- 1Department of Bio-and Environmental Sciences, Technische Universität Dresden–International Institute Zittau, Zittau, Germany
- 2Enzyme Technology Unit, Brandenburg University of Technology, Cottbus, Germany
Unspecific peroxygenases (UPOs) are secreted fungal enzymes with promiscuity for oxygen transfer and oxidation reactions. Functionally, they represent hybrids of P450 monooxygenases and heme peroxidases; phylogenetically they belong to the family of heme-thiolate peroxidases. Two UPOs from the basidiomycetous fungi Agrocybe aegerita (AaeUPO) and Marasmius rotula (MroUPO) converted 35 out of 40 compounds listed as EPA priority pollutants, including chlorinated benzenes and their derivatives, halogenated biphenyl ethers, nitroaromatic compounds, polycyclic aromatic hydrocarbons (PAHs) and phthalic acid derivatives. These oxygenations and oxidations resulted in diverse products and—if at all—were limited for three reasons: (i) steric hindrance caused by multiple substitutions or bulkiness of the compound as such (e.g., hexachlorobenzene or large PAHs), (ii) strong inactivation of aromatic rings (e.g., nitrobenzene), and (iii) low water solubility (e.g., complex arenes). The general outcome of our study is that UPOs can be considered as extracellular counterparts of intracellular monooxygenases, both with respect to catalyzed reactions and catalytic versatility. Therefore, they should be taken into consideration as a relevant biocatalytic detoxification and biodegradation tool used by fungi when confronted with toxins, xenobiotics and pollutants in their natural environments.
Introduction
The most important classes of organic pollutants in the environment are mineral oil constituents as well as halogenated and nitrated products of petrochemicals. Enzymatic transformation and degradation of such recalcitrant compounds, many of them xenobiotics in sensu stricto, generally proceeds via two modes: reductive and oxidative attack (Spain, 1995; Durán and Esposito, 2000; Ye et al., 2004). Oxidoreductases, (e.g., dehydrogenases and oxygenases/[per]oxidases, respectively) play key roles in both degradative strategies and have been well studied (Durán and Esposito, 2000). Oxidases, oxygenases and peroxidases (POX) can be classified according to their co-substrates; the most important of them—involved in the aerobic degradation of numerous organic pollutants—are shortly discussed below.
Polyphenol oxidases, i.e., laccase (LAC) and tyrosinase (TYR), are copper containing enzymes that catalyze the oxidation of phenolic compounds with dioxygen (O2) as electron acceptor and without the need of additional co-enzymes, such as NAD(P)H. They are found in almost all domains of aerobic life and fulfill diverse metabolic functions (Ullrich and Hofrichter, 2007). Whereas, LAC does not directly incorporate oxygen into substrates, TYR can do so along with phenol/catechol oxidation (Jergil et al., 1983; Majcherczyk et al., 1998). Nevertheless, also LAC may indirectly oxyfunctionalize molecules, for example, polycyclic aromatic hydrocarbons (PAHs) or phenols, via one-electron oxidation followed by disproportionation and water addition (Majcherczyk et al., 1998; Wu et al., 2008).
Oxidases using O2 and reduced co-enzymes (e.g., NAD(P)H or FADH2) as oxygen donor (electron acceptor) and electron donor, respectively, are usually referred to as oxygenases (Guengerich, 2001). In dependence on the number of oxygen atoms introduced into the substrate molecule, monooxygenases, and dioxygenases are distinguished. Cytochrome-P450 monooxygenases (P450s) are heme-thiolate proteins where a porphyrin moiety (iron protoporphorin IX; heme) is ligated via a cysteine residue to the polypeptide chain (α-helix) of the apo-enzyme (Munro et al., 2012). The protein superfamily of P450s is highly diverse and comprises versatile intracellular biocatalysts found in all domains of life (Anzenbacher and Anzenbacherová, 2001; Meunier et al., 2004; Munro et al., 2012), and even virally encoded P450s have been described (Lamb et al., 2009). P450s usually utilize NAD(P)H as electron-delivering co-substrate but some of them can also catalyze monooxygenations with peroxides as oxygen donor via the so-called shunt pathway (Munro et al., 2012). While some P450s play specific anabolic roles, e.g., in sterol biosynthesis (Lepesheva and Waterman, 2004), others are rather unspecific and involved in the metabolism of xenobiotics, toxins and drugs (Anzenbacher and Anzenbacherová, 2001; Guengerich, 2001).
Multicomponent monooxygenases (BMMs) represent another family of versatile biocatalysts transferring oxygen to various substrates (Leahy et al., 2003). Thus, toluene 4-monooxygenase and methane monooxygenase are capable of oxygenating—in addition to their eponymous substrates—diverse alkenes and arenes including hardly reactive benzene (Whited and Gibson, 1991; Sazinsky et al., 2004). BMMs have only been found (and characterized) in bacteria and archaea so far (Notomista et al., 2003). Other non-heme monooxygenases contain flavin as prosthetic group (FMOs) (van Berkel et al., 2006; Huijbers et al., 2014). They activate O2 with a reduced flavin cofactor to form a peroxyflavin that attacks the substrate (van Berkel et al., 2006). As P450s, FMOs are found in bacteria and eukaryotes (van Berkel et al., 2006).
Oxygenases that catalyze the incorporation of the entire O2 molecule are called dioxygenases (DIOXs). Most DIOXs are iron containing enzymes, e.g., Rieske-type DIOXs (also referred to as arene DIOXs) (Bugg and Ramaswamy, 2008). Rieske-type DIOXs contain a [2Fe-2S] cluster and preferably catalyze the formation of cis-dihydroxylated metabolites (Ferraro et al., 2005). Arene DIOXs are capable of oxidizing inactivated arenes, such as toluene, benzene and even nitrobenzene (Lessner et al., 2002; Bagnéris et al., 2005).
All types of oxygenases can be involved in the detoxification and biodegradation of organic pollutants and xenobiotics by microorganisms and often they initiate catabolic pathways resulting in the utilization of these compounds as soles carbon and energy sources (Fewson, 1988; Copley, 2000). In this context, the incorporation of oxygen does not only activate the molecules but also increases the compounds' water solubility and hence their bioavailability. That way, many compounds listed as EPA priority pollutants, such as benzene and its derivatives, become accessible to enzymatic attack by other enzymes (e.g., ring-fission enzymes, POX or LAC) upon hydroxylation.
Secreted peroxidases, such as fungal lignin peroxidase (LIP), manganese peroxidase (MNP), and versatile peroxidase (VP), plant horseradish peroxidase (HRP), animal dehaloperoxidase and lactoperoxidase as well as bacterial and fungal dye-decolorizing peroxidases (DYPs), are typical degradative and detoxifying biocatalysts that utilize hydrogen peroxide as electron acceptor (Camarero et al., 1999; Piontek et al., 2001; Hofrichter, 2002; Osborne et al., 2009; Strittmatter et al., 2011). They all contain heme as prosthetic group that is linked via a proximal histidine to the polypeptide chain (heme-imidazole POX) (Ullrich and Hofrichter, 2007). Heme peroxidases are known from all kingdoms of life (Vlasits et al., 2010). Because of the involvement of some of them in lignin biodegradation (MNP, LIP, and VP), which opens an eco-physiological niche for specialized fungi (basidiomycetous white-rotters and litter-decomposers) and is of general interest for the pulp and paper sector, they have been intensely studied over the last three decades (Kirk and Farrell, 1987; Hofrichter, 2002; Martínez et al., 2009). Interestingly, it has recently been proposed that the appearance of fungal ligninolytic peroxidases led to the end of the carboniferous period (Floudas et al., 2012). In addition to lignin, these enzymes were found to efficiently oxidize diverse organic pollutants as well and hence were proposed to be part of unspecific bioremediation/bioattenuation systems in nature (Pointing, 2001; Hammel and Cullen, 2008; Qayyum et al., 2009; Harms et al., 2011).
Unlike the ligninolytic heme peroxidases, the heme iron of chloroperoxidase (CPO) from the ascomycete Caldariomyces (Leptoxyphium) fumago (Dawson, 1988) is linked to a cysteine (heme-thiolate peroxidase-HTP), as in the case of P450s. In 2004, a new HTP type was discovered, which is presently known as unspecific peroxygenase (UPO) (Ullrich et al., 2004; Ullrich and Hofrichter, 2005; Hofrichter and Ullrich, 2006) representing a functional hybrid of peroxidases and P450s (Hofrichter and Ullrich, 2006; Hofrichter et al., 2015). Besides prototypical peroxidase reactions like one electron oxidations, UPO transfers hydrogen peroxide-borne oxygen and catalyze various hydroxylations, e.g., of aromatic and aliphatic hydrocarbons (Kluge et al., 2009, 2012; Aranda et al., 2010; Kinne et al., 2010; Peter et al., 2011; Karich et al., 2013). Moreover, epoxidation, sulfoxidation, heterocyclic N-oxidation, and ether cleavage (O-dealkylation) have been reported (Ullrich et al., 2008; Aranda et al., 2009; Kinne et al., 2009b; Kluge et al., 2012), and moreover, UPO has catalase and haloperoxidase activities (Hofrichter et al., 2015). Thus, UPO combines features of LAC/POX (one-electron oxidation), monooxygenases (incorporation of one oxygen atom into the substrate) and POX/catalase (H2O2 as co-substrate) and hence represents a multifunctional type of oxidoreductase with almost catalytic promiscuity (Pandya et al., 2014; Hofrichter et al., 2015).
UPO genes are ubiquitous in the fungal kingdom (Eumycota) and beyond that, have only been found in the (super) phyllum of heteroconta (e.g., in fungus-like Peronosporales belonging to the former class of oomycetes and in a few diatoms) (Pecyna, 2016). Horizontal gene transfer from ascomycetes was proposed to be the most probable explanation for the occurrence of UPO genes in the latter organisms, leading to the conclusion that they are an autapomorphic feature in the kingdom of fungi (Pecyna, 2016).
Phylogenetically, Unspecific peroxygenases (UPOs) can be classified into two large groups/families, which differ, among others, in molecular size: (i) group I, the short UPOs with an average mass of 29 kDa and (ii) group II, the long UPOs with an average mass of 44 kDa (compare Figure 1; Hofrichter et al., 2015). The latter are exclusively found in ascomycetes and basidiomycetes, while the former are distributed among all fungal phyla (compare Figure 1; Hofrichter et al., 2015). The herein studied UPOs of Agrocybe aegerita (AaeUPO) and Marasmius rotula (MroUPO) belong to the long and short basidiomycetous UPOs, respectively. Interestingly, the above-mentioned CPO, which had been an “orphan” among the heme peroxidases for decades, can now be classified into group I of UPOs/HTPs as well.
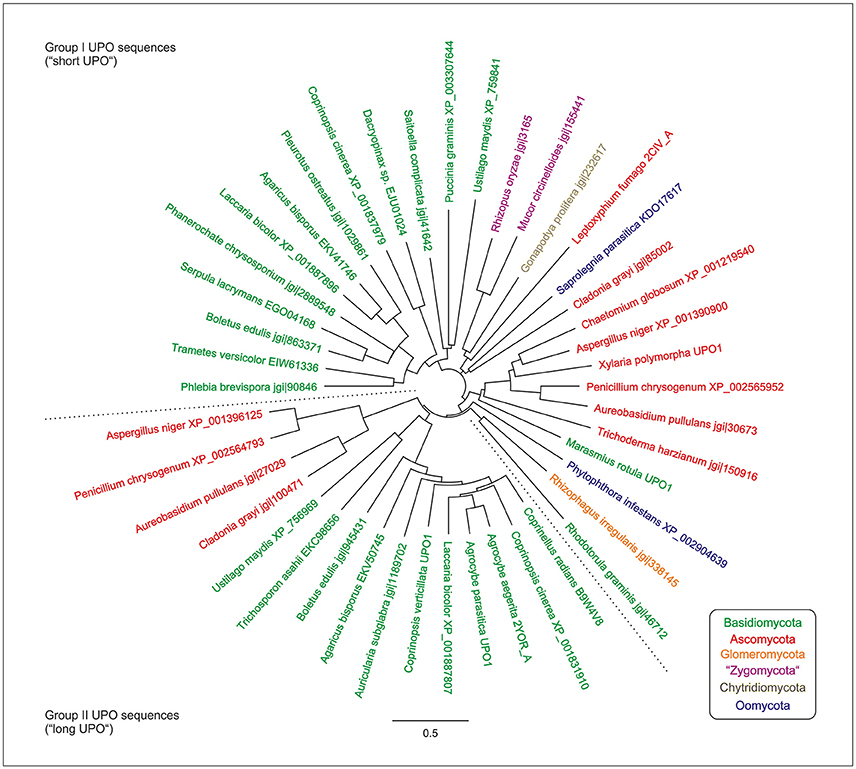
Figure 1. Neighbor-joining phylogenetic tree of UPO/HTP-sequences using Jukes-Cantor genetic distances; updated according to Hofrichter et al. (2015). Green, Basidiomycota; red, Ascomycota; blue, “Oomycota” (Peronosporales, Saprolegiales); purple, “Zygomycota” (Mucoromycotina); dark blue, Chytridiomycota; and orange, Glomeromycota. The dotted lines separate UPO sequences of groups I and II (short and long UPOs, respectively).
Against the background of widespread occurrence of UPOs in the fungal kingdom and their catalytic versatility, it is worth to study the conversion of a representative number of organic pollutants by these enzymes. So we have tested here 44 substrates, of which 40 are listed as EPA priority pollutants including chlorinated benzenes, halogenated biphenyl ethers, nitroaromatics, PAHs and phthalates (USEPA, 1979).
Materials and Methods
Enzyme Preparation and Chemicals
AaeUPO and MroUPO were prepared as described by Ullrich et al. (2004) and Gröbe et al. (2011), respectively. Final enzyme preparations had specific activities of 98 and 28 U/mg for AaeUPO and MroUPO, respectively. Chemicals used were purchased from Sigma Aldrich-Germany (Munich, Germany) with the highest purity available.
Enzyme Assay and Reaction Setup
Enzymatic activity of UPOs was routinely assayed by following the oxidation of veratryl alcohol to veratraldehyde at 310 nm (ε310 = 9,300 M−1 cm−1) in a buffered reaction mixture (pH 7.0) according to Ullrich et al. (2004). Photometric measurements were performed using a Cary Bio 50 spectrophotometer (Varian Inc., Walnut Creek, CA, USA).
Enzymatic reactions were performed in triplicate in 1.5-mL HPLC vials containing 50 mM potassium phosphate buffer (pH 7) and acetronitrile at concentrations between 5 and 30% vol/vol. The substrate concentration ranged from 0.1 to 1 mM, e.g., in the case of large PAHs and phenolic compounds, respectively. The total reaction volume varied between 0.5 and 1 mL. Reactions were started by addition of H2O2 (final concentration 0.5–2 mM). H2O2 was added via syringe pumps over 30 min or, in the case of large PAHs, over 2 h. In additional reaction setups, ascorbic acid (4 mM) was added to the reaction mixtures to prevent polymerization starting from intermediate phenoxy radicals formed by one-electron oxidation (Kinne et al., 2009a). The final enzyme activity (AaeUPO and MroUPO) in the reaction mixtures ranged between 0.5 and 1 U/mL (veratryl alcohol units measured at pH 7). Controls for each reaction setup were run without enzyme. Detailed information on the reaction setups, analytical methods and some specific results (e.g., HPLC elution profiles) are given in the Supplementary section.
Sample Preparation
Samples from the reaction mixtures were collected 30 min after the reaction had started and stopped by injection into an HPLC system or by addition of 1 mM sodium azide. To ensure dissolving, in a few cases, the reaction mixtures were diluted with acetonitrile (e.g., for the analysis of phthalic acid derivatives or small PAHs, such as acenaphthene) or acetone (e.g., for large PAHs, such as benzo[a]pyrene or benz[a]anthracene) prior to injection.
HPLC Analyses
Reaction products were analyzed by HPLC with MS and/or UV-Vis detection using an Agilent Series 1,200 instrument equipped with a diode array detector (Agilent Technologies Deutschland GmbH, Böblingen, Germany). The HPLC system was generally equipped with reversed phase columns; i.e., Luna C18(2), Synergi polar, Kinetex C18 and Kinetex PFP (each supplied by Phenomenex, Aschaffenburg, Germany). An electrospray ionization mass spectrometer (6310 IonTrap, Agilent Technologies Germany GmbH), in negative and positive mode, was used to determine mass-to-charge ratios of substrates tested and metabolites formed in the course of UPO reactions.
Oxidation products were identified by comparing their retention times, mass and spectral data with authentic standards (so far available) and with literature data. In the absence of standards or reference data, metabolites were tentatively assigned according to their mass and UV-Vis spectra.
Results and Discussion
The main outcome of the enzymatic oxidation tests with two fungal UPOs and diverse EPA organopollutants is summarized in Table 1. This includes the respective type of substrate functionalization (incorporation of oxygen, release of functional groups, one-electron oxidation, etc.), the relative conversion of the tested compounds in semi-quantitative form (five levels of conversion) and the products formed. Polymerization products were observed in all cases, in which phenolic groups were present in the substrates or emerged in the course of the reaction as intermediates (with the exception of 2,4-dinitrophenol).
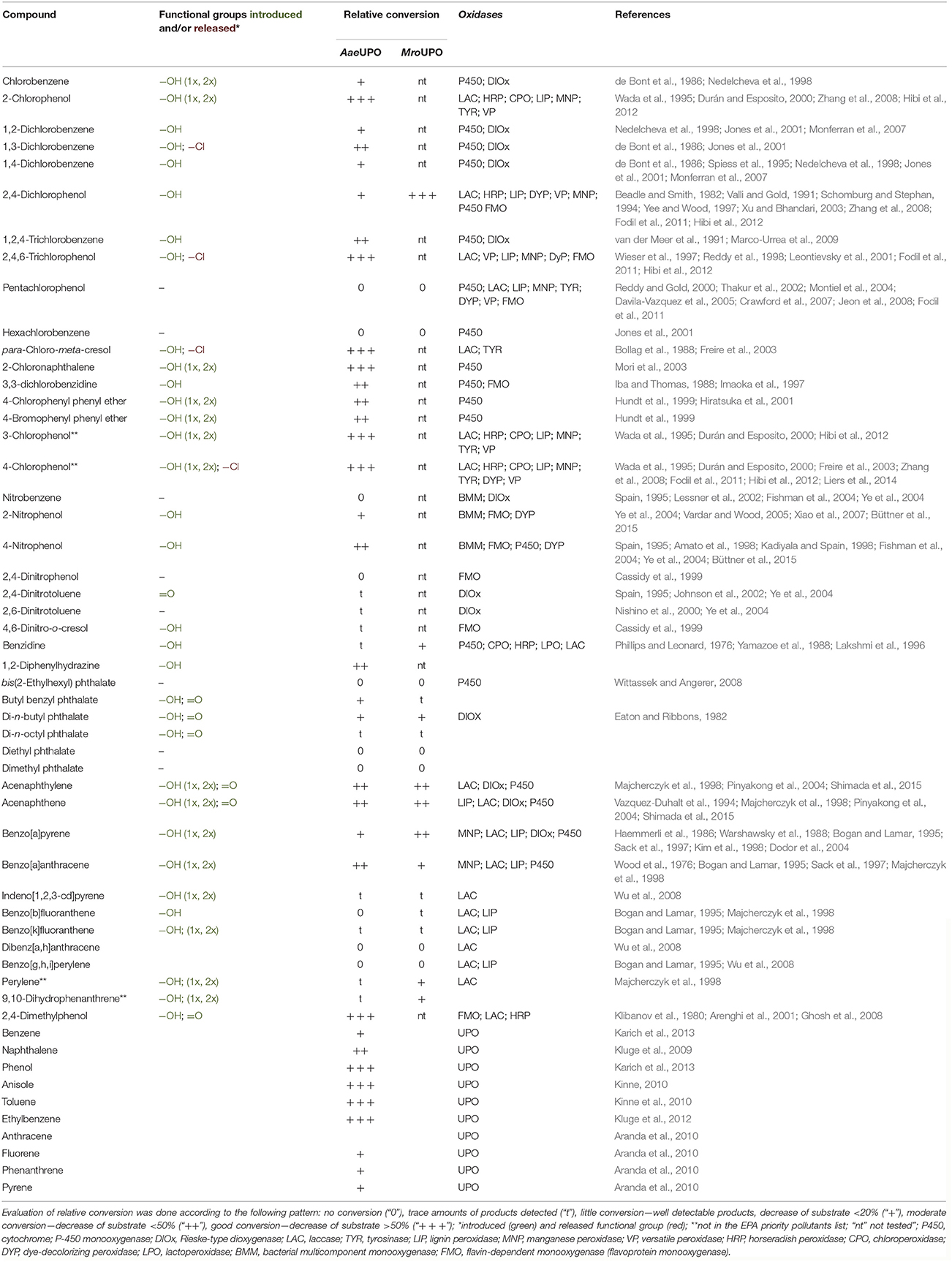
Table 1. EPA priority pollutants (and a few other recalcitrant compounds) tested with respect to their conversion by UPOs, including references to literature data of other oxidoreductases.
Chlorobenzene and Its Derivatives
Chlorobenzene (1) was oxygenated by AaeUPO to give 2-chlorophenol (2) and 4-chlorophenol (3) as major products; oxygenation at the meta position of 1 and thus formation of 3-chlorophenol (4) was not observed. Further oxidation of 2 led to 3-chlorocatechol (5) and chlorohydroquinone (6), whereas oxidation of 3 gave 4-chlorocatechol (7); 5, 6, and 7 are direct oxygenation products of 2, 3, or 4, respectively (Figures 2A,B). p-Benzoquinone (8) was detected (Figure 2A) when ascorbic acid was omitted from the reaction mixture of 3; vice versa, 8 was not observed in the presence of ascorbic acid. Hence 8 must be a product deriving from two consecutive or parallel enzymatic one-electron oxidations, which represents a type of oxidative dehalogenation known from LAC and POX (Hammel and Tardone, 1988; Osborne et al., 2007; Kordon et al., 2010). Hydrogen abstraction at the phenolic function of 3 would give a phenoxy radical. Two of the latter can disproportionate to 3 and an arene cation (Ullrich and Hofrichter, 2007). A nucleophile, e.g., water, may add to the aromatic cation and subsequent elimination of hydrochloric acid leaves 8 behind, analogously to the Ritter reaction (Krimen and Cota, 2004). The pathway described resembles the enzymatic dehalogenation of 3 described for dehaloperoxidases (Osborne et al., 2009). Masses of triple hydroxylated products arising from 5 to 7 were detected in low amounts; however, their unambiguous identification was not possible, due to the lacking of authentic standards.
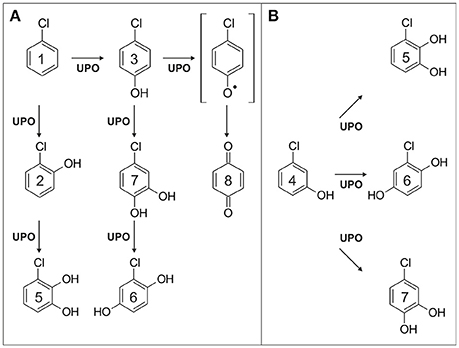
Figure 2. Proposed reaction scheme of chlorobenzene (scheme A, 1) and 3-chlorophenol oxidation (scheme B; 4) catalyzed by AaeUPO; formation of the phenoxy radical is postulated.
Oxygenation of three dichlorobenzenes was indicated by the detection of the corresponding dichlorophenols in the reaction mixture. In the case of 1,3-dichlorophenol, dehalogenation occurred in a second step upon oxygenation giving rise to chlorohydroquinone. The reaction cascade is assumed to proceed analogously to the dehalogenation of 3. Dehalogenation products were also observed when p-chloro-m-cresol and 2,4,6-trichlorophenol were applied as UPO substrates.
Interestingly, the conversion of chlorinated benzenes did not follow the expected reaction sequence; thus the introduction of chlorine substituents usually decreases the charge density of the aromatic system and hence inactivates the latter. However, all three dichlorobenzenes and 1,2,3-trichlorobenzene where more effectively oxidized by AaeUPO than 1. A possible explanation for that finding could be the “steric fixation” of the substrate molecule inside the heme pocket, positively affected by two or more chlorine substituents, resulting in a closer distance to the enzyme's reactive compound I and/or less motion within the heme pocket. To our best knowledge, only P450s and DIOX have been reported to oxygenate mono- and dichlorinated benzenes (de Bont et al., 1986; Spiess et al., 1995; Nedelcheva et al., 1998; Jones et al., 2001; Monferran et al., 2007).
All three tested chlorophenols were oxygenated by AaeUPO. This was most evident when ascorbic acid was present in the reaction mixtures, which prevented polymerizing side activities. Chlorocatechols (i.e., 5 and 7 and not chlorohydroquinones) were the major products deriving from the oxygenation of 3 and 4. This is an interesting fact, since chlorocatechols are the substrates of ring-cleaving DIOX within intracellular degradation pathways of chlorinated arenes (Kaschabek et al., 1998; Moiseeva et al., 2002). Thus, we can consider UPOs being involved in fungal catabolic routes of chloroaromatics, with the advantage that toxic chlorophenols will not have to be taken up into the hyphae (Mars et al., 1997). Chlorophenols and chlorocatechols can additionally serve as substrates for one-electron oxidations and thus, besides oxygenases (Beadle and Smith, 1982; Xu and Bhandari, 2003), several POX and phenol oxidases (LAC, TYR) were found to oxidize chlorinated phenols and their derivatives to reactive phenoxy radicals (Xu and Bhandari, 2003; Zhang et al., 2008; Hibi et al., 2012).
Neither oxygenation nor one-electron oxidation was observed when hexachlorobenzene (HCB) and pentachlorophenol (PCP) were applied as substrates for AaeUPO and MroUPO. They are the only halogenated compounds tested here that were not converted. Chlorine substituents in higher number may protect the arene C-atoms from attack by UPO's compound I via steric hindrance and/or the impossibility to find a suitable point of attack. On the other hand, some P450s were found to be able to oxygenate both HCB and PCP (Jones et al., 2001; Crawford et al., 2007), and the phenolic functionality of PCP makes it susceptible to one-electron oxidation catalyzed by phenol oxidases and POX (Reddy and Gold, 2000; Montiel et al., 2004; Jeon et al., 2008; Fodil et al., 2011). All other tested halogenated compounds (compare Table 1) served also as substrates for UPOs, but are not explicitly discussed here; more pieces of information are given in the Supplementary section.
Benzoquinones and polymerization products emerged in all reaction setups where ascorbic acid was omitted. The latter acted as radical scavenger that reduced chlorinated phenoxy radicals formed via one-electron oxidation (peroxidative activity of UPO) and prevented that way radical coupling (Niki, 1991).
Nitroarenes
The charge density at the aromatic ring is reduced by nitro substituents; thus, with regard to electrophilic attack, nitroarenes are strongly deactivated compounds (McDaniel and Brown, 1958; Spain, 1995). This property is reflected by the low reactivity of UPOs toward nitroaromatic compounds (compare Table 1) and consequently, oxygenation of nitrobenzene was not observed. On the other hand, 2-nitrophenol and 4-nitrophenol served as substrates and were oxidized into the corresponding dihydroxylated nitrobenzenes, which in turn underwent one-electron oxidation resulting in the formation of coupling products. A second nitro group (e.g., 2,4-dinitrophenol), however, made an enzymatic attack by UPOs impossible. Trace amounts of oxidation products were found when 2,4-dinitrotoluene (9) and 4,6-dinitro-o-cresol were applied as substrates. Since the electron density at the aromatic ring of 9 is lower than in 2,4-dinitrophenol, it is assumed that hydroxylation took place at toluene's methyl group, which in case of 2,6-dinitrotoluene is shielded by two flanking nitro groups preventing attack by UPO compound I. A second indication for the oxidation at the benzylic carbon of 9 is the mass shift of “+14” for one of the products detected, which cannot be explained by aromatic ring hydroxylation. In consequence, we conclude that 9 was attacked by two consecutive two-electron oxidations (compare Figure 3) resulting in the formation of 2,4-dinitrobenzaldehyde via the corresponding benzyl alcohol and gem-diol (aldehyde hydrate) intermediates. This finding confirms similar observations previously made for 4-nitrotoluene oxidation by AaeUPO (Kinne et al., 2010).
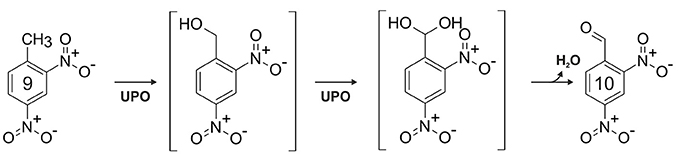
Figure 3. Proposed oxidation sequence for 2,4-dinitrotoluene (9) leading to 2,4-dinitrobenzaldehyde (10).
Overall, these results are not surprising when considering literature data of other enzymes. Only a few oxidoreductases are able to oxidize nitrobenzene, e.g., a few DIOXs and BMMs (Spain, 1995; Fishman et al., 2004). The latter was also found to oxidize nitrophenols (Fishman et al., 2004). Furthermore, oxygenation of nitrophenols was reported for some P450s and FMOs (Cassidy et al., 1999; Ye et al., 2004), whereas one-electron oxidation of nitrophenols can be realized by high-redox potential POX, e.g., DYP (Büttner et al., 2015). In contrast, reductive pathways for nitroaromatics are widely distributed in nature and have been well summarized in previous reviews (Spain, 1995; Ye et al., 2004).
Phthalate Esters
In the course of a screening, six phthalate esters (PEs) were tested for oxygenation by AaeUPO and MroUPO and three of them were converted: butyl benzyl phthalate, di-n-butyl and di-n-octyl phthalate. In case of the latter, only trace amounts of products could be detected. Analogously to 2,4-dinitrotoluoene, the “+14” mass shift of products rules out that the oxygen insertion occurred at the aromatic ring and thus oxygenation at the β-position of the alkyl moieties (i.e., butyl or octyl) is most plausible (Peter et al., 2011). No conversion was observed for bis(2-ethyl-hexyl) phthalate and the short chain PEs, such as dimethyl phthalate and diethyl phthalate.
Most studies dealing with the degradation of PE have used whole cells (bacterial or fungal pure or mixed cultures). Hydrolysis of the ester bond by esterases was the typical reaction observed (Wang et al., 1995, 2003; Staples et al., 1997). The ring of phthalic acid can be oxidized by a specific bacterial DIOX resulting in the formation the corresponding catechol (Eaton and Ribbons, 1982); side chain oxidation of PEs was also reported for human PE metabolism probably realized by liver P450s (Wittassek and Angerer, 2008). Wittassek and Angerer (2008) reported the oxidation of long chain PE, e.g., di(2-ethyl-hexyl) phthalate, by a P450 and emphasized that short chain PEs, (e.g., dimethyl and diethyl) phthalate, were not oxidized by this enzyme; an observation that corresponds with our findings here.
Polycyclic Aromatic Hydrocarbons
Eleven PAHs were tested for the conversion by AaeUPO and MroUPO. The majority of them was in fact oxygenated and oxidized by both UPOs with the exception of bulky dibenz[a,h]anthracene and benzo[g,h,i]perylene; benzo[b]fluoranthene was a substrate for MroUPO only. In dependence on the particular PAH, the extent of product formation reached from trace amounts (e.g., benzo[k]fluoranthene) to substantial amounts (e.g., acenaphthylene).
From all PAHs tested, acenapthylene (14) was oxidized to the highest extent by both UPOs. The major product detected was a monohydroxylated metabolite (m/z +16) with a UV-Vis spectrum resembling that of 1-naphthol (Kluge et al., 2009) (data in suplement). Hence, we assume that oxygenation of 14 proceeded via a 4,5-epoxy acenaphthylene intermediate to give 5-hydroxy acenaphthylene, analogously to naphthalene oxygenation catalyzed by UPO (Kluge et al., 2009). Another interesting finding was the detection of acenaphthenone (13) in the reaction setup of 14. Oxygenation of 14 led to 1-hydroxy-acenaphthylene (15) that is the enolic form of 13. Again, an epoxide intermediate can be postulated, i.e., 1,2-epoxy acenapthene (compare Figure 4).
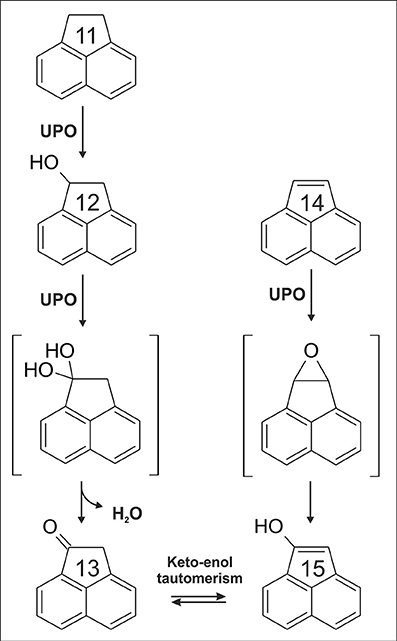
Figure 4. Proposed reaction sequence for the formation of acenaphthenone (13) deriving from acenaphthene (11) or acenaphthylene (14).
Thirteen (13) was also a product deriving from acenaphthene (11) that was next to 14 the best PAH substrate. Oxygenation of a sp3-carbon (aliphatic carbon) may give 1-acenaphthol (12); via a second attack at the same carbon (i.e., at C1 position), a geminal diol intermediate (gem-diol, carbonyl hydrate) may be formed that is in equilibrium via spontaneous dehydration with the corresponding ketone (13, Figure 4). Similar to 14, oxygenation at the aromatic system of 11 was observed as well. However, in contrast to the reaction setup of 14, two oxygenation products were detected with UV-Vis spectra resembling those of 1-naphthol and 2-naphthol (Kluge et al., 2009). The reported oxidation pathway of P450s for 11 and 14 is rather similar to the reaction sequences proposed herein, including the proof of 1,2-epoxy-acenapthene formation (only the formation of 13 was not ascertained for P450s) (Shimada et al., 2015). Conversion of all other (positively) tested PAHs was evident by detection of products with mass shifts of +16 or +32, representing mono- and dihydroxylated products, respectively.
Water solubility and thus bioavailability decreases with increasing size of PAHs and this fact was reflected by their decreasing relative conversion by UPOs the bigger they were (compare Table 2). It has to be noted that the two UPOs tested accomplished the formation of different PAH products and patterns; thus, MroUPO was capable of hydroxylating more bulky PAHs than AaeUPO. This can be explained by the wider heme channel of MroUPOs (11 Å) compared to the relatively narrow channel of AaeUPO (7 Å) (Poraj-Kobielska, 2013; Piontek et al., 2017), which limits AaeUPO to oxidize PAHs that are larger than 6 Å in diameter (e.g., dibenz[a,h]anthracene or benzo[g,h,i]perylene). The results of the UPO-catalyzed conversion of selected PAHs in relation to some physicochemical properties are summarized in Table 2. Aranda and coworkers had already reported about the successful conversion of several PAHs and related compounds by AaeUPO in 2010 (Aranda et al., 2010); four of these PAHs are also listed in Table 2, namely anthracene, fluorene, phenanthrene, and pyrene.
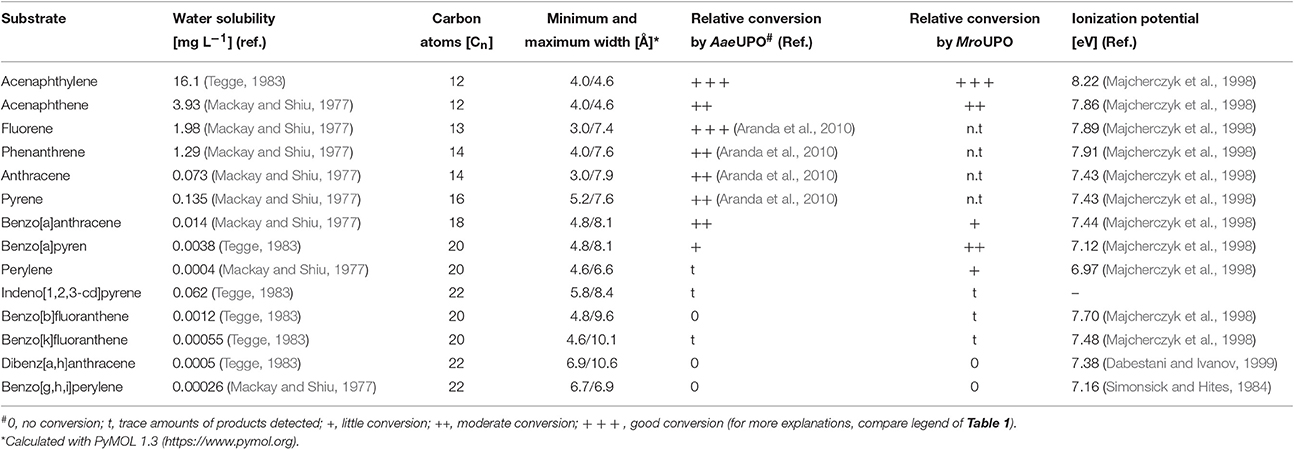
Table 2. Conversion of selected PAHs by AaeUPO and MroUPO with reference to their water solubility and molecular size.
Typical enzymes capable of catalyzing the oxygenation of PAHs are P450s and DIOX (Shimada et al., 1989; Pinyakong et al., 2004). Among fungi, especially P450s were shown to directly oxygenate PAHs (van Gorcom et al., 1998). However, PAHs can be also attacked by enzymes catalyzing one-electron oxidations, such as different POX and LAC, whereat their oxidizability will depend on the ionization potential (IP) and the presence of suitable redox mediators (Sack et al., 1997; Majcherczyk et al., 1998; Johannes and Majcherczyk, 2000; Haritash and Kaushik, 2009). The reaction of these enzymes leads, via instable aryl cations and water addition, to the formation of PAH quinones, in particular in the case of 4-ring and 5-ring PAHs with IP <7.7 eV (Hammel, 1995; Steffen et al., 2003). Quinoid products (whose formation would have been indicated by a mass shift of m/z +28), however, were not detected in our study and the main reaction products of 4- and 5-ring PAHs oxidized by UPOs were mono-hydroxylated products. Thus, substantial one-electron oxidation can be ruled out and hence, the oxyfunctionalizations observed had to be the result of true oxygen transfers (and not of water addition) (Hammel et al., 1986). This finding is largely consistent with the data presented by Aranda et al. (2010) for smaller PAHs, although quinones (e.g., anthraquinone) were observed as minor products. When the data presented herein are being compared with literature data, it becomes evident that quinones are detectable in decreasing order beginning with benzene>naphthalene>anthracene>4- and 5-ring PAHs (e.g., benz[a]anthracene and perylene, respectively); hence the formation of quinoid products from arenes, catalyzed by UPOs, is inversely proportional to the size of the aromatic system (Kluge et al., 2007; Aranda et al., 2010; Karich et al., 2013).
Oxidation of PAHs by ligninolytic peroxidases (e.g., MNP, LIP) and LAC is strictly dependent on the substrates' IP and high-molecular mass PAHs, e.g., benzo[g,h,i]perylene or benzo[a]pyrene, were found to be faster oxidized than smaller PAHs, some of which cannot be oxidized at all (e.g., phenanthrene and fluoranthene) (Steffen et al., 2003). In contrast, UPOs favor low-molecular mass PAHs over high-molecular ones as oxygen acceptor and the substrate IP seems to be of minor relevance for the extent of conversion (Table 2).
Other Substrates
Benzidine (18), 1,2-diphenylhydrazine (16) and 2,4-dimethylphenol do not really fit into the above classification of potential UPO substrates; therefore, we deal with them herein separately. Treatment of 18 samples with AaeUPO and MroUPO mainly resulted in the formation of coupling products. However, in the case of MroUPO, an oxygenation product of 18 was detectable as well. Again, the larger heme channel of MroUPO (compared to AaeUPO) may explain that fact. We assume that 3-hydroxybenzidine (19) was the product formed, which is most plausible to proceed via a lateral oxidative attack on 18. Azo derivatives based on 18 have been widely used as textile dyes and some of them are known to be carcinogenic (Lowry et al., 1980). Thus, 18 has been subject of various (eco)toxicological studies with focus on its carcinogenicity. In this context, oxygenation/hydroxylation of 18 by P450s as well as its POX-catalyzed oxidation to benzidine diimine ensuing binding to DNA were described (Yamazoe et al., 1988; Lakshmi et al., 1996). To supplement this, we have shown here that UPOs are capable of catalyzing these reactions as well.
Studying 16-conversion turned out to be rather difficult for several reasons. The compound instantaneously autoxidizes in aqueous solution to cis- and trans-azobenzene (17) (Riggin and Howard, 1979) or it rearranges to 18 and diphenyline (Hammond and Shine, 1950; Ghigo et al., 2011); the latter reaction, however, is acid-dependent and was therefore not observed under the conditions applied here. Furthermore, authentic standards of potential 16 products are commercially not available and it was impossible to ionize 16 in a way to get a quality mass spectrum. Nevertheless, one product with a mass spectrum shift of “+32” in relation to 16 was detected and might be the result of two hydroxylations at the benzene rings of 16. Two products most probably deriving from the oxygenation of spontaneously formed 17 were additionally detected. The first product's mass spectrum shifted by m/z+16 in relation to 17 (and by m/z+14 compared to 16). The latter mass (+14) could hypothetically stand for a single keto function, which is, however, impossible to emerge at the aromatic rings of 16. Therefore, it may rather represent an oxygenation product of 17 (a hydroxy-azobenzene, 20). Logically, the second product (mass shift m/z+32 compared to 17) presumably resulted from a second hydroxylation of 17 (or it may represent a quinone of a double hydroxylated 16). However, whether cis- or trans-17 served as initial substrate, could not be exactly found out but the decrease of trans-17 in samples containing AaeUPO (compare Supplementary Material) implies the latter. A proposed pathway for spontaneous and UPO-catalyzed hydroxylation of 16 is given in Figure 5. Mammalian metabolism of 17 and derivatives usually proceeds via reductive pathways and 16 typically rearranges to 18 (Walker, 1970; Levine, 1991). Hydroxylation of 17 or 16 by mammalian P450 was not observed (Bray et al., 1951).
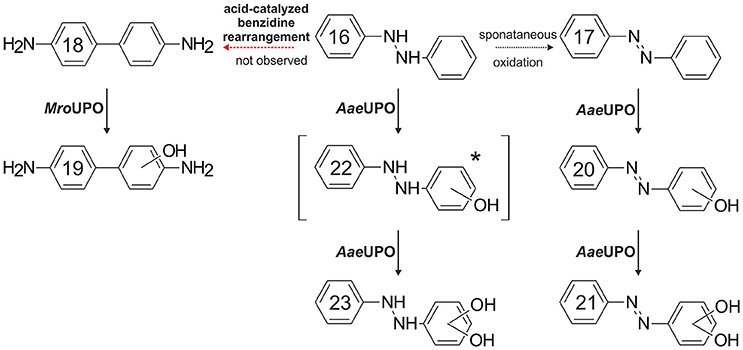
Figure 5. Proposed reaction sequence for the UPO-catalyzed hydroxylation of 1,2-diphenylhydrazine (16) and azobenzene (17). Detection of a double-hydroxylated 1,2-diphenylhydrazine would require a preceding hydroxylation of 16; however, a mono-hydroxylated 1,2-diphenylhydrazine (22) was not detected [*]. (16) spontaneously oxidized to trans- and cis-azobenzene (17); subsequent oxygenation catalyzed by AaeUPO resulted in single and double hydroxylated azobenzenes (20 and 21, respectively). An acid-catalyzed benzidine (18) rearrangement of 16 was not observed. Oxygenation of 18 was catalyzed by MroUPO only.
In the reaction setup of 2,4-dimethylphenol with AaeUPO, polymerization products, oxygenation products and combinations of both were found. This finding is not surprising, since methylphenols (cresols) are well-known substrates both for one-electron oxidations by POX (or LAC) (Klibanov et al., 1980; Ghosh et al., 2008) and for oxyfunctionalizations by P450s (Yan et al., 2005) and other monooxygenases (Arenghi et al., 2001). Thus, our results fit well to these reports and supplement an own previous study dealing with benzene oxidation by AaeUPO, in the course of which phenol emerged as an intermediate that was rapidly further converted (Karich et al., 2013).
Concluding Remarks
The majority of organopollutants tested here (i.e., 35 out of 44 substances, compare Table 1)—including xenobiotics, such as chlorinated benzenes and their derivatives, halogenated biphenyl ethers, nitroaromatic compounds, polycyclic aromatics and phthalates—were oxidatively converted by two fungal model UPOs. UPO-catalyzed oxidations were limited for three main reasons: (i) steric hindrance caused by the number of substituents or general bulkiness of the compound, e.g., hexachlorobenzene or large PAHs, such as benzo[g,h,i]perylene; (ii) strong inactivation of the aromatic ring by electron-withdrawing groups, e.g., nitrobenzene, and (iii) low bioavailability (water solubility) of the potential substrate.
Currently, 41 EPA priority pollutants have been reported to be oxidized by UPOs including the herein tested compounds and several other substances investigated in previous studies. Intensely investigated AaeUPO was found to oxygenate and oxidize numerous substance classes (Aranda et al., 2009, 2010; Kluge et al., 2009, 2012; Kinne et al., 2010; Peter et al., 2011; Poraj-Kobielska et al., 2011; Karich et al., 2013; Poraj-Kobielska, 2013) and at present, as much as 300 aromatic, heterocyclic, aliphatic and alicyclic compounds have been identified to serve as substrates for this enzyme (Hofrichter et al., 2015). This fact points out that UPOs are highly versatile oxidoreductases with an exceptional broad substrate spectrum. Besides UPOs, only P450s realize a comparable catalytic promiscuity for oxyfunctionalization reactions (compare Table 1). In fact, UPOs share with P450s the heme-thiolate as prosthetic group as well as highly reactive compound-I and protonated compound-II intermediates in the catalytic cycle (Yosca et al., 2017). On the other hand, UPOs and P450s do not share any sequence homology and act in different micro-environments (extracellularly vs. intracellularly). Maybe the catalytic systems of P450s and UPOs complement each other in a suitable way by eliminating similar, often toxic compounds inside and outside of fungal cells, respectively. Thus, UPOs are secretory enzymes using the rather simple co-substrate (H2O2) that can be generated outside the fungal hyphae, while P450s—as membrane-bound or cytosolic enzymes—use a complex accessory machinery, which allows their precise action in different hyphal compartments. In other words, UPOs can directly interact with the fungus' micro-environment and do rather “dirty catalytic jobs,” whereas P450s are responsible for the fine-tuning of similar reactions in the cells. UPOs may represent an extracellular equivalent to intracellular P450s, in which they function as a universal fungal detoxification system (“extracellular fungal liver”) that can oxidize plant ingredients, microbial metabolites and xenobiotics. The high number of putative UPO genes distributed among rather different ecological and phylogenetic groups of fungi (compare Figure 1) may strongly support this assumption.
Until now, all substrate conversion studies regarding UPOs have been carried out in cell-free systems with isolated enzyme preparations and it is still unclear, under which circumstances UPOs are induced and expressed in fungi under natural conditions. So it is rather difficult to appraise, which roles (others than detoxification) these enzymes may still play. Whilst the actual physiological functions of UPOs in individual fungi will still have to be elucidated, the wealth of catalyzed reactions is without doubt and in any case, interesting from the environmental and biotechnological points of view. Notably, UPOs do not only complement P450 activities but they may also support the action of extracellular fungal enzyme systems catalyzing one-electron oxidations, as needed for lignin and humus decomposition (i.e., POX and LAC reactions).
Future studies on UPOs will have to focus, amongst others, on the conditions, under which the production and secretion of UPOs are induced and how their activities can be stimulated in different ecological and phylogenetical groups of fungi. Because UPO genes are widely distributed in the whole fungal kingdom and fungi indeed permeate the living scene, a powerful tool may become available to foster bioattenuation processes, such as the self-cleaning function of soils.
Author Contributions
Conceived and designed the experiments: AK, RU, and MH. Performed the experiments: AK. Wrote the manuscript, supervised, and discussed the experiments and data: AK, RU, KS, and MH.
Funding
This research was supported by the EU projects INDOX (KBBE-2013-7-613549) and ENZOX2 (H2020-BBI-PPP-2015-2-1-720297). The funders did not influence the study design, data collection and analysis, the decision to publish, or preparation of the manuscript.
Conflict of Interest Statement
The authors declare that the research was conducted in the absence of any commercial or financial relationships that could be construed as a potential conflict of interest.
Acknowledgments
We thank Henrik Lund† (in memoriam) for numberless scientific discussions and his strong commitment to peroxygenases.
Supplementary Material
The Supplementary Material for this article can be found online at: http://journal.frontiersin.org/article/10.3389/fmicb.2017.01463/full#supplementary-material
Abbreviations
UPO, unspecific peroxygenase; Aae, Agrocybe aegerita; Mro, Marasmius rotula; POX, peroxidases; LAC, laccase; TYR, tyrosinase; PAH, polycyclic aromatic hydrocarbons; P450, cytochrome-P450 monooxygenase; BMM, Multicomponent monooxygenase; TMO, toluene 4-monooxygenase; DIOX, dioxygenase; LIP, lignin peroxidase; MNP, manganese peroxidase; VP, versatile peroxidase; HRP, horseradish peroxidase; DYP, dye-decolorizing peroxidase; CPO, chloroperoxidase; HCB, hexachlorobenzene; PCP, pentachlorophenol; PE, phthalate esters; IP, ionization potential.
References
Amato, G., Longo, V., Mazzaccaro, A., and Gervasi, P. G. (1998). Chlorzoxazone 6-hydroxylase and p-nitrophenol Hhydroxylase as the most suitable activities for assaying cytochrome P450 2E1 in cynomolgus monkey liver. Drug Metab. Dispos. 26, 483–489.
Anzenbacher, P., and Anzenbacherová, E. (2001). Cytochromes P450 and metabolism of xenobiotics. Cell. Mol. Life Sci. 58, 737–747. doi: 10.1007/PL00000897
Aranda, E., Kinne, M., Kluge, M., Ullrich, R., and Hofrichter, M. (2009). Conversion of dibenzothiophene by the mushrooms Agrocybe aegerita and Coprinellus radians and their extracellular peroxygenases. Appl. Microbiol. Biotechnol. 82, 1057–1066. doi: 10.1007/s00253-008-1778-6
Aranda, E., Ullrich, R., and Hofrichter, M. (2010). Conversion of polycyclic aromatic hydrocarbons, methyl naphthalenes and dibenzofuran by two fungal peroxygenases. Biodegradation 21, 267–281. doi: 10.1007/s10532-009-9299-2
Arenghi, F. L. G., Berlanda, D., Galli, E., Sello, G., and Barbieri, P. (2001). Organization and regulation of meta cleavage pathway genes for toluene and o-xylene derivative degradation in Pseudomonas stutzeri OX1. Appl. Environ. Microbiol. 67, 3304–3308. doi: 10.1128/AEM.67.7.3304-3308.2001
Bagnéris, C., Cammack, R., and Mason, J. R. (2005). Subtle difference between benzene and toluene dioxygenases of Pseudomonas putida. Appl. Environ. Microbiol. 71, 1570–1580. doi: 10.1128/AEM.71.3.1570-1580.2005
Beadle, C. A., and Smith, A. R. W. (1982). The purification and properties of 2,4-dichlorophenol hydroxylase from a strain of Acinetobacter species. Eur. J. Biochem. 123, 323–332. doi: 10.1111/j.1432-1033.1982.tb19771.x
Bogan, B. W., and Lamar, R. T. (1995). One-electron oxidation in the degradation of creosote polycyclic aromatic hydrocarbons by Phanerochaete chrysosporium. Appl. Environ. Microbiol. 61, 2631–2635.
Bollag, J. M., Shuttleworth, K. L., and Anderson, D. H. (1988). Laccase-mediated detoxification of phenolic compounds. Appl. Environ. Microbiol. 54, 3086–3091.
Bray, H., Clowes, R., and Thorpe, W. (1951). The metabolism of azobenzene and p-hydroxyazobenzene in the rabbit. Biochem. J. 49:lxv.
Bugg, T. D. H., and Ramaswamy, S. (2008). Non-heme iron-dependent dioxygenases: unravelling catalytic mechanisms for complex enzymatic oxidations. Curr. Opin. Chem. Biol. 12, 134–140. doi: 10.1016/j.cbpa.2007.12.007
Büttner, E., Ullrich, R., Strittmatter, E., Piontek, K., Plattner, D. A., Hofrichter, M., et al. (2015). Oxidation and nitration of mononitrophenols by a DyP-type peroxidase. Arch. Biochem. Biophys. 574, 86–92. doi: 10.1016/j.abb.2015.03.003
Camarero, S., Sarkar, S., Ruiz-Dueñas, F. J., Martınez, M. A. J., and Martınez, Á.T. (1999). Description of a versatile peroxidase involved in the natural degradation of lignin that has both manganese peroxidase and lignin peroxidase substrate interaction sites. J. Biol. Chem. 274, 10324–10330. doi: 10.1074/jbc.274.15.10324
Cassidy, M. B., Lee, H., Trevors, J. T., and Zablotowicz, R. B. (1999). Chlorophenol and nitrophenol metabolism by Sphingomonas sp UG30. J. Ind. Microbiol. Biotechnol. 23, 232–241. doi: 10.1038/sj.jim.2900749
Copley, S. D. (2000). Evolution of a metabolic pathway for degradation of a toxic xenobiotic: the patchwork approach. Trends Biochem. Sci. 25, 261–265. doi: 10.1016/S0968-0004(00)01562-0
Crawford, R., Jung, C., and Strap, J. (2007). The recent evolution of pentachlorophenol (PCP)-4-monooxygenase (PcpB) and associated pathways for bacterial degradation of PCP. Biodegradation 18, 525–539. doi: 10.1007/s10532-006-9090-6
Dabestani, R., and Ivanov, I. N. (1999). A compilation of physical, spectroscopic and photophysical properties of polycyclic aromatic hydrocarbons. Photochem. Photobiol. 70, 10–34.
Davila-Vazquez, G., Tinoco, R., Pickard, M. A., and Vazquez-Duhalt, R. (2005). Transformation of halogenated pesticides by versatile peroxidase from Bjerkandera adusta. Enzyme Microbial Technol. 36, 223–231. doi: 10.1016/j.enzmictec.2004.07.015
Dawson, J. (1988). Probing structure-function relations in heme-containing oxygenases and peroxidases. Science 240, 433–439. doi: 10.1126/science.3358128
de Bont, J. A., Vorage, M. J., Hartmans, S., and van den Tweel, W. J. (1986). Microbial degradation of 1,3-dichlorobenzene. Appl. Environ. Microbiol. 52, 677–680.
Dodor, D. E., Hwang, H.-M., and Ekunwe, S. I. N. (2004). Oxidation of anthracene and benzo[a]pyrene by immobilized laccase from Trametes versicolor. Enzyme Microbial Technol. 35, 210–217. doi: 10.1016/j.enzmictec.2004.04.007
Durán, N., and Esposito, E. (2000). Potential applications of oxidative enzymes and phenoloxidase-like compounds in wastewater and soil treatment: a review. Appl. Catal. B Environ. 28, 83–99. doi: 10.1016/S0926-3373(00)00168-5
Eaton, R. W., and Ribbons, D. W. (1982). Metabolism of dibutylphthalate and phthalate by Micrococcus sp. strain 12B. J. Bacteriol. 151, 48–57.
Ferraro, D. J., Gakhar, L., and Ramaswamy, S. (2005). Rieske business: structure–function of rieske non-heme oxygenases. Biochem. Biophys. Res. Commun. 338, 175–190. doi: 10.1016/j.bbrc.2005.08.222
Fewson, C. A. (1988). Biodegradation of xenobiotic and other persistent compounds: the causes of recalcitrance. Trends Biotechnol. 6, 148–153. doi: 10.1016/0167-7799(88)90084-4
Fishman, A., Tao, Y., Bentley, W. E., and Wood, T. K. (2004). Protein engineering of toluene 4-monooxygenase of Pseudomonas mendocina KR1 for synthesizing 4-nitrocatechol from nitrobenzene. Biotechnol. Bioeng. 87, 779–790. doi: 10.1002/bit.20185
Floudas, D., Binder, M., Riley, R., Barry, K., Blanchette, R. A., Henrissat, B., et al. (2012). The paleozoic origin of enzymatic lignin decomposition reconstructed from 31 fungal genomes. Science 336, 1715–1719. doi: 10.1126/science.1221748
Fodil, D., Badis, A., Jaouadi, B., Zaraî, N., Ferradji, F. Z., and Boutoumi, H. (2011). Purification and characterization of two extracellular peroxidases from Streptomyces sp. strain AM2, a decolorizing actinomycetes responsible for the biodegradation of natural humic acids. Int. Biodeterior. Biodegradation 65, 470–478. doi: 10.1016/j.ibiod.2011.01.009
Freire, R. S., Ferreira, M. M. C., Durán, N., and Kubota, L. T. (2003). Dual amperometric biosensor device for analysis of binary mixtures of phenols by multivariate calibration using partial least squares. Anal. Chim. Acta 485, 263–269. doi: 10.1016/S0003-2670(03)00414-8
Ghigo, G., Osella, S., Maranzana, A., and Tonachini, G. (2011). The mechanism of the acid-catalyzed benzidine rearrangement of hydrazobenzene: a theoretical study. European J. Org. Chem. 2011, 2326–2333. doi: 10.1002/ejoc.201001636
Ghosh, J. P., Taylor, K. E., Bewtra, J. K., and Biswas, N. (2008). Laccase-catalyzed removal of 2,4-dimethylphenol from synthetic wastewater: effect of polyethylene glycol and dissolved oxygen. Chemosphere 71, 1709–1717. doi: 10.1016/j.chemosphere.2008.01.002
Gröbe, G., Ullrich, R., Pecyna, M., Kapturska, D., Friedrich, S., Hofrichter, M., et al. (2011). High-yield production of aromatic peroxygenase by the agaric fungus Marasmius rotula. AMB Express 1:31. doi: 10.1186/2191-0855-1-31
Guengerich, F. P. (2001). Common and uncommon cytochrome P450 reactions related to metabolism and chemical toxicity. Chem. Res. Toxicol. 14, 611–650. doi: 10.1021/tx0002583
Haemmerli, S. D., Leisola, M. S., Sanglard, D., and Fiechter, A. (1986). Oxidation of benzo(a)pyrene by extracellular ligninases of Phanerochaete chrysosporium. Veratryl alcohol and stability of ligninase. J. Biol. Chem. 261, 6900–6903.
Hammel, K. E. (1995). Mechanisms for polycyclic aromatic hydrocarbon degradation by ligninolytic fungi. Environ. Health Perspect. 103(Suppl. 5), 41–43. doi: 10.1289/ehp.95103s441
Hammel, K. E., and Cullen, D. (2008). Role of fungal peroxidases in biological ligninolysis. Curr. Opin. Plant Biol. 11, 349–355. doi: 10.1016/j.pbi.2008.02.003
Hammel, K. E., Kalyanaraman, B., and Kirk, T. K. (1986). Oxidation of polycyclic aromatic hydrocarbons and dibenzo[p]-dioxins by Phanerochaete chrysosporium ligninase. J. Biol. Chem. 261, 16948–16952.
Hammel, K. E., and Tardone, P. J. (1988). The oxidative 4-dechlorination of polychlorinated phenols is catalyzed by extracellular fungal lignin peroxidases. Biochemistry 27, 6563–6568. doi: 10.1021/bi00417a055
Hammond, G. S., and Shine, H. J. (1950). The mechanism of the benzidine rearrangement. i. the effect of acid concentration on rate. J. Am. Chem. Soc. 72, 220–221. doi: 10.1021/ja01157a062
Haritash, A. K., and Kaushik, C. P. (2009). Biodegradation aspects of Polycyclic Aromatic Hydrocarbons (PAHs): a review. J. Hazard. Mater. 169, 1–15. doi: 10.1016/j.jhazmat.2009.03.137
Harms, H., Schlosser, D., and Wick, L. Y. (2011). Untapped potential: exploiting fungi in bioremediation of hazardous chemicals. Nat Rev Micro 9, 177–192. doi: 10.1038/nrmicro2519
Hibi, M., Hatahira, S., Nakatani, M., Yokozeki, K., Shimizu, S., and Ogawa, J. (2012). Extracellular oxidases of Cerrena sp. complementarily functioning in artificial dye decolorization including laccase, manganese peroxidase, and novel versatile peroxidases. Biocatal. Agric. Biotechnol. 1, 220–225. doi: 10.1016/j.bcab.2012.03.003
Hiratsuka, N., Wariishi, H., and Tanaka, H. (2001). Degradation of diphenyl ether herbicides by the lignin-degrading basidiomycete Coriolus versicolor. Appl. Microbiol. Biotechnol. 57, 563–571. doi: 10.1007/s002530100789
Hofrichter, M. (2002). Review: lignin conversion by manganese peroxidase (MnP). Enzyme Microb. Technol. 30, 454–466. doi: 10.1016/S0141-0229(01)00528-2
Hofrichter, M., Kellner, H., Pecyna, M. J., and Ullrich, R. (2015). Fungal unspecific peroxygenases: heme-thiolate proteins that combine preoxidase and cytochrome p450 properties. Adv. Exp. Med. Biol. 851, 341–368. doi: 10.1007/978-3-319-16009-2_13
Hofrichter, M., and Ullrich, R. (2006). Heme-thiolate haloperoxidases: versatile biocatalysts with biotechnological and environmental significance. Appl. Microbiol. Biotechnol. 71, 276–288. doi: 10.1007/s00253-006-0417-3
Huijbers, M. M. E., Montersino, S., Westphal, A. H., Tischler, D., and van Berkel, W. J. H. (2014). Flavin dependent monooxygenases. Arch. Biochem. Biophys. 544, 2–17. doi: 10.1016/j.abb.2013.12.005
Hundt, K., Jonas, U., Hammer, E., and Schauer, F. (1999). Transformation of diphenyl ethers by Trametes versicolor and characterization of ring cleavage products. Biodegradation 10, 279–286. doi: 10.1023/A:1008384019897
Iba, M. M., and Thomas, P. E. (1988). Activation of 3,3′-dichlorobenzidine in rat liver microsomes to mutagens: involvement of cytochrome P-450d. Carcinogenesis 9, 717–723. doi: 10.1093/carcin/9.5.717
Imaoka, S., Yoneda, Y., Matsnda, T., Degawa, M., Fukushima, S., and Funae, Y. (1997). Mutagenic activation of urinary bladder carcinogens by CYP4B1 and the presence of CYP4B1 in bladder mucosa. Biochem. Pharmacol. 54, 677–683. doi: 10.1016/S0006-2952(97)00216-5
Jeon, J.-R., Murugesan, K., Kim, Y.-M., Kim, E.-J., and Chang, Y.-S. (2008). Synergistic effect of laccase mediators on pentachlorophenol removal by Ganoderma lucidum laccase. Appl. Microbiol. Biotechnol. 81, 783–790. doi: 10.1007/s00253-008-1753-2
Jergil, B., Lindbladh, C., Rorsman, H., and Rosengren, E. (1983). Dopa oxidation and tyrosine oxygenation by human melanoma tyrosinase. Acta Derm. Venereol. 63, 468–475.
Johannes, C., and Majcherczyk, A. (2000). Natural mediators in the oxidation of polycyclic aromatic hydrocarbons by laccase mediator systems. Appl. Environ. Microbiol. 66, 524–528. doi: 10.1128/AEM.66.2.524-528.2000
Johnson, G. R., Jain, R. K., and Spain, J. C. (2002). Origins of the 2,4-dinitrotoluene pathway. J. Bacteriol. 184, 4219–4232. doi: 10.1128/JB.184.15.4219-4232.2002
Jones, J. P., O'Hare, E. J., and Wong, L.-L. (2001). Oxidation of polychlorinated benzenes by genetically engineered CYP101 (cytochrome P450cam). Eur. J. Biochem. 268, 1460–1467. doi: 10.1046/j.1432-1327.2001.02018.x
Kadiyala, V., and Spain, J. C. (1998). A two-component monooxygenase catalyzes both the hydroxylation of p-nitrophenol and the oxidative release of nitrite from 4-nitrocatechol in Bacillus sphaericus JS905. Appl. Environ. Microbiol. 64, 2479–2484.
Karich, A., Kluge, M., Ullrich, R., and Hofrichter, M. (2013). Benzene oxygenation and oxidation by the peroxygenase of Agrocybe aegerita. AMB Express 3:5. doi: 10.1186/2191-0855-3-5
Kaschabek, S. R., Kasberg, T., Müller, D., Mars, A. E., Janssen, D. B., and Reineke, W. (1998). Degradation of chloroaromatics: purification and characterization of a novel type of chlorocatechol 2,3-dioxygenase of Pseudomonas putida GJ31. J. Bacteriol. 180, 296–302.
Kim, J. H., Stansbury, K. H., Walker, N. J., Trush, M. A., Strickland, P. T., and Sutter, T. R. (1998). Metabolism of benzo[a]pyrene and benzo[a]pyrene-7,8-diol by human cytochrome P450 1B1. Carcinogenesis 19, 1847–1853. doi: 10.1093/carcin/19.10.1847
Kinne, M. (2010). The Extracellular Peroxygenase of the Agaric Fungus Agrocybe aegerita: Catalytic Properties and Physiological Background with Particular Emphasis on Ether Cleavage. IHI Zittau.
Kinne, M., Poraj-Kobielska, M., Aranda, E., Ullrich, R., Hammel, K. E., Scheibner, K., et al. (2009a). Regioselective preparation of 5-hydroxypropranolol and 4′-hydroxydiclofenac with a fungal peroxygenase. Bioorg. Med. Chem. Lett. 19, 3085–3087. doi: 10.1016/j.bmcl.2009.04.015
Kinne, M., Poraj-Kobielska, M., Ralph, S. A., Ullrich, R., Hofrichter, M., and Hammel, K. E. (2009b). Oxidative cleavage of diverse ethers by an extracellular fungal peroxygenase. J. Biol. Chem. 284, 29343–29349. doi: 10.1074/jbc.M109.040857
Kinne, M., Zeisig, C., Ullrich, R., Kayser, G., Hammel, K. E., and Hofrichter, M. (2010). Stepwise oxygenations of toluene and 4-nitrotoluene by a fungal peroxygenase. Biochem. Biophys. Res. Commun. 397, 18–21. doi: 10.1016/j.bbrc.2010.05.036
Kirk, T. K., and Farrell, R. L. (1987). Enzymatic “Combustion”: the microbial degradation of lignin. Annu. Rev. Microbiol. 41, 465–501. doi: 10.1146/annurev.mi.41.100187.002341
Klibanov, A. M., Alberti, B. N., Morris, E. D., and Felshin, L. M. (1980). Enzymatic removal of toxic phenols and anilines from waste waters. J. Appl. Biochem. 2:5, 414–421.
Kluge, M., Ullrich, R., Dolge, C., Scheibner, K., and Hofrichter, M. (2009). Hydroxylation of naphthalene by aromatic peroxygenase from Agrocybe aegerita proceeds via oxygen transfer from and intermediary epoxidation. Appl. Microbiol. Biotechnol. 81, 1071–1076. doi: 10.1007/s00253-008-1704-y
Kluge, M., Ullrich, R., Scheibner, K., and Hofrichter, M. (2007). Spectrophotometric assay for detection of aromatic hydroxylation catalyzed by fungal haloperoxidase–peroxygenase. Appl. Microbiol. Biotechnol. 75, 1473–1478. doi: 10.1007/s00253-007-0942-8
Kluge, M., Ullrich, R., Scheibner, K., and Hofrichter, M. (2012). Stereoselective benzylic hydroxylation of alkylbenzenes and epoxidation of styrene derivatives catalyzed by the peroxygenase of Agrocybe aegerita. Green Chem. 14, 440–446. doi: 10.1039/C1GC16173C
Kordon, K., Mikolasch, A., and Schauer, F. (2010). Oxidative dehalogenation of chlorinated hydroxybiphenyls by laccases of white-rot fungi. Int. Biodeterior. Biodegradation 64, 203–209. doi: 10.1016/j.ibiod.2009.10.010
Krimen, L. I., and Cota, D. J. (2004). “The Ritter Reaction,” in Organic Reactions. ed S. E. Denmark (Chichester: John Wiley & Sons, Inc.) doi: 10.1002/0471264180.or017.03
Lakshmi, V. M., Zenser, N. T., Hsu, F. F., Mattammal, M. B., Zenser, T. V., and Davis, B. B. (1996). NADPH-dependent oxidation of benzidine by rat liver. Carcinogenesis 17, 1941–1947. doi: 10.1093/carcin/17.9.1941
Lamb, D. C., Lei, L., Warrilow, A. G. S., Lepesheva, G. I., Mullins, J. G. L., Waterman, M. R., et al. (2009). The first virally encoded cytochrome P450. J. Virol. 83, 8266–8269. doi: 10.1128/JVI.00289-09
Leahy, J. G., Batchelor, P. J., and Morcomb, S. M. (2003). Evolution of the soluble diiron monooxygenases. FEMS Microbiol. Rev. 27, 449–479. doi: 10.1016/S0168-6445(03)00023-8
Leontievsky, A., Myasoedova, N., Baskunov, B., Golovleva, L., Bucke, C., and Evans, C. (2001). Transformation of 2,4,6-trichlorophenol by free and immobilized fungal laccase. Appl. Microbiol. Biotechnol. 57, 85–91. doi: 10.1007/s002530100756
Lepesheva, G. I., and Waterman, M. R. (2004). CYP51—the omnipotent P450. Mol. Cell. Endocrinol. 215, 165–170. doi: 10.1016/j.mce.2003.11.016
Lessner, D. J., Johnson, G. R., Parales, R. E., Spain, J. C., and Gibson, D. T. (2002). Molecular characterization and substrate specificity of nitrobenzene dioxygenase from comamonas sp. strain JS765. Appl. Environ. Microbiol. 68, 634–641. doi: 10.1128/AEM.68.2.634-641.2002
Levine, W. G. (1991). Metabolism of AZO dyes: implication for detoxication and activation. Drug Metab. Rev. 23, 253–309. doi: 10.3109/03602539109029761
Liers, C., Aranda, E., Strittmatter, E., Piontek, K., Plattner, D. A., Zorn, H., et al. (2014). Phenol oxidation by DyP-type peroxidases in comparison to fungal and plant peroxidases. J. Mol. Catal. B Enzymatic 103, 41–46. doi: 10.1016/j.molcatb.2013.09.025
Lowry, L. K., Tolos, W. P., Boeniger, M. F., Nony, C. R., and Bowman, M. C. (1980). Chemical monitoring of urine from workers potentially exposed to benzidine-derived azo dyes. Toxicol. Lett. 7, 29–36. doi: 10.1016/0378-4274(80)90081-8
Mackay, D., and Shiu, W. Y. (1977). Aqueous solubility of polynuclear aromatic hydrocarbons. J. Chem. Eng. Data 22, 399–402. doi: 10.1021/je60075a012
Majcherczyk, A., Johannes, C., and Hüttermann, A. (1998). Oxidation of polycyclic aromatic hydrocarbons (PAH) by laccase of Trametes versicolor. Enzyme Microb. Technol. 22, 335–341. doi: 10.1016/S0141-0229(97)00199-3
Marco-Urrea, E., Pérez-Trujillo, M., Caminal, G., and Vicent, T. (2009). Dechlorination of 1,2,3- and 1,2,4-trichlorobenzene by the white-rot fungus Trametes versicolor. J. Hazard. Mater. 166, 1141–1147. doi: 10.1016/j.jhazmat.2008.12.076
Mars, A. E., Kasberg, T., Kaschabek, S. R., van Agteren, M. H., Janssen, D. B., and Reineke, W. (1997). Microbial degradation of chloroaromatics: use of the meta-cleavage pathway for mineralization of chlorobenzene. J. Bacteriol. 179, 4530–4537. doi: 10.1128/jb.179.14.4530-4537.1997
Martínez, Á. T., Ruiz-Dueñas, F. J., Martínez, M. J., del Río, J. C., and Gutiérrez, A. (2009). Enzymatic delignification of plant cell wall: from nature to mill. Curr. Opin. Biotechnol. 20, 348–357. doi: 10.1016/j.copbio.2009.05.002
McDaniel, D. H., and Brown, H. C. (1958). An extended table of hammett substitutent constants based on the ionization of substituted benzoic acids. J. Org. Chem. 23, 420–427. doi: 10.1021/jo01097a026
Meunier, B., de Visser, S. P., and Shaik, S. (2004). Mechanism of oxidation reactions catalyzed by cytochrome P450 enzymes. Chem. Rev. 104, 3947–3980. doi: 10.1021/cr020443g
Moiseeva, O. V., Solyanikova, I. P., Kaschabek, S. R., Gröning, J., Thiel, M., Golovleva, L. A., et al. (2002). A new modified ortho cleavage pathway of 3-chlorocatechol degradation by rhodococcus opacus 1CP: genetic and biochemical evidence. J. Bacteriol. 184, 5282–5292. doi: 10.1128/JB.184.19.5282-5292.2002
Monferran, M. V., Wunderlin, D. A., Nimptsch, J., and Pflugmacher, S. (2007). Biotransformation and antioxidant response in Ceratophyllum demersum experimentally exposed to 1,2- and 1,4-dichlorobenzene. Chemosphere 68, 2073–2079. doi: 10.1016/j.chemosphere.2007.02.016
Montiel, A. M., Fernández, F. J., Marcial, J., Soriano, J., Barrios-González, J., and Tomasini, A. (2004). A fungal phenoloxidase (tyrosinase) involved in pentachlorophenol degradation. Biotechnol. Lett. 26, 1353–1357. doi: 10.1023/B:BILE.0000045632.36401.86
Mori, T., Kitano, S., and Kondo, R. (2003). Biodegradation of chloronaphthalenes and polycyclic aromatic hydrocarbons by the white-rot fungus Phlebia lindtneri. Appl. Microbiol. Biotechnol. 61, 380–383. doi: 10.1007/s00253-003-1253-3
Munro, A. W., Girvan, H. M., Mason, A. E., Dunford, A. J., and McLean, K. J. (2012). What makes a P450 tick? Trends Biochem. Sci. 38, 140–150. doi: 10.1016/j.tibs.2012.11.006
Nedelcheva, V., Gut, I., Souček, P., and Frantık, E. (1998). Cytochrome P450 catalyzed oxidation of monochlorobenzene, 1,2- and 1,4-dichlorobenzene in rat, mouse, and human liver microsomes. Chem. Biol. Interact. 115, 53–70. doi: 10.1016/S0009-2797(98)00058-1
Niki, E. (1991). Action of ascorbic acid as a scavenger of active and stable oxygen radicals. Am. J. Clin. Nutr. 54, 1119S–1124S.
Nishino, S. F., Paoli, G. C., and Spain, J. C. (2000). Aerobic degradation of dinitrotoluenes and pathway for bacterial degradation of 2,6-dinitrotoluene. Appl. Environ. Microbiol. 66, 2139–2147. doi: 10.1128/AEM.66.5.2139-2147.2000
Notomista, E., Lahm, A., Di Donato, A., and Tramontano, A. (2003). Evolution of bacterial and archaeal multicomponent monooxygenases. J. Mol. Evol. 56, 435–445. doi: 10.1007/s00239-002-2414-1
Osborne, R. L., Coggins, M. K., Raner, G. M., Walla, M., and Dawson, J. H. (2009). The mechanism of oxidative halophenol dehalogenation by amphitrite ornata dehaloperoxidase is initiated by H2O2 binding and involves two consecutive one-electron steps: role of ferryl intermediates. Biochemistry 48, 4231–4238. doi: 10.1021/bi900367e
Osborne, R. L., Coggins, M. K., Terner, J., and Dawson, J. H. (2007). Caldariomyces fumago chloroperoxidase catalyzes the oxidative dehalogenation of chlorophenols by a mechanism involving two one-electron steps. J. Am. Chem. Soc. 129, 14838–14839. doi: 10.1021/ja0746969
Pandya, C., Farelli, J. D., Dunaway-Mariano, D., and Allen, K. N. (2014). Enzyme promiscuity: engine of evolutionary innovation. J. Biol. Chem. 289, 30229–30236. doi: 10.1074/jbc.R114.572990
Pecyna, M. (2016). Molekularbiologische Charakterisierung von Häm-thiolat- und DYP-type-Peroxidasen ausgewählter Basidiomyceten. PhD PhD, TU-Dresden.
Peter, S., Kinne, M., Wang, X., Ullrich, R., Kayser, G., Groves, J. T., et al. (2011). Selective hydroxylation of alkanes by an extracellular fungal peroxygenase. FEBS J. 278, 3667–3675. doi: 10.1111/j.1742-4658.2011.08285.x
Phillips, L. E., and Leonard, T. J. (1976). Benzidine as a substrate for measuring phenoloxidase activity in crude cell-free extracts of Schizophyllum commune. Mycologia 68, 277–285. doi: 10.2307/3758999
Pinyakong, O., Habe, H., Kouzuma, A., Nojiri, H., Yamane, H., and Omori, T. (2004). Isolation and characterization of genes encoding polycyclic aromatic hydrocarbon dioxygenase from acenaphthene and acenaphthylene degrading Sphingomonas sp. strain A4. FEMS Microbiol. Lett. 238, 297–305. doi: 10.1111/j.1574-6968.2004.tb09770.x
Piontek, K., Smith, A. T., and Blodig, W. (2001). Lignin peroxidase structure and function. Biochem. Soc. Trans. 29, 111–116. doi: 10.1042/bst0290111
Piontek, K., Strittmatter, E., Dimitrova, E., Zámocký, M., Kiebist, J., Ullrich, R., et al. (2017). Extensive substrate palette – exclusive products: crystal structure of a dimeric peroxygenase from Marasmius rotula. J Biol Chem.
Pointing, S. (2001). Feasibility of bioremediation by white-rot fungi. Appl. Microbiol. Biotechnol. 57, 20–33. doi: 10.1007/s002530100745
Poraj-Kobielska, M. (2013). Conversion of Pharmaceuticals and Other Drugs by Fungal Peroxygenases: Umsetzung von Pharmazeutika und Psychoaktiven Substanzen mit Pilzlichen Peroxygenasen. Dissertation, Technical University Dresden. Available online at: http://www.qucosa.de/recherche/frontdoor/?tx_slubopus4frontend[id]=11333
Poraj-Kobielska, M., Kinne, M., Ullrich, R., Scheibner, K., Kayser, G., Hammel, K. E., et al. (2011). Preparation of human drug metabolites using fungal peroxygenases. Biochem. Pharmacol. 82, 789–796. doi: 10.1016/j.bcp.2011.06.020
Qayyum, H., Maroof, H., and Yasha, K. (2009). Remediation and treatment of organopollutants mediated by peroxidases: a review. Crit. Rev. Biotechnol. 29, 94–119. doi: 10.1080/07388550802685306
Reddy, G. V. B., and Gold, M. H. (2000). Degradation of pentachlorophenol by Phanerochaete chrysosporium: intermediates and reactions involved. Microbiology 146, 405–413. doi: 10.1099/00221287-146-2-405
Reddy, G. V. B., Sollewijn Gelpke, M. D., and Gold, M. H. (1998). Degradation of 2,4,6-trichlorophenol by Phanerochaete chrysosporium: involvement of reductive dechlorination. J. Bacteriol. 180, 5159–5164.
Riggin, R. M., and Howard, C. C. (1979). Determination of benzidine, dichlorobenzidine, and diphenylhydrazine in aqueous media by high performance liquid chromatography. Anal. Chem. 51, 210–214. doi: 10.1021/ac50038a014
Sack, U., Hofrichter, M., and Fritsche, W. (1997). Degradation of polycyclic aromatic hydrocarbons by manganese peroxidase of Nematoloma frowardii. FEMS Microbiol. Lett. 152, 227–234. doi: 10.1111/j.1574-6968.1997.tb10432.x
Sazinsky, M. H., Bard, J., Di Donato, A., and Lippard, S. J. (2004). Crystal structure of the toluene/o-xylene monooxygenase hydroxylase from Pseudomonas stutzeri OX1: insight into the substrate specificity, substrate channeling, and active site tuning of multicomponent monooxygenases. J. Biol. Chem. 279, 30600–30610. doi: 10.1074/jbc.M400710200
Schomburg, D., and Stephan, D. (1994). “2,4-Dichlorophenol 6-monooxygenase,” in Enzyme Handbook, eds D. Schomburg and D. Stephan (Berlin; Heidelberg: Springer), 481–485.
Shimada, T., Martin, M. V., Pruess-Schwartz, D., Marnett, L. J., and Guengerich, F. P. (1989). Roles of individual human cytochrome P-450 enzymes in the bioactivation of benzo(a)pyrene, 7,8-dihydroxy-7,8-dihydrobenzo(a)pyrene, and other dihydrodiol derivatives of polycyclic aromatic hydrocarbons. Cancer Res. 49, 6304–6312.
Shimada, T., Takenaka, S., Murayama, N., Yamazaki, H., Kim, J.-H., Kim, D., et al. (2015). Oxidation of acenaphthene and acenaphthylene by human cytochrome P450 enzymes. Chem. Res. Toxicol. 28, 268–278. doi: 10.1021/tx500505y
Simonsick, W. J. Jr., and Hites, R. A. (1984). Analysis of isomeric polycyclic aromatic hydrocarbons by charge-exchange chemical ionization mass spectrometry. Anal. Chem. 56, 2749–2754. doi: 10.1021/ac00278a028
Spain, J. C. (1995). Biodegradation of nitroaromatic compounds. Annu. Rev. Microbiol. 49, 523–555. doi: 10.1146/annurev.mi.49.100195.002515
Spiess, E., Sommer, C., and Görisch, H. (1995). Degradation of 1,4-dichlorobenzene by Xanthobacter flavus 14p1. Appl. Environ. Microbiol. 61, 3884–3888.
Staples, C. A., Peterson, D. R., Parkerton, T. F., and Adams, W. J. (1997). The environmental fate of phthalate esters: a literature review. Chemosphere 35, 667–749. doi: 10.1016/S0045-6535(97)00195-1
Steffen, K. T., Hatakka, A., and Hofrichter, M. (2003). Degradation of benzo[a]pyrene by the litter-decomposing basidiomycete stropharia coronilla: role of manganese peroxidase. Appl. Environ. Microbiol. 69, 3957–3964. doi: 10.1128/AEM.69.7.3957-3964.2003
Strittmatter, E., Plattner, D. A., and Piontek, K. (2011). “Dye-Decolorizing Peroxidase (DyP),” in Encyclopedia of Inorganic and Bioinorganic Chemistry, ed R. A. Scott (Chichester: John Wiley & Sons, Ltd). doi: 10.1002/9781119951438.eibc2276
Tegge, G. (1983). “Biotechnology, A comprehensive treatise in 8 volumes,” in Biomass, Microorganisms for Special Applications, Microbial Products I, Energy from Renewable Resources. Vol. 3, eds H. J. Rehm, G. Reed and H. Dellweg (Weinheim: Verlag Chemie; Deerfield Beach–Basel Starch-Stärke), 367.
Thakur, I. S., Verma, P., and Upadhayaya, K. (2002). Molecular cloning and characterization of pentachlorophenol-degrading monooxygenase genes of Pseudomonas sp. from the chemostat. Biochem. Biophys. Res. Commun. 290, 770–774. doi: 10.1006/bbrc.2001.6239
Ullrich, R., Dolge, C., Kluge, M., and Hofrichter, M. (2008). Pyridine as novel substrate for regioselective oxygenation with aromatic peroxygenase from Agrocybe aegerita. FEBS Lett. 582, 4100–4106. doi: 10.1016/j.febslet.2008.11.006
Ullrich, R., and Hofrichter, M. (2005). The haloperoxidase of the agaric fungus Agrocybe aegerita hydroxylates toluene and naphthalene. FEBS Lett. 579, 6247–6250. doi: 10.1016/j.febslet.2005.10.014
Ullrich, R., and Hofrichter, M. (2007). Enzymatic hydroxylation of aromatic compounds. Cell. Mol. Life Sci. 64, 271–293. doi: 10.1007/s00018-007-6362-1
Ullrich, R., Nüske, J., Scheibner, K., Spantzel, J., and Hofrichter, M. (2004). Novel haloperoxidase from the agaric basidiomycete Agrocybe aegerita oxidizes aryl alcohols and aldehydes. Appl. Environ. Microbiol. 70, 4575–4581. doi: 10.1128/AEM.70.8.4575-4581.2004
USEPA (1979). Priority Pollutant List [Online]. Washington, DC: United States Environmental Protection Agency. Available online at: https://www.epa.gov/sites/production/files/2015-09/documents/priority-pollutant-list-epa.pdf (Accessed 07.13. 2016).
Valli, K., and Gold, M. H. (1991). Degradation of 2,4-dichlorophenol by the lignin-degrading fungus Phanerochaete chrysosporium. J. Bacteriol. 173, 345–352. doi: 10.1128/jb.173.1.345-352.1991
van Berkel, W. J. H., Kamerbeek, N. M., and Fraaije, M. W. (2006). Flavoprotein monooxygenases, a diverse class of oxidative biocatalysts. J. Biotechnol. 124, 670–689. doi: 10.1016/j.jbiotec.2006.03.044
van der Meer, J. R., van Neerven, A. R., de Vries, E. J., de Vos, W. M., and Zehnder, A. J. (1991). Cloning and characterization of plasmid-encoded genes for the degradation of 1,2-dichloro-, 1,4-dichloro-, and 1,2,4-trichlorobenzene of Pseudomonas sp. strain P51. J. Bacteriol. 173, 6–15. doi: 10.1128/jb.173.1.6-15.1991
van Gorcom, R. F., van den Hondel, C. A., and Punt, P. J. (1998). Cytochrome P450 enzyme systems in fungi. Fungal Genet. Biol. 23, 1–17. doi: 10.1006/fgbi.1997.1021
Vardar, G., and Wood, T. K. (2005). Alpha-subunit positions methionine 180 and glutamate 214 of Pseudomonas stutzeri OX1 toluene-o-xylene monooxygenase influence catalysis. J. Bacteriol. 187, 1511–1514. doi: 10.1128/JB.187.4.1511-1514.2005
Vazquez-Duhalt, R., Westlake, D. W. S., and Fedorak, P. M. (1994). Lignin peroxidase oxidation of aromatic compounds in systems containing organic solvents. Appl. Environ. Microbiol. 60, 459–466.
Vlasits, J., Jakopitsch, C., Bernroitner, M., Zamocky, M., Furtmüller, P. G., and Obinger, C. (2010). Mechanisms of catalase activity of heme peroxidases. Arch. Biochem. Biophys. 500, 74–81. doi: 10.1016/j.abb.2010.04.018
Wada, S., Ichikawa, H., and Tastsumi, K. (1995). Removal of phenols and aromatic amines from wastewater by a combination treatment with tyrosinase and a coagulant. Biotechnol. Bioeng. 45, 304–309. doi: 10.1002/bit.260450404
Walker, R. (1970). The metabolism of azo compounds: a review of the literature. Food Cosmet. Toxicol. 8, 659–676. doi: 10.1016/S0015-6264(70)80455-2
Wang, J., Liu, P., and Qian, Y. (1995). Microbial degradation of di-n-butyl phthalate. Chemosphere 31, 4051–4056. doi: 10.1016/0045-6535(95)00282-D
Wang, Y., Fan, Y., and Gu, J.-D. (2003). Aerobic degradation of phthalic acid by Comamonas acidovoran Fy-1 and dimethyl phthalate ester by two reconstituted consortia from sewage sludge at high concentrations. World J. Microbiol. Biotechnol. 19, 811–815. doi: 10.1023/A:1026021424385
Warshawsky, D., Radike, M., Jayasimhulu, K., and Cody, T. (1988). Metabolism of benzo(A)pyrene by a dioxygenase enzyme system of the freshwater green alga Selenastrum capricornutum. Biochem. Biophys. Res. Commun. 152, 540–544. doi: 10.1016/S0006-291X(88)80071-8
Whited, G. M., and Gibson, D. T. (1991). Toluene-4-monooxygenase, a three-component enzyme system that catalyzes the oxidation of toluene to p-cresol in Pseudomonas mendocina KR1. J. Bacteriol. 173, 3010–3016. doi: 10.1128/jb.173.9.3010-3016.1991
Wieser, M., Wagner, B., Eberspächer, J., and Lingens, F. (1997). Purification and characterization of 2,4,6-trichlorophenol-4-monooxygenase, a dehalogenating enzyme from Azotobacter sp. strain GP1. J. Bacteriol. 179, 202–208. doi: 10.1128/jb.179.1.202-208.1997
Wittassek, M., and Angerer, J. (2008). Phthalates: metabolism and exposure. Int. J. Androl. 31, 131–138. doi: 10.1111/j.1365-2605.2007.00837.x
Wood, A. W., Levin, W., Lu, A. Y. H., Ryan, D., West, S. B., Lehr, R. E., et al. (1976). Mutagenicity of metabolically activated benzo[a]anthracene 3,4-dihydrodiol: evidence for bay region activation of carcinogenic polycyclic hydrocarbons. Biochem. Biophys. Res. Commun. 72, 680–686. doi: 10.1016/S0006-291X(76)80093-9
Wu, Y., Teng, Y., Li, Z., Liao, X., and Luo, Y. (2008). Potential role of polycyclic aromatic hydrocarbons (PAHs) oxidation by fungal laccase in the remediation of an aged contaminated soil. Soil Biol. Biochem. 40, 789–796. doi: 10.1016/j.soilbio.2007.10.013
Xiao, Y., Zhang, J.-J., Liu, H., and Zhou, N.-Y. (2007). Molecular characterization of a novel ortho-nitrophenol catabolic gene cluster in Alcaligenes sp. strain NyZ215. J. Bacteriol. 189, 6587–6593. doi: 10.1128/JB.00654-07
Xu, F., and Bhandari, A. (2003). Retention and extractability of phenol, cresol, and dichlorophenol exposed to two surface soils in the presence of horseradish peroxidase enzyme. J. Agric. Food Chem. 51, 183–188. doi: 10.1021/jf025852s
Yamazoe, Y., Zenser, T. V., Miller, D. W., and Kadlubar, F. F. (1988). Mechanism of formation and structural characterization of DNA adducts derived from peroxidative activation of benzidine. Carcinogenesis 9, 1635–1641. doi: 10.1093/carcin/9.9.1635
Yan, Z., Zhong, H. M., Maher, N., Torres, R., Leo, G. C., Caldwell, G. W., et al. (2005). Bioactivation of 4-methylphenol (p-cresol) via cytochrome p450-mediated aromatic oxidation in human liver microsomes. Drug Metabol. Disposition 33, 1867–1876. doi: 10.1124/dmd.105.006387
Ye, J., Singh, A., and Ward, O. (2004). Biodegradation of nitroaromatics and other nitrogen-containing xenobiotics. World J. Microbiol. Biotechnol. 20, 117–135. doi: 10.1023/B:WIBI.0000021720.03712.12
Yee, D. C., and Wood, T. K. (1997). 2,4-Dichlorophenol degradation using Streptomyces viridosporus T7A lignin peroxidase. Biotechnol. Prog. 13, 53–59. doi: 10.1021/bp960091x
Yosca, T. H., Ledray, A. P., Ngo, J., and Green, M. T. (2017). A new look at the role of thiolate ligation in cytochrome P450. J. Biol. Inorganic Chem. 22, 209–220. doi: 10.1007/s00775-016-1430-3
Keywords: EC 1.11.2.1, peroxidase, xenobiotics, chlorobenzene, nitroaromatics, polycyclic aromatic hydrocarbons, fungi
Citation: Karich A, Ullrich R, Scheibner K and Hofrichter M (2017) Fungal Unspecific Peroxygenases Oxidize the Majority of Organic EPA Priority Pollutants. Front. Microbiol. 8:1463. doi: 10.3389/fmicb.2017.01463
Received: 16 June 2017; Accepted: 20 July 2017;
Published: 09 August 2017.
Edited by:
Fabrice Martin-Laurent, Institut National de la Recherche Agronomique (INRA), FranceReviewed by:
Lígia O. Martins, Universidade Nova de Lisboa, PortugalYonghong Bi, Institute of Hydrobiology (CAS), China
Copyright © 2017 Karich, Ullrich, Scheibner and Hofrichter. This is an open-access article distributed under the terms of the Creative Commons Attribution License (CC BY). The use, distribution or reproduction in other forums is permitted, provided the original author(s) or licensor are credited and that the original publication in this journal is cited, in accordance with accepted academic practice. No use, distribution or reproduction is permitted which does not comply with these terms.
*Correspondence: Alexander Karich, YWxleGFuZGVyLmthcmljaEB0dS1kcmVzZGVuLmRl