- 1Department of Biology, University of Alabama at Birmingham, Birmingham, AL, United States
- 2Gateway Antarctica, University of Canterbury, Christchurch, New Zealand
- 3National Institute of Polar Research, Tachikawa, Japan
- 4Carl Sagan Center, SETI Institute, Mountain View, CA, United States
In this study, we report the distribution of microbial taxa and their predicted metabolic functions observed in the top (U1), middle (U2), and inner (U3) decadal growth laminae of a unique large conical microbial mat from perennially ice-covered Lake Untersee of East Antarctica, using NextGen sequencing of the 16S rRNA gene and bioinformatics tools. The results showed that the U1 lamina was dominated by cyanobacteria, specifically Phormidium sp., Leptolyngbya sp., and Pseudanabaena sp. The U2 and U3 laminae had high abundances of Actinobacteria, Verrucomicrobia, Proteobacteria, and Bacteroidetes. Closely related taxa within each abundant bacterial taxon found in each lamina were further differentiated at the highest taxonomic resolution using the oligotyping method. PICRUSt analysis, which determines predicted KEGG functional categories from the gene contents and abundances among microbial communities, revealed a high number of sequences belonging to carbon fixation, energy metabolism, cyanophycin, chlorophyll, and photosynthesis proteins in the U1 lamina. The functional predictions of the microbial communities in U2 and U3 represented signal transduction, membrane transport, zinc transport and amino acid-, carbohydrate-, and arsenic- metabolisms. The Nearest Sequenced Taxon Index (NSTI) values processed through PICRUSt were 0.10, 0.13, and 0.11 for U1, U2, and U3 laminae, respectively. These values indicated a close correspondence with the reference microbial genome database, implying high confidence in the predicted metabolic functions of the microbial communities in each lamina. The distribution of microbial taxa observed in each lamina and their predicted metabolic functions provides additional insight into the complex microbial ecosystem at Lake Untersee, and lays the foundation for studies that will enhance our understanding of the mechanisms responsible for the formation of these unique mat structures and their evolutionary significance.
Introduction
Microbial mats are often considered to be modern day analogs of ancient organo-sedimentary structures such as microbialites and stromatolites, and the geological record indicates their origin as early as ∼3.5 Ga BP (Gerdes, 2010; Seckbach and Oren, 2010). Morphological diversity and longevity of stromatolitic formations from the Archaean and throughout the Proterozoic indicate its success as a growth strategy, and comparisons with extant examples of microbial mats can explain the possible reasons behind this success (Grotzinger and Knoll, 1999; Hofmann et al., 1999). Modern microbial mats often represent metabolically layered structures, capable of oxygenic photosynthesis at the top of a mat where light is most abundant (Foster and Green, 2011). In the deeper segments in the mats, reduced light and depleted oxygen enables the growth of organisms capable of a cascade of anaerobic metabolisms (Kühl and Fenchel, 2000). Such layering is often visible as color changes, with an orange surface from abundant light-protective carotenoid pigments, transitioning to green light-harvesting phycobilin and chlorophyll pigments and pink layers corresponding to photosynthetic sulfur bacteria in anoxic zones. There are many examples of this spatial organization of microbial communities with defined metabolic roles in the literature, and such close proximity of interacting metabolic structures allows for diffusion to be an effective coupling mechanism that provides key advantages to this mat structure (Seckbach and Oren, 2010). Viewed as an integrated unit, the microbial mat shows metabolic coupling, facilitated by this one-dimensional, organized structure, that allows a more efficient utilization of resources than any one organism could do alone (Stahl, 1995; Paerl and Pinckney, 1996; Franks and Stolz, 2009). Indeed, some argue that the organized complexity and physiological cooperativity of a microbial mat is analogous to that of eukaryotic tissues (Webb et al., 2003; Guerrero and Berlanga, 2010).
These laminated microbial mats are often best developed under extreme conditions such as thermal springs, sulfur springs, high salinity and icy polar environments where conditions preclude large metazoan bioturbators that would otherwise disrupt mat formation (Seckbach and Oren, 2010). Microbial mats in ice-covered Antarctic lakes are thought to be particularly well developed as, in addition to the exclusion of higher eukaryotes through extreme conditions and isolation, they are also protected from physical perturbation – wind and wave forcing – by thick, perennial ice cover (Priscu and Foreman, 2009; Cavicchioli, 2015). To date, most of our understanding of microbial mats in the Antarctic continent has come from perennially ice-covered lakes in the McMurdo Dry Valleys (Priscu and Foreman, 2009; Jungblut et al., 2016). In particular, benthic photosynthetic cyanobacterial mats cohabited by heterotrophic bacterial communities have been implicated as the key biological components in maintaining the lake’s productivity and nutrient cycling (Laybourn-Parry and Pearce, 2007; Quesada et al., 2008). However, in addition to the McMurdo Dry Valleys, a wide range of freshwater lakes have been described in the East Antarctic ice plateau, ice-free oases and foothills of the Transantarctic Mountains. Among these, Lake Untersee (71.34°S, 13.45°E) is the largest of the perennially ice-covered lakes in the interior of central Queen Maud Land (Loopmann et al., 1988; Wand et al., 1997, 2006). The lake presents an exclusively microbial ecosystem and consists of unique conical microbial mats that appear as smooth cones, rising to ∼0.5 m above the lake bottom, unlike anything reported thus far from other Antarctic or temperate lakes (Andersen et al., 2011).
Microbial community structures in ice-covered Antarctic lakes have been studied using a range of culture-dependent and culture-independent molecular techniques (Laybourn-Parry and Pearce, 2007; Laybourn-Parry and Wadham, 2014). For instance, microbial community compositions have been investigated in (1) ice core samples from Lake Vida by clone library, and denaturing gradient gel electrophoresis (DGGE) culture-independent approaches targeting the 16S rRNA gene (Mosier et al., 2007); (2) water column samples from Lake Hoare by culturing chemoorganotrophic isolates on media (Clocksin et al., 2007) and molecular probe of SYBR gold (Thurman et al., 2012); (3) water samples from Lake Bonney by restriction fragment length polymorphism (RFLP) analysis (Glatz et al., 2006); and (4) benthic microbial mat samples from Lake Joyce by microscopic analysis (Hawes et al., 2011). Besides microbial mats in marine and other tropical ecosystems (Mobberley et al., 2012; Louyakis et al., 2017), NextGen sequencing technology has been applied to other ecosystems in Antarctica including lakes, sediments, soils, endoliths, and hypoliths. Examples include: (1) benthic mats from Lake Fryxell using Illumina Miseq platform (Jungblut et al., 2016), (2) water samples from Ace Lake using pyrosequencing (Lauro et al., 2011), (3) water samples from Lake Fryxell and Bonney using pyrosequencing (Vick-Majors et al., 2014), (4) soils, endoliths and hypoliths of Victoria Valley in Eastern Antarctica using pyrosequencing (Van Goethem et al., 2016), (5) surface sediments of King George Island and Bransfield Strait of Northwestern Antarctic Peninsula by pyrosequencing (Franco et al., 2017), (6) sediments of freshwater inland lakes and estuarine environment of Byers Peninsula in Antarctica (Gugliandolo et al., 2016), and (7) ice and freshwater water samples from lakes in Schirmacher Oasis of East Antarctica (Mojib et al., 2009).
In this study, we report the taxonomic composition of the microbial community and their predicted metabolic functions of the top (U1), middle (U2), and inner (U3) laminae of a large conical mat from Lake Untersee. We have used NextGen sequencing technology and bioinformatics tools on the community DNA targeting the 16S rRNA gene. The objective of this study was to describe microbial taxa in each of the top three laminae and use a taxonomy-based bioinformatics tool with the appropriate validation method to predict the likely metabolic processes performed by distinct and concentrically distributed microbial communities. We believe the results from this study will help understand the mechanisms for the formation of these unique laminated conical mats in the ecosystem of Lake Untersee.
Materials and Methods
Description of Lake Untersee and Sample Collection
Located in the Gruber Mountains, Lake Untersee is 563 m above sea level, 6.5 km long and 2.5 km wide, with an area of 11.4 km2 and dammed at its North end by the terminus of the Anuchin Glacier. The lake floor is mostly covered with photosynthetic benthic microbial mats with a conspicuous distribution of vertically rising pigmented large conical mats. Microbial pigments are concentrated near the tops of cones, which appear purple/pink color due to phycoerythrin (Andersen et al., 2011). These mats are soft, composed of organic-rich alternating clear and mineral-rich opaque ∼0.5 mm-thick laminations with a high inheritance of microbes, showing no evidence of lithification (Andersen et al., 2011).
A single core of a conical mat from Lake Untersee (Figures 1A,B) was collected by scientific divers utilizing SCUBA by accessing the lake ecosystem through a hole made on the ∼3.5 m surface ice using methods described by Andersen (2007). The core was collected by gently inserting a 50 mm diameter sterile polycarbonate core tube through the top of a conical mat structure, then sealing it with rubber stoppers and returning it to the surface. The core was stored in Antarctic Logistics Centre International (ALCI), Cape Town, South Africa facility first at -20°C in a walk-in freezer and then transported to UAB in dry ice and kept in -20°C freezer until used. The top three laminae (herein U1, top lamina; U2, middle lamina; and U3, inner lamina) were separated from the core and selected individually (Figures 1C,D) for sequencing. Each lamina was carefully separated using sterile forceps and rinsed with distilled water to avoid carryover of microorganisms between laminae before DNA extraction.
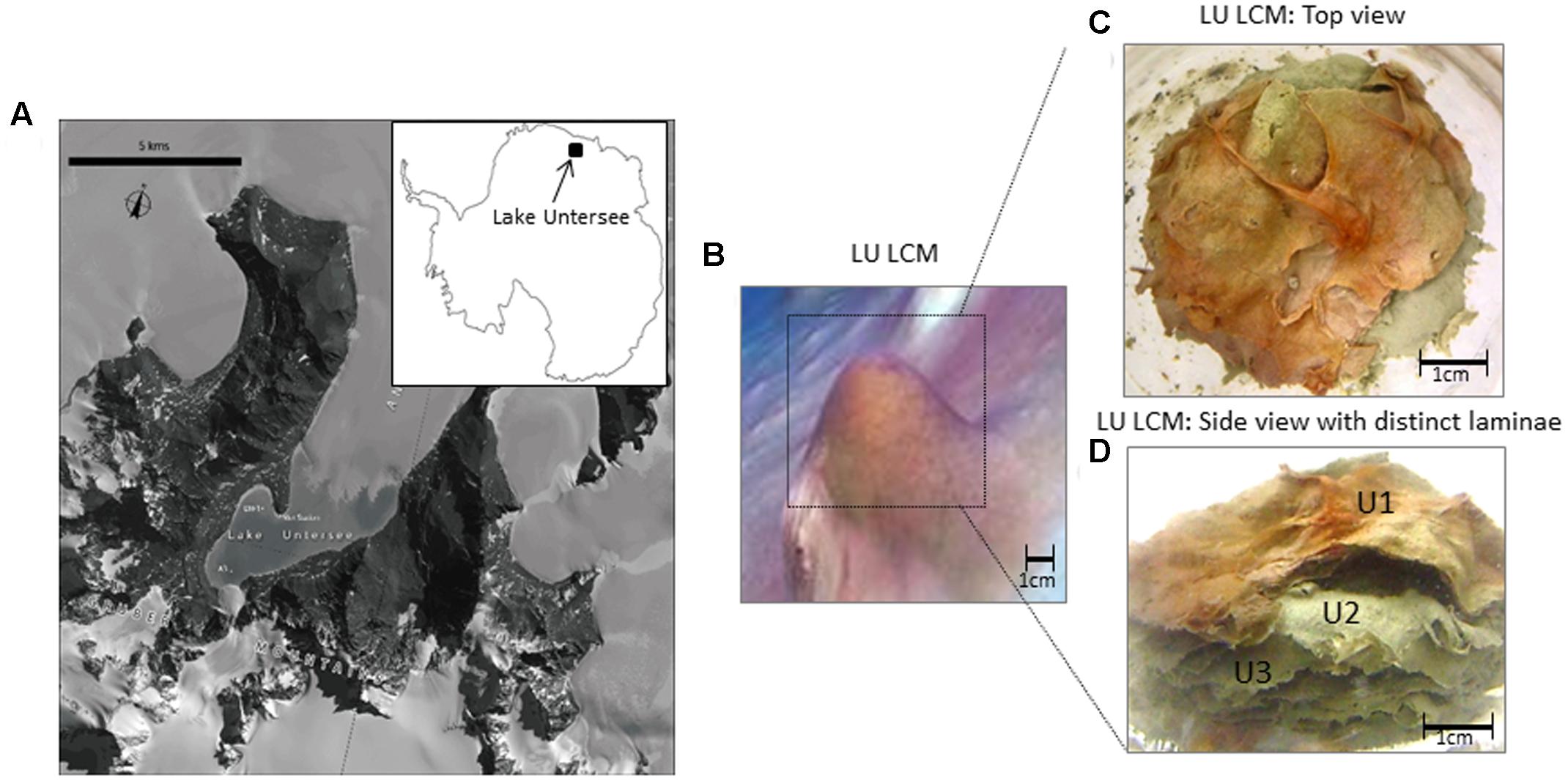
FIGURE 1. Geographical location, structure and laminae of a large conical mat of Lake Untersee, Antarctica. (A) Geographical location and satellite image map of Lake Untersee (71.34° S 13.27° E) Antarctica. (Satellite imagery copyright DigitalGlobe, Inc. Provided by NGA Commercial Imagery Program). (B) Underwater photograph of a large conical microbial mat in Lake Untersee. (C) Large conical microbial mat in Lake Untersee used in this study showing the top layer with bright-colored predominantly pigmented cyanobacteria. (D) Individual lamina of a large conical microbial mat from Lake Untersee used in this study. Notice the top layer consisted of bright colored pigmentation primarily from cyanobacteria. (LU, Lake Untersee; LCM, Large Conical Mat); (U1 = top lamina; U2 = middle lamina, and U3 = inner lamina).
Purification of Community DNA
Five samples (∼1 cm2 each) from different segments of each lamina of the large conical mat were sliced with a sterile scalpel, transferred into separate microcentrifuge tubes consisting of beads (MO BIO Laboratories Inc., Carlsbad, CA, United States), and homogenized in a high velocity bead beater (BIO101/Savant FastPrep FP120 – Qiagen, Inc., Valencia, CA, United States). DNA was then purified using the PowerBiofilm®DNA isolation Kit (MO BIO Laboratories) (Abbasian et al., 2015). The large conical mats used in this study are unique, found only in Lake Untersee. Therefore, to minimize sample collection while obtaining sufficient DNA to investigate the microbial composition of the mat laminae, we used five spatially representative samples from each lamina, and pooled the purified DNA into single samples for amplicon sequencing. The concentration and the quality of the pooled DNA from each mat lamina was determined by using a Lambda II spectrophotometer (Perkin Elmer, Norwalk, Conn.) followed by agarose gel electrophoresis (1% wt/vol agarose in 1X Tris-Acetate-EDTA (TAE) buffer, pH 7.8) to confirm that each sample consists of mostly high molecular weight DNA (>2 kbp) (Ausubel et al., 1987). The DNA was then dried in a Savant Speedvac Evaporator SVC 100H and stored at 4°C until used for sequencing.
Parallel Bacterial Tag-Encoded FLX-Amplicon Pyrosequencing (bTEFAP)
Bacterial tag-encoded FLX amplicon pyrosequencing (bTEFAP) was performed as described previously using the primers 341F (5′–CCTACGGGAGGCAGCAG–3′) (Muyzer et al., 1993) and 907R (5′–CCGTCAATTCMTTTGAGTTT–3′) (Lane et al., 1985) targeting the V3–V5 region of the 16S rRNA (Dowd et al., 2008a; Suchodolski et al., 2009; Finegold et al., 2010; Smith et al., 2010; Andreotti et al., 2011; Koo et al., 2014a,b). For cyanobacterial diversity, oligonucleotide primers CYA106F (5′- CGGACGGGTGAGTAACGCGTGA-3′) and CYA781R (5′-GACTACWGGGGTATCTAATCCCWTT-3′) (Nübel et al., 1997) targeting the 16S rRNA gene were used. First, a sequencing library was established by using a one-step PCR method with a total of 30 cycles of template DNA amplification with the HotStarTaq Plus Master Mix kit (Qiagen, Inc., Valencia, CA, United States), and amplicons originating and extending from the aforementioned bacteria- and cyanobacteria-specific primers. Then, tag-encoded FLX amplicon pyrosequencing was performed on a Roche 454 FLX instrument with Titanium reagents following the Titanium procedures and RTL protocols at the Research and Testing Laboratory (Lubbock, TX, United States)1 (Dowd et al., 2008b). The raw sequence reads have been deposited on the publicly available database located at the SRA of NCBI under accession number SRP078055.
Sequence Processing Using Bioinformatics Tools
All total of 15,999 raw barcoded pyrosequence reads from the bacterial and cyanobacterial 16S rRNA genes from U1, U2, and U3 laminae were merged into fna, qual, and mapping file formats (Table 1). Then, the split_libraries.py command was used to split the sequence libraries based upon the barcodes specified in the mapping file by using the Quantitative Insights into Microbial Ecology (QIIME, v1.8.0) pipeline (Caporaso et al., 2010b). Chimera sequence reads were removed using identify_chimeric_seqs.py module of USEARCH (Edgar, 2010). The poor-quality sequence reads that did not match sufficiently with the primer sequences, barcode sequences, or contained any ambiguous reads, or homopolymers of more than 8 bp, or had average quality scores of less than 28 were excluded from the rest of the analysis (Kunin et al., 2010) resulting into a total of 14,137 sequence reads (Table 1). The quality of sequence reads in all samples were evaluated by PRINSEQ (Schmieder and Edwards, 2011). The result showed a normally distributed sequence length (mean sequence length value of 380.78 ± 72.60 bp) and GC content (mean value of 54.55 ± 2.24%). The majority of the sequences had a mean Phred quality score of 32–33, and no occurrence of ambiguous nucleotides (N) (Schmieder and Edwards, 2011). After the trimming processes, reads were clustered into a total of 1,630 unique Operational Taxonomic Units (OTUs) at a 3% distance using UCLUST (Table 1) (Edgar, 2010). Then, representative sequences were selected from each OTU and aligned using PyNAST (Caporaso et al., 2010a). The Ribosomal Database Program (RDP) classifier2, trained using the Greengenes (v13.8) database3 (McDonald et al., 2012), was used to assign taxonomy for each representative sequence at a confidence threshold of 80% (0.8) (Wang et al., 2007). A single OTU table consisting of all OTUs, taxonomic and abundance information was used to generate stacked column bar graphs of relative abundance using Microsoft Excel software (Microsoft, Seattle, WA, United States). In order to normalize sequence reads across all samples, the “single_rarefaction.py” command in QIIME was used, which resulted in an even sampling depth of 4,460 reads per sample. The subsampled sequence reads were then used for downstream Alpha [observed OTUs, Shannon diversity index (Shannon et al., 1964), and Simpson diversity index (Simpson, 1949)], as well as Beta (principle coordinate analysis (PCoA) plot) diversity analyses. The real_edge table and real_node_table files generated from QIIME (v1.8.0) were exported into Cytoscape (v2.8.2) (Shannon et al., 2003) and an edge-weighted force-directed eweight layout was selected to generate OTU network (Pope et al., 2012). Top 25 most highly abundant taxa at the family level were selected, and then used to generate the heatmap using “heatmap.2” function in R package4.
Oligotyping Analysis
Oligotyping (v1.0) bioinformatics tools was used to assign an oligotype identity from similarly clustered sequence reads based on their nucleotide variation (Eren et al., 2013, 2014; Schmidt et al., 2014). In this study, family Cytophagaceae, Opitutaceae, and Xanthomonadaceae, and genus Phormidium and Leptolyngbya sequence reads were extracted separately from the “seq.fna” file generated through the QIIME pipeline (v1.8.0), and the five extracted sequence reads were used separately to calculate Shannon entropy (Shannon et al., 1964) using “entropy-analysis” command embedded in oligotyping (v1.0). Since the number of sequences from the five extracted sequence files were below 5,000 per reads, a value of the minimum count of the most abundant unique sequence in an oligotype (M) was set up as 5 for Opitutaceae, 3 for Phormidium, 2 for Cytophagaceae and Leptolyngbya, 1 for Xanthomonadaceae, and a value of the minimum actual abundance of an oligotype across all layer samples (A) was set up as 10 for Opitutaceae or 0 for Cytophagaceae, Xanthomonadaceae, Phormidium, and Leptolyngbya to conduct oligotyping (v1.0) (Menke et al., 2014). Oligotypes that did not meet these criteria were removed, and ≥80% reads were retained for each oligotyping results. After completing the analysis, each oligotype representative sequence was subjected to BLAST search in NCBI non-redundant (nr) database5.
PICRUSt Analysis for Predicted Metabolic Functions
Phylogenetic Investigation of Communities by Reconstruction of Unobserved States (PICRUSt v1.0.06) (Langille et al., 2013) analysis was conducted to determine the predicted metabolic functions of the microbial communities in three large conical mat laminae. Cyanobacteria and heterotrophic bacterial sequences were separated from the seq.fna file and each was used for the PICRUSt analysis. As suggested in the PICRUSt (v1.0.0) protocol, OTUs were closed-referenced picked against the Greengenes (v13.5) database at a 97% identity from each sequence file. The resultant OTU table was then used to predict metabolic functions by referencing the Kyoto Encyclopedia of Genes and Genome (KEGG) Orthology (KO) Database7 (Kanehisa and Goto, 2000; Kanehisa et al., 2014), using the “predict_metagenomes.py” command in PICRUSt (v1.0.0). The likely accuracy of the PICRUSt predictions was estimated using the Nearest Sequenced Taxon Index (NSTI) value, which is the average branch length separating OTUs in each sample from the reference genome (Langille et al., 2013). The NSTI value is considered to be the standard method for validation of the PICRUSt-predicted KEGG functional groups (Langille et al., 2013; Staley et al., 2014; Zeng et al., 2015; Hu et al., 2016; Lokesh and Kiron, 2016; Lopes et al., 2016; Yuan et al., 2016). Low NSTI values imply a close relationship to organisms in the known microbial reference genome databases, representing high accuracy of the predicted KEGG functional groups. A pairwise statistical comparison of the predicted metabolic functions in the three laminae (U1 vs. U2; U1 vs. U3; and U2 vs. U3) was carried out using STAMP (Parks et al., 2014), two sided G-test (w/Yates’) + Fisher’s statistical test with the DP: asymptotic-CC confidence interval method with the Benjamini–Hochberg FDR multiple test correction using a P-value of <0.05 (Mason et al., 2012; Li et al., 2014).
Results
Taxonomic Distribution in Top Three Laminae
The relative abundances of microbial taxa identified to the most resolvable level (up to family or genus) in each of the three laminae are elaborated in Figure 2. The U1 lamina represented by the highest relative abundances of members from phylum cyanobacteria (91.2%). Within cyanobacteria, Phormidium (65.8%) was found to be a dominant taxon followed by Leptolyngbya (11.1%), and Pseudanabaena (1.8%) (Figure 2). Pyrosequencing using the bacteria-specific primers on the U1 lamina produced a negligible number of poor quality sequence reads (NCBI accession number: SRP104174). Additionally, the cyanobacteria-specific primers used on the same U1 sample showed a low occurrence of sequences belonging to heterotrophs (<1%).
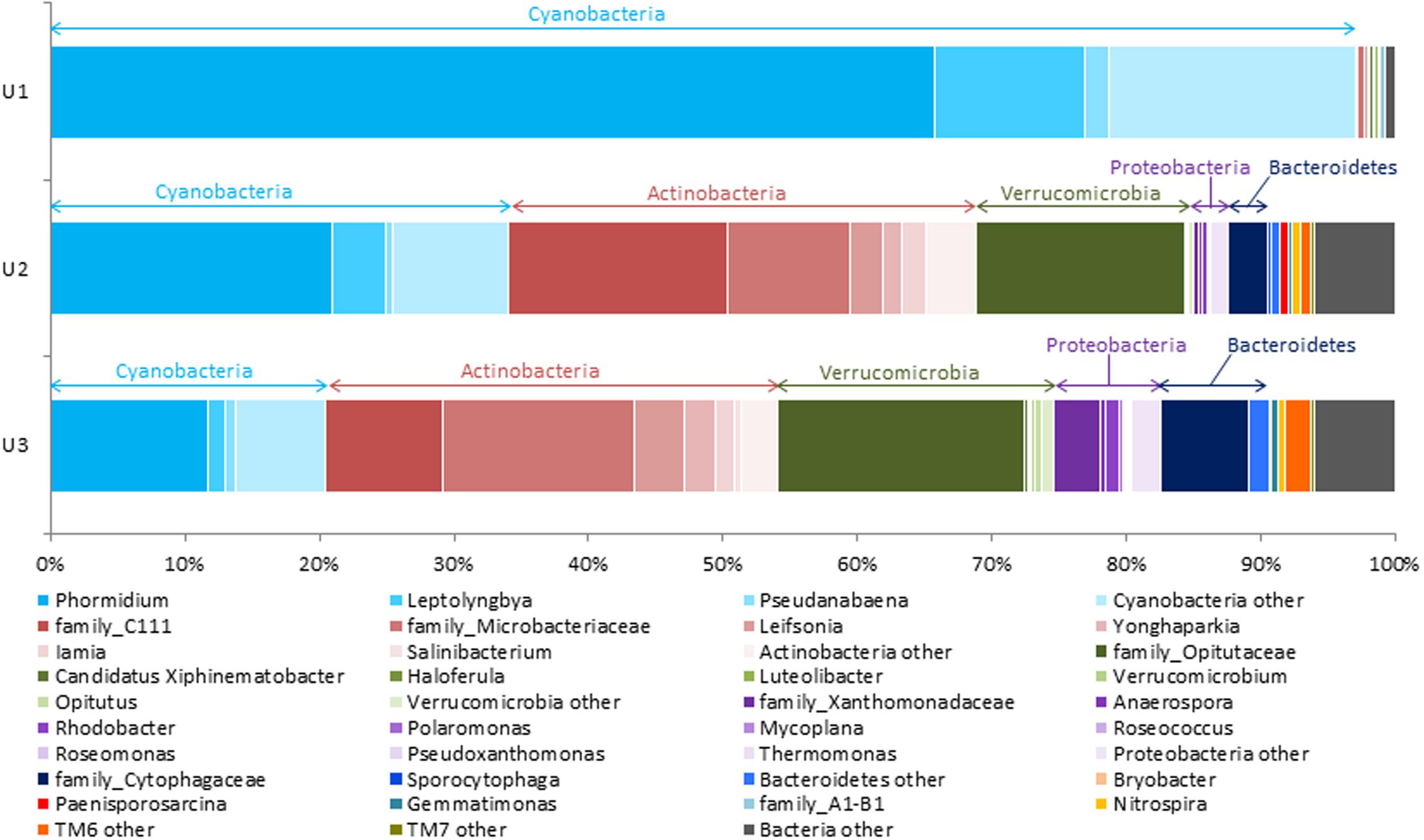
FIGURE 2. Stacked column bar graph representing the relative abundance and distribution of the most abundant taxa up to genus level across the three laminae in this study. The figure shows that while phylum Cyanobacteria was mostly dominant in U1, the relative abundance of Cyanobacteria decreased through U2 and U3. At the highest resolution, Phormidium was found to be the most abundant taxa within the Cyanobacteria in the all laminae. The U3 had a higher abundance of Microbacteriaceae, Opitutaceae, Xanthomonadaceae, and Cytophagaceae as compared to U2, except for C111, the abundance of which was found to be decreased in U3. Sequence data analyses was performed using QIIME (v1.8.0), and the stacked column bar graph was generated using Microsoft Excel software (Microsoft, Seattle, WA, United States).
The U2 and U3 laminae were dominated by heterotrophic bacterial taxa. Within the heterotrophs, family C111 and Microbacteriaceae, Opitutaceae, Cytophagaceae, and Xanthomonadaceae were found to be the highly abundant taxa in U2 and U3 laminae (Figure 2). At a higher taxa resolution (up to genus level) from within these families, Leifsonia, Iamia, Rhodobacter, and Opitutus were detected in both U2 and U3 laminae (Figure 2).
Further, Actinobacteria (34.4%) and Verrucomicrobia (16%) were found to be the dominant heterotrophic bacterial taxa, and cyanobacteria were represented by relatively low abundances of Phormidium (20.6%), Leptolyngbya (4%), and Pseudanabaena: (0.5%) in the U2 lamina (Figure 2). The taxonomic distribution of heterotrophic microbial communities in the U3 lamina revealed high relative abundances of Actinobacteria (33.3%) and Verrucomicrobia (20.4%), and low relative abundances of cyanobacteria representing Phormidium (11.5%), Leptolyngbya (1.3%), and Pseudanabaena (0.8%) (Figure 2). Interestingly, Polaromonas, Roseomonas, and Thermomonas were only detected in the U3 lamina (Figure 2). A detailed taxonomic list at the genus level for each of the three laminae has been included in the Supplementary Table 1. The overall phylum and genus level taxonomic distribution of cyanobacteria and heterotrophic bacteria and their relative abundances in U1, U2, and U3 laminae have been elaborated in Supplementary Figures 1A–H, respectively.
Differentiation of Taxa Using Oligotyping Method
To improve the resolution of the taxa up to species level, sequences extracted from Phormidium, Leptolyngbya, Cytophagaceae, Opitutaceae, and Xanthomonadaceae from the three laminae were subjected to oligotyping followed by BLAST analyses (Figure 3 and Supplementary Table 2). Total sequences, the number of oligotypes, and the number of shared oligotype for these taxa were elaborated in Table 2. Family C111 and Microbacteriaceae were excluded from oligotyping analysis due to the high variation among each of the representative sequences, as well as limited genera information available for these families within 16S rRNA reference taxonomic databases (e.g., Greengenes). The representative oligotypes, their diversity and relative abundances in U1, U2, and U3 laminae are listed in Figure 3, and in Supplementary Table 2. BLAST results of the representative oligotypes from the Phormidium taxon showed that Phormidium cf. uncinatum CYN108 and Phormidium sp. Ant-Orange were found to be the most highly abundant taxa in three laminae (Figure 3A and Supplementary Table 2). Phormidium autumnale, Phormidium cf. uncinatum CAWBG523, and Phormidium spp. were also found in each of the three laminae (Figure 3A and Supplementary Table 2). Leptolyngbya antarctica ANT and Leptolyngbya sp. were found to be the most abundant taxa in U1, U2, and U3 laminae (Figure 3B and Supplementary Table 2). Uncultured Bacteroidetes, Cytophaga sp., and Flexibacter aggregans were found to be highly abundant in U2 and U3 laminae (Figure 3C and Supplementary Table 2). There were no sequences assigned to Cytophagaceae in U1 lamina (Figure 3C). All oligotypes identified within Opitutaceae in U1, U2, and U3 laminae closely matched with genus Opitutus spp. (Figure 3D and Supplementary Table 2). In addition, Pseudoxanthomonas sp., Pseudomonas sp., Xanthomonas sp., and Stenotrophomonas sp. belonging to Family Xanthomonadaceae were highly dominant in U2 and U3 laminae (Figure 3E and Supplementary Table 2). The identity and the e-value of each taxa resulting from the BLAST analysis have been listed in Supplementary Table 2.
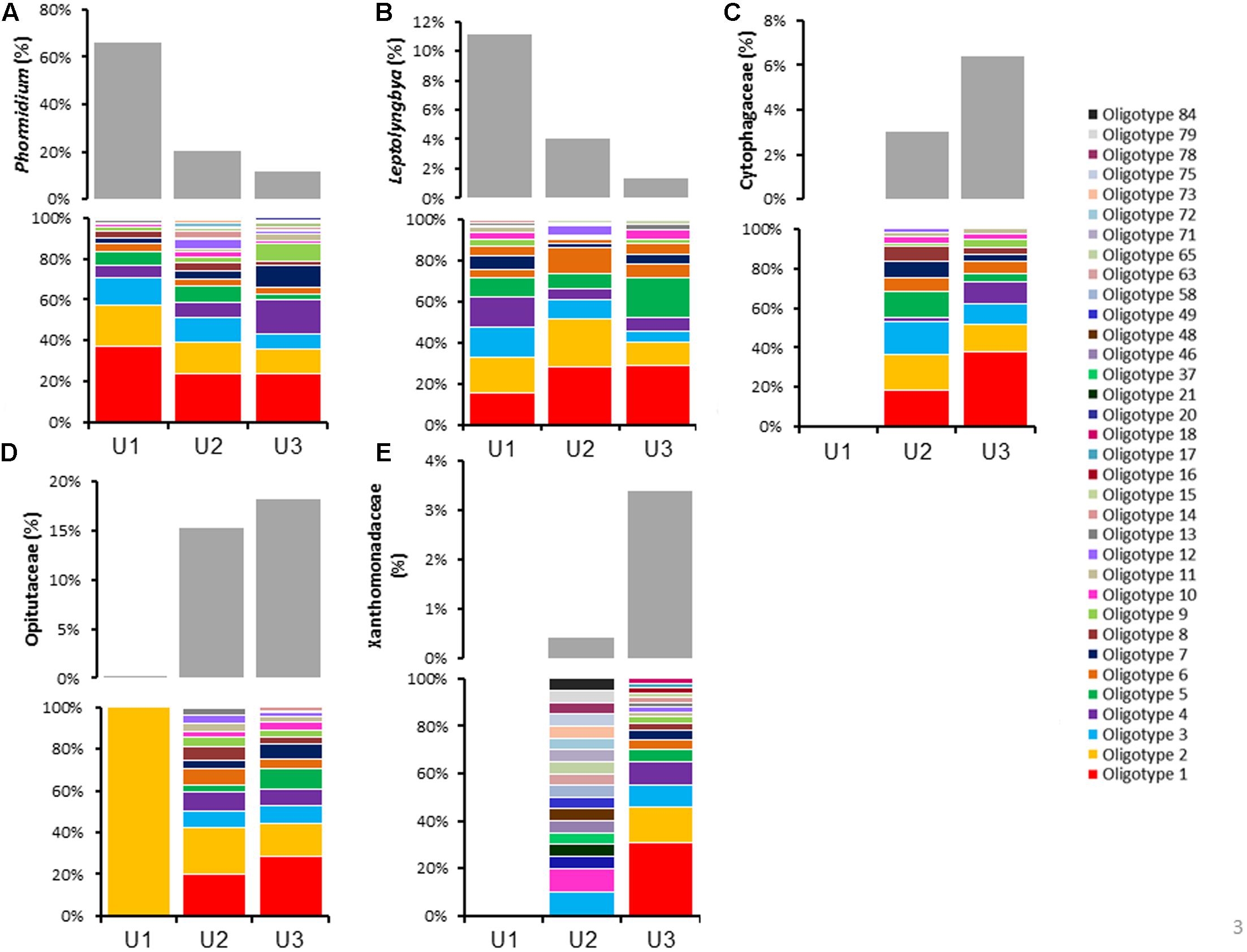
FIGURE 3. Oligotype profiles describing the distribution and abundances of each dominant microbial taxon in three laminae (U1, U2, and U3). Color bars within each (but not between) graph represent the oligotypes within (A) Phormidium, (B) Leptolyngbya, (C) Cytophagaceae, (D) Opitutaceae, and (E) Xanthomonadaceae. The gray bars above each color graph represent the abundance of sequence reads that were assigned to different bacterial taxa. Oligotyping analyses were performed using the open-source pipeline for oligotyping, available at http://oligotyping.org. Note that no Cytophagaceae and Xanthomonadaceae sequences were identified in U1 lamina, as shown in graphs (C,E).
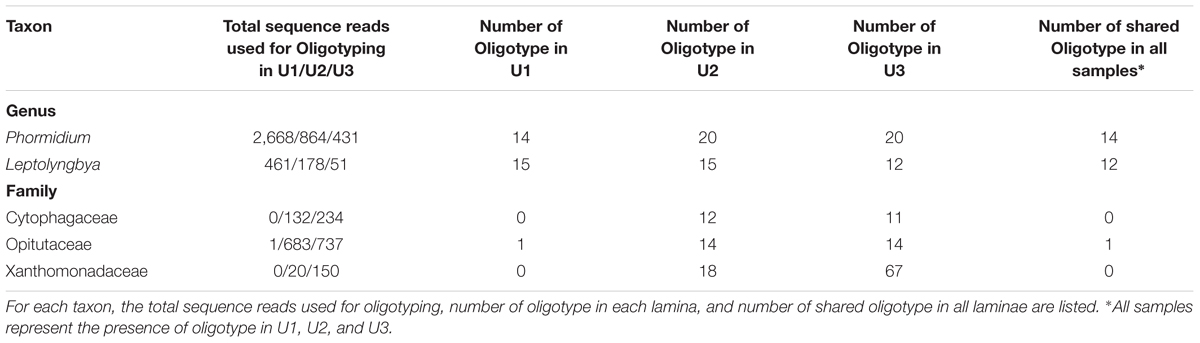
TABLE 2. Oligotyping results for the U1, U2, and U3 laminae of a large conical mat samples from Lake Untersee used in this study.
Alpha and Beta Diversity Estimation of the Microbial Communities
A heatmap was constructed to represent the microbial abundances and compositions of top 25 taxa at family level in U1, U2, and U3 laminae (Figure 4). The relative abundance for each bacterial taxon was shown by color intensity, as depicted by the legend elaborated in Figure 4.
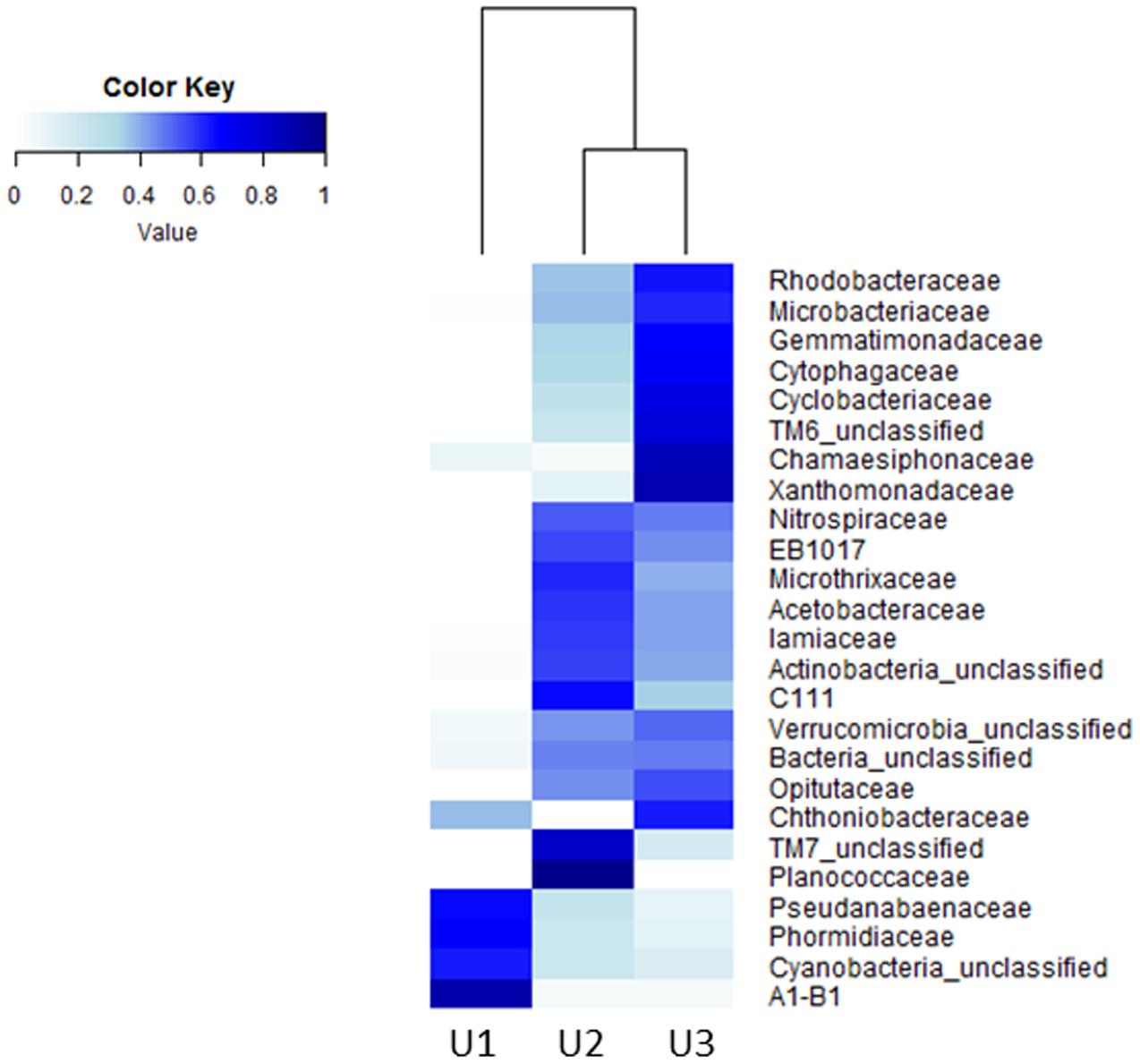
FIGURE 4. Heatmap generated by using the “heatmap.2” function in R package to show the microbial compositions of the top 25 taxa at family level in U1, U2, and U3 of a conical microbial mat from Lake Untersee, Antarctica. The rows indicate the bacterial taxa and the columns represent the three mat laminae used in this study.
The Shannon diversity index (Shannon et al., 1964) and Simpson diversity index (Simpson, 1949) showed microbial diversity in each of the three laminae (Table 1). The weighted UniFrac generated OTU network (Figure 5A) and PCoA plots (Figure 5B) show distribution of the observed microbial taxa in U1, U2, and U3 laminae. The results showed that unique cyanobacterial OTUs clustered in the U1 lamina and a large number of common heterotrophic bacterial OTUs in U2 and U3 laminae (Figure 5A), which is also supported by the PCoA plot (Figure 5B). The rarefaction curves of sequences from all three laminae were saturated with respect to the species richness and diversity (Supplementary Figure 2).
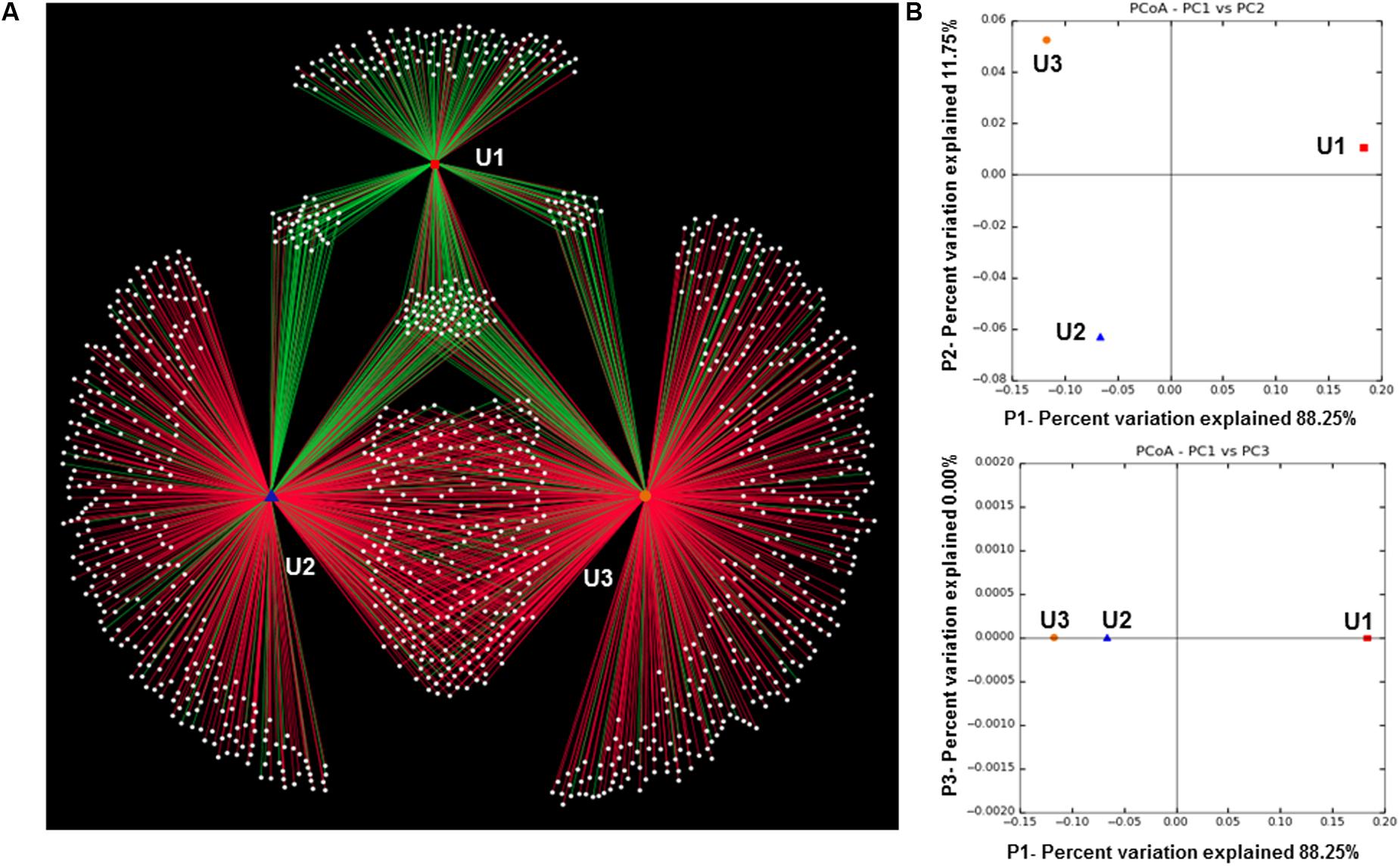
FIGURE 5. (A) Operational Taxonomic Units (OTU) network generated through QIIME (v1.8.0) and Cytoscape (v2.8.2). The green and red edges from the peripheral white nodes are OTUs representing cyanobacteria and heterotrophic bacteria, respectively. The Edge-Weighted Spring-Embedded Eweights format was used to generate the OTU network map; (B) two PCoA plots generated by QIIME (v1.8.0) representing significant differences in bacterial community cluster among U1, U2, and U3 of the conical microbial mat from Lake Untersee, Antarctica.
Predicted Metabolic Functions Using PICRUSt
Predicted metabolic functions of the microbial communities in U1, U2, and U3 laminae were investigated by using PICRUSt (v 1.0.0) (Figure 6). The KEGG level 2 results showed that the U1 lamina had a high number of sequences assigned to energy metabolism such as carbon fixation in photosynthetic organisms; oxidative phosphorylation; photosynthesis; and methane, nitrogen, and sulfur metabolism. The U2 and U3 laminae depicted functional categories such as amino acid and carbohydrate metabolisms, and membrane transport proteins (Figure 6 and Supplementary Table 3). Results using PICRUSt on cyanobacteria collectively showed functional categories of transporters, photosynthesis proteins, ABC transporters, purine metabolism, peptidases, DNA repair and recombination proteins, photosynthesis, porphyrin and chlorophyll metabolisms, and photosynthesis-antenna proteins at KEGG level 3 (Supplementary Figure 3 and Table 4). The functional categories collectively for the heterotrophic bacteria at KEGG level 3 showed a high number of sequences in transporters, ABC transporters, DNA repair and recombination proteins, purine metabolism, phosphotransferase system (PTS), and a high number of sequences for ribosome and two-component systems (Supplementary Figure 3 and Table 4). Detailed PICRUSt results of additional metabolic functions at KEGG levels 2 and 3 have been elaborated in Supplementary Figure 3 and Tables 3, 4.
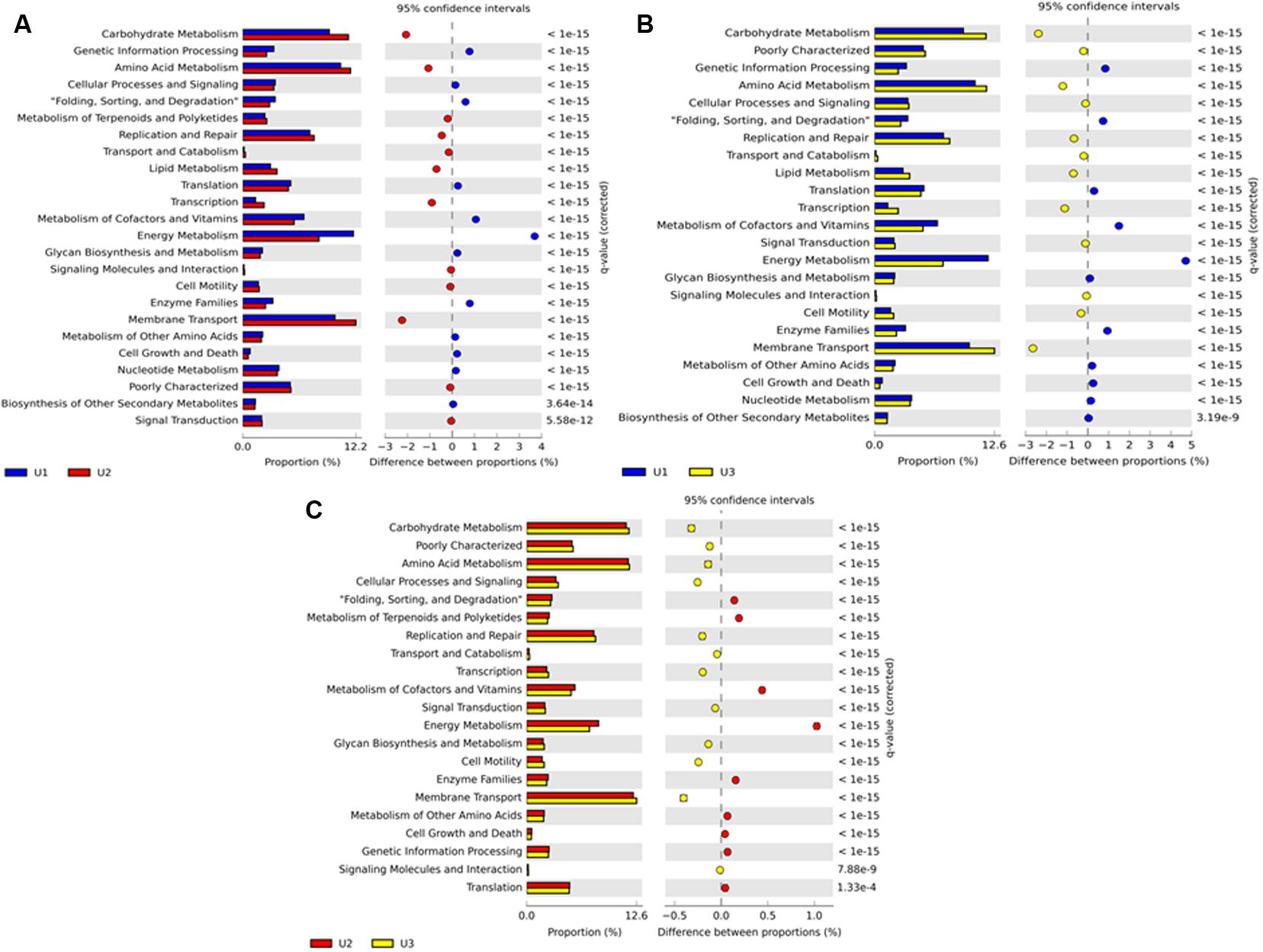
FIGURE 6. Stacked column bar graph representing the predicted metabolic attributes compare between (A) U1 and U2; (B) U1 and U3; and (C) U2 and U3 laminae of a large conical mat from Lake Untersee, Antarctica. All sequence reads were used to predict functions against the KEGG database (http://www.genome.jp/kegg/), which is implemented in PICRUSt (http://picrust.github.io/picrust/) bioinformatics software package. The mean Nearest Sequenced Taxon Index (NSTI) value for all three laminae was 0.11 ± 0.017 s.d.
The NSTI value was determined for each lamina (0.10 in U1; 0.13 in U2; and 0.11 in U3) and the mean value calculated to be 0.11 ± 0.017 s.d. for all laminae. These values are either comparable or better than the mean NSTI value ranging from 0.10 ± 0.016 s.d. to 0.23 ± 0.02 s.d. reported for microbial communities of samples collected from diverse environments (Langille et al., 2013; Staley et al., 2014; Zeng et al., 2015; Hu et al., 2016; Lokesh and Kiron, 2016; Lopes et al., 2016; Yuan et al., 2016). These values indicate that the predicted metabolic functions displayed by the observed microbial taxa in each of the three laminae are close to the known microbial reference genome databases, and thus imply a higher accuracy of the predictions. Predictive microbial functions along with the microbial taxonomic classification of the microbial communities of U1, U2, and U3 laminae of the conical mat have been summarized in Figure 7.
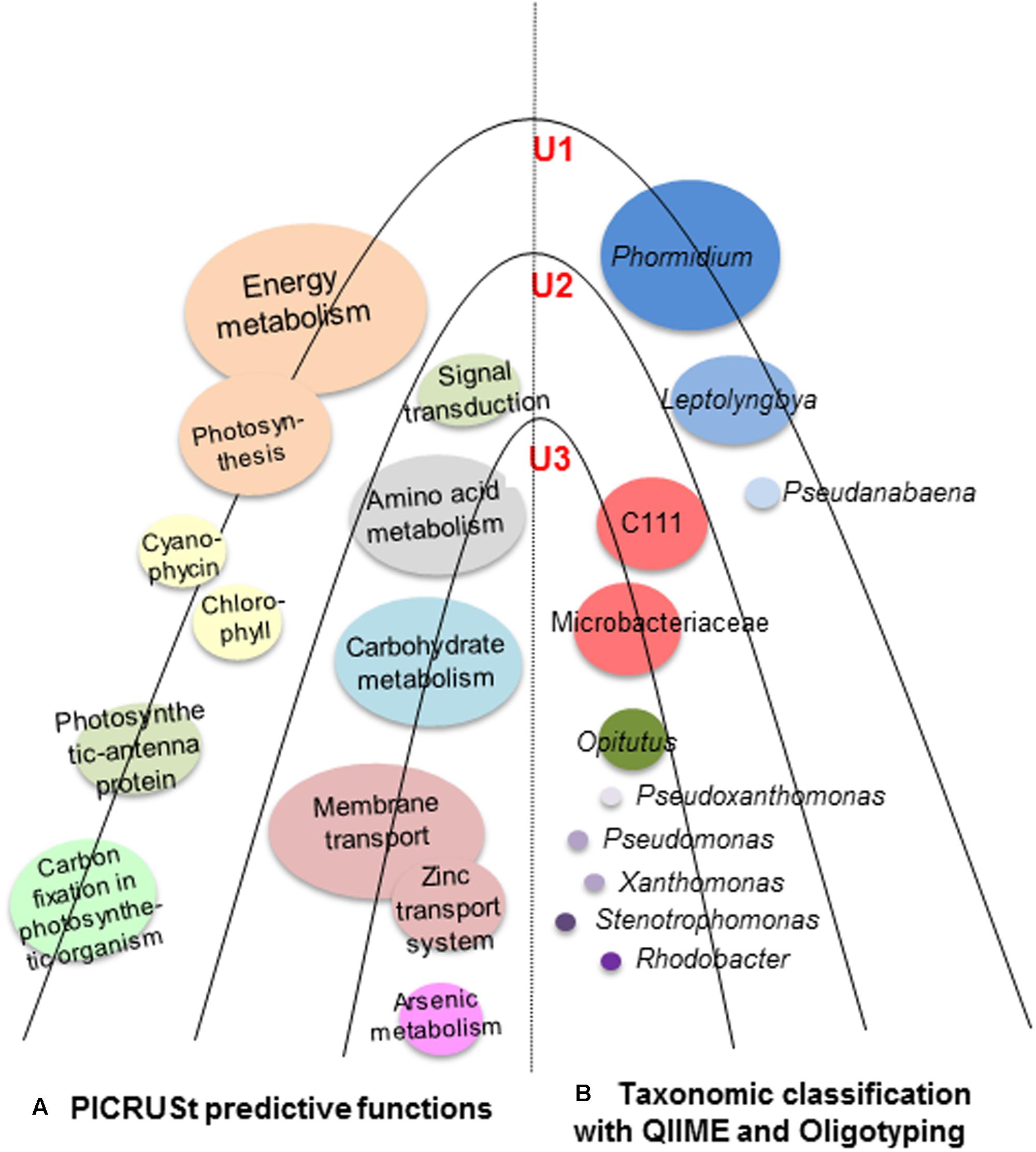
FIGURE 7. Schematic diagram of U1, U2 and U3 laminae of a large conical mat from Lake Untersee, Antarctica. This diagram shows (A) predicted metabolic functions (PICRUSt) elucidating the metabolism of the carbon, sulfur, and nitrogen in the conical mat; and (B) microbial taxonomic classification of the microbial communities in the conical mat was analyzed by both QIIME and Oligotyping. The U1 lamina was dominated by cyanobacteria, which contributed to high energy metabolism. The U2 and U3 laminae were dominated by heterotrophic bacteria, which were involved in various metabolic activities, particularly membrane transport, amino acid and carbohydrate metabolism. The mean Nearest Sequenced Taxon Index (NSTI) value for all three laminae was 0.11 ± 0.017 s.d.
Discussion
The mats in Antarctic ice-covered lakes are comprised of complex microbial communities and are composed primarily of cyanobacteria and heterotrophs (Andersen et al., 2011; Mackey et al., 2015; Jungblut et al., 2016). The large conical mats of Lake Untersee form distinct laminae with each layer being ∼0.5 mm in thickness, and a top lamina dominated by distinctive pigmented photosynthetic cyanobacteria (Andersen et al., 2011). Since these laminae are easily distinguishable, we have explored the taxonomic composition and predicted metabolic functions of microbial communities of the top three laminae (U1, U2, and U3) by using NextGen sequencing technology and bioinformatics tools. The results showed that cyanobacteria dominated the U1 lamina (Figures 2, 4, 5). More specifically, Phormidium sp. was the most prevalent cyanobacteria, followed by Leptolyngbya sp. and Pseudanabaena sp. in these laminae (Figures 2, 3). These cyanobacterial taxa have previously been reported to occur in other benthic microbial mats, including the low irradiance environments of perennially ice-covered Antarctic lakes (Oguni and Takahashi, 1989; Taton et al., 2003; Jungblut et al., 2010; Andersen et al., 2011; Vincent and Quesada, 2012; Zhang et al., 2015). Hawes et al. (2013) demonstrated the composition of cyanobacterial communities of the microbial mats in areas recently flooded due to rising lake levels at Lake Vanda in the McMurdo Dry Valleys. They showed that the upper 2–4 laminae were dominated by orange-brown pigmented myxoxanthophyll intertwined with Leptolyngbya trichomes. Up to six laminae below the top layer were inhabited by phycobilin-rich green/pink-pigmented Leptolyngbya, Oscillatoria and Phormidium morphotypes. In a separate study, Sutherland and Hawes (2009) explored whether the changes in the cyanobacterial community composition in annual growth layers could potentially indicate proxies of past growth performances of microbial mats in various depths of Lake Hoare of the McMurdo Dry Valleys. The results from this study showed that there was no apparent effect of the species composition of cyanobacterial communities along with organic matter and extracellular polysaccharides in mats from all depths. Hawes et al. (2016) described the development of microbial mats in response to variations in irradiance in Lake Hoare of McMurdo Dry Valleys. This study revealed that photosynthetic biomass and species composition in mats remain remarkably stable for long period. The accrual of laminae and their thicknesses were directly correlated with the intensity of irradiance passing through the ice cover of this lake. The results from these studies corroborated our observation of the overall distribution of high abundances of Leptolyngbya and Phormidium in the U1 lamina of Lake Untersee large conical mats.
Further oligotyping analysis within Phormidium sp. showed three dominant species, P. autumnale, P. cf. uncinatum and P. Ant-Orange in U1, U2, and U3 laminae (Figure 3A and Supplementary Table 2). Particularly, the heightened observance of P. autumnale in the U1 lamina in this study corroborated previously reported microscopic and other biochemical analyses methods (Andersen et al., 2011). P. autumnale is a gliding photosynthetic cyanobacterium and is known to manifest horizontal to upward gliding movement along mucous-like extracellular polymeric substances (EPS), which is driven by changes in the microscale gradient of nutrients in their surrounding environment (Geurts, 1976; Freytet and Plet, 1996). Thus, the relatively high abundance of Phormidium, particularly P. autumnale in our study indicates perhaps their crucial role in the formation of the vertically oriented, uniquely structured large conical mats in Lake Untersee. However, such functions need to be verified by future experiments, to demonstrate gliding behavior, growth rate and gene regulation (Hawes et al., 2013).
Oligotyping analysis within Leptolyngbya sp. revealed two abundant species, L. antarctica ANT and L. sp. CENA538 (Figure 3B and Supplementary Table 2). Specifically, the dominant presence of L. antarctica ANT across all three laminae supports previous reports of this cyanobacterium occurring in the majority of benthic microbial communities of Antarctic lakes (Komárek, 2007; Zhang et al., 2015; Jungblut et al., 2016). The straight and irregularly coiled L. antarctica has been reported to form massively large mats particularly in the benthic environments of continually frozen lakes (Komárek, 2007). Their dominance in Lake Untersee’s limited photosynthetically active radiation (PAR) and UV-B environment could be attributed to the previously reported study where exposing Leptolyngbya-dominated microbial mats to increased UV-B relative PAR (400–700 nm) resulted in both significantly lower chlorophyll a concentrations and higher photochemical inhibition in the mats (George et al., 2001).
Lastly, the oligotyping analysis of the third most abundant cyanobacteria in our study, Pseudanabaena sp., revealed two species: Pseudanabaena catenata UAM 412, and Pseudanabaena minima CHAB705. Our observation corroborated previously reported studies of their widespread distribution in Antarctic aquatic ecosystems, but to a lesser abundance as compared to Phormidium and Leptolyngbya (Taton et al., 2006; Hawes et al., 2013).
Given the prominent abundance of the three aforementioned cyanobacteria in the U1 lamina, one can associate the notable observances of PICRUSt-predicted functional categories to these taxa as photosynthetic organisms, namely energy metabolism, cyanophycin, chlorophyll, photosynthesis protein, photosynthetic-antenna protein, and carbon fixation (Figures 6, 7, Supplementary Figure 3, and Tables 3, 4). Furthermore, our PICRUSt results support previous reports that have demonstrated that cyanobacteria are involved in photosynthetic activities (Couradeau et al., 2012) and cyanophycin production, as mat-builders in all microbialites (White et al., 2015).
The taxonomic compositions in the U2 and U3 laminae revealed a diverse group of heterotrophic bacteria with a high abundance of Actinobacteria, Verrucomicrobia, Proteobacteria, and Bacteroidetes (Figures 2, 4, 5), which were comparable to the microbial mat communities in marine and freshwater ecosystems such as Shark Bay in Western Australia (Allen et al., 2009; Goh et al., 2009); Highbourne Cay in Bahamas (Reid et al., 2000; Myshrall et al., 2010; Mobberley et al., 2013); Pavilion Lake (Laval et al., 2000; White, 2014); Clinton Creek in Canada (Power et al., 2011; White, 2014); and Highborne Cay in Northern Exumas, Bahamas (Mobberley et al., 2012; Louyakis et al., 2017). As reported in other studies, the heightened abundance of Actinobacteria in Lake Untersee microbial mats could imply a possible role in recycling organic components from decomposed cyanobacteria from the top (U1) to the inner laminae (U2 and then U3) (Goodfellow and Williams, 1983; Glöckner et al., 2000; Rondon et al., 2000; Zwart et al., 2002; Cottrell et al., 2005; Warnecke et al., 2005; Ward and Bora, 2006; Ventura et al., 2007; Philosof et al., 2009). The sequence data of U1 lamina using the bacteria-specific primers revealed a negligible abundance of heterotrophs. Subsequent bioinformatics analyses were unable to process the sequence reads. The raw sequences reads for the U1 lamina representing bacterial taxa are available at NCBI under accession number SRP104174.
The oligotyping analysis within Verrucomicrobia showed the diverse psychrotolerant, obligatory alkaliphilic, polysaccharide-utilizing ultramicrobacterium Opitutus spp., including Opitutus terrae, of family Opitutaceae (Figure 3D and Supplementary Table 2) (Wilhelm et al., 2011; Larose et al., 2013). Considering their preference to anaerobic habitats, and anaerobic nitrogen fixation metabolism reported in previous studies (Wilhelm et al., 2011; Larose et al., 2013), the observation of Opitutaceae in the inner laminae led us to predict an oxygen-depleted microenvironment within the large conical mats of Lake Untersee. However, the existence of such a microenvironment and the nitrogen fixation properties of Opitutus terrae in the inner laminae requires further investigation.
Previous reports have suggested the ability of Cytophaga to enzymatically lyse cyanobacteria and Gram-positive bacteria, in addition to performing photooxidation through respiration and promoting nitrogen fixation by lowering the oxygen concentrations in the surrounding environment (Marshall, 1989; Madigan et al., 2008). Thus, the existence of Cytophagaceae in Lake Untersee mats in U2 and U3 laminae could demonstrate a possible mutually beneficial relationship with cyanobacteria, but such function needs to be verified by future studies.
PICRUSt analysis of the observed heterotrophic bacterial taxa showed increased predicted metabolic functions in U2 and U3 lamina, which have previously been reported to be common properties of heterotrophic bacterial communities (Figures 6, 7, Supplementary Figure 1, and Tables 3, 4) (Newton et al., 2011; Varin et al., 2012). Previous reports showed that Actinobacteria and Proteobacteria are especially capable of conducting carbon metabolism, membrane transport system including zinc transport, and the stress response regulatory system (Vikram et al., 2016), which supports our PICRUSt results. Interestingly, PICRUSt predicted an elevated activity of arsenic metabolism in the U3 lamina, a mechanism that has been previously suggested to be a prominent characteristic in ancient microbial mats that are over 2.7 billion years old (Sforna et al., 2014; White et al., 2015). The mean NSTI values of our samples using PICRUSt was 0.11 ± 0.017 s.d., which is similar to or better than previously reported studies on the microbiota of: seepweed Suaeda salsa (Amaranthaceae) (NSTI = 0.17 ± 0.02 s.d.), Atlantic salmon skin (NSTI range from 0.10 ± 0.016 s.d. to 0.17 ± 0.065 s.d.), rabbit fecal samples (NSTI = 0.195 ± 0.05 s.d.), gut microbiota of miniature piglets (NSTI = 0.1469 ± 0.01902 s.d.); and environmental samples such as: hypersaline mat microbiome (mean NSTI = 0.23 ± 0.07 s.d.), soil samples from cold deserts of the Antarctic McMurdo Dry Valleys and hot deserts of the Southwestern United States (mid-range NSTI = 0.17 ± 0.02 s.d.), and rhizosphere microbial communities (mean NSTI = 0.23 ± 0.02 s.d.). Based on these NSTI values we infer with high confidence that our predicted metabolic functions are robustly supported by the reference microbial genome database (Fierer et al., 2012; Langille et al., 2013; Staley et al., 2014; Zeng et al., 2015; Hu et al., 2016; Lokesh and Kiron, 2016; Lopes et al., 2016; Yuan et al., 2016).
In summary, the large conical mats of Lake Untersee have thus far been considered unique amongst all reported perennially ice-covered lakes in the Antarctic continent (Andersen et al., 2011, 2015). These mats offer distinct laminae harboring diverse taxonomic compositions. The distribution of microbial communities showed high abundance of photosynthetic cyanobacteria in the outer U1 lamina. A high abundance of P. autumnale with gliding properties along the EPS-enriched U1 lamina indicate this species as a likely contributor for the vertical structure of these large conical mats. Predicted metabolic functions of the phototrophic cyanobacterial community in the U1 lamina showed this community capable of producing nutrients by photosynthesis. The U2 and U3 laminae consisted of a high abundance of heterotrophic bacterial taxa, of which their predicted metabolic functions determined by PICRUSt are likely involved in the decomposition of organics and metabolites. Further shotgun metagenomics analysis would substantiate detailed metabolic traits in each of these microbial communities, which could serve as an ideal model system to better understand the microbial processes that possibly contribute to the formation of these structures analogous to the ancient stromatolites.
Author Contributions
HK conducted the bioinformatics analysis of the NGS data. DA, YT, and IH conducted field work in Antarctica including the collection and processing of a conical stromatolite mat sample from Lake Untersee. NM conducted DNA extraction and pursued the DNA samples to Research and Testing Laboratory (Lubbock, TX, United States) for Pyrosequencing. AB and DA conceived the study. HK, JH, and AB wrote the manuscript and all authors contributed and commented wherever appropriate in the manuscript. AB oversaw the overall progress of this study.
Funding
Primary support for this research was provided by the Tawani Foundation of Chicago (6803099), the Trottier Family Foundation, NASA’s Exobiology and Astrobiology Programs (NNX08AO19G), and the Arctic and Antarctic Research Institute/Russian Antarctic Expedition.
Conflict of Interest Statement
The authors declare that the research was conducted in the absence of any commercial or financial relationships that could be construed as a potential conflict of interest.
Acknowledgments
We thank UAB Heflin Center for Genomic Sciences for the next-generation sequencing, UAB Cheaha HPC and HTC grid for NGS data analyses, and Matthew Pace, Mathew Thompson and T. D. Todd of CAS IT; Troy Campbell and Chris McMurray of Dell EMC (Dell Latitude 12 Rugged Extreme 7214) for computer support. Antarctic logistics support was provided by the Antarctic Logistics Centre International (ALCI), Cape Town, South Africa.
Supplementary Material
The Supplementary Material for this article can be found online at: http://journal.frontiersin.org/article/10.3389/fmicb.2017.01347/full#supplementary-material
Footnotes
- ^www.researchandtesting.com
- ^http://rdp.cme.msu.edu/
- ^http://greengenes.secondgenome.com/
- ^http://CRAN.R-project.org/package=gplots
- ^http://blast.ncbi.nlm.nih.gov/Blast.cgi
- ^http://picrust.github.io/picrust/
- ^http://www.genome.jp/kegg/ko.html
References
Abbasian, F., Lockington, R., Mallavarapu, M., and Naidu, R. (2015). A pyrosequencing-based analysis of microbial diversity governed by ecological conditions in the Winogradsky column. World J. Microbiol. Biotechnol. 31, 1–12. doi: 10.1007/s11274-015-1861-y
Allen, M., Goh, F., Burns, B., and Neilan, B. (2009). Bacterial, archaeal and eukaryotic diversity of smooth and pustular microbial mat communities in the hypersaline lagoon of Shark Bay. Geobiology 7, 82–96. doi: 10.1111/j.1472-4669.2008.00187.x
Andersen, D. (2007). “Antarctic inland waters: scientific diving in the perennially ice-covered lakes of the McMurdo Dry Valleys and Bunger Hills,” in Proceedings of the International Polar Diving Workshop, eds M. A. Lang and M. D. J. Sayer (Washington, DC: Smithsonian Institution), 213.
Andersen, D., Sumner, D., Hawes, I., Webster-brown, J., and McKay, C. (2011). Discovery of large conical stromatolites in Lake Untersee, Antarctica. Geobiology 9, 280–293. doi: 10.1111/j.1472-4669.2011.00279.x
Andersen, D. T., McKay, C. P., and Lagun, V. (2015). Climate conditions at perennially ice-covered lake untersee, East Antarctica. J. Appl. Meteorol. 54, 1393–1412. doi: 10.1175/JAMC-D-14-0251.1
Andreotti, R., de León, A. A. P., Dowd, S. E., Guerrero, F. D., Bendele, K. G., and Scoles, G. A. (2011). Assessment of bacterial diversity in the cattle tick Rhipicephalus (Boophilus) microplus through tag-encoded pyrosequencing. BMC Microbiol. 11:6. doi: 10.1186/1471-2180-11-6
Ausubel, F. M., Brent, R., Kingston, R. E., Moore, D. D., Smith, J. G., Sideman, J. G., et al. (1987). Short Protocols in Molecular Biology: A Compendium of Methods from Current Protocols in Molecular Biology. New York, NY: John Wiley & Sons, Inc.
Caporaso, J. G., Bittinger, K., Bushman, F. D., DeSantis, T. Z., Andersen, G. L., and Knight, R. (2010a). PyNAST: a flexible tool for aligning sequences to a template alignment. Bioinformatics 26, 266–267. doi: 10.1093/bioinformatics/btp636
Caporaso, J. G., Kuczynski, J., Stombaugh, J., Bittinger, K., Bushman, F. D., Costello, E. K., et al. (2010b). QIIME allows analysis of high-throughput community sequencing data. Nat. Methods. 7, 335–336. doi: 10.1038/nmeth.f.303
Cavicchioli, R. (2015). Microbial ecology of Antarctic aquatic systems. Nat. Rev. Microbiol. 13, 691–706. doi: 10.1038/nrmicro3549
Clocksin, K. M., Jung, D. O., and Madigan, M. T. (2007). Cold-active chemoorganotrophic bacteria from permanently ice-covered Lake Hoare, McMurdo Dry Valleys, Antarctica. Appl. Environ. Microbiol. 73, 3077–3083. doi: 10.1128/AEM.00085-07
Cottrell, M. T., Waidner, L. A., Yu, L., and Kirchman, D. L. (2005). Bacterial diversity of metagenomic and PCR libraries from the Delaware River. Environ. Microbiol. 7, 1883–1895. doi: 10.1111/j.1462-2920.2005.00762.x
Couradeau, E., Benzerara, K., Gérard, E., Moreira, D., Bernard, S., Brown, G. E., et al. (2012). An early-branching microbialite cyanobacterium forms intracellular carbonates. Science 336, 459–462. doi: 10.1126/science.1216171
Dowd, S. E., Sun, Y., Secor, P. R., Rhoads, D. D., Wolcott, B. M., James, G. A., et al. (2008a). Survey of bacterial diversity in chronic wounds using Pyrosequencing, DGGE, and full ribosome shotgun sequencing. BMC Microbiol. 8:43. doi: 10.1186/1471-2180-8-43
Dowd, S. E., Sun, Y., Wolcott, R. D., Domingo, A., and Carroll, J. A. (2008b). Bacterial tag-encoded FLX amplicon pyrosequencing (bTEFAP) for microbiome studies: bacterial diversity in the ileum of newly weaned Salmonella-infected pigs. Foodborne Pathol. Dis. 5, 459–472. doi: 10.1089/fpd.2008.0107
Edgar, R. C. (2010). Search and clustering orders of magnitude faster than BLAST. Bioinformatics 26, 2460–2461. doi: 10.1093/bioinformatics/btq461
Eren, A. M., Borisy, G. G., Huse, S. M., and Welch, J. L. M. (2014). Oligotyping analysis of the human oral microbiome. Proc. Natl. Acad. Sci. U.S.A. 111, E2875–E2884. doi: 10.1073/pnas.1409644111
Eren, A. M., Maignien, L., Sul, W. J., Murphy, L. G., Grim, S. L., Morrison, H. G., et al. (2013). Oligotyping: differentiating between closely related microbial taxa using 16S rRNA gene data. Methods Ecol. Evol. 4, 1111–1119. doi: 10.1111/2041-210X.12114
Fierer, N., Leff, J. W., Adams, B. J., Nielsen, U. N., Bates, S. T., Lauber, C. L., et al. (2012). Cross-biome metagenomic analyses of soil microbial communities and their functional attributes. Proc. Natl. Acad. Sci. U.S.A. 109, 21390–21395. doi: 10.1073/pnas.1215210110
Finegold, S. M., Dowd, S. E., Gontcharova, V., Liu, C., Henley, K. E., Wolcott, R. D., et al. (2010). Pyrosequencing study of fecal microflora of autistic and control children. Anaerobe 16, 444–453. doi: 10.1016/j.anaerobe.2010.06.008
Foster, J. S., and Green, S. J. (2011). “Microbial diversity in modern stromatolites,” in Stromatolites: Interaction of Microbes with Sediments, eds V. Tewari and J. Seckbach (Dordrecht: Springer), 383–405. doi: 10.1007/978-94-007-0397-1_17
Franco, D. C., Signori, C. N., Duarte, R. T., Nakayama, C. R., Campos, L. S., and Pellizari, V. H. (2017). High prevalence of gammaproteobacteria in the sediments of admiralty bay and north bransfield Basin, Northwestern Antarctic Peninsula. Front. Microbiol 8:153. doi: 10.3389/fmicb.2017.00153
Franks, J., and Stolz, J. F. (2009). Flat laminated microbial mat communities. Earth Sci. Rev. 96, 163–172. doi: 10.1016/j.earscirev.2008.10.004
Freytet, P., and Plet, A. (1996). Modern freshwater microbial carbonates: the Phormidium stromatolites (tufa-travertine) of southeastern Burgundy (Paris Basin, France). Facies 34, 219–237. doi: 10.1007/BF02546166
George, A. L., Murray, A. W., and Montiel, P. O. (2001). Tolerance of Antarctic cyanobacterial mats to enhanced UV radiation. FEMS Microbiol. Ecol. 37, 91–101. doi: 10.1111/j.1574-6941.2001.tb00856.x
Gerdes, G. (2010). “What are microbial mats?,” in Microbial Mats, eds J. Seckbach and A. Oren (Dordrecht: Springer), 3–25. doi: 10.1007/978-90-481-3799-2_1
Geurts, M.-A. (1976). Genèse et stratigraphie des travertins de fond de vallée en Belgique. Acta Geogr. Louv. 16:66.
Glatz, R., Lepp, P., Ward, B., and Francis, C. (2006). Planktonic microbial community composition across steep physical/chemical gradients in permanently ice-covered Lake Bonney, Antarctica. Geobiology 4, 53–67. doi: 10.1111/j.1472-4669.2006.00057.x
Glöckner, F. O., Zaichikov, E., Belkova, N., Denissova, L., Pernthaler, J., Pernthaler, A., et al. (2000). Comparative 16S rRNA analysis of lake bacterioplankton reveals globally distributed phylogenetic clusters including an abundant group of actinobacteria. Appl. Environ. Microbiol. 66, 5053–5065. doi: 10.1128/AEM.66.11.5053-5065.2000
Goh, F., Allen, M. A., Leuko, S., Kawaguchi, T., Decho, A. W., Burns, B. P., et al. (2009). Determining the specific microbial populations and their spatial distribution within the stromatolite ecosystem of Shark Bay. ISME J. 3, 383–396. doi: 10.1038/ismej.2008.114
Goodfellow, M., and Williams, S. (1983). Ecology of actinomycetes. Ann. Rev. Microbiol. 37, 189–216. doi: 10.1146/annurev.mi.37.100183.001201
Grotzinger, J. P., and Knoll, A. H. (1999). Stromatolites in Precambrian carbonates: evolutionary mileposts or environmental dipsticks? Annu. Rev. Earth Planet. Sci. 27, 313–358. doi: 10.1146/annurev.earth.27.1.313
Guerrero, R., and Berlanga, M. (2010). The hidden side of the prokaryotic cell: rediscovering the microbial world. Int. Microbiol. 10, 157–168. doi: 10.2436/20.1501.01.23
Gugliandolo, C., Michaud, L., Giudice, A. L., Lentini, V., Rochera, C., Camacho, A., et al. (2016). Prokaryotic community in lacustrine sediments of byers Peninsula (Livingston Island, Maritime Antarctica). Microb. Ecol. 71, 387–400. doi: 10.1007/s00248-015-0666-8
Hawes, I., Jungblut, A. D., Obryk, M. K., and Doran, P. T. (2016). Growth dynamics of a laminated microbial mat in response to variable irradiance in an Antarctic lake. Freshw. Biol. 61, 396–410. doi: 10.1111/fwb.12715
Hawes, I., Sumner, D., Andersen, D., and Mackey, T. (2011). Legacies of recent environmental change in the benthic communities of Lake Joyce, a perennially ice-covered Antarctic lake. Geobiology 9, 394–410. doi: 10.1111/j.1472-4669.2011.00289.x
Hawes, I., Sumner, D. Y., Andersen, D. T., Jungblut, A. D., and Mackey, T. J. (2013). Timescales of growth response of microbial mats to environmental change in an ice-covered Antarctic lake. Biology 2, 151–176. doi: 10.3390/biology2010151
Hofmann, H., Grey, K., Hickman, A., and Thorpe, R. (1999). Origin of 3.45 Ga coniform stromatolites in Warrawoona group, Western Australia. Geol. Soc. Am. Bull. 111, 1256–1262. doi: 10.1130/0016-7606(1999)111<1256:OOGCSI>2.3.CO;2
Hu, J., Nie, Y., Chen, J., Zhang, Y., Wang, Z., Fan, Q., et al. (2016). Gradual changes of gut microbiota in weaned miniature piglets. Front. Microbiol. 7:1727. doi: 10.3389/fmicb.2016.01727
Jungblut, A. D., Hawes, I., Mackey, T. J., Krusor, M., Doran, P. T., Sumner, D. Y., et al. (2016). Microbial mat communities along an oxygen gradient in a perennially ice-covered Antarctic lake. Appl. Environ. Microbiol. 82, 620–630. doi: 10.1128/AEM.02699-15
Jungblut, A. D., Lovejoy, C., and Vincent, W. F. (2010). Global distribution of cyanobacterial ecotypes in the cold biosphere. ISME J. 4, 191–202. doi: 10.1038/ismej.2009.113
Kanehisa, M., and Goto, S. (2000). KEGG: kyoto encyclopedia of genes and genomes. Nucleic Acids Res. 28, 27–30. doi: 10.1093/nar/28.1.27
Kanehisa, M., Goto, S., Sato, Y., Kawashima, M., Furumichi, M., and Tanabe, M. (2014). Data, information, knowledge and principle: back to metabolism in KEGG. Nucleic Acids Res. 42, D199–D205. doi: 10.1093/nar/gkt1076
Komárek, J. (2007). Phenotype diversity of the cyanobacterial genus Leptolyngbya in the maritime Antarctic. Polish Polar Res. 28, 211–231.
Koo, H., Mojib, N., Huang, J. P., Donahoe, R. J., and Bej, A. K. (2014a). Bacterial community shift in the coastal Gulf of Mexico salt-marsh sediment microcosm in vitro following exposure to the Mississippi Canyon Block 252 oil (MC252). 3 Biotech 5, 379–392. doi: 10.1007/s13205-014-0233-x
Koo, H., Mojib, N., Thacker, R. W., and Bej, A. K. (2014b). Comparative analysis of bacterial community-metagenomics in coastal Gulf of Mexico sediment microcosms following exposure to Macondo oil (MC252). Antonie Van Leeuwenhoek 106, 993–1009. doi: 10.1007/s10482-014-0268-3
Kühl, M., and Fenchel, T. (2000). Bio-optical characteristics and the vertical distribution of photosynthetic pigments and photosynthesis in an artificial cyanobacterial mat. Microb. Ecol. 40, 94–103. doi: 10.1007/s002480000061
Kunin, V., Engelbrektson, A., Ochman, H., and Hugenholtz, P. (2010). Wrinkles in the rare biosphere: pyrosequencing errors can lead to artificial inflation of diversity estimates. Environ. Microbiol. 12, 118–123. doi: 10.1111/j.1462-2920.2009.02051.x
Lane, D. J., Pace, B., Olsen, G. J., Stahl, D. A., Sogin, M. L., and Pace, N. R. (1985). Rapid determination of 16S ribosomal RNA sequences for phylogenetic analyses. Proc. Natl. Acad. Sci. U.S.A. 82, 6955–6959. doi: 10.1073/pnas.82.20.6955
Langille, M. G., Zaneveld, J., Caporaso, J. G., McDonald, D., Knights, D., Reyes, J. A., et al. (2013). Predictive functional profiling of microbial communities using 16S rRNA marker gene sequences. Nat. Biotechnol. 31, 814–821. doi: 10.1038/nbt.2676
Larose, C., Prestat, E., Cecillon, S., Berger, S., Malandain, C., Lyon, D., et al. (2013). Interactions between snow chemistry, mercury inputs and microbial population dynamics in an arctic snowpack. PLoS ONE 8:e79972. doi: 10.1371/journal.pone.0079972
Lauro, F. M., DeMaere, M. Z., Yau, S., Brown, M. V., Ng, C., Wilkins, D., et al. (2011). An integrative study of a meromictic lake ecosystem in Antarctica. ISME J. 5, 879–895. doi: 10.1038/ismej.2010.185
Laval, B., Cady, S. L., Pollack, J. C., McKay, C. P., Bird, J. S., Grotzinger, J. P., et al. (2000). Modern freshwater microbialite analogues for ancient dendritic reef structures. Nature 407, 626–629. doi: 10.1038/35036579
Laybourn-Parry, J., and Pearce, D. A. (2007). The biodiversity and ecology of Antarctic lakes: models for evolution. Philos. Trans. R. Soc. Lond. B 362, 2273–2289. doi: 10.1098/rstb.2006.1945
Laybourn-Parry, J., and Wadham, J. L. (2014). Antarctic Lakes. Oxford: Oxford University Press. doi: 10.1093/acprof:oso/9780199670499.001.0001
Li, L.-G., Cai, L., Zhang, X.-X., and Zhang, T. (2014). Potentially novel copper resistance genes in copper-enriched activated sludge revealed by metagenomic analysis. Appl. Microbiol. Biotechnol. 98, 10255–10266. doi: 10.1007/s00253-014-5939-5
Lokesh, J., and Kiron, V. (2016). Transition from freshwater to seawater reshapes the skin-associated microbiota of Atlantic salmon. Sci. Rep. 6:19707. doi: 10.1038/srep19707
Loopmann, A., Kaup, E., Klokov, V., Simonov, I., and Haendel, D. (1988). “The bathymetry of some lakes of the Antarctic oases Schirmacher and Untersee,” in Limnological Studies in Queen Maud Land, ed. J. Martin (Tallinn: Eesti NSV Teaduste Akadeemia), 6–14.
Lopes, L. D., e Silva, M. D. C. P., and Andreote, F. D. (2016). Bacterial abilities and adaptation toward the rhizosphere colonization. Front. Microbiol. 7:1341. doi: 10.3389/fmicb.2016.01341
Louyakis, A. S., Mobberley, J. M., Vitek, B. E., Visscher, P. T., Hagan, P. D., Reid, R. P., et al. (2017). A study of the microbial spatial heterogeneity of Bahamian Thrombolites using molecular, biochemical, and stable isotope analyses. Astrobiology 17, 413–430. doi: 10.1089/ast.2016.1563
Mackey, T., Sumner, D., Hawes, I., Jungblut, A., and Andersen, D. (2015). Growth of modern branched columnar stromatolites in Lake Joyce, Antarctica. Geobiology 13, 373–390. doi: 10.1111/gbi.12138
Madigan, M. T., Martinko, J. M., Dunlap, P. V., and Clark, D. P. (2008). Brock biology of microorganisms. Int. Microbiol. 11, 65–73.
Marshall, K. (1989). “Cyanobacterial-heterotrophic bacterial interaction,” in Microbial Mats: Physiological Ecology of Benthic Microbial Communities, eds Y. Cohen and E. Rosenberg (Washington, DC: American Society for Microbiology), 239–245.
Mason, O. U., Hazen, T. C., Borglin, S., Chain, P. S., Dubinsky, E. A., Fortney, J. L., et al. (2012). Metagenome, metatranscriptome and single-cell sequencing reveal microbial response to Deepwater Horizon oil spill. ISME J. 6, 1715–1727. doi: 10.1038/ismej.2012.59
McDonald, D., Price, M. N., Goodrich, J., Nawrocki, E. P., DeSantis, T. Z., Probst, A., et al. (2012). An improved greengenes taxonomy with explicit ranks for ecological and evolutionary analyses of bacteria and archaea. ISME J. 6, 610–618. doi: 10.1038/ismej.2011
Menke, S., Wasimuddin, M. M., Melzheimer, J., Mfune, J. K., Heinrich, S., Thalwitzer, S., et al. (2014). Oligotyping reveals differences between gut microbiomes of free-ranging sympatric Namibian carnivores (Acinonyx jubatus, Canis mesomelas) on a bacterial species-like level. Front. Microbiol. 5:526. doi: 10.3389/fmicb.2014.00526
Mobberley, J. M., Khodadad, C. L., and Foster, J. S. (2013). Metabolic potential of lithifying cyanobacteria-dominated thrombolitic mats. Photosynth. Res. 118, 125–140. doi: 10.1007/s11120-013-9890-6
Mobberley, J. M., Ortega, M. C., and Foster, J. S. (2012). Comparative microbial diversity analyses of modern marine thrombolitic mats by barcoded pyrosequencing. Environ. Microbiol. 14, 82–100. doi: 10.1111/j.1462-2920.2011.02509.x
Mojib, N., Huang, J., Hoover, R. B., Pikuta, E. V., Storrie-Lombardi, M., Sattler, B., et al. (2009). “Diversity of bacterial communities in the lakes of Schirmacher Oasis, Antarctica. Paper Presented at: SPIE Optical Engineering+ Applications, (Bellingham, WA: SPIE).
Mosier, A. C., Murray, A. E., and Fritsen, C. H. (2007). Microbiota within the perennial ice cover of Lake Vida, Antarctica. FEMS Microbiol. Ecol. 59, 274–288. doi: 10.1111/j.1574-6941.2006.00220.x
Muyzer, G., de Waal, E. C., and Uitterlinden, A. G. (1993). Profiling of complex microbial populations by denaturing gradient gel electrophoresis analysis of polymerase chain reaction-amplified genes coding for 16S rRNA. Appl. Environ. Microbiol. 59, 695–700.
Myshrall, K., Mobberley, J., Green, S., Visscher, P., Havemann, S., Reid, R., et al. (2010). Biogeochemical cycling and microbial diversity in the thrombolitic microbialites of Highborne Cay, Bahamas. Geobiology 8, 337–354. doi: 10.1111/j.1472-4669.2010.00245.x
Newton, R. J., Jones, S. E., Eiler, A., McMahon, K. D., and Bertilsson, S. (2011). A guide to the natural history of freshwater lake bacteria. MIcrobiol. Mol. Biol. Rev. 75, 14–49. doi: 10.1128/MMBR.00028-10
Nübel, U., Garcia-Pichel, F., and Muyzer, G. (1997). PCR primers to amplify 16S rRNA genes from cyanobacteria. Appl. Environ. Microbiol. 63, 3327–3332.
Oguni, A., and Takahashi, E. (1989). “Floristic studies on algae from inland waters of Antarctica: II. Lake O-ike, West Ongul Island,” in Proceedings of the NIPR Symposium on Polar Biology, Vol. 2 (Tokyo: National Institute of Polar Research), 154–166.
Paerl, H. W., and Pinckney, J. (1996). A mini-review of microbial consortia: their roles in aquatic production and biogeochemical cycling. Microb. Ecol. 31, 225–247. doi: 10.1007/BF00171569
Parks, D. H., Tyson, G. W., Hugenholtz, P., and Beiko, R. G. (2014). STAMP: statistical analysis of taxonomic and functional profiles. Bioinformatics 30, 3123–3124. doi: 10.1093/bioinformatics/btu494
Philosof, A., Sabehi, G., and Béjà, O. (2009). Comparative analyses of actinobacterial genomic fragments from Lake Kinneret. Environ. Microbiol. 11, 3189–3200. doi: 10.1111/j.1462-2920.2009.02024.x
Pope, P. B., Mackenzie, A. K., Gregor, I., Smith, W., Sundset, M. A., McHardy, A. C., et al. (2012). Metagenomics of the svalbard reindeer rumen microbiome reveals abundance of polysaccharide utilization loci. PLoS ONE 7:e38571. doi: 10.1371/journal.pone.0038571
Power, I., Wilson, S., Dipple, G., and Southam, G. (2011). Modern carbonate microbialites from an asbestos open pit pond, Yukon, Canada. Geobiology 9, 180–195. doi: 10.1111/j.1472-4669.2010.00265.x
Priscu, J., and Foreman, C. (2009). “Lakes of Antarctica,” in Encyclopedia of Inland Waters, Vol. 2, ed. G. E. Likens (Oxford: Elsevier Press), 555–566. doi: 10.1016/b978-012370626-3.00038-7
Quesada, A., Fernández-Valiente, E., Hawes, I., and Howard-Williams, C. (2008). “Benthic primary production in polar lakes and rivers,” in Polar Lakes and Rivers – Arctic and Antarctic Aquatic Ecosystems, eds W. F. Vincent and J. Laybourn-Parry (Oxford: Oxford University Press), 179–196. doi: 10.1093/acprof:oso/9780199213887.003.0010
Reid, R. P., Visscher, P. T., Decho, A. W., Stolz, J. F., Bebout, B., Dupraz, C., et al. (2000). The role of microbes in accretion, lamination and early lithification of modern marine stromatolites. Nature 406, 989–992. doi: 10.1038/35023158
Rondon, M. R., August, P. R., Bettermann, A. D., Brady, S. F., Grossman, T. H., Liles, M. R., et al. (2000). Cloning the soil metagenome: a strategy for accessing the genetic and functional diversity of uncultured microorganisms. Appl. Environ. Microbiol. 66, 2541–2547. doi: 10.1128/AEM.66.6.2541-2547.2000
Schmidt, V. T., Reveillaud, J., Zettler, E., Mincer, T. J., Murphy, L., and Amaral-Zettler, L. A. (2014). Oligotyping reveals community level habitat selection within the genus Vibrio. Front. Microbiol. 5:563. doi: 10.3389/fmicb.2014.00563
Schmieder, R., and Edwards, R. (2011). Quality control and preprocessing of metagenomic datasets. Bioinformatics 27, 863–864. doi: 10.1093/bioinformatics/btr026
Seckbach, J., and Oren, A. (2010). Microbial Mats: Modern and Ancient Microorganisms in Stratified Systems. Berlin: Springer Science & Business Media. doi: 10.1007/978-90-481-3799-2
Sforna, M. C., Philippot, P., Somogyi, A., van Zuilen, M. A., Medjoubi, K., Schoepp-Cothenet, B., et al. (2014). Evidence for arsenic metabolism and cycling by microorganisms 2.7 billion years ago. Nat. Geosci. 7, 811–815. doi: 10.1038/ngeo2276
Shannon, C. E., Weaver, W., Blahut, R. E., and Hajek, B. (1964). The Mathematical Theory of Communication. Urbana, IL: University of Illinois Press.
Shannon, P., Markiel, A., Ozier, O., Baliga, N. S., Wang, J. T., Ramage, D., et al. (2003). Cytoscape: a software environment for integrated models of biomolecular interaction networks. Genome. Res. 13, 2498–2504. doi: 10.1101/gr.1239303
Smith, D. M., Snow, D. E., Rees, E., Zischkau, A. M., Hanson, J. D., Wolcott, R. D., et al. (2010). Evaluation of the bacterial diversity of pressure ulcers using bTEFAP pyrosequencing. BMC Med. Genomics 3:41. doi: 10.1186/1755-8794-3-41
Stahl, D. (1995). Application of phylogenetically based hybridization probes to microbial ecology. Mol. Ecol. 4, 535–542. doi: 10.1111/j.1365-294X.1995.tb00254.x
Staley, C., Gould, T. J., Wang, P., Phillips, J., Cotner, J. B., and Sadowsky, M. J. (2014). Core functional traits of bacterial communities in the Upper Mississippi River show limited variation in response to land cover. Front. Microbiol. 5:414. doi: 10.3389/fmicb.2014.00414
Suchodolski, J. S., Dowd, S. E., Westermarck, E., Steiner, J. M., Wolcott, R. D., Spillmann, T., et al. (2009). The effect of the macrolide antibiotic tylosin on microbial diversity in the canine small intestine as demonstrated by massive parallel 16S rRNA gene sequencing. BMC Microbiol. 9:210. doi: 10.1186/1471-2180-9-210
Sutherland, D. L., and Hawes, I. (2009). Annual growth layers as proxies of past growth conditions for benthic microbial mats in a perennially ice-covered Antarctic lake. FEMS Microbiol. Ecol. 67, 279–292. doi: 10.1111/j.1574-6941.2008.00621.x
Taton, A., Grubisic, S., Brambilla, E., De Wit, R., and Wilmotte, A. (2003). Cyanobacterial diversity in natural and artificial microbial mats of Lake Fryxell (McMurdo Dry Valleys, Antarctica): a morphological and molecular approach. Appl. Environ. Microbiol. 69, 5157–5169. doi: 10.1128/AEM.69.9.5157-5169.2003
Taton, A., Grubisic, S., Ertz, D., Hodgson, D. A., Piccardi, R., Biondi, N., et al. (2006). Polyphasic study of antarctic cyanobacterial strains. J. Phycol. 42, 1257–1270. doi: 10.1111/j.1529-8817.2006.00278.x
Thurman, J., Parry, J., Hill, P. J., Priscu, J. C., Vick, T. J., Chiuchiolo, A., et al. (2012). Microbial dynamics and flagellate grazing during transition to winter in Lakes Hoare and Bonney, Antarctica. FEMS Microbiol. Ecol. 82, 449–458. doi: 10.1111/j.1574-6941.2012.01423.x
Van Goethem, M. W., Makhalanyane, T. P., Valverde, A., Cary, S. C., and Cowan, D. A. (2016). Characterization of bacterial communities in lithobionts and soil niches from Victoria Valley, Antarctica. FEMS Microbiol. Ecol. 92:fiw051. doi: 10.1093/femsec/fiw051
Varin, T., Lovejoy, C., Jungblut, A. D., Vincent, W. F., and Corbeil, J. (2012). Metagenomic analysis of stress genes in microbial mat communities from Antarctica and the High Arctic. Appl. Environ. Microbiol. 78, 549–559. doi: 10.1128/AEM.06354-11
Ventura, M., Canchaya, C., Tauch, A., Chandra, G., Fitzgerald, G. F., Chater, K. F., et al. (2007). Genomics of Actinobacteria: tracing the evolutionary history of an ancient phylum. Microbiol. Mol. Biol. Rev. 71, 495–548. doi: 10.1128/MMBR.00005-07
Vick-Majors, T. J., Priscu, J. C., and Amaral-Zettler, L. A. (2014). Modular community structure suggests metabolic plasticity during the transition to polar night in ice-covered Antarctic lakes. ISME J. 8, 778–789. doi: 10.1038/ismej.2013.190
Vikram, S., Guerrero, L. D., Makhalanyane, T. P., Le, P. T., Seely, M., and Cowan, D. A. (2016). Metagenomic analysis provides insights into functional capacity in a hyperarid desert soil niche community. Environ. Microbiol. 18, 1875–1888. doi: 10.1111/1462-2920.13088
Vincent, W. F., and Quesada, A. (2012). “Cyanobacteria in high latitude lakes, rivers and seas,” in Ecology of Cyanobacteria II: Their Diversity in Space and Time, ed. B. A. Whitton (New York, NY: Springer), 371–385. doi: 10.1007/978-94-007-3855-3_13
Wand, U., Samarkin, V. A., Nitzsche, H.-M., and Hubberten, H.-W. (2006). Biogeochemistry of methane in the permanently ice-covered Lake Untersee, central Dronning Maud Land, East Antarctica. Limnol. Oceanogr. 51, 1180–1194. doi: 10.4319/lo.2006.51.2.1180
Wand, U., Schwarz, G., Brüggemann, E., and Bräuer, K. (1997). Evidence for physical and chemical stratification in Lake Untersee (central Dronning Maud Land, East Antarctica). Antarct. Sci. 9, 43–45. doi: 10.1017/S0954102097000060
Wang, Q., Garrity, G. M., Tiedje, J. M., and Cole, J. R. (2007). Naive Bayesian classifier for rapid assignment of rRNA sequences into the new bacterial taxonomy. Appl. Environ. Microbiol. 73, 5261–5267. doi: 10.1128/AEM.00062-07
Ward, A. C., and Bora, N. (2006). Diversity and biogeography of marine actinobacteria. Curr. Opin. Microbiol. 9, 279–286. doi: 10.1016/j.mib.2006.04.004
Warnecke, F., Sommaruga, R., Sekar, R., Hofer, J. S., and Pernthaler, J. (2005). Abundances, identity, and growth state of actinobacteria in mountain lakes of different UV transparency. Appl. Environ. Microbiol. 71, 5551–5559. doi: 10.1128/AEM.71.9.5551-5559.2005
Webb, J. S., Givskov, M., and Kjelleberg, S. (2003). Bacterial biofilms: prokaryotic adventures in multicellularity. Curr. Opin. Microbiol. 6, 578–585. doi: 10.1016/j.mib.2003.10.014
White, R. A. III. (2014). Metagenomic and Genomic Analyses of Modern Freshwater Microbialites: Unmasking a Community of Complex Metabolic Potential. Ph.D. thesis, Vancouver, BC: University of British Columbia.
White, R. A. III, Chan, A. M., Gavelis, G. S., Leander, B. S., Brady, A., Slater, G. F., et al. (2015). Metagenomic analysis suggests modern freshwater microbialites harbor a core and distinct microbial community. Front. Microbiol. 6:1531. doi: 10.3389/fmicb.2015.01531
Wilhelm, R. C., Niederberger, T. D., Greer, C., and Whyte, L. G. (2011). Microbial diversity of active layer and permafrost in an acidic wetland from the Canadian High Arctic. Can. J. MIcrobiol. 57, 303–315. doi: 10.1139/w11-004
Yuan, Z., Druzhinina, I. S., Labbé, J., Redman, R., Qin, Y., Rodriguez, R., et al. (2016). Specialized microbiome of a halophyte and its role in helping non-host plants to withstand salinity. Sci. Rep. 6:32467. doi: 10.1038/srep32467
Zeng, B., Han, S., Wang, P., Wen, B., Jian, W., Guo, W., et al. (2015). The bacterial communities associated with fecal types and body weight of rex rabbits. Sci. Rep. 5:9342. doi: 10.1038/srep09342
Zhang, L., Jungblut, A., Hawes, I., Andersen, D., Sumner, D., and Mackey, T. (2015). Cyanobacterial diversity in benthic mats of the McMurdo Dry Valley lakes, Antarctica. Polar Biol. 38, 1097–1110. doi: 10.1007/s00300-015-1669-0
Keywords: East Antarctica, cyanobacteria, heterotrophic bacteria, mat lamina, QIIME, PICRUSt
Citation: Koo H, Mojib N, Hakim JA, Hawes I, Tanabe Y, Andersen DT and Bej AK (2017) Microbial Communities and Their Predicted Metabolic Functions in Growth Laminae of a Unique Large Conical Mat from Lake Untersee, East Antarctica. Front. Microbiol. 8:1347. doi: 10.3389/fmicb.2017.01347
Received: 01 February 2017; Accepted: 03 July 2017;
Published: 04 August 2017.
Edited by:
Virginia Helena Albarracín, Center for Electron Microscopy (CIME), ArgentinaReviewed by:
Antonio Quesada, Universidad Autonoma de Madrid, SpainMaria Cecilia Rasuk, PROIMI-CONICET, Argentina
Copyright © 2017 Koo, Mojib, Hakim, Hawes, Tanabe, Andersen and Bej. This is an open-access article distributed under the terms of the Creative Commons Attribution License (CC BY). The use, distribution or reproduction in other forums is permitted, provided the original author(s) or licensor are credited and that the original publication in this journal is cited, in accordance with accepted academic practice. No use, distribution or reproduction is permitted which does not comply with these terms.
*Correspondence: Asim K. Bej, YWJlakB1YWIuZWR1 Dale T. Andersen, ZGFuZGVyc2VuQHNldGkub3Jn
†Present address: Nazia Mojib, School of Biological Sciences, Georgia Institute of Technology, Atlanta, GA, United States