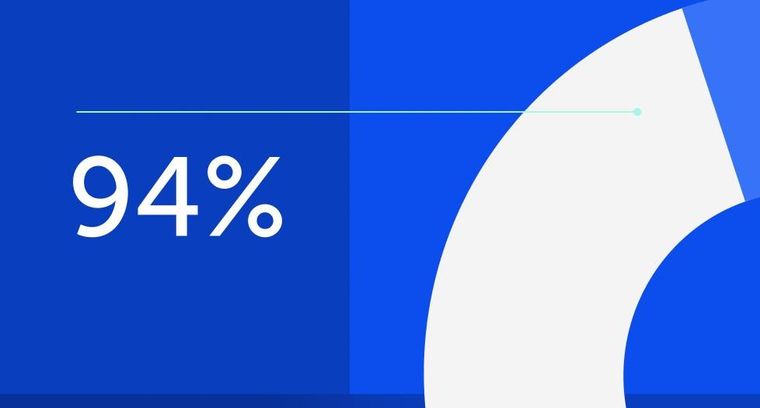
94% of researchers rate our articles as excellent or good
Learn more about the work of our research integrity team to safeguard the quality of each article we publish.
Find out more
ORIGINAL RESEARCH article
Front. Microbiol., 14 July 2017
Sec. Extreme Microbiology
Volume 8 - 2017 | https://doi.org/10.3389/fmicb.2017.01336
This article is part of the Research TopicThermophilic and Halophilic Extremophiles in Eurasian EnvironmentsView all 12 articles
Thioarsenates are common arsenic species in sulfidic geothermal waters, yet little is known about their biogeochemical traits. In the present study, a novel sulfate-reducing bacterial strain Desulfotomaculum TC-1 was isolated from a sulfidic hot spring in Tengchong geothermal area, Yunnan Province, China. The arxA gene, encoding anaerobic arsenite oxidase, was successfully amplified from the genome of strain TC-1, indicating it has a potential ability to oxidize arsenite under anaerobic condition. In anaerobic arsenite oxidation experiments inoculated with strain TC-1, a small amount of arsenate was detected in the beginning but became undetectable over longer time. Thioarsenates (AsO4-xSx2- with x = 1–4) formed with mono-, di- and tri-thioarsenates being dominant forms. Tetrathioarsenate was only detectable at the end of the experiment. These results suggest that thermophilic microbes might be involved in the formation of thioarsenates and provide a possible explanation for the widespread distribution of thioarsenates in terrestrial geothermal environments.
High concentrations of arsenic have been reported in global terrestrial hot springs (McKenzie et al., 2001; Cleverley et al., 2003; Aiuppa et al., 2006; Pascua et al., 2007; Landrum et al., 2009; McCleskey et al., 2010). Traditionally, the predominant form of inorganic arsenic in aqueous environments is arsenate [As(V) as H2AsO4- and HAsO42-] and arsenite [As(III) as H3AsO30 and H2AsO3-] in oxic and anoxic environments, respectively (Oremland, 2003). However, recently pentavalent arsenic-sulfur species, so-called thioarsenates (AsO4-xSx2- with x = 1–4), have also been reported as important arsenic species in a number of sulfidic geothermal environments (Wilkin et al., 2003; Stauder et al., 2005; Planer-Friedrich et al., 2007, 2009; Härtig and Planer-Friedrich, 2012; Hug et al., 2014). For example, Hug et al. (2014) found that di- (x = 2) and tri-thioarsenates (x = 3) represented up to 25% of total arsenic in an acidic-sulfidic hot spring in New Zealand. Keller et al. (2014) investigated the arsenic speciation in natural alkaline-sulfidic geothermal waters (pH 8.56–9.60) and found that sulfide concentration and pH are the predominant factors determining the arsenic species distribution.
Extensive studies have shown that microbial activities can strongly influence the speciation and mobility of arsenic in natural environments through arsenic oxidation and reduction (Oremland et al., 2005; Paez-Espino et al., 2009). Most (if not all) of known arsenite-oxidizing microorganisms contain arsenite oxidases, which catalyze the transformation of arsenite [As(III)] to arsenate [As(V)] (Lett et al., 2012). The arsenite oxidases are encoded by aioA and arxA genes for aerobic and anaerobic arsenite-oxidizing bacteria, respectively. Thus the aioA and arxA genes have become molecular biomarkers to study the distribution and activity of arsenite-oxidizing bacteria in natural environments (Hamamura et al., 2009, 2010, 2014; Zargar et al., 2012; Engel et al., 2013; Jiang et al., 2014; Wu et al., 2015; Hernandez-Maldonado et al., 2016). Recently, it is speculated that filamentous microbial mats might play an important role in thioarsenate transformation in an alkaline, sulfidic hot spring in Yellowstone National Park, which is the first evidence showing microbially mediated thioarsenate species transformation by (hyper) thermophilic prokaryotes (Härtig and Planer-Friedrich, 2012). A subsequent investigation showed that the thermophilic microbial mats were mainly composed of Aquificales represented by Thermocrinis spp. and Sulfurihydrogenibium spp. (Planer-Friedrich et al., 2015). However, little is known about which microbial group was involved in the observed thioarsenate species transformation. In addition, one geochemical study on arsenic speciation in the Tengchong geothermal zone (TGZ) of Yunnan Province, China reported that thioarsenates are widely distributed in the high-sulfidic Tengchong hot springs (Guo et al., 2017). Thus, the TGZ hot springs are suitable sites for retrieving microorganisms potentially involved in thioarsenates transformation.
In the present study, we provided biological evidence on the potential involvement of a novel sulfate-reducing bacterium isolated from a TGZ hot spring, designated as Desulfotomaculum sp. TC-1, in the formation of thioarsenates. The strain TC-1 cells coupled sulfate reduction with arsenite oxidation, in which thioarsenates, instead of arsenate, were the main products. The results in this study suggested that microbial activities may be involved in the formation of thioarsenates in geothermal features and thus could explain the reported distribution of thioarsenates in sulfidic hot springs.
The TGZ is located at the collision boundary between the Indian and Eurasian plates. The TGZ is known for its various geothermal features, which contains more than 800 hot springs (Du et al., 2005; Guo and Wang, 2012). Previous studies have shown that Tengchong hot springs host very diverse microbial communities (Hedlund et al., 2012; Hou et al., 2013; Song et al., 2013a; Briggs et al., 2014), which play important roles in the elemental cycling (e.g., carbon, nitrogen, sulfur) and arsenic transformation (Jiang et al., 2010, 2014; Song et al., 2013b; Li et al., 2015; Wu et al., 2015; Yang et al., 2015; Chen et al., 2016). A hot spring (N24.95318°; E98.43838°) was found downstream of the Dagunguo (DGG) spring in the Rehai Geothermal National Park in the TGZ and was therefore named Dagunguo-2 (DGG-2) (Supplementary Figure S1) (Guo et al., 2017). In June 2014, water temperature and pH were measured in the field with a portable meter (LaMotte, Chestertown, MD, United States). Water chemistry (S2-, Fe2+, NO2- and NH4+) measurements were performed by using Hach kits (Hach Company, Loveland, CO, United States). Sediment samples of the sampled hot spring were aseptically collected for cultivation.
Hungate techniques were used for enrichment and isolation. The hot spring sediment samples were transferred into 25 mL Balch tubes containing 5 mL DSMZ medium 63, which was pre-prepared anaerobically with the headspace filled with 100% N2 gas. In situ enrichments (by putting the culture tubes in the hot spring) were incubated for 48 h and then the resulting enrichment cultures were transported to laboratory for further isolation and purification. To avoid light effects, the balch tubes were covered with foil in situ and ex situ during incubation. Inoculation, sampling, and isolation were performed in an anaerobic glove box (COY Laboratory Products, Grass Lake, MI, United States) with aseptic techniques (The gas chamber was filled with 100% N2). The incubation temperature was 60°C. Cultures with positive growth (as indicated by the formation of black ferrous iron sulfide) were transferred three times for further purification. Isolation was performed by using the rolling-tube method with a high-melting-point agar, GELRITE gellan gum (Sigma) (Hungate and Macy, 1973).
The morphology of strain TC-1 cells was examined with a Zeiss Supra 55 SAPPHIRE scanning electron microscope (SEM) using 7–10 keV accelerating voltage and 8.5 mm working distance. SEM sample preparation and observation were performed according to previously described methods (Zhao et al., 2013, 2015).
Total DNA of strain TC-1 was extracted with Bacterial DNA extraction kit (ABigen, Hangzhou, China) according to manufacturer’s protocol. The 16S rRNA and arxA genes were amplified with the primer sets of Bac27F/Univ1492R and arxA_Deg_F_B (5′-CCA TCW SCT GGR ACG AGG CCY TSG-3′)/arxA_Deg_R_B (5′-GTW GTT GTA GGG GCG GAA S-3′) (Zargar et al., 2012), respectively. PCR amplification, sequencing, and phylogenetic analysis of the 16S rRNA and arxA genes were performed as previously described (Weisburg et al., 1991; Zargar et al., 2010, 2012). The arxA and 16S rRNA gene sequences of strain TC-1 were deposited in the GenBank under accession numbers of KX242336 and KX242337, respectively.
Strain TC-1 was grown at 60°C in anoxic DSMZ 63 medium (1 L) made of solution A [K2HPO4 0.5 g; NH4Cl,1.0 g; Na2SO4,1.0 g; CaCl2 × 2 H2O, 0.1 g; MgSO4 × 7 H2O, 2.0 g; Na-DL-lactate, 2.0 g; yeast extract, 1.0 g; Na-resazurin solution (0.1% w/v), 0.5 ml; distilled water, 980.0 ml], solution B (FeSO4 × 7 H2O, 0.5 g; Distilled water, 10.0 ml) and solution C (ascorbic acid, 0.1 g; distilled water, 10.0 ml). The initial pH was 6.8, and pH varied less than 0.2 units during growth. To remove oxygen, the growth medium was boiled for >10 min, purged with N2 upon cooling and immediately transferred into an anoxic chamber (100% N2) for dispensing into glass serum bottles, which were subsequently sealed with thick butyl rubber stoppers. The sets of experiments were inoculated with TC-1 in DSMZ 63 medium with solution B replaced by 0.5 mM arsenite; Two types of abiotic controls were set up: one was in the DSMZ 63 medium with solution B replaced by 5 mM sulfide (Na2S⋅9 H2O, Sigma–Aldrich) and 0.5 mM arsenite (Sigma–Aldrich), and the other was in the DSMZ 63 medium with solution B replaced by 5 mM sulfide and 0.5 mM arsenate (Sigma–Aldrich). All experimental treatments were performed in triplicate. Liquid sampling for arsenic measurement was performed with aseptic syringes in an anaerobic chamber according to a previous method (Planer-Friedrich et al., 2015).
Total arsenic concentration and arsenic speciation (arsenite and arsenate) were measured according to previously described methods (Wu et al., 2015). Briefly, total arsenic concentration was measured with inductively coupled plasma atomic emission spectroscopy (ICP-AES) (iCAP ICP Spectrometer, Thermo Fisher Scientific, United States) with argon torch and iTeva software (Thermo Fisher Scientific, United States). Arsenic speciation (arsenite and arsenate) was determined with high performance liquid chromatography (HPLC)-atomic fluorescence spectroscopy (AFS). If the sum of measured As(III) and As (V) was not equal to total arsenic, those samples were oxidized by H2O2, in which As(III) and Thio-arsenate will be transferred to As(V) (Pettine et al., 1999), and then were measured for total As by ICP-AES.
The relative abundances of four major thioarsenic species in the samples, including H3AsSO3, H3AsS2O2, H3AsS3O and H3AsS4, were identified by injecting a liquid sample (5 μL) into a liquid chromatography-high resolution mass spectrometry (LC-HRMS, Q Exactive, Thermo Scientific, Germany) according to previously described methods (Chang et al., 2015; Yang et al., 2016). The mobile phase contained 50% acetonitrile (v/v) and 0.1% acetic acid (m/v) with a flow rate of 0.25 mL min-1. The mass spectrometer system was operated with a heated electrospray ionization (HESI) source in a negative ion mode with a spray voltage of -3.2 kV, an S-lens RF level of 50%, a capillary temperature of 300°C, and a mass resolution of 70,000. The runtime was 2 min for each sample. The mass tolerance of the Precursor ion was below 5 ppm. Mass spectra were processed by using the Xcalibur 2.1 software (Thermo Scientific). The relative abundance of each thioarsenic species was calculated according to their corresponding chromatographic peak area.
The pH and temperature of the Dagunguo-2 hot spring were 5.5 and 58.3°C, respectively. The spring water contained S2- (1.53 μM), Fe2+ (5 μM), NO2- (0.065 μM), and NH4+ (37.8 μM).
One strain was obtained and designated as strain TC-1 (Supplementary Figure S2). Phylogenetic analysis on the basis of 16S rRNA gene sequence identified strain TC-1as a close (sequence identity: 99.7%) relative of a sulfate-reducing bacterium Desulfotomaculum carboxydivorans CO-1-SRB, which was isolated from a sludge of an anaerobic bioreactor treating paper mill wastewater (Pettine et al., 1999) (see Figure 1A and Table 1). The arxA gene of strain TC-1 was successfully amplified and was closely related (sequence identity: 99%) to those recovered from Tukh Lake (represented by HJ1A27 in Figure 1B). The morphology of Desulfotomaculum TC-1 cells was rod-shaped with rounded ends, 0.8–1.5 μm in length and 0.2–0.4 μm in width (Supplementary Figure S2). The optimum growth temperature and pH for TC-1 were 60°C and 6.8, respectively. The optimum growth temperature was consistent with the environmental condition of the spring where the TC-1 strain was isolated (57°C). Under optimum conditions, the doubling time of strain TC-1 was approximately 30 h. Strain TC-1 cannot grow on As(III) in the DSMZ 63 medium (without lactate).
FIGURE 1. Phylogenetic trees of 16S rRNA (A) and the deduced amino acid sequences of ArxA (B) encoded by arxA genes of strain TC-1 showing their relatedness to its close relatives in the GenBank. The GenBank accession numbers are listed in parentheses. Bootstrap values (per 1000 trials) > 50% are indicated. NJ/ML/ME indicates Neighbor-Joining/Maximum Likelihood/Minimum-Evolution algorithms.
TABLE 1. Physiological, phylogenetic and phenotypic comparisons between strain TC-1 and known arxA gene-containing strains.
Arsenic speciation was examined for 84 h after inoculation with strain TC-1 (Figure 2). Arsenite (0.5 mM) was nearly exhausted in 60 h. As arsenite was consumed, some amount of arsenate was initially detected after 24 h (Figure 2). After 60 h, both arsenite and arsenate were not detectable in the experimental tubes, but the total arsenic in the solution remained unchanged, indicating no formation of insoluble arsenic precipitates. In the abiotic control containing Na2S and arsenite, white flocs formed in solution immediately. The resulting white flocs were separated by high speed centrifugation (12,000 rpm), and then were observed by SEM-EDS for element mapping. The results showed that arsenite could react with Na2S to form the flocs which contained S and As (Supplementary Figure S3). The resulted supernatant was treated with H2O2 followed by ICP-AES measurement, but no arsenic was detected (data not shown).
FIGURE 2. Time-course variations of arsenite and arsenate concentrations and total arsenic concentration during anaerobic arsenite oxidation by strain TC-1. Triplicate samples were performed, and error bars were smaller than the sizes of the symbols.
The LC-HRMS analysis showed that the missing arsenite had been transformed to thioarsenic species (mono-thioarsenate [HAsSO3]2-, di-thioarsenate [HAsVS2O2]2-, tri-thioarsenate [HAsS3O]2- and tetra-thioarsenate [HAsS4]2-) (Figure 3), and the relative concentrations of each identified thioarsenic species varied during the oxidation process: In the first 60 h, monothioarsenate, di-thioarsenate and tri-thioarsenate were dominant species in the solution, while no tetra-thioarsenate was detected. As arsenite oxidation experiment proceeded, a small amount of tetra-thioarsenate was detected at 84 h (at the end of the experiment) (Figure 3). In the abiotic control containing Na2S and arsenate, only a tiny amount of di-thioarsenate was observed, but other thioarsenic species (i.e., monothioarsenate, tri-thioarsenate, and tetra-thioarsenate) were not detected.
FIGURE 3. Time-course variation of thioarsenate composition (expressed as the corresponding chromatographic peak area of each identified thioarsenic species) produced during anaerobic arsenite oxidation by strain TC-1.
High levels of arsenic has been extensively reported in global terrestrial hot springs. Thus, terrestrial hot springs are an excellent setting for investigating arsenic biogeochemical cycling (Qin et al., 2009). In geothermal environments, microbially mediated aerobic arsenite oxidation has been frequently reported (Oremland, 2003), while few studies up to date discovered anaerobic arsenite oxidation by microbes. For example, Rhine et al. (2006) reported anaerobic arsenite oxidation phenomenon by novel denitrifying isolates from an arsenic contaminated industrial soil, while no function genes related to anaerobic arsenite oxidation were amplified; Zhao et al. (2015) reported anaerobic arsenite oxidation by an autotrophic arsenite-oxidizing bacterium from an arsenic-contaminated paddy soil and aioA gene was successfully amplified from those pure cultures, although the aioA gene is putatively involved in aerobic arsenic oxidation (Lett et al., 2012). Zargar et al. (2010) identified a novel arsenite oxidase gene, arxA, from Alkalilimnicola ehrlichii strain MLHE-1, an anaerobic arsenite-oxidizing bacterium from Mono Lake. However, little is reported on anaerobic arsenite oxidation by microbes in geothermal features. To our best knowledge, the only case of microbially mediated anaerobic arsenite oxidation in hot springs was reported in a hot spring (temperature 43°C) biofilm on the shore of the Paoha Island in Mono Lake (Kulp et al., 2008), which showed anaerobic photosynthetic arsenic(III) oxidation by strain Ectothiorhodospira strain PHS-1. However, in the present study, strain TC-1 can anaerobically oxidize arsenite at high temperature (60°C) independent of light or photosynthesis, indicating that photosynthesis-independent, microbially mediated anaerobic arsenic oxidation could take place in geothermal features.
To our best knowledge, strain TC-1 is the first known sulfate-reducing strain containing arxA gene. To date, only four other known arxA gene-containing strains have been obtained in pure cultures (Table 1) and they all fall within α-Proteobacteria, among which A. ehrlichii MLHE-1, Halorhodospira halophila SL1, and Halomonas sp. ANAO-440 were isolated from alkaline and/or saline environments, while Ectothiorhodospira strain PHS-1 was retrieved from a geothermal feature (temperature 43°C) (Oremland et al., 2002; Kulp et al., 2008; Zargar et al., 2010, 2012; Challacombe et al., 2013; Hamamura et al., 2014; Hernandez-Maldonado et al., 2016). In contrast, strain TC-1 belongs to Firmicutes and is the only known strain within Firmicutes possessing the arxA gene. The affiliation of strain TC-1 with Firmicutes indicated that the microbes involved in anaerobic arsenic oxidation may be more phylogenetically diverse than currently known. It is possible that the arxA gene of strain TC-1 might have originated from other microbes via horizontal gene transfer as in strain PHS-1 (Zargar et al., 2012).
It is notable that thioarsenates could be formed by sulfate reducing bacteria under anaerobic conditions in hot springs. Previous work on arsenic speciation in geothermal environments reported the dominance of As(III) and As(V) in the bulk arsenic speciation (Ballantyne and Moore, 1988; Yokoyama et al., 1993; Macur et al., 2004). While studies with improved sample preservation techniques revealed that thioarsenate species were present or even abundant in geothermal features (Wilkin et al., 2003; Stauder et al., 2005; Planer-Friedrich et al., 2007; Wallschläger and Stadey, 2007; Guo et al., 2017), which can possibly make up to more than 50% of total dissolved arsenic in sulfidic waters (Wilkin et al., 2003; Hug et al., 2014). Most (if not all) of the geothermal features with reported high thioarsenates were sulfidic. The potential underlying reason for the formation of thioarsenates could be explained by the following equation:
in which arsenate reacted with sulfide, leading to the formation of thioarsenate (Planer-Friedrich et al., 2015). The reactant arsenate could be extant or derived from arsenite oxidation. Commonly anoxic condition dominates sulfidic habitats, thus anaerobic arsenite oxidation could take place to produce arsenate. In the present study, strain TC-1 could oxidize As(III) to As(V), which reacted with S2- or HS- and thus formed thioarsenate species. This reaction could also explain the wide distribution of thioarsenates in sulfidic aquifers (Wood et al., 2002; Wilkin et al., 2003; Bostick et al., 2005; Hollibaugh et al., 2005; Stauder et al., 2005; Wallschläger and Stadey, 2007), although no exact reasons were provided for the predominance of thioarsenates in those previous studies. The present study provides evidence for possible microbial involvement in the formation of thioarsenates in hot springs.
Thioarsenate may be an important arsenic species in sulfidic and arsenic-rich environments (Hollibaugh et al., 2005; Hug et al., 2014; Guo et al., 2017). Based on the results presented above, high arsenic geological settings (e.g., groundwater and acid mine drainage that have the potential of sulfate reducing process) may contain significant amounts of thioarsenates, which to date have received little attention. Thioarsenates are more toxic than arsenate and tri-thioarsenate is almost as bioavailable and toxic as arsenite (Hinrichsen et al., 2015). Thus more attention should be paid to thioarsenates in high arsenic, sulfidic habitats. Previous studies have shown that arsenic in solution could be removed through combination with sulfide minerals derived from microbial SO42- reduction (Moore et al., 1988; Rittle et al., 1995; Kirk et al., 2004), and that the enhanced SO42- reduction may be useful for arsenic remediation (Rittle et al., 1995; Newman et al., 1997; Castro et al., 1999; Macy et al., 2000; Jong and Parry, 2003; Lee et al., 2005; Saunders et al., 2005; Keimowitz et al., 2007; Saunders et al., 2008; Kirk et al., 2010; Luo et al., 2013). However, recently an experiment with permeable reactive barriers (PRB) was performed to test the effect of arsenic remediation in the presence microbial sulfate reduction, and found that up to 47% of total As initially present in the sediment was leached out in the form of mobile thio-As species (Kumar et al., 2016). Thus, more cautions should be taken on the geochemical behaviors of arsenic and sulfate in the environment (where the arsenite and arsenate have the potential to transform to mobile thioarsenates) when sulfate reducing bacteria are employed for arsenic remediation (Burton et al., 2014; Stucker et al., 2014).
GW and HJ conceived and designed the experiments. LH and ZC isolated the strain. GW, YP, WG, HD, WS, and QG performed the experiments. GW analyzed the data. All of the authors assisted in writing the manuscript, discussed the results and commented on the manuscript.
The authors declare that the research was conducted in the absence of any commercial or financial relationships that could be construed as a potential conflict of interest.
This work was supported by the National Natural Science Foundation of China grants (41502318, 41422208, 41521001, 41572335, 41630103), the Key Project of International Cooperation of China Ministry of Science and Technology (No. 2013DFA31980), and Special Foundation for Basic Research Program of China Ministry of Science and Technology (MOST) (No. 2015FY110100), and the Fundamental Research Funds for the Central Universities, China University of Geosciences (Wuhan) (No.CUGL170215).
The Supplementary Material for this article can be found online at: http://journal.frontiersin.org/article/10.3389/fmicb.2017.01336/full#supplementary-material
FIGURE S1 | The location (A) and geothermal features (B) of DGG-2 in the Rehai Geothermal Field, Tengchong, Yunnan, China.
FIGURE S2 | Scanning electron microscope images of strain Desulfotomaculum sp. TC-1 cells (Scale bar = 1 μm).
FIGURE S3 | Scanning electron microscope images of flocs and the S-As distribution in floc as indicated by the yellow line in (A). (A–C) Indicate the SEM morphology of the flocs and the EDX multi element mapping/line scanning spectra for As (arsenic) and S (sulfur) elements, respectively.
Aiuppa, A., Avino, R., Brusca, L., Caliro, S., Chiodini, G., D’alessandro, W., et al. (2006). Mineral control of arsenic content in thermal waters from volcano-hosted hydrothermal systems: insights from island of Ischia and Phlegrean Fields (Campanian Volcanic Province, Italy). Chem. Geol. 229, 313–330. doi: 10.1016/j.chemgeo.2005.11.004
Ballantyne, J. M., and Moore, J. N. (1988). Arsenic geochemistry in geothermal systems. Geochim. Cosmochim. Acta 52, 475–483. doi: 10.1016/0016-7037(88)90102-0
Bostick, B., Fendorf, S., and Brown, G. (2005). In situ analysis of thioarsenite complexes in neutral to alkaline arsenic sulphide solutions. Mineral. Mag. 69, 781–795. doi: 10.1180/0026461056950288
Briggs, B. R., Brodie, E. L., Tom, L. M., Dong, H., Jiang, H., Huang, Q., et al. (2014). Seasonal patterns in microbial communities inhabiting the hot springs of Tengchong, Yunnan Province, China. Environ. Microbiol. 16, 1579–1591. doi: 10.1111/1462-2920.12311
Burton, E. D., Johnston, S. G., and Kocar, B. D. (2014). Arsenic mobility during flooding of contaminated soil: the effect of microbial sulfate reduction. Environ. Sci. Technol. 48, 13660–13667. doi: 10.1021/es503963k
Castro, J. M., Wielinga, B. W., Gannon, J. E., and Moore, J. N. (1999). Stimulation of sulfate-reducing bacteria in lake water from a former open-pit mine through addition of organic wastes. Water Environ. Res. 71, 218–223. doi: 10.2175/106143098X121806
Challacombe, J. F., Majid, S., Deole, R., Brettin, T. S., Bruce, D., Delano, S. F., et al. (2013). Complete genome sequence of Halorhodospira halophila SL1. Stand. Genomic Sci. 8, 206–214. doi: 10.4056/sigs.3677284
Chang, Q., Peng, Y. E., Dan, C., Shuai, Q., and Hu, S. (2015). Rapid in situ identification of bioactive compounds in plants by in vivo nanospray high-resolution mass spectrometry. J. Agric. Food Chem. 63, 2911–2918. doi: 10.1021/jf505749n
Chen, S., Peng, X., Xu, H., and Ta, K. (2016). Nitrification of archaeal ammonia oxidizers in a high-temperature hot spring. Biogeosciences 13, 2051–2060. doi: 10.5194/bg-13-2051-2016
Cleverley, J. S., Benning, L. G., and Mountain, B. W. (2003). Reaction path modelling in the As–S system: a case study for geothermal As transport. Appl. Geochem. 18, 1325–1345. doi: 10.1016/S0883-2927(03)00054-4
Du, J., Liu, C., Fu, B., Ninomiya, Y., Zhang, Y., Wang, C., et al. (2005). Variations of geothermometry and chemical-isotopic compositions of hot spring fluids in the Rehai geothermal field, southwestern China. J. Volcanol. Geotherm. Res. 142, 243–261. doi: 10.1016/j.jvolgeores.2004.11.009
Engel, A. S., Johnson, L. R., and Porter, M. L. (2013). Arsenite oxidase gene diversity among Chloroflexi and Proteobacteria from El Tatio Geyser Field, Chile. FEMS Microbiol. Ecol. 83, 745–756. doi: 10.1111/1574-6941.12030
Guo, Q., Planer-Friedrich, B., Liu, M., Li, J., Zhou, C., and Wang, Y. (2017). Arsenic and thioarsenic species in the hot springs of the Rehai magmatic geothermal system, Tengchong volcanic region, China. Chem. Geol. 453, 12–20. doi: 10.1016/j.chemgeo.2017.02.010
Guo, Q., and Wang, Y. (2012). Geochemistry of hot springs in the Tengchong hydrothermal areas, Southwestern China. J. Volcanol. Geotherm. Res. 215, 61–73. doi: 10.1016/j.jvolgeores.2011.12.003
Hamamura, N., Itai, T., Liu, Y., Reysenbach, A. L., Damdinsuren, N., and Inskeep, W. P. (2014). Identification of anaerobic arsenite-oxidizing and arsenate-reducing bacteria associated with an alkaline saline lake in Khovsgol, Mongolia. Environ. Microbiol. Rep. 6, 476–482. doi: 10.1111/1758-2229.12144
Hamamura, N., Macur, R., Korf, S., Ackerman, G., Taylor, W., Kozubal, M., et al. (2009). Linking microbial oxidation of arsenic with detection and phylogenetic analysis of arsenite oxidase genes in diverse geothermal environments. Environ. Microbiol. 11, 421–431. doi: 10.1111/j.1462-2920.2008.01781.x
Hamamura, N., Macur, R. E., Liu, Y., Inskeep, W. P., and Reysenbach, A.-L. (eds). (2010). “Distribution of Aerobic Arsenite Oxidase Genes within the Aquificales,” Interdisciplinary Studies on Environmental Chemistry—Biological Responses to Contaminants, (Tokyo: TERRAPUB), 47–55.
Härtig, C., and Planer-Friedrich, B. (2012). Thioarsenate transformation by filamentous microbial mats thriving in an alkaline, sulfidic hot spring. Environ. Sci. Technol. 46, 4348–4356. doi: 10.1021/es4015458
Hedlund, B. P., Cole, J. K., Williams, A. J., Hou, W., Zhou, E., Li, W., et al. (2012). A review of the microbiology of the Rehai geothermal field in Tengchong, Yunnan Province, China. Geosci. Front. 3, 273–288. doi: 10.1016/j.gsf.2011.12.006
Hernandez-Maldonado, J., Sanchez-Sedillo, B., Stoneburner, B., Boren, A., Miller, L. G., Mccann, S., et al. (2016). The genetic basis of anoxygenic photosynthetic arsenite oxidation. Environ. Microbiol. 19, 130–141. doi: 10.1111/1462-2920.13509
Hinrichsen, S., Geist, F., and Planer-Friedrich, B. (2015). Inorganic and methylated thioarsenates pass the gastrointestinal barrier. Chem. Res. Toxicol. 28, 1678–1680. doi: 10.1021/acs.chemrestox.5b00268
Hollibaugh, J. T., Carini, S., Gürleyük, H., Jellison, R., Joye, S. B., Lecleir, G., et al. (2005). Arsenic speciation in Mono Lake, California: response to seasonal stratification and anoxia. Geochim. Cosmochim. Acta 69, 1925–1937. doi: 10.1016/j.gca.2004.10.011
Hou, W., Wang, S., Dong, H., Jiang, H., Briggs, B. R., Peacock, J. P., et al. (2013). A comprehensive census of microbial diversity in hot springs of Tengchong, Yunnan Province China using 16S rRNA gene pyrosequencing. PLoS ONE 8:e53350. doi: 10.1371/journal.pone.0053350
Hug, K., Maher, W. A., Stott, M. B., Krikowa, F., Foster, S., and Moreau, J. W. (2014). Microbial contributions to coupled arsenic and sulfur cycling in the acid-sulfide hot spring Champagne Pool, New Zealand. Front. Microbiol. 5:569. doi: 10.3389/fmicb.2014.00569
Hungate, R., and Macy, J. (1973). The roll-tube method for cultivation of strict anaerobes. Bull. Ecol. Res. Comm. 17, 123–126.
Jiang, H., Huang, Q., Dong, H., Wang, P., Wang, F., Li, W., et al. (2010). RNA-based investigation of ammonia-oxidizing archaea in hot springs of Yunnan Province, China. Appl. Environ. Microbiol. 76, 4538–4541. doi: 10.1128/AEM.00143-10
Jiang, Z., Li, P., Jiang, D., Wu, G., Dong, H., Wang, Y., et al. (2014). Diversity and abundance of the arsenite oxidase gene aioA in geothermal areas of Tengchong, Yunnan, China. Extremophiles 18, 161–170. doi: 10.1007/s00792-013-0608-7
Jong, T., and Parry, D. L. (2003). Removal of sulfate and heavy metals by sulfate reducing bacteria in short-term bench scale upflow anaerobic packed bed reactor runs. Water Res. 37, 3379–3389. doi: 10.1016/S0043-1354(03)00165-9
Keimowitz, A., Mailloux, B., Cole, P., Stute, M., Simpson, H., and Chillrud, S. (2007). Laboratory investigations of enhanced sulfate reduction as a groundwater arsenic remediation strategy. Environ. Sci. Technol. 41, 6718–6724. doi: 10.1021/es061957q
Keller, N. S., Stefánsson, A., and Sigfússon, B. (2014). Arsenic speciation in natural sulfidic geothermal waters. Geochim. Cosmochim. Acta 142, 15–26. doi: 10.1016/j.gca.2014.08.007
Kirk, M. F., Holm, T. R., Park, J., Jin, Q., Sanford, R. A., Fouke, B. W., et al. (2004). Bacterial sulfate reduction limits natural arsenic contamination in groundwater. Geology 32, 953–956. doi: 10.1130/G20842.1
Kirk, M. F., Roden, E. E., Crossey, L. J., Brealey, A. J., and Spilde, M. N. (2010). Experimental analysis of arsenic precipitation during microbial sulfate and iron reduction in model aquifer sediment reactors. Geochim. Cosmochim. Acta 74, 2538–2555. doi: 10.1016/j.gca.2010.02.002
Kulp, T. R., Hoeft, S. E., Asao, M., Madigan, M. T., Hollibaugh, J. T., Fisher, J. C., et al. (2008). Arsenic(III) fuels anoxygenic photosynthesis in hot spring biofilms from Mono Lake, California. Science 321, 967–970. doi: 10.1126/science.1160799
Kumar, N., Couture, R.-M., Millot, R., Battaglia-Brunet, F., and Rose, J. (2016). Microbial sulfate reduction enhances arsenic mobility downstream of zerovalent-iron-based Permeable Reactive Barrier. Environ. Sci. Technol. 50, 7610–7617. doi: 10.1021/acs.est.6b00128
Landrum, J., Bennett, P., Engel, A., Alsina, M., Pastén, P., and Milliken, K. (2009). Partitioning geochemistry of arsenic and antimony, El Tatio Geyser Field, Chile. Appl. Geochem. 24, 664–676. doi: 10.1016/j.apgeochem.2008.12.024
Lee, M., Saunders, J. A., Wilkin, R. T., and Mohammad, S. (2005). “Geochemical modeling of arsenic speciation and mobilization: implications for bioremediation,” in Advances in Arsenic Research: Integration of Experimental and Observational Studies and Implications for Mitigation, ed. P. A. O’Day (Washington, DC: American Chemical Society), 398–413.
Lett, M. C., Muller, D., Lievremont, D., Silver, S., and Santini, J. (2012). Unified nomenclature for genes involved in prokaryotic aerobic arsenite oxidation. J. Bacteriol. 194, 207–208. doi: 10.1128/JB.06391-11
Li, H., Yang, Q., Li, J., Gao, H., Li, P., and Zhou, H. (2015). The impact of temperature on microbial diversity and AOA activity in the Tengchong Geothermal Field, China. Sci. Rep. 5:17056. doi: 10.1038/srep17056
Luo, T., Tian, H., Guo, Z., Zhuang, G., and Jing, C. (2013). Fate of arsenate adsorbed on nano-TiO2 in the presence of sulfate reducing bacteria. Environ. Sci. Technol. 47, 10939–10946. doi: 10.1021/es400883c
Macur, R. E., Langner, H. W., Kocar, B. D., and Inskeep, W. P. (2004). Linking geochemical processes with microbial community analysis: successional dynamics in an arsenic-rich, acid-sulphate-chloride geothermal spring. Geobiology 2, 163–177. doi: 10.1111/j.1472-4677.2004.00032.x
Macy, J. M., Santini, J. M., Pauling, B. V., O’neill, A. H., and Sly, L. I. (2000). Two new arsenate/sulfate-reducing bacteria: mechanisms of arsenate reduction. Arch. Microbiol. 173, 49–57. doi: 10.1007/s002030050007
McCleskey, R. B., Nordstrom, D. K., Susong, D. D., Ball, J. W., and Taylor, H. E. (2010). Source and fate of inorganic solutes in the Gibbon River, Yellowstone National Park, Wyoming, USA. II. Trace element chemistry. J. Volcanol. Geotherm. Res. 196, 139–155. doi: 10.1016/j.jvolgeores.2010.05.004
McKenzie, E. J., Brown, K. L., Cady, S. L., and Campbell, K. A. (2001). Trace metal chemistry and silicification of microorganisms in geothermal sinter, Taupo Volcanic Zone, New Zealand. Geothermics 30, 483–502. doi: 10.1016/s0375-6505(01)00004-9
Moore, J. N., Ficklin, W. H., and Johns, C. (1988). Partitioning of arsenic and metals in reducing sulfidic sediments. Environ. Sci. Technol. 22, 432–437. doi: 10.1021/es00169a011
Newman, D. K., Beveridge, T. J., and Morel, F. M. M. (1997). Precipitation of arsenic trisulfide by Desulfotomaculum auripigmentum. Appl. Environ. Microbiol. 63, 2022–2028.
Oremland, R. S., Hoeft, S. E., Santini, J. M., Bano, N., Hollibaugh, R. A., and Hollibaugh, J. T. (2002). Anaerobic oxidation of arsenite in Mono Lake water and by a facultative, arsenite-oxidizing chemoautotroph, strain MLHE-1. Appl. Environ. Microbiol. 68, 4795–4802. doi: 10.1128/AEM.68.10.4795-4802.2002
Oremland, R. S., Kulp, T. R., Blum, J. S., Hoeft, S. E., Baesman, S., Miller, L. G., et al. (2005). A microbial arsenic cycle in a salt-saturated, extreme environment. Science 308, 1305–1308. doi: 10.1126/science.1110832
Paez-Espino, D., Tamames, J., De Lorenzo, V., and Canovas, D. (2009). Microbial responses to environmental arsenic. Biometals 22, 117–130. doi: 10.1007/s10534-008-9195-y
Pascua, C. S., Minato, M., Yokoyama, S., and Sato, T. (2007). Uptake of dissolved arsenic during the retrieval of silica from spent geothermal brine. Geothermics 36, 230–242. doi: 10.1016/j.geothermics.2007.03.001
Pettine, M., Campanella, L., and Millero, F. J. (1999). Arsenite oxidation by H2O2 in aqueous solutions. Geochim. Cosmochim. Acta 63, 2727–2735. doi: 10.1016/S0016-7037(99)00212-4
Planer-Friedrich, B., Fisher, J. C., Hollibaugh, J. T., Suss, E., and Wallschlager, D. (2009). Oxidative transformation of trithioarsenate along alkaline geothermal drainages - abiotic versus microbially mediated processes. Geomicrobiol. J. 26, 339–350. doi: 10.1080/01490450902755364
Planer-Friedrich, B., Härtig, C., Lohmayer, R., Suess, E., Mccann, S. H., and Oremland, R. (2015). Anaerobic chemolithotrophic growth of the Haloalkaliphilic bacterium strain MLMS-1 by disproportionation of monothioarsenate. Environ. Sci. Technol. 49, 6554–6563. doi: 10.1021/acs.est.5b01165
Planer-Friedrich, B., London, J., Mccleskey, R. B., Nordstrom, D. K., and Wallschlager, D. (2007). Thioarsenates in geothermal waters of yellowstone national park: determination, preservation, and geochemical importance. Environ. Sci. Technol. 41, 5245–5251. doi: 10.1021/es070273v
Qin, J., Lehr, C. R., Yuan, C., Le, X. C., Mcdermott, T. R., and Rosen, B. P. (2009). Biotransformation of arsenic by a Yellowstone thermoacidophilic eukaryotic alga. Proc. Natl. Acad. Sci. U.S.A. 106, 5213–5217. doi: 10.1073/pnas.0900238106
Rhine, E. D., Phelps, C. D., and Young, L. Y. (2006). Anaerobic arsenite oxidation by novel denitrifying isolates. Environ. Microbiol. 8, 899–908. doi: 10.1111/j.1462-2920.2005.00977.x
Rittle, K. A., Drever, J. I., and Colberg, P. J. S. (1995). Precipitation of arsenic during bacterial sulfate reduction. Geomicrobiol. J. 13, 1–11. doi: 10.1080/01490459509378000
Saunders, J. A., Lee, M.-K., Shamsudduha, M., Dhakal, P., Uddin, A., Chowdury, M., et al. (2008). Geochemistry and mineralogy of arsenic in (natural) anaerobic groundwaters. Appl. Geochem. 23, 3205–3214. doi: 10.1016/j.apgeochem.2008.07.002
Saunders, J. A., Lee, M.-K., Wolf, L. W., Morton, C. M., Feng, Y., Thomson, I., et al. (2005). Geochemical, microbiological, and geophysical assessments of anaerobic immobilization of heavy metals. Bioremediat. J. 9, 33–48. doi: 10.1080/01490459709378044
Song, Z., Wang, F., Zhi, X., Chen, J., Zhou, E., Liang, F., et al. (2013a). Bacterial and archaeal diversities in Yunnan and Tibetan hot springs. China. Environ. Microbiol. 15, 1160–1175. doi: 10.1111/1462-2920.12025
Song, Z., Wang, L., Wang, F., Jiang, H., Chen, J., Zhou, E., et al. (2013b). Abundance and diversity of archaeal accA gene in hot springs in Yunnan Province, China. Extremophiles 17, 871–879. doi: 10.1007/s00792-013-0570-4
Stauder, S., Raue, B., and Sacher, F. (2005). Thioarsenates in sulfidic waters. Environ. Sci. Technol. 39, 5933–5939. doi: 10.1021/es048034k
Stucker, V. K., Silverman, D. R., Williams, K. H., Sharp, J. O., and Ranville, J. F. (2014). Thioarsenic species associated with increased arsenic release during biostimulated subsurface sulfate reduction. Environ. Sci. Technol. 48, 13367–13375. doi: 10.1002/etc.2155
Wallschläger, D., and Stadey, C. J. (2007). Determination of (oxy) thioarsenates in sulfidic waters. Anal. Chem. 79, 3873–3880. doi: 10.1021/ac070061g
Weisburg, W. G., Barns, S. M., Pelletier, D. A., and Lane, D. J. (1991). 16S ribosomal DNA amplification for phylogenetic study. J. Bacteriol. 173, 697–703. doi: 10.1128/jb.173.2.697-703.1991
Wilkin, R. T., Wallschläger, D., and Ford, R. G. (2003). Speciation of arsenic in sulfidic waters. Geochem. Trans. 4:1. doi: 10.1186/1467-4866-4-1
Wood, S. A., Tait, C. D., and Janecky, D. R. (2002). A Raman spectroscopic study of arsenite and thioarsenite species in aqueous solution at 25 C. Geochem. Trans. 3, 31–39. doi: 10.1186/1467-4866-3-31
Wu, G., Jiang, H., Dong, H., Huang, Q., Yang, J., Webb, L., et al. (2015). Distribution of arsenite-oxidizing bacteria and its correlation with temperature in hot springs of the Tibetan-Yunnan geothermal zone in western China. Geomicrobiol. J. 32, 482–493. doi: 10.1080/01490451.2014.938206
Yang, J., Zhou, E., Jiang, H., Li, W., Wu, G., Huang, L., et al. (2015). Distribution and diversity of aerobic carbon monoxide-oxidizing bacteria in geothermal springs of China, the Philippines, and the United States. Geomicrobiol. J. 32, 903–913. doi: 10.1080/01490451.2015.1008605
Yang, Y., Peng, Y. E., Chang, Q., Dan, C., Guo, W., and Wang, Y. (2016). Selective identification of organic iodine compounds using liquid chromatography–high resolution mass spectrometry. Anal. Chem. 88, 1275–1280. doi: 10.1021/acs.analchem.5b03694
Yokoyama, T., Takahashi, Y., and Tarutani, T. (1993). Simultaneous determination of arsenic and arsenious acids in geothermal water. Chem. Geol. 103, 103–111. doi: 10.1016/0009-2541(93)90294-S
Zargar, K., Conrad, A., Bernick, D. L., Lowe, T. M., Stolc, V., Hoeft, S., et al. (2012). ArxA, a new clade of arsenite oxidase within the DMSO reductase family of molybdenum oxidoreductases. Environ. Microbiol. 14, 1635–1645. doi: 10.1111/j.1462-2920.2012.02722.x
Zargar, K., Hoeft, S., Oremland, R., and Saltikov, C. W. (2010). Identification of a novel arsenite oxidase gene, arxA, in the haloalkaliphilic, arsenite-oxidizing bacterium Alkalilimnicola ehrlichii strain MLHE-1. J. Bacteriol. 192, 3755–3762. doi: 10.1128/jb.00244-10
Zhao, L., Dong, H., Kukkadapu, R., Agrawal, A., Liu, D., Zhang, J., et al. (2013). Biological oxidation of Fe (II) in reduced nontronite coupled with nitrate reduction by Pseudogulbenkiania sp. strain 2002. Geochim. Cosmochim. Acta 119, 231–247. doi: 10.1016/j.gca.2013.05.033
Keywords: thioarsenate, hot springs, anaerobic arsenite oxidation, arxA gene, sulfate-reducing bacterium
Citation: Wu G, Huang L, Jiang H, Peng Y, Guo W, Chen Z, She W, Guo Q and Dong H (2017) Thioarsenate Formation Coupled with Anaerobic Arsenite Oxidation by a Sulfate-Reducing Bacterium Isolated from a Hot Spring. Front. Microbiol. 8:1336. doi: 10.3389/fmicb.2017.01336
Received: 17 April 2017; Accepted: 30 June 2017;
Published: 14 July 2017.
Edited by:
Robert Duran, University of Pau and Pays de l’Adour, FranceReviewed by:
Ronald Oremland, United States Geological Survey, United StatesCopyright © 2017 Wu, Huang, Jiang, Peng, Guo, Chen, She, Guo and Dong. This is an open-access article distributed under the terms of the Creative Commons Attribution License (CC BY). The use, distribution or reproduction in other forums is permitted, provided the original author(s) or licensor are credited and that the original publication in this journal is cited, in accordance with accepted academic practice. No use, distribution or reproduction is permitted which does not comply with these terms.
*Correspondence: Hongchen Jiang, amlhbmdoQGN1Zy5lZHUuY24=
Disclaimer: All claims expressed in this article are solely those of the authors and do not necessarily represent those of their affiliated organizations, or those of the publisher, the editors and the reviewers. Any product that may be evaluated in this article or claim that may be made by its manufacturer is not guaranteed or endorsed by the publisher.
Research integrity at Frontiers
Learn more about the work of our research integrity team to safeguard the quality of each article we publish.