- 1Department of Environmental Health Sciences, Arnold School of Public Health, University of South Carolina, Columbia, SC, United States
- 2School of Engineering and Physical Sciences, Heriot-Watt University, Edinburgh, United Kingdom
Microbial cells (i.e., bacteria, archaea, microeukaryotes) in oceans secrete a diverse array of large molecules, collectively called extracellular polymeric substances (EPSs) or simply exopolymers. These secretions facilitate attachment to surfaces that lead to the formation of structured ‘biofilm’ communities. In open-water environments, they also lead to formation of organic colloids, and larger aggregations of cells, called ‘marine snow.’ Secretion of EPS is now recognized as a fundamental microbial adaptation, occurring under many environmental conditions, and one that influences many ocean processes. This relatively recent realization has revolutionized our understanding of microbial impacts on ocean systems. EPS occur in a range of molecular sizes, conformations and physical/chemical properties, and polysaccharides, proteins, lipids, and even nucleic acids are actively secreted components. Interestingly, however, the physical ultrastructure of how individual EPS interact with each other is poorly understood. Together, the EPS matrix molecules form a three-dimensional architecture from which cells may localize extracellular activities and conduct cooperative/antagonistic interactions that cannot be accomplished efficiently by free-living cells. EPS alter optical signatures of sediments and seawater, and are involved in biogeomineral precipitation and the construction of microbial macrostructures, and horizontal-transfers of genetic information. In the water-column, they contribute to the formation of marine snow, transparent exopolymer particles (TEPs), sea-surface microlayer biofilm, and marine oil snow. Excessive production of EPS occurs during later-stages of phytoplankton blooms as an excess metabolic by product and releases a carbon pool that transitions among dissolved-, colloidal-, and gel-states. Some EPS are highly labile carbon forms, while other forms appear quite refractory to degradation. Emerging studies suggest that EPS contribute to efficient trophic-transfer of environmental contaminants, and may provide a protective refugia for pathogenic cells within marine systems; one that enhances their survival/persistence. Finally, these secretions are prominent in ‘extreme’ environments ranging from sea-ice communities to hypersaline systems to the high-temperatures/pressures of hydrothermal-vent systems. This overview summarizes some of the roles of exopolymer in oceans.
Overview
Microorganisms (e.g., bacteria, archaea, microeukaryotes) reside in ocean systems in an assortment of physical states ranging from free-living cells to complex communities attached to surfaces and to each other (Moran, 2015; Brussaard et al., 2016). Over the span of different ocean environments, microbial flora take up dissolved organics and ions, and then secrete polymeric organic compounds. These secretions, called exopolymers or extracellular polymeric substances (EPSs), are abundant and become mixed with other forms of organic matter within ocean systems. It was recognized early on, that under the fluctuating, and often less-predictable conditions of natural systems (compared to those of a laboratory culture flask), the attachment of microbes to surfaces, or to each other, offers a degree of environmental stability not experienced by free-living (non-attached) cells (ZoBell and Allen, 1935). An initial understanding of the purposeful secretion of EPS and their potential stabilizing effects for microbial cells initially emerged during the last century. It is now realized and mostly accepted that many bacteria and other microorganisms occur in a biofilm state; either attached to surfaces or as suspended-aggregates in the water column. EPS, the subject of this overview, consist of a wide range of molecules and provide selective adaptations for the cells that produce them, which in turn, influence broader ocean processes (Figure 1).
EPS: A Microbial Adaptation for Aggregation and Attachment
Extracellular polymeric substance are purposefully produced by microbes: (a) as secretions of biofilms that secure attachment and enhance their local environment, and/or (b) as metabolic-excess waste products. The differences between these two processes is easily discernable but becomes important when addressing the provenance of organic matter and the roles that EPS contribute to ocean systems. It is important to point out that EPS are not an essential component to microbial life (i.e., cells can survive and grow without them), but rather their secretion strongly enhances the survival, metabolic efficiency and adaptation of cells.
The Biofilm State
The term ‘biofilm’ was coined long ago (Costerton et al., 1987), and refers to microbial cells that have attached to a surface or aggregated with each other, and have secreted a gelatinous matrix of EPS. The ability of a microbial cell, such as a bacterium, to attach, secrete EPS and form a biofilm under laboratory conditions, is well-established. The secretion of EPS (by cells) is a key emergent property of the biofilm (Flemming and Wingender, 2010; Flemming et al., 2016); the property that directly influences adaptations that cells utilize to enhance their efficiency and survival. The secretion of an EPS matrix represents, in the broadest sense, an extension of the cell. The presence of EPS facilitates the self-organization of cells into localized communities, and provides biofilm cells with an enhanced capability for: trapping other organics and localizing their digestion by extracellular enzymes, coordinating cell–cell chemical communication [quorum sensing (QS)], facilitating gene-exchange, and provides a degree of physical stability. The EPS often form a localization matrix for other molecules, keeping them in spatial proximity to cells where they can be efficiently utilized.
It is now generally recognized within microbiology that the ‘biofilm state’ is an omnipresent feature of microbial flora in most environments (Hall-Stoodley et al., 2004). Biofilms occur under a wide range of conditions and environments, and whose influences span aquatic, terrestrial, the epi- and endo-biont communities of plants and animals, which can be commensal, symbiotic or pathogenic. The cells within a biofilm can move, and periodically reorient themselves in relation to one another, and in doing so can resist invasion by other cells (Houry et al., 2012). The EPS matrix of biofilms provides a three-dimensional architecture framework that allows the arrangements of cells movements relative to other microbes as well as positioning among sharp geochemical gradients (Decho, 2000b). This will not be discussed further here, but directly contributes to the remarkable plasticity of biofilm cells. The EPS form a matrix of largely anionic molecules near cells, affording them with a proximal environment that is more stabilizing, and conducive to manipulation by the cell (Table 1), and one that contributes to broader ocean processes. However, in this overview we will not discuss biofilms as systems, except with regard to their secretion of EPS.
Finally, it is important to note that in ocean systems, the microbial communities of aggregates suspended in the water-column, and the sea-surface slick communities of oceans are also biofilms, since these communities contain EPS, and exhibit differing levels of organization. EPS are also secreted as a ‘metabolic by product.’ These are most apparent during the later stages of phytoplankton blooms, and will be discussed further below. Taken together, microbial extracellular secretions are now thought to comprise a large portion of the bioavailable carbon pool in oceans, especially in dissolved forms. The total amount of microbially produced EPS, although difficult to measure accurately and precisely, is likely to be very substantial.
Dissolved and Particulate Organic Carbon in the Ocean
Organic matter in seawater constitutes a complex mixture of compounds in a dissolved and particulate form – respectively, dissolved organic matter (DOM) and particulate organic matter (POM). Both forms serve a source of carbon and nutrients to heterotrophic microorganisms, including to mixotrophic eukaryotic phytoplankton and filter feeders. DOM is the dominant form of carbon in the oceans that can originate from any number of sources, much of which is produced in situ by marine microorganisms (largely eukaryotic phytoplankton and bacteria) and is derived from terrestrial inputs via transportation from river effluents and surface runoff. DOM comprises up to 700 Gt of carbon in the ocean, which is a staggering amount of dissolved organic carbon (DOC); so much so that 1% annual change of it in the ocean can produce as much CO2 as that from fossil fuel combustion per annum (Hedges, 2002). Up to 70% of DOM in the oceans averages a molecular weight of <1 kDa and is defined as low-molecular-weight DOM (Benner, 2002), the bulk of which is refractory (Bauer et al., 2002) and difficult to chemically characterize down to the molecular level. The high-molecular-weight fraction of DOM (>1 kDa) in the oceans contributes about 30% of DOC. It is more labile and thus more readily degraded (Amon and Benner, 1994; Guo et al., 1994).
Depending on its physical state in seawater (gel, colloidal, or particulate form), DOC/POC can serve as a surface to which microorganisms attach. Marine snow, which comprises aggregates of >500 μm, is formed in the upper water-column when dead and dying phytoplankton cells come together with other planktonic microorganisms within a matrix of biopolymers (Alldredge et al., 1993; Tiselius and Kuylenstierna, 1996). Marine snow is one form of POC that is a key component of the biological pump in the ocean that participates in the redistribution of carbon in marine systems and principally in the flux of fixed carbon to the sea floor (Shanks and Trent, 1980; Shanks and Reeder, 1993; Long and Azam, 2001b). The processing of organic matter, such as marine snow, by bacteria in the ocean significantly affects its vertical flux from the upper water column to the ocean floor, and in turn impacting the global cycling of carbon and the planet’s climate (Simon et al., 2002). The transport of organic carbon via sinking of POC from the sea surface to the seafloor is another major component of the “biological pump,” which globally contributes in the exports of ca. 10 Gt C per year from the euphotic zone and accounts for 20% of ocean primary production (Treguer et al., 2003). However, at depths approaching 2000 m, this flux or organic carbon decreases to about 1% as the other 19% is mineralized and cycled by the “microbial loop.”
In oceanography, organic matter in seawater is operationally defined as “dissolved” (i.e., DOM) if it passes through a 0.7 μm pore size filter; that which is retained on the filter is defined as POM. The diversity of dissolved organic carbon in seawater ranges from ‘truly’ dissolved molecules, such as glucose, to colloidal and transparent gel-like matter, and can also include microorganisms (e.g., micro-algae, bacteria, archaea, viruses) if they too pass through a 0.7 μm pore size filter. The introduction of sensitive analytical techniques for analyzing seawater, such as high-performance liquid chromatography (HPLC) (Mopper et al., 1992) have increased our understanding of the major classes of DOM in the ocean. Methods to recover and characterize DOM and POM are described by Wurl (2009).
Water Column
DOC and POC
The world’s oceans contain a total DOC content that is comparable in mass to the carbon in atmospheric CO2 (Hansell and Carlson, 1998). The oceanic DOC pool comprises a wide spectrum of compounds, much of which is chemically uncharacterized – it could be regarded as a ‘black hole’ in terms of our relatively poor understanding of its chemical composition and from what biogenic sources this massive pool of organic carbon molecules originate. At least among the chemical constituents of oceanic DOC that have been characterized, three major compound classes have been identified: carbohydrates (mono- and polysaccharides or EPS), proteins, and lipids. Much of the DOC in the ocean water column exists as EPS biopolymers (ca. 10–25% of total oceanic DOM) that undergo reversible transition between colloidal and dissolved phases (Verdugo, 1994; Chin et al., 1998). Based on its predominance throughout the world ocean, it has important implications in microbial interactions and biogeochemical cycles.
Extracellular Polymeric Substance
The synthesis and extracellular release of EPS by eukaryotic phytoplankton and bacteria forms a major component to the total DOC pool in the ocean (Verdugo, 1994; Aluwihare et al., 1997). EPS can serve a variety of functions, such as in the binding and fate of trace metal-nutrient species, the solubilisation of hydrophobic organic chemicals, and in biofilm formation (Decho, 1990; Santschi et al., 1998). Compared to EPS produced by marine eukaryotic phytoplankton (Bhaskar and Bhosle, 2005) and non-marine bacteria (Ford et al., 1991), EPS produced by marine bacteria generally contains higher levels of uronic acids, notably D-glucuronic and D-galacturonic acid (Kennedy and Sutherland, 1987). This renders these macromolecules highly polyanionic (negatively charged), which may be attributable to any number of anionic groups (e.g., COO-, C–O-, SO4-) and consequently quite reactive in their potential to interact with other chemical species (Kennedy and Sutherland, 1987). Nonetheless, the EPS released by some eukaryotic phytoplankton species can also be rich in uronic acids, such as that produced by the coccolithophore Emiliania huxleyi, which contains up to 20% galacturonic acids of total sugar content (De Jong et al., 1979).
The polyanionic nature of EPS serves important ecological functions in marine systems. These include microbial adhesion and biofilm formation (Thavasi and Banat, 2014), the emulsification of hydrocarbon oils and influencing their biodegradation (Gutierrez et al., 2013), or mediating the fate and mobility of heavy metals and trace metal nutrients (Bhaskar and Bhosle, 2005; Gutierrez et al., 2008, 2012). This wide spectrum of functional activity is reflected not merely in the complex chemistry of these molecules, but also in the diversity of bacterial genera producing them (Thavasi et al., 2011). Overall, the composition of marine EPS varies due to the producing species and physiological stage (Myklestad, 1977; Grossart et al., 2007).
A number of reports have described marine bacterial EPS binding heavy and toxic metal ions such as Cd, Cr, Pb, Ni, Cu, Al, and Ur (Zosim et al., 1983; Beech and Cheung, 1995; Schlekat et al., 1998; Iyer et al., 2005; Bhaskar and Bhosle, 2006; Gutierrez et al., 2008). Whilst the rationale to many of these studies was commercial, a few have addressed the ecological implications of marine EPS in biogeochemical cycles. In two studies by Loaec et al. (1997, 1998), the authors reported on the heavy metal-binding capacity of EPS produced by hydrothermal vent bacteria, and showed that this might represent a survival strategy for the bacteria by reducing their exposure to toxic metals released from the hydrothermal vents. Major elemental constituents of seawater, such as Na, Mg, Ca, K, Sr and Si, have been shown to be adsorbed by marine bacterial EPS (Gutierrez et al., 2008). What ecological implications this may have in marine systems, or indeed to the producing organisms, remains to be more-fully understood.
A key role of polyanionic EPS, particularly in the euphotic zone, is in its potential role in controlling soluble iron (Fe3+) bioavailability. Studies in recent years have shown single anionic residues, such as glucuronic and galacturonic acids (Hassler and Schoemann, 2009; Hassler et al., 2011b), and purified marine bacterial EPS containing high levels of uronic acids (Gutierrez et al., 2008; Hassler et al., 2011a), can effectively bind Fe3+ and promote the uptake of this trace metal by eukaryotic phytoplankton (Hassler et al., 2011b; Gutierrez et al., 2012). The implications of this are significant because of the abundance of EPS in the ocean (Verdugo et al., 2004) and because Fe3+ is an essential trace metal that limits primary production in up to 40% of the open ocean (Martin et al., 1994; Boyd et al., 2007).
A large fraction of the EPS produced by bacteria in the ocean is of glycoprotein composition (Long and Azam, 1996; Verdugo et al., 2004). The amino acid and peptide components found associated with these glycoprotein biopolymers have been shown to confer amphiphilic characteristics to these macromolecules (Verdugo et al., 2004; Gutierrez et al., 2009), and which could explain, at least in part, their ability to interact with hydrophobic species, such as oil hydrocarbons.
Transparent Exopolymer Particle
A special class of EPS that are described as mucopolysaccharides is transparent exopolymer particles (TEPs). It is operational defined based on being retained by a filter with a pore size of >0.4 μm (Alldredge et al., 1993), and based on this, TEP are defined as gel particles. TEP exists in the water column suspended in colloidal form, likely formed via the aggregation of smaller EPS molecules (Engel et al., 2004). Aggregation may be mediated by the bridging of divalent cation (Ca2+, Mg2+) and half-ester sulfate (OSO3-) moieties of acidic monomers that constitute individual EPS molecules. TEP is transparent, but because these gel particles are rich in acidic sugars they can be observed under the light microscope after staining with the cationic copper phthalocyanine dye Alcian Blue at pH 2.5 (Alldredge et al., 1993).
The abundances of TEP in the ocean water column are on average in the order of 106 per L of seawater, and can reach as high as 108 per L (Passow, 2002; Bhaskar and Bhosle, 2005), particularly during periods of phytoplankton blooms. The contribution of TEP to the pool of POC in the upper water column in the Atlantic and Adriatic during certain periods of the year has been shown to be quite significant (Engel and Passow, 2001). A fraction of the TEP pool in the ocean is proteinaceous. It is referred to as Coomassie stainable particles (CSPs) because these gel particles can be stained with the amino acid-specific dye Coomassie Brilliant Blue and observed under the light microscope (Long and Azam, 1996). The abundances of CSP in coastal waters range between 106 and 108 per L of seawater (Long and Azam, 1996).
Transparent exopolymer particle contribute significantly to what is described as the marine gel phase. Verdugo et al. (2004) suggested this phase to span a large size spectrum, from colloids to particles of several 100s of micrometers. Its formation has been described to originate from the spontaneous aggregation of DOM molecules into POM within minutes in seawater (Chin et al., 1998) – a process that may involve crosslinks facilitated by cation bridging between DOM molecules.
Microbial Associates
Particulate organic matter can be described as a “hot spot” for microbial (esp. bacterial) activities in the water column, containing a rich microbial community with abundances reaching up to two orders of magnitude higher than in the surrounding seawater environment (Alldredge et al., 1986; Herndl, 1988). The establishment of a bacterial community within and surrounding (biofilm) POM leads to various levels of microbial interaction that include mutualism and antagonism (Long and Azam, 2001a), as well as cooperative behavior such as QS (Gram et al., 2002). A study assessing the phylogenetic diversity of POM-associated versus free-living bacteria from a site ca. 5 km offshore the Santa Barbara coast revealed distinct differences between these communities, with primarily members of the Cytophaga, Planctomyces, and Gammaproteobacteria dominating aggregate particles, whereas Alphaproteobacteria dominated the free-living fraction (DeLong et al., 1993). Bacteria associated with POM have been shown to exhibit high activities for a range of extracellular enzymes (Hoppe et al., 2002; Simon et al., 2002), likely contributing to the hydrolysis of the POM aggregates. Whilst rich in microbial diversity and abundance, POM accounts for only <10% of total bacterial abundance and production in the marine water column, with the majority of bacterial cells occurring in a free-living state.
Studies using oxygen microelectrodes to measure dissolved oxygen in POM aggregates have shown that even the tiniest of marine snow particles can contain anoxic environments (Alldredge and Cohen, 1987; Alldredge and Silver, 1988; Ploug et al., 1997; Ploug, 2008). The high extracellular enzyme activities by bacteria associated with POM will deplete oxygen concentrations that create anoxic micro-niches within the aggregates, potentially supporting the growth of obligate anaerobic or microaerophilic microorganisms (Bianchi et al., 1992). It may therefore, be expected that diverse aerobic and anaerobic microorganisms associated with marine snow aggregates would colonize different niches of the aggregates. The formation of an oxygen gradient, which is increasingly more anoxic toward the interior of the aggregates, would pose a strong influence on the stratification of the microbial community. Essentially, the interior of aggregates will be enriched with obligate and/or facultative anaerobes.
Air–Water Interface
Surface Water Droplet Formation, Sea Spray, and Cloud Formation
Biological processes on the sea surface of the ocean can have a direct effect on atmospheric processes, such as modulating CO2 exchange and release of cloud condensation nuclei (CCN), that in turn influence the Earth’s climate. CCN are atmospheric particles that serve as nuclei for the formation of cloud droplets by taking up water vapor because they are sufficiently soluble. In the past decade there has been an increasing body of evidence supporting the hypothesis that atmospheric marine aerosols contain the same organic species that are found in oceanic DOM (Leck and Bigg, 2005; Bigg, 2007; Facchini et al., 2008). Seawater DOM, much of which comprises phytoplankton exudates and bacterial EPS, can be ejected into the atmosphere when bubbles at the sea surface burst (Bigg, 2007; Leck and Bigg, 2008). Bubbles can form by a number of physical forces at the sea surface, ranging from raindrops to breaking waves, which then burst and produce submicron droplets that disperse as aerosol into the atmosphere and carrying with it marine organic species such as microbial cells and DOM. A study by Kuznetsova et al. (2005) showed that TEP and CSP can accumulate in the sea surface microlayer and subsequently, through bubble bursting, become transported to the atmosphere as marine aerosol. The authors showed that the aerosols contained a large number of semitransparent gel-like particles, in addition to microorganisms, organic and inorganic matter. The semitransparent gel-like particles (primarily TEP and CSP) in the aerosols all contained amino acids, and based on D/L ratios of these acids it was suggested that they originated from phytoplankton exudates.
Several studies have shown that the organic species entrained within marine aerosols collected from various remote ocean sites are of a size range between 70 and 200 nm in diameter. The dominant size range between 50 and 100 nm (Tyree et al., 2007; Fuentes et al., 2010; Hultin et al., 2010), is reminiscent of EPS gels found on the sea surface (Bigg, 2007; Bigg and Leck, 2008; Leck and Bigg, 2008). In a review by Hawkins and Russell (2010) covering over 10 years of measurements of ocean-derived aerosol, the authors concluded that the organic species within marine aerosol is composed of EPS, proteins and amino acids, as well as microorganisms and their components. Facchini et al. (2008) suggested that the solubility continuum of phytoplankton exudates found in seawater is also reflected in marine aerosol, and there is a growing body of evidence supporting the hypothesis that phytoplankton exudates contribute to the formation of CNN (O’Dowd et al., 2004; Russell et al., 2010). Upon its entry into the atmosphere through bubble bursting, the entrained organic gel aggregates within the aerosol particles either directly contribute to the CCN pool in the marine boundary layer (MBL) or after they are degraded by ultraviolet light or acidification in the atmosphere.
Recent research by the DROPPS consortium, funded through the Gulf of Mexico Research Initiative (GOMRI) program, is carrying out experiments attempting to recreate the sea surface microlayer to investigate the potential for petrocarbon (crude oil) to enter the atmosphere. Initial results of this work reveal that crude oil droplets, formed by treatment with dispersants, can burst through physical forces and form aerosolized droplets containing crude oil. This oil-containing aerosol could be carried long distances by wind in the atmosphere and potentially pose health threats to humans and wildlife when inhaled or upon coming in contact with skin.
Marine Oil Snow
Marine oil snow (MOS) is essentially marine snow, with the exception that it distinctively contains oil hydrocarbons. Current knowledge recognizes its formation to be confined to the sea surface where oil slicks form in the event of an oil spill, but further work is needed to determine if MOS can also form in the subsurface. MOS can be described as a mucilaginous organic matter with a “fluffy” or gelatinous off-white appearance that contains oil droplets embedded within its amorphous matrix. Previous reports described evidence of MOS formation during the Ixtoc-I (Boehm and Fiest, 1980; Jernelöv and Lindén, 1981; Patton et al., 1981) and Tsesis (Johansson et al., 1980) oil spills (Teal and Howarth, 1984). However, MOS only recently received considerable attention when copious quantities of it, of macroscopic cm-size dimensions, were observed within 2 weeks of the Deepwater Horizon (DWH) blowout in the Gulf of Mexico – a spill recorded as the worst oil spill disaster in US history. MOS was encountered frequently around the vicinity of surface oil slicks at DWH (Niu et al., 2011; Passow et al., 2012). It eventually sank to the seafloor in the Gulf of Mexico – a process described termed MOSSFA (Marine Oil Snow Sedimentation and Flocculent Accumulation), which contributed a significant role in the export of crude oil (ca. 14% of the oil released at DWH) to the sediment (Valentine et al., 2014).
Conjecture still surrounds MOS genesis at DWH and during the Ixtoc-I and Tsesis oil spills, but its formation and sedimentation appears to have been directly associated with the influx of crude oil. In roller-bottle experiments performed under conditions attempting to simulate sea surface oil slicks at the DWH spill, the presence of crude oil was shown to be an important factor in triggering MOS formation, and that MOS acted as hotspots for microorganism and oil-degrading enzyme activities (Ziervogel et al., 2012; Gutierrez et al., 2013). Bacterial and eukaryotic phytoplankton cells and/or their produced polymers (e.g., EPS) have been reported to induce MOS formation (Passow et al., 2012; Gutierrez et al., 2013; Passow, 2016), whilst there are reports describing conflicting results on the role of dispersants in this respect (Baelum et al., 2012; Fu et al., 2014; Kleindienst et al., 2015; Passow, 2016; Suja et al., 2017). Figure 2A shows MOS formation in a roller-bottle incubation containing synthetic seawater amended with crude oil and Alteromonas sp. strain TK-46(2) – an oil-degrading and EPS-producing bacterial strain that was found enriched in surface oil slicks in the Gulf of Mexico during the DWH spill (Gutierrez et al., 2013). Like TEP, MOS particles can be rich in acidic sugars of polysaccharides, such that may be produced by EPS-producing bacteria like strain TK-46(2) (Figure 2B).
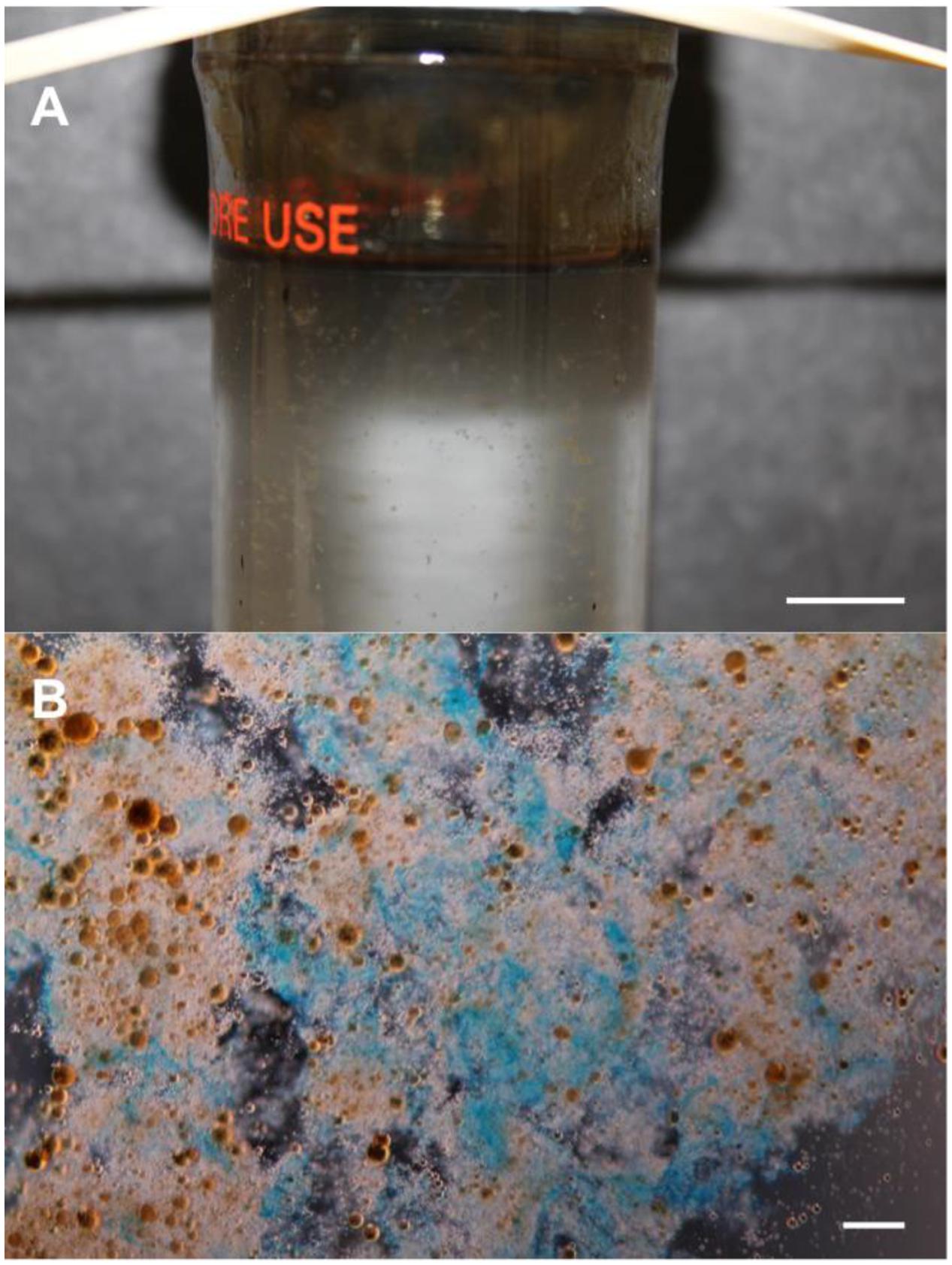
FIGURE 2. Formation of marine oil snow (MOS). (A) A roller-bottle incubation showing the formation of MOS in synthetic seawater amended with crude oil and inoculated with the EPS-producing (and oil-degrading) bacterium Alteromonas sp. strain TK-46(2) that was isolated from sea-surface oil slicks during the Deepwater Horizon oil spill. (B) Light micrograph of MOS aggregates, after staining with the cationic copper phthalocyanine dye Alcian Blue showing that the aggregates are partially composed of polysaccharide. The orange-brown spheres in (B) are emulsified oil droplets embedded within and adsorbed to the amorphous matrix of the aggregates. Scale bars = 10 mm (A), and = 10 μm (B).
Despite the interest in MOS formation as a product of spilled oil into the Gulf of Mexico, the microorganisms associated with MOS particles have received less attention. During incubations with uncontaminated deep-water samples collected during the active phase of the DWH oil spill and amended with the dispersant Corexit, Baelum et al. (2012) reported the formation of MOS, which was dominated by members of the genus Colwellia. In a more in-depth study of the bacterial community associated with MOS, Arnosti et al. (2015) showed that MOS particles contained a bacterial community that was distinctly different from that found freely living (i.e., not associated to MOS) in the surrounding seawater environment. The MOS-associated community was dominated by oil-degrading and EPS-producing members of the Gammaproteobacteria, principally Cycloclasticus, Congregibacter, Haliela, Halomonas and Marinobacter, and included diverse members of the Alphaproteobacteria (principally the Roseobacter clade) and some members within the Bacteroidetes and Planctomycetes. Using CARD-FISH (catalyzed reporter deposition – fluorescence in situ hybridization), MOS particles formed in incubations with Macondo crude oil and the dispersant Corexit were dominated by members of the class Gammaproteobacteria, including the order Alteromonadales, which comprises oil-degrading and EPS-producing taxa (Kleindienst et al., 2015). Using Illumina MiSeq sequencing, Suja et al. (2017) showed MOS particles formed in subarctic waters to be enrichment with oil-degrading (Alcanivorax, Cycloclasticus, Thalassolituus, Marinobacter) and EPS-producing (Halomonas, Pseudoalteromonas, Alteromonas) bacteria, and included major representation by Psychrobacter and Cobetia with putative oil-degrading/EPS-producing qualities. Collectively, these studies indicate that MOS are hotspots where oil-degrading and EPS-producing bacteria are enriched, and the latter may provide a clue on the role of these organisms in MOS formation through their synthesis and release of ‘sticky’ EPS.
Whilst significant knowledge gaps exist in our understanding on MOS formation and its subsequent sedimentation to the sea floor, the influx of crude oil and its interaction with planktonic microorganisms, as well as with dissolved and colloidal organic polymers, such as TEP, and with nutrient and suspended mineral discharges from river effluents, appear to be important factors that warrant further investigation (see Daly et al., 2016 for a review).
Turnover and Stability
Dissolved organic carbon in the ocean can be classified into three broadly defined pools of carbon based on their turnover times: labile, semilabile, and refractory. Collectively, the concentrations of these three DOC pools typically range from 60 to 90 μmol/L in the upper ocean water column, decreasing with depth to 40 μmol/L in the deep sea (Hansell, 2002). Fluctuations in the concentration of DOC in the water column occurs over certain periods of the year, due largely to periods of elevated photosynthetic production since this is the main source that fuels each of these three classes of DOC. The combined effect of biological and physical processes that alter the concentrations of these three pools of DOC is represented in the size-reactivity-continuum model (Amon and Benner, 1996; Benner and Amon, 2015), for which microbial processing of these molecules is the major mechanism that leads to rendering them progressively more recalcitrant (Jiao et al., 2010).
Labile DOC
Labile DOC in seawater comprises substrates with short residence times (minutes to days) since they are consumed almost as quickly as they are produced or released into the water column. An example of a common labile substrate in seawater is glucose, which is on average found at concentrations from 0.001 to 1.0 μmol/L, depending on the ocean region (Rich et al., 1996, 1997; Benner, 2002; Skoog et al., 2002). Other mono-sugar substrates (monosaccharides) also exist in the water column at concentrations typically ranging from 0.002 to 0.8 μmol C/L (Benner, 2002). On average across the oceans, glucose is the most abundant simple sugar, with concentrations as high as 187 nM measured in unfiltered water of the Gulf of Mexico, and 490 nM in high-molecular-weight DOM of the equatorial Pacific (Skoog and Benner, 1997). Glucose has been shown to contribute significantly in supporting a major fraction of bacterial growth in many ocean systems (Rich et al., 1996, 1997; Grossart and Simon, 2002), though other studies have shown glucose to play a less significant role in this respect (Keil and Kirchman, 1999; Skoog et al., 1999, 2002; Kirchman et al., 2001). Turnover rates for glucose can depend on the ocean environment, including the availability of certain nutrients, varying from rapid (hours to days) to relatively slow (100s of days). For example, surface waters limited by inorganic phosphorous can limit bacterial consumption of labile DOC, such as glucose, and result in a longer-than-average residence time of the endogenous pool of the labile DOC (Thingstad et al., 1997).
With respect to the chemical composition of POC in seawater, the abundance of glucose may be related to the major roles that this monosaccharide plays in phytoplankton biology – polymers of glucose (glucans) are major storage compounds in phytoplankton. Galactose is the second most abundant sugar in seawater, and polymers of it (galactans) are major structural components of phytoplankton cell walls (Romankevich, 1984).
Another simple carbohydrate that also contributes to the total pool of labile DOC in the ocean is mannitol. It is one of the most abundant sugar alcohol compounds in nature (Stoop et al., 1996); it is found in bacteria, fungi, algae and higher plants, where it often acts as a compatible solute, among conferring other functions. In ocean systems, mannitol is a major product of photosynthetic organisms, like algae, whereupon this polyol is released following cell lysis to join the pool of labile DOC in the ocean.
Carbohydrate concentrations in seawater can be as high as 10 μmol/L and contribute a significant fraction to the pool of labile substrates. Dissolved polysaccharides, such as EPS produced by bacteria and algae, form a major fraction of the total carbohydrates in the water column (Benner, 2002). Since concentrations of monosaccharides are typically 10-fold lower than dissolved polysaccharides, this suggests they are likely cycled more rapidly. Polysaccharides nonetheless contribute to fueling a major fraction of bacterial activity in some marine environments. On average, however, concentrations of labile DOC are very low (<1 μmol/L), constituting less than 1% of total organic carbon in the upper water column of the ocean. These substrates could potentially sustain oligotrophic microbial populations in regions of poor nutrient availability, such as in the open ocean. Nonetheless, the labile DOC pool is continuously replenished on a yearly basis by trophic (phytoplankton and bacterial excretion) and non-trophic (viral lysis, grazing) processes (Nagata, 2000).
Semilabile DOC
Approximately half of the total pool of DOC in the upper ocean water column is classed as semilabile, and comprises substrates that are consumed over weeks to months. Concentrations of semilabile DOC typically range from 20 to 30 μmol/L in the ocean water column, and because it is consumed over this median time scale, it assumes that this pool of DOC is important in supporting bacterial growth over seasonal to annual time scales (Carlson et al., 1994; Repeta and Aluwihare, 2006). In some ocean systems, such as the Sargasso Sea, the total semilabile DOC can account for as much as 89% of the total DOC (Carlson et al., 1994). In this study, up to 50% of this semilabile DOC was found to be more resistant to microbial degradation over weeks to months.
Interestingly, DOC produced in high-nutrient environments has been observed to be less susceptible to microbial degradation than that produced in low-nutrient environments. This may relate to the chemical qualities of the DOC produced in these contrasting environments – DOC produced in high-nutrient waters may be more nutrient rich than that produced in low-nutrient waters (Church, 2008). Semilabile DOC can accumulate as a result of inorganic nutrient limitation of bacterial growth (Thingstad et al., 1997), primarily from a limitation in (Cotner et al., 1997; Rivkin and Anderson, 1997; Thingstad et al., 1998; Zohary and Robarts, 1998; Caron et al., 2000). Other studies, however, have not found evidence to support the hypothesis that inorganic nutrient limitation of bacterial growth leads to accumulation of semilabile DOC. Rather, the chemical nature of this DOC class, specifically acting as a poor substrate for bacterial degradation, likely contributes to its accumulation in the water column. This is especially the case in the upper water column where DOC concentrations are higher than in the mesopelagic. This could influence the microbial communities in these contrasting regions of the water column where microbes in the upper water column have been found to degrade semilabile DOC less rapidly than those communities found in the mesopelagic (Carlson et al., 2004).
Refractory DOC
Much of the DOC in the ocean consists of low-molecular-weight solutes of <1000 Da, the majority of which comprises the refractory pool of DOC that is resistant to microbial degradation over time scales of 1000s of years – anywhere between 4000 and 6000 years (Williams and Druffel, 1987) – approaching or exceeding that of ocean circulation (Benner et al., 1992). Whilst concentrations of labile and semilabile DOC vary with depth, that of refractory DOC averages ca. 40 μmol/L throughout the water column with little to no variation with depth, and likely contributes insignificantly as a source of carbon and energy to bacterioplankton. However, a fraction of refractory DOC at the sea surface is destroyed by ultraviolet irradiation, which results in the release of labile DOC for heterotrophs to consume (Moran and Zepp, 2000). The refractory pool contributes to the sequestration of enormous quantities of carbon and acts as a carbon sink in the water column (Hedges, 2002). A recent study combining organic matter size, 14C age and elemental composition of DOC estimated that small refractory molecules in the ocean are produced by microorganisms and at a rate of 0.24 PgC per year, which is on par in magnitude to the burial of organic carbon in sediments (Walker et al., 2016).
Major sources of this refractory DOC pool include complex cell fragments and other high-molecular-weight biopolymers produced by cells that are partially or almost totally recalcitrant to biodegradation. During viral lysis of eukaryotic phytoplankton cells, cellular components that are highly refractory to degradation are released into the water column. Similarly, phage-mediated lysis of bacterial cells leads to the release of outer-membrane proteins of the cell envelope, which also are very resistant to degradation. Conversely, highly labile cell components, such as nucleic acids and amino acids, are recycled in the photic zone.
A proportion of the DOC released through cell lysis, including that produced extracellularly by living cells, is converted by chemical mechanisms into humic substances. This complex material is quite resistant to biodegradative processes and contributes to the total pool of refractory organic matter in the water column. Either by agglomeration of these humic substances, or their attachment to other sinking particles, a proportion of this refractory material eventually sinks to the ocean floor and becomes buried. Not all refractory DOC in the marine water column, however, sinks to the seafloor. Rather, most of it remains suspended and circulating in the ocean for years to millennia. In fact, of the fixed organic carbon formed by primary production, only a very small fraction reaches the seafloor; much of it (>99%) is remineralized in the water column through microbial action.
Extracellular polymeric substance produced by marine bacteria, even that which is freshly produced, can be somewhat refractory to microbial degradation or chemical analysis (Ogawa et al., 2001). This is believed to be related to the presence of uronic acids (Anton et al., 1988; Bejar et al., 1996) or glycosidic linkages of hexosamines (Biermann, 1988). Such constituents can confer on EPS molecules a high resistance to degradation under acid hydrolysis conditions that are used to chemically analyze them. Some studies analyzing the chemical nature of EPS isolated from cultured marine bacterial strains have shown a major proportion of these macromolecules (up to 80%) can be unaccounted for by chemical analysis (Gutierrez et al., 2007a,b, 2008).
Physical/Chemical Properties of EPS
Extracellular polymeric substance comprise an expanding plethora of biochemical molecules that interact in many ways and that are, as yet, poorly understood. EPS consist of an array of molecules, ranging from quite large (e.g., >100 kDa) to much smaller (e.g., <10 kDa) polymers. Some of the molecules contribute to the structural stability, gel properties, and pliancy of the greater matrix (Flemming, 2016). We must determine how different types of molecules in this matrix interact with each other in order to provide the observed functional roles of the EPS matrix. An entire rethinking of the extracellular milieu of microorganisms will likely be required. An insightful examination is provided by Neu and Lawrence (2016).
Composition and Physical Properties
From the standpoint of microbial cells, the EPS, especially when in a gel state, form a three-dimensional matrix or scaffold within which cells can orient themselves relative to one another. The presence of certain polymers can afford the matrix physical stability. Polymers such as amyloids and/or eDNA (discussed below) can serve as an architectural framework for the EPS. Each type of EPS component can offer different physical properties (Chew et al., 2014). The physical ultrastructure of the EPS matrix has been very difficult to image in a fully hydrated state (Decho, 1999), owing to its delicate nature. Excellent pioneering efforts have been conducted by Dohnalkova et al. (2011) using a unique cryo-TEM approach. Recent developments in cryo-TEM and -SEM may provide further insights into this complex matrix.
Bacterial and microalgal EPS exist in nature in a range of different physical states, most of which are operationally defined. Capsules consist of polymers that closely surround individual or multiple cells and often serve a protective role (Whitfield, 2006). Further away from cells, EPS can exist as tight, dense-gels, to a continuum of physical states from looser-slime to truly dissolved forms. Dissolved forms, in the absence of cells, may condense to microgels in the open ocean (Chin et al., 1998; Verdugo et al., 2004; Verdugo and Santschi, 2010); a process that is of significant importance and has been well-summarized in a review (Verdugo, 2012). While differences in these physical states are relatively arbitrary, they likely serve very different functions to the microbial cells secreting them. The physical state of EPS results from a combination of the polymer concentrations, types and abundances of ions, composition, and steric availability of functional groups on polymers. Recently, this has been reviewed in greater detail (Neu and Lawrence, 2016).
Some microbial EPS can exhibit significant viscoelastic properties, being able to stretch and retract in response to an applied force such as intermittent water flow (Fabbri and Stoodley, 2016). This property offers the microbial cells contained within a biofilm with a certain degree of physical flexibility and mechanical resiliency (Peterson et al., 2015). This flexibility is important to the ability of cells to persist on a surface or as an aggregate in the water-column.
Compositional information is a useful starting point for investigating EPS. Strictly speaking, the term EPS is an operational designation that refers to the milieu of ‘larger’ molecules contained in the extracellular matrix in proximity to cells (Wotton, 2004). Many of the molecules contained in natural EPS cannot be conveniently characterized as either protein, lipid or carbohydrate. For this reason, it has been called the ‘dark matter’ of biofilms (Flemming et al., 2007). It is now known that the EPS matrix can be quite heterogeneous, especially over small spatial scales (see Microdomains within EPS Matrices). It is for this reason that analyses of ‘bulk’ samples will not capture the smaller-scale variability that are critical to understanding the physical and chemical properties of the in situ matrix. When EPS from natural biofilms, for example, are extracted and then reconstituted, the physical (e.g., gel, viscocity, rheology, etc.) properties of the reconstituted EPS often do not readily resemble those of the original biofilm. (In much the same way, a cell cannot be taken apart via extractions, and then put back together as a functioning cell.) This suggests that the molecules within the matrix may have important molecular organization either by purposeful design or by result of the environment. It is important from a functional standpoint, to determine which molecules and molecular interactions contribute to physical properties such as gel formation, rheology, and diffusion-slowing, and chemical properties, such as sorption.
Finally, while matrix is actively secreted by cells, the properties of EPS may be changed post-secretion, modified by geochemical, enzymatic, and photochemical processes, and additionally contain trapped or sorbed molecules. These modifications may have dramatic effects on their physical properties. Thus, EPS under natural conditions exist in a ‘continuum’ of compositional and partial degradation states. Thus, it is imperative that future non-destructive approaches (e.g., Raman spectroscopy) are developed for characterizations of EPS in situ.
Laboratory studies of bacteria secreting EPS show that cells typically produce sugar monomers that are exported, then assembled to the existing polymer outside of the outer membrane (Whitfield, 2006; Sutherland, 2016). Polymers may consist of single sugar monomers, called homopolymers, or consist of several monomers linked together to form a repeating unit, called heteropolymers. Since several types of repeating units may be generated, this provides the cell with the capability to alter the physical chemical properties by mixing different amounts of repeating units. By changing the building blocks (i.e., repeating units), the polymer composition can be varied. This allows the polymer to have a variable composition and provides the cell the capability to modify its extracellular polymers in response to changing conditions.
At present, there are no specific biosignatures that can be assigned reliably for detection of EPS, simply because similar glycol-based compounds are produced throughout biological systems for many purposes. The secretion of carbohydrates and other glycosylated polymers is not unique to bacteria or even microorganisms; rather, it is a universal biological strategy employed by microalgae, fungi, invertebrate and vertebrate animals, and plants (Underwood and Paterson, 2003).
Extracellular polymeric substance have often been considered synonymous with ‘exopolysaccharides’ and acronym EPS. This was partially based on the carbohydrate-focus of investigators at the time, and additionally due to the artifact of carbon-rich culture conditions that were used to grow bacteria and obtain abundant quantities of EPS. Much has been learned regarding polysaccharide chemistry from these seminal studies. While polysaccharides are a major component of many natural EPS, they are only a component. The EPS matrix is now known to contain several different major groups of molecules, whose roles and involvement are still under study, and will be discussed briefly below. Hence, much discussion has evolved about what exactly constitutes the EPS matrix. Three points emerge as one studies the EPS of natural systems: (1) Many different types of molecules interact to provide the physical structure, impart chemical properties, and even actively manipulate EPS properties for the cell; (2) EPS-compositional studies should not be limited to culture-based systems; and (3) EPS under natural conditions will likely exist in a continuum of partial-degradation states.
Polysaccharides
The polysaccharide components of EPS are perhaps the best-studied to date. Common carbohydrate components that are often found in EPS include monomers such as D-glucose, D-galactose, D-mannose, L-fucose, L-rhamnose, D-glucuronic acid, D-galacturonic acid, L-guluronic acid, D-mannuronic acid, N-acetyl-D-glucosamine, and N-acetyl-D-galactosamine (Sutherland, 2001, 2016). Polysaccharides such as cellulose, alginic acid, dextran, xanthan, and Vibrio exopolysaccharide (VPS) are examples of polysaccharides produced by bacteria (for reviews see Decho, 1990; Wotton, 2004; Serra et al., 2013; Hobley et al., 2015).
The exopolysaccharide portion can exert significant net effects on physical and sorptive properties of EPS (Salek and Gutierrez, 2016). The presence of polar negative-charged groups such as carboxyls, phosphates and sulfate esters can provide negative charges (Thornton et al., 2007; Gutierrez et al., 2009). In certain microbial mat systems, highly sulfated exopolysaccharides have been isolated, and contained up to near 30% (wt/wt) in sulfate (Moppert et al., 2009).
In the presence of (positive) divalent cations (e.g., Ca2+, Mg2+) they can form cation bridges with adjacent polymers having also negative functional groups. EPS having abundant uronic acids often complex in this manner. Direct linkages between adjacent EPS can also occur. For example, linkages between a cationic polysaccharide and (anionic) extracellular-DNA has been shown to contribute to the physical stability of bacterial biofilms (Jennings et al., 2015). The abilities of EPS to link with each other is dependent, in part, on pH, the presence of appropriate functional groups, and the steric availability of functional groups (Decho, 1990; Ulrich, 2009).
Microdomains within EPS Matrices
The natural EPS matrix is now known to be heterogeneous over small spatial scales (e.g., μm, even nm). In order to describe small, localized areas that exhibited different properties than the broader matrix, polysaccharide chemists long ago developed the term ‘microdomain.’ The microdomain concept was extended to EPS (Decho, 2000a) to begin understanding why intact EPS exhibited very different properties than those of the extracted bulk EPS. Microdomains can result from localized concentrations of certain monomeric components of polymers, and/or differential binding of adjacent polymers to each other. Evidence for microdomains within in situ expolymer matrices has been evidenced by the careful combination of fluorescent lectin probes and confocal scanning laser microscopy (Lawrence et al., 2007, 2016; Aldeek et al., 2013). Microdomains are now realized to contribute to the smaller-scale heterogeneity that is observed within both suspended aggregate- and attached-biofilms. The presence of microdomains re-enforces the idea that the EPS matrix is not an amorphous, homogeneous entity, but rather can be structured at several different levels (Mayer et al., 1999; Decho, 2000a; Lawrence et al., 2003).
Proteins
Proteinacious moieties are common in natural EPS matrices and occur in a variety of molecular forms such as peptides, amino-sugars, glycoproteins, proteoglycans, and amyloid proteins (Gutierrez et al., 2007a,b; Fong and Yildiz, 2015; Zhang et al., 2015). They also can be grouped by their functions and properties such as extracellular enzymes (e-enzymes), membrane vesicle proteins, adhesins, amyloids, hydrophobins, and amphiphiles (Hobley et al., 2013). It is not well-understood yet, how proteins interact with other matrix molecules to accomplish these apparent functions.
While many proteins and peptides are easily hydrolyzed by microbial heterotrophy, certain proteins and peptides can be quite refractory to degradation. It is now realized that structured refractory complexes, called amyloid fibrils are a common component of EPS. These have not received much attention in oceanic environments but may contribute to a number of forms of refractory organic matter, and the refractory portions of EPS. Here, they may have important functions. Amyloids may form an important, refractory structural component of the EPS matrix (Gebbink et al., 2005; Larsen et al., 2007; Zheng et al., 2015).
Amyloids are loosely defined as any fibrillary polypeptide aggregate having a cross-β-quaternary structure (Fandrich, 2007), which self-assemble under the right environmental conditions. Amyloid fibrils consist of sets of 4–6 peptides linked together in a twisting, helical (i.e., rope-like) structure that is held together by non-covalent associations. A growing body of evidence supports the idea that amyloid fibrils, sometimes called curli fibers, may be formed from many different proteins (and peptides) and are a generic structure of peptide chain. While amyloid formation has been linked to many human disease processes (Barnhart and Chapman, 2006; van Gerven et al., 2015), they occur in natural microbial systems as a component of EPS. Their formation, at present, is thought to result from non-biological processes (Romero et al., 2010).
Extracellular e-DNA
A growing body of research now acknowledges the presence of extracellular forms of deoxyribonucleic acids (eDNA), and their role as an important structural component of the biofilm matrix (Böckelmann et al., 2005). Historically, eDNA was thought to result largely from the lysis of cells or release of plasmids. However, seminal studies by Whitchurch et al. (2002) showed the presence of eDNA as a part of the EPS. Concentrations of eDNA in sediments are often 3–4 orders of magnitude higher than those in the water-column, and suggest a role in the cycling of P in marine systems (Dell’Anno and Corinaldesi, 2004). Both eDNA and extracellular nucleases, together, may influence the physical consistency of biofilm EPS (Rice et al., 2007; Seper et al., 2011). Results of other studies indicated secretion by bacteria of eDNA is an active process (Nishimura et al., 2003; Steinberger and Holden, 2005; Suzuki et al., 2009; Gloag et al., 2013; Okshevsky and Meyer, 2013; Tang et al., 2013). The postulated roles suggest that eDNA may be a bacterial strategy that serves as an abundant structural scaffold within EPS. For example, in non-marine systems, the Gram-negative bacterium Pseudomonas aeruginosa uses eDNA bound to a specific cationic extracellular polysaccharide pel to provide structural stability to the EPS of biofilm (Jennings et al., 2015). Others have suggested an electron-transfer conduit, or substratum for the controlled movement of bound e-Enzymes (Flemming et al., 2007). Further studies await empirical testing of these ideas. However, an interesting caveat is that a recent study by Dell’Anno and Danovaro (2005) showed that DNA sorbed to sediments constituted an important source of phosphorus in normally P-limited deep-sea ecosystems. A review on eDNA pools in marine sediments summarizes many important aspects (Torti et al., 2015). Finally, DNA has been implicated in long-distance electron transfer processes (Giese, 2002). A pertinent question that warrants investigation is: does this offer the possibility for long-distance transfer of extracellular electrons through the EPS matrix?
Modifications Post-secretion
Extracellular polymeric substance, once-secreted by cells, are subjected to substantial environmental modifications, perhaps in predictable manners. Degradation of EPS may consist of a multi-step process. The steps likely represent the degradation of different components, ranging from highly labile to relatively refractory, which have different compositions and/or steric availabilities (to extracellular enzymes). A study of EPS produced within lithifying microbial mats showed that initial hydrolyses involved a rapid, and possibly selective, utilization by heterotrophs of certain sugar monomers, and LMW compounds. Certain components of the EPS, such as the uronic acids were highly labile to mat bacteria (Decho et al., 2005). Initial heterotrophic degradation of EPS was fueled by the large pool of LMW organics released by cyanobacteria during photosynthesis. This pool was consumed within 4–6 h post-daylight. The results indicated that a rapid, initial degradation occurred, followed by a much slower decomposition, leaving behind a more “refractory remnant” that persist for extended periods. As alluded to above, specific components of EPS such as many polysaccharides should exhibit relatively rapid turnover rates, when compared to more refractory components such as amyloid proteins. Finally, degradation will be affected by the physical properties of the EPS such as their gel versus solution states.
Sorption, Trapping, and Diffusion-Slowing Properties
Diffusion is a key process in the design of the microbial cell, as it is the primary means by which small organic molecules and ions may be taken up by cells. Diffusion is also of relevance in the movement of signal molecules (i.e., autoinducers) involved in QS, extracellular enzymes, and antimicrobial agents. EPS can be broadly considered a sorptive sponge for the binding, trapping and concentration of organics and ions (Decho, 1990). Over the small spatial scales of biofilms, the EPS matrix influences the diffusion process. Diffusion is a multi-faceted process that is influenced by temperature, ionic concentrations, etc. A major driver in diffusion, of course, is the relative concentration gradient (Brogioli and Vailati, 2001). The density and properties of the EPS can influence diffusion rates (of ions or molecules) so they can range negligible (compared to diffusion in pure water at the same temperature) to having significantly slowed diffusivities (Decho, 2015).
Although bulk measurements of diffusivity may be estimated, it is difficult to determine how the smaller-scale variability in EPS densities influence diffusion at these scales. The natural matrix of EPS within aggregates or surface biofilms is often filled with channels, which by microbial design or by environmental influence, enhances mass transfer to/from cells.
A number of investigators have carefully measured diffusion rate constants by monitoring the movement of fluorescent molecules over time using confocal scanning laser microscopy and other approaches (Lawrence et al., 1994; Guiot et al., 2002; Stewart, 2002; De Beer et al., 2004; Waharte et al., 2010; Neu and Lawrence, 2014). Lawrence et al. (1994) initially used fluorescent molecules and confocal scanning laser microscopy to examine diffusivities and found them to be variable, ranging from those of pure water (d = 1.0) to extreme diffusion-slowing effects (d = 0.02) by the matrix. From a practical standpoint, one can assume there will be variability within an aggregate or attached biofilm. Collectively, these studies have shown that considerable changes occur in the movement of molecules and ions over microspatial distances (i.e., μms), which can relate to the observed heterogeneity that occurs within biofilms (Stewart and Franklin, 2008).
Physical trapping of organic and inorganic colloids, and nanoparticles also occurs in the EPS matrix. The viscoelastic nature and the dispersed arrangements of EPS at the surface-most fringes of biofilms make them ideal for the physical trapping of colloids, small particles, and/or sorption of ions and molecules. Sorption is influenced by a number of factors. These include pH, the forms and concentrations of ion(s), and the type(s) of ligands (binding sites) and associations (e.g., ionic- and covalent-bonds, van der Waals forces, etc.). The pH can have a strong effect on ionic binding, especially with regard to many EPS (Braissant et al., 2007, 2009; Gutierrez et al., 2008). In general, acidic pH tends to inhibit ion binding, while neutral or basic pH tend to promote binding. A caveat is that not all ions bind equally. A second caveat is that certain functional groups bind ions more efficiently at a given pH. For example, as the pH rises to near neutral, more complexation may occur to carboxyl sites. The increase in binding of divalent cations often results in a more cohesive polymeric gel structure.
This pH-dependent sorption process has practical importance in ocean systems (and biofilms) because the most abundant divalent ions in seawater are Ca2+ and Mg2+. These ions often form suitable (bi-dentate) bridges between adjacent EPS molecules having carboxylic acid groups, and can contribute to gel formation or floc (marine snow) formation in the water column. However, other important ions (at a given pH) may ‘outcompete’ Ca2+ and Mg2+ for binding sites. The binding of transition and other metals, such as Th, Cd, Cu, Ag, Fe, and Se, to EPS isolated from different environments, such as hydrothermal vents, microbial mats, and other areas, has been described (Schlekat et al., 1998; Zhang et al., 2008; Moppert et al., 2009; Deschatre et al., 2013). Metal binding to EPS of surface floc material (i.e., marine snow) in the surface waters of oceans, and subsequent sinking of flocs may result in significant vertical transport (flux) of trace elements to ocean floor, a process of global biogeochemical significance (as mentioned above).
While ocean seawater is often pH 7.8–8.2, the range of pH over smaller spatial (and temporal) scales can be quite dramatic. In microbial mat systems, where highly active bacterial respiration and photosynthesis occur, the pH has been shown to vary from pH 6.0–10.0 over a 24 h (i.e., diel) cycle (Visscher et al., 2000; Des Marais, 2003; Baumgartner et al., 2006). This is due to net photosynthesis during daylight (which raise pH), and net respiration during darkness (which lowers pH). This can result in regular diel changes in the complexation of ions, and hence influence the physical stability of EPS over a 24 h cycle. The diffusion-slowing properties of EPS contribute to the sharp geochemical gradients often observed within aggregate and attached biofilms (Baumgartner et al., 2006).
Optical Properties
The biofilm is an organic gel coating (of EPS) on a surface (or within a suspended aggregate) with a collage of cells, and sorbed or localized molecules and ions, colloids, and particulates. All of these different components, individually or interactively, will influence the optical properties (i.e., refraction, scattering, and absorption of photons) of the broader surface (or water). The EPS can be thought of as a “semi-translucent” gel having different densities. Several processes act in concert to alter the optical properties of sediments. First, the polymers themselves appear to decrease the reflectance of the surface. In sediment systems, this can alter the amount of light entering the sediments. This is due to a combination of two processes. First, the gel polymers increase the spacing between sediment grains. This allows more light to enter in the spaces between grains, rather than being reflected from closely packed sediments. Second, the gel state of the polymer acts as a ‘photon trap’ because it mediates a change in refractive index, relative to seawater. This enhances the forward-scattering of photons, relative to back-scattering. This has been termed the ‘biofilm gel-effect’ (Decho et al., 2003). The functional value is that light may be more homogenously scattered around photosynthetic cells, and allow cells to conduct photosynthesis deeper in sediments (or mats).
As light interacts with a surface, photons are either reflected, scattered, refracted and/or absorbed. Reflectance involves back-scattering of photons at a fixed angle (relative to incident direction of photon). Often photons are ‘scattered’ at many angles relative to the incident. Refraction involves continuing through the surface, but altering the angle (relative to the incident) resulting in a change in refractive index. Absorbance involves the capture of photon energy by surface molecules or atoms. Absorbed photons may be re-emitted, as fluorescence (within pico-sec to nano-secs after absorbance), or released as heat. However, the biofilm is not simply a translucent gel but rather a three-dimensional matrix harboring cells, sorbed, or localized molecules (e.g., scytonemins, amino acids, etc.), colloids, and particulates. Biological chromophores (molecules that absorb light near specific wavelengths) include the purines and pyrimidines of DNA, the ‘ringed’ amino acids (tyrosine, phenylalanine, etc.), and other molecules. The sea surface layer is known to harbor EPS gels (Wurl and Holmes, 2008). Of special interest will be how the sea-surface layers of EPS influence photon penetration into the underlying water.
Localization of Microbial Extracellular Processes
Quorum Sensing
Do microbial communities communicate and coordinate activities? Classical microbiology during much of the past century has taught us to understand microbes simply as individual cells. Recently, however, a growing body of evidence supports the idea that bacteria often act in groups, rather than as individuals. When bacteria are attached, their proximity to each other results in the development of interactive relationships ranging from antagonistic to agonistic, and even altruistic. These interactions are often chemically mediated but are tempered by the ever-changing conditions of their local environment. The diffusion-slowing properties of the EPS matrix facilitates the development of such relationships among cells in a way that cannot be accomplished by free-living planktonic cells.
Quorum sensing is a type of bacterial cell–cell communication that involves the exchange of chemical signals among nearby cells to coordinate behaviors that are best conducted in groups (Fuqua et al., 1996). It involves the production, detection, and response by cells to diffusible signaling molecules (i.e., autoinducers). Autoinducers accumulate in the proximal environment as the bacterial population increases. When autoinducer concentration reaches a threshold-level, cells collectively alter gene expression. Many group activities such as bioluminescence, antibiotic production, and EPS secretion (Camilli and Bassler, 2006) are regulated by QS.
Quorum sensing can also be utilized by cells for ‘diffusion-sensing’ (Redfield, 2002). This allows bacteria to sense the diffusional properties of its proximal environment, presumably to ‘make decisions’ whether to conduct more metabolically costly processes, such as production and release of extracellular enzymes, plasmids, antibiotics, etc. (Ruparell et al., 2016). Together, these two processes, quorum- and diffusion-sensing, have been termed ‘efficiency sensing’ (Hense et al., 2007).
The foundation for cell–cell cooperative interactions originally was proposed for explaining the bioluminescence by a marine luminescent bacterium, previously Photobacterium, renamed Vibrio fischeri, and then Aliivibrio fischeri, which was isolated from a small Hawaiian squid (Ruby and Nealson, 1977; Nyholm et al., 2000). Studies progressively showed that autoinducer molecules, upon reaching a threshold concentration in the medium, triggered changes in gene expression that resulted in bacterial luminescence. Luminescence by populations of symbiotic bacteria, localized in the light organs of the squid, afforded it a selective advantage against predation. The ability to communicate, coordinate, and act as groups, however, does not relinquish the cells as an individual unit. Microbial cells can (and do) still act as individual cells. This amazing flexibility likely contributes to the tremendous success and resiliency of bacteria.
In open surface-water ocean environments, QS can have large-scale effects, especially when in overwhelming abundances. An obvious example of this was the ‘milky ocean’ that was observed at night by satellite off of Somalia, Africa (Nealson and Hastings, 2004; Miller et al., 2005). The milky ocean, which was 100s of square km in size, was due to QS-triggered bioluminescence in ocean surface populations of bacteria.
Several different classes of chemical signals exist. Most were described from the study of infection-causing bacteria. These include acylhomoserine lactones (AHL), unique oligopeptides, furanosyl borate diesters (Autoinducer-2), and gamma-butyrolactones (Waters and Bassler, 2005). The AHLs comprise a class of approximately 18 different types of signal molecules that are released into the surrounding environment, and eventually bind to an intracellular receptor protein, whose complex then triggers changes in gene expression (Churchill and Chen, 2011). AHLs in marine environments have been found in sponges (Taylor et al., 2004), microbial mats (McLean et al., 1997; Decho et al., 2009, 2010) and marine snow (Hmelo et al., 2011). Interestingly, signals such as AHLs are prone to inactivation under certain environmental conditions such as high pH (>8.0) (Decho et al., 2009; Hmelo and van Mooy, 2009). It is not known how fluctuating conditions (e.g., pH, oxidants, desiccation, photocatalytic degradation) in natural environments influence chemical signaling and coordination of microbial activities (Horswill et al., 2007; Decho et al., 2009, 2011; Frey et al., 2010). Signaling, however, will likely be localized and most pronounced within EPS matrices, and within planktonic aggregates (Gram et al., 2002; Wagner-Dobler et al., 2005; Hmelo et al., 2011; Amin et al., 2015; Jatt et al., 2015).
Signaling using AHLs, for example, is not limited to heterotrophic bacteria. Rather it has been found in photosynthetic cyanobacteria (Sharif et al., 2008) and Archaea (Zhang et al., 2012). Importantly, it is now realized that this form of cell–cell communication can occur in single-species population, but may also be utilized by inter-Kingdom consortia, such as plant-microbe and animal–microbe associations. Contrastingly, molecules that may act as signals for some bacteria, may act as antibiotics against other bacteria (Kaufmann et al., 2005; Davies et al., 2006; Schertzer et al., 2009; Johnson et al., 2016). A final note is that currently only several classes of signaling molecules (e.g., AHLs, AI-2, and peptides, diffusible signal factors (DSFs), etc.) are known (Schaefer et al., 2008; Papenfort and Bassler, 2016, for review). However, QS and similar interactions via chemical signaling are likely to occur using a variety of signals; most of which may be unknown at present.
Extracellular Vesicles and Gene-Exchange
Bacteria possess the capability to bud-off portions of their cell membranes (Schooling et al., 2009; Biller et al., 2014), which are then released as extracellular vesicles. The vesicles provide bacteria with the ability to package molecules within a surrounding lipid membrane, and release them in their surrounding extracellular environment, and localize them within the EPS matrix. This can provide a protective ‘minefield’ against antibiotics, preserve extracellular signals and plasmids, and provide other functions as well. The presence of extracellular vesicles within biofilms, and specifically the EPS matrix, is now realized to be quite common (Mashburn and Whiteley, 2005; Biller et al., 2014). The vesicle composition is often similar to the plasma membrane (Gram-positives) or outer cell membrane (in Gram-negatives), but additionally contain specific proteins as part of the vesicle. The vesicles can package a wide range of molecules such as eDNA, RNA, e-enzymes, antibiotics, and signal molecules, and likely provide protective effects for the packaged molecules they carry (Mashburn-Warren et al., 2008; Schooling et al., 2009).
Gene exchange is an important process among bacteria. The EPS matrix can enhance gene exchange among cells for several reasons. First, conjugation (i.e., a uni-directional exchange of plasmids via a pilus connecting two cells) requires extended contact for a prolonged period of time (approximately 20 min). In open-water systems, this is difficult due to Brownian motion constraints. When localized in a three-dimensional EPS matrix, two cells can remain relatively stationary for prolonged periods of time, which can facilitate conjugative gene exchange. Second, extracellular DNA, used in transformation, can be rapidly degraded once outside of the cell, or strongly sorbed to sediment particles. When DNA is immobilized within EPS, its persistence can be enhanced, and thus increase chances for transformational exchange of DNA among cells. Direct measurements of these two processes, to our knowledge, are not yet available.
Extracellular Enzymes (e-Enzymes) and Hydrolysis products
Degradation of organic matter and its mineralization to CO2 is a fundamental process of bacteria. In many ocean environments, bacteria produce e-enzymes to partially hydrolyze organic matter that becomes sorbed or trapped by the EPS (Hoppe et al., 2001). When conducted efficiently, with minimal loss to the surrounding water, the biofilm can be an efficient external digestion system for the microbial community (Flemming and Wingender, 2010).
The localization of e-enzymes is a process that is important in open water aggregate- as well as attached-biofilms. In order for efficient diffusional uptake, both enzymes and their hydrolysis products must remain localized in proximity to cells (e.g., approximately 30 μm). EPS provide a matrix to localize both enzymes and their hydrolysis products relatively close to cells. It is not known, however, how e-enzymes remain localized within the EPS matrix. Are they attached (bonded) to polymers with active sites exposed? In studies of other systems, bacteria are known to localize polysaccharases and other e-enzymes (Sutherland, 1999, 2016). e-Enzymes are also known to be contained within extracellular vesicles, localized within the expolymer matrix (Mashburn and Whiteley, 2005; Mashburn-Warren et al., 2008; Elhanawy et al., 2014), and e-DNA nucleases were found in Vibrio cholerae biofilms (Seper et al., 2011). Indeed, elevated microbial activities, such as enzymatic activities, have been reported in marine snow particles at higher-levels than those in surrounding sea water (Smith et al., 1992; Ploug et al., 1999; Grossart et al., 2003; Jatt et al., 2015), thus suggesting that marine snow are hotspots for remineralization of organic and inorganic materials (Azam and Long, 2001; Thornton et al., 2010).
Insight has been provided through studies of other systems (Tielen et al., 2013). They showed that extracellular lipase was protected against heat denaturation via complexation with the EPS alginate. Using molecular modeling they were able to show that e-enzymes can be physically bound to EPS, however, the enzyme/EPS bond must occur away from active sites on the enzyme. Finally, this bonding provides enhanced stability against denaturation. However, questions remain, such as: how are enzymatic activities maintained outside the cell? Empirical evidence has been relatively limited. Do the functional equivalents of extracellular chaperones help to maintain activities (i.e., prevent denaturation) of e-enzymes? It is also not known how extracellular enzymes may modify the EPS themselves.
EPS in Microbial Mats and Mineral Precipitation
Microbialites are benthic microbial deposits (Burne and Moore, 1987). Microbial mats, a type of microbialite, are the longest-lived ecosystems that are known to have existed on Earth. Certain fossilized microbialites extend far back in the fossil record (Sprachta et al., 2001; for review, see Chagas et al., 2016). They are the earliest known macro-fossil evidence of life in the geological record, extending back an estimated 3.4–3.7 gy (Tice and Lowe, 2004; Nutman et al., 2016). They dominate the fossil record for 3 gy, which represents over 80% of the time life has existed on Earth (Allwood et al., 2007). Recently, the precipitation process has been studied at nanometer spatial scales (Benzerara et al., 2006).
Microbial mats typically exhibit a distinct vertical layering of microbial functional groups that is strongly influenced by externally influenced gradients such as light and geochemical conditions (Des Marais, 2003; Vasconcelos et al., 2006; Franks and Stolz, 2009). In most cases, mats are examples of actively metabolizing, highly organized microbial communities, and constitute “high-yield” systems where resources are efficiently recycled amongst its members (Visscher and Stolz, 2005). These systems, therefore, offer excellent platforms from which to study how EPS may influence the precipitation of carbonate minerals. Thrombolites (Mobberley et al., 2015) and tufa deposits (Zippel and Neu, 2011; Dupraz et al., 2013) are other forms of microbialites.
Extracellular polymeric substances are abundantly present in microbialites, such as mats and contribute to the metabolic efficiency of mat communities (Neu, 1994). This occurs through their diffusion-slowing properties, light-attenuation, and abilities to influence 3D-architecture, chemical communication, extracellular enzymatic hydrolyses, and biogeochemical mineral precipitation. The details of how this relate to the molecular-scale interaction occurring between ions and the EPS are not, as yet, fully understood.
Carbonate Precipitation
Mats are well-known for their association with the biogeochemical precipitation of minerals such as carbonates (e.g., calcite, aragonite) (Decho, 2010). Present-day examples of precipitating mats include tufa mats, marine stromatolites, and marine thrombolites (see Dupraz et al., 2009, for review; Glunk et al., 2009; Tourney and Ngwenya, 2014). There are several different mechanisms known to directly or indirectly influence precipitation within mat environments (Visscher and Stolz, 2005). Microbial communities drive the basic alkalinity engine, which when coupled to the organic matrix of mats, results in biogeomineral precipitation (Dupraz and Visscher, 2005). Activities of several microbial groups, such as cyanobacteria, sulfate reducers, and anoxygenic phototrophs, can ‘promote precipitation,’ while other groups (e.g., aerobic heterotrophs, sulfur oxidizers, and fermenters) can ‘promote dissolution’ (Dupraz et al., 2009). Precipitation of CaCO3 occurs in seawater that is near or exceeding supersaturation of carbonate ions, and has basic pH conditions (Arp et al., 2001, 2003). Cyanobacterial activities, for example, will raise the pH during daylight photosynthesis, which favors localized carbonate precipitation (Gautret et al., 2004; Ludwig et al., 2005). Specific moieties on EPS, such as acidic groups, can act as nuclei for subsequent CaCO3 precipitation (Braissant et al., 2003; Bhaskar and Bhosle, 2005; Obst et al., 2009). Even bacterial cells themselves can serve as nucleation sites for precipitation (Varenyam et al., 2010). EPS can bind substantial amounts of free Ca2+ (Braissant et al., 2007) and other minerals such as phosphate and sulfate (Gallagher et al., 2013) from the surrounding water. This can result in precipitation of EPS-associated minerals such as apatite [Ca5(PO4)3(F,Cl,OH)], struvite (MgNH4PO4⋅6H2O), dolomite [CaMg(CO3)2], and aragonite (Gallagher et al., 2012, 2013).
Under some conditions, however, EPS can inhibit precipitation, or even contribute to carbonate dissolution. Cation-binding by EPS removes free Ca2+ ions from solution, through depletion of carbonate minerals from the proximal surroundings. Acidic amino acids, such as aspartic or glutamic acids, and carboxylated polysaccharides (i.e., groups of uronic acids) can act as strong inhibitors of CaCO3 precipitation (Kawaguchi and Decho, 2002a,b; Dupraz et al., 2004; Gautret and Trichet, 2005). The functional groups and their steric availability (to bind ions) are key in this process (Rieger et al., 2007; Yang et al., 2008). The role(s) in carbonate precipitation of specific microbial clades such as sulfate reducers, however, remains controversial (Meister, 2013), but likely involves different types of community interactions under normal marine, hypersaline, and alkaline conditions (Gallagher et al., 2014).
Marine stromatolites are microbial mats having repeating layers of precipitated micritic laminae produced through interaction of the microbial communities and the environment (Krumbein, 1983). In present day, they occur in only a few limited marine environments such as the Bahamas (Reid et al., 2000; Paerl et al., 2001) and Shark Bay in Western Australia (Goh et al., 2009). Studies extending for over a decade have examined present-day open-water, subtidal, marine stromatolites at Highborne Cay (Bahamas) and showed the surface microbial community consisted of several distinct mat stages (i.e., termed Types 1, 2, and 3), each having very different phenotypic characteristics (Reid et al., 2000). The EPS produced during these stages had very different properties and influenced the microbial communities within. In the Type 1 stage, the community exhibited “high growth.” It consisted of dense cyanobacteria with high EPS production that grew (upward) quickly, consuming resources (Decho et al., 2005). The abundant EPS resulted in a “sticky” surface that trapped ooid grains (i.e., sediment) washing over the mats during high wave actions; a process that propagated the continued upward growth of the mat. The EPS contained ligands to chelate much of the available free Ca2+ ions. The net result was that EPS in the Type 1 mat inhibited CaCO3 precipitation (Visscher et al., 2000). When a Type 1 mat transitions to a Type 2 mat, sulfate-reducing bacteria (SRB) increase in their relative abundances. EPS, initially produced by the cyanobacteria, are consumed by SRBs, then re-secreted as different EPS, a process which enhances localized precipitation (Visscher et al., 2000; Decho et al., 2005). Infared (FT-IR) spectral analyses of EPS extracted from the precipitate closely resembles those extracted from SRB mat isolates (Braissant et al., 2009). Notably, the EPS properties change in the Type 2 mat, becoming “less sticky.” These studies illustrated how the EPS properties of the different mat could influence their properties and growth. The Type 2 mat resembles a classic microbial mat, with less EPS production (or accumulation), and little/no upward growth. The cyanobacteria, SRB, sulfur-oxidizing bacteria (SOB), and aerobic heterotrophs become spatially organized, and exhibit a closer metabolically coupling.
With rising levels of atmospheric CO2, efforts are beginning to examine if certain carbonate-precipitating bacteria can be used to sequester (and store) CO2 (Paul et al., 2017) and understand how microbial mat processes influence C storage (Bouton et al., 2016).
Sediment Stabilization and Fossil Evidence of Mats
Sediment fluxes in marine systems are affected by many parameters, including sediment grain sizes, physically cohesive muds, and biologically cohesive microbial extracellular polymers (Gerbersdorf et al., 2009; Grabowski et al., 2011). EPS concentrations in marine sediments vary considerably (Underwood et al., 1995, 2004). EPS, especially those from microphytobenthos (e.g., diatomaceous mats), are important in cohesive sediment stability, and resistance against erosion and resuspension (Grant and Gust, 1987; Paterson, 1989; Smith and Underwood, 1998, 2000; Tolhurst et al., 2002; Underwood and Paterson, 2003; Hanlon et al., 2006). Levels of EPS present in sediments can be a crucial variable to sediment stability (Malarkey et al., 2015). However, more EPS isn’t always better. Interesting experimental studies by Paterson et al. (2008) showed that lower levels of EPS were more efficient in increasing erosional thresholds than abundant EPS conditions. While is well-established that the cohesive properties of EPS contribute to sediment stability, it is however not well-understood how the molecular-scale interactions of EPS themselves contribute to their cohesiveness (Paterson et al., 2008). Sediment-inhabiting small animals may indirectly influence sediment stability. For example, increased EPS production was shown to occur in the presence of a grazing nematode (Hubas et al., 2010). Initial studies suggest that QS (see above) may be involved in biofilm formation in certain diatoms (Yang et al., 2016) and offers the possibility that QS may contribute to the sediment stabilization process.
Finally, the very same bedform patterns that contribute to sediment stability in present-day sediments (e.g., ripples) also are considered as fossil evidences of sediment stabilization. These include patterns such as microbially induced sedimentary structures (MISSs), which are considered to be indirect fossil remnants that illustrate the very earliest vestiges of microbial mat life through geologic time (Noffke et al., 2001, 2013; Noffke, 2010).
Animal–microbial Interactions and Food-webs
Feeding Studies
It was realized early on that biofilms, and more-specifically their EPS, can represent a potentially labile carbon source for animals ingesting microbial cells (Decho, 1990). Since many small animals, present in both the water column and sediments of ocean systems, ingest microbial flora as a food source, they will coincidently ingest the closely associated EPS during the feeding process. Many invertebrate taxa are filter-feeders (i.e., straining suspended particles from the water) or deposit-feeders (i.e., ingesting sediments and their organics), therefore will consume microbial flora and their associated EPS as a food. Initial feeding experiments addressing EPS utilization by marine animals were conducted using EPS that were isolated from bacterial cultures grown in the presence of radioactively labeled (14C) substrates. Once the EPS were separated from cells and residual label, EPS were mixed with sediments and fed to animals. Results indicated that EPS comprised a highly labile carbon food source. Examples of such studies involved copepods (Decho and Moriarty, 1990), polychaete worms (Decho and Lopez, 1993), bivalves (Harvey and Luoma, 1985), and sea stars (Hoskins et al., 2003). Biofilms are even known to be grazed upon by benthic foraminifera (Bernhard and Bowser, 1992).
Extracellular polymeric substance can act as a ‘sorptive sponge.’ This is due to their ability to bind metals, other ions, and even relatively hydrophobic organic contaminants such as pesticides, which is attributed to the presence of charged moieties (i.e., positive or negatively charged functional groups) or hydrophobic moieties. This can result in the efficient trophic-transfer of metals and pesticides to consumer animals that are ingesting EPS. Together, these moieties serve to sorb and/or trap, and concentrate environmental contaminants. When animals ingest the matrix, coincidentally during their feeding on sediments, cells or flocs, they will consume the sorbed metals and organics as well. EPS have been shown to be an efficient trophic-transfer vehicle for sorbed metals in amphipods (Schlekat et al., 1998, 1999, 2000; Selck et al., 1999), and organic compounds such as pesticides, although most of the latter work has even been conducted in freshwater systems (Widenfalk et al., 2008; Lundqvist et al., 2010, 2012).
Binding of Nanoparticles to EPS and Biofilms
In ocean systems, both natural (e.g., geological) processes and anthropogenic processes result in the generation of extremely small particulates called nanoparticles. Nanoparticles measure 1–100 nm in at least one dimension, and have different physicochemical properties than larger particles. In oceans, nanoparticles occur in the form of metal contaminants, organics, and even degraded plastics and potentially may have long water-column residence times owing to their small sizes. A number of studies are indicating that nanoparticles are efficiently concentrated by biofilms, and more specifically their EPS (Battin et al., 2009; Ferry et al., 2009; Fabrega et al., 2011; Nevius et al., 2012; Ikuma et al., 2014, 2015). Further studies have shown that nanoparticles are taken up by protozoans (Holbrook et al., 2008; Werlin et al., 2011; Mortimer et al., 2016). Finally, the bioavailability and bioaccumulation of nanoparticles occurs in marine (and freshwater) animals such as snails, bivalves and oligochaetes (for review, see Luoma et al., 2014), all of which coincidently ingest EPS during feeding processes. Therefore, EPS can work as a trophic transfer vehicle for nanoparticles to enter food webs.
EPS Capsules, Survival of Digestion, and the Gut Microbiome
Bacteria often possess EPS capsules surrounding their cells. The presence of capsules is a protective measure for the cells. Studies conducted by Plante and colleagues showed that encapsulated bacteria were less susceptible to digestion during detritivore- and deposit-feeding (Plante et al., 1990; Plante and Schriver, 1998; Plante, 2000). DePas et al. (2014) showed that protection is afforded to biofilm bacteria during grazing by the nematode Caenorhabditis elegans.
Finally, one understudied aspect of trophic interactions regarding biofilms that is gaining attention involves the roles of resident gut bacteria in consumer animals (including humans). The presence of these bacteria, whose densities and activities may be quite substantial, is often facilitated by EPS capsules and biofilms. Gut bacteria are a source of many new genes, and the diversity and ecological principles driving these microbiomes will be an interesting future area of study (Dorosz et al., 2016).
The Larval Settlement Process and Biofouling
Virtually any type of surface, when placed in seawater becomes fouled with organisms ranging from bacteria to animals and algae; a process known as biofouling (Lewin, 1984). Bacteria and other microbes are generally the initial colonizing organisms of a surface. The presence of a bacterial biofilm often sets the stage for subsequent larval settlement (Tran and Hadfield, 2011). Understanding how biofilms interact with larval settlement is important to the broader biofouling process, which constitutes a costly ocean-engineering problem.
Studies in marine systems suggest that larval settlement is also a multistep process, which involves initial sensing of specific chemical cues, initial settlement and “tasting” of the surface, and finally more-permanent settlement. The initial sensing step of waterborne cues is a concentration-dependent process.
Presently, data suggest that in many cases, the larval settlement cues are molecules that are produced by adult conspecifics and are concentrated within the surface biofilm matrix or, in some cases, may be produced by the biofilms themselves (Unabia and Hadfield, 1999; Bao et al., 2007). Cue(s) can be multifunctional, acting as agonists, antagonists, or toxins (Ferrer and Zimmer, 2012; Guezennec et al., 2012). Behaviors and responses of larvae to cues are often species-dependent.
Settlement cues may be localized within the biofilm, and more-specifically by the EPS matrix. Diatom biofilms and the possible involvement of heat-stable settlement cues are involved in the settlement of the polychaete Hydroides elegans (Lam et al., 2003). Hydroides sp. are examples of the initial colonizers of open surfaces in warmer water regions. Once they are set, the complexity of colonizing species increases. Settlement cues may be produced by the biofilm itself but has been challenging to verify. The beneficial effect of a specific epibiotic bacterial biofilm on marine animal or plant hosts has been suggested (Holmström et al., 1992; Tran and Hadfield, 2011). Coralline red algae, for example, are highly inductive surfaces for the settlement of marine invertebrates, and are now realized to be strongly influenced by the surface microbial flora and their cues (Nielsen et al., 2015).
In contrast, the inhibition of larval settlement seems to be influenced by waterborne or biofilm-associated molecules. This is important to the potential control of biofouling. Early studies noted that many marine animals and macroalgae exhibit reduced biofouling, and suggested that chemical defenses may be involved (Holmström et al., 2002; Rao et al., 2007). More recently, studies have shown that specific chemical inhibitors can be produced by either the host organism (e.g., algae, animal) or by biofilm bacteria growing on the surface of the host (Lau and Qian, 2000). These have included both large and small molecules. In tunicates, proteins produced by biofilm bacteria inhibit further colonization by bacteria (Holmström et al., 1992). In a series of landmark studies, de Nys et al. (2009) showed the macroalga Delissia pulchra, was not subject to biofouling. This inhibition (of biofouling) operates by jamming the cell–cell chemical communication pathways of bacteria. As mentioned above, many gram-negative bacteria use AHLs in chemical communication. Release of specific AHL analogs, which are similar in molecular design can “jam” the QS pathway(s) of AHLs. Small halogenated furanones, resembling AHLs, interfered with chemical signaling in bacteria. An ecological function of antifouling molecules produced by plants and animals was postulated (and was tested) by Kjelleberg and colleagues (Franks et al., 2006). Harder et al. (2002) found large (>100 kDa) polysaccharide-containing molecules, produced by both a host macroalga (Ulva sp.) and Vibrio sp. bacteria, to inhibit larval settlement. These molecules act as a broad-spectrum inhibitor for settlement. This has touched off substantial exploration for chemically based inhibitors of biofouling in both nature and medicine by many laboratories. This infers the complex interaction in biofouling among host organisms, bacterial biofilms, and chemical cues. Since the biofouling of marine surfaces has both positive and negative effects to hatcheries, this area has an emerging impact on aquaculture processes (Joyce and Utting, 2015; Camacho-Chab et al., 2016). Finally, work is in progress to understand how climate change may affect processes such as larval settlement (Whalan and Webster, 2014).
Extreme Ocean Environments
In extreme and fluctuating conditions, microbes surround themselves with EPS in an effort to add stability to their extracellular environment. The physiological plasticity of microbial cells, combined with their EPS-based adaptations allow microbial life to succeed at the boundaries of where other forms of life can survive.
Low-temperature Sea-ice Communities
In polar regions, metabolic processes are slowed by relatively cold temperatures. It is here that microbes also employ EPS to their advantage. Earlier, pioneering studies in Antarctic systems showed the presence of specific ‘anti-freeze proteins’ (i.e., glycoproteins) within the blood plasma of fish (Devries, 1971). The proteins would bind to ice crystals as they formed and prevent further growth of damaging ice crystals in the blood. This realization launched many subsequent studies of other organisms, including bacteria.
In Arctic and Antarctic systems, the presence of EPS play key roles as cryoprotectants, for attachment to sea-ice interfaces, and to survive enclosure in ice (Underwood et al., 2010, 2013). Studies of bacterial isolates from sea-ice systems have demonstrated that certain glycoproteins and exopolysaccharides act as cryoprotectants, which inhibit ice crystal nucleation, in addition to securing the attachment of cells to the ice surface (Nichols C. A. et al., 2005; Nichols C. M. et al., 2005; Marx et al., 2009; Ewert and Deming, 2014). EPS and TEP become routinely embedded in sea-ice (Meiners et al., 2003; Collins et al., 2008) and contribute to the survival of microbial cells in these environments (Krembs et al., 2002, 2011; Liu et al., 2013; Boetius et al., 2015). EPS, including TEP can account for the majority of the carbon pool in sea-ice, which is later released during melting (Miller et al., 2011; Wurl et al., 2011), and can even make their way into atmospheric ice (Wilson et al., 2015).
High-temperature Hydrothermal Vents
Since their initial discovery in 1977, ocean hydrothermal vent systems have received much scientific attention. They are located near specific regions of the ocean spreading centers of tectonic plates, where geothermally heated fluids, enriched in minerals, hydrogen sulfide, ammonia and methane are released and mix with much colder surrounding seawater. Mineral deposits form as chimneys, and are surrounded by islands of intense biological activity, where chemosynthetic bacteria and archaea form the base of a food web having a diversity of often unique animals (Tunnicliffe, 1991). For these reasons, they have been considered as a possible site for the origin of life on Earth, and as an analog for study in the exploration for possible life elsewhere.
Isolates of bacteria from vent systems demonstrate the capacity for abundant EPS production, perhaps to sequester dissolved minerals and other metals from the surrounding water (Raguénès et al., 1997a,b; Guezennec et al., 1998; Rougeaux et al., 1999, 2001; Guezennec, 2002). EPS, derived from isolate cultures, typically have uronic acid contents as high as 40%, and relatively high molecular masses (Guezennec, 2002). It is not yet understood, however, how the EPS may influence the microenvironment of the bacteria and archaea, in terms of e-enzymes, 3D-microspatial development of their communities, and microspatial acidification (to solubilize metal ions). It is not known if the EPS matrix facilitates these processes, and actually may serve to inhibit their precipitation, similarly to those in some shallow-water carbonate environments?
Hypersaline Environments and Desiccation
Hypersaline systems, such as salt ponds, salterns, and hypersaline lagoons, contain well-developed microbial mats. Many of these systems occur in proximity or directly connected to ocean systems, while others are inland. Examples of hypersaline systems are numerous and a few include Salt Pond, San Salvador, Bahamas (Pinckney and Paerl, 1997); Guerrero Negro, Baha California Sur, Mexico (Ley et al., 2006); Laguna Tebenquiche, Salar de Atama, Chile (Fernandez et al., 2016); Don Juan Pond [McMurdo Dry Valleys, Antarctica (Dickson et al., 2013)]; Dead Sea (Oren, 1994); Solar Lake, Sinai, Egypt (Teske et al., 1998); Hamelin Pool, Shark Bay, Western Australia (Goh et al., 2009); Polynesian islands (Rougeaux et al., 2001; Richert et al., 2005; Moppert et al., 2009).
The hypersaline environment presents unique challenges to microorganisms, especially in terms of fluctuations in ion concentrations and osmolarity. In addition, many hypersaline mats are exposed to intermittent and/or progressive desiccation. A lack of available water, during the desiccation process, can kill a bacterial cell, largely through denaturation of proteins and destabilization of cell membranes (see Potts, 1994, for review). EPS are an abundant component of such mats (Benninghoff et al., 2016), and likely provide a degree of protection to mat microbial flora against ion fluctuations and desiccation (Potts, 1994; Shaw et al., 2003; Decho, 2016). Interestingly, studies have shown that bacteria can survive in a desiccated state in salt crystal for 250 my (Vreeland et al., 2000).
In hypersaline mats, EPS occur in the form of capsules surrounding individual cells, or a larger EPS matrix surrounding many cells in a biofilm, which can buffer cells against either dessication or rapid changes in water potential. Salinities in hypersaline ponds are often >300 g/L (e.g., seawater is approximately 32 g/L), but can reach as high as 440 g/L, often with concentrations of individual ions not matching those observed for typical seawater. Here, mat communities often experience extended periods (i.e., days to months) of desiccation that often is followed by a rapid rehydration due to seasonal rain events.
Selective saltation also plays a role in the ability of mats to cope with increasing salinities. This allows less-soluble minerals to be removed from solution, as a function of concentration. The evaporation process that occurs throughout the dry season serves to increase ionic concentrations and promotes the selective precipitation of salts on the mat surface. As ionic concentrations increase, there is a sequential salting-out occurring of less-soluble minerals. For example, much of the Ca2+ is typically removed as gypsum (CaSO4 2H2O) or smaller amounts of calcite (CaCO3). Gypsum begins to precipitate when salinity concentrations reach about 160 ppt. At very high ionic concentrations (>300 ppt) NaCl begins to precipitate.
Some EPS may condense with increasing salinity, and even form a hydrophobic barrier on the surface of the biofilm. This may result in enhanced protection during subsequent desiccation. It has been proposed that the exclusion of ions occurs via the EPS matrix in response to increasing salinity, which is designed to reduce osmotic stress and conserve water within the mat (Decho, 2016). Desiccation is a process occurring in many areas of marine environments. On the fringes of ocean systems, specifically on the upper reaches of rocky intertidal zones, intermittent desiccation is a common process. The roles of EPS in stabilizing microbial communities require further investigation.
Summary: EPS Research Looking Forward
The growing awareness of microbial EPS and their influences on ocean processes are evidenced in this special issue and offers many avenues for future research. It is emphasized here that the secretion of EPS is an adaptive response employed by microbes to enhance their metabolic efficiency and survival. An extensive literature on EPS and biofilms that is available in other areas of microbiology may have relevance to ocean studies.
Finally, there were many aspects of EPS that were not covered in this relatively short overview, but are important to understanding the dynamics of microbial extracellular biology. For example, we have not addressed: (1) EPS as electron-transfer vehicles; (2) the concentration of viruses; (3) molecular pathways of EPS secretion; and (4) the roles of biofilms in the search for life elsewhere. In addition, we anticipate that the roles of EPS in ocean systems will be integrated into the fundamental microbiology of the ocean, and into larger-scale topics such as global climate change, biotechnological applications of EPS, and the search for novel antibiotics and other medicinal compounds.
Author Contributions
All authors listed, have made substantial, direct and intellectual contribution to the work, and approved it for publication.
Conflict of Interest Statement
The authors declare that the research was conducted in the absence of any commercial or financial relationships that could be construed as a potential conflict of interest.
Acknowledgments
The authors wish to thank the many colleagues and collaborators, whose in-depth discussions have initially fueled, productively challenged and gradually refined many of the ideas put forth here. This work was supported, in part, by grants from the U.S. National Science Foundation to AD: Collaborative Research in Chemistry (CRC-0526821), Environmental Genomics (En-Gen-0723707) and BioMaterials (DMR-1608151) Programs.
References
Aldeek, F., Schneider, R., Fontaine-Aupart, M. P., Mustin, C., Lécart, S., Merlin, C., et al. (2013). Patterned hydrophobic domains in the exopolymer matrix of Shewanella oneidensis MR-1 biofilms. Appl. Environ. Microbiol. 79, 1400–1402. doi: 10.1128/AEM.03054-12
Alldredge, A. L., and Cohen, Y. (1987). Can microscale chemical patches persist in the sea? Microelectrode study of marine snow, fecal pellets. Science 235, 689–691. doi: 10.1126/science.235.4789.689
Alldredge, A. L., Cole, J. J., and Caron, D. A. (1986). Production of heterotrophic bacteria inhabiting macroscopic organic aggregates (marine snow) from surface waters. Limnol. Oceanogr. 31, 68–78. doi: 10.4319/lo.1986.31.1.0068
Alldredge, A. L., Passow, U., and Logan, B. E. (1993). The abundance and significance of a class of large, transparent organic particles in the ocean. Deep Sea Res. 40, 1131–1140. doi: 10.1016/0967-0637(93)90129-Q
Alldredge, A. L., and Silver, M. W. (1988). Characteristics, dynamics and significance of marine snow. Prog. Oceanogr. 20, 41–82. doi: 10.1016/0079-6611(88)90053-5
Allwood, A. C., Walter, M. R., Burch, I. W., and Kamber, B. S. (2007). 3.43 billion-year-old stromatolite reef from the Pilbara Craton of Western Australia: ecosystem-scale insights to early life on Earth. Precambr. Res. 158, 198–227. doi: 10.1016/j.precamres.2007.04.013
Aluwihare, L. I., Repeta, D. J., and Chen, R. F. (1997). A major biopolymeric component of dissolved organic carbon in surface seawater. Nature 387, 166–169. doi: 10.1038/387166a0
Amin, S. A., Hmelo, L. R., van Tol, H. M., Durham, B. P., Carlson, L. T., Heal, K. R., et al. (2015). Interaction and signaling between a cosmopolitan phytoplankton and associated bacteria. Nature 522, 98–101. doi: 10.1038/nature14488
Amon, R. M. W., and Benner, R. (1994). Rapid cycling of high-molecular-weight dissolved organic matter in the ocean. Nature 369, 549–552. doi: 10.1038/369549a0
Amon, R. M. W., and Benner, R. (1996). Bacterial utilization of different size classes of dissolved organic matter. Limnol. Oceanogr. 41, 41–51. doi: 10.4319/lo.1996.41.1.0041
Anton, J., Meseguer, I., and Rodriguezvalera, F. (1988). Production of an extracellular polysaccharide by Haloferax-mediterranei. Appl. Environ. Microbiol. 54, 2381–2386.
Arnosti, C., Ziervogel, K., Yang, T., and Teske, A. (2015). Oil-derived marine aggregates – hot spots of polysaccharide degradation by specialized bacterial communities. Deep Sea Res. II 129, 179–186. doi: 10.1016/j.dsr2.2014.12.008
Arp, G., Reimer, A., and Reitner, J. (2001). Photosynthesis-induced biofilm calcification and calcium concentration in Phanerozoic oceans. Science 292, 1701–1704. doi: 10.1126/science.1057204
Arp, G., Reimer, A., and Reitner, J. (2003). Microbialite formation in seawater of increased alkalinity, Satonda Crater Lake, Indonesia. J. Sed. Res. 73, 105–127. doi: 10.1306/071002730105
Azam, F., and Long, R. A. (2001). Oceanography – Sea snow microcosms. Nature 414, 495–498. doi: 10.1038/35107174
Baelum, J., Borglin, S., Chakraborty, R., Fortney, J. L., Lamendella, R., Mason, O. U., et al. (2012). Deep-sea bacteria enriched by oil and dispersant from the Deepwater Horizon spill. Environ. Microbiol. 14, 2405–2416. doi: 10.1111/j.1462-2920.2012.02780.x
Bao, W.-Y., Satuito, C. G., Yang, J.-L., and Kitamura, H. (2007). Larval settlement and metamorphosis of the mussel Mytilus galloprovincialis in response to biofilms. Mar. Biol. 150, 565–574. doi: 10.1007/s00227-006-0383-4
Barnhart, M. M., and Chapman, M. R. (2006). Curli biogenesis and function. Annu. Revs. Microbiol. 60, 131–147. doi: 10.1146/annurev.micro.60.080805.142106
Battin, T. J., von der Kammer, F., Weilhartner, A., Ottofuelling, S., and Hofmann, T. (2009). Nanostructured TiO2: transport behavior and effects on aquatic microbial communities under environmental conditions. Environ. Sci. Technol. 43, 8098–8104. doi: 10.1021/es9017046
Bauer, J. E., Druffel, E. R. M., Wolgast, D. M., and Griffin, S. (2002). Temporal and regional variability in sources and cycling of DOC and POC in the northwest Atlantic continental shelf and slope. Deep Sea Res. II 49, 4387–4419. doi: 10.1016/S0967-0645(02)00123-6
Baumgartner, L. K., Reid, R. P., Dupraz, C., Decho, A. W., Buckley, D. H., Spear, J. R., et al. (2006). Sulfate reducing bacteria in microbial mats: changing paradigms, new discoveries. Sed. Geol. 185, 131–145. doi: 10.1016/j.sedgeo.2005.12.008
Beech, I. B., and Cheung, C. W. S. (1995). Interactions of EPS produced by sulphate-reducing bacteria with metal ions. Int. Biodeter. Biodegrad. 35, 59–72. doi: 10.1016/0964-8305(95)00082-G
Bejar, V., Calvo, C., Moliz, J., Diaz-Martinez, F., and Quesada, E. (1996). Effect of growth conditions on the rheological properties and chemical composition of Volcaniella eurihalina exopolysaccharide. Appl. Biochem. Biotechnol. 59, 77–86. doi: 10.1007/BF02787859
Benner, R. (2002). “Chemical composition and reactivity,” in Biogeochemistry of Marine Dissolved Organic Matter, eds D. A. Hansell and C. A. Carslon (San Diego, CA: Academic Press), 59–90. doi: 10.1146/annurev-marine-010213-135126
Benner, R., and Amon, R. M. W. (2015). The size-reactivity continuum of major bioelements in the ocean. Annu. Rev. Mar. Sci. 7, 185–205. doi: 10.1146/annurev-marine-010213-135126
Benner, R., Pakulski, J. D., McCarthy, M., Hedges, J. I., and Hatcher, P. G. (1992). Bulk chemical characteristics of dissolved organic matter in the ocean. Science 255, 1561–1564. doi: 10.1126/science.255.5051.1561
Benninghoff, J. C., Wingender, J., Flemming, H.-C., and Siebers, B. (2016). “Biofilms X-treme: composition of extracellular polymeric substances in Archaea,” in The Perfect Slime -Microbial Extracellular Polymeric Substances (EPS), eds H.-C. Flemming, T. R. Neu, and J. Wingender (London: IWA Publishers), 301–317.
Benzerara, K., Menguy, N., Lopez-Garcia, P., Yoon, T.-H., Kazmierczak, J., Typiszczak, T., et al. (2006). Nanoscale detection of organic signatures in carbonate microbialites. Proc. Natl. Acad. Sci. U.S.A. 103, 9440–9445. doi: 10.1073/pnas.0603255103
Bernhard, J. M., and Bowser, S. S. (1992). Bacterial biofilms as a trophic resource for certain benthic foraminifera. Mar. Ecol. Prog. Ser. 83, 263–272. doi: 10.3354/meps083263
Bhaskar, P. V., and Bhosle, N. B. (2005). Microbial extracellular polymeric substances in marine biogeochemical processes. Curr. Sci. 88, 45–53.
Bhaskar, P. V., and Bhosle, N. B. (2006). Bacterial extracellular poly- meric substances (EPS): a carrier of heavy metals in the marine food-chain. Environ. Int. 32, 191–198. doi: 10.1016/j.envint.2005.08.010
Bianchi, M., Marty, D., Teyssié, J. L., and Fowler, S. W. (1992). Strictly aerobic and anaerobic bacteria associated with sinking particulate matter and zooplankton fecal pellets. Mar. Ecol. Prog. Ser. 88, 55–60. doi: 10.3354/meps088055
Biermann, C. J. (1988). Hydrolysis and other cleavages of glycosidic linkages in polysaccharides. Adv. Carbohydr. Chem. Biochem. 46, 251–271. doi: 10.1016/S0065-2318(08)60168-7
Bigg, E. K. (2007). Sources, nature, and influence on climate of marine airborne particulates. Environ. Chem. 4, 155–161. doi: 10.1071/EN07001
Bigg, E. K., and Leck, C. (2008). The composition of fragments of bubbles bursting at the ocean surface. J. Geophys. Res. 113, D11209. doi: 10.1029/2007jd009078
Biller, S. J., Schubotz, F., Roggensack, S. E., Thompson, A. W., Summons, R. E., and Chisholm, S. W. (2014). Bacterial vesicles in marine ecosystems. Science 343, 183–186. doi: 10.1126/science.1243457
Böckelmann, U., Janke, A., Kuhn, R., Neu, T. R., Wecke, J., Lawrence, J. R., et al. (2005). Bacterial extracellular DNA forming a defined network-like structure. FEMS Microbiol. Lett. 262, 31–38. doi: 10.1111/j.1574-6968.2006.00361.x
Boehm, P. D., and Fiest, D. L. (1980). “Aspects of the transport of petroleum hydrocarbons to the offshore benthos during the Ixtoc-I blowout in the Bay of Campeche,” in Proceedings of the Symposium on the Preliminary Results from the September, 1979 Pierce/Research IXTOC-1Cruises, (Boulder, CO: NOAA).
Boetius, A., Anesio, A. M., Deming, J. W., Mikucki, J. A., and Rapp, J. Z. (2015). Microbial ecology of the cryosphere: sea ice and glacial habitats. Nat. Revs. Microbiol. 13, 677–690. doi: 10.1038/nrmicro3522
Bouton, A., Vennin, E., Boulle, J., Pace, A., Bourillot, R., Thomazo, C., et al. (2016). Linking the distribution of microbial deposits from the Great Salt Lake (Utah, USA) to tectonic and climatic processes. Biogeosciences 13, 5511–5526. doi: 10.5194/bg-13-5511-2016
Boyd, P. W., Jickells, T., Law, C. S., Blain, S., Boyle, E. A., Buesseler, K. O., et al. (2007). Mesoscale iron enrichment experiments 1993–2005: synthesis and future directions. Science 315, 612–617. doi: 10.1126/science.1131669
Braissant, O., Cailleau, G., Dupraz, C., and Verrechia, E. P. (2003). Bacterially induced mineralization of calcium carbonate in terrestrial environments: the role of exopolysaccharides and amino acids. J. Sed. Res. 73, 485–490. doi: 10.1306/111302730485
Braissant, O., Decho, A. W., Dupraz, C., Glunk, C., Przekop, K. M., and Visscher, P. T. (2007). Exopolymeric substances of sulfate-reducing bacteria: Interactions with calcium at alkaline pH and implication for formation of carbonate minerals. Geobiology 5, 401–411. doi: 10.1111/j.1472-4669.2007.00117.x
Braissant, O., Decho, A. W., Przekop, K. M., Gallagher, K. L., Glunk, C., Dupraz, C., et al. (2009). Characteristics and turnover of exopolymeric substances in a hypersaline microbial mat. FEMS Microbiol. Ecol. 67, 293–307. doi: 10.1111/j.1574-6941.2008.00614.x
Brogioli, D., and Vailati, A. (2001). Diffusive mass transfer by non-equilibrium fluctuations: Fick’s Law revisited. Phys. Revs. 63:012105. doi: 10.1103/PhysRevE.63.012105
Brussaard, C. P. D., Bidle, K. D., Pedrós-Alió, C., and Legrand, C. (2016). The interactive microbial ocean. Nat. Microbiol. 2:16255. doi: 10.1038/nmicrobiol.2016.255
Burne, R. V., and Moore, L. S. (1987). Microbialites: organosedimentary deposits of benthic microbial communities. Palaios 2, 241–254. doi: 10.2307/3514674
Camacho-Chab, J. C., Lango-Reynoso, F., del Refugio Castañeda-Chávez, M., Galaviz-Villa, I., Hinojosa-Garro, D., and Ortega-Morales, B. O. (2016). Implications of extracellular polymeric substance matrices of microbial habitats associated with coastal aquaculture systems. Water 8:369. doi: 10.3390/w8090369
Camilli, A., and Bassler, B. L. (2006). Bacterial small-molecule signaling pathways. Science 311, 1113–1116. doi: 10.1126/science.1121357
Carlson, C. A., Ducklow, H. W., and Michaels, A. F. (1994). Annual flux of dissolved organic carbon from the euphotic zone in the northwestern Sargasso Sea. Nature 371, 405–408. doi: 10.1038/371405a0
Carlson, C. A., Giovannoni, S. J., Hansell, D. A., Goldberg, S. J., Parsons, R., and Vergin, K. (2004). Interactions among dissolved organic carbon, microbial processes, and community structure in the mesopelagic zone of the northwestern Sargasso Sea. Limnol. Oceanogr. 49, 1073–1083. doi: 10.4319/lo.2004.49.4.1073
Caron, D. A., Lim, E. L., Sanders, R. W., Dennett, M. R., and Berninger, U. G. (2000). Responses of bacterioplankton and phytoplankton to organic carbon and inorganic nutrient additions in contrasting oceanic ecosystems. Aquat. Microbiol. Ecol. 22, 175–184. doi: 10.3354/ame022175
Chagas, A. A. P., Webb, G. E., Burne, R. V., and Southam, G. (2016). Modern lacustrine microbialites: toward a synthesis of aqueous and carbonate geochemistry and mineralogy. Earth Sci. Revs. 162, 338–363. doi: 10.1016/j.earscirev.2016.09.012
Chew, S. C., Kundukad, B., Seviour, T., van der Maarel, J. R. C., Yang, L., Rice, S. A., et al. (2014). Dynamic remodeling of microbial biofilms by functionally distinct exopolysaccharides. MBio 5:e1536-14. doi: 10.1128/mBio.01536-14
Chin, W.-C., Orellana, M. V., and Verdugo, P. (1998). Spontaneous assembly of marine dissolved organic matter into polymer gels. Nature 391, 568–572. doi: 10.1038/35345
Church, M. J. (2008). “Resource control of bacterial dynamics in the sea,” in Microbial Ecology of the Oceans, 2nd Edn, ed. D. L. Kirchman (Hoboken, NJ: John Wiley & Sons, Inc).
Churchill, M. E. A., and Chen, L. (2011). Structural basis of acyl-homoserine lactone-dependent signaling. Chem. Rev. 111, 68–85. doi: 10.1021/cr1000817
Collins, R. E., Carpenter, S. D., and Deming, J. W. (2008). Spatial heterogeneity and temporal dynamics of particles, bacteria, and pEPS in Arctic winter sea ice. J. Mar. Syst. 74, 902–917. doi: 10.1016/j.jmarsys.2007.09.005
Costerton, J. W., Cheng, K. J., Geesey, G. G., Ladd, T. I., Nickel, J. C., Dasgupta, M., et al. (1987). Bacterial biofilms in nature and disease. Annu. Rev. Microbiol. 41, 435–464. doi: 10.1146/annurev.mi.41.100187.002251
Cotner, J. B., Ammerman, J. W., Peele, E. R., and Bentzen, E. (1997). Phosphorus-limited bacterioplankton growth in the Sargasso Sea. Aquat. Microbiol. Ecol. 13, 141–149. doi: 10.3354/ame013141
Daly, K. L., Passow, U., Chanton, J., and Hollander, D. (2016). Assessing the impacts of oil-associated marine snow formation and sedimentation during and after the Deepwater Horizon oil spill. Anthropocene 13, 18–33. doi: 10.1016/j.ancene.2016.01.006
Davies, J., Spiegelman, G. B., and Grace, Y. (2006). The world of subinhibitory antibiotic concentrations. Curr. Opin. Microbiol. 9, 445–453. doi: 10.1016/j.mib.2006.08.006
De Beer, D., Stoodley, P., Roe, F., and Lewandowski, Z. (2004). Effects of biofilm structure on oxygen distribution and mass transport. Biotechnol. Bioeng. 43, 1131–1133. doi: 10.1002/bit.260431118
De Jong, E., van Rens, L., Westbroek, P., and Bosch, L. (1979). Biocalcification by the marine alga Emiliania huxleyi (Lohmann) Kamptner. Eur. J. Biochem. 99, 559–567. doi: 10.1111/j.1432-1033.1979.tb13288.x
de Nys, R., Steinberg, P. D., Willemsen, P., Dworjanyn, S. A., Gabelish, C. L., and King, R. J. (2009). Broad spectrum effects of secondary metabolites from the red alga Delisea pulchra in antifouling assays. Biofouling 8, 259–271. doi: 10.1080/08927019509378279
Decho, A. W. (1990). Microbial exopolymer secretions in ocean environments: their role(s) in food webs and marine processes. Oceanogr. Mar. Biol. Ann. Rev. 28, 73–153.
Decho, A. W. (1999). Imaging an alginate polymer gel matrix using atomic force microscopy. Carbohydr. Res. 315, 330–333. doi: 10.1016/S0008-6215(99)00006-3
Decho, A. W. (2000a). “Exopolymer microdomains as a structuring agent for heterogeneity with microbial biofilms,” in Microbial Sediments, eds R. E. Riding and S. M. Awramik (Berlin: Springer-Verlag Press), 9–15.
Decho, A. W. (2000b). Microbial biofilms in intertidal systems: an overview. Cont. Shelf Res. 20, 1257–1273. doi: 10.1016/S0278-4343(00)00022-4
Decho, A. W. (2010). Overview of biopolymer-induced mineralization: what goes on in biofilms? Ecol. Eng. 36, 137–144. doi: 10.1016/j.ecoleng.2009.01.003
Decho, A. W. (2015). “Localization of quorum sensing by extracellular polymeric substances (EPS): considerations of in situ signaling,” in The Physical Basis of Bacterial Quorum Communication, ed. S. J. Hagen (New York, NY: Springer), doi: 10.1007/978-1-4939-1402-9_6
Decho, A. W. (2016). “Unique and baffling aspects of the matrix: EPS syneresis and glass formation during desiccation,” in The Perfect Slime, eds H.-C. Flemming, T. R. Neu, and J. Wingender (London: IWA Publishing), 207–226. doi: 10.2166/9781780407418
Decho, A. W., Frey, R. L., and Ferry, J. L. (2011). Chemical challenges to bacterial AHL signaling in the environment. Chem. Rev. 111, 86–99. doi: 10.1021/cr100311q
Decho, A. W., Kawaguchi, T., Allison, M. A., Louchard, E. M., Reid, R. P., Stephens, C., et al. (2003). Sediment properties influencing upwelling spectral reflectance signatures: the “biofilm gel effect”. Limnol. Oceanogr. 48, 431–443. doi: 10.4319/lo.2003.48.1_part_2.0431
Decho, A. W., and Lopez, G. R. (1993). Exopolymer microenvironments of microbial flora: multiple and interactive effects on trophic relationships. Limnol. Oceanogr. 38, 1633–1645. doi: 10.4319/lo.1993.38.8.1633
Decho, A. W., and Moriarty, D. J. W. (1990). Bacterial exopolymer utilization by a harpacticoid copepod: a methodology and results. Limnol. Oceanogr. 35, 1039–1049. doi: 10.4319/lo.1990.35.5.1039
Decho, A. W., Norman, R. S., and Visscher, P. T. (2010). Quorum sensing in natural environmental: emerging views from microbial mats. Trends Microbiol. 18, 73–80. doi: 10.1016/j.tim.2009.12.008
Decho, A. W., Visscher, P. T., Ferry, J., Kawaguchi, T., He, L., Przekop, K. M., et al. (2009). Autoinducers extracted from microbial mats reveal a surprising diversity of N-acylhomoserine lactones (AHLs) and abundance changes that may relate to diel pH. Environ. Microbiol. 11, 409–420. doi: 10.1111/j.1462-2920.2008.01780.x
Decho, A. W., Visscher, P. T., and Reid, R. P. (2005). Production and cycling of natural microbial EPS (EPS) within a marine stromatolite. Palaeogeogr. Palaeoclimat. Palaeoecol. 219, 71–86. doi: 10.1016/j.palaeo.2004.10.015
Dell’Anno, A., and Corinaldesi, C. (2004). Degradation and turnover of extracellular DNA in marine sediments: ecological and methodological considerations. Appl. Environ. Microbiol. 70, 4384–4386. doi: 10.1128/AEM.70.7.4384-4386.2004
Dell’Anno, A., and Danovaro, R. (2005). Extracellular DNA plays a role in deep-sea ecosystem functioning. Science 309, 2179. doi: 10.1126/science.1117475
DeLong, E. F., Franks, D. G., and Alldredge, A. L. (1993). Phylogenetic diversity of aggregate-attached vs. free-living marine bacterial assemblages. Limnol. Oceanogr. 38, 924–934. doi: 10.4319/lo.1993.38.5.0924
DePas, W. H., Syed, A. K., Sifuentes, M., Lee, J. S., Warshaw, D., Saggar, V., et al. (2014). Biofilm formation protects Escherichia coli against killing by Caenorhabditis elegans and Myxococcus xanthus. Appl. Environ. Microb. 80, 7079–7087. doi: 10.1128/AEM.02464-14
Des Marais, D. J. (2003). Biogeochemistry of hypersaline microbial mats illustrates the dynamics of modern microbial ecosystems and the early evolution of the biosphere. Biol. Bull. 204, 160–167. doi: 10.2307/1543552
Deschatre, M., Ghillebaert, F., Guezennec, J., and Colin, C. S. (2013). Sorption of copper(II) and silver(1) by four bacterial exopolysaccharides. Appl. Biochem. Biotechnol. 171, 1313–1327. doi: 10.1007/s12010-013-0343-7
Devries, A. L. (1971). Glycoproteins as biological antifreeze agents in antarctic fishes. Science 172, 1152–1155. doi: 10.1126/science.172.3988.1152
Dickson, J. L., Head, J. W., Levy, J. S., and Marchant, D. R. (2013). Don Juan Pond, Antarctica: near-surface CaCl2-brine feeding Earth’s most saline lake and implications for Mars. Sci. Rep. 3:1166. doi: 10.1038/srep01166
Dohnalkova, A. C., Marshall, M. J., Arey, B. W., Williams, K. H., Buck, E. C., and Fredrickson, J. K. (2011). Imaging hydrated microbial extracellular polymers: comparative analysis by electron microscopy. Appl. Environ. Microbiol. 77, 1254–1262. doi: 10.1128/AEM.02001-10
Dorosz, J. N. A., Castro-Mejia, J. L., Hansen, L. H., Nielsen, D. S., and Skovgaard, A. (2016). Different microbiomes associated with the copepods Acartia tonsa and Temora longicornis from the same marine environment. Aquat. Microb. Ecol. 78, 1–9. doi: 10.3354/ame01799
Drake, L. A., Doblin, M. A., and Dobbs, F. C. (2007). Potential microbial bioinvasions via ships’ ballast water, sediment, and biofilm. Mar. Poll. Bull. 55, 333–341. doi: 10.1016/j.marpolbul.2006.11.007
Dupraz, C., Fowler, A., Tobias, C., and Visscher, P. T. (2013). Stomatolitic knobs in Storr’s Lake (San Salvador, Bahamas): a model system for formation and alteration of laminae. Geobiology 11, 527–548. doi: 10.1111/gbi.12063
Dupraz, C., Reid, R. P., Braissant, O., Decho, A. W., Norman, R. S., and Visscher, P. T. (2009). Processes of carbonate precipitation in modern microbial mats. Earth Sci. Revs. 96, 141–162. doi: 10.1016/j.earscirev.2008.10.005
Dupraz, C., and Visscher, P. T. (2005). Microbial lithification in marine stromatolites and hypersaline mats. Trends Microbiol. 13, 429–438. doi: 10.1016/j.tim.2005.07.008
Dupraz, C., Visscher, P. T., Baumgartner, L. K., and Reid, R. P. (2004). Microbe-mineral interactions: early carbonate precipitation in a hypersaline lake (Eleuthera Island, Bahamas). Sedimentology 51, 745–765. doi: 10.1111/j.1365-3091.2004.00649.x
Dupuy, C., Mallet, C., Guizien, K., Montanié, H., Bréret, M., Mornet, F., et al. (2014). Sequential resuspension of biofilm components (Viruses, prokaryotes and protists) as measured by erodimetry experiments in the Brouage mudflat (French Atlantic coast). J. Sea Res. 92, 56–65. doi: 10.1016/j.seares.2013.12.002
Elhanawy, W., Debelyy, M. O., and Feldman, M. F. (2014). Preferential packing of acidic glycosidases and proteases into bacteriodes outer membrane vesicles. mBio 5:e00909-14. doi: 10.1128/mBio.00909-14
Engel, A., and Passow, U. (2001). Carbon and nitrogen content of transparent exopolymer particles (TEP) in relation to their Alcian Blue adsorption. Mar. Ecol. Progr. Ser. 219, 1–10. doi: 10.3354/meps219001
Engel, A., Thoms, S., Riebesell, U., Rochelle-Newall, E., and Zondervan, I. (2004). Polysaccharide aggregation as a potential sink of marine dissolved organic carbon. Nature 428, 929–932. doi: 10.1038/nature02453
Ewert, M., and Deming, J. W. (2014). Bacterial responses to fluctuations and extremes in temperature and brine salinity at the surface of Arctic winter sea ice. FEMS Microbiol. Ecol. 89, 476–489. doi: 10.1111/1574-6941.12363
Fabbri, S., and Stoodley, P. (2016). “Mechanical properties of biofilms,” in The Perfect Slime, eds H.-C. Flemming, T. R. Neu, and J. Wingender (London: IWA Publishing), 153–177. doi: 10.2166/9781780407418
Fabrega, J., Zhang, R., Renshaw, J. C., Liu, W.-T., and Lead, J. R. (2011). Impact of silver nanoparticles on natural marine biofilm bacteria. Chemosphere 85, 961–966. doi: 10.1016/j.chemosphere.2011.06.066
Facchini, M. C., Rinaldi, M., Decesari, S., Carbone, C., Finessi, E., Mircea, M., et al. (2008). Primary submicron marine aerosol dominated by insoluble organic colloids and aggregates. Geophys. Res. Lett. 35, L17814. doi: 10.1029/2008gl034210
Fandrich, M. (2007). On the structural definition of amyloid fibrils and other polypeptide aggregates. Cell. Mol. Life Sci. 64, 2066–2078. doi: 10.1007/s00018-007-7110-2
Fernandez, A. B., Rasuk, M. C., Visscher, P. T., Contreras, M., Novoa, F., Poire, D. G., et al. (2016). Microbial diversity in sediment ecosystems (evaporate domes, microbial mats and crusts) of hypersaline Laguna Tebenquiche, Salar de Atama, Chile. Front. Microbiol. 7:1284. doi: 10.3389/fmicb.2016.01284
Ferrer, R. P., and Zimmer, R. K. (2012). Community ecology and the evolution of molecules of keystone significance. Biol. Bull. 223, 167–177. doi: 10.1086/BBLv223n2p167
Ferry, J. L., Craig, P., Hexel, C., Sisco, P., Frey, R., Pennington, P. L., et al. (2009). Transfer of gold nanoparticles from the water column to the estuarine food web. Nat. Nanotechnol. 4, 441–444. doi: 10.1038/nnano.2009.157
Flemming, H.-C. (2016). “The perfect slime – and the ‘dark matter’ of biofilms,” in The Perfect Slime: Microbial Extracellular Polymeric Substances (EPS), eds H.-C. Flemming, T. R. Neu, and J. Wingender (London: IWA Publishers), 1–14. doi: 10.2166/9781780407418
Flemming, H. C., Neu, T. R., and Wozniak, D. (2007). The EPS matrix: The house of biofilm cells. J. Bacteriol. 189, 7945–7947. doi: 10.1128/JB.00858-07
Flemming, H. C., and Wingender, J. (2010). The biofilm matrix. Nat. Rev. Microbiol. 8, 623–633. doi: 10.1038/nrmicro2415
Flemming, H.-C., Wingender, J., Kjelleberg, S., Steinberg, P., Rice, S., and Szewzyk, U. (2016). Biofilms: an emergent form of microbial life. Nat. Rev. Microbiol. 14, 563–575. doi: 10.1038/nrmicro.2016.94
Fong, J. N. C., and Yildiz, F. H. (2015). Biofilm matrix proteins. Microbiol. Spectr. 3. doi: 10.1128/microbiolspec.MB-0004-2014
Ford, T., Sacco, E., Black, J., Kelley, T., Goodacre, R. C., and Berkeley, R. C. W. (1991). Characterization of EPS of aquatic bacteria by pyrolysis-mass spectrometry. Appl. Environ. Microbiol. 57, 1595–1601.
Franks, A., Egan, S., Holmstrom, C., James, S., Lappin-Scott, H., and Kjelleberg, S. (2006). Inhibition of fungal colonization by Pseudoalteromonas tunicata provides a competitive advantage during surface colonization. Appl. Environ. Microbiol. 72, 6079–6087. doi: 10.1128/AEM.00559-06
Franks, J., and Stolz, J. F. (2009). Flat laminated microbial mat communities. Earth Sci. Rev. 96, 163–172. doi: 10.1016/j.earscirev.2008.10.004
Frey, R. L., He, L., Cui, Y., Decho, A. W., Kawaguchi, T., Ferguson, P. L., et al. (2010). Reaction of N-acylhomoserine lactones with hydroxyl radicals: rates, products, and effects on signaling activity. Environ. Sci. Technol. 44, 7465–7469. doi: 10.1021/es100663e
Fu, J., Gong, Y., Zhao, X., O’Reilly, S. E., and Zhao, D. (2014). Effects of oil and dispersants on formation of marine oil snow and transport of oil hydrocarbons. Environ. Sci. Technol. 48, 14392–14399. doi: 10.1021/es5042157
Fuentes, E., Coe, H., Green, D., de Leeuw, G., and McFiggans, G. (2010). On the impacts of phytoplankton-derived organic matter on the production of marine aerosol – Part 1: source fluxes. Atmos. Chem. Phys. 10, 9295–9317. doi: 10.5194/acp-10-9295-2010
Fuqua, W. C., Winans, S. C., and Greenberg, E. P. (1996). Census and consensus in bacterial ecosystems: the LuxR/Luxl family of quorum sensing transcriptional regulators. Annu. Rev. Microbiol. 50, 727–751. doi: 10.1146/annurev.micro.50.1.727
Gallagher, K. L., Braissant, O., Kading, T. J., Dupraz, C., and Visscher, P. T. (2013). Phosphate-related artifacts in carbonate mineralization experiments. J. Sed. Res. 83, 37–49. doi: 10.2110/jsr.2013.9
Gallagher, K. L., Dupraz, C., and Visscher, P. T. (2014). Two opposing effects of sulfate reduction on carbonate precipitation in normal marine, hypersaline, and alkaline environments – Comment. Geology 42, e313–e314. doi: 10.1130/g34639c.1
Gallagher, K. L., Kading, T. J., Braissant, O., Dupraz, C., and Visscher, P. T. (2012). Inside the alkalinity engine: the role of electron donors in the organomineralization potential of sulfate-reducing bacteria. Geobiology 10, 518–530. doi: 10.1111/j.1472-4669.2012.00342.x
Gautret, P., Camoin, G., Golubic, S., and Sprachta, S. (2004). Biochemical control of calcium carbonate precipitation in modern lagoonal microbialites, Tikehau atoll, French Polynesia. J. Sed. Res. 74, 462–478. doi: 10.1306/012304740462
Gautret, P., and Trichet, J. (2005). Automicrites in modern cyanobacterial stromatolitic deposits of Rangiroa, Tuamotu Archipelago, French Polynesia: biochemical parameters underlaying their formation. Sed. Geol. 178, 55–73. doi: 10.1016/j.sedgeo.2005.03.012
Gebbink, M. F. B. G., Claessen, D., Bouma, B., Dijkhuizen, L., and Wösten, H. A. (2005). Amyloids – a functional coat for microorganisms. Nat. Rev. Microbiol. 3, 333–341. doi: 10.1038/nrmicro1127
Gerbersdorf, S. U., Bittner, R., Lubarsky, H., Manz, W., and Paterson, D. M. (2009). Microbial assemblages as ecosystem engineers of sediment stability. J. Soils Sed. 9, 640–652. doi: 10.1007/s11368-009-0142-5
Giese, B. (2002). Long-distance electron transfer through DNA. Annu. Rev. Biochem. 71, 51–70. doi: 10.1146/annurev.biochem.71.083101.134037
Gloag, E. S., Turnbull, L., Huang, A., Vallotton, P., Wang, H., Nolan, L. M., et al. (2013). Self-organization of bacterial biofilms is facilitated by extracellular DNA. Proc. Natl. Acad. Sci. U.S.A. 110, 11541–11546. doi: 10.1073/pnas.1218898110
Glunk, C., Dupraz, C., Braissant, O., Gallagher, K. L., Verrecchia, E. P., and Visscher, P. T. (2009). Microbially mediated carbonate precipitation in a hypersaline lake, Big Pond (Eleuthera, Bahamas). Sedimentology 58, 720–736. doi: 10.1111/j.1365-3091.2010.01180.x
Goh, F., Allen, M. A., Leuko, S., Kawaguchi, T., Decho, A. W., Burns, B. P., et al. (2009). Determining the specific microbial populations and their spatial distribution within the stromatolite ecosystem of Shark Bay. ISME J. 3, 383–396. doi: 10.1038/ismej.2008.114
Grabowski, R. C., Droppo, I. G., and Wharton, G. (2011). Erodibility of cohesive sediment: the importance of sediment properties. Earth Sci. Rev. 105, 101–120. doi: 10.1016/j.earscirev.2011.01.008
Gram, L., Grossart, H.-P., Schlingloff, A., and Kiorboe, T. (2002). Possible quorum sensing in marine snow bacteria: production of acylated homoserine lactones by Roseobacter strains isolated from marine snow. Appl. Environ. Microbiol. 68, 4111–4116. doi: 10.1128/AEM.68.8.4111-4116.2002
Grant, J., and Gust, G. (1987). Prediction of coastal sediment stability from photopigment content of mats of purple sulfur bacteria. Nature 330, 244–246. doi: 10.1038/330244a0
Grossart, H.-P., Engel, A., Arnosti, C., De La Rocha, C., Murray, A., and Passow, U. (2007). Microbial dynamics in autotrophic and heterotrophic seawater mesocosms. III. Organic matter fluxes. Aquat. Microb. Ecol. 49,143–156. doi: 10.3354/ame01140
Grossart, H.-P., Kiørboe, T., Tang, K., and Ploug, H. (2003). Bacterial colonization of particles: growth and interactions. Appl. Environ. Microbiol. 69, 3500–3509. doi: 10.1128/AEM.69.6.3500-3509.2003
Grossart, H. P., and Simon, M. (2002). Bacterioplankton dynamics in the Gulf of Aqaba and the Northern Red Sea in early spring. Mar. Ecol. Prog. Ser. 239, 263–276. doi: 10.3354/meps239263
Guezennec, J. (2002). Deep-sea hydrothermal vents: a new source of innovative bacterial exopolysaccharides of biotechnological interests. J. Indust. Microbiol. Biotechnol. 29, 204–208. doi: 10.1038/sj.jim.7000298
Guezennec, J., Herry, J. M., Kouzayha, A., Bachere, E., Mittelman, M., and Fontaine, M. N. B. (2012). Exopolysaccharides from unusual marine environments inhibit early stages of biofouling. Int. Biodeterior. Biodegrad. 66, 1–7. doi: 10.1016/j.ibiod.2011.10.004
Guezennec, J., Pignet, P., Lijour, Y., Gentric, E., Ratiskol, J., and Colliec-Jouault, S. (1998). Sulfation and depolymerization of a bacterial exopolysaccharide of hydrothermal origin. Carbohydr. Polymers 37, 19–24. doi: 10.1016/S0144-8617(98)00006-X
Guiot, E., Georges, P., Brun, A., Fontaine-Aupart, M. P., Bellon-Fontaine, M. N., and Briandet, R. (2002). Heterogeneity of diffusion inside microbial biofilms determined by fluorescence correlation spectroscopy under two-photon excitation. Photochem. Photobiol. 75, 570–578. doi: 10.1562/0031-8655(2002)075<0570:HODIMB>2.0.CO;2
Guizien, K., Dupuy, C., Ory, P., Montainié, H., Hartmann, H., Chatelain, M., et al. (2014). Microorganism dynamics during a rising tide: disentangling effects of resuspension and mixing with offshore waters above an intertidal mudflat. J. Mar. Syst. 129, 178–188. doi: 10.1016/j.jmarsys.2013.05.010
Guo, L., Coleman, C. H. J., and Santschi, P. H. (1994). The distribution of colloidal and dissolved organic carbon in the Gulf of Mexico. Mar. Chem. 45, 105–119. doi: 10.1016/0304-4203(94)90095-7
Gutierrez, T., Berry, D., Yang, T., Mishamandani, S., McKay, L., Teske, A., et al. (2013). Role of bacterial exopolysaccharides (EPS) in the fate of the oil released during the Deepwater Horizon oil spill. PLoS ONE 8:e67717. doi: 10.1371/journal.pone.0067717
Gutierrez, T., Biller, D. V., Shimmield, T., and Green, D. H. (2012). Metal binding properties of the EPS produced by Halomonas sp. TG39 and its potential in enhancing trace element bioavailability to eukaryotic phytoplankton. Biometals 25, 1185–1194. doi: 10.1007/s10534-012-9581-3
Gutierrez, T., Morris, G., and Green, D. H. (2009). Yield and physicochemical properties of EPS from Halomonas sp. strain TG39 identifies a role for protein and anionic residues (sulfate and phosphate) in emulsification of n-hexadecane. Biotechnol. Bioeng. 103, 207–216. doi: 10.1002/bit.22218
Gutierrez, T., Mulloy, B., Bavington, C., Black, K., and Green, D. H. (2007a). Partial purification and chemical characterization of a glycoprotein (putative hydrocolloid) emulsifier produced by a marine Antarctobacter species. Appl. Microbiol. Biotechnol. 76, 1017–1026. doi: 10.1007/s00253-007-1091-9
Gutierrez, T., Shimmield, T., Haidon, C., Black, K., and Green, D. H. (2007b). Glycoprotein emulsifiers from two marine Halomonas species: chemical and physical characterization. J. Appl. Microbiol. 103, 1716–1727. doi: 10.1111/j.1365-2672.2007.03407.x
Gutierrez, T., Mulloy, B., Black, K., and Green, D. H. (2008). Emulsifying and metal ion binding activity of a glycoprotein exopolymer produced by Pseudoalteromonas sp strain TG12. Appl. Environ. Microbiol. 74, 4867–4876. doi: 10.1128/AEM.00316-08
Hall-Stoodley, L., Costerton, J. W., and Stoodley, P. (2004). Bacterial biofilms: from the natural environment to infectious diseases. Nat. Rev. Microbiol. 2, 95–108. doi: 10.1038/nrmicro821
Hanlon, A. R. M., Bellinger, B., Haynes, K., Xiao, G., Hofmann, T. A., Gretz, M. R., et al. (2006). Dynamics of extracellular polymeric substance (EPS) production and loss in an estuarine, diatom-dominated, microalgal biofilm over a tidal emersion-immersion period. Limnol. Oceanogr. 51, 79–93. doi: 10.4319/lo.2006.51.1.0079
Hansell, D. A. (2002). “DOC in the global ocean carbon cycle,” in Biogeochemistry of Marine Dissolved Organic Matter, eds D. A. Hansell and C. A. Carlson (San Diego, CA: Academic Press), 685–715. doi: 10.1016/B978-012323841-2/50017-8
Hansell, D. A., and Carlson, C. A. (1998). Deep-ocean gradients in the concentration of dissolved organic carbon. Nature 395, 263–268. doi: 10.1038/26200
Harder, T., Lam, C., and Qian, P. Y. (2002). Induction of larval settlement in the polychaete Hydroides elegans by marine bioWlms: an investigation of monospecifc diatom Wlms as settlement cues. Mar. Ecol. Prog. Ser. 229, 105–112. doi: 10.3354/meps229105
Harvey, R. W., and Luoma, S. N. (1985). Effect of adherent bacteria and bacterial cellular polymers upon assimilation by Macoma balthica of sediment bound Cd, Zn, and Ag. Mar. Ecol. Prog. Ser. 22, 281–289. doi: 10.3354/meps022281
Hassler, C. S., Alasonati, E., Mancuso-Nichols, C. A., and Slaveykova, V. I. (2011a). Exopolysaccharides produced by bacteria isolated from the pelagic Southern Ocean—role of Fe binding, chemical reactivity, and bioavailability. Mar. Chem. 123, 88–98. doi: 10.1016/j.marchem.2010.10.003
Hassler, C. S., Schoemann, V., Mancuso-Nichols, C., Butler, E. C. V., and Boyd, P. W. (2011b). Saccharides enhance iron bioavailability to Southern Ocean phytoplankton. Proc. Natl. Acad. Sci. U.S.A. 108, 1076–1081. doi: 10.1073/pnas.1010963108
Hassler, C. S., and Schoemann, V. (2009). Bioavailability of organically bound Fe to model phytoplankton of the Southern Ocean. Biogeosciences 6, 2281–2296. doi: 10.5194/bg-6-2281-2009
Hawkins, L. N., and Russell, L. M. (2010). Polysaccharides, proteins, and phytoplankton fragments: four chemically distinct types of marine primary organic aerosol classified by single particle spectromicroscopy. Adv. Meteorol. 2010, 612132. doi: 10.1155/2010/612132
Hedges, J. I. (2002). “Why dissolved organic matter,” in Biochemistry of Marine Dissolved Organic Matter, eds D. A. Hansell and C. A. Carlson (San Diego, CA: Academic Press), 685–715.
Hense, B. A., Kuttler, C., Muller, J., Rothballer, M., Hartmann, A., and Kreft, J.-U. (2007). Does efficiency sensing unify diffusion and quorum sensing? Nat. Rev. Microbiol. 5, 230–239.
Herndl, G. (1988). Ecology of amorphous aggregations (marine snow) in the northern Adriatic Sea: 2. Microbial density and activity in marine snow and its implication to overall pelagic processes. Mar. Ecol. Prog. Ser. 48, 265–275. doi: 10.3354/meps048265
Hmelo, L., Mincer, T. J., and Van Mooy, B. A. S. (2011). Possible influence of bacterial quorum sensing on the hydrolysis of sinking particulate organic carbon in marine environments. Environ. Microbiol. Rep. 3, 682–688. doi: 10.1111/j.1758-2229.2011.00281.x
Hmelo, L., and van Mooy, B. A. S. (2009). Kinetic constraints on acylated homoserine lactone-based quorum sensing in marine environments. Aquat. Microb. Ecol. 54, 127–133. doi: 10.3354/ame01261
Hobley, L., Harkins, C., MacPhee, C. E., and Stanley-Wall, N. R. (2015). Giving structure to the biofilm matrix: and overview of individual strategies and emerging common themes. FEMS Microbiol. Rev. 39, 649–669. doi: 10.1093/femsre/fuv015
Hobley, L., Ostrowski, A., Rao, F. V., Bromley, K. M., Porter, M., Prescott, A. R., et al. (2013). BslA is a self-assembling bacterial hydrophobin that coats the Bacillus subtilus biofilm. Proc. Natl. Acad. Sci. U.S.A. 110, 13600–13605. doi: 10.1073/pnas.1306390110
Holbrook, R. D., Murphy, K. E., Morrow, J. B., and Cole, K. D. (2008). Trophic transfer of nanoparticles in a simplified invertebrate food web. Nat. Nanotechnol. 3, 352–355. doi: 10.1038/nnano.2008.110
Holmström, C., Egan, S., Franks, A., McCloy, S., and Kjelleberg, S. (2002). Antifouling activities expressed by marine surface associated Pseudoalteromonas species. FEMS Microbiol. Ecol. 41, 47–58. doi: 10.1111/j.1574-6941.2002.tb00965.x
Holmström, C., Rittschof, D., and Kjelleberg, S. (1992). Inhibition of settlement by larvae of Balanus amphitrite and Ciona intestinalis by a surface-colonizing marine bacterium. Appl. Environ. Microbiol. 58, 2111–2115.
Hoppe, H.-G., Arnosti, C., and Herndl, G. F. (2001). “Ecological significance of bacterial enzymes in the marine environment,” in Enzymes in the Environment, eds R. G. Burns and R. P. Dick (Basel: Marcel Dekker), 73–107.
Hoppe, H.-G., Gocke, K., Koppe, R., and Begler, C. (2002). Bacterial growth and primary production along a north-south transect of the Atlantic Ocean. Nature 416, 168–171. doi: 10.1038/416168a
Horswill, A. R., Stoodley, P., Stewart, P. S., and Parsek, M. R. (2007). The effect of the chemical, biological, and physical environment on quorum sensing in structured microbial communities. Anal. Bioanal. Chem. 387, 371–380. doi: 10.1007/s00216-006-0720-y
Hoskins, D. L., Stancyk, S. E., and Decho, A. W. (2003). Utilization of algal and bacterial extracellular polymeric secretions (EPS) by the deposit-feeding brittlestar Amphipholis gracillima (Echinodermata). Mar. Ecol. Prog. Ser. 247, 93–101. doi: 10.3354/meps247093
Houry, A., Gohar, M., Deschamps, J., Tischendko, E., Aymerich, S., Gruss, A., et al. (2012). Bacterial swimmers that infiltrate and take over the biofilm matrix. Proc. Natl. Acad. Sci. U.S.A. 109, 13088–13093. doi: 10.1073/pnas.1200791109
Hubas, C., Sachidhanandam, C., Rybarcczyk, H., Lubarsky, H. V., Rigaux, A., Moens, T., et al. (2010). Bacterivorous nematodes stimulate microbial growth and exopolymer production in marine sediment microcosms. Mar. Ecol. Prog. Ser. 419, 85–94. doi: 10.3354/meps08851
Hultin, K. A. H., Nilsson, E. D., Krejci, R., Mårtensson, E. M., Ehn, M., and Hagström, Å. (2010). In situ laboratory sea spray production during the Marine Aerosol Production 2006 cruise on the northeastern Atlantic Ocean. J. Geophys. Res. 115, D06201. doi: 10.1029/2009jd012522
Ikuma, K., Decho, A. W., and Lau, B. L. T. (2015). When nanoparticles meet biofilms- interactions guiding the environmental fate and accumulation of nanoparticles. Front. Microbiol. 6:591. doi: 10.3389/fmicb.2015.00591
Ikuma, K., Madden, A. S., Decho, A. W., and Lau, B. L. T. (2014). Deposition of nanoparticles onto polysaccharide-coated surfaces: implications for nanoparticle–biofilm interactions. Environ. Sci. Nano 1, 117–122. doi: 10.1039/c3en00075c
Iyer, A., Mody, K., and Bhavanath, J. (2005). Biosorption of heavy metals by a marine bacterium. Mar. Poll. Bull. 50, 340–343. doi: 10.1016/j.marpolbul.2004.11.012
Jatt, A. N., Tang, K., Liu, J., Zhang, Z., and Zhang, X.-H. (2015). Quorum sensing in marine snow and its possible influence on production of extracellular hydrolytic enzymes in marine snow bacterium Pantoea ananatis B9. FEMS Microbiol. Ecol. 91, 1–13. doi: 10.1093/femsec/fiu030
Jennings, L. K., Storek, K. M., Ledvina, H. E., Coulon, C., Marmont, L. S., Sadovskaya, I., et al. (2015). Pel is a cationic exopolysaccharide that cross-links extracellular DNA in the Pseudomonas aeruginosa biofilm matrix. Proc. Natl. Acad. Sci. U.S.A. 112, 11353–11358. doi: 10.1073/pnas.1503058112
Jernelöv, A., and Lindén, O. (1981). Ixtoc I: a case study of the world’s largest oil spill. AMBIO 10, 299–306.
Jiao, N., Herndl, G. J., Hansell, D. A., Benner, R., Kattner, G., Wilhelm, S. W., et al. (2010). Microbial production of recalcitrant dissolved organic matter: long-term carbon storage in the global ocean. Nat. Rev. Microbiol. 8, 593–599. doi: 10.1038/nrmicro2386
Johansson, S., Larsson, U., and Boehm, P. (1980). The Tsesis oil spill impact on the pelagic ecosystem. Mar. Pollut. Bull. 11, 284–293. doi: 10.1016/0025-326X(80)90166-6
Johnson, W. M., Soule, M. C. K., and Kujawinski, E. B. (2016). Evidence for quorum sensing and differential metabolite production by a marine bacterium in response to DMSP. ISME J. 10, 2304–2316. doi: 10.1038/ismej.2016.6
Joyce, A., and Utting, S. (2015). The role of exopolymers in hatcheries: an overlooked factor in hatchery hygiene and feed quality. Aquaculture 446, 122–131. doi: 10.1016/j.aquaculture.2015.04.037
Kaufmann, G. F., Sartorio, R., Lee, S.-H., Rogers, C. J., Meijler, M. M., Moss, J. A., et al. (2005). Revisiting quorum sensing: discovery of additional chemical and biological functions for 3-oxo-N-acylhomoserine lactones. Proc. Natl. Acad. Sci. U.S.A. 102, 309–314. doi: 10.1073/pnas.0408639102
Kawaguchi, T., and Decho, A. W. (2002a). A laboratory investigation of cyanobacterial extracellular polymeric secretions (EPS) in influencing CaCO3 polymorphism. J. Cryst. Growth 240, 230–235. doi: 10.1016/S0022-0248(02)00918-1
Kawaguchi, T., and Decho, A. W. (2002b). Characterization of extracellular polymeric secretions (EPS) from modern soft marine stromatolites (Bahamas) and its inhibitory effect on CaCO3 precipitation Prep. Biochem. Biotechnol. 32, 51–63.
Keil, R. G., and Kirchman, D. L. (1999). Utilization of dissolved protein and amino acids in the northern Sargasso Sea. Aquat. Microb. Ecol. 18, 293–300. doi: 10.3354/ame018293
Kennedy, A. F. D., and Sutherland, I. W. (1987). Analysis of bacterial exopolysaccharides. Biotechnol. Appl. Biochem. 9, 12–19.
Kirchman, D. L., Meon, B., Ducklow, H. W., Carlson, C. A., Hansell, D. A., and Steward, G. F. (2001). Glucose fluxes and concentrations of dissolved combined neutral sugars (polysaccharides) in the Ross Sea and Polar Front Zone, Antarctica. Deep Sea Res. II 48, 4179–4197. doi: 10.1016/S0967-0645(01)00085-6
Kleindienst, S., Seidel, M., Ziervogel, K., Grim, S., Loftis, K., Harrison, S., et al. (2015). Chemical dispersants can suppress the activity of natural oil-degrading microorganisms. Proc. Natl. Acad. Sci. U.S.A. 112, 14900–14905. doi: 10.1073/pnas.1507380112
Krembs, C., Eicken, H., and Deming, J. W. (2011). Exopolymer alteration of physical properties of sea ice and implications for ice habitability and biogeochemistry in a warmer Arctic. Proc. Natl. Acad. Sci. U.S.A. 108, 3653–3658. doi: 10.1073/pnas.1100701108
Krembs, C., Eicken, H., Junge, K., and Deming, J. W. (2002). High concentrations of exopolymeric substances in Arctic winter sea ice: implication for the polar ocean carbon cycle and cryoprotection of diatoms. Deep Sea Res. II 49, 2163–2181. doi: 10.1016/S0967-0637(02)00122-X
Krumbein, W. E. (1983). Stromatolites – the challenge of a term in space and time. Precambr. Res. 20, 493–531. doi: 10.1016/0301-9268(83)90087-6
Kuznetsova, M., Lee, C., and Aller, J. (2005). Characterization of the proteinaceous matter in marine aerosols. Mar. Chem. 96, 359–377. doi: 10.1007/s00216-012-6363-2
Lam, C., Harder, T., and Qian, P. Y. (2003). Induction of larval settlement in the polychaete Hydroides elegans by surface-associated settlement cues of marine benthic diatoms. Mar. Ecol. Prog. Ser. 263, 83–92. doi: 10.3354/meps263083
Larsen, P., Nielsen, J. L., Dueholm, M., Wetzel, R., Otzen, D., and Nielsen, P. H. (2007). Amyloid adhesins are abundant in natural biofilms. Environ. Microbiol. 9, 3077–3090. doi: 10.1111/j.1462-2920.2007.01418.x
Lau, S. C. K., and Qian, P. Y. (2000). Inhibitory effect of phenolic compounds and marine bacteria on larval settlement of the barnacle Balanus amphitrite Darwin. Biofouling 16, 47–58. doi: 10.1080/08927010009378429
Lawrence, J. R., Swerhone, G. D. W., Kuhlicke, U., and Neu, T. R. (2007). In situ-evidence for microdomains in the polymer matrix of bacterial microcolonies. Can. J. Microbiol. 53, 450–458. doi: 10.1139/W06-146
Lawrence, J. R., Swerhone, G. D. W., Kuhlicke, U., and Neu, T. R. (2016). In situ evidence for metabolic and chemical microdomains in the structured polymer matrix of bacterial microcolonies. FEMS Microbiol. Ecol. 92:fiw183. doi: 10.1093/femsec/fiw183
Lawrence, J. R., Swerhone, G. D. W., Leppard, G. G., Araki, T., Zhang, X., West, M. M., et al. (2003). Scanning transmission X-ray, laser scanning and transmission electron microscopy mapping of the exopolymeric matrix of microbial biofilms. Appl. Environ. Microbiol. 69, 5543–5554. doi: 10.1128/AEM.69.9.5543-5554.2003
Lawrence, J. R., Wolfaardt, G. M., and Korber, D. R. (1994). Determination of diffusion coefficients in biofilms by confocal laser microscopy. Appl. Environ. Microbiol. 60, 1166–1173.
Leck, C., and Bigg, E. K. (2005). Evolution of the marine aerosol – a new perspective. Geophys. Res. Lett. 32, L19803. doi: 10.1029/2005GL023651
Leck, C., and Bigg, E. K. (2008). Comparison of sources and nature of the tropical aerosol with the summer high Arctic aerosol. Tellus 60B, 118–126. doi: 10.1111/j.1600-0889.2007.00315.x
Lewin, R. (1984). Microbial adhesion is a sticky problem. Science 224, 375–377. doi: 10.1126/science.6143401
Ley, R. E., Harris, J. K., Wilcox, J., Spear, J. R., Miller, S. R., Bebout, B. M., et al. (2006). Unexpected diversity and complexity from the Guerrero Negro hypersaline microbial mat. Appl. Environ. Microbiol. 72, 3685–3695. doi: 10.1128/AEM.72.5.3685-3695.2006
Liu, S.-B., Chen, X.-L., He, H.-L., Zhang, X.-Y., Xie, B.-B., Yu, Y., et al. (2013). Structure and ecological roles of a novel exopolysaccharide from the Arctic sea ice bacterium Pseudoalteromonas sp. Strain SM20310. Appl. Environ. Microbiol. 79, 224–230. doi: 10.1128/AEM.01801-12
Loaec, M., Olier, R., and Guezennec, J. (1997). Uptake of lead, cadmium and zinc by a novel bacterial exopolysaccharide. Water Res. 31, 1171–1179. doi: 10.1016/S0043-1354(96)00375-2
Loaec, M., Olier, R., and Guezennec, J. (1998). Chelating properties of bacterial exopolysaccharides from deep-sea hydrothermal vents. Carbohydr. Polymers 35, 65–70. doi: 10.1016/S0144-8617(97)00109-4
Long, R. A., and Azam, F. (1996). Abundant protein-containing particles in the sea. Aquat. Microb. Ecol. 10, 213–221. doi: 10.3354/ame010213
Long, R. A., and Azam, F. (2001a). Antagonistic interactions among marine pelagic bacteria. Appl. Environ. Microbiol. 67, 4975–4983.
Long, R. A., and Azam, F. (2001b). Microscale patchiness of bacterioplankton assemblage richness in seawater. Aquat. Microb. Ecol. 26, 103–113. doi: 10.3354/ame026103
Ludwig, R., Al-Horani, F. A., de Beer, D., and Jonkers, H. M. (2005). Photosynthesis controlled calcification in a hypersaline microbial mat. Limnol. Oceanogr. 50, 1836–1843. doi: 10.4319/lo.2005.50.6.1836
Lundqvist, A., Bertilsson, S., and Goedkoop, W. (2010). Effects of extracellular polymeric and humic substances on chlorpyrifos bioavailability to Chironomus riparius. Ecotoxicology 19, 614–622. doi: 10.1007/s10646-009-0430-2
Lundqvist, A., Bertilsson, S., and Goedkoop, W. (2012). Interactions with DOM and biofilms affect the fate and bioavailability of insecticides to invertebrate grazers. Ecotoxicology 21, 2398–2408. doi: 10.1007/s10646-012-0995-z
Luoma, S. N., Khan, F. R., and Croteau, M.-N. (2014). Bioavailability and bioaccumulation of metal-based engineered nanomaterials in aquatic environments: concepts and processes. Nanosci. Environ. 7, 157–193. doi: 10.1016/b978-0-08-099408-6.00005-0
Malarkey, J., Baas, J. H., Hope, J. A., Aspden, R. J., Parsons, D. R., Peakall, J., et al. (2015). The pervasive role of biological cohesion in bedform development. Nat. Commun. 6:6257. doi: 10.1038/ncomms7257
Martin, J. H., Coale, K. H., Johnson, K. S., Fitzwater, S. E., Gordon, R. M., Tanner, S. J., et al. (1994). Testing the iron hypothesis in ecosystems of the equatorial Pacific-Ocean. Nature 371, 123–129. doi: 10.1038/371123a0
Marx, J. G., Carpenter, S. D., and Deming, J. W. (2009). Production of cryoprotectant extracellular polymeric substances (EPS) by the marine psychrophilic bacterium Colwellia psychrerythraea strain 34H under extreme conditions. Can. J. Microbiol. 55, 63–72. doi: 10.1139/W08-130
Mashburn, L. M., and Whiteley, M. (2005). Membrane vesicles traffic signals and facilitate group activities in a prokaryote. Nature 437, 422–425. doi: 10.1038/nature03925
Mashburn-Warren, L., McLean, R. J. C., and Whiteley, M. (2008). Gram-negative outer membrane vesicles: beyond the cell surface. Geobiology 6, 214–219. doi: 10.1111/j.1472-4669.2008.00157.x
Mayer, C., Moritz, R., Kirschner, C., Borchard, W., Maibaum, R., Wingender, J., et al. (1999). The role of intermolecular interaction: studies on model systems for bacterial biofilms. Int. J. Biol. Macromol. 26, 3–16. doi: 10.1016/S0141-8130(99)00057-4
McLean, R. J. C., Whiteley, M., Stickler, D. J., and Fuqua, W. C. (1997). Evidence of autoinducer activity in naturally occurring biofilms. FEMS Microbiol. Lett. 154, 259–263. doi: 10.1111/j.1574-6968.1997.tb12653.x
Meiners, K., Gradinger, R., Fehling, J., Civitarese, G., and Spindler, M. (2003). Vertical distribution of exopolymer particles in sea ice of the Fram Strait (Arctic) during autumn. Mar. Ecol. Prog. Ser. 248, 1–13. doi: 10.3354/meps248001
Meister, P. (2013). Two opposing effects of sulfate reduction on carbonate precipitation in normal marine, hypersaline, and alkaline environments. Geology 41, 499–502. doi: 10.1130/G34185.1
Miller, L. A., Papkyriakow, T. N., Collins, R. E., Deming, J. W., Ehn, J. K., MacDonald, R. W., et al. (2011). Carbon dynamics in sea ice: a winter flux time series. J. Geophys. Res. 116:C02028. doi: 10.1029/2009jc006058
Miller, S. D., Haddock, S. H. D., Elvidge, C. D., and Lee, T. F. (2005). Detection of a bioluminescent milky sea from space. Proc. Natl. Acad. Sci. U.S.A. 102, 14181–14184. doi: 10.1073/pnas.0507253102
Mobberley, J. M., Khodadad, C. L. M., Visscher, P. T., Reid, R. P., Hagan, P., and Foster, J. S. (2015). Inner workings of thrombolites: spatial gradients of metabolic activity as revealed by metatranscriptome profiling. Sci. Rep. 5:12601. doi: 10.1038/srep12601
Mopper, K., Schultz, C. A., Chevolot, L., Germain, C., Revuelta, R., and Dawson, R. (1992). Determination of sugars in unconcentrated seawater and other natural waters by liquid chromatography and pulsed amperometric detection. Environ. Sci. Technol. 26, 133–138. doi: 10.1021/es00025a014
Moppert, X., Le Costaouec, T., Raguénès, G., Simon-Colin, C., Crassous, P., Costa, B., et al. (2009). Investigations into the uptake of copper, iron and selenium by a highly-sulphated bacterial exopolysaccharide isolated from microbial mats. J. Indust. Microbiol. Biotechnol. 36, 599–604. doi: 10.1007/s10295-009-0529-8
Moran, M. A. (2015). Microbiome: the global ocean microbiome. Science 350:aac8455. doi: 10.1126/science.aac8455
Moran, M. A., and Zepp, R. G. (2000). “UV radiation effects on microbes and microbial processes,” in Microbial Ecology of the Oceans, 1st Edn, ed. D. L. Kirchman (Hoboken, NJ: Wile-Liss), 201–228.
Mortimer, M., Petersen, E. J., Buchholz, B. A., Orias, E., and Holden, P. A. (2016). Bioaccumulation of multiwall Carbon nanotubes in Tetrahymena thermophila by direct feeding or trophic transfer. Environ. Sci. Technol. 50, 8876–8885. doi: 10.1021/acs.est.6b01916
Myklestad, S. (1977). Production of carbohydrates by marine planktonic diatoms. II. Influence of the N/P ratio in the growth medium on the assimilation ratio, growth rate and production of cellular and extracellular carbohydrates by Chaetoceros affinis var Willei (Gran) Hustedt and Skeletonema costatum (Grev) Cleve. J. Exp. Mar. Biol. Ecol. 29, 161–179. doi: 10.1016/0022-0981(77)90046-6
Nagata, T. (2000). “Production mechanisms of dissolved organic matter,” in Microbial Ecology of the Oceans, 1st Edn, ed. D. L. Kirchman (Hoboken, NJ: Wiley-Liss), 121–152.
Nealson, K. H., and Hastings, J. W. (2004). Quorum sensing on a global scale: massive numbers of bioluminsescent bacteria make milky seas. Appl. Environ. Microbiol. 72, 2295–2297. doi: 10.1128/AEM.72.4.2295-2297.2006
Neu, T. R. (1994). “Biofilms and microbial mats,” in Biostabilization of Sediments, eds W. E. Krumbein, D. M. Paterson, and L. J. Stal (Oldenburg: BIS-Verlag), 9–17.
Neu, T. R., and Lawrence, J. R. (2014). Advanced techniques for in situ analysis of the biofilm matrix (structure, composition, dynamics) by means of laser scanning microscopy. Methods Mol. Biol. 1147, 43–64. doi: 10.1007/978-1-4939-0467-9_4
Neu, T. R., and Lawrence, J. R. (2016). “The extracellular matrix – an intractable part of biofilm systems,” in The Perfect Slime -Microbial Extracellular Polymeric Substances (EPS), eds H.-C. Flemming, T. R. Neu, and J. Wingender (London: IWA Publishers), 25–60.
Nevius, B. A., Chen, Y. P., Chen Ferry, J. L., and Decho, A. W. (2012). Surface-functionalization effects on uptake of fluorescent polystyrene nanoparticles by model biofilms. Ecotoxicology 21, 2205–2213. doi: 10.1007/s10646-012-0975-3
Nichols, C. A., Guezennec, J., and Bowman, J. P. (2005). Bacterial exopolysaccharides from extreme marine environments with special consideration of the Southern Ocean, sea ice, and deep-sea hydrothermal vents: a review. Mar. Biotechnol. 7, 253–271. doi: 10.1007/s10126-004-5118-2
Nichols, C. M., Lardière, S. G., Bowman, J. P., Nichols, P. D., Gibson, J. A. E., and Guézennec, J. (2005). Chemical characterization of exopolysaccharide for Antarctic marine bacteria. Microb. Ecol. 49, 578–589. doi: 10.1007/s00248-004-0093-8
Nielsen, S. J., Harder, T., and Steinberg, P. D. (2015). Sea urchin larvae decipher the epiphytic bacterial community composition when selecting sites for attachment and metamorphosis. FEMS Microbiol. Ecol. 91, 1–9. doi: 10.1093/femsec/fiu011
Nishimura, S., Tanaka, T., Fujita, K., Itaya, M., Hiraishi, A., and Kikuchi, Y. (2003). Extracellular DNA and RNA produced by a marine photosynthetic bacterium Rhodovulum sulfidophilum. Nucleic Acids Res. 3(Suppl.), 279–280. doi: 10.1093/nass/3.1.279
Niu, H., Li, Z., Lee, K., Kepkay, P., and Mullin, J. V. (2011). Modelling the transport of oil-mineral-aggregates (OMAs) in the marine environment and assessment of their potential risks. Environ. Model Assess. 16, 61–75. doi: 10.1007/s10666-010-9228-0
Noffke, N. (2010). Microbial Mats in Sandy Deposits from the Archean to Today. Heidelberg: Springer, 196.
Noffke, N., Christian, D., Wacey, D., and Hazen, R. M. (2013). Microbially induced sedimentary structures recording an ancient ecosystem in the ca. 3.48 billion-year-old dresser formation, Pilbara, Western Australia. Astrobiology 13, 1103–1124. doi: 10.1089/ast.2013.1030
Noffke, N., Gerdes, G., Klenke, T., and Krumbein, W. E. (2001). Microbially induced sedimentary structures- A new category within the classification of primary sedimentary structures. J. Sed. Res. 71, 649–656. doi: 10.1306/2DC4095D-0E47-11D7-8643000102C1865D
Nutman, A. P., Bennett, V. C., Friend, C. R. L., van Kranendonk, M. J., and Chivas, A. R. (2016). Rapid emergence of life shown by discovery of 3,700-million-year-old microbial structures. Nature 537, 535–538. doi: 10.1038/nature19355
Nyholm, S. V., Stabb, E. V., Ruby, E. G., and McFall-Ngai, M. J. (2000). Establishment of an animal bacterial association: recruiting symbiotic vibrios from the environment. Proc. Natl. Acad. Sci. U.S.A. 97, 10231–10235. doi: 10.1073/pnas.97.18.10231
Obst, M., Dynes, J. J., Lawrence, J. R., Swerhone, G. D. W., Benzerara, K., Karunakaran, C., et al. (2009). Precipitation of amorphous CaCO3 (aragonite-like) by cyanobacteria: a STXM study of the influence of EPS on the nucleation process. Geochim. Cosmchim. Acta 73, 4180–4198. doi: 10.1016/j.gca.2009.04.013
O’Dowd, C. D., Facchini, M. C., Cavalli, F., Ceburnis, D., Mircea, M., Decesari, S., et al. (2004). Biogenically-driven organic contribution to marine aerosol. Nature 431, 676–680. doi: 10.1038/nature02959
Ogawa, H., Amagai, Y., Koike, I., Kaiser, K., and Benner, R. (2001). Production of refractory dissolved organic matter by bacteria. Science 292, 917–920. doi: 10.1126/science.1057627
Okshevsky, M., and Meyer, R. L. (2013). The role of extracellular DNA in the establishment, maintenance and perpetuation of bacterial biofilms. Crit. Revs. Microbiol. 41, 341–352. doi: 10.3109/1040841X.2013.841639
Oren, A. (1994). The ecology of extremely halophilic archaea. FEMS Microbiol. Rev. 13, 415–439. doi: 10.1111/j.1574-6976.1994.tb00060.x
Paerl, H. W., Steppe, T. F., and Reid, R. P. (2001). Bacterially-mediated precipitation in marine Stromatolites. Environ. Microbiol. 3, 123–130. doi: 10.1046/j.1462-2920.2001.00168.x
Papenfort, K., and Bassler, B. L. (2016). Quorum sensing signal-response systems in Gram-negative bacteria. Nat. Revs. Microbiol. 14, 576–588. doi: 10.1038/nrmicro.2016.89
Passow, U. (2002). Transparent exopolymer particles (TEP) in aquatic environments. Progr. Oceanogr. 55, 287–333. doi: 10.1016/S0079-6611(02)00138-6
Passow, U. (2016). Formation of rapidly-sinking, oil-associated marine snow. Deep Sea Res. II Top. Stud. Oceanogr. 129, 232–240. doi: 10.1016/j.dsr2.2014.10.001
Passow, U., Ziervogel, K., Asper, V., and Diercks, A. (2012). Marine snow formation in the aftermath of the deepwater horizon oil spill in the Gulf of Mexico. Environ. Res. Lett. 7, 1–11. doi: 10.1088/1748-9326/7/3/035301
Paterson, D. M. (1989). Short-term changes in the erodibility of intertidal cohesive sediments related to the migratory behavior of epipelic diatoms. Limnol. Oceanogr. 34, 223–234. doi: 10.4319/lo.1989.34.1.0223
Paterson, D. M., Aspden, R. J., Visscher, P. T., Consalvey, M., Andres, M. S., Decho, A. W., et al. (2008). Light dependent biostabilisation of sediments by stromatolite assemblages. PLoS ONE 3:e3176. doi: 10.1371/journal.pone.0003176
Patton, J. S., Rigler, M. W., Boehm, P. D., and Fiest, D. L. (1981). Ixtoc I oil spill: flaking of surface mousse in the Gulf of Mexico. Nature 290, 235–238. doi: 10.1038/290235a0
Paul, V., Mormile, M. R., and Wronkiewicz, D. J. (2017). Impact of elevated CO2 concentrations on carbonate mineral precipitation ability of sulfate-reducing bacteria and implications for CO2 sequestration. Appl. Geochem. 78, 250–271. doi: 10.1016/j.apgeochem.2017.01.010
Peterson, B. W., He, Y., Ren, Y., Zerdoum, A., Libera, M. R., Sharma, P. K., et al. (2015). Viscoelasticity of biofilms and their recalcitrance to mechanical and chemical challenges. FEMS Microbiol. Rev. 39, 234–245. doi: 10.1093/femsre/fuu008
Pinckney, J. L., and Paerl, H. W. (1997). Anoxygenic photosynthesis and nitrogen fixation by a microbial mat community in a Bahamian hypersaline lagoon. Appl. Environ. Microbiol. 63, 420–426.
Plante, C. (2000). Role of bacterial exopolymeric capsules in protection from deposit feeder ingestion. Aquat. Microb. Ecol. 21, 211–219. doi: 10.3354/ame021211
Plante, C. J., Jumars, P. A., and Baross, J. A. (1990). Digestive associations between marine detritivores and bacteria. Ann. Rev. Ecol. Syst. 21, 29–44. doi: 10.1146/annurev.es.21.110190.000521
Plante, C. J., and Schriver, A. G. (1998). Differential lysis of sedimentary bacteria by Arenicola marina L.: examination of cell wall structure and exopolymeric capsules as correlates. J. Exp. Mar. Biol. Ecol. 229, 35–52. doi: 10.1016/S0022-0981(98)00039-2
Ploug, H. (2008). Cyanobacterial surface blooms formed by Aphanizomenon sp. and Nodularia spumigena in the Baltic Sea: small-scale fluxes, pH, and oxygen micro-environments. Limnol. Oceanogr. 53, 914–921. doi: 10.4319/lo.2008.53.3.0914
Ploug, H., Grossart, H. P., Azam, F., and Jørgensen, B. B. (1999). Photosynthesis, respiration, and carbon turnover in sinking marine snow from surface waters of the Southern California Bight: implications for the carbon cycle in the ocean. Mar. Ecol. Prog. Ser. 179, 1–11. doi: 10.3354/meps179001
Ploug, H., Kühl, M., Buchholz-Cleven, B., and Jørgensen, B. B. (1997). Anoxic aggregates - an ephemeral phenomenon in the pelagic environment? Aquat. Microb. Ecol. 13, 285–294. doi: 10.3354/ame013285
Raguénès, G., Christen, R., Guézennec, J., Pignet, P., and Barbier, G. (1997a). Vibrio diabolicus sp.nov., a new polysaccharide-secreting organism isolated from a deep-sea vent polychaete annelid, Alvinella pompejana. Int. J. Syst. Bacteriol. 47, 989–995.
Raguénès, G., Ruimy, R., Pignet, P., Christen, R., Loaec, M., Rougeaux, H., et al. (1997b). Alteromonas infernus sp. nov., a new polysaccharide producing bacterium isolated from a deep-sea hydrothermal vent. J. Appl. Bacteriol. 82, 422–430.
Rao, D., Webb, J. S., Holmstrom, C., Case, R., Low, A., Steinberg, P., et al. (2007). Low densities of epiphytic bacteria from the marine alga Ulva australis inhibit settlement of fouling organisms. Appl. Environ. Microbiol. 73, 7844–7852. doi: 10.1128/AEM.01543-07
Redfield, R. J. (2002). Is quorum sensing a side effect of diffusion sensing? Trends Microbiol. 10, 365–370.
Reid, R. P., Visscher, P. T., Decho, A. W., Stolz, J. F., Bebout, B. M., Dupraz, C., et al. (2000). The role of microbes in accretion, lamination and early lithication of modern marine stromatolites. Nature 406, 989–992. doi: 10.1038/35023158
Repeta, D. J., and Aluwihare, L. I. (2006). Radiocarbon analysis of neutral sugars in high-molecular-weight dissolved organic carbon: implications for organic carbon cycling. Limnol. Oceanogr. 51, 1045–1053. doi: 10.4319/lo.2006.51.2.1045
Rice, K. C., Mann, E. E., Endres, J. L., Weiss, E. C., Cassat, J. E., Smeltzer, M. S., et al. (2007). The cidA murein hydrolase regulator contributes to DNA release and biofilm development in Staphylococcus aureus. Proc. Natl. Acad. Sci., U.S.A. 104, 8113–8118. doi: 10.1073/pnas.0610226104
Rich, J., Gosselin, M., Sherr, E., Sherr, B., and Kirchman, D. L. (1997). High bacterial production, uptake and concentrations of dissolved organic matter in the Central Arctic Ocean. Deep-Sea Res. II 44, 1645–1663. doi: 10.1016/S0967-0645(97)00058-1
Rich, J. H., Ducklow, H. W., and Kirchman, D. L. (1996). Concentrations and uptake of neutral monosaccharides along 140°W in the Equatorial Pacific: contribution of glucose to heterotrophic bacterial activity and the POM flux. Limnol. Oceanogr. 41, 595–604. doi: 10.4319/lo.1996.41.4.0595
Richert, L., Golubic, S., Le Guédès, R., Ratiskol, J., Payri, C., and Guezennec, J. (2005). Characterization of exopolysaccharides produced by cyanobacteria isolated from polynesian microbial mats. Curr. Microbiol. 51, 379–384. doi: 10.1007/s00284-005-0069-z
Rieger, J., Frechen, T., Cox, G., Heckmann, W., Schmidt, C., and Thieme, J. (2007). Precursor structures in the crystallization/precipitation processes of CaCO3 and control of particle formation by polyelectrolytes. Faraday Discuss. 136, 265–277. doi: 10.1039/b701450c
Rivkin, R. B., and Anderson, M. R. (1997). Inorganic nutrient limitation of oceanic bacterioplankton. Limnol. Oceanogr. 42, 730–740. doi: 10.1038/nature07236
Romankevich, E. A. (1984). Geochemistry of Organic Matter in the Ocean. Berlin: Springer-Verlag. doi: 10.1007/978-3-642-49964-7
Romero, D., Aguilar, C., Losick, R., and Kolter, R. (2010). Amyloid fibers provide structural integrity to Bacillus subtilus biofilms. Proc. Natl. Acad. Sci. U.S.A. 107, 2230–2234. doi: 10.1073/pnas.0910560107
Rougeaux, H., Guezennec, M., Mao Che, L., Payri, C., Deslandes, E., and Guezennec, J. (2001). Microbial communities and exopolysaccharides from Polynesian mats. Mar. Biotechnol. 3, 181–187. doi: 10.1007/s101260000063
Rougeaux, H., Kervarec, N., Pichon, R., and Guezennec, J. (1999). Structure of the exopolysaccharide of Vibrio diabolicus isolated from a deep-sea hydrothermal vent. Carbohydr. Res. 322, 40–45. doi: 10.1016/S0008-6215(99)00214-1
Ruby, E. G., and Nealson, K. H. (1977). Luminous bacterium that emits yellow light. Science 196, 432–434. doi: 10.1126/science.850787
Ruparell, A., Dubern, J. F., Ortori, C. A., Harrison, F., Halliday, N. M., and Emtage, A. (2016). The fitness burden imposed by synthesizing quorum sensing signals. Sci. Rep. 6:33101. doi: 10.1038/srep33101
Russell, L. M., Hawkins, L. N., Frossard, A. A., Quinn, P. K., and Bates, T. S. (2010). Carbohydrate-like composition of submicron atmospheric particles and their production from ocean bubble bursting. Proc. Natl. Acad. Sci. U.S.A. 107, 6652–6657. doi: 10.1073/pnas.0908905107
Salek, K., and Gutierrez, T. (2016). Surface-active biopolymers from marine bacteria for potential biotechnological applications. AIMS Microbiol. 2, 92–107. doi: 10.3934/microbiol.2016.2.92
Santschi, P. H., Guo, L., Means, J. C., and Ravichandran, M. (1998). “Natural organic matter binding of trace metal and trace organic contaminants in estuaries,” in Biogeochemistry of Gulf of Mexico Estuaries, eds T. S. Bianchi, J. R. Pennock, and R. Twilley (New York, NY: Wiley), 347–380.
Schaefer, A. L., Greenberg, E. P., Oliver, C. M., Oda, Y., Huang, J. J., Bittan-Banin, G., et al. (2008). A new class of homoserine lactone quorum sensing signals. Nature 454, 595–596. doi: 10.1038/nature07088
Schertzer, J. W., Boulette, M. L., and Whiteley, M. (2009). More than a signal: non-signaling properties of quorum sensingmolecules. Trends Microbiol. 17, 189–195. doi: 10.1016/j.tim.2009.02.001
Schlekat, C. E., Decho, A. W., and Chandler, G. T. (1998). Sorption of cadmium to bacterial extracellular polymeric sediment coatings under estuarine conditions. Environ. Toxicol. Chem. 17, 1867–1874. doi: 10.1002/etc.5620170930
Schlekat, C. E., Decho, A. W., and Chandler, G. T. (1999). Dietary assimilation of cadmium associated with bacterial exopolymer sediment coatings by the estuarine amphipod Leptocheirus pluinulosus: effects of Cd concentration and salinity. Mar. Ecol. Prog. Ser. 183, 205–216. doi: 10.3354/meps183205
Schlekat, C. E., Decho, A. W., and Chandler, G. T. (2000). Bioavailability of particle-associated Ag, Cd, and Zn to the estuarine amphipod, Leptocheirus plumulosus, through dietary ingestion. Limnol. Oceanogr. 45, 11–21. doi: 10.1371/journal.pone.0064060
Schooling, S. R., Hubley, A., and Beveridge, T. J. (2009). Interaction of DNA with biofilm-derived membrane vesicles. J. Bacteriol. 191, 4097–4102. doi: 10.1128/JB.00717-08
Selck, H., Decho, A. W., and Forbes, V. E. (1999). Effects of chronic metal exposure and sediment organic matter on digestive absorption efficiencies of cadmium by the deposit-feeding polychaete Capitella species I. Environ. Toxicol. Chem. 18, 1289–1297. doi: 10.1002/etc.5620180631
Seper, A., Fengler, V. H. I., Roier, S., Wolinski, H., Kohlwein, S. D., Bishop, A. L., et al. (2011). Extracellular nucleases and extracellular DNA play important roles in Vibrio cholera biofilm formation. Mol. Microbiol. 82, 1015–1037. doi: 10.1111/j.1365-2958.2011.07867.x
Serra, D. O., Richter, A. M., and Hengge, R. (2013). Cellulose as an architectural element in spatially structured Escherichia coli biofilms. J. Bact. 195, 5540–5554. doi: 10.1128/JB.00946-13
Shanks, A. L., and Reeder, M. L. (1993). Reducing microzones and sulfide production in marine snow. Mar. Ecol. Prog. Ser. 96, 43–47. doi: 10.3354/meps096043
Shanks, A. L., and Trent, J. D. (1980). Marine snow: sinking rates and potential role in vertical flux. Deep Sea Res. Part A. Oceanogr. Res. Papers 27, 137–143. doi: 10.1016/0198-0149(80)90092-8
Sharif, D. I., Gallon, J., Smith, C. J., and Dudley, E. (2008). Quorum sensing in cyanobacteria: N-octanoyl-homoserine lactone release and response, by the epilithic colonial cyanobacterium Gloeotheca PCC6909. ISME J. 2, 1171–1182. doi: 10.1038/ismej.2008.68
Shaw, E., Hill, D. R., Brittain, N., Wright, D. J., Täuber, U., Marand, H., et al. (2003). Unusual water flux in the extracellular polysaccharide of the cyanobacterium Nostoc commune. Appl Environ. Microbiol. 69, 5679–5684. doi: 10.1128/AEM.69.9.5679
Simon, M., Grossart, H.-P., Schweitzer, B., and Ploug, H. (2002). Microbial ecology of organic aggregates in aquatic ecosystems. Aquat. Microb. Ecol. 28, 175–211. doi: 10.3354/ame028175
Skoog, A., and Benner, R. (1997). Aldoses in various size fractions of marine organic matter: implications for carbon cycling. Limnol. Oceanogr. 42, 1803–1813. doi: 10.4319/lo.1997.42.8.1803
Skoog, A., Biddanda, B., and Benner, R. (1999). Bacterial utilization of dissolved glucose in the upper water column of the Gulf of Mexico. Limnol. Oceanogr. 44, 1625–1633. doi: 10.3389/fmicb.2013.00318
Skoog, A., Whitehead, K., Sperling, F., and Junge, K. (2002). Microbial glucose uptake and growth along a horizontal nutrient gradient in the North Pacific. Limnol. Oceanogr. 47, 1676–1683. doi: 10.4319/lo.2002.47.6.1676
Smith, D. C., Simon, M., Alldredge, A. L., and Azam, F. (1992). Intense hydrolytic enzyme activity on marine aggregates and implications for rapid particle dissolution. Nature 359, 139–142. doi: 10.1038/359139a0
Smith, D. J., and Underwood, G. J. C. (1998). Exopolymer production by intertidal epipelic diatoms. Limnol. Oceanogr. 43, 1578–1591. doi: 10.4319/lo.1998.43.7.1578
Smith, D. J., and Underwood, G. J. C. (2000). The production of extracellular carbohydrates by estuarine benthic diatoms: the effects of growth phase and light and dark treatment. J. Phycol. 36, 321–333. doi: 10.1046/j.1529-8817.2000.99148.x
Sprachta, S., Camoin, G., Golubic, S., and Le Campion, T. (2001). Microbialites in a modern lagoonal environment: nature and distribution, Tikehau atoll (French Polynesia). Palaeogeogr. Palaeoclimatol. Palaeoecol. 175, 103–124. doi: 10.1016/S0031-0182(01)00388-1
Steinberger, R. E., and Holden, P. A. (2005). Extracellular DNA in single and multiple-species unsaturated biofilms. Appl. Environ. Microbiol. 71, 5404–5410. doi: 10.1128/AEM.71.9.5404-5410.2005
Stewart, P. S. (2002). Diffusion in biofilms. J. Bacteriol. 185, 1485–1491. doi: 10.1128/JB.185.5.1485-1491.2003
Stewart, P. S., and Franklin, M. J. (2008). Physiological heterogeneity in biofilms. Nat. Revs. Microbiol. 6, 199–210. doi: 10.1038/nrmicro1838
Stoop, J. M. H., Williamson, J. D., and Pharr, D. M. (1996). Mannitol metabolism in plants: a method for coping with stress. Trends Plant Sci. 1, 139–144. doi: 10.1016/S1360-1385(96)80048-3
Suja, L. D., Summers, S., and Gutierrez, T. (2017). Role of EPS, dispersant and nutrients on the microbial response and MOS formation in the Subarctic Northeast Atlantic. Front. Microbiol. 8:676. doi: 10.3389/fmicb.2017.00676
Sutherland, I. W. (1999). “Polysaccharases in biofilms – sources – action – consequences,” in Microbial Extracellular Polymeric Substances, eds J. Wingender, T. R. Neu, and H.-C. Flemming (Berlin: Springer Press), 201–216. doi: 10.1007/978-3-642-60147-7_11
Sutherland, I. W. (2001). The biofilm matrix – an immobilized but dynamic microbial environment. Trends Microbiol. 9, 222–227. doi: 10.1016/S0966-842X(01)02012-1
Sutherland, I. W. (2016). “EPS – a complex mixture,” in The Perfect Slime – Microbial Extracellular Polymeric Substances, eds H.-C. Flemming,J. Wingender, and T. R. Neu (London: IWA Publishers), 15–24.
Suzuki, H., Daimon, M., Awano, T., Umekage, S., Tanaka, T., and Kikuchi, Y. (2009). Characterization of extracellular DNA production and flocculation of the marine photosynthetic bacterium Rhodovulum sulfidophilum. Appl. Microbiol. Biotechnol. 84, 349–356. doi: 10.1007/s00253-009-2031-7
Tang, L., Schramm, A., Neu, T. R., Revsbech, N. P., and Meyer, R. L. (2013). Extracellular DNA in adhesion and biofilm formation of four environmental isolates: a quantitative study. FEMS Microbiol. Ecol. 86, 394–403. doi: 10.1111/1574-6941.12168
Taylor, M. W., Schupp, P. J., Baillie, H. J., Charlton, T. S., de Nys, R., Kjelleberg, S., et al. (2004). Evidence for acyl homoserine lactone signal production in bacteria associated with marine sponges. Appl. Environ. Microbiol. 70, 4387–4389. doi: 10.1128/AEM.70.7.4387-4389.2004
Teal, J. M., and Howarth, R. W. (1984). Oil spill studies: a review of ecological effects. Environ. Manag. 8, 27–44. doi: 10.1007/BF01867871
Teske, A., Ramsing, N. B., Habicht, K., Fukul, M., Küver, J., Jørgensen, B. B., et al. (1998). Sulfate-reducing bacteria and their activities in cyanobacterial mats of Solar lake (Sinai. Egypt). Appl. Environ. Microbiol. 64, 2943–2951.
Thavasi, R., and Banat, I. M. (2014). “Biosurfactant and bioemulsifiers from marine sources-,” in Biosurfactants: Research Trends and Applications, Chap. 5, eds C. N. Mulligan, S. K. Sharma, and M. A. Hardback (Boca Raton, FL: CRC Press), 125–146. doi: 10.1201/b16383-6
Thavasi, R., Jayalakshmi, S., and Banat, I. M. (2011). “Biosurfactant from marine bacterial isolates,” in current Research Technology and Education Topics in Applied Microbiology and Microbial Biotechnology Book Series –, Vol. 2, ed. A. Mendez-Vilas (Badajoz: Formatex Research Center), 1367–1373.
Thingstad, T. F., Hagström,Å, and Rassoulzadegan, F. (1997). Accumulation of degradable DOC in surface waters: is it caused by a malfunctioning microbial loop? Limnol. Oceanogr. 42, 398–404. doi: 10.4319/lo.1997.42.2.0398
Thingstad, T. F., Li Zweifel, U., and Rassoulzadegan, F. (1998). P limitation of heterotrophic bacteria and phytoplankton in the northwest Mediterranean. Limnol. Oceanogr. 43, 88–94. doi: 10.4319/lo.1998.43.1.0088
Thornton, D. C. O., Fejes, E. M., Dimarco, S. F., and Clancy, K. M. (2007). Measurement of acid polysaccharides in marine and freshwater samples using alcian blue. Limnol. Oceanogr. Method. 5, 73–87. doi: 10.4319/lom.2007.5.73
Thornton, D. C. O., Kopac, S. M., and Long, R. A. (2010). Production and enzymatic hydrolysis of carbohydrates in intertidal sediments. Aquat. Microb. Ecol. 60, 109–125. doi: 10.3354/ame01403
Tice, M. M., and Lowe, D. R. (2004). Photosynthetic microbial mats in the 3,416-Myr-old ocean. Nature 431, 522–523. doi: 10.1038/nature02888
Tielen, P., Rosenau, F., Wilhelm, S., Jaeger, K.-C., Flemming, H.-C., and Wingender, J. (2013). Extracellular enzymes affect biofilm formation in mucoid Pseudomonas aeruginosa. BMC Microbiol. 159:221–228.
Tiselius, P., and Kuylenstierna, B. (1996). Growth and decline of a diatom spring bloom phytoplankton species composition, formation of marine snow and the role of heterotrophic dinoflagellates. J. Plank. Res. 18, 133–155. doi: 10.1093/plankt/18.2.133
Tolhurst, T. J., Gust, G., and Paterson, D. M. (2002). The influence of an extracellular polymeric substance (EPS) on cohesive sediment stability. Proc. Marine Sci. 5, 409–425. doi: 10.1016/S1568-2692(02)80030-4
Torti, A., Lever, M. A., and Jorgensen, B. B. (2015). Origin, dynamics and implications of extracellular DNA pools in marine sediments. Mar. Genom. 24, 185–196. doi: 10.1016/j.margen.2015.08.007
Tourney, J., and Ngwenya, B. T. (2014). The role of bacterial extracellular polymeric substances in geomicrobiology. Chem. Geol. 386, 115–132. doi: 10.1128/AEM.06568-11
Tran, C., and Hadfield, M. G. (2011). Larvae of Pocillopora damicornis (Anthozoa) settle and metamorphose in response to surface-biofilm bacteria. Mar. Ecol. Prog. Ser. 433, 85–96. doi: 10.3354/meps09192
Treguer, P., Legendre, L., Rivkin, R. T., Ragueneau, O., and Dittert, N. (2003). “Water column biogeochemistry below the euphotic zone,” in Ocean Biogeochemistry: A Synthesis of the Joint Global Ocean Flux Study (JGOFS), ed. J. R. M. Fasham (Berlin: Springer-Verlag), 145–156.
Tunnicliffe, V. (1991). The biology of hydrothermal vents: ecology and evolution. Oceanogr. Mar. Biol. Ann. Rev. 29, 319–408.
Tyree, C. A., Hellion, V. M., Alexandrova, O. A., and Allen, J. O. (2007). Foam droplets generated from natural and artificial seawaters. J. Geophys. Res. 112:D12204. doi: 10.1029/2006jd007729
Ulrich, M. (2009). Bacterial Polysaccharides: Current Innovations and Future Trends. Norwich: Caister Academic Press.
Unabia, C. R. C., and Hadfield, M. G. (1999). Role of bacteria in larval settlement and metamorphosis of the polychaete Hydroides elegans. Mar. Biol. 133, 55–64. doi: 10.1007/s002270050442
Underwood, G. J. C., Aslam, S. N., Niemi, A., Norman, L., Meiners, K. M., Laybourn-Parry, J., et al. (2013). Broad-scale predictability of carbohydrates and EPS in Antarctic and Arctic sea ice. Proc. Natl. Acad. Sci. U.S.A. 110, 15734–15739. doi: 10.1073/pnas.1302870110
Underwood, G. J. C., Boulcott, M., Raines, C. A., and Waldron, K. (2004). Environmental effects on exopolymer production by marine benthic diatoms – dynamics, changes in composition and pathways of production. J. Phycol. 40, 293–304. doi: 10.1111/j.1529-8817.2004.03076.x
Underwood, G. J. C., Fietz, S., Papadimitriou, S., Thomas, D. N., and Dieckmann, G. S. (2010). Distribution and composition of dissolved extracellular polymeric substances (EPS) in Antarctic sea ice. Mar. Ecol. Prog. Ser. 404, 1–19. doi: 10.3354/meps08557
Underwood, G. J. C., and Paterson, D. M. (2003). The importance of extracellular carbohydrate production by marine epipelic diatoms. Adv. Bot. Res. 40, 184–240. doi: 10.1016/S0065-2296(05)40005-1
Underwood, G. J. C., Paterson, D. M., and Parkes, R. J. (1995). The measurement of microbial carbohydrate EPS from intertidal sediments. Limnol. Oceanogr. 40, 1243–1253. doi: 10.4319/lo.1995.40.7.1243
Valentine, D. L., Fisher, G. B., Bagby, S. C., Nelson, R. K., Reddy, C. M., Sylva, S. P., et al. (2014). Fallout plume of submerged oil from Deepwater Horizon. Proc. Natl. Acad. Sci. U.S.A 111, 15906–15911. doi: 10.1073/pnas.1414873111
van Gerven, N., Klein, R. D., Hultgren, S. J., and Remaut, H. (2015). Bacterial amyloid formation: structural insights into curli biogenesis. Trends Microbiol. 23, 693–706. doi: 10.1016/j.tim.2015.07.010
Varenyam, A., Mukherjee, A., and Reddy, M. S. (2010). Characterization of two urease-producing and calcifying Bacillus spp. Isolated from cement. J. Microbiol. Biotechnol. 20, 1571–1576. doi: 10.4014/jmb.1006.06032
Vasconcelos, C., Warthmann, R., McKenzie, J., Visscher, P. T., Bittermann, A. G., and van Lith, Y. (2006). Lithifying microbial mats in lagoa vermelha, brazil: modern precambrian relics? Sed. Geol. 185, 175–183. doi: 10.1016/j.sedgeo.2005.12.022
Verdugo, P. (1994). Polymer gel phase transition in condensation-decondensation of secretory products. Adv. Polymer Sci. 110, 145–156. doi: 10.1007/BFb0021131
Verdugo, P. (2012). Marine microgels. Ann. Rev. Marine Sci. 4, 375–400. doi: 10.1146/annurev-marine-120709-142759
Verdugo, P., Alldredge, A. L., Azam, F., Kirchman, D. L., Passow, U., and Santschi, P. (2004). The oceanic gel phase: a bridge in the DOM-POM continuum. Mar. Chem. 92, 67–85. doi: 10.1016/j.marchem.2004.06.017
Verdugo, P., and Santschi, P. (2010). Polymer dynamics of DOC networks and gel formation in seawater. Deep-Sea Res. II. 57, 1486–1493. doi: 10.1016/j.dsr2.2010.03.002
Visscher, P. T., Reid, R. P., and Bebout, B. M. (2000). Microscale observations of sulfate reduction: evidence of microbial activity forming lithified micritic laminae in modern marine stromatolites. Geology 28, 919–922. doi: 10.1130/0091-7613(2000)28<919:MOOSRC>2.0.CO;2
Visscher, P. T., and Stolz, J. F. (2005). Microbial mats as bioreactors: populations, processes, and products. Palaeogeo. Palaeoclim. Palaeoecol. 219, 87–100. doi: 10.1016/j.palaeo.2004.10.016
Vreeland, R. H., Rosenzweig, W. D., and Powers, D. W. (2000). Isolation of a 250 million-year-old halotolerant bacterium from a primary salt crystal. Nature 407, 897–900. doi: 10.1038/35038060
Wagner-Dobler, I., Thiel, V., Eberl, L., Allgaier, M., Bodor, A., Meyer, S., et al. (2005). Discovery of complex mixtures of novel long-chain quorum sensing signals in free-living and host-associated marine alphaproteobacteria. Chembiochem 6, 2195–2206. doi: 10.1002/cbic.200500189
Waharte, F., Steenkeste, K., Briandet, R., and Fontaine-Aupart, M. P. (2010). Diffusion measurements inside biofilms by image-based fluorescence recovery after photobleaching (FRAP) analysis with a commercial confocal laser scanning microscope. Appl. Environ. Microbiol. 76, 5860–5869. doi: 10.1128/AEM.00754-10
Walker, B. D., Beaupré, S. R., Guilderson, T. P., McCarthy, M. D., and Druffel, E. R. M. (2016). Pacific carbon cycling constrained by organic matter size, age and composition relationships. Nat. Geosci. 9, 888–891. doi: 10.1038/ngeo2830
Waters, C. M., and Bassler, B. L. (2005). Quorum sensing: cell-to-cell communication in bacteria. Annu. Revs. Cell Dev. Biol. 21, 319–346. doi: 10.1146/annurev.cellbio.21.012704.131001
Werlin, R., Priester, J. H., Mielke, R. E., Kramer, S., Jackson, S., Stoimenov, P. K., et al. (2011). Biomagnification of cadmium selenide quantum dots in a simple experimental microbial food chain. Nat. Nanotechnol. 6, 65–71. doi: 10.1038/nnano.2010.251
Whalan, S., and Webster, N. S. (2014). Sponge larval settlement cues: the role of microbial biofilms in a warming ocean. Sci. Rep. 4:4072. doi: 10.1038/srep04072
Whitchurch, C. B., Tolker-Nielsen, T., Ragas, P. C., and Mattick, J. S. (2002). Extracellular DNA required for bacterial biofilm formation. Science 295:1487. doi: 10.1126/science.295.5559.1487
Whitfield, C. (2006). Biosynthesis and assembly of capsular polysaccharides in Escherichia coli. Annu. Rev. Biochem. 75, 39–68. doi: 10.1146/annurev.biochem.75.103004.142545
Widenfalk, A., Lundqvist, A., and Goedkoop, W. (2008). Sediment microbes and biofilms increase the bioavailability of chlorpyrifos in Chironomus riparius (Chironomidae, Diptera). Ecotox. Environ. Saf. 71, 490–497. doi: 10.1016/j.ecoenv.2007.10.028
Williams, P. M., and Druffel, E. R. M. (1987). Radiocarbon in dissolved organic matter in the central North Pacific Ocean. Nature 330, 246–248. doi: 10.1038/330246a0
Wilson, T. W., Ladino, L. A., Alpert, P. A., Breckels, M. N., Brooks, I. M., Browse, J., et al. (2015). A marine biogenic source of atmospheric ice-nucleating particles. Nature 525, 234–238. doi: 10.1038/nature14986
Wotton, R. S. (2004). The ubiquity and many roles of EPS (EPS) in aquatic systems. Oceanogr. Mar. Biol. Annu. Revs. 42, 57–94. doi: 10.1201/9780203507810.ch3
Wurl, O. (2009). Practical Guidelines for the Analysis of Seawater. Boca Raton, FL: CRC Press. doi: 10.1201/9781420073072
Wurl, O., and Holmes, M. (2008). The gelatinous nature of the sea-surface microlayer. Mar. Chem. 110, 89–97. doi: 10.1016/j.marchem.2008.02.009
Wurl, O., Miller, L., and Vagle, S. (2011). Production and fate of transparent exopolymer particles in the ocean. J. Geophys. Res. 116:C00H13. doi: 10.1111/j.1462-2920.2012.02873.x
Yang, C., Fang, S., Chen, D., Wang, J., Liu, F., and Xia, C. (2016). The possible role of bacterial signal molecules N-acyl homoserine lactones in the formation of diatom-biofilm (Cylindrotheca sp.). Mar. Poll. Bull. 107, 118–124. doi: 10.1016/j.marpolbul.2016.04.010
Yang, M., Stipp, S. L. S., and Harding, J. (2008). Biological control on calcite crystallization by polysaccharides. Cryst. Growth Des. 8, 4066–4074. doi: 10.1016/j.jsb.2015.03.007
Zhang, G., Zhang, F., Ding, G., Li, J., Guo, X., Zhu, J., et al. (2012). Acyl homoserine lactone-based quorum sensing in a methanogenic archaeon. ISME J. 6, 1336–1344. doi: 10.1038/ismej.2011.203
Zhang, S., Xu, C., and Santschi, P. H. (2008). Chemical composition and Thorium-234 (IV) binding of extracellular polymeric substances (EPS) by the marine diatom Amphora sp. Mar. Chem. 106, 967–976.
Zhang, W., Sun, J., Ding, W., Lin, J., Tian, R., Lu, L., et al. (2015). Extracellular matrix-associated proteins form an integral and dynamic system during Pseudomonas aeruginosa biofilm development. Front. Cell. Infect. Microbiol. 5:40. doi: 10.3389/fcimb.2015.00040
Zheng, G., Vad, B. S., Dueholm, M. S., Christiansen, G., Nilsson, M., Tolker-Nielsen, T., et al. (2015). Functional bacterial amyloid increases Pseudomonas biofilm hydrophobicity and stiffness. Front. Microbiol. 6:1099. doi: 10.3389/fmicb.2015.01099
Ziervogel, K., McKay, L., Rhodes, B., Osburn, C. L., Dickson-Brown, J., Arnosti, C., et al. (2012). Microbial activities and dissolved organic matter dynamics in oil- contaminated surface seawater from the Deepwater Horizon oil spill site. PLoS ONE 7:e34816. doi: 10.1371/journal.pone.0034816
Zippel, B., and Neu, T. R. (2011). Characterization of glycoconjugates of extracellular polymeric substances in Tufa-associated biofilms by using fluorescence lectin-binding analysis. Appl. Environ. Microbiol. 77, 505–516. doi: 10.1128/AEM.01660-10
ZoBell, C. E., and Allen, E. C. (1935). The significance of marine bacteria in the fouling of submerged surfaces. J. Bact. 29, 239–251.
Zohary, T., and Robarts, R. D. (1998). Experimental study of microbial P limitation in the eastern Mediterranean. Limnol. Oceanogr. 43, 387–395. doi: 10.1007/s00248-015-0713-5
Keywords: EPS, biofilm, organic matter, oceans research, bacteria
Citation: Decho AW and Gutierrez T (2017) Microbial Extracellular Polymeric Substances (EPSs) in Ocean Systems. Front. Microbiol. 8:922. doi: 10.3389/fmicb.2017.00922
Received: 06 March 2017; Accepted: 08 May 2017;
Published: 26 May 2017.
Edited by:
Télesphore Sime-Ngando, Centre National de la Recherche Scientifique (CNRS), FranceReviewed by:
Varenyam Achal, East China Normal University, ChinaEric D. van Hullebusch, UNESCO-IHE Institute for Water Education, Netherlands
Copyright © 2017 Decho and Gutierrez. This is an open-access article distributed under the terms of the Creative Commons Attribution License (CC BY). The use, distribution or reproduction in other forums is permitted, provided the original author(s) or licensor are credited and that the original publication in this journal is cited, in accordance with accepted academic practice. No use, distribution or reproduction is permitted which does not comply with these terms.
*Correspondence: Alan W. Decho, YXdkZWNob0BtYWlsYm94LnNjLmVkdQ==