of the mechanism of non-self-recognition-mediated PCD (Dementhon et al., 2003). However, inactivation of idi-4 did not affect HI (Dementhon et al., 2004) and evidence suggests that fungal autophagy plays a protective role during some HI reactions (Pinan-Lucarre et al., 2003, 2005), similar to what has been observed in some plant models (Liu et al., 2005).
An example of a HI system in filamentous fungi for which the molecular mechanism of death is relatively well characterized is the het-s/het-S system of P. anserina (Saupe, 2011). In this HI system, the het-s allele encodes a prion protein (the HET-s protein) that can exist as a soluble monomer, in a state termed [Het-s*], or as infectious aggregates (a prion state), termed [Het-s]. Importantly, the [Het-s*] form can be transformed to the prion state when in contact with [Het-s]. Prion-infected [Het-s] strains trigger HI with [Het-S] strains, while strains containing the non-prion form, [Het-s*], do not. Cell death is induced when the HET-S protein is activated to form a pore-forming toxin upon interaction with the HET-s prion protein (Coustou et al., 1997; Daskalov et al., 2015, 2016), highlighting that this cell death process has molecular similarities with mammalian necroptosis (Daskalov et al., 2016, see below, in Fungal Nod-Like Receptors- Molecular Hubs for Cell Death Signaling and beyond?).
PCD in Response to Stress
In addition to PCD associated with reproduction, aging and HI in fungi, the response to external stressors can also induce PCD. In fact, activation of PCD is a major treatment strategy for fungal infections in both animals and plants (Ramsdale, 2008). PCD can be triggered by incubating cells with cell death-inducing agents such as staurosporine, phytosphingosine, farnesol or acetic acid, as well as with UV light, oxidative stress and antifungal agents (Figure 1). Table 2 (and references therein) summarizes the wide array of stressors that induce PCD in filamentous fungi. It also denotes the different signaling and effector molecules that have been shown to be involved in the mode of action of each of the stressors. For more in-depth information on some of the signaling pathways, the readers are referred to the original publications. Cells treated with these stressors show typical apoptotic markers like DNA fragmentation, nuclear condensation, chromatin condensation and nuclear degradation as well as ROS accumulation and positive Annexin V and negative PI staining (Sharon et al., 2009). Increasing environmental stress can be associated with a shift from regulated toward uncontrolled death (Phillips et al., 2003).
PCD during Plant Infection
Fungal PCD has also been implicated in plant infection (Figure 1). For example, in the rice blast pathogen Magnaporthe grisea, autophagic cell death of conidia is essential for infection via a specialized type of cell (appressorium), which is required to penetrate the outer cuticle of leaves and stems of rice plants (Wilson and Talbot, 2009). Appressorium morphogenesis requires a mitotic event, nuclear migration, and death of the asexual spore that initiates the infection (Veneault-Fourrey et al., 2006). Conidial death is mediated by autophagy since disruption of the autophagy-mediator gene MgATG8 prevents cell death (Liu et al., 2007; Kershaw and Talbot, 2009); the MgATG8 mutant forms appressoria, but is unable to penetrate plant tissues and cause disease.
Cell death has also been observed in necrotrophic fungi in response to the host defense machinery during plant infection. For example, Arabidopsis cells produce a phytoalexin called camalexin that induces cell death in the necrotrophic fungus Botrytis cinerea (Shlezinger et al., 2011b). Overexpression of the anti-apoptotic gene BcBIR1 in B. cinerea confers enhanced pathogenicity and resistance to cell death (Shlezinger et al., 2011b), while a ΔBcBIR1 mutant exhibits the opposite phenotype, i.e., hypersensitivity to cell death and reduced virulence. Another plant defense compound, hexanoic acid, induces cell death in B. cinerea through BcNma, the orthologue of the mammalian pro-apoptotic protein HtrA2 (Roze and Linz, 1998; Finkelshtein et al., 2011; Shlezinger et al., 2011b). Tomato cells produce the saponin α-tomatine, a sesquiterpene glycoside with fungicidal activity that induces ROS- and metacaspase-dependent cell death in Fusarium oxysporum (Ito et al., 2007). In addition, during host-pathogen interactions, fungal cells may manipulate the mechanisms of plant PCD for their benefit. For example, Alternaria alternata produces a mycotoxin called AAL, with structural similarities to sphinganine, that induces cell death in tomato cells by interfering with the sphingolipid biosynthesis (Brandwagt et al., 2000), leading to the accumulation of dihydrosphingosine and cell death.
Molecular Mediators of Regulated Cell Death
Calcium (Ca2+), the Mitochondrial Electron Transport Chain and ROS
Among the different molecules and pathways identified as playing a role during fungal cell death, Ca2+-triggered signaling seems to be frequently involved in responding to treatment with different compounds (Figure 2). Transient or stable modifications in the cytosolic levels of Ca2+ can operate both as pro-survival or pro-death signals. To regulate the intracellular concentration of Ca2+, cells chelate, compartmentalize or remove the ion using active (pumps and transporters) and passive (Ca2+-binding proteins) systems (Berridge et al., 2000; Carafoli, 2002; Clapham, 2007; Cerella et al., 2010). In S. cerevisiae, the elevation of cytosolic Ca2+ levels is a common response to antifungal agents, including the plant essential oils carvacrol (Rao et al., 2010) and eugenol (Roberts et al., 2012), amiodarone (Gupta et al., 2003; Pozniakovsky et al., 2005) and ER stress-inducing agents like tunicamycin and azole drugs (Bonilla et al., 2002; Martin et al., 2011). In N. crassa, a rise in the cytosolic levels of Ca2+ was also associated with treatment with chitosan (Palma-Guerrero et al., 2009), antifungal proteins PAF (Binder et al., 2010), and PAF26 (Munoz et al., 2012), 2,4-diacetylphloroglucinol (Troppens et al., 2013), plant defensins (Munoz et al., 2014), and staurosporine (Goncalves et al., 2014a, 2015). Staurosporine is a natural alkaloid that has traditionally been used to inhibit protein kinases, and which induce apoptosis in mammalian cells through both caspase-dependent and caspase-independent mechanisms (Belmokhtar et al., 2001). Phospholipase C seems to be a pivotal player coordinating recruitment of Ca2+ to the cytosol during staurosporine-induced cell death as cells lacking the phospolipase C gene plc-2 exhibit increased survival and a staurosporine-induced cytosolic Ca2+ signature is abolished (Goncalves et al., 2014a). The importance of extracellular Ca2+ during staurosporine-induced fungal PCD is supported by the observation that cell death in N. crassa is exacerbated in Ca2+-free medium and inhibited when excess Ca2+ is present (Gonçalves et al., 2014). Consistent with these observations, a similar protection from fungal PCD was observed by the presence of an excessive amount of extracellular Ca2+ in occidiofungin-treated S. cerevisiae cells and chitosan-treated N. crassa cells (Lopez-Moya et al., 2016; Robinson et al., 2017).
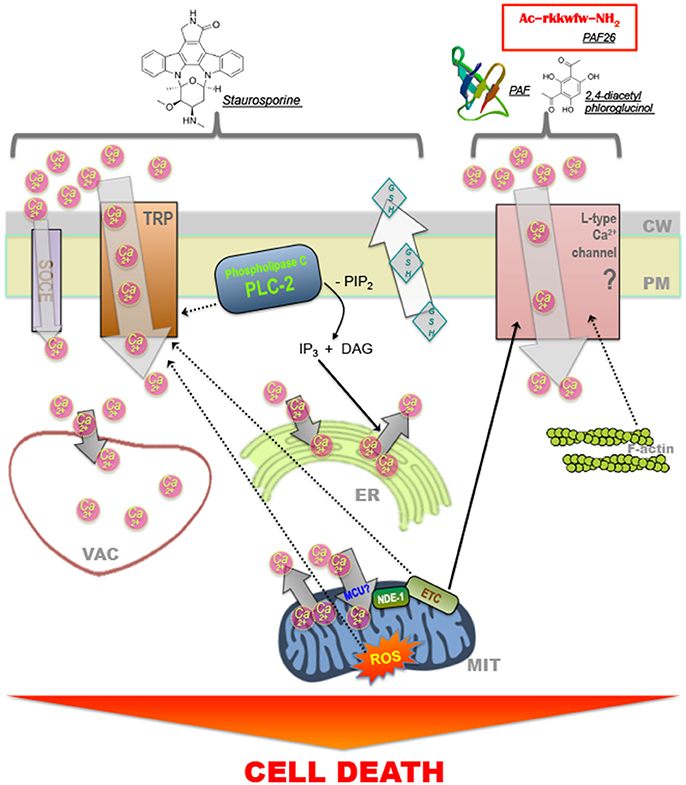
Figure 2. Model for Ca2+ transport and signaling involved in cell death in filamentous fungi. Model for staurosporine induced modifications in intracellular Ca2+ dynamics that precede induction of cell death in N. crassa (Goncalves et al., 2014a). The phospholipase C-family protein PLC-2 plays a pivotal role during the process, which occurs via the recruitment of Ca2+ from the extracellular space, through a TRP-like channel via store-operated Ca2+ entry (SOCE) and from internal stores such as the endoplasmic reticulum (via a IP3-activated channel) or the mitochondria; ROS formation is necessary for the recruitment of Ca2+ to the cytosol (Goncalves et al., 2015). Vacuoles, other acidic vesicles, the endoplasmic reticulum and mitochondria uptake Ca2+ to buffer the concentration of ions in the cytosol. The antifungal peptides Penicillium AntiFungal (Binder et al., 2010) and PAF26 (Munoz et al., 2012) and 2,4- diacetylphloroglucinol (Troppens et al., 2013) also induce cell death and the recruitment of Ca2+ to the cytosol. In this case, Ca2+ recruitment occurs, at least partially, through L-type Ca2+ channels, which are affected by the stability of the intracellular F-actin network. The mitochondrial electron transport chain (ETC) regulates increases in intracellular Ca2+ during cell death (Munoz et al., 2012; Goncalves et al., 2015). Dashed arrows indicate that a relationship between the two elements has been suggested but not fully proven.
The mitochondrial respiratory chain of N. crassa is involved in the regulation of intracellular Ca2+ dynamics upon treatment with staurosporine (Goncalves and Videira, 2015; Goncalves et al., 2015). More specifically, deletion of certain subunits of complex I, namely NUO51 and NUO14, disrupts Ca2+ signaling after treatment with staurosporine and results in hypersensitivity to the drug (Castro et al., 2010). Chemical disruption of other different components of the mitochondrial respiratory chain also led to defective Ca2+ responses during staurosporine-induced cell death (Goncalves et al., 2015).
In addition to changes in Ca2+ levels, the export of GSH and the accumulation of ROS are determinant events during fungal cell death. For example, ROS accumulation has been shown to occur after treatment with staurosporine (Castro et al., 2010), azole drugs (Shirazi and Kontoyiannis, 2013a,b), PAF (Leiter et al., 2005), farnesol (Semighini et al., 2006a; Liu et al., 2010), and during HI (Hutchison et al., 2009). In addition, N. crassa cells, like higher eukaryotes, export GSH which seems to be a cause rather than a consequence during the execution of staurosporine- and phytosphingosine-induced cell death (Fernandes et al., 2013). This phenomenon leads to an imbalance of intracellular GSH/GSSG ratio that favors the accumulation of ROS and the oxidation of cellular components. Importantly, staurosporine-induced cell death is prevented by the supplementation of exogenous GSH or its precursor N-acetyl-cysteine (NAC) (Castro et al., 2010). Interestingly, addition of exogenous GSH or NAC blocked the staurosporine-induced intracellular Ca2+ signature, indicating that it is dependent on ROS (Goncalves et al., 2015).
The BCL-2 Family of Proteins and Other Members of the Intrinsic Cell Death Pathway
Most of the common regulators and executioners of mammalian apoptosis do not have evident homologs in fungal genomes (Sharon et al., 2009; Shlezinger et al., 2012). However, it is clear that fungi undergo an apoptotic-like cell death in response to several stimuli, suggesting that proteins with low (or no) sequence similarity might behave as functional analogs of the mammalian mediators of cell death. In line with this, heterologous expression of mammalian BCL-2 family members in fungi can induce or prevent cell death (Sato et al., 1994; Longo et al., 1997; Xu and Reed, 1998; Polcic and Forte, 2003; Barhoom and Sharon, 2007). Filamentous fungi possess more proteins with homology to cell death-related proteins of mammalian cells as compared to S. cerevisiae (Fedorova et al., 2005). Moreover, the similarity between some proteins involved in cell death in humans and filamentous fungi is higher than the similarity of these same proteins between yeast and filamentous fungal species (Fedorova et al., 2005). In addition to the fact that many filamentous fungi have been extensively used as model organisms for cell biology, these two features make them especially attractive to study PCD. Sequence conservation between mammalian and fungal cell death mediators is normally domain-centered, with conservation of elements important for an apoptotic role and divergence of the remainder of the protein sequence (Sharon et al., 2009; Shlezinger et al., 2012).
Using an in silico domain-based procedure, Shlezinger et al. identified putative fungal mediators of cell death including the BIR (baculovirus inhibitor of apoptosis protein repeat) domain (Shlezinger et al., 2011a). One BIR-containing protein was identified in B. cinerea (BcBir1) and described as an anti-apoptotic molecule involved in interactions with host plants (Shlezinger et al., 2011b). A pro-apoptotic protein called Bxi1/Ybh3 containing a BH3-like signature at the C-terminal portion, triggered PCD in S. cerevisiae (Buttner et al., 2011). BH3 domains are present in some members of the BCL-2 family (Chipuk et al., 2010). Homologues of Bxi1 in other fungi were predicted but functional verification has not been tested. Recently, an orthologue of the mammalian Bax inhibitor 1, MrBI-1 in Metarhizium robertsii was shown to be involved in the anti-death response of this fungal species to farnesol (Chen et al., 2015).
The mitochondrial component of mammalian apoptosis (classically termed the intrinsic pathway) is more conserved in fungi. Cytochrome c functions as an electron carrier of the mitochondrial inter-membrane space, but becomes a pro-apoptogenic molecule upon induction of cell death (Tait and Green, 2010). In S. cerevisiae, cytochrome c (Cyc1) is released from mitochondria upon exposure to acetic acid (Ludovico et al., 2002; Pereira et al., 2007). In filamentous fungal species, this phenomenon was shown to occur in response to treatment with the anti-fungal peptides D(KLAKLAK)2 (Barbu et al., 2013) and WH1 fungin (Qi et al., 2010), as well as upon exposure to a combined treatment of azole drugs and tacrolimus (Shirazi and Kontoyiannis, 2013a).
In mammals, AIF and EndoG are DNA nucleases that normally reside in the mitochondria where they play a role in the maintenance of the respiratory chain (Vahsen et al., 2004) and in mitochondrial DNA replication (Cote and Ruiz-Carrillo, 1993), respectively. In mammalian cells, upon induction of apoptosis, these two molecules translocate to the nucleus and cleave DNA (Tait and Green, 2010). In yeast, the AIF homolog, Aif1, also migrates to the nucleus in response to a variety of stresses where it causes DNA condensation and fragmentation (Wissing et al., 2004). In N. crassa, aif disruption leads to increased resistance to phytosphingosine and hydrogen peroxide (Castro et al., 2008). In A. nidulans, aifA is induced by farnesol and deletion of aifA confers increased sensitivity to this drug and hydrogen peroxide (Savoldi et al., 2008; Dinamarco et al., 2010). These apparently contrasting results might be linked to compensatory mechanisms since AIF belongs to a small family of proteins that also includes the AIF-homologous mitochondrion-associated inducers of death (AMIDs). Human AMID (or PRG3) is a NAD(P)H oxidase that induces caspase-independent apoptosis (Wu et al., 2002). In N. crassa, the AIF-family is composed of AIF, AMID, and AMID-2 (Carneiro et al., 2012a). AIF and AMID proteins are related to the alternative NAD(P)H dehydrogenases present in the mitochondria of fungi, plants, protists and bacteria and are particularly relevant because they oxidize NAD(P)H, reduce quinones and serve as entry points for electrons into the respiratory chain (Videira and Duarte, 2002; Carneiro et al., 2012a). Disruption of N. crassa AMID results in increased sensitivity to phytosphingosine and hydrogen peroxide (Castro et al., 2008), while single or multiple deletion of the different alternative NAD(P)H dehydrogenases results in abnormal accumulation of ROS and altered sensitivity to cell death stimuli (Goncalves and Videira, 2015). In P. anserina, deletion of amid, amid2, and aif2 results in an increase in lifespan (Hamann et al., 2007; Brust et al., 2010).
The mammalian EndoG seems to be involved in mitochondrial DNA replication (Cote and Ruiz-Carrillo, 1993), but is released from the mitochondria in a caspase-independent manner and cleaves nuclear DNA during apoptosis (Tait and Green, 2010). In yeast, Nuc1 seems to behave similarly to its human EndoG homolog, as it translocates to the nucleus after exposure of yeast cells to hydrogen peroxide (Burhans and Weinberger, 2007). However, in A. nidulans, the EndoG homolog NucA is not involved in farnesol-induced cell death (de Castro Pimentel Figueiredo et al., 2011). In animals, HtrA2/Omi is a mitochondrial pro-apoptotic protein that binds to inhibitor of apoptosis proteins and alleviates their inhibitory effect on caspases (Tait and Green, 2010). In yeast, its homolog Nma111, although not mitochondrial, but rather nucleus-localized, is also pro-apoptotic (Fahrenkrog et al., 2004). In B. cinerea, genetic manipulation of the HtrA2/Omi homolog BcNma by overexpression or deletion leads to enhanced or reduced appearance of apoptotic markers, respectively (Finkelshtein et al., 2011). However, the overexpression and mutant strains responded similarly to wild type cells when exposed to different cell death-inducing stresses.
Metacaspases
In animals, caspases are synthesized as inactive zymogens (procaspases) and are activated upon a cell death stimulus. Based on their structure and function, caspases can be classified in three groups: inflammatory, initiator and effector caspases (Fuentes-Prior and Salvesen, 2004; Pop and Salvesen, 2009). Two caspase-related families have also been identified: the metacaspases and the paracaspases (Uren et al., 2000). Metacaspases (as well as paracaspases) have the catalytic histidine/cysteine dyad and secondary structure predictions indicate the presence of the caspase/hemoglobinase fold (Uren et al., 2000; Vercammen et al., 2007). Metacaspases possess sequence similarity with mammalian caspases, in particular in the catalytic p20 and p10 domains. However, metacaspases have a major difference with caspases: instead of cleaving after aspartic acid residues, they cleave after arginine or lysine residues (Vercammen et al., 2004, 2006; Watanabe and Lam, 2005). In humans, paracaspases are involved in the development of MALT lymphoma, but apparently not in cell death execution (Uren et al., 2000). Recently, it has been suggested that paracaspases are a subclass of metacaspases (Hulpiau et al., 2016). Metacaspases have been traditionally divided in two types (I and II). Type II metacaspases are found only in plants, while the distribution of type I metacaspases is more widespread across eukaryotes (Carmona-Gutierrez et al., 2010b). Type I metacaspases possess an N′-terminal region evocative of the pro-domain of initiator and inflammatory caspases with a proline-rich repeat motif (Uren et al., 2000). Type II metacaspases lack such a pro-domain (Uren et al., 2000; Vercammen et al., 2004). Recently, a third type of metacaspase, type III, containing a rearrangement of domain structures between N- and C-terminus has been suggested in phytoplanktonic protists (Choi and Berges, 2013).
Although differing opinions regarding the nomenclature and function of metacaspases occur (Vercammen et al., 2007; Carmona-Gutierrez et al., 2010b; Enoksson and Salvesen, 2010), more recent evidence points to a clear role during cell death. In plants, the conserved Tudor Staphylococcal Nuclease (TSN) was described as a natural substrate of the type II metacaspase of Picea abies mcII-Pa (Sundstrom et al., 2009); mcII-Pa activity on TSN is associated with cell death during stress and development. The Arabidopsis thaliana Type I metacaspases AtMC1 and AtMC2 antagonistically control PCD in response to pathogen attack (Coll et al., 2010).
In S. cerevisiae, the sole metacaspase Yca1 (also described as Mca1) is cleaved like a typical caspase upon treatment with hydrogen peroxide, demonstrating its role as pro-death molecule (Madeo et al., 2002). However, recent work has shown that Yca1 can play a cytoprotective role during removal of misfolded proteins, which lead to yeast life span extension (Hill et al., 2014; Liu, 2014). In the filamentous fungus Aspergillus fumigatus, apoptotic-like cell death occurring after exhaustion of the carbon source and entry into the stationary phase of growth is associated with intracellular activity against caspase-1 and -8 substrates and is blocked by the pan-caspase inhibitor Z-VAD-fmk (Mousavi and Robson, 2003). However, the two metacaspases identified in the A. fumigatus genome (CasA and CasB) do not participate in the response to hydrogen peroxide, amphotericin B or phytosphingosine-induced cell death (Cheng et al., 2003; Mousavi and Robson, 2004) as a ΔcasA ΔcasB mutant showed a similar sensitivity to pro-apoptotic stimuli as a wild type strain (Richie et al., 2007). In P. anserina, disruption of either or both of the predicted metacaspases (PaMCA1 and PaMCA2) led to an increase in lifespan, particularly the ΔPaMca1 mutant (Hamann et al., 2007). Metacaspase-dependent activity occurred in senescent but not in juvenile cultures, while 15 day-old mycelia from the ΔPaMca1 mutant was more resistant to hydrogen peroxide. In N. crassa, the two predicted metacaspases (MCA-1A and MCA-1B) are not required for HI-related cell death (Hutchison et al., 2009), although transcripts for both metacaspases increased during HI and mca-1A is overexpressed in response to phytosphingosine (Videira et al., 2009). Metacaspases have also been linked to the response of other filamentous fungi to a number of stimuli (Table 2).
PARP-1 (poly ADP-ribose polymerase) is involved in DNA repair and is a target of caspases during mammalian apoptosis (Dantzer et al., 2000; Tait and Green, 2010). PARP homologs are absent in yeast but are present in filamentous fungi (Fedorova et al., 2005). The A. nidulans PARP homolog PrpA plays a role in the response to DNA damage and is required for farnesol-induced cell death (Semighini et al., 2006b). In A. nidulans, metacaspases have been implicated in degradation of a PARP-like protein in cell death associated with asexual sporulation processes (Thrane et al., 2004). PARP was also described as a substrate of P. anserina metacaspases (Strobel and Osiewacz, 2013).
Fungal NOD-Like Receptors–Molecular Hubs for Cell Death Signaling and beyond?
Recently, several PCD-controlling molecular players in fungi have been identified that are analogous to NLR proteins [Nucleotide-Binding Domain (NBD) and Leucine-Rich Repeats (LRRs)], also termed NOD-like receptors (Figure 3), which are major constituents of innate immune defenses in plants and metazoans (Proell et al., 2008; Duxbury et al., 2016; Jones et al., 2016; Kim et al., 2016). In plants, NLRs [or R (resistance) proteins] control a characteristic cell death reaction, termed the hypersensitive response, which prevents the spread of pathogenic agents inside plant tissues (Jones and Dangl, 2006). In metazoans, the activity of some NLRs can trigger apoptosis or lead to a highly inflammatory form of PCD known as pyroptosis (Da Silva Correia et al., 2007; Larock and Cookson, 2013; Jones et al., 2016). NLR proteins are intracellular, multi-domain molecular sensors, detecting endogenous or exogenous danger signals or pathogen (or microbial)-associated molecular patterns (PAMPs or MAMPs) (Proell et al., 2008). Most NLR proteins have a modular domain organization with a central NBD domain, an N-terminal effector domain—crucial for downstream signaling—and a C-terminal LRR-containing domain (Jones et al., 2016). Two different types of NBD domains can be encountered; the NB-ARC type (Nucleotide-Binding Domain shared by APAF-1, R proteins and CED-4) mostly present in plants, and the NACHT type (shared by the apoptosis inhibitory protein NAIP and the proteins CIITA, HET-E and TP1), which are predominant in metazoans (Jones et al., 2016). In the current paradigm for NLR functioning, the NLR sensors undergo an oligomerization process mediated by the NACHT/NB-ARC domains resulting in a transition from inactive to active state. The formation of a multimeric signaling platform results in proximity-induced activation of the N-terminal domains, which directly or indirectly induce specific molecular responses (Danot et al., 2009). The similar architectures of plant and metazoan NLRs is believed to be the result of evolutionary convergence rather than orthology (Urbach and Ausubel, 2017).
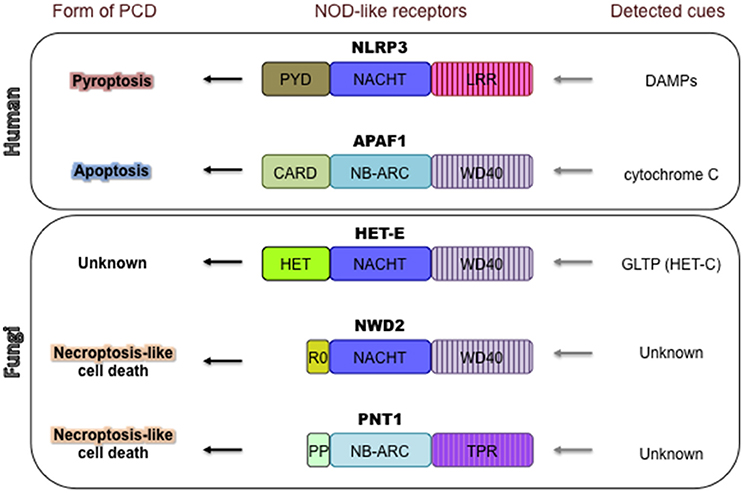
Figure 3. NOD-like receptors control various forms of PCD in mammals and filamentous fungi. NLRP3 and APAF1, given as examples, control pyroptosis and apoptosis, respectively. NLRP3 activity is triggered by various DAMPs (damage-associated molecular patterns), which leads to highly inflammatory pyroptotic cell death (Jones et al., 2016). APAF1 controls the intrinsic apoptotic cell death pathway in mammals (Shakeri et al., 2017). APAF1 is activated by cytochrome C, released from damaged mitochondria. Three examples are given of fungal NOD-like receptors controlling PCD. HET-E controls the HI PCD reaction in the ascomycete P. anserina. PCD is triggered by incompatible allelic variants of HET-C, a glycolipid transfer protein (GLTP). The downstream signaling pathway of HET-E activation and specific mechanism of cell death are currently unclear. Some fungal NLRs (e.g., NWD2 of P. anserina or PNT1 of Chaetomium globosum) use short amyloid motifs (R0 or PP (pseudo-palindromic) respectively) to induce a cell death reaction that has been linked to necroptosis—inflammatory PCD in metazoans (Loquet and Saupe, 2017). The molecular cues and events activating NWD2 and PNT1 are not yet identified.
In fungi, more than 5600 putative NLR-like genes (“NLR-like” is used to signify architectures not containing LRRs) have been bioinformatically identified in 198 genomes, corresponding to 164 different species (Dyrka et al., 2014). NLR-like genes are present in all branches of the fungal kingdom with the exception of yeasts (Dyrka et al., 2014). The majority of fungal NLR-like genes encode a NACHT type of NBD, although NB-ARC domains are also present (1:5 ratio with NACHT). Three different types of super-structure-forming repeats (ANK, TPR, or WD40) are most often found at the C-terminus of fungal NLRs. Some of these C-terminal repeat-containing domains define the signal specificity of the receptors and possibly, as suspected for plant and metazoan NLRs, negatively regulate the NBD-mediated oligomerization process (Chevanne et al., 2010; Daskalov et al., 2015). For various fungal NLR-like proteins, intraspecific variations in repeat number have been reported (Dyrka et al., 2014) and positive diversifying selection on codons within the WD40 repeat region of NLR-like HI-inducing proteins HET-E, HET-D, HET-R have been identified (Paoletti et al., 2007). The process of inter- and intragenic repeat shuffling and positive diversifying selection acting on sequence level have been proposed to lead to an extremely diverse and vast non-self-recognition repertoire for this class of receptors (Paoletti et al., 2007; Chevanne et al., 2010).
The N-terminal effector domains of NLR proteins often serve to recruit additional molecular players important for signaling (Vajjhala et al., 2017). In metazoan NLRs, some N-terminal domains initiate the formation of high-order filaments termed “specks” of an adapter protein called ASC, which leads to cell death through the activation of various caspases (Hoss et al., 2017; Vajjhala et al., 2017). The nucleated-polymerization principle (or prion-like principle) of activation described for ASC is proposed to have broader implications in metazoan innate-immunity (Cai et al., 2014). The fungal NLR-like proteins bear an impressive variety of N-terminal domains with at least 12 different described classes, some of which encode putative lipases, phosphorylases and pore-forming toxin activities (Dyrka et al., 2014). One frequently found effector domain in the fungal NLR-like proteins is the HET domain (for example, in HET-E, HET-D, and HET-R) (Paoletti and Clave, 2007). The HET domain is instrumental for the HI PCD reaction, but its molecular function is currently unknown. However, it has been reported to show similarity to an effector domain termed TIR (Toll/interleukin-1 receptor) identified in various plant and metazoan innate immunity proteins (Paoletti and Clave, 2007; Dyrka et al., 2014; Duxbury et al., 2016). TIR domains function as adaptor domains mediating homotypic protein-protein interactions initiating PCD-associated signaling cascades (Ve et al., 2015).
In some instances, genes that encode for NLR effector domains lack any other regulatory motif (Daskalov et al., 2012; Loquet and Saupe, 2017). In this case, the effector activity can be controlled by an NLR protein encoded by an adjacent gene (Daskalov et al., 2015). The interaction between the NLR and the effector protein is dependent on a short amyloid-forming motif/domain with prion-like features (Daskalov et al., 2015; Loquet and Saupe, 2017) that serves to transduce the signal from the activated NLR toward the effector (Figure 3) (Saupe et al., 2016). Such NLR/effector functional units using amyloid-based signal transduction coexist with “all-in-one” architectures (where the effector is an integral part of the NLR) in a single fungal genome. Furthermore, some of the signaling amyloids have diversified into distinct families that share a specific primary sequence pattern while others appear to have acquired the signaling role in a convergent manner (Daskalov et al., 2016; Loquet and Saupe, 2017).
The NLR-based amyloid signal transduction has been evolutionarily linked to a form of PCD in metazoans termed necroptosis (Daskalov et al., 2016; Grootjans et al., 2017). Necroptotic cell death relies on the protein kinases RIP1 and RIP3, which form amyloid-based signaling complexes mediated by a short (~19aa) motif termed RHIM (RIP homotypic interaction motif) (Rebsamen et al., 2009; Orozco et al., 2014). The RHIM motif seems to be evolutionary related to some of the signaling amyloids attached to the fungal NLR proteins (Kajava et al., 2014; Daskalov, 2016). In addition, both signaling pathways (NLR-based amyloid signaling and necroptosis) led to PCD by plasma membrane damage through a conserved α-helical domain—named HeLo or HeLo-like in fungi and 4HB in metazoans (part of the necroptosis executioner protein MLKL) (Seuring et al., 2012; Hildebrand et al., 2014). Furthermore, the fungal HeLo and HeLo-like domains (and by extension the 4HB domain of MLKL) have homology with an RPW8-related CCR-type N-terminal domain of some plant NLRs, for which a membrane-targeting function in a context of innate immune response has also been reported (Collier et al., 2011; Wang et al., 2013; Daskalov et al., 2016; Zhong and Cheng, 2016). Thus, it seems likely that some PCD molecular pathways in all three major eukaryotic kingdoms have conserved mechanisms of cell death execution. Future studies are needed to understand the degree to which the molecular mechanisms have been conserved.
Taken together, these observations also point to a possible role in innate immunity for at least some fungal NLRs (Paoletti and Saupe, 2009). However, the large number of NLR-like proteins encoded in fungal genomes and their extreme architectural diversity suggests that this family of proteins may be involved in a variety of molecular responses, some of which may be important outside of the immune context and possibly not resulting in cell death.
Concluding Remarks
PCD in filamentous fungi has been identified as a crucial component of the invasion and colonization of host cells, for non-self-recognition mechanisms, during sexual and asexual differentiation and in aging. Additionally, PCD can be induced by treatment of fungi with a variety of chemicals and environmental onslaughts. These observations indicate that filamentous fungi have harnessed mechanisms of regulated death in response to a variety of factors, suggesting that multicellularity growth habits of these organisms have necessitated the evolution of PCD diversity. The multicellular nature of filamentous fungi vs. the unicellular lifestyle of yeast makes filamentous species particularly relevant models of PCD. This adds to the fact that a number of filamentous fungi are particularly easy to manipulate and maintain and because there is a wide assortment of available molecular, biochemical and genetic tools, they are very attractive options for the study of complex biological processes such as PCD. Some of the challenges that we predict will have to be overcome by researchers in the fungal PCD field include the discrimination of different cell death categories in fungi as well as their respective biochemical and morphological hallmarks. Nonetheless, the recent identification of molecular players in both recognition and execution of PCD has provided the groundwork for further investigations into both commonalities and differences in metazoan and fungal cell death.
Author Contributions
APG, JH, AD, AV, and NLG collectively wrote and edited the paper. JH constructed Figure 1, APG constructed Figure 2, and AD constructed Figure 3.
Conflict of Interest Statement
The authors declare that the research was conducted in the absence of any commercial or financial relationships that could be construed as a potential conflict of interest.
Acknowledgments
AV was supported by FCT Portugal (PEst-C/SAU/LA0002/2013 and FCOMP-01-0124-FEDER-037277), the European Regional Development Fund (NORTE-07-0124-FEDER-000003) and a grant from the University of Porto (PP_IJUP2011-20). APG was supported by a grant from the National Science Foundation to NLG (MCB1412411). NLG, JH, and AD were supported by the Laboratory Directed Research and Development Program of Lawrence Berkeley National Laboratory under U.S. Department of Energy Contract No. DE-AC02-05CH11231.
References
Adam, A. L., Kohut, G., and Hornok, L. (2008). Fphog1, a HOG-type MAP kinase gene, is involved in multistress response in Fusarium proliferatum. J. Basic Microbiol. 48, 151–159. doi: 10.1002/jobm.200700403
Almeida, B., Silva, A., Mesquita, A., Sampaio-Marques, B., Rodrigues, F., and Ludovico, P. (2008). Drug-induced apoptosis in yeast. Biochim. Biophys. Acta 1783, 1436–1448. doi: 10.1016/j.bbamcr.2008.01.005
Azevedo, M. M., Almeida, B., Ludovico, P., and Cassio, F. (2009). Metal stress induces programmed cell death in aquatic fungi. Aquat. Toxicol. 92, 264–270. doi: 10.1016/j.aquatox.2009.02.010
Baltazar Lde, M., Soares, B. M., Carneiro, H. C., Avila, T. V., Gouveia, L. F., Souza, D. G., et al. (2013). Photodynamic inhibition of Trichophyton rubrum: in vitro activity and the role of oxidative and nitrosative bursts in fungal death. J. Antimicrob. Chemother. 68, 354–361. doi: 10.1093/jac/dks414
Barbu, E. M., Shirazi, F., Mcgrath, D. M., Albert, N., Sidman, R. L., Pasqualini, R., et al. (2013). An antimicrobial peptidomimetic induces mucorales cell death through mitochondria-mediated apoptosis. PLoS ONE 8:e76981. doi: 10.1371/journal.pone.0076981
Barhoom, S., and Sharon, A. (2007). Bcl-2 proteins link programmed cell death with growth and morphogenetic adaptations in the fungal plant pathogen Colletotrichum gloeosporioides. Fungal Genet. Biol. 44, 32–43. doi: 10.1016/j.fgb.2006.06.007
Belmokhtar, C. A., Hillion, J., and Segal-Bendirdjian, E. (2001). Staurosporine induces apoptosis through both caspase-dependent and caspase-independent mechanisms. Oncogene 20, 3354–3362. doi: 10.1038/sj.onc.1204436
Bernhardt, D., Hamann, A., and Osiewacz, H. D. (2014). The role of mitochondria in fungal aging. Curr. Opin. Microbiol. 22, 1–7. doi: 10.1016/j.mib.2014.09.007
Berridge, M. J., Lipp, P., and Bootman, M. D. (2000). The versatility and universality of calcium signalling. Nat. Rev. Mol. Cell Biol. 1, 11–21. doi: 10.1038/35036035
Biella, S., Smith, M. L., Aist, J. R., Cortesi, P., and Milgroom, M. G. (2002). Programmed cell death correlates with virus transmission in a filamentous fungus. Proc. Biol. Sci. 269, 2269–2276. doi: 10.1098/rspb.2002.2148
Binder, U., Chu, M., Read, N. D., and Marx, F. (2010). The antifungal activity of the Penicillium chrysogenum protein PAF disrupts calcium homeostasis in Neurospora crassa. Eukaryotic Cell 9, 1374–1382. doi: 10.1128/EC.00050-10
Bok, J. W., Ishida, K., and Griffiths, A. J. (2003). Ultrastructural changes in Neurospora cells undergoing senescence induced by kalilo plasmids. Mycologia 95, 500–505. doi: 10.1080/15572536.2004.11833095
Bonilla, M., Nastase, K. K., and Cunningham, K. W. (2002). Essential role of calcineurin in response to endoplasmic reticulum stress. EMBO J. 21, 2343–2353. doi: 10.1093/emboj/21.10.2343
Bowman, J. C., Hicks, P. S., Kurtz, M. B., Rosen, H., Schmatz, D. M., Liberator, P. A., et al. (2002). The antifungal echinocandin caspofungin acetate kills growing cells of Aspergillus fumigatus in vitro. Antimicrob. Agents Chemother. 46, 3001–3012. doi: 10.1128/AAC.46.9.3001-3012.2002
Brandwagt, B. F., Mesbah, L. A., Takken, F. L., Laurent, P. L., Kneppers, T. J., Hille, J., et al. (2000). A longevity assurance gene homolog of tomato mediates resistance to Alternaria alternata f. sp. lycopersici toxins and fumonisin B1. Proc. Natl. Acad. Sci. U.S.A. 97, 4961–4966. doi: 10.1073/pnas.97.9.4961
Brust, D., Hamann, A., and Osiewacz, H. D. (2010). Deletion of PaAif2 and PaAmid2, two genes encoding mitochondrial AIF-like oxidoreductases of Podospora anserina, leads to increased stress tolerance and lifespan extension. Curr. Genet. 56, 225–235. doi: 10.1007/s00294-010-0295-1
Burhans, W. C., and Weinberger, M. (2007). Yeast endonuclease G: complex matters of death, and of life. Mol. Cell 25, 323–325. doi: 10.1016/j.molcel.2007.01.030
Buttner, S., Ruli, D., Vogtle, F. N., Galluzzi, L., Moitzi, B., Eisenberg, T., et al. (2011). A yeast BH3-only protein mediates the mitochondrial pathway of apoptosis. EMBO J. 30, 2779–2792. doi: 10.1038/emboj.2011.197
Cai, X., Chen, J., Xu, H., Liu, S., Jiang, Q. X., Halfmann, R., et al. (2014). Prion-like polymerization underlies signal transduction in antiviral immune defense and inflammasome activation. Cell 156, 1207–1222. doi: 10.1016/j.cell.2014.01.063
Carafoli, E. (2002). Calcium signaling: a tale for all seasons. Proc. Natl. Acad. Sci. U.S.A. 99, 1115–1122. doi: 10.1073/pnas.032427999
Carmona-Gutierrez, D., Eisenberg, T., Buttner, S., Meisinger, C., Kroemer, G., and Madeo, F. (2010a). Apoptosis in yeast: triggers, pathways, subroutines. Cell Death Differ. 17, 763–773. doi: 10.1038/cdd.2009.219
Carmona-Gutierrez, D., Frohlich, K. U., Kroemer, G., and Madeo, F. (2010b). Metacaspases are caspases. Doubt no more. Cell Death Differ 17, 377–378. doi: 10.1038/cdd.2009.198
Carneiro, P., Duarte, M., and Videira, A. (2012a). Characterization of apoptosis-related oxidoreductases from Neurospora crassa. PLoS ONE 7:e34270. doi: 10.1371/journal.pone.0034270
Carneiro, P., Duarte, M., and Videira, A. (2012b). Disruption of alternative NAD(P)H dehydrogenases leads to decreased mitochondrial ROS in Neurospora crassa. Free Radic. Biol. Med. 52, 402–409. doi: 10.1016/j.freeradbiomed.2011.10.492
Castro, A., Lemos, C., Falcao, A., Fernandes, A. S., Glass, N. L., and Videira, A. (2010). Rotenone enhances the antifungal properties of staurosporine. Eukaryotic Cell 9, 906–914. doi: 10.1128/EC.00003-10
Castro, A., Lemos, C., Falcao, A., Glass, N. L., and Videira, A. (2008). Increased resistance of complex I mutants to phytosphingosine-induced programmed cell death. J. Biol. Chem. 283, 19314–19321. doi: 10.1074/jbc.M802112200
Cerella, C., Diederich, M., and Ghibelli, L. (2010). The dual role of calcium as messenger and stressor in cell damage, death, and survival. Int. J. Cell Biol. 2010, 546163. doi: 10.1155/2010/546163
Chen, C., and Dickman, M. B. (2005). Proline suppresses apoptosis in the fungal pathogen Colletotrichum trifolii. Proc. Natl. Acad. Sci. U.S.A. 102, 3459–3464. doi: 10.1073/pnas.0407960102
Chen, Y., Duan, Z., Chen, P., Shang, Y., and Wang, C. (2015). The Bax inhibitor MrBI-1 regulates heat tolerance, apoptotic-like cell death, and virulence in Metarhizium robertsii. Sci. Rep. 5:10625. doi: 10.1038/srep10625
Cheng, C. H., Yang, C. A., and Peng, K. C. (2012). Antagonism of Trichoderma harzianum ETS 323 on Botrytis cinerea mycelium in culture conditions. Phytopathol 102, 1054–1063. doi: 10.1094/PHYTO-11-11-0315
Cheng, J., Park, T. S., Chio, L. C., Fischl, A. S., and Ye, X. S. (2003). Induction of apoptosis by sphingoid long-chain bases in Aspergillus nidulans. Mol. Cell. Biol. 23, 163–177. doi: 10.1128/MCB.23.1.163-177.2003
Chevanne, D., Saupe, S. J., Clave, C., and Paoletti, M. (2010). WD-repeat instability and diversification of the Podospora anserina hnwd non-self recognition gene family. BMC Evol. Biol. 10:134. doi: 10.1186/1471-2148-10-134
Chipuk, J. E., Moldoveanu, T., Llambi, F., Parsons, M. J., and Green, D. R. (2010). The BCL-2 family reunion. Mol. Cell 37, 299–310. doi: 10.1016/j.molcel.2010.01.025
Choi, C. J., and Berges, J. A. (2013). New types of metacaspases in phytoplankton reveal diverse origins of cell death proteases. Cell Death Dis. 4:e490. doi: 10.1038/cddis.2013.21
Clarke, P. G., and Clarke, S. (1996). Nineteenth century research on naturally occurring cell death and related phenomena. Anat. Embryol. 193, 81–99. doi: 10.1007/BF00214700
Colabardini, A. C., De Castro, P. A., De Gouvea, P. F., Savoldi, M., Malavazi, I., Goldman, M. H., et al. (2010). Involvement of the Aspergillus nidulans protein kinase C with farnesol tolerance is related to the unfolded protein response. Mol. Microbiol. 78, 1259–1279. doi: 10.1111/j.1365-2958.2010.07403.x
Coll, N. S., Vercammen, D., Smidler, A., Clover, C., Van Breusegem, F., Dangl, J. L., et al. (2010). Arabidopsis type I metacaspases control cell death. Science 330, 1393–1397. doi: 10.1126/science.1194980
Collier, S. M., Hamel, L. P., and Moffett, P. (2011). Cell death mediated by the N-terminal domains of a unique and highly conserved class of NB-LRR protein. Mol. Plant Microbe Interact. 24, 918–931. doi: 10.1094/MPMI-03-11-0050
Cote, J., and Ruiz-Carrillo, A. (1993). Primers for mitochondrial DNA replication generated by endonuclease G. Science 261, 765–769. doi: 10.1126/science.7688144
Coustou, V., Deleu, C., Saupe, S., and Begueret, J. (1997). The protein product of the het-s heterokaryon incompatibility gene of the fungus Podospora anserina behaves as a prion analog. Proc. Natl. Acad. Sci. U.S.A. 94, 9773–9778. doi: 10.1073/pnas.94.18.9773
Da Silva Correia, J., Miranda, Y., Leonard, N., Hsu, J., and Ulevitch, R. J. (2007). Regulation of Nod1-mediated signaling pathways. Cell Death Differ. 14, 830–839. doi: 10.1038/sj.cdd.4402070
Danot, O., Marquenet, E., Vidal-Ingigliardi, D., and Richet, E. (2009). Wheel of Life, Wheel of Death: a mechanistic insight into signaling by STAND proteins. Structure 17, 172–182. doi: 10.1016/j.str.2009.01.001
Dantzer, F., De La Rubia, G., Menissier-De Murcia, J., Hostomsky, Z., De Murcia, G., and Schreiber, V. (2000). Base excision repair is impaired in mammalian cells lacking Poly(ADP-ribose) polymerase-1. Biochem 39, 7559–7569. doi: 10.1021/bi0003442
Daskalov, A. (2016). On the evolutionary trajectories of signal-transducing amyloids in fungi and beyond. Prion 10, 362–368. doi: 10.1080/19336896.2016.1228506
Daskalov, A., Habenstein, B., Martinez, D., Debets, A. J., Sabate, R., Loquet, A., et al. (2015). Signal transduction by a fungal NOD-like receptor based on propagation of a prion amyloid fold. PLoS Biol. 13:e1002059. doi: 10.1371/journal.pbio.1002059
Daskalov, A., Habenstein, B., Sabate, R., Berbon, M., Martinez, D., Chaignepain, S., et al. (2016). Identification of a novel cell death-inducing domain reveals that fungal amyloid-controlled programmed cell death is related to necroptosis. Proc. Natl. Acad. Sci. U.S.A. 113, 2720–2725. doi: 10.1073/pnas.1522361113
Daskalov, A., Heller, J., Herzog, S., Fleissner, A., and Glass, N. L. (2017). Molecular mechanisms regulating cell fusion and heterokaryon formation in filamentous fungi. Microbiol. Spectr. 5. doi: 10.1128/microbiolspec.FUNK-0015-2016. Available online at: http://www.asmscience.org/docserver/fulltext/microbiolspec/5/2/FUNK-0015-2016.pdf?expires=1505258237&id=id&accname=esid054099&checksum=68CF1CBE42A67B8F7F9F536284885B0B
Daskalov, A., Paoletti, M., Ness, F., and Saupe, S. J. (2012). Genomic clustering and homology between HET-S and the NWD2 STAND protein in various fungal genomes. PLoS ONE 7:e34854. doi: 10.1371/journal.pone.0034854
de Castro Pimentel Figueiredo, B., de Castro, P. A., Dinamarco, T. M., Goldman, M. H., and Goldman, G. H. (2011). The Aspergillus nidulans nucA(EndoG) homologue is not involved in cell death. Eukaryotic Cell 10, 276–283. doi: 10.1128/EC.00224-10
Debets, A. J. M., and Griffiths, A. J. F. (1998). Polymorphism of het-genes prevents resource plundering in Neurospora crassa. Mycol. Res. 102, 1343–1349. doi: 10.1017/S095375629800639X
Debets, F., Yang, X., and Griffiths, A. J. (1994). Vegetative incompatibility in Neurospora: its effect on horizontal transfer of mitochondrial plasmids and senescence in natural populations. Curr. Genet. 26, 113–119. doi: 10.1007/BF00313797
Dementhon, K., Iyer, G., and Glass, N. L. (2006). VIB-1 is required for expression of genes necessary for programmed cell death in Neurospora crassa. Eukaryotic Cell 5, 2161–2173. doi: 10.1128/EC.00253-06
Dementhon, K., Paoletti, M., Pinan-Lucarre, B., Loubradou-Bourges, N., Sabourin, M., Saupe, S. J., et al. (2003). Rapamycin mimics the incompatibility reaction in the fungus Podospora anserina. Eukaryotic Cell 2, 238–246. doi: 10.1128/EC.2.2.238-246.2003
Dementhon, K., Saupe, S. J., and Clave, C. (2004). Characterization of IDI-4, a bZIP transcription factor inducing autophagy and cell death in the fungus Podospora anserina. Mol. Microbiol. 53, 1625–1640. doi: 10.1111/j.1365-2958.2004.04235.x
Dinamarco, T. M., Pimentel Bde, C., Savoldi, M., Malavazi, I., Soriani, F. M., Uyemura, S. A., et al. (2010). The roles played by Aspergillus nidulans apoptosis-inducing factor (AIF)-like mitochondrial oxidoreductase (AifA) and NADH-ubiquinone oxidoreductases (NdeA-B and NdiA) in farnesol resistance. Fungal Genet. Biol. 47, 1055–1069. doi: 10.1016/j.fgb.2010.07.006
Duarte, M., and Videira, A. (2009). Effects of mitochondrial complex III disruption in the respiratory chain of Neurospora crassa. Mol. Microbiol. 72, 246–258. doi: 10.1111/j.1365-2958.2009.06643.x
Duarte, M., and Videira, A. (2012). Defective valyl-tRNA synthetase hampers the mitochondrial respiratory chain in Neurospora crassa. Biochem. J. 448, 297–306. doi: 10.1042/BJ20120963
Dufour, E., Boulay, J., Rincheval, V., and Sainsard-Chanet, A. (2000). A causal link between respiration and senescence in Podospora anserina. Proc. Natl. Acad. Sci. U.S.A. 97, 4138–4143. doi: 10.1073/pnas.070501997
Duxbury, Z., Ma, Y., Furzer, O. J., Huh, S. U., Cevik, V., Jones, J. D., et al. (2016). Pathogen perception by NLRs in plants and animals: Parallel worlds. Bioessays 38, 769–781. doi: 10.1002/bies.201600046
Dyrka, W., Lamacchia, M., Durrens, P., Kobe, B., Daskalov, A., Paoletti, M., et al. (2014). Diversity and variability of NOD-like receptors in fungi. Genome Biol. Evol. 6, 3137–3158. doi: 10.1093/gbe/evu251
Eastwood, M. D., Cheung, S. W., Lee, K. Y., Moffat, J., and Meneghini, M. D. (2012). Developmentally programmed nuclear destruction during yeast gametogenesis. Dev. Cell 23, 35–44. doi: 10.1016/j.devcel.2012.05.005
Ellis, H. M., and Horvitz, H. R. (1986). Genetic control of programmed cell death in the nematode C. elegans. Cell 44, 817–829. doi: 10.1016/0092-8674(86)90004-8
Ellis, R. E., Yuan, J. Y., and Horvitz, H. R. (1991). Mechanisms and functions of cell death. Annu. Rev. Cell Biol. 7, 663–698. doi: 10.1146/annurev.cb.07.110191.003311
Emri, T., Molnar, Z., and Pocsi, I. (2005). The appearances of autolytic and apoptotic markers are concomitant but differently regulated in carbon-starving Aspergillus nidulans cultures. FEMS Microbiol. Lett. 251, 297–303. doi: 10.1016/j.femsle.2005.08.015
Engelberg-Kulka, H., Amitai, S., Kolodkin-Gal, I., and Hazan, R. (2006). Bacterial programmed cell death and multicellular behavior in bacteria. PLoS Genet. 2:e135. doi: 10.1371/journal.pgen.0020135
Enoksson, M., and Salvesen, G. S. (2010). Metacaspases are not caspases–always doubt. Cell Death Differ. 17:1221. doi: 10.1038/cdd.2010.45
Fabrizio, P., Battistella, L., Vardavas, R., Gattazzo, C., Liou, L. L., Diaspro, A., et al. (2004). Superoxide is a mediator of an altruistic aging program in Saccharomyces cerevisiae. J. Cell Biol. 166, 1055–1067. doi: 10.1083/jcb.200404002
Fahrenkrog, B., Sauder, U., and Aebi, U. (2004). The S. cerevisiae HtrA-like protein Nma111p is a nuclear serine protease that mediates yeast apoptosis. J. Cell Sci. 117, 115–126. doi: 10.1242/jcs.00848
Falcone, C., and Mazzoni, C. (2016). External and internal triggers of cell death in yeast. Cell. Mol. Life Sci. 73, 2237–2250. doi: 10.1007/s00018-016-2197-y
Fedorova, N. D., Badger, J. H., Robson, G. D., Wortman, J. R., and Nierman, W. C. (2005). Comparative analysis of programmed cell death pathways in filamentous fungi. BMC Genomics 6:177. doi: 10.1186/1471-2164-6-177
Fernandes, A. S., Castro, A., and Videira, A. (2013). Reduced glutathione export during programmed cell death of Neurospora crassa. Apoptosis 18, 940–948. doi: 10.1007/s10495-013-0858-y
Fernandes, A. S., Goncalves, A. P., Castro, A., Lopes, T. A., Gardner, R., Glass, N. L., et al. (2011). Modulation of fungal sensitivity to staurosporine by targeting proteins identified by transcriptional profiling. Fungal Genet Biol. 48, 1130–1138. doi: 10.1016/j.fgb.2011.09.004
Finkelshtein, A., Shlezinger, N., Bunis, O., and Sharon, A. (2011). Botrytis cinerea BcNma is involved in apoptotic cell death but not in stress adaptation. Fungal Genet. Biol. 48, 621–630. doi: 10.1016/j.fgb.2011.01.007
Franco, F. P., Santiago, A. C., Henrique-Silva, F., De Castro, P. A., Goldman, G. H., Moura, D. S., et al. (2014). The sugarcane defense protein SUGARWIN2 causes cell death in Colletotrichum falcatum but not in non-pathogenic fungi. PLoS ONE 9:e91159. doi: 10.1371/journal.pone.0091159
Fuchs, Y., and Steller, H. (2011). Programmed cell death in animal development and disease. Cell 147, 742–758. doi: 10.1016/j.cell.2011.10.033
Fuchs, Y., and Steller, H. (2015). Live to die another way: modes of programmed cell death and the signals emanating from dying cells. Nat. Rev. Mol. Cell Biol. 16, 329–344. doi: 10.1038/nrm3999
Fuentes-Prior, P., and Salvesen, G. S. (2004). The protein structures that shape caspase activity, specificity, activation and inhibition. Biochem. J. 384, 201–232. doi: 10.1042/BJ20041142
Fujita, K. I., Tatsumi, M., Ogita, A., Kubo, I., and Tanaka, T. (2014). Anethole induces apoptotic cell death accompanied by reactive oxygen species production and DNA fragmentation in Aspergillus fumigatus and Saccharomyces cerevisiae. FEBS J. 281, 1304–1313. doi: 10.1111/febs.12706
Gaddameedi, R. R., Burgula, S., Sairam, M., and Singh, S. S. (2011). Role of insulin in Cr(VI)-mediated genotoxicity in Neurospora crassa. Lett. Appl. Microbiol. 53, 14–21. doi: 10.1111/j.1472-765X.2011.03058.x
Galluzzi, L., Bravo-San Pedro, J. M., Vitale, I., Aaronson, S. A., Abrams, J. M., Adam, D., et al. (2015). Essential vs. accessory aspects of cell death: recommendations of the NCCD 2015. Cell Death Differ. 22, 58–73. doi: 10.1038/cdd.2014.137
Galluzzi, L., Vitale, I., Abrams, J. M., Alnemri, E. S., Baehrecke, E. H., Blagosklonny, M. V., et al. (2012). Molecular definitions of cell death subroutines: recommendations of the Nomenclature Committee on Cell Death 2012. Cell Death Differ. 19, 107–120. doi: 10.1038/cdd.2011.96
Glass, N. L., and Dementhon, K. (2006). Non-self recognition and programmed cell death in filamentous fungi. Curr. Opin. Microbiol. 9, 553–558. doi: 10.1016/j.mib.2006.09.001
Glass, N. L., and Kaneko, I. (2003). Fatal attraction: nonself recognition and heterokaryon incompatibility in filamentous fungi. Eukaryotic Cell 2, 1–8. doi: 10.1128/EC.2.1.1-8.2003
Goncalves, A. P., and Videira, A. (2015). Mitochondrial type II NAD(P)H dehydrogenases in fungal cell death. Microb Cell 2, 68–73. doi: 10.15698/mic2015.03.192
Goncalves, A. P., Cordeiro, J. M., Monteiro, J., Lucchi, C., Correia-De-Sa, P., and Videira, A. (2015). Involvement of mitochondrial proteins in calcium signaling and cell death induced by staurosporine in Neurospora crassa. Biochim. Biophys. Acta 1847, 1064–1074. doi: 10.1016/j.bbabio.2015.05.011
Goncalves, A. P., Cordeiro, J. M., Monteiro, J., Munoz, A., Correia-De-Sa, P., Read, N. D., et al. (2014a). Activation of a TRP-like channel and intracellular Ca2+ dynamics during phospholipase-C-mediated cell death. J. Cell Sci. 127, 3817–3829. doi: 10.1242/jcs.152058
Goncalves, A. P., Hall, C., Kowbel, D. J., Glass, N. L., and Videira, A. (2014b). CZT-1 is a novel transcription factor controlling cell death and natural drug resistance in Neurospora crassa. G3 (Bethesda). 4, 1091–1102. doi: 10.1534/g3.114.011312
Gonçalves, A. P., Monteiro, J., Lucchi, C., Kowbel, D. J., Cordeiro, J. M., Correia-De-Sa, P., et al. (2014). Extracellular calcium triggers unique transcriptional programs and modulates staurosporine-induced cell death in Neurospora crassa. Microbial Cell 1, 289–302. doi: 10.15698/mic2014.09.165
Griffiths, A. J., and Bertrand, H. (1984). Unstable cytoplasms in Hawaiian strains of Neurospora intermedia. Curr. Genet. 8, 387–398. doi: 10.1007/BF00419828
Grootjans, S., Vanden Berghe, T., and Vandenabeele, P. (2017). Initiation and execution mechanisms of necroptosis: an overview. Cell Death Differ. 24, 1184–1195. doi: 10.1038/cdd.2017.65
Gupta, S. S., Ton, V. K., Beaudry, V., Rulli, S., Cunningham, K., and Rao, R. (2003). Antifungal activity of amiodarone is mediated by disruption of calcium homeostasis. J. Biol. Chem. 278, 28831–28839. doi: 10.1074/jbc.M303300200
Hamann, A., Brust, D., and Osiewacz, H. D. (2007). Deletion of putative apoptosis factors leads to lifespan extension in the fungal ageing model Podospora anserina. Mol. Microbiol. 65, 948–958. doi: 10.1111/j.1365-2958.2007.05839.x
Hammond, T. M., Rehard, D. G., Xiao, H., and Shiu, P. K. (2012). Molecular dissection of Neurospora Spore killer meiotic drive elements. Proc. Natl. Acad. Sci. U.S.A. 109, 12093–12098. doi: 10.1073/pnas.1203267109
Hengartner, M. O., and Horvitz, H. R. (1994). C. elegans cell survival gene ced-9 encodes a functional homolog of the mammalian proto-oncogene bcl-2. Cell 76, 665–676. doi: 10.1016/0092-8674(94)90506-1
Herker, E., Jungwirth, H., Lehmann, K. A., Maldener, C., Frohlich, K. U., Wissing, S., et al. (2004). Chronological aging leads to apoptosis in yeast. J. Cell Biol. 164, 501–507. doi: 10.1083/jcb.200310014
Hildebrand, J. M., Tanzer, M. C., Lucet, I. S., Young, S. N., Spall, S. K., Sharma, P., et al. (2014). Activation of the pseudokinase MLKL unleashes the four-helix bundle domain to induce membrane localization and necroptotic cell death. Proc. Natl. Acad. Sci. U.S.A. 111, 15072–15077. doi: 10.1073/pnas.1408987111
Hill, S. M., Hao, X., Liu, B., and Nystrom, T. (2014). Life-span extension by a metacaspase in the yeast Saccharomyces cerevisiae. Science 344, 1389–1392. doi: 10.1126/science.1252634
Hoss, F., Rodriguez-Alcazar, J. F., and Latz, E. (2017). Assembly and regulation of ASC specks. Cell. Mol. Life Sci. 74, 1211–1229. doi: 10.1007/s00018-016-2396-6
Hulpiau, P., Driege, Y., Staal, J., and Beyaert, R. (2016). MALT1 is not alone after all: identification of novel paracaspases. Cell. Mol. Life Sci. 73, 1103–1116. doi: 10.1007/s00018-015-2041-9
Hutchison, E. A., Bueche, J. A., and Glass, N. L. (2012). Diversification of a protein kinase cascade: IME-2 is involved in nonself recognition and programmed cell death in Neurospora crassa. Genetics 192, 467–482. doi: 10.1534/genetics.112.142612
Hutchison, E., Brown, S., Tian, C., and Glass, N. L. (2009). Transcriptional profiling and functional analysis of heterokaryon incompatibility in Neurospora crassa reveals that reactive oxygen species, but not metacaspases, are associated with programmed cell death. Microbiology 155, 3957–3970. doi: 10.1099/mic.0.032284-0
Ito, S., Ihara, T., Tamura, H., Tanaka, S., Ikeda, T., Kajihara, H., et al. (2007). alpha-Tomatine, the major saponin in tomato, induces programmed cell death mediated by reactive oxygen species in the fungal pathogen Fusarium oxysporum. FEBS Lett. 581, 3217–3222. doi: 10.1016/j.febslet.2007.06.010
Jacobson, D. J., Beurkens, K., and Klomparens, K. L. (1998). Microscopic and ultrastructural examination of vegetative incompatibility in partial diploids heterozygous at het loci in Neurospora crassa. Fungal Genet. Biol. 23, 45–56. doi: 10.1006/fgbi.1997.1020
Jones, J. D., and Dangl, J. L. (2006). The plant immune system. Nature 444, 323–329. doi: 10.1038/nature05286
Jones, J. D., Vance, R. E., and Dangl, J. L. (2016). Intracellular innate immune surveillance devices in plants and animals. Science 354:aaf6395. doi: 10.1126/science.aaf6395
Julien, O., and Wells, J. A. (2017). Caspases and their substrates. Cell Death Differ. 24, 1380–1389. doi: 10.1038/cdd.2017.44
Kajava, A. V., Klopffleisch, K., Chen, S., and Hofmann, K. (2014). Evolutionary link between metazoan RHIM motif and prion-forming domain of fungal heterokaryon incompatibility factor HET-s/HET-s. Sci. Rep. 4:7436. doi: 10.1038/srep07436
Katz, M. E., Braunberger, K. S., and Kelly, J. M. (2016). Role of HxkC, a mitochondrial hexokinase-like protein, in fungal programmed cell death. Fungal Genet. Biol. 97, 36–45. doi: 10.1016/j.fgb.2016.11.002
Kerr, J. F., Wyllie, A. H., and Currie, A. R. (1972). Apoptosis: a basic biological phenomenon with wide-ranging implications in tissue kinetics. Br. J. Cancer 26, 239–257. doi: 10.1038/bjc.1972.33
Kershaw, M. J., and Talbot, N. J. (2009). Genome-wide functional analysis reveals that infection-associated fungal autophagy is necessary for rice blast disease. Proc. Natl. Acad. Sci. U.S.A. 106, 15967–15972. doi: 10.1073/pnas.0901477106
Kim, Y. K., Shin, J. S., and Nahm, M. H. (2016). NOD-Like Receptors in Infection, Immunity, and Diseases. Yonsei Med. J. 57, 5–14. doi: 10.3349/ymj.2016.57.1.5
Kretschmer, M., Klose, J., and Kronstad, J. W. (2012). Defects in mitochondrial and peroxisomal beta-oxidation influence virulence in the maize pathogen Ustilago maydis. Eukaryotic Cell 11, 1055–1066. doi: 10.1128/EC.00129-12
Krizsan, K., Bencsik, O., Nyilasi, I., Galgoczy, L., Vagvolgyi, C., and Papp, T. (2010). Effect of the sesterterpene-type metabolites, ophiobolins A and B, on zygomycetes fungi. FEMS Microbiol. Lett. 313, 135–140. doi: 10.1111/j.1574-6968.2010.02138.x
Kroemer, G., El-Deiry, W. S., Golstein, P., Peter, M. E., Vaux, D., Vandenabeele, P., et al. (2005). Classification of cell death: recommendations of the Nomenclature Committee on Cell Death. Cell Death Differ 12(Suppl. 2), 1463–1467. doi: 10.1038/sj.cdd.4401724
Kroemer, G., Galluzzi, L., Vandenabeele, P., Abrams, J., Alnemri, E. S., Baehrecke, E. H., et al. (2009). Classification of cell death: recommendations of the Nomenclature Committee on Cell Death 2009. Cell Death Differ. 16, 3–11. doi: 10.1038/cdd.2008.150
Krohn, N. G., Brown, N. A., Colabardini, A. C., Reis, T., Savoldi, M., Dinamarco, T. M., et al. (2014). The Aspergillus nidulans ATM kinase regulates mitochondrial function, glucose uptake and the carbon starvation response. G3 4, 49–62. doi: 10.1534/g3.113.008607
Kuida, K., Zheng, T. S., Na, S., Kuan, C., Yang, D., Karasuyama, H., et al. (1996). Decreased apoptosis in the brain and premature lethality in CPP32-deficient mice. Nature 384, 368–372. doi: 10.1038/384368a0
Lamkanfi, M., Festjens, N., Declercq, W., Vanden Berghe, T., and Vandenabeele, P. (2007). Caspases in cell survival, proliferation and differentiation. Cell Death Differ. 14, 44–55. doi: 10.1038/sj.cdd.4402047
Larock, C. N., and Cookson, B. T. (2013). Burning down the house: cellular actions during pyroptosis. PLoS Pathog. 9:e1003793. doi: 10.1371/journal.ppat.1003793
Laun, P., Pichova, A., Madeo, F., Fuchs, J., Ellinger, A., Kohlwein, S., et al. (2001). Aged mother cells of Saccharomyces cerevisiae show markers of oxidative stress and apoptosis. Mol. Microbiol. 39, 1166–1173. doi: 10.1111/j.1365-2958.2001.02317.x
Leiter, E., Szappanos, H., Oberparleiter, C., Kaiserer, L., Csernoch, L., Pusztahelyi, T., et al. (2005). Antifungal protein PAF severely affects the integrity of the plasma membrane of Aspergillus nidulans and induces an apoptosis-like phenotype. Antimicrob. Agents Chemother. 49, 2445–2453. doi: 10.1128/AAC.49.6.2445-2453.2005
Li, H., Zhou, H., Luo, Y., Ouyang, H., Hu, H., and Jin, C. (2007). Glycosylphosphatidylinositol (GPI) anchor is required in Aspergillus fumigatus for morphogenesis and virulence. Mol. Microbiol. 64, 1014–1027. doi: 10.1111/j.1365-2958.2007.05709.x
Li, L., Gu, W., Liang, C., Liu, Q., Mello, C. C., and Liu, Y. (2012). The translin-TRAX complex (C3PO) is a ribonuclease in tRNA processing. Nat. Struct. Mol. Biol. 19, 824–830. doi: 10.1038/nsmb.2337
Liu, B. (2014). The Yin and Yang of a metacaspase. Cell Cycle 13, 3471–3472. doi: 10.4161/15384101.2014.980697
Liu, P., Luo, L., Guo, J., Liu, H., Wang, B., Deng, B., et al. (2010). Farnesol induces apoptosis and oxidative stress in the fungal pathogen Penicillium expansum. Mycologia 102, 311–318. doi: 10.3852/09-176
Liu, X. H., Lu, J. P., and Lin, F. C. (2007). Autophagy during conidiation, conidial germination and turgor generation in Magnaporthe grisea. Autophagy 3, 472–473. doi: 10.4161/auto.4339
Liu, Y., Schiff, M., Czymmek, K., Talloczy, Z., Levine, B., and Dinesh-Kumar, S. P. (2005). Autophagy regulates programmed cell death during the plant innate immune response. Cell 121, 567–577. doi: 10.1016/j.cell.2005.03.007
Longo, V. D., Ellerby, L. M., Bredesen, D. E., Valentine, J. S., and Gralla, E. B. (1997). Human Bcl-2 reverses survival defects in yeast lacking superoxide dismutase and delays death of wild-type yeast. J. Cell Biol. 137, 1581–1588. doi: 10.1083/jcb.137.7.1581
Longo, V. D., Mitteldorf, J., and Skulachev, V. P. (2005). Programmed and altruistic ageing. Nat. Rev. Genet. 6, 866–872. doi: 10.1038/nrg1706
Lopez-Moya, F., Kowbel, D., Nueda, M. J., Palma-Guerrero, J., Glass, N. L., and Lopez-Llorca, L. V. (2016). Neurospora crassa transcriptomics reveals oxidative stress and plasma membrane homeostasis biology genes as key targets in response to chitosan. Mol. Biosyst. 12, 391–403. doi: 10.1039/C5MB00649J
Loquet, A., and Saupe, S. J. (2017). Diversity of amyloid motifs in NLR signaling in fungi. Biomolecules 7:38. doi: 10.3390/biom7020038
Lorin, S., Dufour, E., and Sainsard-Chanet, A. (2006). Mitochondrial metabolism and aging in the filamentous fungus Podospora anserina. Biochim. Biophys. Acta 1757, 604–610. doi: 10.1016/j.bbabio.2006.03.005
Low, C. P., and Yang, H. (2008). Programmed cell death in fission yeast Schizosaccharomyces pombe. Biochim. Biophys. Acta 1783, 1335–1349. doi: 10.1016/j.bbamcr.2008.02.002
Lu, B. C., Gallo, N., and Kues, U. (2003). White-cap mutants and meiotic apoptosis in the basidiomycete Coprinus cinereus. Fungal Genet. Biol. 39, 82–93. doi: 10.1016/S1087-1845(03)00024-0
Ludovico, P., Rodrigues, F., Almeida, A., Silva, M. T., Barrientos, A., and Corte-Real, M. (2002). Cytochrome c release and mitochondria involvement in programmed cell death induced by acetic acid in Saccharomyces cerevisiae. Mol. Biol. Cell 13, 2598–2606. doi: 10.1091/mbc.E01-12-0161
Madeo, F., Frohlich, E., and Frohlich, K. U. (1997). A yeast mutant showing diagnostic markers of early and late apoptosis. J. Cell Biol. 139, 729–734. doi: 10.1083/jcb.139.3.729
Madeo, F., Herker, E., Maldener, C., Wissing, S., Lachelt, S., Herlan, M., et al. (2002). A caspase-related protease regulates apoptosis in yeast. Mol. Cell 9, 911–917. doi: 10.1016/S1097-2765(02)00501-4
Man, S. M., Karki, R., and Kanneganti, T. D. (2017). Molecular mechanisms and functions of pyroptosis, inflammatory caspases and inflammasomes in infectious diseases. Immunol. Rev. 277, 61–75. doi: 10.1111/imr.12534
Marek, S. M., Wu, J., Glass, N. L., Gilchrist, D. G., and Bostock, R. M. (2003). Nuclear DNA degradation during heterokaryon incompatibility in Neurospora crassa. Fungal Genet. Biol. 40, 126–137. doi: 10.1016/S1087-1845(03)00086-0
Martin, D. C., Kim, H., Mackin, N. A., Maldonado-Baez, L., Evangelista, C. C. Jr., Beaudry, V. G., et al. (2011). New regulators of a high affinity Ca2+ influx system revealed through a genome-wide screen in yeast. J. Biol. Chem. 286, 10744–10754. doi: 10.1074/jbc.M110.177451
Mousavi, S. A., and Robson, G. D. (2003). Entry into the stationary phase is associated with a rapid loss of viability and an apoptotic-like phenotype in the opportunistic pathogen Aspergillus fumigatus. Fungal Genet. Biol. 39, 221–229. doi: 10.1016/S1087-1845(03)00047-1
Mousavi, S. A., and Robson, G. D. (2004). Oxidative and amphotericin B-mediated cell death in the opportunistic pathogen Aspergillus fumigatus is associated with an apoptotic-like phenotype. Microbiol 150, 1937–1945. doi: 10.1099/mic.0.26830-0
Munkres, K. D. (1976). Ageing of Neurospora crassa. III. Induction of cellular death and clonal senescence of an inositol-less mutant by inositol starvation and the protective effect of dietary antioxidants. Mech. Ageing Dev. 5, 163–169. doi: 10.1016/0047-6374(76)90016-6
Munoz, A., Bertuzzi, M., Bettgenhaeuser, J., Iakobachvili, N., Bignell, E. M., and Read, N. D. (2015). Different stress-induced calcium signatures are reported by aequorin-mediated calcium measurements in living cells of Aspergillus fumigatus. PLoS ONE 10:e0138008. doi: 10.1371/journal.pone.0138008
Munoz, A., Chu, M., Marris, P. I., Sagaram, U. S., Kaur, J., Shah, D. M., et al. (2014). Specific domains of plant defensins differentially disrupt colony initiation, cell fusion and calcium homeostasis in Neurospora crassa. Mol. Microbiol. 92, 1357–1374. doi: 10.1111/mmi.12634
Munoz, A., Harries, E., Contreras-Valenzuela, A., Carmona, L., Read, N. D., and Marcos, J. F. (2013). Two functional motifs define the interaction, internalization and toxicity of the cell-penetrating antifungal peptide PAF26 on fungal cells. PLoS ONE 8:e54813. doi: 10.1371/journal.pone.0054813
Munoz, A., Marcos, J. F., and Read, N. D. (2012). Concentration-dependent mechanisms of cell penetration and killing by the de novo designed antifungal hexapeptide PAF26. Mol. Microbiol. 85, 89–106. doi: 10.1111/j.1365-2958.2012.08091.x
Muzaffar, S., Bose, C., Banerji, A., Nair, B. G., and Chattoo, B. B. (2016). Anacardic acid induces apoptosis-like cell death in the rice blast fungus Magnaporthe oryzae. Appl. Microbiol. Biotechnol. 100, 323–335. doi: 10.1007/s00253-015-6915-4
Nitsche, B. M., Jorgensen, T. R., Akeroyd, M., Meyer, V., and Ram, A. F. (2012). The carbon starvation response of Aspergillus niger during submerged cultivation: insights from the transcriptome and secretome. BMC Genomics 13:380. doi: 10.1186/1471-2164-13-380
Oberst, A., Bender, C., and Green, D. R. (2008). Living with death: the evolution of the mitochondrial pathway of apoptosis in animals. Cell Death Differ. 15, 1139–1146. doi: 10.1038/cdd.2008.65
Orozco, S., Yatim, N., Werner, M. R., Tran, H., Gunja, S. Y., Tait, S. W., et al. (2014). RIPK1 both positively and negatively regulates RIPK3 oligomerization and necroptosis. Cell Death Differ. 21, 1511–1521. doi: 10.1038/cdd.2014.76
Osiewacz, H. D. (2011). Mitochondrial quality control in aging and lifespan control of the fungal aging model Podospora anserina. Biochem. Soc. Trans. 39, 1488–1492. doi: 10.1042/BST0391488
Osiewacz, H. D., and Bernhardt, D. (2013). Mitochondrial quality control: impact on aging and life span—a mini-review. Gerontology 59, 413–420. doi: 10.1159/000348662
Osiewacz, H. D., and Borghouts, C. (2000). Mitochondrial oxidative stress and aging in the filamentous fungus Podospora anserina. Ann. N.Y. Acad. Sci. 908, 31–39. doi: 10.1111/j.1749-6632.2000.tb06633.x
Palkova, Z., and Vachova, L. (2006). Life within a community: benefit to yeast long-term survival. FEMS Microbiol. Rev. 30, 806–824. doi: 10.1111/j.1574-6976.2006.00034.x
Palma-Guerrero, J., Huang, I. C., Jansson, H. B., Salinas, J., Lopez-Llorca, L. V., and Read, N. D. (2009). Chitosan permeabilizes the plasma membrane and kills cells of Neurospora crassa in an energy dependent manner. Fungal Genet. Biol. 46, 585–594. doi: 10.1016/j.fgb.2009.02.010
Paoletti, M., and Clave, C. (2007). The fungus-specific HET domain mediates programmed cell death in Podospora anserina. Eukaryotic Cell 6, 2001–2008. doi: 10.1128/EC.00129-07
Paoletti, M., and Saupe, S. J. (2009). Fungal incompatibility: evolutionary origin in pathogen defense? Bioessays 31, 1201–1210. doi: 10.1002/bies.200900085
Paoletti, M., Saupe, S. J., and Clave, C. (2007). Genesis of a fungal non-self recognition repertoire. PLoS ONE 2:e283. doi: 10.1371/journal.pone.0000283
Pereira, C., Camougrand, N., Manon, S., Sousa, M. J., and Corte-Real, M. (2007). ADP/ATP carrier is required for mitochondrial outer membrane permeabilization and cytochrome c release in yeast apoptosis. Mol. Microbiol. 66, 571–582. doi: 10.1111/j.1365-2958.2007.05926.x
Perkins, D. D. (1975). The use of duplication-generating rearrangements for studying heterokaryon incompatibility genes in Neurospora. Genetics 80, 87–105.
Phillips, A. J., Sudbery, I., and Ramsdale, M. (2003). Apoptosis induced by environmental stresses and amphotericin B in Candida albicans. Proc. Natl. Acad. Sci. U.S.A. 100, 14327–14332. doi: 10.1073/pnas.2332326100
Pinan-Lucarre, B., Balguerie, A., and Clave, C. (2005). Accelerated cell death in Podospora autophagy mutants. Eukaryotic Cell 4, 1765–1774. doi: 10.1128/EC.4.11.1765-1774.2005
Pinan-Lucarre, B., Paoletti, M., Dementhon, K., Coulary-Salin, B., and Clave, C. (2003). Autophagy is induced during cell death by incompatibility and is essential for differentiation in the filamentous fungus Podospora anserina. Mol. Microbiol. 47, 321–333. doi: 10.1046/j.1365-2958.2003.03208.x
Plesofsky, N. S., Levery, S. B., Castle, S. A., and Brambl, R. (2008). Stress-induced cell death is mediated by ceramide synthesis in Neurospora crassa. Eukaryotic Cell 7, 2147–2159. doi: 10.1128/EC.00147-08
Plesofsky-Vig, N., and Brambl, R. (1995). Disruption of the gene for hsp30, an alpha-crystallin-related heat shock protein of Neurospora crassa, causes defects in thermotolerance. Proc. Natl. Acad. Sci. U.S.A. 92, 5032–5036. doi: 10.1073/pnas.92.11.5032
Polcic, P., and Forte, M. (2003). Response of yeast to the regulated expression of proteins in the Bcl-2 family. Biochem. J. 374, 393–402. doi: 10.1042/bj20030690
Pop, C., and Salvesen, G. S. (2009). Human caspases: activation, specificity, and regulation. J. Biol. Chem. 284, 21777–21781. doi: 10.1074/jbc.R800084200
Pozniakovsky, A. I., Knorre, D. A., Markova, O. V., Hyman, A. A., Skulachev, V. P., and Severin, F. F. (2005). Role of mitochondria in the pheromone- and amiodarone-induced programmed death of yeast. J. Cell Biol. 168, 257–269. doi: 10.1083/jcb.200408145
Proell, M., Riedl, S. J., Fritz, J. H., Rojas, A. M., and Schwarzenbacher, R. (2008). The Nod-like receptor (NLR) family: a tale of similarities and differences. PLoS ONE 3:e2119. doi: 10.1371/journal.pone.0002119
Qi, G., Zhu, F., Du, P., Yang, X., Qiu, D., Yu, Z., et al. (2010). Lipopeptide induces apoptosis in fungal cells by a mitochondria-dependent pathway. Peptides 31, 1978–1986. doi: 10.1016/j.peptides.2010.08.003
Raju, N. B., and Perkins, D. D. (2000). Programmed ascospore death in the homothallic ascomycete Coniochaeta tetraspora. Fungal Genet. Biol. 30, 213–221. doi: 10.1006/fgbi.2000.1217
Ramsdale, M. (2008). Programmed cell death in pathogenic fungi. Biochim. Biophys. Acta 1783, 1369–1380. doi: 10.1016/j.bbamcr.2008.01.021
Ramsdale, M. (2012). Programmed cell death in the cellular differentiation of microbial eukaryotes. Curr. Opin. Microbiol. 15, 646–652. doi: 10.1016/j.mib.2012.09.005
Rao, A., Zhang, Y., Muend, S., and Rao, R. (2010). Mechanism of antifungal activity of terpenoid phenols resembles calcium stress and inhibition of the TOR pathway. Antimicrob. Agents Chemother. 54, 5062–5069. doi: 10.1128/AAC.01050-10
Ratcliff, W. C., Denison, R. F., Borrello, M., and Travisano, M. (2012). Experimental evolution of multicellularity. Proc. Natl. Acad. Sci. USA. 109, 1595–1600. doi: 10.1073/pnas.1115323109
Rayner, A. D. M. (1996). Interconnectedness and individualism in fungal mycelia. Cambridge: Cambridge University Press.
Rebsamen, M., Heinz, L. X., Meylan, E., Michallet, M. C., Schroder, K., Hofmann, K., et al. (2009). DAI/ZBP1 recruits RIP1 and RIP3 through RIP homotypic interaction motifs to activate NF-kappaB. EMBO Rep. 10, 916–922. doi: 10.1038/embor.2009.109
Richie, D. L., Miley, M. D., Bhabhra, R., Robson, G. D., Rhodes, J. C., and Askew, D. S. (2007). The Aspergillus fumigatus metacaspases CasA and CasB facilitate growth under conditions of endoplasmic reticulum stress. Mol. Microbiol. 63, 591–604. doi: 10.1111/j.1365-2958.2006.05534.x
Rizet, G. (1953). Impossibility of obtaining uninterrupted and unlimited multiplication of the ascomycete Podospora anserina. C. R. Hebd. Seances Acad. Sci. 237, 838–840.
Roberts, S. K., Mcainsh, M., and Widdicks, L. (2012). Cch1p mediates Ca2+ influx to protect Saccharomyces cerevisiae against eugenol toxicity. PLoS ONE 7:e43989. doi: 10.1371/journal.pone.0043989
Robinson, C. A., Denison, C., Burkenstock, A., Nutter, C., and Gordon, D. M. (2017). Cellular conditions that modulate the fungicidal activity of occidiofungin. J. Appl. Microbiol. 123, 380–391. doi: 10.1111/jam.13496
Roze, L. V., and Linz, J. E. (1998). Lovastatin triggers an apoptosis-like cell death process in the fungus Mucor racemosus. Fungal Genet. Biol. 25, 119–133. doi: 10.1006/fgbi.1998.1093
Sato, T., Hanada, M., Bodrug, S., Irie, S., Iwama, N., Boise, L. H., et al. (1994). Interactions among members of the Bcl-2 protein family analyzed with a yeast two-hybrid system. Proc. Natl. Acad. Sci. U.S.A. 91, 9238–9242. doi: 10.1073/pnas.91.20.9238
Saupe, S. J. (2000). Molecular genetics of heterokaryon incompatibility in filamentous ascomycetes. Microbiol. Mol. Biol. Rev. 64, 489–502. doi: 10.1128/MMBR.64.3.489-502.2000
Saupe, S. J. (2011). The [Het-s] prion of Podospora anserina and its role in heterokaryon incompatibility. Semin. Cell Dev. Biol. 22, 460–468. doi: 10.1016/j.semcdb.2011.02.019
Saupe, S. J., Jarosz, D. F., and True, H. L. (2016). Amyloid Prions in Fungi. Microbiol Spectr. 4. doi: 10.1128/microbiolspec.FUNK-0029-2016. Available online at: http://www.asmscience.org/docserver/fulltext/microbiolspec/4/6/FUNK-0029-2016.pdf?expires=1505258350&id=id&accname=esid054099&checksum=D544DE58F2F086E73C7891CAEE7ECACF
Savi, G. D., Vitorino, V., Bortoluzzi, A. J., and Scussel, V. M. (2013). Effect of zinc compounds on Fusarium verticillioides growth, hyphae alterations, conidia, and fumonisin production. J. Sci. Food Agric. 93, 3395–3402. doi: 10.1002/jsfa.6271
Savoldi, M., Malavazi, I., Soriani, F. M., Capellaro, J. L., Kitamoto, K., Da Silva Ferreira, M. E., et al. (2008). Farnesol induces the transcriptional accumulation of the Aspergillus nidulans Apoptosis-Inducing Factor (AIF)-like mitochondrial oxidoreductase. Mol. Microbiol. 70, 44–59. doi: 10.1111/j.1365-2958.2008.06385.x
Semighini, C. P., Hornby, J. M., Dumitru, R., Nickerson, K. W., and Harris, S. D. (2006a). Farnesol-induced apoptosis in Aspergillus nidulans reveals a possible mechanism for antagonistic interactions between fungi. Mol. Microbiol. 59, 753–764. doi: 10.1111/j.1365-2958.2005.04976.x
Semighini, C. P., Murray, N., and Harris, S. D. (2008). Inhibition of Fusarium graminearum growth and development by farnesol. FEMS Microbiol. Lett. 279, 259–264. doi: 10.1111/j.1574-6968.2007.01042.x
Semighini, C. P., Savoldi, M., Goldman, G. H., and Harris, S. D. (2006b). Functional characterization of the putative Aspergillus nidulans poly(ADP-ribose) polymerase homolog PrpA. Genetics 173, 87–98. doi: 10.1534/genetics.105.053199
Seuring, C., Greenwald, J., Wasmer, C., Wepf, R., Saupe, S. J., Meier, B. H., et al. (2012). The mechanism of toxicity in HET-S/HET-s prion incompatibility. PLoS Biol. 10:e1001451. doi: 10.1371/journal.pbio.1001451
Severin, F. F., Meer, M. V., Smirnova, E. A., Knorre, D. A., and Skulachev, V. P. (2008). Natural causes of programmed death of yeast Saccharomyces cerevisiae. Biochim. Biophys. Acta 1783, 1350–1353. doi: 10.1016/j.bbamcr.2008.02.001
Shakeri, R., Kheirollahi, A., and Davoodi, J. (2017). Apaf-1: Regulation and function in cell death. Biochimie 135, 111–125. doi: 10.1016/j.biochi.2017.02.001
Sharon, A., Finkelstein, A., Shlezinger, N., and Hatam, I. (2009). Fungal apoptosis: function, genes and gene function. FEMS Microbiol. Rev. 33, 833–854. doi: 10.1111/j.1574-6976.2009.00180.x
Shi, M., Chen, L., Wang, X. W., Zhang, T., Zhao, P. B., Song, X. Y., et al. (2012). Antimicrobial peptaibols from Trichoderma pseudokoningii induce programmed cell death in plant fungal pathogens. Microbiology 158, 166–175. doi: 10.1099/mic.0.052670-0
Shirazi, F., and Kontoyiannis, D. P. (2013a). The calcineurin pathway inhibitor tacrolimus enhances the in vitro activity of azoles against mucorales via apoptosis. Eukaryotic Cell 12, 1225–1234. doi: 10.1128/EC.00138-13
Shirazi, F., and Kontoyiannis, D. P. (2013b). Mitochondrial respiratory pathways inhibition in Rhizopus oryzae potentiates activity of posaconazole and itraconazole via apoptosis. PLoS ONE 8:e63393. doi: 10.1371/journal.pone.0063393
Shirazi, F., Kontoyiannis, D. P., and Ibrahim, A. S. (2015). Iron starvation induces apoptosis in Rhizopus oryzae in vitro. Virulence 6, 121–126. doi: 10.1080/21505594.2015.1009732
Shlezinger, N., Doron, A., and Sharon, A. (2011a). Apoptosis-like programmed cell death in the grey mould fungus Botrytis cinerea: genes and their role in pathogenicity. Biochem. Soc. Trans. 39, 1493–1498. doi: 10.1042/BST0391493
Shlezinger, N., Goldfinger, N., and Sharon, A. (2012). Apoptotic-like programed cell death in fungi: the benefits in filamentous species. Front. Oncol. 2:97. doi: 10.3389/fonc.2012.00097
Shlezinger, N., Minz, A., Gur, Y., Hatam, I., Dagdas, Y. F., Talbot, N. J., et al. (2011b). Anti-apoptotic machinery protects the necrotrophic fungus Botrytis cinerea from host-induced apoptotic-like cell death during plant infection. PLoS Pathog. 7:e1002185. doi: 10.1371/journal.ppat.1002185
Silar, P. (2005). Peroxide accumulation and cell death in filamentous fungi induced by contact with a contestant. Mycol. Res. 109, 137–149. doi: 10.1017/S0953756204002230
Song, C., Chen, Q., Wu, X., Zhang, J., and Huang, C. (2014). Heat stress induces apoptotic-like cell death in two Pleurotus species. Curr. Microbiol. 89, 611–616. doi: 10.1007/s00284-014-0634-4
Strauss, B. S. (1958). Cell death and unbalanced growth in Neurospora. J. Gen. Microbiol. 18, 658–669. doi: 10.1099/00221287-18-3-658
Strobel, I., and Osiewacz, H. D. (2013). Poly(ADP-ribose) polymerase is a substrate recognized by two metacaspases of Podospora anserina. Eukaryotic Cell 12, 900–912. doi: 10.1128/EC.00337-12
Sukhanova, E. I., Rogov, A. G., Severin, F. F., and Zvyagilskaya, R. A. (2012). Phenoptosis in yeasts. Biochemistry 77, 761–775. doi: 10.1134/S0006297912070097
Sundstrom, J. F., Vaculova, A., Smertenko, A. P., Savenkov, E. I., Golovko, A., Minina, E., et al. (2009). Tudor staphylococcal nuclease is an evolutionarily conserved component of the programmed cell death degradome. Nat. Cell Biol. 11, 1347–1354. doi: 10.1038/ncb1979
Tait, S. W., and Green, D. R. (2010). Mitochondria and cell death: outer membrane permeabilization and beyond. Nat. Rev. Mol. Cell Biol. 11, 621–632. doi: 10.1038/nrm2952
Tao, L., Gao, N., Chen, S., and Yu, J. H. (2010). The choC gene encoding a putative phospholipid methyltransferase is essential for growth and development in Aspergillus nidulans. Curr. Genet. 56, 283–296. doi: 10.1007/s00294-010-0300-8
Terrile, M. C., Mansilla, A. Y., Albertengo, L., Rodriguez, M. S., and Casalongue, C. A. (2014). Nitric-oxide-mediated cell death is triggered by chitosan in Fusarium eumartii spores. Pest Manag. Sci. 71, 668–674. doi: 10.1002/ps.3814
Thrane, C., Kaufmann, U., Stummann, B. M., and Olsson, S. (2004). Activation of caspase-like activity and poly (ADP-ribose) polymerase degradation during sporulation in Aspergillus nidulans. Fungal Genet. Biol. 41, 361–368. doi: 10.1016/j.fgb.2003.11.003
Tian, J., Ban, X., Zeng, H., He, J., Chen, Y., and Wang, Y. (2012). The mechanism of antifungal action of essential oil from dill (Anethum graveolens L.) on Aspergillus flavus. PLoS ONE 7:e30147. doi: 10.1371/journal.pone.0030147
Tian, J., Wang, Y. Z., Lu, Z. Q., Sun, C. H., Zhang, M., Zhu, A. H., et al. (2016). Perillaldehyde, a promising antifungal agent used in food preservation, triggers apoptosis through a metacaspase-dependent pathway in Aspergillus flavus. J. Agr. Food Chem. 64, 7404–7413. doi: 10.1021/acs.jafc.6b03546
Troppens, D. M., Chu, M., Holcombe, L. J., Gleeson, O., O'gara, F., Read, N. D., et al. (2013). The bacterial secondary metabolite 2,4-diacetylphloroglucinol impairs mitochondrial function and affects calcium homeostasis in Neurospora crassa. Fungal Genet. Biol. 56, 135–146. doi: 10.1016/j.fgb.2013.04.006
Tsiatsiani, L., Van Breusegem, F., Gallois, P., Zavialov, A., Lam, E., and Bozhkov, P. V. (2011). Metacaspases. Cell Death Differ. 18, 1279–1288. doi: 10.1038/cdd.2011.66
Turner, B. C., and Perkins, D. D. (1979). Spore killer, a chromosomal factor in Neurospora that kills meiotic products not containing it. Genetics 93, 587–606.
Umar, M. H., and Griensven Van, L. J. L. D. (1997). Morphogenetic cell death in developing primordia of Agaricus bisporus. Mycologia 89, 274–277. doi: 10.2307/3761082
Urbach, J. M., and Ausubel, F. M. (2017). The NBS-LRR architectures of plant R-proteins and metazoan NLRs evolved in independent events. Proc. Natl. Acad. Sci. U.S.A. 114, 1063–1068. doi: 10.1073/pnas.1619730114
Uren, A. G., O'rourke, K., Aravind, L. A., Pisabarro, M. T., Seshagiri, S., Koonin, E. V., et al. (2000). Identification of paracaspases and metacaspases: two ancient families of caspase-like proteins, one of which plays a key role in MALT lymphoma. Mol. Cell 6, 961–967. doi: 10.1016/S1097-2765(00)00094-0
Vahsen, N., Cande, C., Briere, J. J., Benit, P., Joza, N., Larochette, N., et al. (2004). AIF deficiency compromises oxidative phosphorylation. EMBO J. 23, 4679–4689. doi: 10.1038/sj.emboj.7600461
Vajjhala, P. R., Ve, T., Bentham, A., Stacey, K. J., and Kobe, B. (2017). The molecular mechanisms of signaling by cooperative assembly formation in innate immunity pathways. Mol. Immunol. 86, 23–37. doi: 10.1016/j.molimm.2017.02.012
Van der Nest, M. A., Olson, A., Lind, M., Velez, H., Dalman, K., Brandstrom Durling, M., et al. (2014). Distribution and evolution of het gene homologs in the basidiomycota. Fungal Genet. Biol. 64, 45–57. doi: 10.1016/j.fgb.2013.12.007
Van Diepeningen, A. D., Debets, A. J., and Hoekstra, R. F. (1997). Heterokaryon incompatibility blocks virus transfer among natural isolates of black Aspergilli. Curr. Genet. 32, 209–217. doi: 10.1007/s002940050268
van Doorn, W. G. (2011). Classes of programmed cell death in plants, compared to those in animals. J. Exp. Bot. 62, 4749–4761. doi: 10.1093/jxb/err196
Ve, T., Williams, S. J., and Kobe, B. (2015). Structure and function of Toll/interleukin-1 receptor/resistance protein (TIR) domains. Apoptosis 20, 250–261. doi: 10.1007/s10495-014-1064-2
Veneault-Fourrey, C., Barooah, M., Egan, M., Wakley, G., and Talbot, N. J. (2006). Autophagic fungal cell death is necessary for infection by the rice blast fungus. Science 312, 580–583. doi: 10.1126/science.1124550
Vercammen, D., Belenghi, B., Van De Cotte, B., Beunens, T., Gavigan, J. A., De Rycke, R., et al. (2006). Serpin1 of Arabidopsis thaliana is a suicide inhibitor for metacaspase 9. J. Mol. Biol. 364, 625–636. doi: 10.1016/j.jmb.2006.09.010
Vercammen, D., Declercq, W., Vandenabeele, P., and Van Breusegem, F. (2007). Are metacaspases caspases? J. Cell Biol. 179, 375–380. doi: 10.1083/jcb.200705193
Vercammen, D., Van De Cotte, B., De Jaeger, G., Eeckhout, D., Casteels, P., Vandepoele, K., et al. (2004). Type II metacaspases Atmc4 and Atmc9 of Arabidopsis thaliana cleave substrates after arginine and lysine. J. Biol. Chem. 279, 45329–45336. doi: 10.1074/jbc.M406329200
Videira, A., and Duarte, M. (2002). From NADH to ubiquinone in Neurospora mitochondria. Biochim. Biophys. Acta 1555, 187–191. doi: 10.1016/S0005-2728(02)00276-1
Videira, A., Kasuga, T., Tian, C., Lemos, C., Castro, A., and Glass, N. L. (2009). Transcriptional analysis of the response of Neurospora crassa to phytosphingosine reveals links to mitochondrial function. Microbiology 155, 3134–3141. doi: 10.1099/mic.0.029710-0
Vogt, C. I. (1842). Untersuchungen über die Entwicklungsgeschichte der Geburtshelferkroete (Alytes obstetricans). Solothurn: Jent & Gassmann.
Vopalenska, I., Hulkova, M., Janderova, B., and Palkova, Z. (2005). The morphology of Saccharomyces cerevisiae colonies is affected by cell adhesion and the budding pattern. Res. Microbiol. 156, 921–931. doi: 10.1016/j.resmic.2005.05.012
Wang, W., Zhang, Y., Wen, Y., Berkey, R., Ma, X., Pan, Z., et al. (2013). A comprehensive mutational analysis of the Arabidopsis resistance protein RPW8.2 reveals key amino acids for defense activation and protein targeting. Plant Cell 25, 4242–4261. doi: 10.1105/tpc.113.117226
Wang, X., Wang, Y., Zhou, Y., and Wei, X. (2014). Farnesol induces apoptosis-like cell death in the pathogenic fungus Aspergillus flavus. Mycologia 106, 881–888. doi: 10.3852/13-292
Watanabe, N., and Lam, E. (2005). Two Arabidopsis metacaspases AtMCP1b and AtMCP2b are arginine/lysine-specific cysteine proteases and activate apoptosis-like cell death in yeast. J. Biol. Chem. 280, 14691–14699. doi: 10.1074/jbc.M413527200
White, K., Grether, M. E., Abrams, J. M., Young, L., Farrell, K., and Steller, H. (1994). Genetic control of programmed cell death in Drosophila. Science 264, 677–683. doi: 10.1126/science.8171319
Wiemer, M., and Osiewacz, H. D. (2014). Effect of paraquat-induced oxidative stress on gene expression and aging of the filamentous ascomycete Podospora anserina. Microbial. Cell 1, 225–240. doi: 10.15698/mic2014.07.155
Wilson, R. A., and Talbot, N. J. (2009). Under pressure: investigating the biology of plant infection by Magnaporthe oryzae. Nat. Rev. Microbiol. 7, 185–195. doi: 10.1038/nrmicro2032
Wissing, S., Ludovico, P., Herker, E., Buttner, S., Engelhardt, S. M., Decker, T., et al. (2004). An AIF orthologue regulates apoptosis in yeast. J. Cell Biol. 166, 969–974. doi: 10.1083/jcb.200404138
Worrall, J. J. (1997). Somatic incompatibility in basidiomycetes. Mycologia 89, 24–36. doi: 10.2307/3761169
Wu, M., Xu, L. G., Li, X., Zhai, Z., and Shu, H. B. (2002). AMID, an apoptosis-inducing factor-homologous mitochondrion-associated protein, induces caspase-independent apoptosis. J. Biol. Chem. 277, 25617–25623. doi: 10.1074/jbc.M202285200
Xu, Q., and Reed, J. C. (1998). Bax inhibitor-1, a mammalian apoptosis suppressor identified by functional screening in yeast. Mol. Cell 1, 337–346. doi: 10.1016/S1097-2765(00)80034-9
Yan, J., Du, T., Zhao, W., Hartmann, T., Lu, H., Lu, Y., et al. (2013). Transcriptome and biochemical analysis reveals that suppression of GPI-anchor synthesis leads to autophagy and possible necroptosis in Aspergillus fumigatus. PLoS ONE 8:e59013. doi: 10.1371/journal.pone.0059013
Yuan, J., Shaham, S., Ledoux, S., Ellis, H. M., and Horvitz, H. R. (1993). The C. elegans cell death gene ced-3 encodes a protein similar to mammalian interleukin-1 beta-converting enzyme. Cell 75, 641–652. doi: 10.1016/0092-8674(93)90485-9
Zhao, J., Gladieux, P., Hutchison, E., Bueche, J., Hall, C., Perraudeau, F., et al. (2015). Identification of allorecognition loci in Neurospora crassa by genomics and evolutionary approaches. Mol. Biol. Evol. 32, 2417–2432. doi: 10.1093/molbev/msv125
Keywords: programmed cell death, filamentous fungi, calcium, ROS, metacaspases, NLR, BCL-2 family
Citation: Gonçalves AP, Heller J, Daskalov A, Videira A and Glass NL (2017) Regulated Forms of Cell Death in Fungi. Front. Microbiol. 8:1837. doi: 10.3389/fmicb.2017.01837
Received: 29 July 2017; Accepted: 07 September 2017;
Published: 21 September 2017.
Edited by:
Alex Andrianopoulos, University of Melbourne, AustraliaReviewed by:
Olaf Kniemeyer, Leibniz-Institut für Naturstoff-Forschung und Infektionsbiologie, Hans Knöll Institut, GermanyVito Valiante, Leibniz-Institut für Naturstoff-Forschung und Infektionsbiologie, Hans Knöll Institut, Germany
Copyright © 2017 Gonçalves, Heller, Daskalov, Videira and Glass. This is an open-access article distributed under the terms of the Creative Commons Attribution License (CC BY). The use, distribution or reproduction in other forums is permitted, provided the original author(s) or licensor are credited and that the original publication in this journal is cited, in accordance with accepted academic practice. No use, distribution or reproduction is permitted which does not comply with these terms.
*Correspondence: N. Louise Glass, TGdsYXNzQGJlcmtlbGV5LmVkdQ==