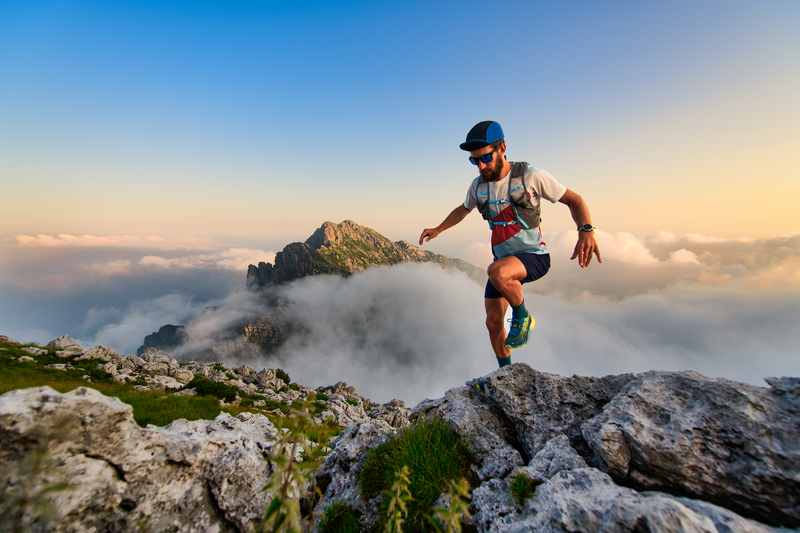
94% of researchers rate our articles as excellent or good
Learn more about the work of our research integrity team to safeguard the quality of each article we publish.
Find out more
ORIGINAL RESEARCH article
Front. Microbiol. , 16 June 2017
Sec. Microbiotechnology
Volume 8 - 2017 | https://doi.org/10.3389/fmicb.2017.01112
This article is part of the Research Topic Microbial Secondary Metabolites: Recent Developments and Technological Challenges View all 26 articles
The O2:CH4 ratio significantly effects nitrogen removal in mixed cultures where aerobic methane oxidation is coupled with denitrification (AME-D). The goal of this study was to investigate nitrogen removal of the AME-D process at four different O2:CH4 ratios [0, 0.05, 0.25, and 1 (v/v)]. In batch tests, the highest denitrifying activity was observed when the O2:CH4 ratio was 0.25. At this ratio, the methanotrophs produced sufficient carbon sources for denitrifiers and the oxygen level did not inhibit nitrite removal. The results indicated that the synergy between methanotrophs and denitrifiers was significantly improved, thereby achieving a greater capacity of nitrogen removal. Based on thermodynamic and chemical analyses, methanol, butyrate, and formaldehyde could be the main trophic links of AME-D process in our study. Our research provides valuable information for improving the practical application of the AME-D systems.
Biological denitrification, following nitrification, is widely used to remove nitrogen from wastewater. It includes four reduction steps: (1) from nitrate () to nitrite (), (2) from to nitric oxide (NO), (3) from NO to nitrous oxide (N2O) and (4) from N2O to dinitrogen (N2) (Zumft, 1997). Theoretically, and can be completely reduced to N2 in the presence of enough carbon sources if the microbes are equipped with full set of denitrification genes (Rittmann and McCarty, 2001). Among the four steps of denitrification, the reduction of soluble nitrite by nitrite reductase into gas is the key step and considered as the symbol of permanent removal of nitrogen from the aquatic ecosystem (Saunders and Kalff, 2001; Philippot and Hallin, 2005). Currently, carbons in the forms of methanol, ethanol and acetate are frequently supplemented for complete denitrification in wastewater treatment systems. However, the addition of external carbon sources inevitably increases the operational cost of wastewater treatment. Methane (CH4), a greenhouse gas, is readily available in many wastewater treatment plants and landfills and has a potential as an electron donor to replace traditional carbon sources for denitrification in nitrate-contaminated wastewater treatment (Modin et al., 2007). It was successfully demonstrated as a carbon source for denitrification in the presence of oxygen for the first time in 1978 (Rhee and Fuhs, 1978). This process was defined as aerobic methane oxidation coupled with denitrification (AME-D) (all the following discussions about this process are based on wastewater treatment systems). The AME-D process is a promising and realistic alternative to conventional biological treatment of nitrate-rich wastewaters. However, the nitrogen removal performance of AME-D systems is required to be higher than conventional systems for practical applications. Basic knowledge regarding operational parameters that affect the AME-D process is highly needed for the system process design.
The AME-D process is a synergistic collaboration between aerobic methanotrophs and denitrifying bacteria. Aerobic methanotrophs are a group of microorganisms capable of utilizing CH4 as a carbon and energy source. These microbes can oxidize CH4 to carbon dioxide (CO2) in the presence of oxygen (O2). Metabolic pathways of methane oxidation in aerobic methanotrophs are comprehensively summarized by other researchers (Trotsenko and Murrell, 2008; Zhu et al., 2016; Figure 1). Briefly, CH4 is initially catalyzed by methane monooxygenase (MMO), soluable MMO (sMMO) or particulate MMO (pMMO), to produce methanol as the first intermediate. Afterwards, methanol is transformed into formaldehyde by methanol dehydrogenase. Formaldehyde may be assimilated into biomass through the ribulose monophosphate pathway or the serine pathway, releasing multi-carbon intermediates such as acetate and citrate. Alternatively, formaldehyde can be dissimilated to CO2 via formate for energy production. It was demonstrated that dissolved organic intermediates, such as methanol (Meschner and Hamer, 1985), formaldehyde (Liu et al., 2014), acetate (Costa et al., 2000), which were released during aerobic methane oxidation, could be used as carbon sources for co-existing denitrifiers. On the metabolic pathways, denitrification in the AME-D process consists of two critical steps that may occur simultaneously or sequentially. The first is methane oxidation to release carbon sources, and the second is the use of these carbon compounds for denitrification (i.e., nitrate or nitrite removal). Therefore, operational parameters that affect the organic carbons excreted by methanotrophs for denitrification would have a critical impact on the nitrogen removal of AME-D process. Because of the well-known inhibition of excessive O2 on denitrification (Zumft, 1997), supply of enough electron donors and well-control of O2 level are two critical strategies to improve nitrogen removal and promote complete denitrification (--NO-N2O-N2) in the AME-D systems.
Figure 1. Traditional metabolism of methane in aerobic methanotrophs (modified from Zhu et al., 2016). pMMO, particulate methane monooxygenase; sMMO, soluble methane monooxygenase; MDH, methanol dehydrogenase; FDHa, formaldehyde dehydrogenase; FDHb, formate dehydrogenase; RuMP, ribulose monophosphate pathway. PQQ, pyrroloquinolinequinone; NAD+, oxidized form of nicotinamide-adenine dinucleotide; NADH, reduced form of nicotinamide-adenine dinucleotide.
It has been demonstrated that the O2:CH4 ratio is an essential parameter to regulate the carbon flow from CH4 to biomass and CO2, which impacts the production of carbon sources for nitrogen removal in the AME-D process. Morinaga et al. (1979) and Costa et al. (2000) discovered that methanotrophic strains could excrete formaldehyde and acetate, when the O2:CH4 ratio was lower than 1.0 (i.e., oxygen-limited). In contrast, no organic metabolites were detected when O2:CH4 ratio was higher than 1.0 (i.e., methane-limited). Kalyuzhnaya et al. (2013) further studied the pathway of intermediates production by aerobic methanotrophs under oxygen-limited conditions and discovered that acetate, lactate, and even hydrogen could be released during novel fermentation-related methanotrophy. Besides, oxygen is another critical factor to influence nitrogen removal in the AME-D process. Aerobic methanotrophs require enough O2 to utilize CH4, whereas excessive O2 will inhibit denitrifiers to reduce nitrogen (Modin et al., 2007). To date, environmental conditions that are favorable for both aerobic methanotrophs and denitrifiers are still unclear. Determination of an optimal O2:CH4 ratio which balances the requirements of carbon source and O2 could be an effective way for improving denitrifying performance in the AME-D process.
The aim of this research was to investigate the impact of the O2:CH4 ratio on nitrogen removal in the AME-D process and to determine the optimal ratio resulting in the highest nitrogen removal performance. It was hypothesized that intermediates released by methanotrophs primarily control nitrogen removal when O2:CH4 ratio is below the optimal value, whereas O2 level mainly controls nitrogen removal when O2:CH4 ratio is over the optimal. According to the stoichiometric (Equations 1 and 2) of the AME-D process (Zhu et al., 2016), the O2:CH4 ratio should be maintained below 1.1:1 (more specifically 0, 0.05, 0.25, and 1.0 in this study) to avoid excessive O2 inhibition on denitrification.
The sludge used in this study was collected from an AME-D culture that was enriched for more than 1 year in a batch bioreactor with continuous CH4 supply. The sludge was pre-incubated in a nitrite-containing medium under anoxic conditions in the dark at 25°C for 3 days to eliminate residual organic carbon sources. Then the sludge was centrifuged at 3,300 g for 5 min and the supernatant was discarded. The sludge pellet was resuspended and washed in sterile phosphate buffer solution (pH = 6.8) for three times to further remove extracellular organic carbons. Subsequently, it was centrifuged at higher speed of 14,000 g for 10 min to ensure complete precipitation of suspended cells. The experiments were initiated when no organic carbon was present in the supernatant. The organic carbon was determined with a Total Organic Carbon (TOC) analyzer (MultiN/C3100, Analytikjena, Germany). After all residual organics were removed, the sludge was re-suspended in the basal medium (Liu et al., 2014) at a mixed liquor suspended solid (MLSS) concentration of 17,735 mg/L. The basal medium contained the following components in 1 liter (L) distilled water: 1,250 mg KHCO3, 50 mg KH2PO4, 300 mg CaCl2·2H2O, 200 mg MgSO4·7H2O, 345 mg NaNO2, 1.0 mL acidic trace element solution and 1.0 mL alkaline trace element solution. Constituents of the acidic and alkaline trace element solution were described by Liu et al. (2014). Inorganic nitrogenous compounds were prepared from NaNO2 to result in a concentration of -N in the basal medium of 70 mg/L. Ammonium () and were also supplied at low concentrations, 0.07 and 3.82 mg/L, respectively, to serve as nitrogen sources for microbes in the AME-D system. The pH of the medium was 7.2.
Batch experiment was conducted using 10 mL of the prepared sludge and 20 mL of the basal medium in a 150 mL glass vial. Freshly prepared mixture of the basal medium and the sludge was sparged with CH4 (99.99%) for 5 min. The vial was crimp-sealed with a butyl rubber stopper. Different volumes of CH4 (0, 6, 24, and 60 mL) were withdrawn from the headspace of the vials with a gas-lock syringe. Afterwards, the same volume of pure O2 (99.99%) was injected into the headspace of vials. The final compositions of O2 and CH4 in the gas mixtures of each treatment were shown in Table 1. Un-inoculated media was used as blank controls to test for leakage and non-biological chemical transformations. Triplicate samples were incubated at 28°C in shaking incubator at 180 rpm in the dark for 60 h. CH4 and nitrogen (-N, -N and -N) consumptions and intermediate production were monitored in each treatment.
Table 1. Experimental set up for aerobic methane oxidation coupled with denitrification (AME-D) process.
The -N, -N, and -N concentrations were determined by ultraviolet spectrophotometry (UV-5300PC, METASH, China). The measurement of volatile suspended solid (VSS) was performed according to standard methods (American Public Health Association, American Water Works Association, Water Pollution Control Federation, 1989). In this study, nitrite removal expressed as mmol nitrite consumed per gram of biomass per day i.e., mmol -N/gVSS/d. To quantify CH4 content in the headspace of each vial, a sample of 0.5 mL was removed from the headspace of the vial with a gas-lock syringe. The sample was analyzed with a gas chromatograph (GC) equipped with a thermal conductivity detector under the conditions previously described by Zhang et al. (2014). Methane oxidation activity was expressed as CH4 consumed per gram of biomass per day i.e., mmol CH4/gVSS/d (Wang et al., 2008). NO concentration was measured by GC-mass spectrometry (6890N GC-5973MS, Agilent, USA) with the methods described by Leone et al. (1994). N2O concentration in the vial headspace was determined by a GC (GC-14B, Shimadzu, Japan) equipped with an electron capture detector and a Porapak Q column maintained at 330 K. Intermediate (methanol, formaldehyde, formate, acetate, and other potential organics) concentrations were determined with a high performance liquid chromatograph using the methods described by Thalasso et al. (1997).
Sludge samples were analyzed for DNA before and after incubation. Three independent DNA extractions of each treatment were performed from 30 mg of sludge using a FastDNA SPIN Kit for Soil (MP Biomedical, LLC, Ohio, USA). The extraction was performed according to the manufacturer's instructions. The concentrations and the quality of DNA samples were measured with a Nanodrop analyzer (Thermo Scientific, Wilmington, DE, USA). Extracted DNA was stored at −20°C prior to subsequent analyses.
The abundance of aerobic methanotrophs and denitrifiers was estimated through quantitative PCR (q-PCR). Due to the low level of mmoX-harboring methanotrophs in the enrichment and high enough Cu concentration (2 μM) in the medium to inhibit the expression of mmoX gene (Takeguchi et al., 1997), the function of sMMO encoded by mmoX gene was considered to be negligible. Therefore, only pmoA gene encoding a subunit of pMMO was used to investigate the abundance variation of aerobic methanotrophs in this study. For denitrifiers, the nirK gene encoding copper nitrite reductase and the nirS gene encoding cytochrome cd1-containing nitrite reductase were used as the biomarkers. The quantification was based on the intensity of SYBR Green dye fluorescence, which can bind to double-stranded DNA. Standard curve for each gene were generated using a 10-fold dilution series of the linearized plasmid standard (10−1–10−6 ng) ranging from 108 to 103 copies. Each qPCR assay (25 μL) included 12.5 μL of 2 × SYBR Premix Ex Taq (Takara, Dalian, China), 1 μL of each forward and reverse primer (20 μM), either 1 μL of template DNA or the standard vector plasmid of the clones grown as single cellular suspension. The optimized thermal conditions and primers used for each gene can be viewed in the Supplementary Table 1. All real-time PCR assays were performed in triplicate for each sample in a Bio-Rad CFX1000 Thermal Cycler. All PCR runs included negative controls that did not contain DNA templates. The gene copy numbers were determined by comparing threshold cycles obtained in each PCR run with those of known standard DNA concentrations. Standard curves were obtained using serial dilutions of linearized plasmids containing cloned pmoA, nirK, and nirS genes.
All data are presented as means and standard deviations. Analysis of variance and least significant difference (LSD) tests at the 5% level were used to determine the statistical significance of different treatments. Any differences with p ≥ 0.05 were not considered as statistically significant. The relationships between nirK/nirS gene copies and the nitrite removals at four treatments were tested with linear regression analyses using SPSS 20.0 for Windows (SPSS Inc., Chicago, IL).
Methane oxidation activity and concentrations of extracellular metabolites (methanol, formaldehyde, formate, and acetate, etc.) were determined under four different O2:CH4 ratios. As shown in Figure 2, methane oxidation activity substantially decreased from 277.80 mmol/gVSS/d to 21.06 mmol/gVSS/d when the O2:CH4 ratio was increased from 0 to 1 (p < 0.05). With the exception of treatment 1 at an O2:CH4 ratio of 0, a similar trend was observed for qPCR data of the pmoA gene. The pmoA gene abundance decreased almost an order of magnitude (from 9.36 × 109 to 1.63 × 109 copies per g dry biomass) when the O2:CH4 ratio was increased from 0.05 to 1 (Figure 2). The three primary metabolites observed in the bulk media were formaldehyde, acetate and citrate. Their concentrations went up from 42 to 76 μg/L (formaldehyde), 11 to 45 μg/L (acetate) and 0 to 28 μg/L (citrate), respectively, when O2:CH4 ratio was increased from 0 to 1 (Figure 3). Methanol, formate and butyrate were considered as three trace metabolites and their concentrations were lower than 1.20 μg/L (Supplementary Figure 1). However, methanol were not detected in the treatment 1 in the absence of O2.
Figure 2. Methane oxidation activities of the consortia and the abundance of pmoA gene at different O2:CH4 ratios in aerobic methane oxidation coupled with denitrification (AME-D) process. T1, Treatment 1 (0% O2 and 100% CH4); T2, Treatment 2 (5% O2 and 95% CH4); T3, Treatment 3 (20% O2 and 80% CH4); T4, Treatment 4 (50% O2 and 50% CH4).
Figure 3. Accumulation of acetate, citrate and formaldehyde in the liquid at different O2:CH4 ratios in aerobic methane oxidation coupled with denitrification (AME-D) process. T1, Treatment 1 (0% O2 and 100% CH4); T2, Treatment 2 (5% O2 and 95% CH4); T3, Treatment 3 (20% O2 and 80% CH4); T4, Treatment 4 (50% O2 and 50% CH4).
The concentrations of -N, -N and -N were measured at the beginning and the end of incubation. Results showed that concentrations of -N and -N decreased slightly by the end of the experiment and were detected in all final samples (Supplementary Table 2). There was a jump in the nitrite removal from 0.53 mmol -N/gVSS/d to 7.32 mmol -N/gVSS/d when the O2:CH4 ratio was increased from 0 to 0.25 (Figure 4). However, the nitrite removal decreased by 53.8% as the O2:CH4 ratio was increased from 0.25 to 1 (p < 0.05). The amount of reduced nitrogen released as NO was very low (0.13–0.32 μM; Table 2). In addition, the percentage of the reduced nitrogen emitted as N2O decreased from 37.96 to 12.30% when the O2:CH4 ratio increased from 0 to 0.25 (Table 2). This percentage at the O2:CH4 ratio of 1 was about 2 times higher than that at the O2:CH4 ratio of 0.25, while this difference was insignificant (p > 0.05; Table 2). The highest nitrite removal and the low percentage of NO-N and N2O-N in total reduced nitrogen were observed at the O2:CH4 ratio of 0.25. Based on the results, the O2:CH4 ratio of 0.25 was proposed as the optimal ratio for this AME-D system.
Figure 4. Nitrite removal of the consortia and the abundance of functional genes at different O2:CH4 ratios in aerobic methane oxidation coupled with denitrification (AME-D) process. T1, Treatment 1 (0% O2 and 100% CH4); T2, Treatment 2 (5% O2 and 95% CH4); T3, Treatment 3 (20% O2 and 80% CH4); T4, Treatment 4 (50% O2 and 50% CH4).
Table 2. Reduction of -N and accumulation of NO-N and N2O-N at different O2:CH4 ratios in aerobic methane oxidation coupled with denitrification (AME-D) process.
Nitrite reduction is catalyzed by nitrite reductase which are found in two different forms: copper nitrite reductase encoded by nirK gene and cytochrome cd1-containing nitrite reductase encoded by nirS gene (Wang et al., 2014). Investigating the difference in nirK and nirS gene abundance might provide further evidences for the variation of nitrite removal. The abundance of nirK gene was more than doubled as the O2:CH4 ratio was increased from 0 to 0.25 (from 3.47 × 1010 copies/gVSS up to 7.91 × 1010 copies/gVSS). However, it descended to 6.23 × 1010 copies/gVSS as the O2:CH4 ratio was further raised to 1. In contrast, copy numbers of nirS gene displayed no significant change (a slight increase from 1.82 copies/gVSS to 2.34 × 1011 copies/gVSS) when O2:CH4 ratio was increased from 0 to 0.25. However, it went down significantly to 7.10 × 1010 copies/gVSS at higher O2:CH4 ratio of 1. In addition, the linear correlation analysis revealed that nirK gene copies were positively correlated with nitrite removal (r2 = 0.8639, p = 0.0707), whereas nirS gene copies had a slightly negative correlation with the nitrite removal (r2 = 0.0121, p = 0.8899; Figure 5). If the nitrite removal was considered as the representation of the denitrifying conditions in the corresponding treatment, the higher nitrite removal indicated the better denitrifying conditions in this treatment. Thereby, the linear coorelation analyses suggest that nirK-type denitrifiers might be more responsive to the denitrifying conditions than nirS-type denitrifiers (Yoshida et al., 2010).
Figure 5. The relationships between nitrite removal and the abundance of nirK/nirS genes in aerobic methane oxidation coupled with denitrification (AME-D) process. Linear regressions were used to test the correlation between nitrite removal and the abundance of nirK/nirS genes.
The results from the batch experiment showed that the peak nitrite removal (7.32 mmol -N/gVSS/d) was obtained at an O2:CH4 ratio of 0.25. As -N and -N are the preferred microbial nitrogen sources (Modin et al., 2007), their presence throughout the incubation period (Supplementary Table 2) indicated that nitrite was negligibly consumed as a nitrogen source for assimilation. Therefore, nitrite removal from the media was through dissimilatory, i.e., denitrification. Additionally, the low percentage of the consumed -N emitted as N2O-N (12.30%) and NO-N (0.20%, Table 2) at the O2:CH4 ratio of 0.25 indicated that enough carbon sources provided by the methanotrophs may allow denitrifiers to reduce almost 87.5% of -N through complete denitrification. At a lower O2:CH4 ratio of 0.25, it was consistent with our hypothesis that nitrite removal were not inhibited by O2, and were stimulated by the increased carbon provided by methanotrophic metabolism. However, nitrite removal was less effective at higher O2:CH4 ratios with higher oxygen concentrations, which was corroborated by the lower abundance of denitrifying genes (Figure 4).
The conclusion that nitrite removal was stimulated by carbon sources released from aerobic methane oxidation at a O2:CH4 ratio lower than or equal to 0.25 could be supported by the variation of intermediate concentrations among four treatments. Concentrations of accumulated intermediates were higher and higher in the bulk media with the O2:CH4 ratios increasing from 0 to 0.25 (Figure 3). It may be attributed to the increased O2 concentration in these treatments. Morinaga et al. (1979) and Costa et al. (2001) demonstrated that the O2 level would significantly impact the consumption and production of metabolites in methane metabolism. However, the effect was not unambiguously determined. Morinaga et al. (1979) observed formaldehyde accumulation under oxygen-limited conditions, whereas Costa et al. (2001) discovered that formaldehyde accumulated under oxygen-excessive conditions. Our results indicated that the increased oxygen level promoted intermediates accumulation in methane metabolism. Low level of available carbon sources for denitrifiers in Treatment 1 resulted in the lowest nitrite removal. Once O2 was largely induced in the headspace in Treatment 2 and 3, concentrations of intermediates increased in the liquid bulk. The observation of simultaneous higher nitrite removal and lower ratio of accumulated N2O-N:consumed -N indicated that complete denitrifying activity was improved by these accumulated intermediates.
The ability of denitrifiers to resist the inhibition of O2 at the optimal O2:CH4 ratio may be due to two reasons. Firstly, the abundance of nirK-harboring denitrifiers was the highest among the four treatments and these denitrifiers are able to tolerate higher O2 levels (Desnues et al., 2007). Secondly, the sludge aggregation/granulation in anoxic micro-environments can lessen the O2 exposure of the denitrifiers. As shown in Figure 6, at the O2:CH4 ratio of 0.25, suspended biomass formed granule-like agglomerates with an average diameter of about 2 mm, while such effect did not occur for the O2:CH4 ratios of 0, 0.05, and 1. Sludge aggregation has also been observed in an AME-D system with an optimized O2 level that had the highest nitrogen removal rate (Thalasso et al., 1997). It can be therefore concluded that sludge aggregation at an optimal O2:CH4 ratio could improve nitrite removal. The spatial distribution of microorganisms and O2 level within the aggregates should be investigated in the future.
Figure 6. Configuration of activated sludge in four treatments at the end of incubation during aerobic methane oxidation coupled with denitrification (AME-D) process. The phenomenon of aggregation is apparent in treatment 3 (T3) while activated sludge in other treatments were dispersive. T1, Treatment 1 (0% O2 and 100% CH4); T2, Treatment 2 (5% O2 and 95% CH4); T3, Treatment 3 (20% O2 and 80% CH4); T4, Treatment 4 (50% O2 and 50% CH4).
With regard to the effect of the O2:CH4 ratio on denitrification, Sun et al. (2013) observed a similar phenomenon that the O2:CH4 ratio affected nitrogen removal of AME-D process in a membrane biofilm bioreactor (MBfR). Their optimal O2:CH4 ratio of 1.5 was considerably higher than the one in this study. It is likely that the spatial arrangement of the microbial community within a well-developed biofilm would allow for greater tolerance to O2, as compared to a suspended culture. They speculated that greater metabolite excretion by the methanotrophs improved nitrate removal performance initially, and that excessive O2 caused the significant drop in the denitrifying rate when O2:CH4 ratio increased to 2.0. The results from this study corroborate the trend that Sun et al. (2013) observed.
Methane oxidation activity dramatically decreased when O2:CH4 ratio increased from 0 to 1 (Figure 2). It was probably due to the large change in methane availability. Li et al. (2014) observed a similar trend with methanotrophic activity of landfill cover soils. The methane oxidation activity at O2:CH4 ratio of 0.25 (4 × 104 ppmv O2/2 × 105 ppmv CH4) was almost 4-fold higher than that at O2:CH4 ratio of 4.00 (2 × 105 ppmv O2/5 × 104 ppmv CH4) (Supplementary Table 3). In their research, methane oxidation activity was much more sensitive to CH4 than O2, and a drop of CH4 concentration would result in a simultaneous decrease of methane oxidation activity. Additionally, copy numbers of the pmoA gene decreased as the methane concentration decreased (Baani and Liesack, 2008; Li et al., 2014). In the current research, CH4 concentration declined from 100 to 50% and O2 concentrations rose from 0 to 50% for the ratios tested. This suggests that a decrease in methane oxidation activity with a substantial increase in O2:CH4 ratio is plausible. However, it is unexpected that the high pmoA gene copy numbers and methane oxidation activity were observed in Treatment 1 in the absence of O2. Rechecking the gaseous composition of the headspace in this treatment at the beginning of the incubation, it was discovered that trace amount (0.012%) of O2 can still be detected after the sludge was sparged with pure CH4 (99.99%). This trace level of O2 may result in the observed CH4 consumption. However, further investigations are still required to focus on examining if anaerobic methane oxidation contributed to CH4 consumption when this trace amount of O2 was depleted.
Acetate, citrate, and formaldehyde were detected as three primary compounds detected during the AME-D process, while methanol, formate, and butyrate occurred in trace quantities. It is difficult to postulate which were the main substrates for the denitrifiers as their levels of consumption relative to production were not known. However, thermodynamic analysis of AME-D process may provide useful information for speculating the actual metabolic pathway (all of the following thermodynamic analyses are based on aerobic methane oxidation coupled with complete denitrification).
Methanol is considered as the most effective intermediate for denitrification according to the review of the AME-D process (Zhu et al., 2016). The reactions (Equations 3–5) related to energy production contained in AME-D process using as the denitrifying electron acceptor were shown in Table 3. These equations are based on one electron equivalent (eeq). Assuming that at least X (<1) mol of methanol is needed by aerobic methanotrophs for their requirement of cell synthesis and maintenance when one mole of CH4 is oxidized to methanol, the remaining part of methanol can be used for denitrification as the electron donor. An energy-balanced equation for the AME-D process can be described as Equation (6).
Table 3. Stoichiometric equations in aerobic methane oxidation coupled with denitrification (AME-D) process based on theoretical hypotheses.
Where ε, is the energy transfer efficiency a value of 0.37 (McCarty, 2007). [ΔGEq.(3) – 0.5ΔGxy] denotes the net energy production for the oxidation from CH4 to methanol after considering the energy input for the mono-oxygenase and the required reducing equivalent, which equals to 47.26 kJ/eeq. ΔGm represents the minimum maintenance energy requirement with a value of about 8.1 kJ/mol oxidized CH4 (Modin et al., 2007). The exhaustive description of calculation processes for [ΔGEq.(3) – 0.5ΔGxy] and ΔGm is presented by Zhu et al. (2016). From Equation (6), the value derived for X is 0.40. Because the energy required by cell synthesis has not been considered during the above calculation, the theoretical maximum quantity of methanol used by denitrifiers was 0.60 mol. This means that the maximum proportion of methanol that can be captured by denitrifiers is 60%. The overall reaction of AME-D process in the presence of was described as Equation (7), a combination of the proposed value and Equations (3–5).
All six substrates detected in this study were potential carbon sources for denitrifiers. In order to understand which substrates were likely the functional intermediates, thermodynamic analysis was performed based on the chemical data associated with the O2:CH4 ratio of 0.25. During the process of thermodynamic derivation, all of organics detected in the bulk liquid were individually chosen as the trophic link of aerobic methane oxidation and denitrification. After several iterations, the same general equation (Equation 8) for the AME-D process, which was in agreement with CH4 and consumption was obtained through three different approaches:
(1) 4.34% of consumed CH4-C flowed to denitrifiers using methanol as the trophic link;
(2) 5.22% of consumed CH4-C flowed to denitrifiers using butyrate as the trophic link;
(3) 6.52% of consumed CH4-C flowed to denitrifiers using formaldehyde as the trophic link. This means that methanol, butyrate and formaldehyde released by aerobic methanotrophs were three possible intermediates that could be used as carbon sources by denitrifiers at an O2:CH4 ratio of 0.25. However, acetate could not be an active carbon source under this condition in our study, although it was a feasible electron donor with highest denitrifying potential (Hallin and Pell, 1998). This conclusion is further supported by the increased copy numbers of nirK genes, which would have decreased if acetate was the main active electron donor for denitrifiers (Li et al., 2015).
Based on the above analysis, it was evident that the percentage of carbon flow from methane to denitrification (4.34–6.52%) was much lower than the ideal flow (60%). Further improvement in the carbon flow is vital to enhance the AME-D denitrification rates.
The effect of the O2:CH4 ratio was demonstrated to significantly impact nitrogen removal during the AME-D process through contribution of the carbon metabolites generated by the methanotrophs and oxygen inhibition. To date, most studies have considered only the individual impact of O2 on the apparent nitrogen removal rate of the AME-D process (Werner and Kayser, 1991; Thalasso et al., 1997; Modin et al., 2010), whereas the combined effect of O2 and CH4 and associated mechanisms are rarely investigated. It is necessary to address the impact of O2:CH4 ratio on CH4 and / metabolism in the AME-D process, rather than the tendency of methane oxidation rates and denitrifying activities under different gaseous environments. The knowledge will allow a better understanding of the specific roles of the O2:CH4 ratio in CH4 and / metabolism. It is expected that this will contribute to well-founded strategies that will improve nitrogen removal, one of bottle-necks in the application of the AME-D process.
In this study, the optimal O2:CH4 ratio for denitrification was found to be 0.25. At this point, denitrifying activity reached the highest level of 7.32 mmol -N/gVSS/d. When the O2:CH4 ratio was below the optimal ratio, nitrite removal was improved with the increased O2:CH4 ratio, presumably due to an increase in available substrates released by aerobic methanotrophs. Methanol, butyrate and formaldehyde were thermodynamically speculated as the main active intermediates of the AME-D process. When the O2:CH4 ratio was above the optimal ratio, nitrite removal was presumably inhibited by the excessive O2. These results indicate that adjusting the O2:CH4 ratio can improve the cooperation between aerobic methanotrophs and denitrifiers to obtain better nitrogen removal performance using the AME-D process.
JZ: contributed to the conception, experimental design, acquisition, analysis, and interpretation of data, and article drafting; XX, MY: analyzed and interpreted data; HW, ZM: contributed to data acquisition; WW: supervised the student and revised the article.
The authors declare that the research was conducted in the absence of any commercial or financial relationships that could be construed as a potential conflict of interest.
We would like to express our appreciation to Dr. P. J. Strong from the Centre for Solid Waste Bioprocessing in the University of Queensland, Prof. Jiayang Cheng from Biological and Agricultural Engineering Department of North Carolina State University, Dr. Faqian sun, Dr. Cheng Wang, Dr. Pengfei Liu, PhD student Xingguo Han and Christina Horn for their help to improve the quality of this manuscript. We additionally thank the reviewers for their valuable suggestions. This work was supported by the China National Critical Project for Science and Technology on Water Pollution and Control under No. 2014ZX07101-012.
The Supplementary Material for this article can be found online at: http://journal.frontiersin.org/article/10.3389/fmicb.2017.01112/full#supplementary-material
American Public Health Association, American Water Works Association, Water Pollution Control Federation (1989). Standard Methods for the Examination of Water and Wastewater, 17th Edn. Washington, D. C: American Water Works Association, Water Pollution Control Federation.
Baani, M., and Liesack, W. (2008). Two isozymes of particulate methane monooxygenase with different methane oxidation kinetics are found in Methylocystis sp. strain SC2. Proc. Natl. Acad. Sci. U.S.A. 105, 10203–10208. doi: 10.1073/pnas.0702643105
Costa, C., Dijkema, C., Friedrich, M., Garcia-Encina, P., Fernandez-Polanco, F., and Stams, A. J. (2000). Denitrification with methane as electron donor in oxygen-limited bioreactors. Appl. Microbiol. Biotechnol. 53, 754–762. doi: 10.1007/s002530000337
Costa, C., Vecherskaya, M., Dijkema, C., and Stams, A. J. M. (2001). The effect of oxygen on methanol oxidation by an obligate methanotrophic bacterium studied by in vivo13C nuclear magnetic resonance spectroscopy. J. Ind. Microbiol. Biot. 26, 9–14. doi: 10.1038/sj.jim.7000075
Desnues, C., Michotey, V. D., Wieland, A., Zhizang, C., Fourcans, A., Duran, R., et al. (2007). Seasonal and diel distributions of denitrifying and bacterial communities in a hypersaline microbial mat (Camargue, France). Water Res. 41, 3407–3419. doi: 10.1016/j.watres.2007.04.018
Hallin, S., and Pell, M. (1998). Metabolic properties of denitrifying bacteria adapting to methanol and ethanol in activated sludge. Water Res. 32, 13–18. doi: 10.1016/S0043-1354(97)00199-1
Kalyuzhnaya, M. G., Yang, S., Rozova, O. N., Smalley, N. E., Clubb, J., Lamb, A., et al. (2013). Highly efficient methane biocatalysis revealed in a methanotrophic bacterium. Nat. Commun. 4:2785. doi: 10.1038/ncomms3785
Leone, A. M., Gustafsson, L. E., Francis, P. L., Persson, M. G., Wiklund, N. P., and Moncada, S. (1994). Nitric oxide is present in exhaled breath in humans: direct GC-MS confirmation. Biochem. Bioph. Res. Commun. 201, 883–887. doi: 10.1006/bbrc.1994.1784
Li, C., Cao, J. S., Ren, H. Q., Li, Y., and Tang, S. Y. (2015). Comparison on kinetics and microbial community among denitrification process fed by different kinds of volatile fatty acids. Process Biochem. 50, 447–455. doi: 10.1016/j.procbio.2015.01.005
Li, H., Chi, Z., Lu, W., and Wang, H. (2014). Sensitivity of methanotrophic community structure, abundance, and gene expression to CH4 and O2 in simulated landfill biocover soil. Environ. Pollut. 184, 347–353. doi: 10.1016/j.envpol.2013.09.002
Liu, J., Sun, F., Wang, L., Ju, X., Wu, W., and Chen, Y. (2014). Molecular characterization of a microbial consortium involved in methane oxidation coupled to denitrification under micro-aerobic conditions. Microb. Biotechnol. 7, 64–76. doi: 10.1111/1751-7915.12097
McCarty, P. L. (2007). Thermodynamic electron equivalents model for bacterial yield prediction: modifications and comparative evaluations. Biotechnol. Bioeng. 97, 377–388. doi: 10.1002/bit.21250
Meschner, K. L., and Hamer, G. (1985). “Denitrification by methanotrophic/metylotrophic bacterial associations in aquatic environments,” in Denitrification in the Nitrogen Cycle, ed H. L. Golterman (New York, NY: Plenum Press), 257–271.
Modin, O., Fukushi, K., Nakajima, F., and Yamamoto, K. (2010). Aerobic methane oxidation coupled to denitrification: kinetics and effect of oxygen supply. J. Environ. Eng-Asce. 136, 211–219. doi: 10.1061/(ASCE)EE.1943-7870.0000134
Modin, O., Fukushi, K., and Yamamoto, K. (2007). Denitrification with methane as external carbon source. Water Res. 41, 2726–2738. doi: 10.1016/j.watres.2007.02.053
Morinaga, Y., Yamanaka, S., Yoshimura, M., Takinami, K., and Hirose, Y. (1979). Methane metabolism of the obligate methane-utilizing bacterium, Methylomonas Flagellata, in methane-limited and oxygen-limited chemostat culture. Agric. Biol. Chem. Tokyo 43, 2453–2458.
Philippot, L., and Hallin, S. (2005). Finding the missing link between diversity and activity using denitrifying bacteria as a model functional community. Curr. Opin. Microbiol. 8, 234–239. doi: 10.1016/j.mib.2005.04.003
Rhee, G. Y., and Fuhs, G. W. (1978). Wastewater denitrification with one-carbon compounds as energy-source. J. Water Pollut. Control Fed. 50, 2111–2119.
Rittmann, B. E., and McCarty, P. L. (2001). Environmental Biotechnology: Principles and Applications. New York, NY: McGraw-Hill Book Co.
Saunders, D. L., and Kalff, J. (2001). Nitrogen Retention in Wetlands, Lakes and Rivers. Hydrobiologia 443, 205–212. doi: 10.1023/A:1017506914063
Sun, F. Y., Dong, W. Y., Shao, M. F., Lv, X. M., Li, J., Peng, L. Y., et al. (2013). Aerobic methane oxidation coupled to denitrification in a membrane biofilm reactor: treatment performance and the effect of oxygen ventilation. Bioresour. Technol. 145, 2–9. doi: 10.1016/j.biortech.2013.03.115
Takeguchi, M., Furuto, T., Sugimori, D., and Okura, I. (1997). Optimization of methanol biosynthesis by Methylosinus trichosporium OB3b: an approachto improve methanol accumulation. Appl. Biochem. Biotech. 68, 143–152. doi: 10.1007/BF02785987
Thalasso, F., Vallecillo, A., GarciaEncina, P., and FdzPolanco, F. (1997). The use of methane as a sole carbon source for wastewater denitrification. Water Res. 31, 55–60. doi: 10.1016/S0043-1354(96)00228-X
Trotsenko, Y. A., and Murrell, J. C. (2008). Metabolic aspects of aerobic obligate methanotrophy. Adv. Appl. Microbiol. 63, 183–229. doi: 10.1016/S0065-2164(07)00005-6
Wang, Y. L., Wu, W. X., Ding, Y., Liu, W., Perera, A., Chen, Y. X., et al. (2008). Methane oxidation activity and bacterial community composition in a simulated landfill cover soil is influenced by the growth of Chenopodium album L. Soil Biol. Biochem. 40, 2452–2459. doi: 10.1016/j.soilbio.2008.06.009
Wang, Z., Zhang, X. X., Lu, X., Liu, B., Li, Y., Long, C., et al. (2014). Abundance and diversity of bacterial nitrifiers and denitrifiers and their functional genes in tannery wastewater treatment plants revealed by high-throughput sequencing. PLoS ONE 9:0113603. doi: 10.1371/journal.pone.0113603
Werner, M., and Kayser, R. (1991). Denitrification with biogas as external carbon source. Water Sci. Technol. 23, 701–708.
Yoshida, M., Ishii, S., Otsuka, S., and Senoo, K. (2010). nirK-harboring denitrifiers are more responsive to denitrification-inducing conditions in rice paddy soil than nirS-harboring bacteria. Microbes Environ. 25, 45–48. doi: 10.1264/jsme2.ME09160
Zhang, X., Kong, J. Y., Xia, F. F., Su, Y., and He, R. (2014). Effects of ammonium on the activity and community of methanotrophs in landfill biocover soils. Syst. Appl. Microbiol. 37, 296–304. doi: 10.1016/j.syapm.2014.03.003
Zhu, J., Wang, Q., Yuan, M. D., Tan, G. Y., Sun, F., Wang, C., et al. (2016). Microbiology and potential applications of aerobic methane oxidation coupled to denitrification (AME-D) process: a review. Water Res. 90, 203–215. doi: 10.1016/j.watres.2015.12.020
Keywords: aerobic methane oxidation, denitrification, O2:CH4 ratio, intermediate accumulation, thermodynamics
Citation: Zhu J, Xu X, Yuan M, Wu H, Ma Z and Wu W (2017) Optimum O2:CH4 Ratio Promotes the Synergy between Aerobic Methanotrophs and Denitrifiers to Enhance Nitrogen Removal. Front. Microbiol. 8:1112. doi: 10.3389/fmicb.2017.01112
Received: 25 December 2016; Accepted: 31 May 2017;
Published: 16 June 2017.
Edited by:
Wen-Jun Li, Sun Yat-sen University, ChinaReviewed by:
Binbin Liu, Centre of Agricultural Resources Research (CAS), ChinaCopyright © 2017 Zhu, Xu, Yuan, Wu, Ma and Wu. This is an open-access article distributed under the terms of the Creative Commons Attribution License (CC BY). The use, distribution or reproduction in other forums is permitted, provided the original author(s) or licensor are credited and that the original publication in this journal is cited, in accordance with accepted academic practice. No use, distribution or reproduction is permitted which does not comply with these terms.
*Correspondence: Weixiang Wu, d2VpeGlhbmdAemp1LmVkdS5jbg==
Disclaimer: All claims expressed in this article are solely those of the authors and do not necessarily represent those of their affiliated organizations, or those of the publisher, the editors and the reviewers. Any product that may be evaluated in this article or claim that may be made by its manufacturer is not guaranteed or endorsed by the publisher.
Research integrity at Frontiers
Learn more about the work of our research integrity team to safeguard the quality of each article we publish.