- Department of Biochemistry, University of Otago, Dunedin, New Zealand
Oomycetes in the genus Phytophthora are among the most damaging plant pathogens worldwide. Two important species are Phytophthora cinnamomi, which causes root rot in thousands of native and agricultural plants, and Phytophthora agathidicida, which causes kauri dieback disease in New Zealand. As is the case for other Phytophthora species, management options for these two pathogens are limited. Here, we have screened over 100 compounds for their anti-oomycete activity, as a potential first step toward identifying new control strategies. Our screening identified eight compounds that showed activity against both Phytophthora species. These included five antibiotics, two copper compounds and a quaternary ammonium cation. These compounds were tested for their inhibitory action against three stages of the Phytophthora life cycle: mycelial growth, zoospore germination, and zoospore motility. The inhibitory effects of the compounds were broadly similar between the two Phytophthora species, but their effectiveness varied widely among life cycle stages. Mycelial growth was most successfully inhibited by the antibiotics chlortetracycline and paromomycin, and the quaternary ammonium salt benzethonium chloride. Copper chloride and copper sulfate were most effective at inhibiting zoospore germination and motility, whereas the five antibiotics showed relatively poor zoospore inhibition. Benzethonium chloride was identified as a promising antimicrobial, as it is effective across all three life cycle stages. While further testing is required to determine their efficacy and potential phytotoxicity in planta, we have provided new data on those agents that are, and those that are not, effective against P. agathidicida and P. cinnamomi. Additionally, we present here the first published protocol for producing zoospores from P. agathidicida, which will aid in the further study of this emerging pathogen.
Introduction
Species in the genus Phytophthora (from the Greek for “plant-destroyers”) are among the most serious threats to native plants and horticultural species alike, with a global economic impact estimated in the billions of dollars per annum (Erwin and Ribeiro, 1996). While they superficially resemble filamentous fungi, Phytophthora are actually oomycetes and therefore more closely related to diatoms and brown algae in the stramenopiles (Gunderson et al., 1987; Thines, 2014). Phytophthora live in plant tissues, soil, or water, and under favorable conditions of temperature, moisture, and nutrition produce sporangia, followed by the release of motile zoospores, allowing infection to spread rapidly among host plants (Judelson and Blanco, 2005).
Two species of particular importance in New Zealand are Phytophthora agathidicida and Phytophthora cinnamomi. P. agathidicida (formerly Phytophthora taxon agathis or PTA) is a root and collar/stem canker pathogen responsible for dieback in kauri (Agathis australis), an iconic tree that is native to New Zealand (Beever et al., 2009; Weir et al., 2015). Kauri is a keystone species that has a profound influence on the surrounding soil, canopy, and biodiversity (Ecroyd, 1982; Wyse et al., 2014). Kauri dieback was first recognized over 40 years ago (Gadgil, 1974) and is continuing to spread through the forests of northern New Zealand, rapidly killing trees of all ages and sizes (Beever et al., 2009). Currently, there are no established treatment or control options for P. agathidicida, although the use of phosphite as a potential treatment for infected trees is being explored (Horner and Hough, 2013; Horner et al., 2015).
Phytophthora cinnamomi is a generalist pathogen capable of infecting thousands of different host plants in wildlands, horticulture and commercial nurseries, resulting in root rot and often death of the host plant (Erwin and Ribeiro, 1996). In New Zealand, the most economically important host is avocado (Persea americana). Globally, its principal food crop hosts are avocado and pineapple, but it is also an important pathogen of ecologically significant native plants throughout the temperate regions of the world. Some of the greatest plant losses are in areas with a Mediterranean climate including parts of the United States, Australia, Mexico, and the Iberian Peninsula (Burgess et al., 2017). It also infects a range of ornamental trees and shrubs and is readily spread through the movement and out-planting of nursery stock (Erwin and Ribeiro, 1996). Disease caused by P. cinnamomi has significant economic impacts on forestry and horticulture; in California alone, the annual loss from P. cinnamomi infections in avocado groves is US$30 million (Erwin and Ribeiro, 1996). P. cinnamomi has been called the “biological bulldozer” for its capacity to destroy plants (Carter, 2004), and it was recently identified as one of the top 10 oomycete pathogens, based on its scientific and economic importance (Kamoun et al., 2015).
Exacerbating the effects of Phytophthora infection is the fact that these species are notoriously difficult to control. While Phytophthora share many traits with the true fungi, they are phylogenetically distinct enough to lack many of the canonical molecular targets of labeled fungicides (Rolando et al., 2016). For example, Phytophthora species lack the biosynthetic pathways for ergosterol and a chitin-based cell wall. The development of resistance is also an ongoing problem (Cohen and Coffey, 1986; Parra and Ristaino, 2001; Gisi and Sierotzki, 2008; Miao et al., 2016). Once an area becomes infected, eradication can require chemical treatment, physical barriers to prevent disease spread, and destruction of host plants (Dunstan et al., 2010). Environmental toxicity of chemical treatments and the logistics of application further complicate disease management and eradication efforts.
In this study, we have sought to identify anti-oomycete compounds that inhibit mycelial growth, zoospore germination, and/or zoospore motility in P. agathidicida and P. cinnamomi, as a first step toward development of more effective control agents. By using phenotype microarray (PM) plates (Bochner et al., 2001) as sources of potential antimicrobials, we screened 120 known antimicrobial chemicals in a high-throughput fashion. Here, we explore the chemical susceptibility of the emerging pathogen, P. agathidicida, and compare its susceptibility profile to these chemicals with the more well-characterized species, P. cinnamomi.
Materials and Methods
Routine Culturing
Phytophthora agathidicida isolate NZFS 3770 and P. cinnamomi isolate NZFS 3910 were sourced from Scion (Rotorua, New Zealand). The two species were routinely cultured at 22°C in darkness on clarified 20% V8-juice agar (V8A). For antibiotic susceptibility testing using PM plates (Biolog Inc, CA, United States), isolates were cultured on potato-dextrose agar (PDA; P. agathidicida) or cornmeal agar (CA; P. cinnamomi) (Becton, Dickinson & Co, NJ, United States). EC50 assays were carried out using CA for both species.
Anti-oomycete Compound Screening
The activities of potential anti-oomycete compounds were tested using a modified version of the Kirby–Bauer disk diffusion assay (Bauer et al., 1966). Test compounds were prepared by adding 20 μL of sterile water to each well of a PM plate. Each plate (PM 21D, 22D, 23A, 24C, and 25D) contains 24 different compounds at four different concentrations. Five microliters of each resuspended compound were added to sterile 6 mm filter disks (Whatman number 1 paper). After sufficient drying, the four filter disks containing a single test compound at different concentrations were placed at regular intervals around the edges of a PDA or CA plate, along with a control disk (sterile water). Each plate was then inoculated by placing a 3 mm agar plug of mycelium (taken from the leading edge of an actively growing mycelial mat) in the center. Each plate was incubated at 20°C in the dark and radial growth was measured after 5 days (P. cinnamomi) or 7 days (P. agathidicida). Three biological replicates (from independently grown cultures) were performed for each compound screened. Any compound that reproducibly gave zones of inhibition that were greater than the control disk and were dose-dependent was scored as a positive.
Determination of EC50 Values for Inhibition of Mycelial Growth
The EC50 values for radial growth inhibition were determined for each compound that inhibited Phytophthora growth in the disk diffusion assays. CA was amended with eight twofold dilutions of the compound of interest, with concentrations ranging from 0.125 to 512 μg/ml. Concentration ranges were chosen based on preliminary growth inhibition assays and differed among compounds. Control plates (CA only) were included for each compound tested, and three biological replicates were performed for each compound and concentration. Each plate was inoculated by placing a 3 mm agar plug of mycelium (taken from the edge of an actively growing mycelial mat) in its center; plates were then incubated at 20°C for 4 days (P. cinnamomi) or 6 days (P. agathidicida). Two perpendicular measurements were taken for each plate and averaged, and the size of the agar plug was subtracted to obtain the final measurement of radial growth. Measurements from test compound plates were subtracted from control plate measurements and converted to inhibition percentages. To estimate EC50 values, compound concentrations were log-transformed, and non-linear regression with curve fitting (by least squares) was carried out using GraphPad Prism version 6.0. All chemicals were purchased from Sigma-Aldrich (MO, United States) except for copper sulfate pentahydrate (Ajax Chemicals, Sydney, Australia) and kanamycin sulfate (Applichem GmbH, Darmstadt, Germany).
Zoospore Germination and Motility
In order to produce sporangia in P. cinnamomi and P. agathidicida, approximately 2 cm2 of agar from the edge of an actively growing mycelial mat was excised and transferred to a Petri dish containing a 1:10 dilution of carrot broth (P. agathidicida) or clarified 20% V8 broth (P. cinnamomi), cut into ∼1 mm2 pieces and then incubated at 25°C in darkness for 24 h. The following day, the broth was removed and each dish was washed four times in sterile Chen–Zentmyer salt solution (for P. cinnamomi; Chen and Zentmyer, 1970) or sterile pond water (for P. agathidicida) for 45 min at a time on the bench (P. agathidicida) or on a rocking table (P. cinnamomi), followed by overnight incubation (without rocking) at 22°C under light. Following sporangium production, zoospore release was induced by removing the liquid and washing each dish three times with sterile Milli-Q water that had been cooled to 4°C. Each wash was for 20 min, with the first two at room temperature and the final wash at 4°C. Wash volumes were 15 ml per dish for the first two washes, and 10 ml for the final wash. Following the final wash, dishes were returned to room temperature for 30–90 min until sufficient numbers (a final concentration of approximately 1 × 103 zoospores/ml) of zoospores had been released.
To assess the effect of each putative anti-oomycete compound on zoospore germination, 200 μl of zoospore suspension were spread on triplicate water agar plates amended with the test compounds. Concentrations of the compounds were as for the mycelial growth inhibition assays, and ranged from 0.125 to 512 μg/ml. Plates were incubated for 12–16 h, and 100 zoospores per plate were counted under a Nikon C-DS dissecting microscope at 40× magnification to determine the percentage that had germinated. Spores were defined as having germinated if the germ tube length was at least twice as long as the spore diameter, as described previously (Liu et al., 2014). The level of germination inhibition was calculated by subtracting the percent germinated at each concentration from that of three control (water agar only) plates.
Zoospore motility assays were based on a method described previously (Hu et al., 2007). One milliliter of zoospore suspension (approximately 1,000 zoospores) was added to triplicate wells in a 24-well plate containing 10 μl of the test compound solution at different concentrations, resulting in five twofold dilutions of the test compound. Control wells were amended with an equal volume of sterile water. Zoospores were kept at room temperature, and observed with the dissecting microscope to determine the time required for all zoospore motility to stop. Observations were made at 5 min intervals for the first hour, then at 30 min intervals until motility had ceased. Wells that had no motile zoospores by the time of the first observation (5 min post-treatment) were scored as 0 (i.e., complete inhibition). Subsequent loss of motility was calculated by dividing the length of time zoospores remained motile by the average motility time in the controls.
Results
High-Throughput Screening for Anti-oomycete Compounds
The activities of potential anti-oomycete compounds were tested using a disk diffusion assay (Bauer et al., 1966), where the zone of inhibition of mycelial growth around filter disks containing the test compounds was assessed (Figure 1). Each species was screened against a panel of Biolog PM plates. In total, the panel of five PM plates contained 120 potential antimicrobial compounds, with each compound present at four concentrations. The panel contained common anti-yeast and antifungal antibiotics, as well as other types of chemicals with antimicrobial activity such as metals, detergents, and inhibitors. The complete list of compounds tested is available in Supplementary Table 1.
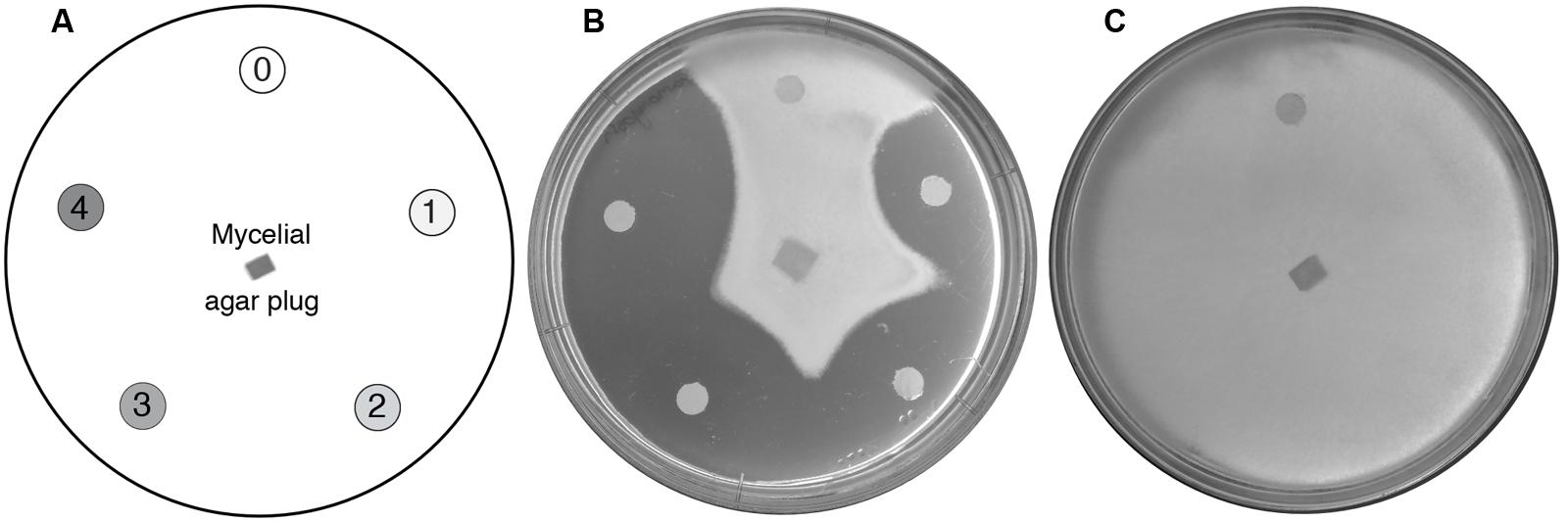
FIGURE 1. High-throughput screening for anti-oomycete compounds. (A) Diagram showing the standard test arrangement. An agar plug of mycelium is used to inoculate an agar plate with disks saturated either with water (control; marked 0) or increasing concentrations of a test compound (in a clockwise direction, marked 1–4). (B) Representative results from a typical experiment. Here, P. agathidicida mycelial growth is inhibited in the presence of increasing concentrations of paromomycin. (C) Control plate showing normal growth of P. agathidicida in the absence of test compounds.
Of the 16 anionic toxins tested, only one (arsenite) had an inhibitory effect. Similarly, only two of the 15 cationic toxins tested, copper (II) chloride and copper (II) sulfate, showed inhibitory effects. None of the chelating agents tested (including dipyridyl, EDTA, and EGTA) showed any inhibitory effects.
Several common antibiotics successfully inhibited growth, particularly at higher concentrations. The majority were aminoglycosides (hygromycin B, kanamycin, neomycin, paromomycin, and tobramycin), which act by inhibiting protein synthesis. Chlortetracycline (a tetracycline antibiotic which also inhibits protein synthesis) and D-cycloserine (an amino acid derivative which inhibits pyridoxal 5′-phosphate dependent enzymes) also inhibited mycelial growth. Of the antibiotics identified here, only neomycin and paromomycin have been previously reported to have anti-oomycete activity against other Phytophthora species (Lee et al., 2005).
In addition to antibiotics, the PM screen included seven common antifungals: three triazoles (fluconazole, myclobutanil, propiconazole), an imidazole (miconazole), a polyene antimycotic (nystatin), a nucleoside analog (5-fluorocytosine) and cycloheximide. None of these showed any inhibitory effects.
Benzethonium chloride, a quaternary ammonium salt often used as a disinfectant, showed strong inhibition of P. agathidicida mycelial growth, and a low level of inhibition against P. cinnamomi.
Thallium (I) acetate and sodium arsenite both showed promising inhibitory effects; however, their non-selective toxicity (Hughes, 2002; Lennartson, 2015) makes them unsuitable for use in the environment so they were not tested further. Similarly, fluorodeoxyuridine (an oncology drug) and trifluoperazine (an antipsychotic) inhibited mycelial growth, but were excluded from further analysis due to their unsuitability for use in the real-world treatment of oomycete infections.
Mycelial Growth Inhibition
Based on the results of our qualitative high-throughput screen, we selected the eight most promising inhibitors for more quantitative testing. To begin, we determined EC50 values for inhibition of mycelial growth. This is important, because mycelial growth assays approximate both systemic growth within the plant, and across root-grafts between plants (i.e., root-to-root contact). Regression analysis resulted in well-supported inhibition curves, with all R2 values >0.9 (Figure 2). The calculated EC50 values for all compounds are presented in Table 1. These values varied widely among the compounds tested, from 0.79 to 180 μg/ml.
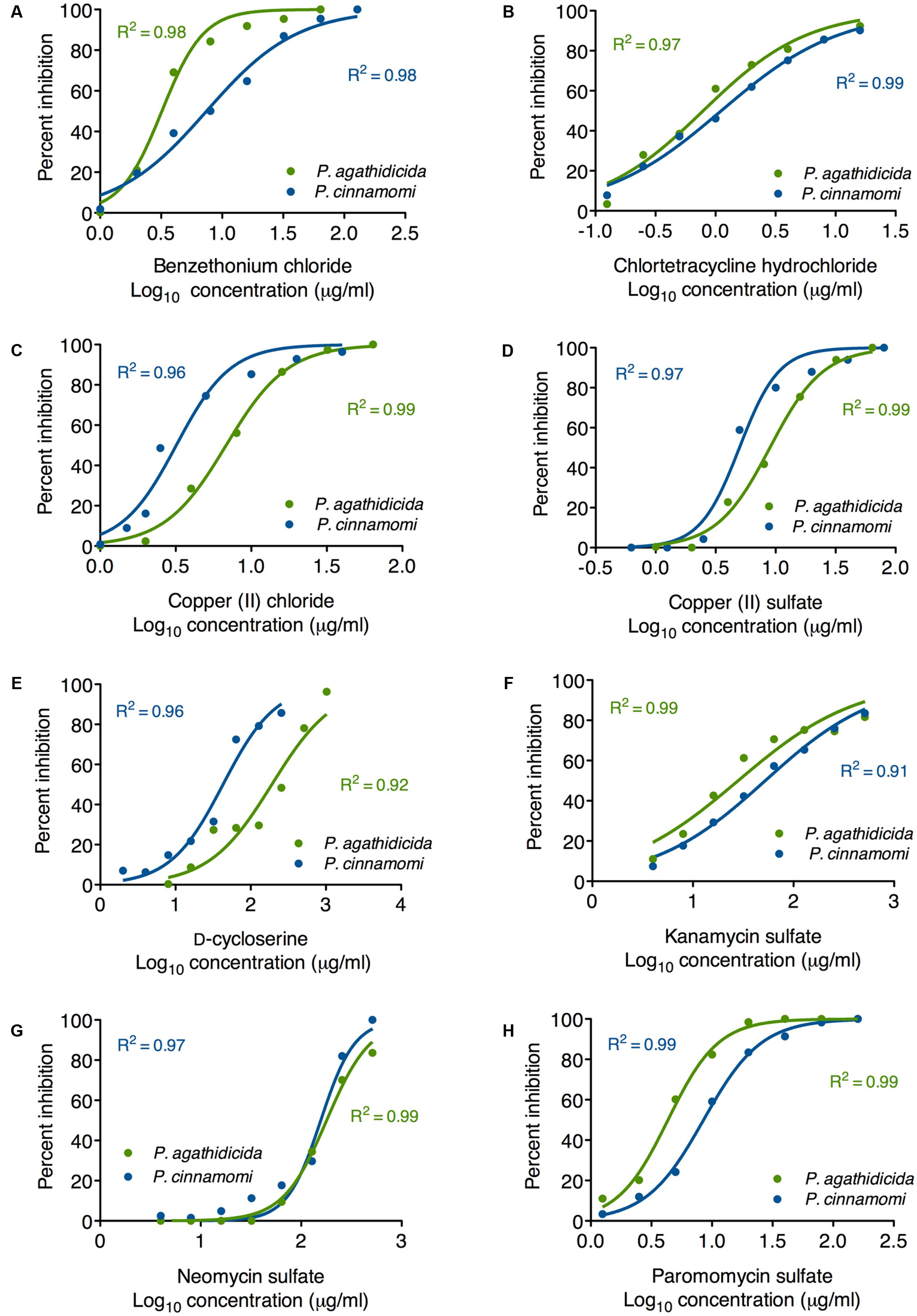
FIGURE 2. Mycelial growth inhibition curves for P. agathidicida (green) and P. cinnamomi (blue). Each panel (A–H) shows the inhibition curves for a single compound, as identified in the label on the x-axis. Data points show the mean of triplicate inhibition assays.
The most effective inhibitor of mycelial growth in both Phytophthora species was chlortetracycline hydrochloride, with EC50 values of 0.79 and 1.1 μg/ml in P. agathidicida and P. cinnamomi, respectively. Benzethonium chloride was also an effective inhibitor, though more so for P. agathidicida, as was seen in the initial high-throughput screen. Both of the copper compounds tested had relatively low EC50 values, and were more effective against P. cinnamomi. EC50 values for the aminoglycoside antibiotics varied widely, with paromomycin by far the most effective. D-cycloserine was a relatively ineffective inhibitor, although it showed the greatest species specificity of any compound tested (almost fivefold more effective against P. cinnamomi than P. agathidicida).
Zoospore Germination Inhibition
In addition to mycelial growth, a useful antimicrobial agent could also inhibit one or more of the other stages in the Phytophthora life cycle (see, for example, Mircetich, 1970 and Judelson and Blanco, 2005). The first step in initiating a new infection is the encystment and germination of a zoospore on the host plant (Judelson and Blanco, 2005). We tested the ability of our eight candidate antimicrobials to inhibit zoospore germination, following a previous protocol (Liu et al., 2014).
As no previous studies have reported laboratory methods for producing P. agathidicida zoospores, several variations on the standard P. cinnamomi protocol were trialed in order to maximize the number of sporangia formed and zoospores released. It was found that excised pieces of mycelial mats grew best when grown in diluted, clarified carrot broth (Erwin and Ribeiro, 1996) rather than V8 broth. Sporangia production was increased when the subsequent washing steps were carried out using sterile (filtered and autoclaved) pond water (pH ∼8.5) rather than the salt solution used for P. cinnamomi. The chemical composition of the pond water used can be found in Supplementary Table 2. Pond water samples from two sources (Rotorua, North Island, New Zealand and Dunedin, South Island, New Zealand) were trialed, with no noticeable differences in sporangium numbers. Adjusting the pH of the pond water up or down, to values between 5 and 10, reduced sporangia production, emphasizing that unadjusted pond water was optimal. As the mycelial mats of P. agathidicida were less adherent than those of P. cinnamomi, carrying out the wash steps on a rocking table tended to dislodge the mats and resulted in decreased numbers of sporangia; therefore wash steps were carried out without rocking. Methods for inducing zoospore release were as for P. cinnamomi, though P. agathidicida often required a longer final incubation step at room temperature before zoospores began to be released.
Germination rates on unamended agar plates ranged from 85 to 98% for P. agathidicida and 57–91% for P. cinnamomi (dashed lines in Figure 3). Germination of P. agathidicida zoospores was inhibited to a greater or lesser extent by five of the eight compounds tested (Figure 3), with the strongest inhibitors being benzethonium chloride (Figure 3A), copper (II) chloride (Figure 3C), and copper (II) sulfate (Figure 3D). All of these compounds inhibited germination completely at concentrations ranging from 10 to 80 μg/ml, with the two copper salts proving the most effective. On the other hand, neomycin and paromomycin only effected partial inhibition of germination at the highest concentrations tested (Figures 3G,H). The other antibiotics (chlortetracycline, D-cycloserine and kanamycin) did not inhibit germination of P. agathidicida zoospores.
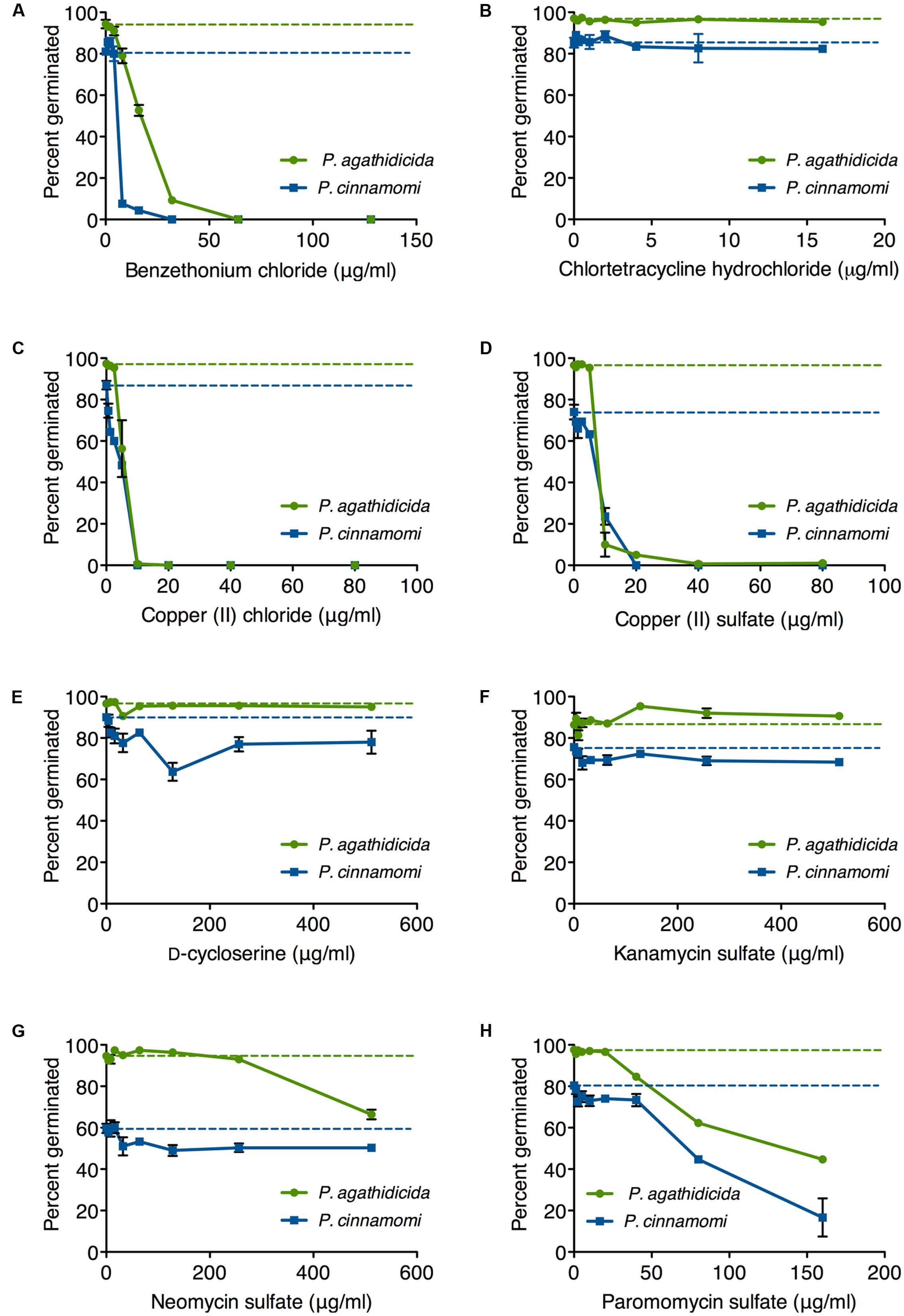
FIGURE 3. Inhibition of zoospore germination in P. agathidicida (green) and P. cinnamomi (blue). Each panel (A–H) shows the inhibition curves for a single compound, as identified in the label on the x-axis. Dashed lines represent the germination rates on unamended agar. Data points are mean values from triplicate germination assays. Error bars are ± standard error of the mean. Where error bars are not visible, they are smaller than the symbol.
The pattern was broadly similar for inhibiting germination of P. cinnamomi zoospores (Figure 3). As with P. agathidicida, the most effective inhibitors were benzethonium chloride (Figure 3A), copper chloride (Figure 3C), and copper sulfate (Figure 3D), all of which prevented germination completely at concentrations between 10 and 80 μg/ml. Paromomycin was the most effective of the antibiotics, although it only reduced the germination rate by fourfold (Figure 3H).
Zoospore Motility Inhibition
A third way of disrupting Phytophthora pathogenicity is to inhibit zoospore motility. Once released from sporangia, zoospores exhibit chemotaxis toward the roots of a new host plant, and, in the case of P. cinnamomi, can swim at approximately 0.5 m/h through water (Allen and Newhook, 1973; Allen and Harvey, 1974). Rendering them immotile would offer a novel containment approach that prevents spread from infected trees.
In control experiments, the untreated zoospores of P. agathidicida and P. cinnamomi remained motile in sterile water for approximately 17 and 20 h on average, respectively. We tested our set of eight anti-oomycete compounds over a range of concentrations. In general, we observed dose dependent responses, with higher compound concentrations leading to faster zoospore encystment. The only exception was D-cycloserine, which had no effect on the motility of P. cinnamomi zoospores at any concentration tested.
In order to quantify the effectiveness of the compounds, we compared the lowest concentration of each that was required to cause complete loss of motility within 5 min of treatment. The concentration required for this rapid inhibition varied widely among compounds (Table 2). Copper chloride, copper sulfate, and benzethonium chloride proved the most effective, with concentrations ≤0.1 μg/ml causing almost immediate loss of motility. At the other extreme, 256 μg/ml D-cycloserine was required to effect such immediate inhibition of P. agathidicida zoospore motility. Similarly, at the highest concentration tested (8 μg/ml), kanamycin failed to inhibit motility within 5 min. Instead, it took 25 min for P. agathidicida zoospores to lose motility at this concentration of kanamycin, and 60 min for P. cinnamomi zoospores. The general trends observed for both Phytophthora species in response to the eight compounds were similar, although P. agathidicida zoospores were over an order of magnitude more sensitive to neomycin than P. cinnamomi (Table 2).
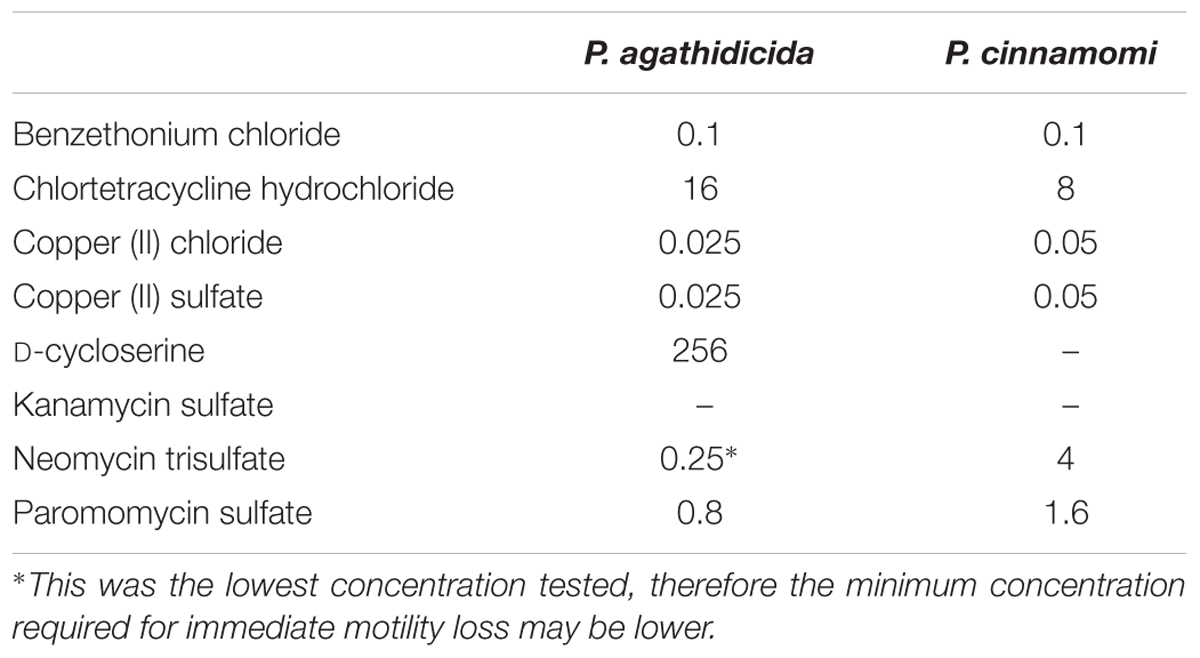
TABLE 2. Minimum concentrations of anti-oomycete compounds (in μg/ml) required to cause zoospores to become immotile within 5 min of treatment.
Discussion
This study provides the first comprehensive screening data on dozens of potential anti-oomycete compounds, for their effectiveness against two species of Phytophthora. In the case of P. cinnamomi, we have extended comparable early work on screening 13 fungicides (Smith, 1979) to incorporate a significantly broader range of compounds. For the emerging pathogen P. agathidicida, we have reported the first such high-throughput screen. By assessing effectiveness against three different stages of the Phytophthora life cycle, we have also explored new opportunities for control of these destructive organisms.
Of the 120 compounds screened, eight showed anti-oomycete activity against both Phytophthora species. P. agathidicida is an emerging pathogen, and none of the inhibitory compounds found here have been reported previously. The EC50 values for mycelial growth inhibition were all within an order of magnitude between the two species, suggesting that similar control options may be available for P. agathidicida as those that are used for P. cinnamomi. However, further study of the various known isolates of P. agathidicida and P. cinnamomi will be necessary in order assess any potential differences in sensitivities.
Five of the eight anti-oomycete compounds identified in our screens were antibiotics that are more commonly associated with antibacterial activity. Antibiotics have been used since the 1950s to control bacterial diseases of various plants. However, with the growing threat of antibiotic resistance, this practice is the subject of debate (McManus et al., 2002; Tripathi and Cytryn, 2017; Wiles, 2017). The inhibitory abilities of the antibiotics identified in this study differed markedly between Phytophthora life cycle stages. This was particularly true of chlortetracycline, which was the most effective mycelial growth inhibitor in both species, but had no effect on zoospore germination rates and showed relatively poor inhibition of zoospore motility. Paromomycin was the only antibiotic tested here that inhibited all three life cycle stages, albeit with less efficacy in zoospores than the copper-containing compounds or benzethonium chloride. Overall, given the limited efficacy of these antibiotics against the different life cycle stages of Phytophthora and the potential for resistance to spread within and between species, our data suggest there is little potential for exploring these antibiotics as treatments for Phytophthora diseases, except perhaps as a last resort.
The most effective compounds across all three life cycle stages that we identified were copper salts and the quaternary ammonium salt benzethonium chloride. The identification of copper in our screens is unsurprising, as copper-based fungicides have traditionally been a mainstay of Phytophthora control (Keast et al., 1985; Howard et al., 1998; Gisi and Sierotzki, 2008). However, it is interesting to note that both copper chloride and copper sulfate were ∼2-fold less effective at inhibiting mycelial growth in P. agathidicida as compared to P. cinnamomi. In New Zealand, copper sprays have been used widely in agricultural, horticultural, and forestry settings to control a range of bacterial and fungal pathogens. For example, copper spraying has become a significant component of the kiwifruit industry’s spray program for providing protection against infection by Pseudomonas syringae pv. actinidiae (Cameron and Sarojini, 2014; Gould et al., 2015). Similarly, copper fungicides are widely used by the New Zealand forestry industry for control of dothistroma needle blight (Bulman et al., 2013) and they have recently been shown to be effective against Phytophthora pluvialis (Rolando et al., 2016). However, concerns around soil accumulation and toxicity limit the use of copper sprays in natural environments (Wightwick et al., 2008; Komarek et al., 2010; Kiaune and Singhasemanon, 2011).
The quaternary ammonium salt benzethonium chloride was also effective across all three life cycle stages, for both species we examined. It showed EC50 values for mycelial growth inhibition of <10 μg/ml, immediate loss of zoospore motility at 0.1 μg/ml, and complete inhibition of germination at 32 μg/ml (P. cinnamomi) or 64 μg/ml (P. agathidicida). To our knowledge, this is the first report of the effectiveness of benzethonium chloride against any Phytophthora species, although other quaternary ammonium compounds have been shown to be effective against P. cinnamomi (Noske and Shearer, 1985). For example, benzalkonium chloride is sold in Australia under the brand name Phytoclean and is designed for use in shoe wash stations and for equipment/tool washdown. While preliminary, our data suggest that benzethonium chloride may be similarly useful, for example, in the shoe wash stations and sanitation kits that are currently employed to prevent the spread of kauri dieback. Before implementation, future work will need to include studies on phytotoxicity and the potential to be used as a soil drench, as was done during the commercialization of Phytoclean.
While our work expands the range of compounds known to possess anti-oomycete activity toward P. cinnamomi, it is particularly interesting with respect to P. agathidicida. As this species is an emerging pathogen that was only formally described in 2015 (Weir et al., 2015), comparatively little research has been carried out on treatment options. To date, phosphorous acid (phosphite) is the only chemical that has been used on kauri trees affected by P. agathidicida, and while this has proved an effective treatment, some signs of phytotoxicity were present at higher treatment concentrations (Horner and Hough, 2013; Horner et al., 2015). The EC50 for mycelial growth inhibition by phosphite was 4 μg/ml (Horner and Hough, 2013), similar to the EC50 we measured for benzethonium chloride (3.2 μg/ml). It must be noted, however, that phosphite acts both by inhibiting pathogen growth and by inducing host defense responses, therefore in vitro assays may overestimate the dose required in planta (Erwin and Ribeiro, 1996). Furthermore, copper chloride, copper sulfate, and benzethonium chloride all inhibited P. agathidicida zoospore motility and germination at low concentrations. Future work will also explore their effects on other stages of the P. agathidicida life cycle, such as the formation of the chlamydospores, oospores, and hyphal aggregates that are produced inside the roots of the hosts, as these may be critical targets for localized disease eradication.
Clearly work remains to be done to combat the threat to kauri posed by P. agathidicida. In this study, we have provided a list of promising anti-oomycete compounds, as well as a protocol for laboratory production of P. agathidicida zoospores. Our results suggest avenues for further investigation, bearing in mind that numerous factors must be considered before employing chemical control to manage plant disease, including: the rate, method, and frequency of application; possible phytotoxicity; and the potential for development of resistance. Ultimately, we hope that this study will facilitate further research into this pathogen, thereby helping to prevent the devastation of some of New Zealand’s most iconic forests.
Author Contributions
MG conceived this study. All authors participated in the experimental design, protocol development, and culturing of Phytophthora. SL and CA conducted all of the inhibition assays. All authors contributed to the writing and all authors have approved the final version.
Funding
This research was supported by a University of Otago Priming Partnerships grant and funding from the New Zealand National Science Challenge: New Zealand’s Biological Heritage Ng- a Koiora Tuku Iho.
Conflict of Interest Statement
The authors declare that the research was conducted in the absence of any commercial or financial relationships that could be construed as a potential conflict of interest.
Acknowledgments
Phytophthora agathidicida isolate NZFS 3770 and P. cinnamomi isolate NZFS 3910 were provided by the Healthy Trees, Healthy Future Research Programme (Scion, Rotorua, New Zealand). We also gratefully acknowledge Drs Rebecca Ganley, Nari Williams, and Peter Scott for protocol advice and their assistance in establishing our Phytophthora research programme at the University of Otago.
Supplementary Material
The Supplementary Material for this article can be found online at: http://journal.frontiersin.org/article/10.3389/fmicb.2017.01340/full#supplementary-material
References
Allen, R. N., and Harvey, J. D. (1974). Negative chemotaxis of zoospores of Phytophthora cinnamomi. J. Gen. Microbiol. 84, 28–38. doi: 10.1099/00221287-84-1-28
Allen, R. N., and Newhook, F. J. (1973). Chemotaxis of zoospores of Phytophthora cinnamomi to ethanol in capillaries of soil pore dimensions. Trans. Br. Mycol. Soc. 61, 287–302. doi: 10.1016/S0007-1536(73)80152-4
Bauer, A. W., Kirby, W. M. M., Sherris, J. C., and Turck, M. (1966). Antibiotic susceptibility testing by a standardized single disk method. Am. J. Clin. Pathol. 57, 592–597.
Beever, R. E., Walpara, N. W., Ramsfield, T. D., Dick, M. A., and Horner, I. J. (2009). “Kauri (Agathis australis) under threat from Phytophthora?,” in Phytophthoras in Forests and Natural Ecosystems. Proceedings of the Fourth Meeting of IUFRO Working Party S07.02.09, General Technical report OSW-GTR-221, eds E. M. Goheen and S. J. Frankel (Albany, CA: USDA Forest Service), 74–85.
Bochner, B. R., Gadzinski, P., and Panomitros, E. (2001). Phenotype microarrays for high-throughput phenotypic testing and assay of gene function. Genome Res. 11, 1246–1255. doi: 10.1101/gr.186501
Burgess, T. I., Scott, J. K., McDougall, K. L., Stukely, M. J., Crane, C., Dunstan, W. A., et al. (2017). Current and projected global distribution of Phytophthora cinnamomi, one of the world’s worst plant pathogens. Glob. Chang Biol. 23, 1661–1674. doi: 10.1111/gcb.13492
Bulman, L. S., Dick, M. A., Ganley, R. J., McDougal, R. L., Schwelm, A., and Bradshaw, R. E. (2013). “Dothistroma needle blight”, in Infectious Forest Diseases, ed. G. Nicolotti and P. Gonthier (Wallingford: CABI), 436–437.
Cameron, A., and Sarojini, V. (2014). Pseudomonas syringae pv. actinidiae: chemical control, resistance mechanisms and possible alternatives. Plant Pathol. 63, 1–11. doi: 10.1111/ppa.12066
Carter, R. E. (2004). Arresting Phytophthora Dieback: The Biological Bulldozer. Ultimo NSW: WWF Australia.
Chen, D. W., and Zentmyer, G. A. (1970). Production of sporangia by Phytophthora cinnamomi in axenic culture. Mycologia 62, 397–402. doi: 10.2307/3757597
Cohen, Y., and Coffey, M. D. (1986). Systemic fungicides and the control of Oomycetes. Annu. Rev. Phytopathol. 24, 311–338. doi: 10.1146/annurev.py.24.090186.001523
Dunstan, W. A., Rudman, T., Shearer, B. L., Moore, N. A., Paap, T., Calver, M. C., et al. (2010). Containment and spot eradication of a highly destructive, invasive plant pathogen (Phytophthora cinnamomi) in natural ecosystems. Biol. Invasion 12, 913–925. doi: 10.1007/s10530-009-9512-6
Ecroyd, C. E. (1982). Biological flora of New Zealand 8. Agathis australis (D. Don) Lindl. (Araucariaceae) Kauri. N. Z. J. Bot. 20, 17–36. doi: 10.1080/0028825X.1982.10426402
Erwin, D. C., and Ribeiro, O. K. (1996). Phytophthora Diseases Worldwide. St. Paul, MN: The American Phytopathological Society.
Gisi, U., and Sierotzki, H. (2008). Fungicide modes of action and resistance in downy mildews. Eur. J. Plant Pathol. 122, 157–167. doi: 10.1007/s10658-008-9290-5
Gould, E. M., Black, M. Z., Clark, G., Tanner, D. J., and Benge, J. (2015). Tools for managing the kiwifruit bacterial canker disease Pseudomonas syringae pv actinidiae (Psa). Acta Hortic. 1105, 39–46. doi: 10.17660/ActaHortic.2015.1105.6
Gunderson, J. H., Elwood, H., Ingold, A., Kindle, K., and Sogin, M. L. (1987). Phylogenetic relationships between chlorophytes, chrysophytes, and oomycetes. Proc. Natl. Acad. Sci. U.S.A. 84, 5823–5827. doi: 10.1073/pnas.84.16.5823
Horner, I. J., and Hough, E. G. (2013). Phosphorous acid for controlling Phytophthora taxon Agathis in kauri: glasshouse trials. N. Z. Plant Prot. 66, 242–248.
Horner, I. J., Hough, E. G., and Horner, M. B. (2015). Forest efficacy trials on phosphite for control of kauri dieback. N. Z. Plant Prot. 68, 7–12.
Howard, K., Colquhoun, I. J., and Hardy, G. (1998). The potential of copper sulphate to control Phytophthora cinnamomi during bauxite mining in Western Australia. Australas. Plant Pathol. 27, 51–58. doi: 10.1071/AP98006
Hu, J., Hong, C., Stromberg, E. L., and Moorman, G. W. (2007). Effects of propamocarb hydrochloride on mycelial growth, sporulation and infection by Phytophthora nicotianae isolates from Virginia nurseries. Plant Dis. 91, 414–420. doi: 10.1094/PDIS-91-4-0414
Hughes, M. F. (2002). Arsenic toxicity and potential mechanisms of action. Toxicol. Lett. 133, 1–16. doi: 10.1016/S0378-4274(02)00084-X
Judelson, H. S., and Blanco, F. A. (2005). The spores of Phytophthora: weapons of the plant destroyer. Nat. Rev. Microbiol. 3, 47–58. doi: 10.1038/nrmicro1064
Kamoun, S., Furzer, O., Jones, J. D., Judelson, H. S., Ali, G. S., Dalio, R. J., et al. (2015). The top 10 oomycete pathogens in molecular plant pathology. Mol. Plant Pathol. 16, 413–434. doi: 10.1111/mpp.12190
Keast, D., Tonkin, C., and Sanfelieu, L. (1985). Effects of copper salts on growth and survival of Phytophthora cinnamomi in vitro and on the antifungal activity of actinomycete populations from the roots of Eucalyptus marginata and Banksia grandis. Austral. J. Bot. 33, 115–129. doi: 10.1071/BT9850115
Kiaune, L., and Singhasemanon, N. (2011). Pesticidal copper (I) oxide: environmental fate and aquatic toxicity. Rev. Environ. Contam. Toxicol. 213, 1–26. doi: 10.1007/978-1-4419-9860-6_1
Komarek, M., Cadkova, E., Chrastny, V., Bordas, F., and Bollinger, J.-C. (2010). Contamination of vineyard soils with fungicides: a review of environmental and toxicological aspects. Environ. Intl. 36, 148–151. doi: 10.1016/j.envint.2009.10.005
Lee, H. B., Kim, Y., Kim, J. C., Choi, G. J., Park, S.-H., Kim, C.-J., et al. (2005). Activity of some aminoglycoside antibiotics against true fungi, Phytophthora and Pythium species. J. Appl. Microbiol. 99, 836–843. doi: 10.1111/j.1365-2672.2005.02684.x
Liu, P., Wang, H., Zhou, Y., Meng, Q., Si, N., Hao, J. J., et al. (2014). Evaluation of fungicides enestroburin and SYP1620 on their inhibitory activities to fungi and oomycetes and systemic translocation in plants. Pestic. Biochem. Physiol. 112, 19–25. doi: 10.1016/j.pestbp.2014.05.004
McManus, P. S., Stockwell, V. O., Sundin, G. W., and Jones, A. L. (2002). Antibiotic use in plant agriculture. Annu. Rev. Phytopathol. 40, 443–465. doi: 10.1146/annurev.phyto.40.120301.093927
Miao, J., Cai, M., Dong, X., Liu, L., Lin, D., Zhang, C., et al. (2016). Resistance assessment for oxathiapiprolin in Phytophthora capsici and the detection of a point mutation (G769W) in PcORP1 that confers resistance. Front. Microbiol. 7:615. doi: 10.3389/fmicb.2016.00615
Mircetich, S. M. (1970). Inhibition of germination of chlamydospores of Phytophthora cinnamomi by some antimicrobial agents in Phytophthora selective media. Can. J. Microbiol. 16, 1227–1230. doi: 10.1139/m70-206
Noske, G. L., and Shearer, B. L. (1985). Quaternary ammonium compounds were more effective than a phenolic compound or sodium hypochlorite in inhibiting growth of Phytophthora cinnamomi (Rands). Austral. Plant Pathol. 14, 37–40. doi: 10.1071/app9850037
Parra, G., and Ristaino, J. B. (2001). Resistance to mefenoxam and metalaxyl among field isolates of Phytophthora capsici causing Phytophthora blight of bell pepper. Plant Dis. 85, 1069–1075. doi: 10.1094/PDIS.2001.85.10.1069
Rolando, C. A., Dick, M. A., Gardner, J., Bader, M. K.-F., and Williams, N. M. (2016). Chemical control of two Phytophthora species infecting the canopy of Monterey pine (Pinus radiata). For. Pathol. 47:e12327. doi: 10.1111/efp.12327
Smith, P. M. (1979). A study of the effects of fungitoxic compounds on Phytophthora cinnamomi in water. Ann. Appl. Biol. 93, 149–157. doi: 10.1111/j.1744-7348.1979.tb06525.x
Thines, M. (2014). Phylogeny and evolution of plant pathogenic oomycetes—a global overview. Eur. J. Plant Pathol. 138, 431–447. doi: 10.1080/19440049.2014.984244
Tripathi, V., and Cytryn, E. (2017). Impact of anthropogenic activities on the dissemination of antibiotic resistance across ecological boundaries. Essays Biochem. 61, 11–21. doi: 10.1042/EBC20160054
Weir, B. S., Paderes, E. P., Anand, N., Uchida, J. Y., Pennycook, S. R., Bellgard, S. E., et al. (2015). A taxonomic revision of Phytophthora Clade 5 including two new species, Phytophthora agathidicida and P. cocois. Phytotaxa 205, 21–38. doi: 10.11646/phytotaxa.205.1.2
Wightwick, A. M., Mollah, M. R., Partington, D. L., and Allinson, G. (2008). Copper fungicide residues in Australian vineyard soils. J. Agric. Food Chem. 56, 2457–2464. doi: 10.1021/jf0727950
Wiles, S. (2017). Antibiotic Resistance: The End of Modern Medicine?. Wellington: Bridget Williams Books.
Keywords: oomycetes, Agathis australis, kauri dieback, avocado root rot, phenotype microarray, zoospore, high-throughput screening
Citation: Lawrence SA, Armstrong CB, Patrick WM and Gerth ML (2017) High-Throughput Chemical Screening Identifies Compounds that Inhibit Different Stages of the Phytophthora agathidicida and Phytophthora cinnamomi Life Cycles. Front. Microbiol. 8:1340. doi: 10.3389/fmicb.2017.01340
Received: 15 March 2017; Accepted: 03 July 2017;
Published: 19 July 2017.
Edited by:
Jesús Mercado-Blanco, Consejo Superior de Investigaciones Científicas (CSIC), SpainReviewed by:
Santa Olga Cacciola, University of Catania, ItalyGiles E. St. J. Hardy, Murdoch University, Australia
Copyright © 2017 Lawrence, Armstrong, Patrick and Gerth. This is an open-access article distributed under the terms of the Creative Commons Attribution License (CC BY). The use, distribution or reproduction in other forums is permitted, provided the original author(s) or licensor are credited and that the original publication in this journal is cited, in accordance with accepted academic practice. No use, distribution or reproduction is permitted which does not comply with these terms.
*Correspondence: Monica L. Gerth, bW9uaWNhLmdlcnRoQG90YWdvLmFjLm56