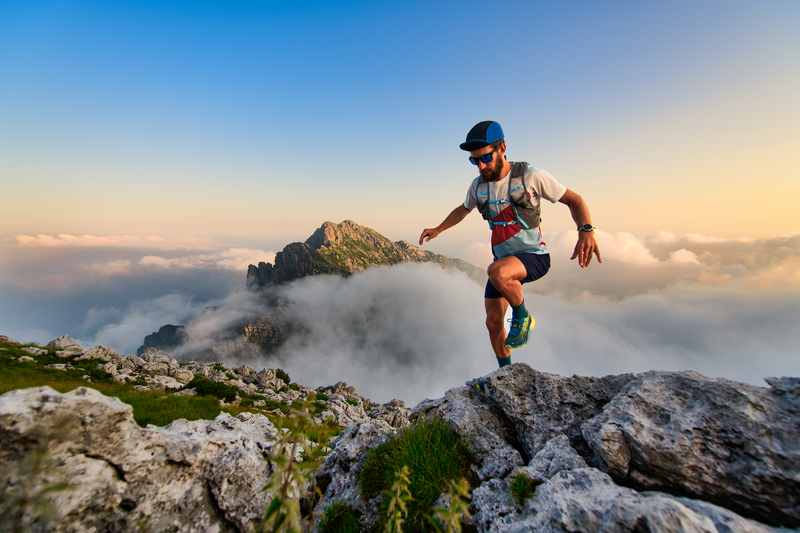
94% of researchers rate our articles as excellent or good
Learn more about the work of our research integrity team to safeguard the quality of each article we publish.
Find out more
ORIGINAL RESEARCH article
Front. Microbiol. , 15 June 2017
Sec. Microbiotechnology
Volume 8 - 2017 | https://doi.org/10.3389/fmicb.2017.01105
Aiming to comprehensively survey the potential pollution of an alpine cryoconite (Jamtalferner glacier, Austria), and its bacterial community structure along with its biodegrading potential, first chemical analyses of persistent organic pollutants, explicitly polychlorinated biphenyls (PCBs) and organochlorine pesticides (OCPs) as well as polycyclic aromatic hydrocarbons (PAHs), revealed a significant contamination. In total, 18 PCB congeners were detected by high resolution gas chromatography/mass spectrometry with a mean concentration of 0.8 ng/g dry weight; 16 PAHs with an average concentration of 1,400 ng/g; and 26 out of 29 OCPs with a mean concentration of 2.4 ng/g. Second, the microbial composition was studied using 16S amplicon sequencing. The analysis revealed high abundances of Proteobacteria (66%), the majority representing α-Proteobacteria (87%); as well as Cyanobacteria (32%), however high diversity was due to 11 low abundant phyla comprising 75 genera. Biodegrading potential of cryoconite bacteria was further analyzed using enrichment cultures (microcosms) with PCB mixture Aroclor 1242. 16S rDNA analysis taxonomically classified 37 different biofilm-forming and PCB-degrading bacteria, represented by Pseudomonas, Shigella, Subtercola, Chitinophaga, and Janthinobacterium species. Overall, the combination of culture-dependent and culture-independent methods identified degrading bacteria that can be potential candidates to develop novel bioremediation strategies.
Most of our biosphere is permanently cold (Morita, 1975; Russell et al., 1990; Margesin and Miteva, 2011). Among these cold environments, snow and glaciers permanently or seasonally cover up to 35% of the Earth’s terrestrial surface area (Miteva, 2008; Anesio and Laybourn-Parry, 2012). In areas of bare glacier ice, the most common water reservoirs are small supraglacial melt depressions known as “cryoconite holes” (Wharton et al., 1985; Southwell, 2014; Takeuchi, 2014). Cryoconites, continuously reported from glaciers worldwide, e.g., Alps, Arctic, Antarctic, Greenland, and Himalayas (Wharton et al., 1981; Kohshima, 1987; De Smet and Van Rompu, 1994; Margesin et al., 2002), cover up to 6% of the glacier surface (Fountain et al., 2004). Cryoconites are presumed to be “ice-cold hot-spots of microbial diversity and activity” (Edwards et al., 2013a) as they are the most biologically active habitats within glacial ecosystems (Säwström et al., 2002). Thus, they provide an ideal environment for a diverse variety of psychrophilic microorganisms, including bacteria, algae, viruses and yeasts (Margesin et al., 2002; Christner et al., 2003; Anesio et al., 2007). Until today, studying cryoconite microbial communities using molecular tools has mainly focused on polar regions (Christner et al., 2003; Foreman et al., 2007; Edwards et al., 2011, 2013a,b; Cameron et al., 2012a,b; Zarsky et al., 2013; Stibal et al., 2015), whereas generally less is known about the microbial diversity of cryoconites on alpine glaciers. Edwards et al. (2013a) reported on a metagenome assembly from cryoconites on the Rotmoosferner in the Austrian Alps, which was dominated by the bacterial phyla Proteobacteria, Bacteroidetes, and Actinobacteria followed by Cyanobacteria. In addition, further comparative studies revealed that cryoconite communities differ due to the properties of the cryoconite debris (Telling et al., 2012) and in relation to glacier-specific factors (Edwards et al., 2011, 2013a,b).
Furthermore, glacial ecosystems are subjected to the fallout and accumulation of black carbon (BC) as well as chemical pollutants, and are thus important indicators of pollution events. The presence of incorporated organic environmental toxins, so called persistent organic pollutants (POPs), such as polychlorinated biphenyls (PCBs) and organochlorine pesticides (OCPs) as well as polycyclic aromatic hydrocarbons (PAHs) is often associated to atmospheric deposition and transport path of air masses (Bizzotto et al., 2009). It was shown that BC offers the most important binding phases for PAHs and PCBs in the environment (Lohmann et al., 2005; Koelmans et al., 2006; Bond et al., 2013), thereby connecting the global cycle of POPs to that of BC. Many POPs and PAHs are carcinogenic and suspected to disturb the development of humans and animals (Koelmans et al., 2006). Additionally, they are long-lasting and can be transported via the atmosphere over long distances. The snowmelt transports these chemicals for years into glacier lakes, where they accumulated in the sediment. Thus, glaciers are secondary sources for re-entry of POPs in the environment (Villa et al., 2003, 2004, 2006). Chemical analyses detected concentrations of up to 43 ng/L for PCBs and up to 168 μg/L for PAHs in contaminated glacial areas (Cappa et al., 2014). From an ecological perspective, glaciers are low-carbon ecosystems, where most of the existing organic carbon is derived from allochthonous inputs (Stibal et al., 2008). Accumulation of organic pollutants can represent a relevant source of nutrients, and thus potentially a hotspot for hydrocarbon-degrading microorganisms (Megharaj et al., 2011). Hydrocarbon-degrading microorganisms comprise less than 0.1% of the microbial communities in uncontaminated environments. In contrast, these organisms can constitute over 90% of the viable microorganisms in polluted ecosystems (Atlas, 1981). Numerous studies have demonstrated the capacity of microorganisms to efficiently degrade a wide range of hydrocarbons, phenol, phenol-related compounds, and petroleum hydrocarbons (Aislabie et al., 1998; Bej et al., 2000; Baraniecki et al., 2002; Samanta et al., 2002; Bergauer et al., 2005; Margesin, 2007; Margesin et al., 2007; Stibal et al., 2012; Kuddus et al., 2013). Thus, bacteria thriving in cold environments, especially in the highly bioactive cryoconites, represent considerable candidates for low-temperature bioremediation besides their key ecological role for nutrient cycling (Margesin, 2007). The potential of such hydrocarbon-degrading microorganisms has already led to the development of bioremediation techniques for contaminated soil and water, but nowadays these procedures are still challenging (Dua et al., 2002).
The present study aimed to identify the microbial community structure of a cryoconite on the alpine Jamtalferner glacier in Austria by focusing on the presence of bacteria regardless of other microorganisms such as fungi and archaea. Analytic measurements of a broad spectrum of harmful compounds, namely PCBs, PAHs and OCPs, were examined to quantify the presence of organic contaminants in the cryoconite. A combination of culture-dependent and culture-independent methods was performed to identify potential biodegrading organisms, which might be relevant for the development of novel bioremediation strategies.
Sampling was performed in September 2006 on Jamtalferner (47.51°N, 10.09°E), a medium sized valley glacier at the southern margin of Jam valley in the Silvretta group near the “Drei-Ländereck” in Austria. It covers an area of 3.5 km2 over a length of about 2.4 km. The lowest part of the glacier is located at an altitude of 2,420 m, the highest at Hintere Jamspitze (3,156 m). The cryoconite sample was collected near the glacier base at 2,700 m above sea level (see Figure 1) using a sterile 500 mL polyethylene terephthalate (PET) bottle and immediately transferred to the lab. The sample was aliquoted into 1 mL in polypropylene (PP) reaction tubes and frozen at -80°C. The sample contained both, cryoconite meltwater and detritus. All subsequent experiments mentioned below were performed within weeks after sampling.
FIGURE 1. Sampling site. (A) Study location on glacier Jamtalferner in Austria. (B) Section of a compass map shows exact sampling site (C) Cryoconite on Jamtalferner, scale bar 8 cm.
The cryoconite sample (10 g wet weight) was slowly thawed on ice before metagenomic DNA was extracted by direct lysis after a modified protocol by Henne et al. (1999) described in detail in Weiland et al. (2010) and Weiland-Bräuer et al. (2017). Total metagenomic DNA was further purified using a Qiagen-tip 100 (Qiagen Plasmid Midi Kit; Qiagen, Hilden, Germany). High-molecular-weight genomic DNA of enriched cultures was isolated from 5 mL overnight cultures using the AquaPure Genomic DNA Kit (Bio-Rad, Munich, Germany).
16S rRNA genes were PCR amplified from 10 ng isolated genomic DNA using the bacteria-specific primer 27F (5′-AGAGTTTGATCCTGGCTCAG-3′) and the universal primer 1492R (5′-GGTTACCTTGTTACGACTT-3′) (Lane, 1991), resulting in a 1.5 kbp PCR fragment. The fragment was cloned into pCRII-TOPO (Invitrogen, Karlsruhe, Germany). DNA sequences were determined by the sequencing facility at the Institute of Clinical Molecular Biology, University of Kiel, Kiel, Germany (IKM) using primer set 27F and 1492R or primer 27F singly.
DNA sequences were taxonomically classified using BLAST network service in the database of NCBI to determine their approximate phylogenetic affiliations. Tree reconstruction was performed with partial or full length 16S rRNA sequences using phylogeny.fr (Dereeper et al., 2008, 2010) with the Maximum-Likelihood method. Sequences were submitted to the NCBI database; accession numbers KT924431–KT924439 and KT931670–KT931706 (see Supplementary Table S2).
Primers used to construct the amplicon library were of the structure 5′-[Roche’s adaptor for long reads (Lib-L)] – [template-specific sequence]-3′. As template specific sequences, forward primer F338 (5′-ACTCCTACGGRAGGCAGCAG-3′) and reverse primer R802 (5′-TACNVGGGTATCTAATCC-3′) were used in a PCR amplification of the V3–V4 hypervariable region of the 16S rRNA gene (Dethlefsen et al., 2008; Claesson et al., 2010). Amplifications were conducted in two duplicate reactions of 50 μL, each containing 20 ng of template DNA and the GoTaq DNA Polymerase kit (Promega, Madison, WI, United States). Cycling conditions were: 94°C for 10 min, followed by 30 cycles of 94°C for 1 min, 44°C for 1 min and 72°C for 1 min, with a final step at 72°C for 10 min. The duplicate reactions were combined, amplicons size-checked and purified using MinElute Gel Extraction kit (Qiagen, Hilden, Germany). Purified amplicons were quantified using Quant-iT PicoGreen kit (Invitrogen, Darmstadt, Germany) and pooled to equimolar amounts. Pyrosequencing was carried out according to the manufacturer’s instructions using the GS FLX Titanium series kit (Sequencing Kit XLR70, Pico Titer Plate Kit 70 × 75, SV emPCR Kit/Lib-A, Maintenance Wash Kit; Roche, Mannheim, Germany) and 1/8 plate was sequenced on a Roche 454 GS-FLX Titanium platform at the IKM in 2007.
Sequence processing was conducted with mothur v1.35.1 (Schloss et al., 2009) as recently described (Langfeldt et al., 2014; Weiland-Bräuer et al., 2015). Taxonomic classification was done using the ribosomal database project trainset release 14 (Cole et al., 2007, 2014). Bacterial sequences were further processed and binned to operational taxonomic units (OTUs) at a 97% similarity level. After the previous filtering and further quality steps, the analyzed cryoconite sample contained 15,479 high quality sequences belonging to 203 OTUs. Sequence data were deposited in the NCBI Sequence Read Archive (accession number PRJNA300438).
The bphA gene encoding the large subunit of the iron–sulfur component of the key enzyme biphenyl dioxygenase for degradation of PCBs was PCR amplified using 10 ng isolated metagenomic DNA as well as genomic DNA of Pseudomonas spp. isolated from microcosms and the GoTaq DNA Polymerase kit (Promega, Madison, WI, United States) in 25 μL total volume. Primers for amplification of the 211 bp universal bphA fragment (Uhlik et al., 2007) and the 1,100 bp bphA gene of five PCB degrading groups (Sanseverino et al., 2002) were used for amplification (see Supplementary Table S1). PCB degrading bacteria in the cryoconite were determined based on gel electrophoresis, followed by sequencing of the PCR products at the sequencing facility at IKM.
Bacteria were isolated from the cryoconite sample by streaking 100 μl of the original cryoconite sample and respective serial dilutions (10-1 to 10-4) on Nutrient Broth (NB, Carl Roth; Karlsruhe, Germany) agar plates, which were incubated at 4, 20, 30, and 37°C for at least 2 days. Obtained colonies were purified at least three times by repeated streaking. Pure cultures were analyzed for 16S rRNA genes and taxonomically classified via BLAST (Basic Local Alignment Search Tool) search using the National Center for Biotechnology Information (NCBI) database.
Enrichment of bacteria on PCB using microcosms was performed according to Macedo et al. (2005) (Supplementary Figure S1). Droplets (2 μL) of Aroclor 1242 (50 mg/kg in transformer oil; Sigma-Aldrich, Munich, Germany) were placed on sterile standard borosilicate glass cover slips (24 mm × 60 mm; thickness, 0.17 mm, Carl Roth, Karlsruhe, Germany). The slides bearing 10 droplets of PCB mixture Aroclor 1242 were placed with the PCB droplets downward on the water surface of a reservoir filled with 20 mL sterile tap water and 20 g (2 mL) cryoconite sample. Three enrichments were started in parallel. The microcosms were kept at 4°C without agitation for 28 days. After 7, 14, and 28 days, three droplets were used for confocal laser scanning microscopy (CLSM) to detect biofilm formation on PCB droplets. In addition, after 28 days, six droplets were used for DNA isolation and the composition of the bacterial community enriched on PCB was analyzed by 16S rRNA gene analysis using the universal bacterial 16S primer pair 27F and 1492R, cloning and sequencing by Sanger sequencing.
Light microscopy of the cryoconite sample was performed with an Axio Scope microscope and Axio Vision software (Zeiss, Jena, Germany). Biofilm formation on PCB droplets of microcosms was monitored after 7, 14, and 28 days of incubation. PCB droplets on the slides were stained with Nile Red for 15 min according to the manufacturer (Sigma-Aldrich, Karlsruhe, Germany). The sample was carefully rinsed twice with MilliQ water and counterstained using the nucleic acid-specific stain Syto9 (Invitrogen, Darmstadt, Germany). Samples were incubated for 15 min at RT. The entire three-dimensional structure of the PCB community was recorded by scanning along depth using a TCS SP CLSM (Leica, Wetzlar, Germany) and recording the stacks of cross sections simultaneously at the corresponding excitation wavelengths. The following settings were used for excitation and recording of emission signals, respectively: Nile Red, 488 and 550 to 700 nm; Syto9, 488 and 500 to 540 nm. For each field of view, an appropriate number of optical slices were acquired with a Z-step of 1 μm. Digital image acquisition, post-processing, analysis of the CLSM optical thin sections and three-dimensional reconstructions were conducted with the corresponding Leica software (provided for the TCS SP CLSM).
The contamination of the cryoconite sample with PCBs, PAHs, and OCPs was analyzed using high resolution gas chromatography/high resolution mass spectrometry (HRGC/HRMS). The executing laboratory is accredited for the analysis of PAHs, PCBs, and OCPs in various matrices (D-PL-14138-02-00). First, a dry matter determination of the cryoconite sample was performed that yielded 1.28 g. The sample was transferred to hydromatrix with 1 mL double-distilled water and rinsed three times with 1 mL double-distilled water followed by extraction using ASE 200. The hydromatrix mixture was filled in a 33 mL extraction cell added with isotopically labeled quantification standards listed in Supplementary Table S3. The cell was extracted with a solvent mixture of n-hexane/acetone (75:25, v:v) at 120°C and 12 MPa. Two static cycles of 10 min were sufficient for complete extraction. Water was removed using anhydrous sodium sulfate. The volume of the extract was reduced to 1 mL for further cleanup using a rotary evaporator.
A chromatography column was filled from bottom to top with 10 g of silica gel (LGC Standards, Wesel, Germany), 5 g Alumina B (with 3% water added) (LGC Standards, Wesel, Germany) and 2 g of anhydrous sodium sulfate. The column was washed prior to the sample addition with 60 mL of hexane/dichloromethane (1:1, v:v). 1 mL of sample extract was applied to the upper layer of sodium sulfate. Compounds were eluted with 100 mL n-hexane/dichloromethane (1:1, v:v) and again concentrated to 1 mL. A glass fiber filter was placed in the lower end of an empty 8 mL SPE glass cartridge. 1 g C18-modified silica gel (Chromabond C18, Macherey-Nagel, Düren, Germany) was added and covered with a further glass fiber filter. The SPE cartridge was conditioned with 5 mL acetonitrile under vacuum. Before sample application, a solvent change was carried out with 0.5 mL acetonitrile under a stream of nitrogen and topped on the SPE. Following, 5 mL acetonitrile was eluting the analytes from the SPE cartridge and concentrated to 0.2–0.3 mL under nitrogen stream. The final eluate was transferred into a vial, where it was reduced to a final volume of 20 μL already containing the respective recovery standards (see Supplementary Table S4). The analytical measurement of PCBs, PAHs, and OCPs potentially present in the analyzed cryoconite sample was determined by HRGC/HRMS. The parameters used for analytical measurements are listed in Supplementary Table S5. In addition to the cryoconite sample, the PET sampling bottle as well as a PP storage tube (see “Study site and sampling”) were analyzed regarding the presence of PCBs, PAHs and OCPs. Therefore, bottle and storage tubes were rinsed with n-hexane/acetone (75:25, v:v), followed by HRGC/HRMS measurement of the solvent.
Chemical analyses of a broad spectrum of potentially harmful, organic substances were performed using HRGC coupled to isotope-dilution HRMS in order to identify and quantify chemical pollutants in the cryoconite sample from Jamtalferner glacier. In total, samples were assayed for 18 PCB congeners, 16 PAHs and 29 OCPs (Tables 1–3). All analyzed PCB congeners were detected in the cryoconite with concentrations ranging from 12 pg/g dry weight (PCB #81) to 3847 pg/g (PCB #138) (Table 1). Concentrations of PAHs were one magnitude higher as PCB quantities; with a minimum of 44 ng/g Acenaphthene and a maximum of 6908 ng/g Fluoranthene (Table 2). All analyzed PAHs were detected in high amounts. Furthermore, 26 out of 29 OCPs were detected in the cryoconite with a notable maximum concentration of 38 ng/g for 4,4′-DDT (Table 3). The additionally analyzed PET sampling bottle as well as the PP storage tubes (see Materials and Methods) did not showed atypical concentrations of PCBs, PAHs, and OCPs (Tables 1–3). To sum up, a significant contamination with a broad spectrum of organic environmental toxins was observed in the surface of the cryoconite on Jamtalferner glacier.
The bacterial community structure of the upper surface of an alpine cryoconite from Jamtalferner glacier was determined by 454 pyrosequencing using the hypervariable regions V3–V4 of the 16S rRNA gene. 15,479 sequences were included in the 16S amplicon analysis after processing and resulted in the identification of 203 different OTUs (97% sequence similarity) (Supplementary Table S6). It was observed that the microbial community was dominated by the phyla Proteobacteria (66%) and Cyanobacteria (32%). Within the most dominant bacterial phyla, a number of classes were present. Of particular mention is the abundance of α-Proteobacteria, which accounted for 87% of all Proteobacteria, with γ-Proteobacteria (11%) and other classes (β-Proteobacteria, 1%; δ- Proteobacteria, 0.06%; unclassified Proteobacteria, 0.01%) accounting for the remainders. However, the observed high diversity of the community was reflected by 11 other phyla present in low abundances, namely Firmicutes, Bacteroidetes, Actinobacteria, Candidatus Saccharibacteria, Acidobacteria, Chloroflexi, Gemmatimonadetes, Planctomycetes, Fusobacteria, Microgenomates (Figure 2). The relative distribution of bacterial genera is depicted in Figure 3. High abundant genera were unclassified α-Proteobacteria (57%), followed by genus GpIIa belonging to phylum Cyanobacteria (32%) and γ-Proteobacterium Pseudomonas (7%). However, these three genera included only 13 OTUs, whereas 190 OTUs were present within the 75 low abundant genera (Figure 3 and Supplementary Table S6). Overall, the community structure of the cryoconite was characterized by high bacterial diversity including both, heterotrophic and phototrophic bacteria.
FIGURE 2. Deep sequencing analysis of the bacterial communities in the alpine cryoconite. The community composition of the cryoconite on Jamtalferner was analyzed using 454 deep sequencing technology (Illumina, Roche) of the V3–V4 region of the 16S rRNA gene. The cryoconite composition is displayed at bacterial phylum level.
FIGURE 3. High bacterial diversity in the alpine cryoconite. Bacterial community composition of the cryoconite displayed at genus level for high abundant (upper) and low abundant genera (lower). Color code reflects phylum affiliation.
A total of 29 cryoconite bacteria were isolated by enrichment on rich medium (NB agar plates) incubated at various temperatures (4, 20, 30, and 37°C). Isolates from different agar plates varying in morphology (shape, color) were verified by several streaks for single clones resulting in 17 taxonomically classified isolates. Sequence analysis identified nine unique isolates based on 99% identity on nucleotide level. All isolates were identified at least at genus level. As illustrated in the phylogenetic tree (Figure 4), Gram-positives were represented by Micrococcus (G30.1, Accession No. KT924434; G37.1, Accession No. KT924436), Staphylococcus (G30.2, Accession No. KT924435), and Bacillus species (G37.2, Accession No. KT924437; G37.3, Accession No. KT924438; G37.4, Accession No. KT924439). Gram-negative bacteria were represented by species of the genera Janthinobacterium (G4.1, Accession No. KT924431) and Pseudomonas (G20.1, Accession No. KT924432; G20.2, Accession No. KT924433). Pertaining to the deep sequencing analysis, almost all NB enriched bacteria detected belonged to the low abundant taxa and their 16S rRNA sequences matched with certain OTUs listed in Supplementary Table S6 (see Supplementary Table S7).
FIGURE 4. Phylogenetic position of identified isolates enriched from the cryoconite. Isolates (in total 9) were enriched from the cryoconite ample on Nutrient Broth medium at 4, 20, 30, and 37°C. The tree was calculated from full-length 16S rRNA gene sequences by the Maximum-Liklihood method. Nearest relatives to the 16S rRNA sequences of isolates were obtained via BLAST search using the NCBI database. The scale bar represents evolutionary distance (substitutions per nucleotide).
Based on the heavy contamination detected on the cryoconite and results of 16S amplicon sequencing, we assumed increased occurrence of biodegrading bacteria in the sample. For identification of remediating bacteria, we experimentally studied solely the degradation of PCBs by a combination of two approaches (i) a metagenomic and (ii) an enrichment-driven approach.
First, the presence of the key gene bphA of the PCB degradation process (encoding the large subunit of biphenyl dioxygenase) was monitored in the metagenomic DNA by PCR amplification (Figure 5A). The 211 bp bphA fragment was successfully amplified in the cryoconite metagenome using universal primers (Figure 5B, lane 1). In addition, using taxa-specific primers resulted in the amplification of the 1,100 bp bphA fragment exclusively for taxa group “Gram-negative 3” containing Pseudomonas and Ralstonia species (Figure 5B, lane 4). Sequence analysis of the cloned 1,100 bp bphA fragment verified the presence of the key gene and enabled classification to Pseudomonas species.
FIGURE 5. Detection of bphA in the metagenome of the cryoconite. (A) Biphenyl-degrading microorganisms are able to degrade several PCB congeners using the same enzymatic system. The catabolic enzymes involved in the biodegradation of PCBs are organized in the bphABCXD operon where the biphenyl dioxygenase BphA is the first key enzyme. (B) Detection of bphA using universal primer bphA 463/674 (lane 1), and specific primer sets for PCB Gram- group1 (lane 2), PCB Gram- group2 (lane 3), PCB Gram- group3 (lane 4), PCB Rhodo group1 (lane 5), PCB Rhodo group2 (lane 6) (see also Supplementary Table S1).
Second, floating dish microcosm experiments were performed to enrich potentially present PCB-degrading bacteria (Supplementary Figure S1). The microcosms were kept at 4°C without agitation for 28 days. After 7, 14, and 28 days, three droplets were analyzed by CLSM to detect biofilm formation on PCB droplets. Figure 6 depicts the formation and development of a bacterial biofilm on PCB droplets. Initially, bacterial cells colonized the glass substratum of the slide within 3 days. Single cells and cell aggregates were detected on the glass substratum close to the PCB droplet, but almost no cells were observed on the droplet. After 7 days, first bacterial cells populated the PCB droplets. Within the next 21 days of incubation, large microbial aggregates were observed on the PCB surface as depicted in Figures 6C,D. Bacterial cells formed aggregates on the PCB droplets, which matured to compact biofilms. After 28 days of incubation, degradation of PCBs was indicated by observing perforated PCB droplets (Figure 6D).
FIGURE 6. Biofilm formation on PCB droplets over time. (A) Light microscope image of the bacterial community of the cryoconite sample. Confocal laser scanning images (CLSMs) demonstrating biofilm formation on PCB droplets over time (B, 7 days; C, 14 days; and D, 28 days of incubation at 4°C). Images are overlays of Syto9 signals of living PCB-degrading bacterial cells (green), and Nile red signals of PCB (Aroclor 1242; red).
To verify the enrichment of bacteria on PCB after 28 days of incubation, six droplets were pooled and used for DNA isolation, followed by 16S rRNA analysis using the universal bacterial primer set 27F and 1492 followed by cloning and Sanger sequencing (see Materials and Methods). Sequence analysis using the NCBI BLAST tool and the multiple sequence alignment tool CLUSTALW resulted in identification of 37 unique sequences (based on 99% identity on nucleotide level) out of a total of 188. The taxonomical classification resulted in the assignment of these sequences to three different phyla Bacteroidetes, Actinobacteria, and Proteobacteria; the latter subdivided into β- and γ-Proteobacteria (Figure 7). Within the phylum Bacteroidetes five sequences were classified to class Sphingobacteriia and genus Chitinophaga. Actinobacteria were exclusively represented by Gram-positive Subtercola species of family Microbacteraceae. Within the class of β-Proteobacteria seven sequences were assigned to genera Duganella, Janthinobacterium, Polaromonas and Variovorax, whereas Shigella, Escherichia, and Pseudomonas were identified within γ-Proteobacteria. Additionally, isolates taxonomically classified to Pseudomonas spp. were analyzed regarding the presence of the bphA gene resulting in identification of the respective gene in isolates PCB32, PCB38, and PCB40 (see Figure 7). Again, almost all enriched bacteria were assigned to the low abundant taxa, except Pseudomonas. 16S sequences of isolates obtained from the Sanger sequencing approach matched with respective OTUs assessed by amplicon sequencing (Supplementary Table S7).
FIGURE 7. Phylogenetic analysis of cold-adapted PCB degraders enriched in microcosms. Phylogenetic tree of 37 bacterial clones enriched from the cryoconite on PCB droplets in microcosms including four identified taxonomic groups. The tree was calculated from partial 16S rRNA gene sequences by the Maximum-Liklihood method 16S rRNA sequences of isolates (PCB1-PCB62) are represented with their nearest relatives obtained via BLAST search using the NCBI database. The scale bar represents evolutionary distance (substitutions per nucleotide).
Since the late 1960s detectable concentrations of POPs and PAHs have been discovered in many environmental matrices, e.g., air, water, and sediments in the Alps, Antarctic, and Arctic (Halsall, 2004; Bassan et al., 2005; Tremolada et al., 2008; Fuoco et al., 2009; Hansen et al., 2014; Pisso et al., 2014; Vecchiato et al., 2015). It is already known that their long range transport and bioaccumulation significantly impacts human health and the environment, thus POP and PAH exposure is supposed to cause developmental defects, chronic illnesses, cancer, and death (Kageson, 1998). Particularly, glaciers have been shown to be secondary emission sources by re-emitting POPs long after atmospheric deposition (Kallenborn et al., 2012), thus the identification and quantification of POPs and PAHs in glaciers is important for the prediction of secondary emission events and corresponding contamination scenarios. So far, chemical detection of POPs and PAHs in cold environments was mostly conducted in glacial ice and sediment cores (Villa et al., 2006; Bizzotto et al., 2009; Nadal et al., 2015). To the best of our knowledge, this is the first study presenting detailed chemical analyses of PCBs, PAHs, and OCPs in a cryoconite.
Almost all POPs identified in the cryoconite from Jamtalferner, including a broad range of PCB congeners and various OCPs, are listed in the Stockholm Convention, a global treaty to protect human health and the environment from these chemicals (Stockholm Convention, 2009). In general, concentrations of POPs and PAHs vary between study sites in the Alps, Arctic, and Antarctic as well as between different altitudes and nutrient sources comprising xenobiotic entry (Bradley et al., 2014). Documented PCB concentrations in pristine glaciers are on average 0.5 ng/L (Bogdal et al., 2009), whereas PCB concentrations in contaminated glacier areas were approximately 20 ng/L (Cappa et al., 2014); and sediment cores even range from 2 to 132,000 ng/g dry weight (Bigus et al., 2013). These reference data already show that a comparison between several studies is difficult because of different measurement methods and units specified for POP and PAH concentrations. Assuming that one kilogram of dry matter as specified for sediments or debris-associated POP/PAH concentrations is equivalent to 1 lt sample volume as indicated for snow, melt water, and ice cores; an approximate comparison can be drawn. Taking this assumption into account, for instance the average concentration of PCBs in the analyzed cryoconite is two magnitudes of order higher than in contaminated glacier areas with up to 3.8 ng/g dry weight (PCB #138, see Table 1). Similar high concentrations were also revealed for PAH and OCP concentrations (Tables 2, 3). In comparison with reference data, our observed PAH and OCP concentrations are similar to those in heavily polluted regions (Blais et al., 2001; Villa et al., 2006; Bigus et al., 2013; Cappa et al., 2014). All those comparisons clearly show the heavy contamination of the analyzed sample from the Jamtalferner cryoconite with a broad range of environmental toxins. It might be suggested that the BC particles act as sorbent for the pollutants leading to detected high concentrations of PCBs, PAHs, and OCPs. Those pollutants can strongly force for the selection of biodegrading bacteria in generally carbon-poor environments, and thus provide potential to develop remediation strategies with so far unknown biodegrading bacteria.
In the meantime, the diversity of microbial communities present in cold environments is well-known (Carpenter et al., 2000; Amato et al., 2007; Simon et al., 2009; Larose et al., 2010; Harding et al., 2011; Gutiérrez et al., 2015). However, previous analyses have primarily focused on culture- and microscopy-based approaches (Margesin et al., 2002, 2007; Edwards et al., 2013b; Singh et al., 2014). As expected, many of the bacteria found in the cryoconite from Jamtalferner in this study are closely related to bacteria obtained from permanently cold environments such as alpine lakes, Antarctic sea ice, and freshwater lakes (Morita, 1975; Margesin et al., 2007; Bowman, 2014). Taxonomical classification revealed that the analyzed cryoconite mainly consisted of Proteobacteria and Cyanobacteria. Within these phyla, Proteobacteria were dominated by α-Proteobacteria; Cyanobacteria exclusively contained genus GpIIa including Prochlorococcus and Synechococcus. The latter are both known as the most important CO2 fixing bacteria on earth (Bryant and Frigaard, 2006) arguing that they are one of the primary producers in the cryoconite. Several other studies also revealed the predominance of proteobacterial lineages within bacterial cryoconite communities (Edwards et al., 2011, 2013a; Cameron et al., 2012b; Franzetti et al., 2013; Zarsky et al., 2013). It is suggested that Proteobacteria are well-adapted to respond to regular environmental variations typical for short active summer seasons in cold environments (Edwards et al., 2014). A key strategy for survival in such cold environments appears to be the extremely efficient scavenging and recycling of nutrients, as demonstrated for the Proteobacteria-dominated ice-shelf microbial mat metagenomes (Varin et al., 2010, 2012). It is possible that Proteobacteria, and in particular α-Proteobacteria, be important players within the Alp cryoconite community. In addition, the identified predominance of Proteobacteria over Cyanobacteria challenges the assumption that Cyanobacteria are the exclusive contributors to primary production within cryoconites (Edwards et al., 2011, 2014). Photosynthetic Proteobacteria, such as Rhodobacter and Erythrobacter, were present in the sequencing data as well as other phototrophic taxa like Gemmatimonas and Chloroflexi. In conclusion, our data demonstrate that a diverse microbial community is present in the cryoconite from Jamtalferner that are well-known in supraglacial environments.
Nowadays, polluted glaciers are considered as the ultimate sink for many POPs (Blais et al., 2001). The breakdown of these hazardous substances into less toxic or non-toxic substances in such an environment could prevent the entry of POPs into the groundwater and biota, and ultimately avoid their accumulation in animals and humans. Currently, bioremediation is accepted as the practicable method to eliminate POPs as well as PAHs from the environment, because of its advantages over other processes as landfill, soil washing, and incineration (Bajaj and Singh, 2015). However, less information is available on the biodegradation of POPs and PAHs in contaminated cold environments, but it is known that low temperatures affect the rate of biodegradation due to adaptation of physical parameters of the contaminants such as increased viscosity, decreased volatilization and reduced bioavailability (Margesin, 2007; Margesin et al., 2007). In addition, cold conditions also influence microbial activity by reducing metabolic turnover rates (Feller, 2010), and thus make bioremediation in remote cold areas more difficult (Bej et al., 2009). Several reports showed the capability of cold-adapted bacteria to biodegrade POPs/PAHs and their metabolic products under low temperature conditions (Jones and De Voogt, 1999; Bergauer et al., 2005; Hesselsoe et al., 2005; Haritash and Kaushik, 2009; Bajaj and Singh, 2015). Cryoconites as important supraglacial niches and microbial hot-spots of diversity and activity are therefore an ideal habitat, where cold-adapted bacteria with remediation potential can be found. The detection of the gene fragment encoding for biphenyl dioxygenase, involved in the aerobic degradation of PCBs, experimentally identified Pseudomonas, one of the major genera in the cryoconite, as potential PCB-degrader. Lastly, the successful enrichment of bacteria in Aroclor 1242 microcosms assigned to genera Pseudomonas, Shigella, Polaromonas, Variovorax, Janthinobacterium, Subtercola, and Chitinophaga as well as the corresponding depletion of PCBs reflected by CLSM imaging indeed demonstrated PCB degradation by cryoconite embedded bacteria. Almost all of the 37 microcosm-enriched bacteria were identified by phylogenetic analyses as close relatives to known biodegraders (see Table 4).
TABLE 4. List of selected bacteria identified in the cryoconite, which are potentially able to biodegrade hydrocarbons.
In addition to our study, the predominance of Proteobacteria as hydrocarbon degraders has also been observed in other studies. In a recent study by Eriksson et al. (2001, 2003), mixed communities of PAH degraders were enriched from alpine soils under both, aerobic and anaerobic conditions resulting in a few predominant bacterial genera, namely Pseudomonas, Sphingomonas, and Variovorax. The predominance of these three taxa was also determined by Saul et al. (2005) in hydrocarbon-contaminated Antarctic soil. Representatives of β-Proteobacteria, like Polaromonas and Variovorax, were also identified as PCB degraders in Aroclor-microcosms. Variovorax was recently identified in the bacterial community of PCB-polluted soil; and both PCB and PAH degradation of Variovorax isolates was described even under aerobic and low temperature conditions (Eriksson et al., 2003). Psychrophilic Polaromonas species often isolated from glacial environments like an alpine cryoconite are already known as hydrocarbon-degrading (e.g., naphthalene, dichloroethene) bacteria with optimal features for application in remediation of contaminated cold environments (Mattes et al., 2008; Margesin et al., 2012; Franzetti et al., 2013).
The present study was conducted with the aim of evaluating both, the level of contamination with the hazardous pollutants PCBs, PAHs, and OCPs as well as the bacterial diversity in a cryoconite on the alpine Jamtalferner glacier in Austria. The obtained results indicate that in carbon-poor environments, like glaciers, the presence of pollutants can strongly force for the selection of strains able to metabolize them. The enrichment of bacteria on PCB droplets confirmed the assumption that pollution causes the stimulation of pollutant-degrading microorganisms (Jordahl et al., 1997; Ding et al., 2009); and thus reflected the level of contamination retrieved in the chemical analyses of the cryoconite. Our results indeed indicate that contaminated glacial areas can be very important reservoirs for bacteria with potential applications in bioremediation of contaminated remote cold areas. Further analyses will be important to elucidate the degradation of more pollutants, e.g., PAHs and PCBs by bacteria, but also by fungi and archaea. Bacterial isolates have to be characterized in more detail concerning their effective degradation capability as well as application in bioremediation processes.
RS and NW-B conceived the experiments. NW-B performed all experiments, except HGRC/HRMS analyses performed by K-WS. MF performed bioinformatics and statistical analyses. NW-B and RS wrote the manuscript.
This work was financially supported by Bundesministerium für Forschung und Bildung within the GenoMik Transfer Netzwerk (ChemBiofilm, support code 0315587B).
The authors declare that the research was conducted in the absence of any commercial or financial relationships that could be construed as a potential conflict of interest.
We thank the Institute of Clinical Molecular Biology in Kiel, Germany, for providing Sanger and pyrosequencing platforms.
The Supplementary Material for this article can be found online at: http://journal.frontiersin.org/article/10.3389/fmicb.2017.01105/full#supplementary-material
Ahmed, M., and Focht, D. D. (1973). Degradation of polychlorinated biphenyls by two species of Achromobacter. Can. J. Microbiol. 19, 47–52. doi: 10.1139/m73-007
Aislabie, J., McLeod, M., and Fraser, R. (1998). Potential for biodegradation of hydrocarbons in soil from the ross dependency, Antarctica. Appl. Microbiol. Biotechnol. 49, 210–214. doi: 10.1007/s002530051160
Amato, P., Hennebelle, R., Magand, O., Sancelme, M., Delort, A.-M., Barbante, C., et al. (2007). Bacterial characterization of the snow cover at Spitzberg, Svalbard. FEMS Microbiol. Ecol. 59, 255–264. doi: 10.1111/j.1574-6941.2006.00198.x
Amund, O. O., Ilori, M. O., and Odetundun, F. R. (1997). Degradation of commercial detergent products by microbial populations of the Lagos lagoon. Folia Microbiol. 42, 353–356. doi: 10.1007/BF02816949
Anesio, A. M., and Laybourn-Parry, J. (2012). Glaciers and ice sheets as a biome. Trends Ecol. Evol. 27, 219–225. doi: 10.1016/j.tree.2011.09.012
Anesio, A. M., Mindl, B., Laybourn-Parry, J., Hodson, A. J., and Sattler, B. (2007). Viral dynamics in cryoconite holes on a high Arctic glacier (Svalbard). J. Geophys. Res. 112, G04531. doi: 10.1029/2006JG000350
Atlas, R. M. (1981). Microbial degradation of petroleum hydrocarbons: an environmental perspective. Microbiol. Rev. 45, 180.
Bacosa, H. P., Suto, K., and Inoue, C. (2012). Bacterial community dynamics during the preferential degradation of aromatic hydrocarbons by a microbial consortium. Int. Biodeterior. Biodegradation 74, 109–115. doi: 10.1016/j.ibiod.2012.04.022
Bajaj, S., and Singh, D. K. (2015). Biodegradation of persistent organic pollutants in soil, water and pristine sites by cold-adapted microorganisms: mini review. Int. Biodeterior. Biodegradation 100, 98–105. doi: 10.1016/j.ibiod.2015.02.023
Baraniecki, C. A., Aislabie, J., and Foght, J. M. (2002). Characterization of Sphingomonas sp. Ant 17, an aromatic hydrocarbon-degrading bacterium isolated from Antarctic soil. Microb. Ecol. 43, 44–54. doi: 10.1007/s00248-001-1019-3
Bassan, R., Belis, C., Heublein, D., Iozza, S., Jakl, T., Jakobi, G., et al. (2005). Monitoring network in the Alpine region for persistent and other organic pollutants: a multinational approach to investigate the contamination of the Alps with organic compounds. Organohalogen Comp. 67, 1839.
Bej, A. K., Aislabie, J., and Atlas, R. M. (2009). Polar Microbiology: the Ecology, Biodiversity and Bioremediation Potential of Microorganisms in Extremely Cold Environments. Boca Raton FL: CRC Press. doi: 10.1201/9781420083880
Bej, A. K., Saul, D., and Aislabie, J. (2000). Cold-tolerant alkane-degrading Rhodococcus species from Antarctica. Polar Biol. 23, 100–105. doi: 10.1007/s003000050014
Bergauer, P., Fonteyne, P.-A., Nolard, N., Schinner, F., and Margesin, R. (2005). Biodegradation of phenol and phenol-related compounds by psychrophilic and cold-tolerant alpine yeasts. Chemosphere 59, 909–918. doi: 10.1016/j.chemosphere.2004.11.011
Bigus, P., Tobiszewski, M., and Namiesnik, J. (2013). Historical records of organic pollutants in sediment cores. Mar. Pollut. Bull. 78, 26–42. doi: 10.1016/j.marpolbul.2013.11.008
Bizzotto, E. C., Villa, S., Vaj, C., and Vighi, M. (2009). Comparison of glacial and non-glacial-fed streams to evaluate the loading of persistent organic pollutants through seasonal snow/ice melt. Chemosphere 74, 924–930. doi: 10.1016/j.chemosphere.2008.10.013
Blais, J. M., Schindler, D. W., Muir, D. C. G., Sharp, M., Donald, D., Lafrenière, M., et al. (2001). Melting glaciers: a major source of persistent organochlorines to subalpine Bow Lake in Banff National Park, Canada. Ambio 30, 410–415. doi: 10.1579/0044-7447-30.7.410
Bogdal, C., Schmid, P., Zennegg, M., Anselmetti, F. S., Scheringer, M., and Hungerbuhler, K. (2009). Blast from the past: melting glaciers as a relevant source for persistent organic pollutants. Environ. Sci. Technol. 43, 8173–8177. doi: 10.1021/es901628x
Bond, T. C., Doherty, S. J., Fahey, D., Forster, P., Berntsen, T., DeAngelo, B., et al. (2013). Bounding the role of black carbon in the climate system: a scientific assessment. J. Geophys. Res. 118, 5380–5552. doi: 10.1002/jgrd.50171
Bowman, J. S. (2014). Life in the Cold Biosphere: The Ecology of Psychrophile Communities, Genomes, and Genes. Seattle, WA: University of Washington.
Bradley, J. A., Singarayer, J. S., and Anesio, A. M. (2014). Microbial community dynamics in the forefield of glaciers. Proc. R. Soc. Lond. B Biol. Sci. 281, 20140882. doi: 10.1098/rspb.2014.0882
Brown, W. A., and Cooper, D. G. (1992). Hydrocarbon degradation by Acinetobacter calcoaceticus RAG-1 using the self-cycling fermentation technique. Biotechnol. Bioeng. 40, 797–805. doi: 10.1002/bit.260400707
Bryant, D. A., and Frigaard, N.-U. (2006). Prokaryotic photosynthesis and phototrophy illuminated. Trends Microbiol. 14, 488–496. doi: 10.1016/j.tim.2006.09.001
Cameron, K. A., Hodson, A. J., and Osborn, A. M. (2012a). Carbon and nitrogen biogeochemical cycling potentials of supraglacial cryoconite communities. Polar Biol. 35, 1375–1393. doi: 10.1007/s00300-012-1178-3
Cameron, K. A., Hodson, A. J., and Osborn, A. M. (2012b). Structure and diversity of bacterial, eukaryotic and archaeal communities in glacial cryoconite holes from the Arctic and the Antarctic. FEMS Microbiol. Ecol. 82, 254–267. doi: 10.1111/j.1574-6941.2011.01277.x
Cappa, F., Suciu, N., Trevisan, M., Ferrari, S., Puglisi, E., and Cocconcelli, P. S. (2014). Bacterial diversity in a contaminated Alpine glacier as determined by culture-based and molecular approaches. Sci. Total Environ. 497, 50–59. doi: 10.1016/j.scitotenv.2014.07.094
Carpenter, E. J., Lin, S., and Capone, D. G. (2000). Bacterial activity in South Pole snow. Appl. Environ. Microbiol. 66, 4514–4517. doi: 10.1128/AEM.66.10.4514-4517.2000
Christner, B. C., Kvitko Ii, B. H., and Reeve, J. N. (2003). Molecular identification of bacteria and eukarya inhabiting an Antarctic cryoconite hole. Extremophiles 7, 177–183. doi: 10.1007/s00792-002-0309-0
Claesson, M. J., Wang, Q., O’Sullivan, O., Greene-Diniz, R., Cole, J. R., Ross, R. P., et al. (2010). Comparison of two next-generation sequencing technologies for resolving highly complex microbiota composition using tandem variable 16S rRNA gene regions. Nucleic Acids Res. 38:e200. doi: 10.1093/nar/gkq873
Cole, J. R., Chai, B., Farris, R. J., Wang, Q., Kulam-Syed-Mohideen, A. S., McGarrell, D. M., et al. (2007). The ribosomal database project (RDP-II): introducing myRDP space and quality controlled public data. Nucleic Acids Res. 35, D169–172. doi: 10.1093/nar/gkl889
Cole, J. R., Wang, Q., Fish, J. A., Chai, B., McGarrell, D. M., Sun, Y., et al. (2014). Ribosomal Database Project: data and tools for high throughput rRNA analysis. Nucleic Acids Res. 42, D633–42. doi: 10.1093/nar/gkt1244
De Smet, W. H., and Van Rompu, E. A. (1994). Rotifera and Tardigrada from some cryoconite holes on a Spitsbergen (Svalbard) glacier. Belg. J. Zool. 124, 27–27.
Deng, M.-C., Li, J., Liang, F. -R., Yi, M., Xu, X.-M., Yuan, J.-P., et al. (2014). Isolation and characterization of a novel hydrocarbon-degrading bacterium Achromobacter sp. HZ01 from the crude oil-contaminated seawater at the Daya Bay, southern China. Mar. Pollut. Bull. 83, 79–86. doi: 10.1016/j.marpolbul.2014.04.018
Dereeper, A., Audic, S., Claverie, J.-M., and Blanc, G. (2010). BLAST-EXPLORER helps you building datasets for phylogenetic analysis. BMC Evol. Biol. 10, 8. doi: 10.1186/1471-2148-10-8
Dereeper, A., Guignon, V., Blanc, G., Audic, S., Buffet, S., Chevenet, F., et al. (2008). Phylogeny.fr: robust phylogenetic analysis for the non-specialist. Nucleic Acids Res. 36, W465–469. doi: 10.1093/nar/gkn180
Dethlefsen, L., Huse, S., Sogin, M. L., and Relman, D. A. (2008). The pervasive effects of an antibiotic on the human gut microbiota, as revealed by deep 16S rRNA sequencing. PLoS Biol. 6:e280. doi: 10.1371/journal.pbio.0060280
Ding, N., Guo, H., Hayat, T., Wu, Y., and Xu, J. (2009). Microbial community structure changes during Aroclor 1242 degradation in the rhizosphere of ryegrass (Lolium multiflorum L.). FEMS Microbiol. Ecol. 70, 305–314. doi: 10.1111/j.1574-6941.2009.00742.x
Dua, M., Singh, A., Sethunathan, N., and Johri, A. K. (2002). Biotechnology and bioremediation: successes and limitations. Appl. Microbiol. Biotechnol. 59, 143–152. doi: 10.1007/s00253-002-1024-6
Edwards, A., Anesio, A. M., Rassner, S. M., Sattler, B., Hubbard, B., Perkins, W. T., et al. (2011). Possible interactions between bacterial diversity, microbial activity and supraglacial hydrology of cryoconite holes in Svalbard. ISME J. 5, 150–160. doi: 10.1038/ismej.2010.100
Edwards, A., Mur, L. A. J., Girdwood, S. E., Anesio, A. M., Stibal, M., Rassner, S. M. E., et al. (2014). Coupled cryoconite ecosystem structure-function relationships are revealed by comparing bacterial communities in alpine and Arctic glaciers. FEMS Microbiol. Ecol. 89, 222–237. doi: 10.1111/1574-6941.12283
Edwards, A., Pachebat, J. A., Swain, M., Hegarty, M., Hodson, A. J., Irvine-Fynn, T. D. L., et al. (2013a). A metagenomic snapshot of taxonomic and functional diversity in an alpine glacier cryoconite ecosystem. Environ. Res. Lett. 8, 11. doi: 10.1088/1748-9326/8/3/035003
Edwards, A., Rassner, S. M., Anesio, A. M., Worgan, H. J., Irvine-Fynn, T. D. L., Williams, H. W., et al. (2013b). Contrasts between the cryoconite and ice-marginal bacterial communities of Svalbard glaciers. Polar Res. 32, 19468. doi: 10.3402/polar.v32i0.19468
Eriksson, M., Ka, J.-O., and Mohn, W. W. (2001). Effects of low temperature and freeze-thaw cycles on hydrocarbon biodegradation in Arctic tundra soil. Appl. Environ. Microbiol. 67, 5107–5112. doi: 10.1128/AEM.67.11.5107-5112.2001
Eriksson, M., Sodersten, E., Yu, Z., Dalhammar, G., and Mohn, W. W. (2003). Degradation of polycyclic aromatic hydrocarbons at low temperature under aerobic and nitrate-reducing conditions in enrichment cultures from northern soils. Appl. Environ. Microbiol. 69, 275–284. doi: 10.1128/AEM.69.1.275-284.2003
Feller, G. (2010). Protein stability and enzyme activity at extreme biological temperatures. J. Physics 22, 323101. doi: 10.1088/0953-8984/22/32/323101
Feng, T., Lin, H., Tang, J., and Feng, Y. (2014). Characterization of polycyclic aromatic hydrocarbons degradation and arsenate reduction by a versatile Pseudomonas isolate. Int. Biodeterior. Biodegradation 90, 79–87. doi: 10.1016/j.ibiod.2014.01.015
Field, J. A., and Sierra-Alvarez, R. (2008). Microbial degradation of chlorinated dioxins. Chemosphere 71, 1005–1018. doi: 10.1016/j.chemosphere.2007.10.039
Foreman, C. M., Sattler, B., Mikucki, J. A., Porazinska, D. L., and Priscu, J. C. (2007). Metabolic activity and diversity of cryoconites in the Taylor Valley, Antarctica. J. Geophys. Res. 112, G04S32. doi: 10.1029/2006JG000358
Fountain, A. G., Tranter, M., Nylen, T. H., Lewis, K. J., and Mueller, D. R. (2004). Evolution of cryoconite holes and their contribution to meltwater runoff from glaciers in the McMurdo Dry Valleys, Antarctica. J. Glaciol. 50, 35–45. doi: 10.3189/172756504781830312
Franzetti, A., Tatangelo, V., Gandolfi, I., Bertolini, V., Bestetti, G., Diolaiuti, G., et al. (2013). Bacterial community structure on two alpine debris-covered glaciers and biogeography of Polaromonas phylotypes. ISME J. 7, 1483–1492. doi: 10.1038/ismej.2013.48
Fuoco, R., Giannarelli, S., Wei, Y., Ceccarini, A., Abete, C., Francesconi, S., et al. (2009). Persistent organic pollutants (POPs) at Ross sea (Antarctica). Microchem. J. 92, 44–48. doi: 10.1016/j.microc.2008.11.004
Gallego, S., Vila, J., Tauler, M., Nieto, J. M., Breugelmans, P., Springael, D., et al. (2014). Community structure and PAH ring-hydroxylating dioxygenase genes of a marine pyrene-degrading microbial consortium. Biodegradation 25, 543–556. doi: 10.1007/s10532-013-9680-z
Gutiérrez, M. H., Galand, P. E., Moffat, C., and Pantoja, S. (2015). Melting glacier impacts community structure of Bacteria, Archaea and Fungi in a Chilean Patagonia fjord. Environ. Microbiol. 17, 3882–3897. doi: 10.1111/1462-2920.12872
Halsall, C. J. (2004). Investigating the occurrence of persistent organic pollutants (POPs) in the arctic: their atmospheric behaviour and interaction with the seasonal snow pack. Environ. Pollut. 128, 163–175. doi: 10.1016/j.envpol.2003.08.026
Hamamura, N., Olson, S. H., Ward, D. M., and Inskeep, W. P. (2006). Microbial population dynamics associated with crude-oil biodegradation in diverse soils. Appl. Environ. Microbiol. 72, 6316–6324. doi: 10.1128/AEM.01015-06
Hansen, K. M., Christensen, J. H., and Brandt, J. (2014). The Effect of Climate Changes Versus Emission Changes on Future Atmospheric Levels of POPs and Hg in the Arctic. Aarhus: Aarhus University.
Harayama, S., and Timmis, K. N. (2012). Catabolism of aromatic hydrocarbons by Pseudomonas. Genet. Bacterial Divers. 151, 152–174.
Harding, T., Jungblut, A. D., Lovejoy, C., and Vincent, W. F. (2011). Microbes in high Arctic snow and implications for the cold biosphere. Appl. Environ. Microbiol. 77, 3234–3243. doi: 10.1128/AEM.02611-10
Haritash, A. K., and Kaushik, C. P. (2009). Biodegradation aspects of polycyclic aromatic hydrocarbons (PAHs): a review. J. Hazard. Mater. 169, 1–15. doi: 10.1016/j.jhazmat.2009.03.137
Hedlund, B. P., Geiselbrecht, A. D., Bair, T. J., and Staley, J. T. (1999). Polycyclic aromatic hydrocarbon degradation by a new marine bacterium, Neptunomonas naphthovorans gen. nov., sp. nov. Appl. Environ. Microbiol. 65, 251–259.
Henne, A., Daniel, R., Schmitz, R. A., and Gottschalk, G. (1999). Construction of environmental DNA libraries in Escherichia coli and screening for the presence of genes conferring utilization of 4-hydroxybutyrate. Appl. Environ. Microbiol. 65, 3901–3907.
Hesselsoe, M., Boysen, S., Iversen, N., Jürgensen, L., Murrell, J. C., McDonald, I., et al. (2005). Degradation of organic pollutants by methane grown microbial consortia. Biodegradation 16, 435–448. doi: 10.1007/s10532-004-4721-2
Hou, L. H., and Dutta, S. K. (2000). Phylogenetic characterization of several para- and meta-PCB dechlorinating Clostridium species: 16S rDNA sequence analyses. Lett. Appl. Microbiol. 30, 238–243. doi: 10.1046/j.1472-765x.2000.00709.x
Jeon, C. O., Park, W., Ghiorse, W. C., and Madsen, E. L. (2004). Polaromonas naphthalenivorans sp. nov., a naphthalene-degrading bacterium from naphthalene-contaminated sediment. Int. J. Syst. Evol. Microbiol. 54, 93–97. doi: 10.1099/ijs.0.02636-0
Jones, K. C., and De Voogt, P. (1999). Persistent organic pollutants (POPs): state of the science. Environ. Pollut. 100, 209–221. doi: 10.1016/S0269-7491(99)00098-6
Jordahl, J. L., Foster, L., Schnoor, J. L., and Alvarez, P. J. J. (1997). Effect of hybrid poplar trees on microbial populations important to hazardous waste bioremediation. Environ. Toxicol. Chem. 16, 1318–1321. doi: 10.1002/etc.5620160630
Kageson, P. (1998). Growth Versus the Environment: Is There a Trade-Off? (Dordrecht: Kluwer Academic Publishers), 300.
Kallenborn, R., Halsall, C., Dellong, M., and Carlsson, P. (2012). The influence of climate change on the global distribution and fate processes of anthropogenic persistent organic pollutants. J. Environ. Monit. 14, 2854–2869. doi: 10.1039/c2em30519d
Koelmans, A. A., Jonker, M. T. O., Cornelissen, G., Bucheli, T. D., Van Noort, P. C. M., and Gustafsson, Ö. (2006). Black carbon: the reverse of its dark side. Chemosphere 63, 365–377. doi: 10.1016/j.chemosphere.2005.08.034
Kohshima, S. (1987). “Glacial biology and biotic communities,” in Evolution and Coadaptation in Biotic Communities. Vol. 77, eds S. Kawano, J. H. Connell, and T. Hidaka (Kyoto: Faculty of Science, Kyoto University), 93–98.
Kuddus, M., Joseph, B., and Ramteke, P. W. (2013). Production of laccase from newly isolated Pseudomonas putida and its application in bioremediation of synthetic dyes and industrial effluents. Biocatal. Agric. Biotechnol. 2, 333–338. doi: 10.1016/j.bcab.2013.06.002
Lane, D. J. (1991). 16S/23S rRNA Sequencing. E. Stackebrandt and M Goodfellow; Nucleic Acid Techniques in Bacterial Systematics. Chichester: John Wiley & Sons, 115–175.
Langfeldt, D., Neulinger, S. C., Heuer, W., Staufenbiel, I., Kunzel, S., Baines, J. F., et al. (2014). Composition of microbial oral biofilms during maturation in young healthy adults. PLoS ONE 9:e87449. doi: 10.1371/journal.pone.0087449
Larose, C., Berger, S., Ferrari, C., Navarro, E., Dommergue, A., Schneider, D., et al. (2010). Microbial sequences retrieved from environmental samples from seasonal Arctic snow and meltwater from Svalbard, Norway. Extremophiles 14, 205–212. doi: 10.1007/s00792-009-0299-2
Leaes, F. L., Daniel, A. P., Mello, G. B., Battisti, V., Bogusz, S., Emanuelli, T., et al. (2006). Degradation of polychlorinated biphenyls (PCBs) by Staphylococcus xylosus in liquid media and meat mixture. Food Chem. Toxicol. 44, 847–854. doi: 10.1016/j.fct.2005.11.008
Li, H., Li, P., Hua, T., Zhang, Y., Xiong, X., and Gong, Z. (2005). Bioremediation of contaminated surface water by immobilized Micrococcus roseus. Environ. Technol. 26, 931–940. doi: 10.1080/09593332608618504
Lohmann, R., MacFarlane, J., and Gschwend, P. (2005). Importance of black carbon to sorption of native PAHs, PCBs, and PCDDs in Boston and New York harbor sediments. Environ. Sci. Technol. 39, 141–148. doi: 10.1021/es049424+
Lovecka, P., Pacovska, I., Stursa, P., Vrchotova, B., Kochankova, L., and Demnerova, K. (2015). Organochlorinated pesticide degrading microorganisms isolated from contaminated soil. New Biotechnol. 32, 26–31. doi: 10.1016/j.nbt.2014.07.003
Lu, X.-Y., Zhang, T., and Fang, H. H.-P. (2011). Bacteria-mediated PAH degradation in soil and sediment. Appl. Microbiol. Biotechnol. 89, 1357–1371. doi: 10.1007/s00253-010-3072-7
Ma, Y., Wang, L., and Shao, Z. (2006). Pseudomonas, the dominant polycyclic aromatic hydrocarbon-degrading bacteria isolated from Antarctic soils and the role of large plasmids in horizontal gene transfer. Environ. Microbiol. 8, 455–465. doi: 10.1111/j.1462-2920.2005.00911.x
Macedo, A. J., Kuhlicke, U., Neu, T. R., Timmis, K. N., and Abraham, W. R. (2005). Three stages of a biofilm community developing at the liquid-liquid interface between polychlorinated biphenyls and water. Appl. Environ. Microbiol. 71, 7301–7309. doi: 10.1128/AEM.71.11.7301-7309.2005
MacRae, I. C., Raghu, K., and Bautista, E. M. (1969). Anaerobic degradation of the insecticide lindane by Clostridium sp. Nature 221, 859–860. doi: 10.1038/221859a0
Mallick, S., Chatterjee, S., and Dutta, T. K. (2007). A novel degradation pathway in the assimilation of phenanthrene by Staphylococcus sp. strain PN/Y via meta-cleavage of 2-hydroxy-1-naphthoic acid: formation of trans-2, 3-dioxo-5-(2′-hydroxyphenyl)-pent-4-enoic acid. Microbiology 153, 2104–2115. doi: 10.1099/mic.0.2006/004218-0
Margesin, R. (2007). Alpine microorganisms: useful tools for low-temperature bioremediation. J. Microbiol. 45, 281–285.
Margesin, R., Gander, S., Zacke, G., Gounot, A. M., and Schinner, F. (2003a). Hydrocarbon degradation and enzyme activities of cold-adapted bacteria and yeasts. Extremophiles 7, 451–458. doi: 10.1007/s00792-003-0347-2
Margesin, R., Labbe, D., Schinner, F., Greer, C. W., and Whyte, L. G. (2003b). Characterization of hydrocarbon-degrading microbial populations in contaminated and pristine Alpine soils. Appl. Environ. Microbiol. 69, 3085–3092. doi: 10.1128/AEM.69.6.3085-3092.2003
Margesin, R., and Miteva, V. (2011). Diversity and ecology of psychrophilic microorganisms. Res. Microbiol. 162, 346–361. doi: 10.1016/j.resmic.2010.12.004
Margesin, R., and Schinner, F. (2001). Biodegradation and bioremediation of hydrocarbons in extreme environments. Appl. Microbiol. Biotechnol. 56, 650–663. doi: 10.1007/s002530100701
Margesin, R., Schinner, F., Marx, J. -C., and Gerday, C. (2007). Psychrophiles: From Biodiversity to Biotechnolgy: From Biodiversity to Biotechnology. Berlin: Springer Science & Business Media.
Margesin, R., Spröer, C., Zhang, D.-C., and Busse, H.-J. (2012). Polaromonas glacialis sp. nov. and Polaromonas cryoconiti sp. nov., isolated from alpine glacier cryoconite. Int, J. Syst. Evol. Microbiol. 62(Pt 11), 2662–2668. doi: 10.1099/ijs.0.037556-0
Margesin, R., Zacke, G., and Schinner, F. (2002). Characterization of heterotrophic microorganisms in alpine glacier cryoconite. Arct. Antarct. Alp. Res. 34, 88–93. doi: 10.2307/1552512
Mattes, T. E., Alexander, A. K., Richardson, P. M., Munk, A. C., Han, C. S., Stothard, P., et al. (2008). The genome of Polaromonas sp. strain JS666: insights into the evolution of a hydrocarbon-and xenobiotic-degrading bacterium, and features of relevance to biotechnology. Appl. Environ. Microbiol. 74, 6405–6416. doi: 10.1128/AEM.00197-08
Megharaj, M., Ramakrishnan, B., Venkateswarlu, K., Sethunathan, N., and Naidu, R. (2011). Bioremediation approaches for organic pollutants: a critical perspective. Environ. Int. 37, 1362–1375. doi: 10.1016/j.envint.2011.06.003
Mera, N., and Iwasaki, K. (2007). Use of plate-wash samples to monitor the fates of culturable bacteria in mercury-and trichloroethylene-contaminated soils. Appl. Microbiol. Biotechnol. 77, 437–445. doi: 10.1007/s00253-007-1152-0
Militon, C., Boucher, D., Vachelard, C., Perchet, G., Barra, V., Troquet, J., et al. (2010). Bacterial community changes during bioremediation of aliphatic hydrocarbon-contaminated soil. FEMS Microbiol. Ecol. 74, 669–681. doi: 10.1111/j.1574-6941.2010.00982.x
Miteva, V. I. (2008). “Microorganisms associated with glaciers,” in Encyclopedia of Snow, Ice and Glaciers, eds V. P. Singh, P. Singh, and U. K. Haritashya (Berlin: Springer), 741–744.
Nadal, M., Marqués, M., Mari, M., and Domingo, J. L. (2015). Climate change and environmental concentrations of POPs: a review. Environ. Res. 143, 177–185. doi: 10.1016/j.envres.2015.10.012
Nogales, B., Moore, E. R., Llobet-Brossa, E., Rossello-Mora, R., Amann, R., and Timmis, K. N. (2001). Combined use of 16S ribosomal DNA and 16S rRNA to study the bacterial community of polychlorinated biphenyl-polluted soil. Appl. Environ. Microbiol. 67, 1874–1884. doi: 10.1128/AEM.67.4.1874-1884.2001
Parales, R. (2015). “Pseudomonas putida F1: a model for the study of chemotaxis by a soil bacterium,” in Proceedings of the 2015 SIMB Annual Meeting and Exhibition: Simb, Denver, CO.
Pisso, I., Eckhardt, S., and Breivik, K. (2014). “Assessment of secondary sources of Persistent Organic Pollutants in the Arctic,” in Proceedings of the EGU General Assembly Conference Abstracts, Vienna, 16073.
Robertson, W. J., Franzmann, P. D., and Mee, B. J. (2000). Spore-forming, Desulfosporosinus-like sulphate-reducing bacteria from a shallow aquifer contaminated with gasolene. J. Appl. Microbiol. 88, 248–259. doi: 10.1046/j.1365-2672.2000.00957.x
Russell, N. J., Harrisson, P., Johnston, I. A., Jaenicke, R., Zuber, M., Franks, F., et al. (1990). Cold adaptation of microorganisms [and discussion]. Philos. Trans. R. Soc. Biol. Sci. 326, 595–611. doi: 10.1098/rstb.1990.0034
Samanta, S. K., Singh, O. V., and Jain, R. K. (2002). Polycyclic aromatic hydrocarbons: environmental pollution and bioremediation. Trends Biotechnol. 20, 243–248. doi: 10.1016/S0167-7799(02)01943-1
Sanseverino, J., Layton, A. C., and Sayler, G. S. (2002). “Detection of polychlorinated biphenyl-degrading organisms in soil,” in Gene Probes. M. A. de Muro and R. Rapley (Berlin: Springer), 193–209.
Saul, D. J., Aislabie, J. M., Brown, C. E., Harris, L., and Foght, J. M. (2005). Hydrocarbon contamination changes the bacterial diversity of soil from around Scott Base, Antarctica. FEMS Microbiol. Ecol. 53, 141–155. doi: 10.1016/j.femsec.2004.11.007
Säwström, C., Mumford, P., Marshall, W., Hodson, A., and Laybourn-Parry, J. (2002). The microbial communities and primary productivity of cryoconite holes in an Arctic glacier (Svalbard 79 N). Polar Biol. 25, 591–596. doi: 10.1007/s00300-002-0388-5
Schloss, P. D., Westcott, S. L., Ryabin, T., Hall, J. R., Hartmann, M., Hollister, E. B., et al. (2009). Introducing mothur: open-source, platform-independent, community-supported software for describing and comparing microbial communities. Appl. Environ. Microbiol. 75, 7537–7541. doi: 10.1128/AEM.01541-09
Sethunathan, N., and Yoshida, T. (1973). Degradation of chlorinated hydrocarbons by Clostridium sp. isolated from lindane-amended, flooded soil. Plant and Soil 38, 663–666. doi: 10.1007/BF00010705
Sharma, P., Chopra, A., Cameotra, S. S., and Suri, C. R. (2010). Efficient biotransformation of herbicide diuron by bacterial strain Micrococcus sp. PS-1. Biodegradation 21, 979–987. doi: 10.1007/s10532-010-9357-9
Simon, C., Wiezer, A., Strittmatter, A. W., and Daniel, R. (2009). Phylogenetic diversity and metabolic potential revealed in a glacier ice metagenome. Appl. Environ. Microbiol. 75, 7519–7526. doi: 10.1128/AEM.00946-09
Singh, P., Singh, S. M., and Dhakephalkar, P. (2014). Diversity, cold active enzymes and adaptation strategies of bacteria inhabiting glacier cryoconite holes of High Arctic. Extremophiles 18, 229–242. doi: 10.1007/s00792-013-0609-6
Stibal, M., Baelum, J., Holben, W. E., Sörensen, S. R., Jensen, A., and Jacobsen, C. S. (2012). Microbial degradation of 2, 4-dichlorophenoxyacetic acid on the Greenland ice sheet. Appl. Environ. Microbiol. 78, 5070–5076. doi: 10.1128/AEM.00400-12
Stibal, M., Schostag, M., Cameron, K. A., Hansen, L. H., Chandler, D. M., Wadham, J. L., et al. (2015). Different bulk and active bacterial communities in cryoconite from the margin and interior of the Greenland ice sheet. Environ. Microbiol. Rep. 7, 293–300. doi: 10.1111/1758-2229.12246
Stibal, M., Tranter, M., Benning, L. G., and Rehák, J. (2008). Microbial primary production on an Arctic glacier is insignificant in comparison with allochthonous organic carbon input. Environ. Microbiol. 10, 2172–2178. doi: 10.1111/j.1462-2920.2008.01620.x
Stockholm Convention (2009). Stockholm Convention on Persistent organic pollutants as amended in 2009. Available at: http://chm.pops.int/Home/tabid/2121/Default.aspx
Su, X., Zhang, Q., Hu, J., Hashmi, M. Z., Ding, L., and Shen, C. (2015). Enhanced degradation of biphenyl from PCB-contaminated sediments: the impact of extracellular organic matter from Micrococcus luteus. Appl. Microbiol. Biotechnol. 99, 1989–2000. doi: 10.1007/s00253-014-6108-6
Sun, W., Sun, X., and Cupples, A. M. (2014). Identification of Desulfosporosinus as toluene-assimilating microorganisms from a methanogenic consortium. Int. Biodeterior. Biodegradation 88, 13–19. doi: 10.1016/j.ibiod.2013.11.014
Takeuchi, N. (2014). “Cryoconite,” in Encyclopedia of Snow, Ice and Glaciers, edsV. P. Singh, and U. K. Haritashya (Berlin: Springer), 168–171.
Telling, J., Anesio, A. M., Tranter, M., Stibal, M., Hawkings, J., Irvine-Fynn, T., et al. (2012). Controls on the autochthonous production and respiration of organic matter in cryoconite holes on high Arctic glaciers. J. Geophys. Res. 117, G01017. doi: 10.1029/2011JG001828
Tremolada, P., Villa, S., Bazzarin, P., Bizzotto, E., Comolli, R., and Vighi, M. (2008). POPs in mountain soils from the Alps and Andes: suggestions for a “precipitation effect” on altitudinal gradients. Water Air Soil Pollut. 188, 93–109. doi: 10.1007/s11270-007-9527-5
Uhlik, O., Jecna, K., Mackova, M., Vlcek, C., Hroudova, M., Demnerova, K., et al. (2009). Biphenyl-metabolizing bacteria in the rhizosphere of horseradish and bulk soil contaminated by polychlorinated biphenyls as revealed by stable isotope probing. Appl. Environ. Microbiol. 75, 6471–6477. doi: 10.1128/AEM.00466-09
Uhlik, O., Strejcek, M., Junkova, P., Sanda, M., Hroudova, M., Vlcek, C., et al. (2007). Matrix-assisted laser desorption ionization (MALDI)-time of flight mass spectrometry-and MALDI biotyper-based identification of cultured biphenyl-metabolizing bacteria from contaminated horseradish rhizosphere soil. Appl. Environ. Microbiol. 77, 6858–6866. doi: 10.1128/AEM.05465-11
Uhlik, O., Wald, J., Strejcek, M., Musilova, L., Ridl, J., Hroudova, M., et al. (2012). Identification of bacteria utilizing biphenyl, benzoate, and naphthalene in long-term contaminated soil. PLoS ONE 7:e40653. doi: 10.1371/journal.pone.0040653
Varin, T., Lovejoy, C., Jungblut, A. D., Vincent, W. F., and Corbeila, J. (2010). Metagenomic profiling of Arctic microbial mat communities as nutrient scavenging and recycling systems. Limnol. Oceanogr. 55, 1901–1911. doi: 10.4319/lo.2010.55.5.1901
Varin, T., Lovejoy, C., Jungblut, A. D., Vincent, W. F., and Corbeil, J. (2012). Metagenomic analysis of stress genes in microbial mat communities from Antarctica and the High Arctic. Appl. Environ. Microbiol. 78, 549–559. doi: 10.1128/AEM.06354-11
Vecchiato, M., Argiriadis, E., Zambon, S., Barbante, C., Toscano, G., Gambaro, A., et al. (2015). Persistent Organic Pollutants (POPs) in Antarctica: occurrence in continental and coastal surface snow. Microchem. J. 119, 75–82. doi: 10.1016/j.microc.2014.10.010
Villa, R., Bonetti, E., Penza, M. L., Iacobello, C., Bugari, G., Bailo, M., et al. (2004). Target-specific action of organochlorine compounds in reproductive and nonreproductive tissues of estrogen-reporter male mice. Toxicol. Appl. Pharmacol. 201, 137–148. doi: 10.1016/j.taap.2004.05.007
Villa, S., Negrelli, C., Finizio, A., Flora, O., and Vighi, M. (2006). Organochlorine compounds in ice melt water from Italian Alpine rivers. Ecotoxicol. Environ. Saf. 63, 84–90. doi: 10.1016/j.ecoenv.2005.05.010
Villa, S., Vighi, M., Casini, S., and Focardi, S. (2003). Pesticide risk assessment in a lagoon ecosystem. Part II: effect assessment and risk characterization. Environ. Toxicol. Chem. 22, 936–942. doi: 10.1002/etc.5620220435
Weiland, N., Löscher, C., Metzger, R., and Schmitz, R. (2010). “Construction and screening of marine metagenomic libraries,” in Metagenomics. eds W. R. Streit and R. Daniel (Berlin: Springer), 51–65. doi: 10.1007/978-1-60761-823-2_3
Weiland-Bräuer, N., Langfeldt, D., and Schmitz, R. A. (2017). Construction and screening of marine metagenomic large insert libraries. Methods Protoc. 1539, 23–42. doi: 10.1007/978-1-4939-6691-2_3
Weiland-Bräuer, N., Neulinger, S. C., Pinnow, N., Künzel, S., Baines, J. F., and Schmitz, R. A. (2015). Composition of bacterial communities associated with Aurelia aurita changes with compartment, life stage, and population. Appl. Environ. Microbiol. 81, 6038–6052. doi: 10.1128/AEM.01601-15
Wharton, R. A. Jr., McKay, C. P., Simmons, G. M. Jr., and Parker, B. C. (1985). Cryoconite holes on glaciers. BioScience, 35, 499–503. doi: 10.2307/1309818
Wharton, R. A. Jr., Vinyard, W. C., Parker, B. C., Simmons, G. M. Jr., and Seaburg, K. G. (1981). Algae in cryoconite holes on Canada Glacier in southern Victorialand, Antarctica. Phycologia 20, 208–211. doi: 10.2216/i0031-8884-20-2-208.1
Whyte, L. G., Bourbonniere, L., and Greer, C. W. (1997). Biodegradation of petroleum hydrocarbons by psychrotrophic Pseudomonas strains possessing both alkane (alk) and naphthalene (nah) catabolic pathways. Appl. Environ. Microbiol. 63, 3719–3723.
Winsley, T. J., Snape, I., McKinlay, J., Stark, J., van Dorst, J. M., Ji, M., et al. (2014). The ecological controls on the prevalence of candidate division TM7 in polar regions. Front. Microbiol. 5:345. doi: 10.3389/fmicb.2014.00345
Wu, Y., Luo, Y., Zou, D., Ni, J., Liu, W., Teng, Y., et al. (2008). Bioremediation of polycyclic aromatic hydrocarbons contaminated soil with Monilinia sp.: degradation and microbial community analysis. Biodegradation 19, 247–257. doi: 10.1007/s10532-007-9131-9
Zarsky, J. D., Stibal, M., Hodson, A., Sattler, B., Schostag, M., Hansen, L. H., et al. (2013). Large cryoconite aggregates on a Svalbard glacier support a diverse microbial community including ammonia-oxidizing archaea. Environ. Res. Lett. 8, 035044. doi: 10.1088/1748-9326/8/3/035044
Keywords: cryoconite, microbial communities, biodegradation, polychlorinated biphenyls (PCBs), polycyclic aromatic hydrocarbon (PAH), organochlorine pesticide (OCP)
Citation: Weiland-Bräuer N, Fischer MA, Schramm K-W and Schmitz RA (2017) Polychlorinated Biphenyl (PCB)-Degrading Potential of Microbes Present in a Cryoconite of Jamtalferner Glacier. Front. Microbiol. 8:1105. doi: 10.3389/fmicb.2017.01105
Received: 13 March 2017; Accepted: 31 May 2017;
Published: 15 June 2017.
Edited by:
Pankaj Kumar Arora, M. J. P. Rohilkhand University, IndiaReviewed by:
Stefano Fedi, Università di Bologna, ItalyCopyright © 2017 Weiland-Bräuer, Fischer, Schramm and Schmitz. This is an open-access article distributed under the terms of the Creative Commons Attribution License (CC BY). The use, distribution or reproduction in other forums is permitted, provided the original author(s) or licensor are credited and that the original publication in this journal is cited, in accordance with accepted academic practice. No use, distribution or reproduction is permitted which does not comply with these terms.
*Correspondence: Ruth A. Schmitz, cnNjaG1pdHpAaWZhbS51bmkta2llbC5kZQ==
Disclaimer: All claims expressed in this article are solely those of the authors and do not necessarily represent those of their affiliated organizations, or those of the publisher, the editors and the reviewers. Any product that may be evaluated in this article or claim that may be made by its manufacturer is not guaranteed or endorsed by the publisher.
Research integrity at Frontiers
Learn more about the work of our research integrity team to safeguard the quality of each article we publish.