- 1Institute of Antibiotics, Huashan Hospital, Fudan University, Shanghai, China
- 2Key Laboratory of Clinical Pharmacology of Antibiotics, National Health and Family Planning Commission, Shanghai, China
With the increasing spread of methicillin-resistant Staphylococcus aureus worldwide, fosfomycin has begun to be used more often, either alone or in combination with other antibiotics, for treating methicillin-resistant S. aureus infections, resulting in the emergence of fosfomycin-resistant strains. Fosfomycin resistance is reported to be mediated by fosfomycin-modifying enzymes (FosA, FosB, FosC, and FosX) and mutations of the target enzyme MurA or the membrane transporter proteins UhpT and GlpT. Our previous studies indicated that the fos genes might not the major fosfomycin resistance mechanism in S. aureus, whereas mutations of glpT and uhpT seemed to be more related to fosfomycin resistance. However, the precise role of these two genes in S. aureus fosfomycin resistance remains unclear. The aim of the present study was to investigate the role of glpT and uhpT in S. aureus fosfomycin resistance. Homologous recombination was used to knockout the uhpT and glpT genes in S. aureus Newman. Gene complementation was generated by the plasmid pRB473 carrying these two genes. The fosfomycin minimal inhibitory concentration (MIC) of the strains was measured by the E-test to observe the influence of gene deletion on antibiotic susceptibility. In addition, growth curves were constructed to determine whether the mutations have a significant influence on bacterial growth. Deletion of uhpT, glpT, and both of them led to increased fosfomycin MIC 0.5 μg/ml to 32 μg/ml, 4 μg/ml, and >1024 μg/ml, respectively. By complementing uhpT and glpT into the deletion mutants, the fosfomycin MIC decreased from 32 to 0.5 μg/ml and from 4 to 0.25 μg/ml, respectively. Moreover, the transporter gene-deleted strains showed no obvious difference in growth curves compared to the parental strain. In summary, our study strongly suggests that mutations of uhpT and glpT lead to fosfomycin resistance in S. aureus, and that uhpT mutation may play a more important role. The high resistance and low biological fitness cost resulting from uhpT and glpT deletion suggest that these strains might have an evolutionary advantage in a fosfomycin-rich clinical situation, which should be closely monitored.
Introduction
Staphylococcus aureus is one of the most common bacterial pathogens worldwide in both community and hospital settings. Methicillin-resistant Staphylococcus aureus (MRSA) is an important multi-resistant pathogen. To date, vancomycin has remained the cornerstone drug in the management of invasive MRSA infections. However, the continuous rise in the vancomycin minimum inhibitory concentration (MIC), known as the “vancomycin MIC creep” phenomenon, poses a significant challenge to MRSA therapy; therefore, fosfomycin has recently been used alone or in combination with other antibiotics in treating MRSA infections (del Rio et al., 2016; VanEperen and Segreti, 2016). Nevertheless, this situation has inevitably led to the emergence of fosfomycin-resistant MRSA strains. In a recent review, the susceptibility of S. aureus to fosfomycin ranged between 33.2 and 100% in the nine available studies (frequency = 91.7%, 95% confidence interval 88.7–94.9%); in seven of the studies susceptibility rate was >90% (Vardakas et al., 2016). According to the CHINET surveillance program in China in 2010, 29.5% of the MRSA clinical isolates were resistant to fosfomycin (Guo et al., 2013). And Yu et al. (2010) reported a fosfomycin susceptible rate of 33.2%.
The mechanisms of action and resistance of fosfomycin have been studied for decades. Fosfomycin was first discovered in 1969 as an effective bactericidal agent against Gram-positive and Gram-negative organisms. The mechanism of action of fosfomycin differs from that of most commonly used antimicrobials. In general, fosfomycin is transported across the bacterial wall primarily with the help of the glycerol-3-phosphate (G-3-P) transport (GlpT) system. In the presence of glucose-6-phosphate (G-6-P), the hexose phosphate uptake transport (UhpT) system is induced, and provides an alternative route to the GlpT system. UhpT are important membrane transporter proteins for small molecules, including fosfomycin (Castaneda-Garcia et al., 2009). When transported into the cytosol of a bacterium, fosfomycin deactivates the target protein UDP-N-acetylglucosamine-3-enolpyruvyltransferase (MurA, encoded by the murA gene), thereby preventing the formation of N-acetylmuramic acid from N-acetylglucosamine and phosphoenolpyruvate, which is the initial step in peptidoglycan chain formation of the bacterial wall (Kahan et al., 1974). The key resistance mechanisms to fosfomycin include the loss or reduced production of transporters, reduced affinity to MurA, and production of fosfomycin-modifying enzymes (Sastry and Doi, 2016).
However, to date, the mechanisms contributing to fosfomycin resistance have been mostly studied in Gram-negative bacteria, with few related studies on Gram-positive bacteria. We have conducted several studies to investigate the fosfomycin resistance mechanisms in Gram-positive cocci, including Enterococcus faecium and S. aureus (Xu et al., 2013; Chen et al., 2014; Fu et al., 2016a,b). These previous studies indicated that the fos gene was not the major mechanism of fosfomycin resistance in MRSA isolates from our hospital, whereas mutations of glpT and uhpT seemed to be more closely related to fosfomycin resistance. However, the exact roles of these two genes in S. aureus fosfomycin resistance remain unclear. Thus, we designed the present study to investigate the roles of glpT and uhpT in S. aureus fosfomycin resistance.
Materials and Methods
Bacterial Strains and Plasmids
The strains and plasmids used in this study are presented in Table 1. The clinical fosfomycin-resistant MRSA strains SA2, SA94, and SA30 were collected from the blood or cerebral spinal fluid of patients at Huashan Hospital and were characterized previously (Fu et al., 2016b). And the strain names are in accordance with that in the Supplementary Table S1 of the previous article (Fu et al., 2016b). Each of the clinical MRSA strains was with a different type of transporter gene mutation (Table 1). The S. aureus strains Newman and RN4220, and the plasmid pKOR1 were used in the homologous recombination assay (Bae and Schneewind, 2005; Wang et al., 2015). In addition, S. aureus ATCC29213 (American Type Tissue Culture Collection, Manassas, VA, United States) was used for the quality control of susceptibility testing. These strains and plasmids were laboratory collection.
Allelic Gene Deletion by Homologous Recombination
Knockout of the transporter genes glpT and uhpT was conducted as previously described (Bae and Schneewind, 2005; Wang et al., 2015). The plasmids and primers used are listed in Tables 1, 2, respectively. Proper gene deletion was verified by analytical polymerase chain reaction (PCR) and sequencing of the genomic DNA at the borders of the PCR-derived regions. Sequencing was then performed to confirm the nucleotides. The amplified fragments were used to construct the homologous recombinant pKOR1-ΔuhpT/glpT with Gateway®BP ClonaseTM II Enzyme mix (Thermo Fisher Scientific, Waltham, MA, United States).
pKOR1-ΔuhpT and pKOR1-ΔglpT were introduced into S. aureus RN4220 by electroporation for modification. The plasmid extracted from strain RN4220 was then introduced into S. aureus Newman. The desired uhpT and glpT deletion mutants were selected as described previously (Bae and Schneewind, 2005).
The successful generation of the Newman-ΔuhpT and Newman-ΔglpT strains was further confirmed by PCR and sequencing. PCRs were performed using the primers attB1-uhpT-up-F/attB2-uhpT-CF and attB1-glpT-up-F/attB2-glpT-CF in the strains S. aureus Newman, Newman-ΔuhpT, and Newman-ΔglpT, respectively.
Construction of the Complemented Strain
Fragments were PCR-amplified from S. aureus Newman using the primers C-uhpT-F/R and C-glpT-F/R. The PCR products and vector pRB473 were double-digested with the designed restriction enzymes BamHI and EcoRI (for uhpT), or BamHI and KpnI (for glpT), and ligation was performed with T4 ligase. The resulting plasmids were transferred into S. aureus RN4220, and then introduced into the deletion and clinical strains with defects on uhpT and/or glpT, SA2, SA94, and SA30.
Antimicrobial Susceptibility Testing
Fosfomycin susceptibility of the knockout and clinical strains with defects on uhpT and/or glpT, and their complemented strains were tested with the E-test (BioMerieux SA, La Balme Les Grotts, France), according to the manufacturer’s guidance. Results were interpreted according to European committee on antimicrobial susceptibility testing criteria (European Committee on Antimicrobial Susceptibility Testing [EUCAST], 2017) (susceptible, ≤32 mg/L; resistant, ≥64 mg/L).
Measurement of Growth Curves
To evaluate the influence of deletion of the transporter genes on bacterial growth, we measured the in vitro growth curves of S. aureus Newman, Newman-ΔuhpT, Newman-ΔglpT, Newman-ΔuhpT&glpT, and the clinical strains. The strains were cultivated in tryptic soy broth overnight at 37°C. The bacterial solution was diluted to an optical density at 600 nm (OD600) of 0.1 and cultivated again. The OD600 was then measured at 0, 2, 4, 6, 8, 10, 12, 14, 16, 18, 20, 22, and 24 h for each strain, by spectrophotometer (UNICO, Shanghai, China). The procedure was repeated three times for each strain, and the mean OD600 values were used to draw the growth curves.
Phenotype Microarray (PM) Analysis
Phenotype Microarray analysis was performed using BIOLOG Phenotype MicroarrayTM (BIOLOG, Hayward, CA, United States) according to the manufacturer’s recommendations. The deletion mutants, namely Newman-ΔuhpT, Newman-ΔglpT, Newman-ΔuhpT&glpT, and the parental strain Newman, were tested with the 96-wells plates PM1 and PM2, containing 190 carbon substrates, including G-6-P (PM1 plate, well C1). To assess the altered phenotypes in carbon metabolism of the deletion mutants, the growth was compared to the parent S. aureus Newman.
Results
The deletion mutants showed considerably increased MIC values to fosfomycin compared to that of the parental strain. The Newman-ΔuhpT&glpT strain, in which both transporter genes were knocked out, showed high-level resistance (MIC > 1024 μg/ml) to fosfomycin, as determined by the E-test (Table 3). When uhpT or glpT was knocked out from S. aureus Newman, the fosfomycin MICs increased from 0.5 to 32 μg/ml or 4 μg/ml, respectively.
Complementing uhpT and glpT led to a reduced fosfomycin MIC in the deletion mutants and the clinical fosfomycin-resistant S. aureus strains with defects at both sites. By complementing plasmid pRB473-uhpT into Newman-ΔuhpT, the strain’s fosfomycin MIC decreased from 32 to 0.5 μg/ml. Similarly, by complementing glpT into Newman-ΔglpT, strain’s fosfomycin MIC decreased from 4 to 0.25 μg/ml. S. aureus SA2, SA94, and SA30 were clinical fosfomycin-resistant strains, with mutations of both uhpT and glpT, uhpT only, and glpT only, respectively. When complemented with the functional transporter genes, the fosfomycin MICs decreased considerably, as shown in Table 3.
In vitro bacterial growth curves of the wild-type strain S. aureus Newman and the deletion mutants were compared to evaluate the potential fitness cost of these resistant-conferring mutations. As shown in Figure 1, no significant depression in growth was observed in Newman-ΔuhpT and Newman-ΔglpT compared to the wild-type strain. However, the strain Newman-ΔuhpT&glpT presented slight growth inhibition compared to the wild-type.
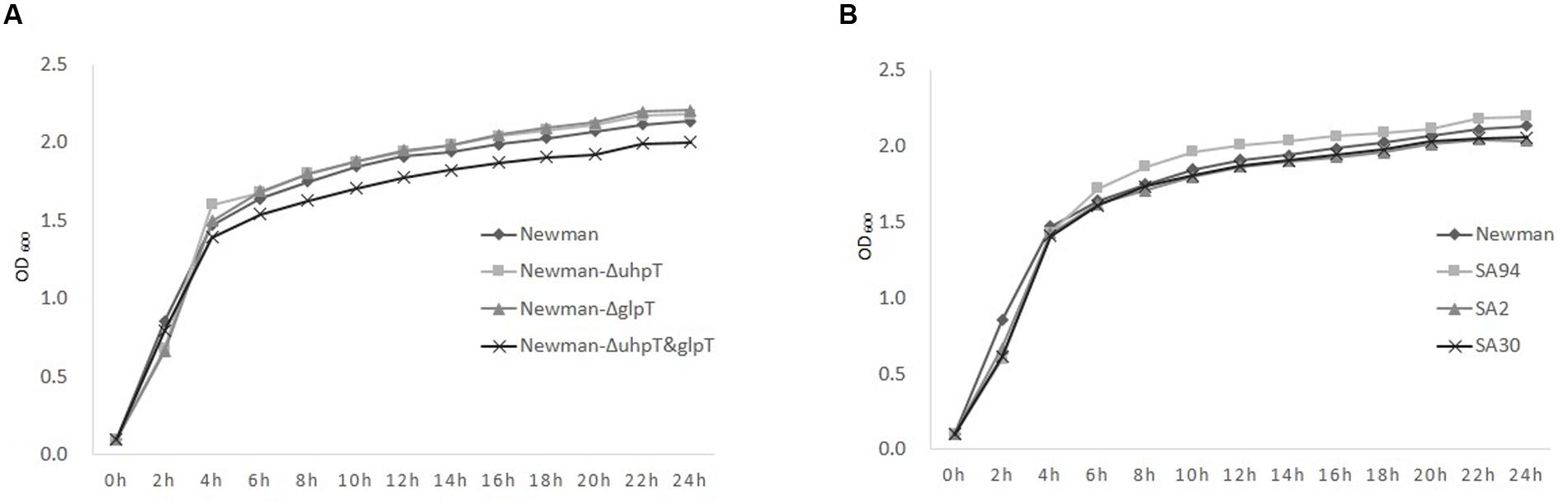
FIGURE 1. The in vitro growth curves of S. aureus strains. The strains were cultivated in tryptic soy broth overnight at 37°C. The bacterial solution was diluted to an optical density at 600 nm (OD600) of 0.1 and cultivated again. The OD600 was then measured at 0, 2, 4, 6, 8, 10, 12, 14, 16, 18, 20, 22, and 24 h to draw the curves. (A) Newman (◆), Newman-ΔuhpT (■), Newman-ΔglpT (▲), and Newman-ΔuhpT&glpT (×). (B) Newman (◆) and the clinical uhpT/glpT mutants: SA2 (▲), with mutations of both glpT and uhpT; SA94 (■), with mutation of uhpT; SA30 (×), with mutation of glpT.
Phenotype Microarray analysis was performed using carbon utilization panels, PM1 and PM2, in 190 carbon substrates. The changes in carbon metabolism were listed in Figure 2. S. aureus Newman showed metabolic advantage over Newman-ΔuhpT and Newman-ΔuhpT&glpT in wells containing G-6-P (Figure 2, PM1, well C1). G-3-P was not included in the substrate list, and there was no obvious change found in Newman-ΔglpT.
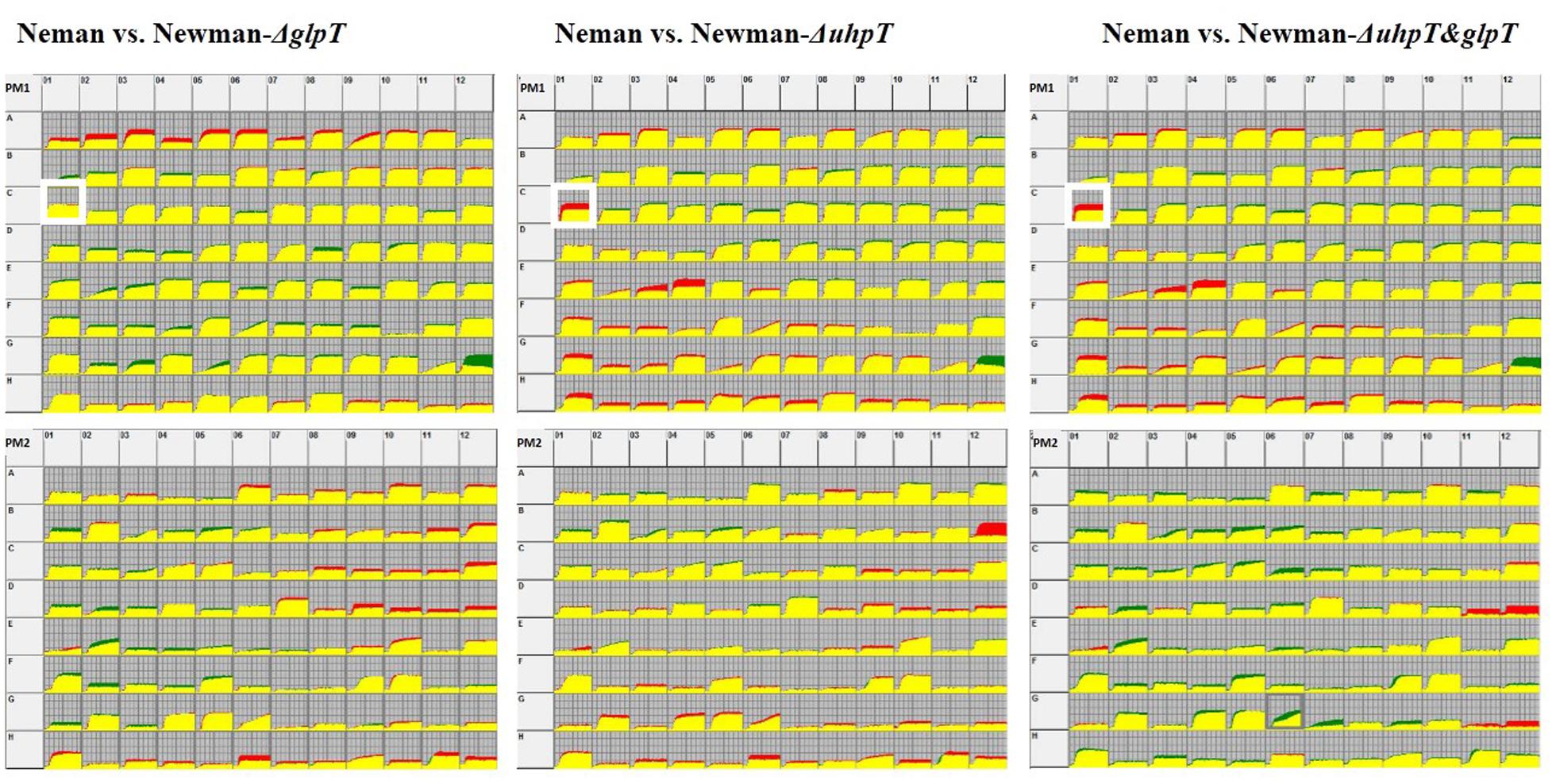
FIGURE 2. PM analysis for carbon metabolism. The strains were grown in a 96-well plate under 37°C over two nights. Carbon utilization kinetics was measured with OmniLog instrument. Data were superimposed using OmniLog software. The kinetic results show consensus data comparing S. aureus Newman and its transporter gene deletion mutants. A metabolic advantage by S. aureus Newman is indicated by red, while a metabolic advantage by the deletion mutants is shown by green. The yellow part indicates that both strains have equal metabolism in the well. The white box around PM1 plate, well C1, highlights the G-6-P metabolism. Detailed substrate information of PM1 and PM2 were shown in the Supplementary Table S1.
Discussion
Intravenous fosfomycin is broadly used in the treatment of multidrug-resistant pathogens in Europe and Asia owing to its unique antibiotic mechanism, high permeability, and high susceptibility rate (Falagas et al., 2009). Falagas et al. (2010) performed fosfomycin susceptibility testing in non-urinary MRSA isolates, among which 99.2% (129/130) were found to be susceptible. The same group reviewed the susceptibility data of Gram-positive cocci, and reported a cumulative susceptibility rate of 87.9% (4240/4892) in S. aureus (Falagas et al., 2009).
Fosfomycin-resistance mechanism has been well described for Gram-negative bacteria such as E. coli (Kim et al., 1996; Horii et al., 1999; Huang et al., 2003; Takahata et al., 2010). In E. coli, GlpT and UhpT are responsible for fosfomycin uptake. Mutations or insertional inactivation in the glpT and/or uhpT genes or their regulatory genes lead to the loss of function of the transporters and fosfomycin resistance. The inactivation of either uhpT or glpT conferred a moderate fosfomycin resistance, (MICs increased from 2 to 8 μg/ml and 32 μg/ml, respectively, compared to the wild type) (Takahata et al., 2010). In P. aeruginosa, the inactivation of glpT produced significant decrease in fosfomycin MIC, from 8 to 1024 μg/ml (Castaneda-Garcia et al., 2009; Takahata et al., 2010). Modification or overexpression of murA, production of fosfomycin-modifying enzymes, are also associated with fosfomycin resistance (Garcia et al., 1995; Kim et al., 1996; Bernat et al., 1997; Horii et al., 1999; Fillgrove et al., 2003; Roberts et al., 2013).
There is less known of fosfomycin resistance mechanism in Gram-positive cocci. In previous works, we collected MRSA clinical strains, and found that only the minority of the fosfomycin-resistant MRSA strains carried the fos gene or murA mutation, while glpT and uhpT mutations were common (82.1%, 55/67, vs. 77.6%, 52/67, respectively) (Fu et al., 2016a,b). This fact indicated that, fosB or murA mutation is not the major contributor to fosfomycin resistance in MRSA, while mutations within the glpT and/or uhpT genes might play an important role in S. aureus fosfomycin resistance. In the present study, we established uhpT and/or glpT deletion mutants. Knocking out both genes resulted in high-level fosfomycin resistance (MIC > 1024 μg/ml). Complementing either of the two genes into the deletion mutants and clinical mutated strains resulted in a decreased fosfomycin MIC. Direct comparison of uhpT and glpT according to the level of increase of the MIC suggested that uhpT has a greater effect on the strain’s MIC to fosfomycin than glpT.
To evaluate the possible, the in vitro fitness cost of the transporter gene mutation, we compared growth curves between the fosfomycin-sensitive wild-type strain, laboratory deletion mutant strain, and clinical strains with defects on uhpT and/or glpT. Previous reports have shown that mutations of uhpT and glpT can compromise the growth of strains of E. coli, Klebsiella pneumoniae, and Proteus mirabilis (Li Pira et al., 1987; Marchese et al., 2003). A probable mechanism might be that mutations of the glpT and/or uhpT transporting systems prevent carbon source getting into the cytoplasm, and therefore disturb cell metabolism (Venkateswaran and Wu, 1972; Nilsson et al., 2003). But in P. aeruginosa, the glpT mutation was found to lead to fosfomycin resistance with no obvious fitness cost (Castaneda-Garcia et al., 2009). In the present study, there was only a slight reduction of growth observed in the strain Newman-ΔuhpT&glpT compared to the wild type, and no significant growth suppression was observed either in the laboratory deletion mutant strains or in clinical strains with defects on uhpT and/or glpT, which is similar as observed in P. aeruginosa. So S. aureus might also compensate the disadvantage in energy obtainment caused by uhpT and/or glpT mutation through other transporting systems. But further study is still in need for verification.
We observed that G-6-P utilization was defected in both Newman-ΔuhpT and Newman-ΔuhpT&glpT. UhpT is the membrane transporter of this substrate, deletion mutants of uhpT showed defects in G-6-P metabolism is as expected. The G-3-P metabolism in S. aureus seems to be more complicated. G-3-P seems to be an intermediate product in carbon/phosphorus metabolism pathway. As another low G+C Gram-positive bacteria, B. subtilis shares similar carbon metabolism pattern as S. aureus. In B. subtilis, G-3-P is produced from glycerol with glycerol kinase. And G-3-P dehydrogenase can oxidize G-3-P to dihydroxyacetone phosphate, an intermediated in glycolysis (Holmberg et al., 1990). We have not observed significant change in metabolism. This may be because that G-3-P utilization defect is easily compensated by other pathways.
In summary, the results of our study strongly suggest that mutations of uhpT and glpT lead to fosfomycin resistance in S. aureus, and that the uhpT mutation may play a more important role. The high resistance and low fitness cost resulting from uhpT and glpT mutations suggest that these mutated strains might have an evolutionary advantage in a fosfomycin-rich clinical situation. The widely observed uhpT or glpT mutation in S. aureus might be a threat in hospital settings. Further studies are needed to evaluate the frequency of S. aureus fosfomycin mutants, and virulence of these mutants.
Author Contributions
Designed and conceived the experiments: YL, XX, and MW. Performed the experiments: SX, ZF, and YZ. Analyzed the data: SX, ZF, and YZ. Wrote and reviewed the manuscript: SX, ZF, XX, and YL.
Funding
This study was supported by grants from the National Natural Science Foundation of China (81171613 to XX and 81120108024 to MW).
Conflict of Interest Statement
The authors declare that the research was conducted in the absence of any commercial or financial relationships that could be construed as a potential conflict of interest.
Acknowledgment
We thank Professor M. Li, Renji Hospital, Shanghai Jiaotong University, for providing strain S. aureus RN4220 and plasmid pKOR1.
Supplementary Material
The Supplementary Material for this article can be found online at: http://journal.frontiersin.org/article/10.3389/fmicb.2017.00914/full#supplementary-material
TABLE S1 | Carbon sources tested in PM1 and PM2 plates.
References
Bae, T., and Schneewind, O. (2005). Allelic replacement in Staphylococcus aureus with inducible counter-selection. Plasmid 55, 58–63. doi: 10.1016/j.plasmid.2005.05.005
Bernat, B. A., Laughlin, L. T., and Armstrong, R. N. (1997). Fosfomycin resistance protein (FosA) is a manganese metalloglutathionetransferase related to glyoxalase I and the extradioldioxygenases. Biochemistry 36, 3050–3055. doi: 10.1021/bi963172a
Castaneda-Garcia, A., Rodriguez-Rojas, A., Guelfo, J. R., and Blazquez, J. (2009). The glycerol-3-phosphate permeaseGlpT is the only fosfomycin transporter in Pseudomonas aeruginosa. J. Bacteriol. 191, 6968–6974. doi: 10.1128/JB.00748-09
Chen, C., Xu, X., Qu, T., Yu, Y., Ying, C., Liu, Q., et al. (2014). Prevalence of the fosfomycin-resistance determinant, fosB3, in Enterococcus faecium clinical isolates from China. J. Med. Microbiol. 63, 1484–1489. doi: 10.1099/jmm.0.077701-0
del Rio, A., Garcia-De-La-Maria, C., Entenza, J. M., Gasch, O., Armero, Y., Soy, D., et al. (2016). Fosfomycin plus beta-Lactams as synergistic bactericidal combinations for experimental endocarditis due to methicillin-resistant and glycopeptide-intermediate Staphylococcus aureus. Antimicrob. Agents Chemother. 60, 478–486. doi: 10.1128/AAC.02139-15
European Committee on Antimicrobial Susceptibility Testing [EUCAST] (2017). Available at: http://www.eucast.org
Falagas, M. E., Maraki, S., Karageorgopoulos, D. E., Kastoris, A. C., Kapaskelis, A., and Samonis, G. (2010). Antimicrobial susceptibility of gram-positive non-urinary isolates to fosfomycin. Int. J. Antimicrob. Agents 35, 497–499. doi: 10.1016/j.ijantimicag.2010.01.010
Falagas, M. E., Roussos, N., Gkegkes, I. D., Rafailidis, P. I., and Karageorgopoulos, D. E. (2009). Fosfomycin for the treatment of infections caused by Gram-positive cocci with advanced antimicrobial drug resistance: a review of microbiological, animal and clinical studies. Expert Opin. Investig. Drugs 18, 921–944. doi: 10.1517/13543780902967624
Fillgrove, K. L., Pakhomova, S., Newcomer, M. E., and Armstrong, R. N. (2003). Mechanistic diversity of fosfomycin resistance in pathogenic microorganisms. J. Am. Chem. Soc. 125, 15730–15731. doi: 10.1021/ja039307z
Fu, Z., Liu, Y., Chen, C., Guo, Y., Ma, Y., Yang, Y., et al. (2016a). Characterization of fosfomycin resistance gene, fosB, in methicillin-resistant Staphylococcus aureus isolates. PLoS ONE 11:e0154829. doi: 10.1371/journal.pone.0154829
Fu, Z., Ma, Y., Chen, C., Guo, Y., Hu, F., Liu, Y., et al. (2016b). Prevalence of fosfomycin resistance and mutations in murA, glpT, and uhpT in methicillin-resistant Staphylococcus aureus strains isolated from blood and cerebrospinal fluid samples. Front. Microbiol. 6:1544. doi: 10.3389/fmicb.2015.01544
Garcia, P., Arca, P., and Evaristo Suarez, J. (1995). Product of fosC, a gene from Pseudomonas syringae, mediates fosfomycin resistance by using ATP as cosubstrate. Antimicrob. Agents Chemother. 39, 1569–1573. doi: 10.1128/AAC.39.7.1569
Guo, Y., Zhu, D., Hu, F., Wang, F., Yu, Y., Yang, Q., et al. (2013). CHINET 2010 surveillance and analysis of antibiotic resistance in Staphylococcus spp. in China. Chin. J. Infect. Chemother. 13, 86–92.
Holmberg, C., Beijer, L., Rutberg, B., and Rutverg, L. (1990). Glycerol catabolism in Bacillus subtilis: nucleotide sequence of the genes encoding glycerol kinase (glpK) and glycerol-e-phosphate dehydrogenase (glpD). J. Gen. Microbiol. 136, 2367–2375. doi: 10.1099/00221287-136-12-2367
Horii, T., Kimura, T., Sato, K., Shibayama, K., and Ohta, M. (1999). Emergence of fosfomycin-resistant isolates of Shiga-like toxin-producing Escherichia coli O26. Antimicrob. Agents Chemother. 43, 789–793.
Huang, Y., Lemieux, M. J., Song, J., Auer, M., and Wang, D. N. (2003). Structure and mechanism of the glycerol-3-phosphate transporter from Escherichia coli. Science 301, 616–620. doi: 10.1126/science.1087619
Kahan, F. M., Kahan, J. S., Cassidy, P. J., and Kropp, H. (1974). The mechanism of action of fosfomycin (phosphonomycin). Ann. N. Y. Acad. Sci. 235, 364–386. doi: 10.1111/j.1749-6632.1974.tb43277.x
Kim, D. H., Lees, W. J., Kempsell, K. E., Lane, W. S., Duncan, K., and Walsh, C. T. (1996). Characterization of a Cys115 to Asp substitution in the Escherichia coli cell wall biosynthetic enzyme UDP-GlcNAcenolpyruvyltransferase (MurA) that confers resistance to inactivation by the antibiotic fosfomycin. Biochemistry 35, 4923–4928. doi: 10.1021/bi952937w
Li Pira, G., Pruzzo, C., and Schito, G. C. (1987). Monuril and modification of pathogenicity traits in resistant microorganisms. Eur. Urol. 13(Suppl. 1), 92–97.
Marchese, A., Gualco, L., Debbia, E. A., Schito, G. C., and Schito, A. M. (2003). In vitro activity of fosfomycin against gram-negative urinary pathogens and the biological cost of fosfomycin resistance. Int. J. Antimicrob Agents 22(Suppl. 2), 53–59. doi: 10.1016/S0924-8579(03)00230-9
Nilsson, A. I., Berg, O. G., Aspevall, O., Kahlmeter, G., and Andersson, D. I. (2003). Biological costs and mechanisms of fosfomycin resistance in Escherichia coli. Antimicrob. Agents Chemother. 47, 2850–2858. doi: 10.1128/AAC.47.9.2850-2858.2003
Roberts, A. A., Sharma, S. V., Strankman, A. W., Duran, S. R., Rawat, M., and Hamilton, C. J. (2013). Mechanistic studies of FosB: a divalent-metal-dependent bacillithiol-S-transferase that mediates fosfomycin resistance in Staphylococcus aureus. Biochem. J. 451, 69–79. doi: 10.1042/BJ20121541
Sastry, S., and Doi, Y. (2016). Fosfomycin: resurgence of an old companion. J. Infect. Chemother. 22, 273–280. doi: 10.1016/j.jiac.2016.01.010
Takahata, S., Ida, T., Hiraishi, T., Sakakibara, S., Maebashi, K., Terada, S., et al. (2010). Molecular mechanisms of fosfomycin resistance in clinical isolates of Escherichia coli. Int. J. Antimicrob. Agents 35, 333–337. doi: 10.1016/j.ijantimicag.2009.11.011
VanEperen, A. S., and Segreti, J. (2016). Empirical therapy in methicillin-resistant Staphylococcus aureus infections: an Up-To-Date approach. J. Infect. Chemother. 22, 351–359. doi: 10.1016/j.jiac.2016.02.012
Vardakas, K. Z., Legakis, N. J., Triarides, N., and Falagas, M. E. (2016). Susceptibility of contemporarty isolates to fosfomycin: a systematic review of the literature. Int. J. Antimicrob. Agents 47, 269–285. doi: 10.1016/j.ijantimicag.2016.02.001
Venkateswaran, P. S., and Wu, H. C. (1972). Isolation and characterization of a phosphonomycin-resistant mutant of Escherichia coli K-12. J. Bacteriol. 110, 935–944.
Wang, W., Chen, J., Chen, G., Du, X., Cui, P., Wu, J., et al. (2015). Transposon mutagenesis identifies novel genes associated with Staphylococcus aureuspersister formation. Front. Microbiol. 6:1437. doi: 10.3389/fmicb.2015.01437
Xu, X., Chen, C., Lin, D., Guo, Q., Hu, F., Zhu, D., et al. (2013). The fosfomycin resistance gene fosB3 is located on a transferable, extrachromosomal circular intermediate in clinical Enterococcus faecium isolates. PLoS ONE 8:e78106. doi: 10.1371/journal.pone.0078106
Keywords: Staphylococcus aureus, fosfomycin, resistance, membrane transporter, glpT, uhpT
Citation: Xu S, Fu Z, Zhou Y, Liu Y, Xu X and Wang M (2017) Mutations of the Transporter Proteins GlpT and UhpT Confer Fosfomycin Resistance in Staphylococcus aureus. Front. Microbiol. 8:914. doi: 10.3389/fmicb.2017.00914
Received: 01 December 2016; Accepted: 04 May 2017;
Published: 19 May 2017.
Edited by:
Axel Cloeckaert, Institut National de la Recherche Agronomique (INRA), FranceReviewed by:
Makoto Kuroda, National Institute of Infectious Diseases, JapanFangyou Yu, First Affiliated Hospital of Wenzhou Medical University, China
Copyright © 2017 Xu, Fu, Zhou, Liu, Xu and Wang. This is an open-access article distributed under the terms of the Creative Commons Attribution License (CC BY). The use, distribution or reproduction in other forums is permitted, provided the original author(s) or licensor are credited and that the original publication in this journal is cited, in accordance with accepted academic practice. No use, distribution or reproduction is permitted which does not comply with these terms.
*Correspondence: Xiaogang Xu, eHV4aWFvZ2FuZ0BmdWRhbi5lZHUuY24= Yang Liu, bGl1eWFuZ0BmdWRhbi5lZHUuY24=