- 1Division of Applied Food System, Major of Food Science and Technology, Seoul Women’s University, Seoul, South Korea
- 2Department of Chemistry and Medical Chemistry, College of Science and Technology, Yonsei University, Wonju, South Korea
Iron or zinc deficiency is one of the most important nutritional disorders which causes health problem. However, food fortification with minerals often induces unacceptable organoleptic changes during preparation process and storage, has low bioavailability and solubility, and is expensive. Nanotechnology surface modification to obtain novel characteristics can be a useful tool to overcome these problems. In this study, the efficacy and potential toxicity of dispersible Fe or Zn supplement coated in dextrin and glycerides (SunActive FeTM and SunActive ZnTM) were evaluated in terms of cytotoxicity, intestinal transport, and bioavailability, as compared with each counterpart without coating, ferric pyrophosphate (FePP) and zinc oxide (ZnO) nanoparticles (NPs), respectively. The results demonstrate that the cytotoxicity of FePP was not significantly affected by surface modification (SunActive FeTM), while SunActive ZnTM was more cytotoxic than ZnO-NPs. Cellular uptake and intestinal transport efficiency of SunActive FeTM were significantly higher than those of its counterpart material, which was in good agreement with enhanced oral absorption efficacy after a single-dose oral administration to rats. These results seem to be related to dissolution, particle dispersibility, and coating stability of materials depending on suspending media. Both SunActiveTM products and their counterpart materials were determined to be primarily transported by microfold (M) cells through the intestinal epithelium. It was, therefore, concluded that surface modification of food fortification will be a useful strategy to enhance oral absorption efficiency at safe levels.
Introduction
Iron deficiency is the most common nutritional disorder often occurs in infants, children, and premenopausal women, causing iron deficiency anemia. Approximately, 20–50% of the world population are reported to be affected by iron deficiency (Beard and Stoltzfus, 2001; Hider and Kong, 2013). Zinc deficiency is another important nutrition-related health problem, which affects about 25% of population around the world (Prasad, 2012; Raya et al., 2016). Since a small portion of iron or zinc in the diet can be absorbed, the deficiencies are mainly associated with low bioavailability of these elements.
Food fortification refers to the addition of essential trace elements and vitamins to food, so as to improve the nutritional quality of the food and to provide a public health benefit with minimal risk to health [World Health Organization (WHO) and Food and Agriculture Organization (FAO)]. This is a relatively simple and efficient way to prevent mineral deficiency. However, mineral fortification in food has challenges because it often causes unacceptable organoleptic changes during preparation process and storage, has a low bioavailability, and is expensive (Hurrell, 2002). For example, water-soluble ferrous sulfate has a good bioavailability, but induces unacceptable color or flavor changes in food (Hurrell, 1997). In contrast, ferric pyrophosphate (FePP) does not induce serious organoleptic change, although it has low bioavailability coming from poor water solubility (Kloots et al., 2004). In case of Zn fortification, zinc sulfate, zinc oxide (ZnO), zinc acetate, and zinc gluconate have been frequently used (Brown and Wuehler, 2000; Brown et al., 2004). Recently, zinc nanoparticle (NP) formulation, like ZnO-NP, has also attracted much attention to enhance intestinal absorption and bioavailability and to reduce undesirable side effects, taking advantage of high surface area to volume ratio and great reactivity as nano-sized materials (Srinivas et al., 2010; Torabi et al., 2013; Zhao et al., 2014; Faiz et al., 2015; Raya et al., 2016). However, ZnO-NP itself tends to be easily precipitated or aggregated, thereby possessing low stability under physiological condition. Moreover, most forms for Zn fortification have unpleasant zinc flavor when applied in the diet.
A modification of surface characteristics can be a fascinating strategy to overcome such disadvantages in food fortification, for example, by using colloidal techniques, emulsification, surface coating, and etc. (Rossi et al., 2014). In this regard, innovative SunActiveTM compounds (Taiyo Kagaku, Yokkaichi, Japan), in which FePP or ZnO-NPs are emulsified by dextrin, surfactants, and lecithin (Nanbu et al., 2000; Honda et al., 2010), are currently available on the market. These techniques provide high dispersion stability in aqueous media, and thus, can be also used for application in liquid foods or drinks without compromising taste. It was reported that SunActive FeTM, an emulsified FePP with dextrin and glycerides, has a similar bioavailability to ferrous sulfate when added to a wheat-milk infant cereal and a yogurt drink (Fidler et al., 2004). Similarly, SunActive ZnTM, an emulsion of ZnO-NPs with maltodextrin and glycerides, was reported to enhance Zn bioavailability in zinc-deficient rats (Ishihara et al., 2008).
Nanotechnology application to food sectors covers techniques or tools used during cultivation, production, processing, or packaging of the food, as defined by the Nanoforum (Joseph and Morrison, 2006). Surface modification of Fe or Zn moiety by SunActiveTM coating, which induces novel characteristics, can be an important nanotechnology strategy, although an average particle size of SunActiveTM products have been reported to be relatively large (∼300 nm) (Fidler et al., 2004; Sakaguchi et al., 2004; Ishihara et al., 2008; Roe et al., 2009). Nevertheless, SunActiveTM coating can be classified as a nano-coating, considering general definition of nanomaterials as “particles with dimensions less than one micrometer (i.e., <1000 nm) that exhibit unique properties not recognized in micron or larger sized particles,” provided by US Food and Drug Administration (Food and Drug Administration [FDA], 2014).
Relatively little information is currently available on physicochemical characteristics and in vitro and in vivo efficacy as well as toxicity of commercially available, nanotechnology applied mineral fortification products. The aim of the present study was, therefore, to answer the question as to whether they have enhanced efficacy, without affecting potential toxicity. We evaluated the cytotoxicity of SunActive FeTM and SunActive ZnTM in human intestinal cells. Furthermore, the efficacy and bioavailability of SunActiveTM products were assessed in terms of cellular uptake, intestinal transport mechanism, and in vivo biokinetics. The physicochemical properties of SunActiveTM products, such as solubility, dispersibility, and coating stability, were also analyzed to explain their biological behaviors. In all experiments, FePP and ZnO-NPs with similar primary particle size compared to SunActiveTM products were used as counterpart materials for comparative study.
Materials and Methods
Materials
SunActive FeTM (FePP 26.6%, dextrin 54.7%, glycerides 5.3%, lecithin 1.3%, and etc.) and SunActive ZnTM (ZnO 30.0%, maltodextrin 62.1%, glyceride 6.0%, lecithin 1.9%, and etc.) were purchased from Taiyo Kagaku (Yokkaichi, Japan). According to the certificate of analysis provided by the manufacturer, both Sunactive FeTM and Sunactive ZnTM were prepared by materials mixing, pasteurization at 80°C, homogenization, filtration, and spray drying. FePP and ZnO-NPs possessing similar primary particle sizes to corresponding SunActiveTM product were purchased from Dr. Paul Lohmann GmbH KG (Emmerthal, Germany) and American Elements, Co. Ltd (Los Angeles, CA, USA), respectively.
Characterization
Crystalline phase of each sample was analyzed by powder X-ray diffractometry (XRD, Bruker AXS D2 Phaser, Billerica, MA, USA) with Cu Kα radiation (λ = 1.5418 Å). The powder sample mounted on poly (methyl methacrylate) holder was scanned from 5 to 80° (2θ) with 0.1 mm equatorial slit and 1.0 mm air scattering slit. For measurement of hydrodynamic radius of materials, aqueous suspension of each sample (1 mg/ml) was subjected to dynamic light scattering (DLS) instrument (ELSZ-1000, Otsuka, Japan). The particle size and morphology of powder samples were examined with field emission-scanning electron microscope (SEM; Quanta 250 FEG, FEI Company, Hillsboro, OR, USA). Each powder was loaded on carbon tape and coated with Pt/Pd for 60 s before SEM measurement.
Solubility and Hydrodynamic Radii in Cell Culture Medium and Simulated Body Fluids
Dissolution of metal species from each sample was evaluated in cell culture minimum essential medium (MEM; Welgene, Gyeongsangbuk-do, South Korea) and simulated body fluids, such as gastric and intestinal solutions. For preparation of simulated gastric solution, 4 g NaCl (Daejung Chemicals & Metals, Gyeonggi-do, South Korea) and 6.4 g pepsin (Sigma–Aldrich, St. Louis, MO, USA) were dissolved in 1,900 ml of distilled and deionized water (DDW), and then the solution was titrated with 1 M HCl until pH 1.5. In order to prepare simulated intestinal solution, pH of simulated gastric solution was adjusted to 6.8 with 0.95 M NaHCO3 (Sigma–Aldrich) solution, and then, 175 mg of bile salt (Sigma–Aldrich) and 50 mg pancreatin (Sigma–Aldrich) were added.
Each sample with designated mass was dispersed in MEM containing 10% fetal bovine serum (FBS), and simulated gastric or intestinal solution. The final concentrations of suspension were 3.72, 1, 3.24, and 1 mg/ml for SunActive FeTM, FePP, SunActive ZnTM, and ZnO-NPs, respectively, to have an equivalent Zn or Fe amount. Each SunActiveTM suspension was prepared to contain an equivalent Fe or Zn amount compared to its counterpart, FePP or ZnO-NPs. After incubation for 24 h, the supernatants were collected by centrifugation (9,240 × g), pre-treated with ultrapure nitric acid, filtered through syringe filter (0.45 μm, Advantec MFS, Inc., Dublin, CA, USA), and then subjected to inductively coupled plasma-atomic emission spectroscopy (JY2000 Ultrace, HORIBA Jobin Yvon, Longjumeau, France).
In order to investigate dispersibility of samples under physiological conditions, hydrodynamic radii in MEM and simulated body fluids were measured. Each suspension was prepared to have 1 mg/ml concentration, and then subjected to DLS measurement (ELSZ-1000).
Cell Culture
Human intestinal epithelial INT-407 cells were provided by Dr. Tae-Sung Kim at Korea University (Seoul, South Korea) and cultured in MEM (Welgene). Caco-2 cells were purchased from the Korean Cell Line Bank (Seoul, South Korea) and cultured in Dulbecco’s Modified Eagle Medium (DMEM; Welgene), under a humidified atmosphere (5% CO2/95% air) at 37°C. The media were supplemented with 10% heat inactivated FBS, 100 units/ml of penicillin, and 100 μg/ml of streptomycin (Welgene).
Cell Proliferation
The effect of materials on cell proliferation was measured with the water soluble tetrazolium salt-1 (WST-1; Roche, Molecular Biochemicals, Mannheim, Germany). Cells (5 × 103 cells/100 μl) were incubated with SunActiveTM products or equivalent amounts of FePP or ZnO-NPs based on Fe or Zn content for 24 h. Then, 10 μl of WST-1 solution (Roche) was added to each well, and cells were further incubated for 4 h. Absorbance was measured using a plate reader at 440 nm (SpectraMax® M3, Molecular Devices, Sunnyvale, CA, USA). Cells incubated in the absence of materials were used as controls.
Clonogenic Assay
Cells (5 × 102 cells/2 ml) were seeded in 6-well plates and incubated overnight at 37°C under a 5% CO2 atmosphere. The medium in the plates was then replaced with fresh medium containing various concentrations of SunActiveTM materials or equivalent amounts of FePP or ZnO-NPs based on Fe or Zn content, and incubation was continued for 7 days. For colony counting, cells were washed with phosphate buffered saline (PBS) and fixed with 90% crystal violet solution (Sigma–Aldrich) for 1 h. After cell washing with DDW and air-drying, colonies consisted of more than 50 cells were counted. Each experiment was done in triplicate and colony numbers in the absence of test materials were used as controls.
LDH Leakage Assay
The release of lactate dehydrogenase (LDH) was monitored with the CytoTox 96 Non-Radioactive Cytotoxicity assay (Promega, Madison, WI, USA). Cells (2 × 104 cells/1 ml) were exposed to SunActiveTM products or equivalent amounts of FePP or ZnO-NPs based on Fe or Zn content for 24 h. The cells were detached with trypsin- ethylenediaminetetraacetic acid (EDTA) treatment, centrifuged, and aliquots (50 μl) of cell culture medium were collected from each well and placed in new microplates. Then, 50 μl of substrate solution was added to each well and the plates were further incubated for 30 min at room temperature. Finally, after adding 50 μl of stop solution, the absorbance at 490 nm was measured with a microplate reader (SpectraMax® M3, Molecular Devices). Cytotoxicity is expressed relative to the basal LDH release from untreated control cells.
Intracellular ROS Production
Intracellular reactive oxygen species (ROS) levels were monitored using a peroxide-sensitive fluorescent probe, 2′,7′-dichlorofluorescein diacetate (H2DCFDA) (Molecular Probes, Inc., Eugene, OR, USA), according to the manufacturer’s guidelines. Briefly, cells (1 × 104 cells/100 μl) were incubated with SunActiveTM products or equivalent amounts of FePP or ZnO-NPs based on Fe or Zn content for 24 h under standard condition as described above, washed with PBS, and incubated with 5 μM H2DCFDA for 60 min at 37°C. After washing with PBS, DCF fluorescence was immediately measured using a fluorescence microplate reader (SpectraMax® M3, Molecular Devices). Excitation and emission wavelengths were 495 and 518 nm, respectively. Cells not treated with test materials were used as controls.
Cellular Uptake
Cells (1 × 106 cells/2 ml) were incubated overnight at 37°C, and then, the medium was replaced with fresh medium containing SunActiveTM products or an equivalent amount of FePP or ZnO-NPs (50 μg/ml Fe or Zn content). After 6 h of incubation, the cells were washed three times with PBS, treated with 5 mM EDTA in PBS for 40 s to detach adsorbed particles on the cell membrane, and further washed with PBS. The cells were harvested by scraping and re-suspended in DDW. After centrifugation, the cell pellets were digested and Fe or Zn concentrations were determined by ICP-AES (JY2000 Ultrace, HORIBA Jobin Yvon), as described in “ICP-AES analysis” below.
Intestinal Transport Mechanism
3D Cell Culture for Intestinal Epithelial Monolayers
The monoculture system of Caco-2 cells, representing the intestinal epithelium monolayers of tight junctions, was prepared as follow; after coating Transwell® inserts (SPL Life Science, Gyeonggi-do, South Korea) with MatrigelTM matrix (Becton Dickinson, Bedford, MA, USA) for 1 h, supernatants were removed, and then inserts were washed with DMEM. Caco-2 cells (4.5 × 105 cells/well) were grown on upper insert sides, cultured for 21 days, and treated with SunActiveTM products or an equivalent amount of FePP or ZnO-NPs (50 μg/ml Fe or Zn content) for 6 h. The concentrations of transported Fe or Zn in basolateral solutions were determined by ICP-AES (JY2000 Ultrace, HORIBA Jobin Yvon).
3D Cell Culture for FAE Model
Non-adherent human Burkitt’s lymphoma Raji B cells were purchased from the Korean Cell Line Bank and grown in RPMI 1640 medium, supplemented with 10% FBS, 1% non-essential amino acids, 1% L-glutamine, 100 units/ml of penicillin, and 100 μg/ml of streptomycin at 37°C in 5% CO2 atmosphere. An in vitro model of human intestinal follicle-associated epithelium (FAE) was prepared according to the method described by des Rieux et al. (2007) to study material transport by microfold (M) cells; Caco-2 cells (1 × 106 cells/well) were grown on upper insert sides in the same manner as described in Caco-2 monoculture system and incubated for 14 days. Raji B cells (1 × 106 cells/well) in DMEM were then added to basolateral insert compartments, and these co-cultures were maintained for 5 days. Apical medium of cell monolayers was then replaced with a particle suspension containing SunActiveTM products or an equivalent amount of FePP or ZnO-NPs (50 μg/ml Fe or Zn content), and treated for 6 h. The concentrations of transported Fe or Zn in basolateral solutions were determined by ICP-AES (JY2000 Ultrace, HORIBA Jobin Yvon).
Animals
Five-week-old female Sprague Dawley (SD) rats weighing 130–150 g were purchased from Nara Biotech, Co., Ltd (Seoul, South Korea). Animals were housed in plastic laboratory animal cages in a ventilated room maintained at 20 ± 2°C and 60 ± 10% relative humidity under a 12 h light/dark cycle. Water and commercial laboratory complete food were provided ad libitum. Animals were allowed to acclimate to the environment for 7 days before treatment. All animal experiments were performed in compliance with the guideline issued by the Animal and Ethics Review Committee of Seoul Women’s University and Ethics Review Committee of Seoul Women’s University approved the procedures used in this study (IACUC-2014A-3).
Biokinetics and Tissue Distribution
Six female rats per group were administered a single dose of SunActive FeTM (1000 mg/kg) or SunActive ZnTM (500 mg/kg) and an equivalent Fe or Zn amount of FePP (320 mg/kg) or ZnO-NPs (156.25 mg/kg) by oral gavage; controls (n = 6) received an equivalent volume of distilled water (DW). To determine plasma Fe or Zn concentrations, blood samples were collected via tail vein at 0, 0.25, 0.5, 1, 2, 4, 6, 10, and 24 h. Blood samples were centrifuged at 3,000 × g for 15 min at 4°C to obtain plasma. Plasma Fe or Zn concentration-time profiles were determined by ICP-AES (JY2000 Ultrace, HORIBA Jobin Yvon). The following biokinetic parameters were calculated using Kinetica version 4.4 (Thermo Fisher Scientific, Waltham, MA, USA): maximum concentration (Cmax), time to maximum concentration (Tmax), area under the plasma concentration-time curve (AUC), half-life (T1/2), and mean residence time (MRT).
For the tissue distribution study, the same doses were orally administered. Tissue samples of kidneys, liver, lungs, and spleen were collected after CO2 euthanasia at 24 h post-administration. Fe or Zn biodistribution was determined by ICP-AES (JY2000 Ultrace, HORIBA Jobin Yvon).
ICP-AES Analysis
Biological samples were pre-digested with 10 ml of ultrapure nitric acid (HNO3) overnight, heated to ∼160°C, and 1 ml of hydrogen peroxide (H2O2) solution was added. The mixtures were heated until the samples were colorless and clear. After dilution with 3 ml of DDW, total Fe or Zn concentrations were determined by ICP-AES (JY2000 Ultrace, HORIBA Jobin Yvon).
Statistical Analysis
Results were expressed as means ± standard deviations. Experimental values were compared with corresponding untreated control values. One-way analysis of variance (ANOVA) with Tukey’s test in SAS Version 9.4 (SAS Institute, Inc., Cary, NC, USA) was used to determine the significances of intergroup differences. Statistical significance was accepted for p values of less than 0.05.
Results
Physicochemical Properties
Physicochemical properties of SunActive FeTM and SunActive ZnTM are displayed in Figures 1, 2, respectively. XRD results show that both SunActive FeTM and FePP had amorphous phase (Figure 1A). Hydrodynamic radii, representing the secondary particle sizes in DDW suspension, were 240 and 410 nm for SunActive FeTM and FePP (Figure 1B), respectively. The SEM images in low magnification exhibited sphere-like morphology for SunActive FeTM, which seems to be attributed to the organic moiety, such as dextrin and glycerides, while FePP showed irregular aggregation (Figure 1C). Magnified images taken at the surface of materials showed comparable primary particle size of ∼50 nm for both SunActive FeTM and FePP (insets in Figure 1C). On the other hand, both SunActive ZnTM and ZnO-NPs had the typical Wurtzite crystal phase [Figure 2A, Joint Committee on Powder Diffraction Standards (JCPDS) No. 36-1451], indicating the existence of ZnO-NPs in SunActive ZnTM. Hydrodynamic radii of SunActive ZnTM and ZnO-NPs were 350 and 750 nm, respectively, indicating higher dispersion degree of SunActive ZnTM (Figure 2B). Similar to SunActive FeTM, SunActive ZnTM showed spherical morphology in low magnification, while ZnO-NPs had irregular and agglomerated shape (Figure 2C). Magnified SEM images at the surface of materials exhibited fairly similar primary particle size of 112 ± 42 and 103 ± 20 nm for SunActive ZnTM and ZnO-NPs, respectively (insets in Figure 2C).
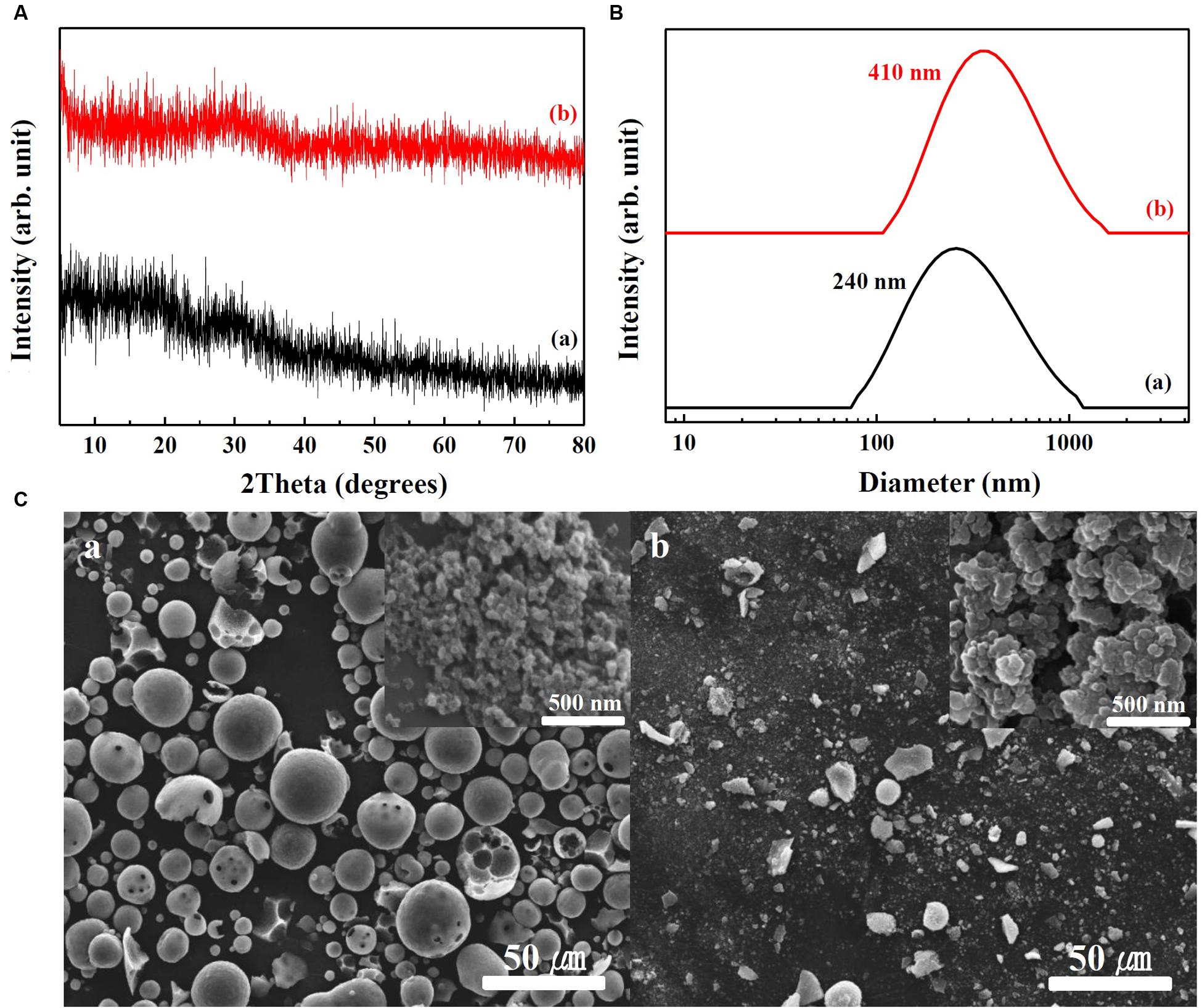
FIGURE 1. (A) XRD patterns, (B) hydrodynamic radii measured by DLS, and (C) SEM images of (a) SunActive FeTM and (b) FePP. Insets in (C-a,b) are magnified images at the surface of materials.
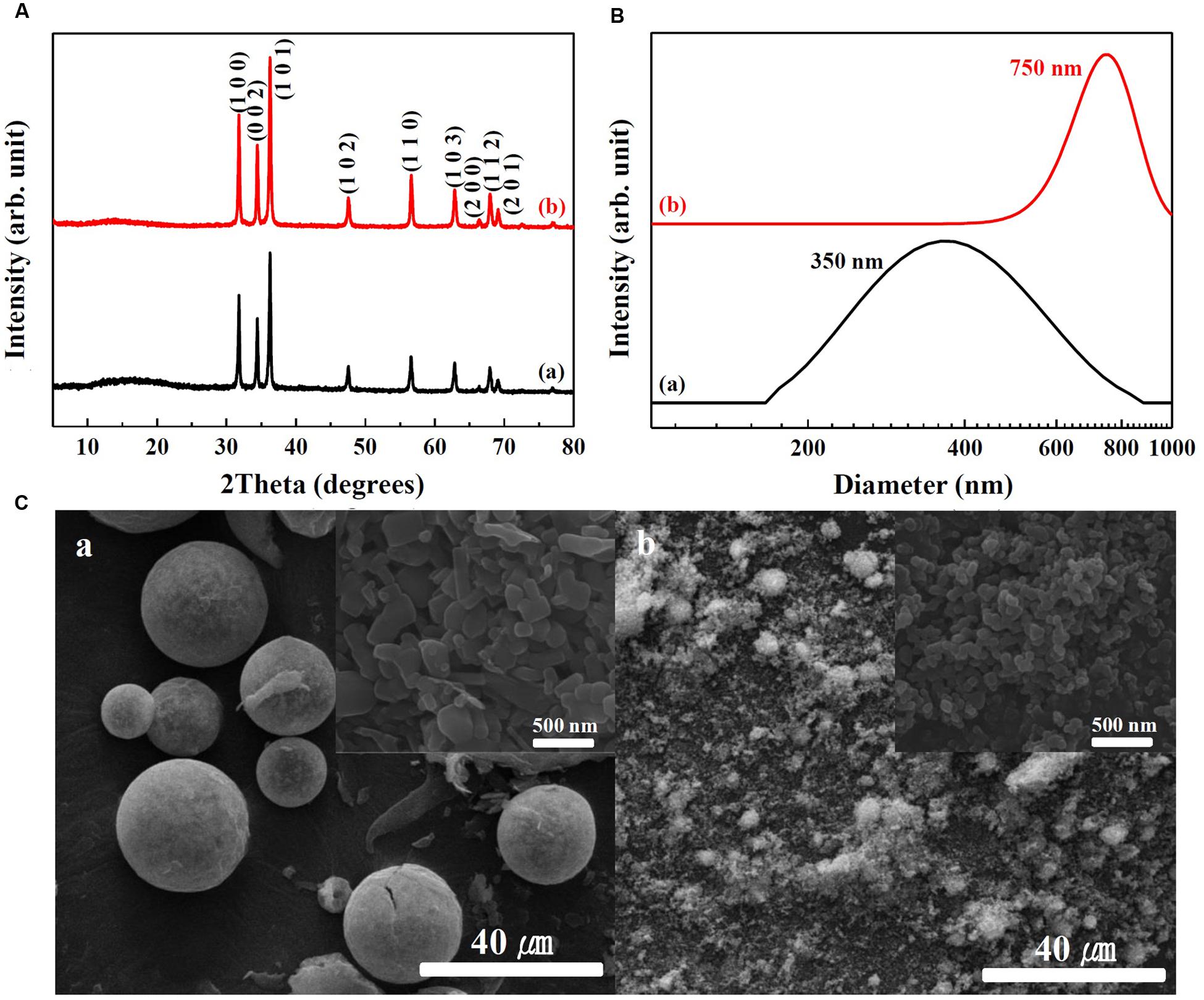
FIGURE 2. (A) XRD patterns, (B) hydrodynamic radii measured by DLS, and (C) SEM images of (a) SunActive ZnTM and (b) ZnO-NPs. Insets in (C-a,b) are magnified images at the surface of materials.
Solubility and Hydrodynamic Radii in Cell Culture Medium and Simulated Body Fluids
Dissolution properties of Fe or Zn from SunActive FeTM or SunActive ZnTM in MEM and simulated gastric or intestinal solutions were compared to those of their counterpart materials (FePP or ZnO-NPs) and summarized in Table 1. There were no significant differences in Fe dissolution between SunActive FeTM and FePP in MEM, although slightly high solubility was found for FePP in simulated gastric fluid. On the other hand, Fe solubility from SunActive FeTM significantly and remarkably increased compared to FePP under intestinal condition. Similar to Fe samples, Zn dissolution from SunActive ZnTM was similar to that from ZnO-NPs in both MEM and gastric solution. Whereas, Zn solubility was slightly higher for ZnO-NPs than SunActive ZnTM in intestinal solution, and both Zn samples showed the highest solubility in gastric solution. In other words, solubilities between SunActiveTM products and their counterparts were similar in MEM, while statistically different solubility was found in simulated intestinal solution.
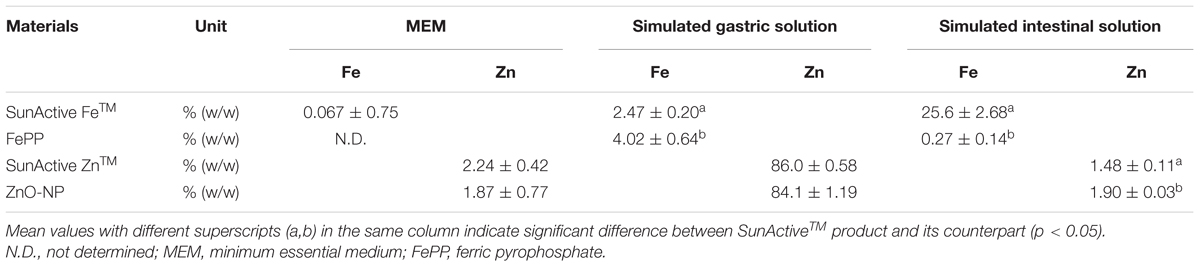
TABLE 1. Dissolution properties of Fe or Zn from SunActiveTM products and their counterpart materials in cell culture medium (MEM) and simulated body fluids.
Hydrodynamic radii of SunActiveTM products and their counterpart materials in MEM and simulated gastric and intestinal solutions were measured by DLS and displayed in Figure 3. The hydrodynamic radii were found to be highly dependent on the type of medium. The hydrodynamic radii of SunActive FeTM, FePP, and SunActive ZnTM in MEM were 340, 460, and 300 nm, respectively (Figure 3A), which were comparable to the values obtained in DDW (Figures 1B, 2B). The hydrodynamic radius of ZnO-NPs in MEM considerably reduced compared to that in DDW, showing 380 nm. Under simulated gastric condition, the hydrodynamic radii of all materials significantly increased and more prominent increase in size was found for Fe samples than Zn samples (Figure 3B). In simulated intestinal fluid (Figure 3C), both SunActive FeTM and FePP had increased hydrodynamic radii and reduced homogeneity compared to those in MEM (Figure 3A). Furthermore, SunActive FeTM showed split distribution in the range from 150 to 1000 nm. In case of SunActive ZnTM and ZnO-NPs, the single distribution patterns and similar hydrodynamic radii were found in intestinal fluid, compared to those in DDW.
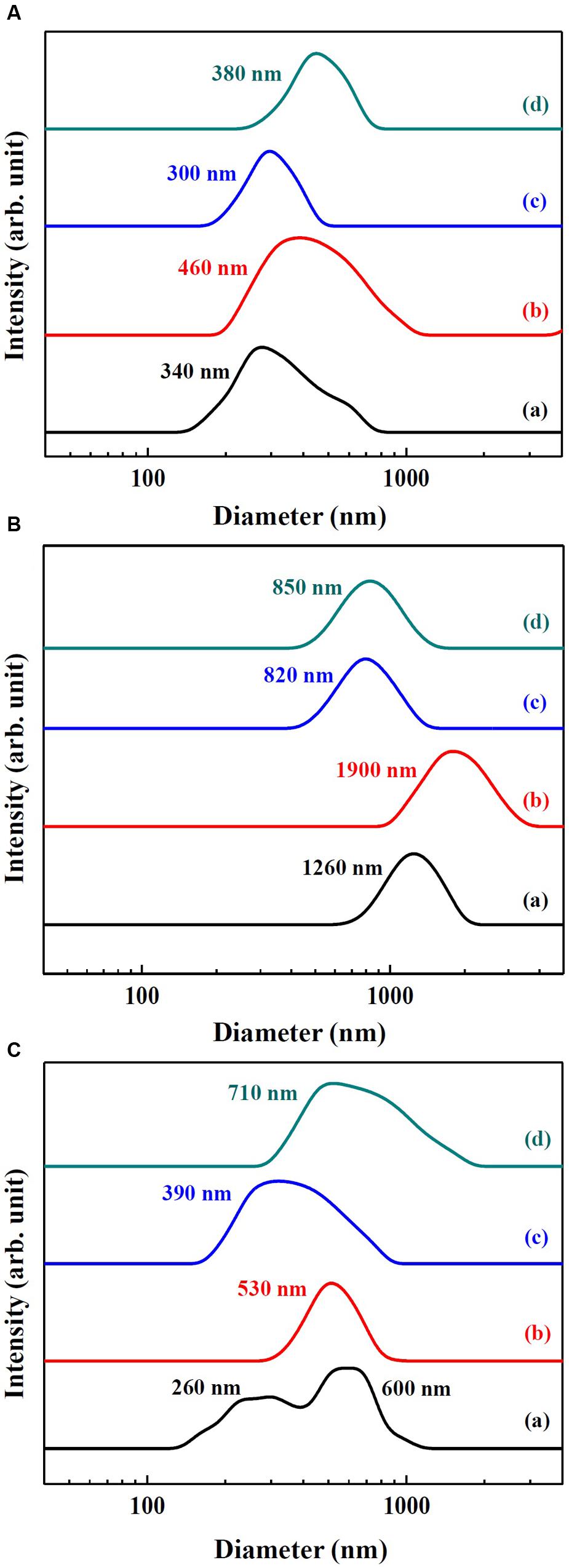
FIGURE 3. Hydrodynamic radius of (a) SunActive FeTM, (b) FePP, (c) SunActive ZnTM, and (d) ZnO-NPs in (A) MEM, (B) simulated gastric solution, and (C) simulated intestinal solution.
Short- and Long-Term Cytotoxicity
Cytotoxicity of SunActive FeTM or SunActive ZnTM was evaluated in human intestinal INT-407 cells in terms of inhibition of cell proliferation and colony-forming ability, and compared to that of each counterpart material. Figure 4A demonstrates that both SunActive FeTM and FePP did not affect cell proliferation and viability up to the highest concentration tested after exposure for 24 h. On the other hand, SunActive ZnTM more remarkably inhibited cell proliferation than ZnO-NPs (Figure 4B). It is worth noting that all cytotoxicity tests were performed based on equivalent Fe or Zn amounts, and the counterpart materials had similar primary particle size to corresponding SunActiveTM products. It was determined that Fe and Zn contents in SunActive FeTM and SunActive ZnTM are 8 and 25%, respectively, and therefore, the highest concentrations as total compounds for the former and the latter were 1000 μg/ml (equivalent to 80 μg/ml Fe content) and 800 μg/ml (equivalent to 200 μg/ml Zn content), respectively.
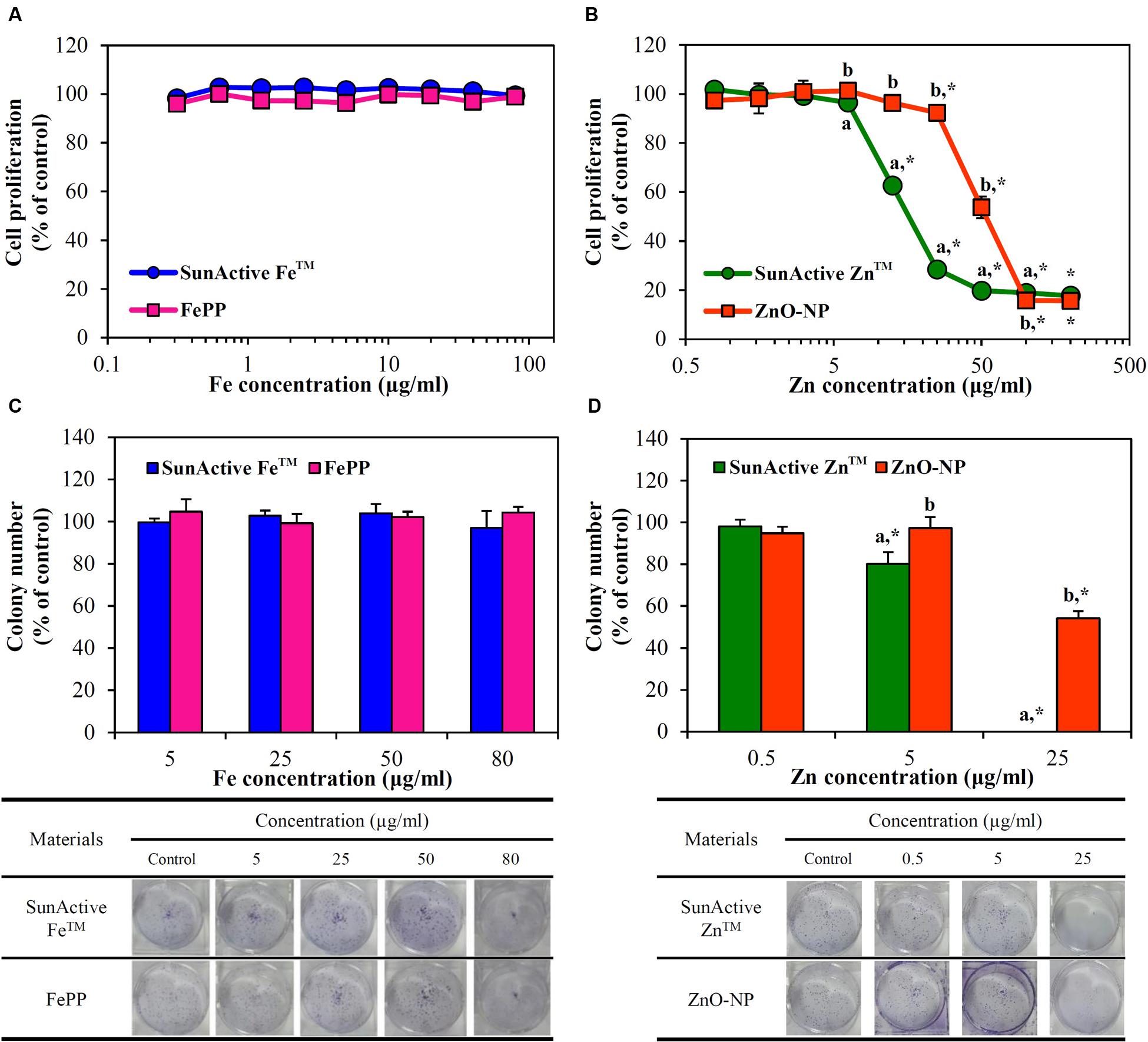
FIGURE 4. Short-term effect of (A) SunActive FeTM and (B) SunActive ZnTM on cell proliferation of INT-407 cells after treatment for 24 h, measured by WST-1 assay. Long-term effect of (C) SunActive FeTM and (D) SunActive ZnTM on colony-forming ability of INT-407 cells incubated for 7 days. FePP and ZnO-NPs were used as counterpart materials for SunActive FeTM and SunActive ZnTM, respectively. Mean values with different letters (a,b) in the same concentrations indicate significant differences between SunActiveTM product and its counterpart material (p < 0.05). ∗ Indicates significant differences from the untreated controls (p < 0.05).
Long-term cytotoxicity was also evaluated by clonogenic assay after treatment for 7 days, which investigates the ability of colony formation of cells. Interestingly, SunActive FeTM and FePP did not affect colony-forming ability at all (Figure 4C). Whereas, SunActive ZnTM remarkably inhibited colony formation at the highest concentration tested (25 μg/ml), as compared to ZnO-NPs (Figure 4D). The same tendency was observed in human intestinal Caco-2 cells exposed to SunActiveTM products (data not shown).
Membrane Damage and ROS Generation
Membrane damage caused by SunActiveTM products was evaluated using LDH leakage assay, which measures released amount of cytosolic LDH into extracellular medium. The same tendency obtained from WST-1 assay was found; neither SunActive FeTM nor FePP did induce membrane damage (Figure 5A), while significantly high LDH leakage from SunActive ZnTM-exposed cells compared to ZnO-NP exposure was found (Figure 5B).
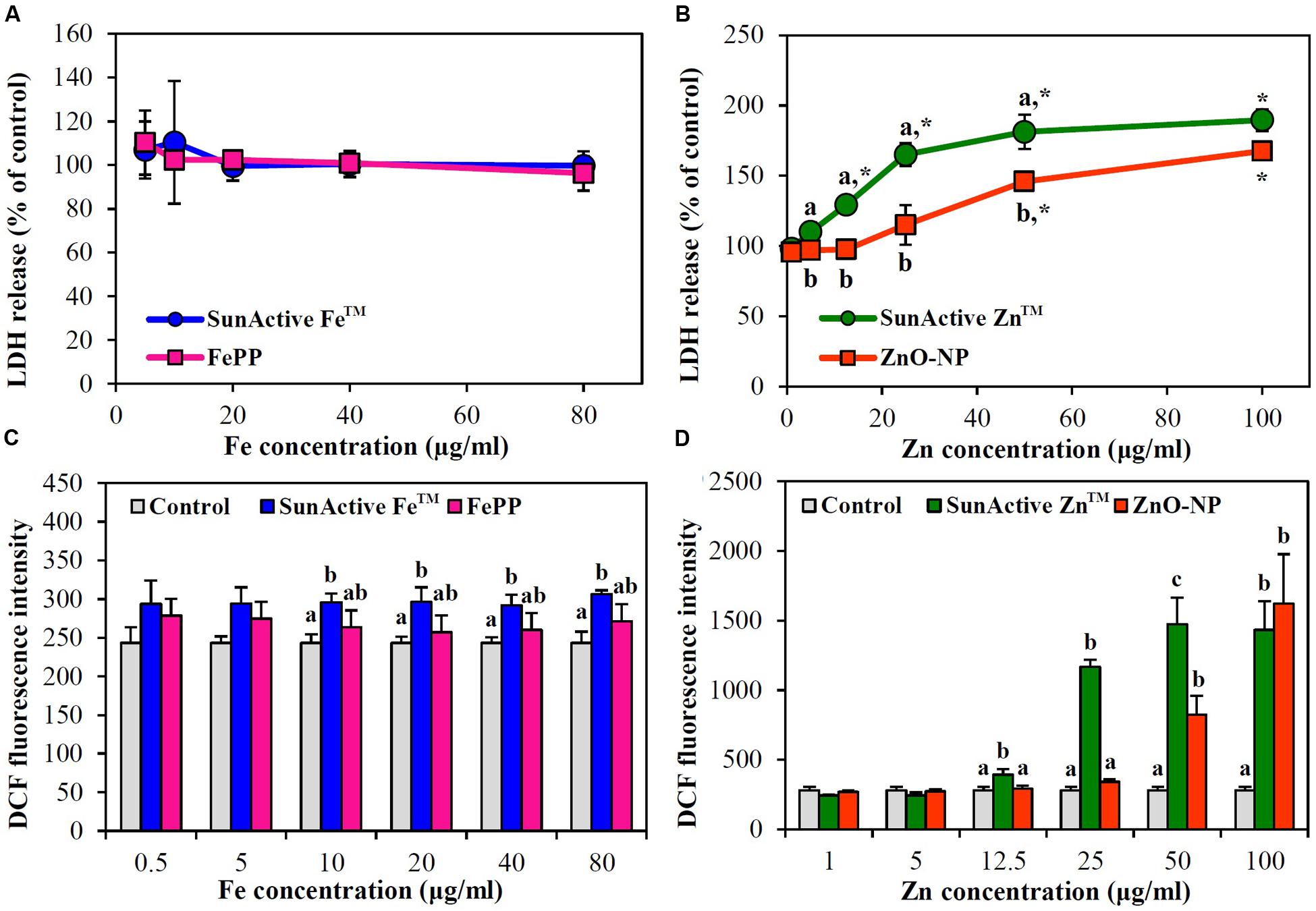
FIGURE 5. Effect of (A,C) SunActive FeTM and (B,D) SunActive ZnTM on (A,B) LDH release and (C,D) ROS generation in INT-407 cells after treatment for 24 h. FePP and ZnO-NPs were used as counterpart materials for SunActive FeTM and SunActive ZnTM, respectively. Mean values with different letter (a,b,c) in the same concentrations indicate significant differences among untreated control, SunActiveTM product, and its counterpart material (p < 0.05). ∗ Indicates significant differences from the untreated controls (p < 0.05).
When intracellular ROS generation was measured with H2DCFDA, slightly but significantly increased ROS levels were detected by SunActive FeTM treatment, whereas, no elevated ROS levels were found in FePP-exposed cells (Figure 5C). Meanwhile, both SunActive ZnTM and ZnO-NPs significantly induced ROS at Zn concentrations of more than 50 μg/ml, but much high ROS levels were induced by SunActive ZnTM at concentration range from 12.5 to 50 μg/ml (Figure 5D). The same tendency was found in Caco-2 cells (data not shown).
Cellular Uptake
Cellular uptake efficacy of SunActiveTM products was evaluated in both INT-407 cells and Caco-2 cells by measuring intracellular Fe or Zn amount by ICP-AES, and compared with that of each counterpart material. Figure 6 shows that both SunActive FeTM and SunActive ZnTM were more efficiently taken up by cells than FePP and ZnO-NPs, respectively. Significantly, high uptake of both materials was found in INT-407 cells (Figures 6C,D) than in Caco-2 cells (Figures 6A,B).
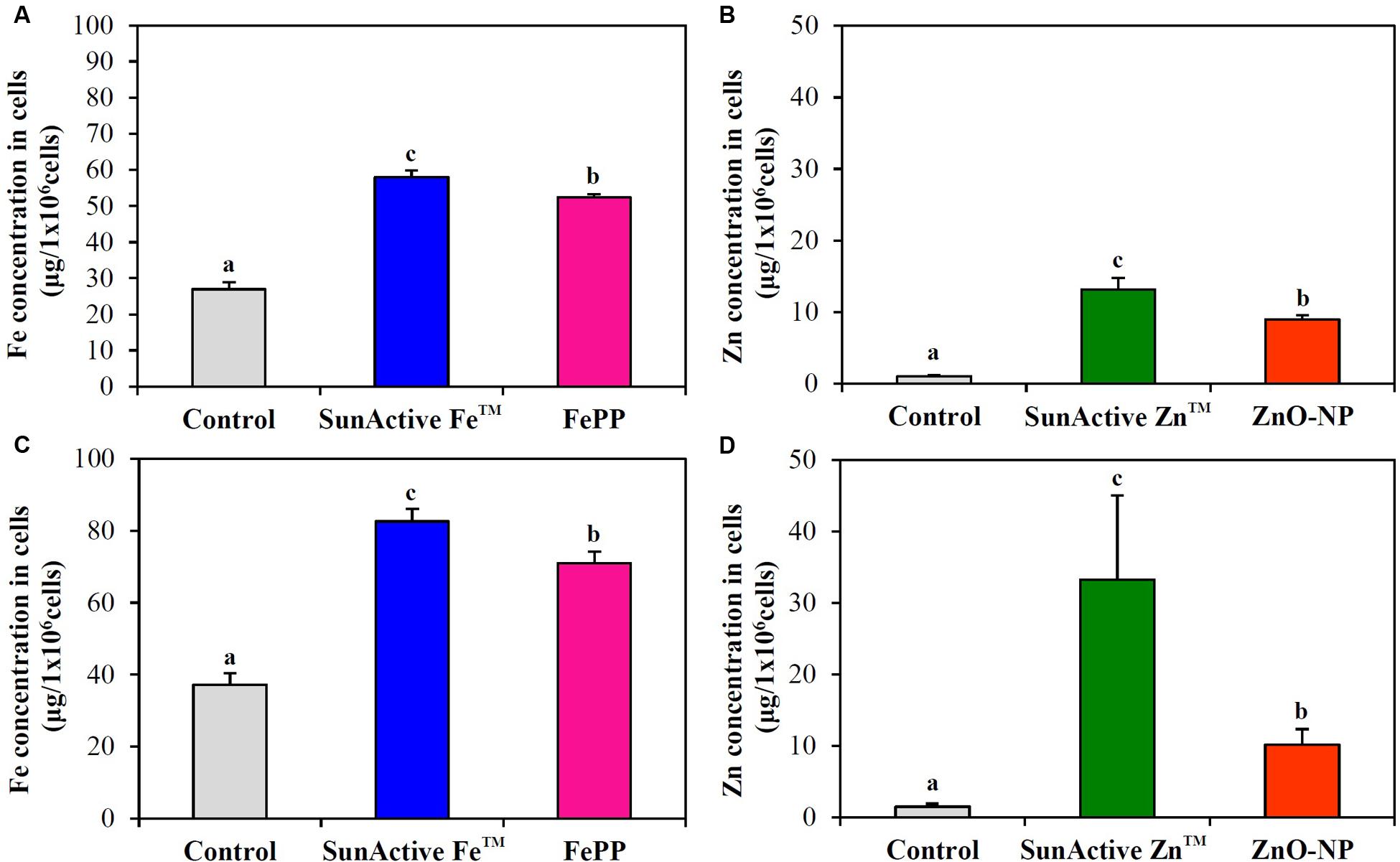
FIGURE 6. Cellular uptake of (A,C) SunActive FeTM and (B,D) SunActive ZnTM in (A,B) Caco-2 cells and in (C,D) INT-407 cells after treatment for 6 h, measured by ICP-AES. FePP and ZnO-NPs were used as counterpart materials for SunActive FeTM and SunActive ZnTM, respectively. Mean values with different letters (a,b,c) in the same figure indicate significant differences among untreated control, SunActiveTM product, and its counterpart material (p < 0.05).
Intestinal Transport Mechanism
Intracellular transport pathway was assessed using in vitro models of human FAE and Caco-2 monolayers, which represent M cells in Peyer’s Patches and intestinal tight junctions, respectively. The results demonstrate that both SunActiveTM materials were primarily transported by M cells, but their significantly elevated transports through Caco-2 monolayers were also found (Figures 7A,B). FePP and ZnO-NPs were also found to be translocated across both M cells and Caco-2 tight junctions. Total intestinal transport amounts of SunActiveTM materials through both M cells and Caco-2 monolayers were pooled and presented in Figures 7C,D. Significantly higher translocation of SunActive FeTM than FePP was found, contrary to SunActive ZnTM showing similar total transport amount compared to ZnO-NPs.
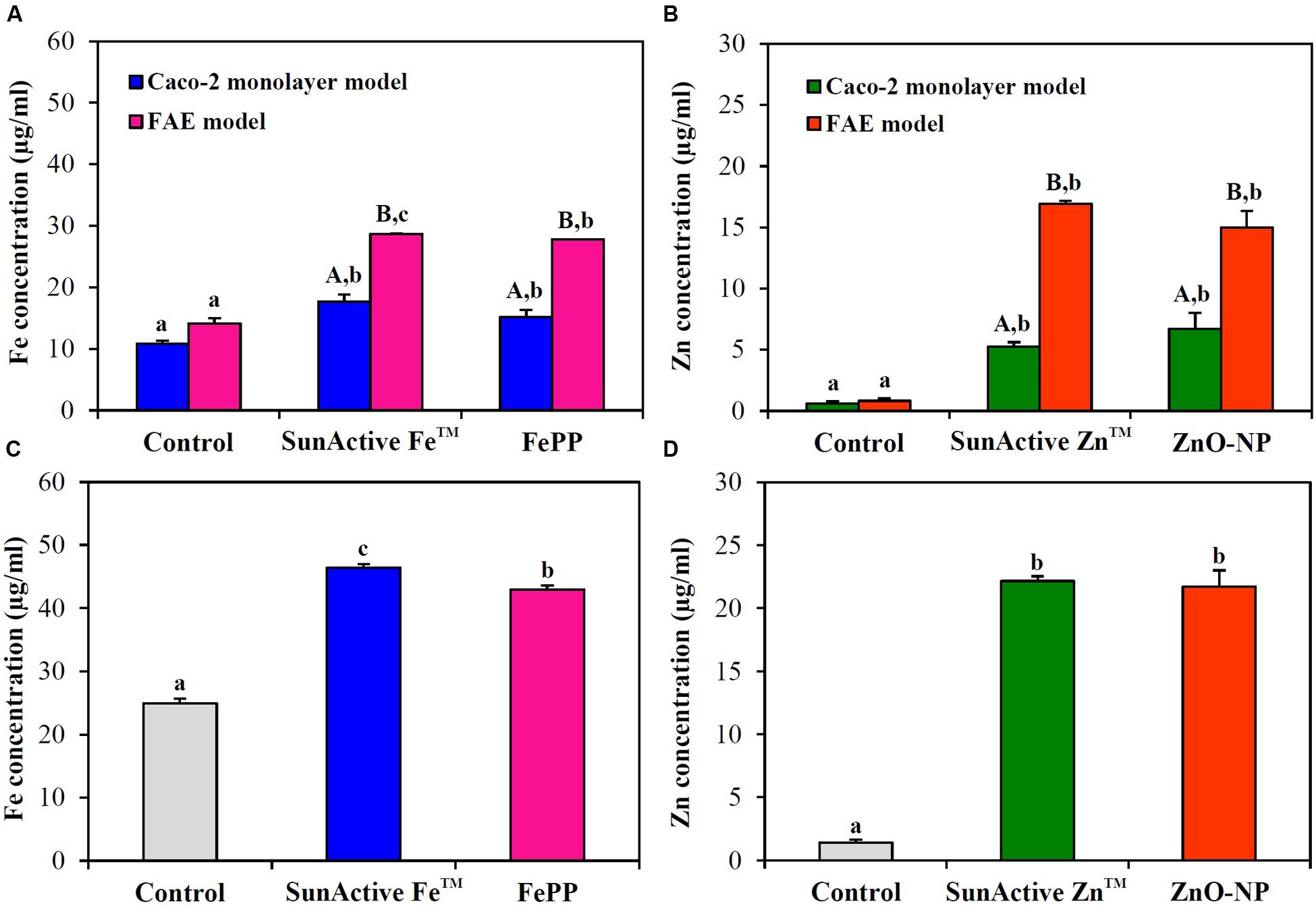
FIGURE 7. Intestinal transport mechanism of (A) SunActive FeTM and (B) SunActive ZnTM evaluated using in vitro models of Caco-2 monolayers and human FAE after treatment for 6 h. Combined total intestinal transport amount of (C) SunActive FeTM and (D) SunActive ZnTM through both Caco-2 monolayers and the FAE model. FePP and ZnO-NPs were used as counterpart materials for SunActive FeTM and SunActive ZnTM, respectively. Mean values with different letters in minuscule (a,b,c) in the same figure indicate significant differences among untreated control, SunActiveTM product, and its counterpart material (p < 0.05). Mean values with different letters in majuscule (A,B) in the same figure indicate significant differences between Caco-2 monolayers and the FAE model (p < 0.05).
Biokinetics and Tissue Distribution
Plasma concentration versus time curves after a single-dose oral administration to rats demonstrate that SunActive FeTM entered the bloodstream more rapidly and greatly than FePP, showing peak concentration at 0.5 h for the former versus 2 h for the latter (Figure 8A). When biokinetic parameters were calculated (Table 2), all kinetic parameters between SunActive FeTM and FePP were significantly different, showing high Cmax, AUC, T1/2, and MRT values, but low Tmax values for SunActive FeTM. On the other hand, almost similar plasma concentration-time profiles between SunActive ZnTM and ZnO-NPs were found (Figure 8B). Biokinetic parameters reveal only slightly high T1/2 values for SunActive ZnTM compared to ZnO-NPs, but there were no statistical significances in Cmax, Tmax, AUC, and MRT values between two materials (p > 0.05). When oral absorption efficiency was calculated based on AUC values (Table 2), absorption of SunActive FeTM (21.1 ± 4.6%) significantly increased, in comparison with that of FePP (9.7 ± 2.4%), while SunActive ZnTM and ZnO-NPs were determined to have 9.1 ± 0.4 and 8.9 ± 1.0% of oral absorptions, respectively, without statistical difference (p > 0.05).
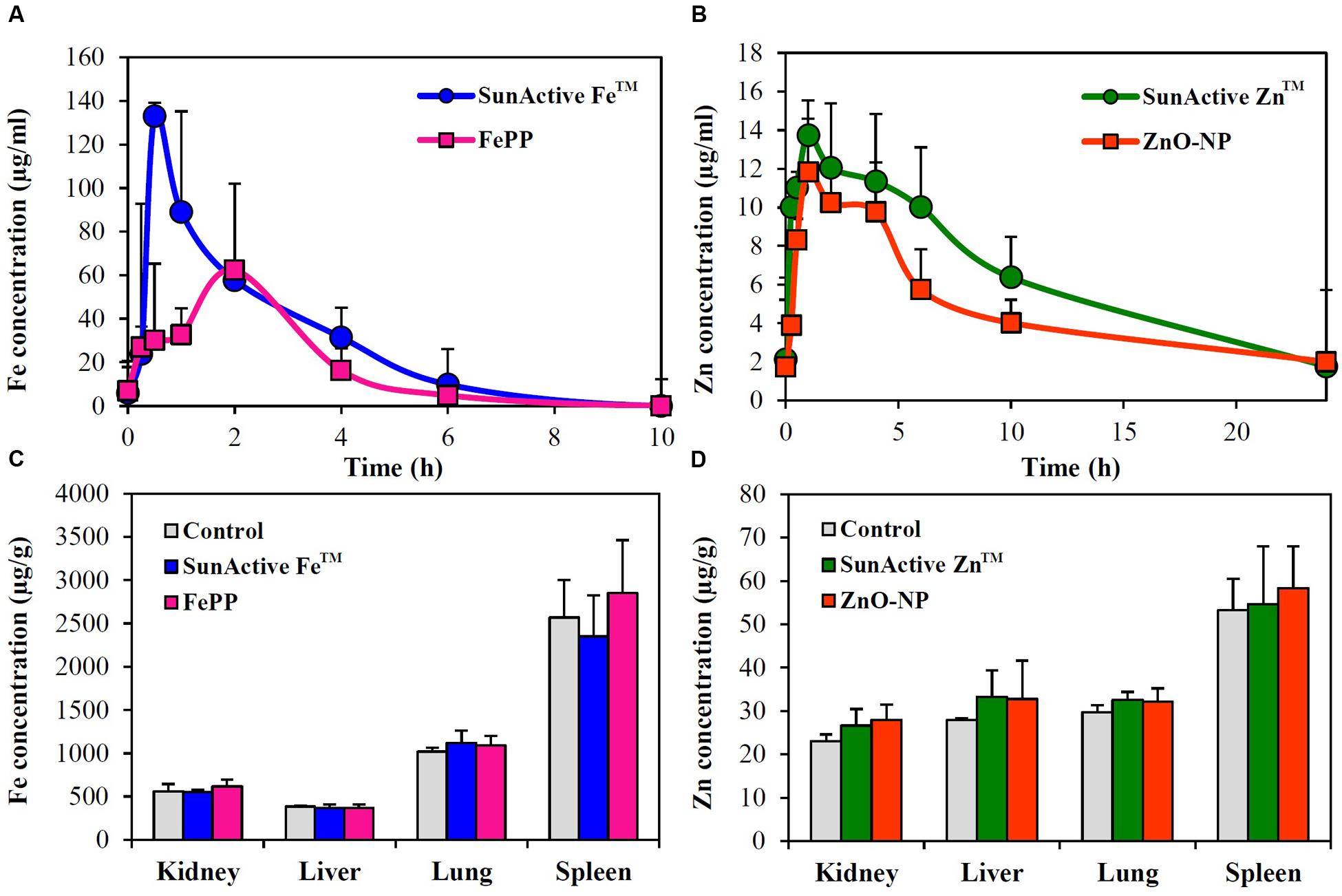
FIGURE 8. Plasma concentration-time profiles of (A) SunActive FeTM (1000 mg/kg) and (B) SunActive ZnTM (500 mg/kg) after a single-dose oral administration to rats. An equivalent amount of FePP (320 mg/kg) or ZnO-NPs (156.25 mg/kg) based on Fe or Zn content was also orally administered for comparative study. Tissue distribution patterns of (C) SunActive FeTM (1000 mg/kg) and (D) SunActive ZnTM (500 mg/kg) after a single-dose oral administration to rats at 24 h post-administration.
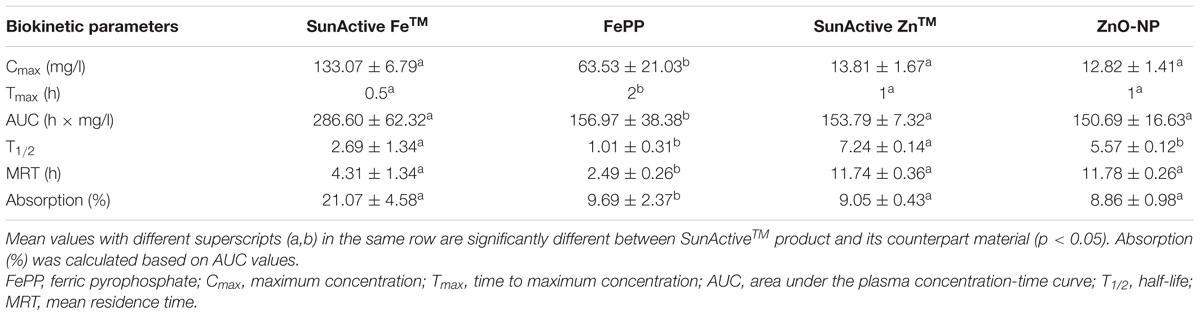
TABLE 2. Biokinetic parameters and oral absorption of SunActiveTM products and their counterpart materials after oral administration of a single-dose to rats.
Tissue distributions of SunActiveTM products and their counterpart materials were also investigated after a single-dose oral administration to rats. Figures 8C,D show no significantly increased Fe or Zn levels in all organs analyzed compared to untreated control groups (p > 0.05).
Discussion
The aim of this study was to investigate the efficacy and potential toxicity of commercially available, surface modified Fe or Zn fortification, coated with low-molecular-carbohydrates (dextrin for SunActive FeTM and maltodextrin for SunActive ZnTM) and glycerides, which enables to have stable and high dispersibility in water, thereby expecting high bioavailability. Hydrodynamic radii obtained from DLS measurement in various media (DDW, MEM, and simulated gastric and intestinal solutions) clearly showed that SunActiveTM coating enhanced dipersibility of pristine materials, FePP and ZnO-NPs (Figures 1B, 2B, 3A–C). However, SunActiveTM products measured from SEM showed micron sizes (Figures 1C, 2C), which seems to be attributed to the agglomeration among coating moieties, such as dextrin (for SunActive FeTM) and maltodextrin (SunActive ZnTM), during drying process for SEM measurement. The sizes of SunActive FeTM and SunActive ZnTM can be, therefore, considered to be 240 and 350 nm, respectively, measured by DLS, rather than particle sizes from SEM images. Furthermore, SunActiveTM coating ensured long-term dispersion stability, while remarkable sedimentation of non-coated FePP and ZnO-NPs was observed (Supplementary Figure 1). It should be noted that the counterparts, FePP and ZnO-NPs were chosen to have the same primary particle size compared to SunActiveTM products. Thus, the reduced hydrodynamic radii of SunActiveTM products compared to counterpart materials are attributed to the organic coating, which reduces particle aggregation. It was also found that the hydrodynamic radius and dispersibility were highly dependent on dispersing medium. For instance, SunActive FeTM and FePP showed significant agglomeration in simulated gastric (Figure 3B) and intestinal fluids (Figure 3C) compared to DDW (Figure 1A) and MEM (Figure 3A). The degree of agglomeration was relatively higher for FePP than for SunActive FeTM. Agglomeration was also strongly related to dispersion stability; FePP showed clear sedimentation in DDW and simulated intestinal solution after 48 h of dispersion, while SunActive FeTM showed retarded sedimentation under all conditions (Supplementary Figure 1). On the other hand, DLS results revealed that SunActive ZnTM showed less agglomeration than ZnO-NPs in MEM (Figure 3A) and simulated gastric and intestinal fluids (Figures 3B,C). This was in good agreement with sedimentation result (Supplementary Figure 1), which was attributed to the agglomeration of particles in each medium.
The cytotoxicity of SunActive FeTM was determined to be not affected by surface coating in terms of short- and long-term inhibition of cell proliferation (Figures 4A,C) as well as membrane damage (Figure 5A). Interestingly, SunActive FeTM did not inhibit colony-forming ability even after exposure for 7 days, implying its low cytotoxicity. It is worth noting that all biological experiments were performed based on the same Fe or Zn content, in order to compare in vivo oral Fe or Zn absorption efficiency of SunActiveTM products with that of each counterpart material. Thus, the highest concentrations tested as total compounds for cytotoxicity experiments were 1000 and 320 μg/ml for SunActive FeTM (8% Fe) and FePP (25% Fe), respectively. Slightly increased intracellular ROS generation was detected in cells exposed to SunActive FeTM compared to FePP (Figure 5C). The slight difference in ROS generation may be related to different particle size in aqueous suspension. Indeed, hydrodynamic radius of SunActive FeTM was smaller than FePP in MEM (Figure 3A-a,b). It is still controversial, but generally accepted that smaller sized NPs induce higher ROS generation (Manke et al., 2013). Nevertheless, overall toxicity results on SunActive FeTM suggest its low cytotoxicity potential, indicating that low cytotoxic nature of FePP was not influenced by surface coating. On the other hand, SunActive ZnTM exhibited higher inhibition of cell proliferation and colony formation (Figures 4B,D), induction of membrane damage (Figure 5B), and ROS generation (Figure 5D) than ZnO-NPs, when cytotoxicity was expressed based on Zn contents. The IC50 values obtained by WST-1 assay were 18.11 and 61.99 μg/ml for SunActive ZnTM and ZnO-NPs as Zn contents, corresponding to 72.45 (25% Zn) and 77.48 μg/ml (80% Zn) as total compounds for the former and the latter, respectively. Hence, the cytotoxicity of SunActive ZnTM is much higher than ZnO-NPs based on Zn content, but similar toxicity between two materials was found when the toxicity was expressed as total compounds. Meanwhile, another critical factor affecting the cytotoxicity is the fate of materials under cell culture condition. It has been well-reported that Zn2+ ions cause high toxicity (Song et al., 2010; Yin et al., 2012; Wang et al., 2014; Jo et al., 2016). Furthermore, Zn2+ ion release from ZnO-NPs was reported to facilitate ROS generation and LDH release (Song et al., 2010). Indeed, the IC50 values for ZnCl2 as Zn2+ ions were 9.54 μg/ml based on Zn content and 19.89 μg/ml as a total compound (data not shown), which are different from those for SunActive ZnTM or ZnO-NPs. In the present study, SunActive ZnTM was more toxic than ZnO-NPs based on Zn content, in spite of similar Zn dissolution property between two materials in MEM (Table 1). Moreover, the solubility of both materials was ∼2% in MEM, indicating their primarily particulate fate. Hence, it is strongly likely that high toxicity of SunActive ZnTM can be related to its small hydrodynamic radius and high dispersibility (Figure 3A), but not to their solubility or fate.
When intracellular uptake efficiency was evaluated by measuring total Fe or Zn concentration using ICP-AES, uptake efficiency in both INT-407 and Caco-2 cells was significantly enhanced by SunActiveTM coatings, in comparison with their counterpart materials (Figure 6). Enhanced cellular uptake of SunActiveTM products might be attributed to their reduced hydrodynamic radii compared to counterpart materials (Figure 3A), which probably causes high toxicity as well (Figures 4, 5). However, this type of experiment reflects only the amount taken up by cells, which can be different from absorption efficacy through the intestinal epithelium after oral administration. Thus, intestinal transport efficacy and mechanism were also assessed using in vitro 3D culture systems, human FAE model and Caco-2 monolayers. The FAE model represents M cells found in the Peyer’s patches, which involve in the intestinal transport of a variety of materials and immune system (Kernéis et al., 1997; Neutra et al., 2001; Jang et al., 2004). Caco-2 monolayers were often used to represent a dense network of intestinal tight junctions (Ma et al., 2005; Hubatsch et al., 2007). The results show that both SunActive FeTM and SunActive ZnTM were primarily transported by M cells, but slight transports by Caco-2 monolayers were also found (Figure 7). FePP and ZnO-NPs were also found to be translocated in the same manner. In other words, intestinal transport mechanism was not affected by surface coating. Since in vivo intestinal transport occurs simultaneously through M cells and tight junctions, it is more reasonable that combined translocation amounts obtained by two different models are compared. As shown in Figures 7C,D, significantly high intestinal transport of SunActive FeTM compared to FePP suggests that surface coating can contribute to enhance intestinal transport of mineral fortification. However, increased translocation of SunActive ZnTM compared to ZnO-NPs was not found. These results indicate that surface modification does not always result in transport efficiency, and that the intestinal transport of materials is governed by various factors, such as particle size, dispersibility, and coating stability.
Plasma concentration-time profiles after a single-dose oral administration to rats were highly consistent with the intestinal transport results. SunActive FeTM remarkably increased oral absorption rate and absorption efficiency, as shown in rapid Tmax and high AUC values (Figure 8A and Table 2). Oral absorption efficacy of SunActive FeTM significantly increased by about 2.2 fold compared to its counterpart material, FePP. This result indicates that surface coating by dextrin and glycerides can enhance oral Fe bioavailability. This seems to be strongly associated with hydrodynamic radius, dispersibility, and solubility. SunActive FeTM had smaller hydrodynamic radius than FePP in all media tested (Figure 3C-a,b), showing better dispersibility than FePP (Supplementary Figure 1), which is likely to facilitate oral absorption of Fe moiety. Furthermore, intestinal solubility of SunActive FeTM dramatically enhanced compared to FePP, and thus, total gastrointestinal solubility of the former significantly increased in comparison with the latter, which surely affects Fe bioavailability (Zariwala et al., 2013). Meanwhile, almost similar biokinetic behaviors between SunActive ZnTM and ZnO-NPs were found, as observed by similar plasma concentration versus time curves and biokinetic parameters (Figure 8B and Table 2). Moreover, oral absorption of SunActive ZnTM did not significantly increase (Table 2), although reduced hydrodynamic radius and enhanced dispersibility compared to ZnO-NPs were observed (Figure 3 and Supplementary Figure 1). It should be noted that major surface coating materials for SunActive FeTM and SunActive ZnTM are dextrin (54.7%) and maltodextrin (62.1%), respectively, and maltodextrin is more easily digestible than dextrin and rapidly absorbed as glucose (Chronakis, 1998). It is, therefore, probable that SunActive ZnTM is easily degradable after oral administration, resulting in its similar oral absorption compared to ZnO-NPs. Contrary to SunActive ZnTM, SunActive FeTM with dextrin coating tends to be less degraded under gastrointestinal condition, which can contribute to enhance Fe bioavailability. It is interesting to note that SunActive ZnTM had similar hydrodynamic radius compared to ZnO-NPs in simulated gastric fluid, while significantly reduced hydrodynamic radius was observed for SunActive FeTM compared to FePP (Figure 3B). Moreover, no significant difference in total gastrointestinal solubility between SunActive ZnTM and ZnO-NPs was found (Table 1), which may explain similar oral Zn absorption between two materials. On the other hand, much higher total gastrointestinal solubility of SunActive ZnTM than SunActive FeTM (Table 1) might also support coating stability of the latter. It is worth noting that biokinetic study was performed to have an equivalent amount of Fe or Zn, based on Fe or Zn contents in all tested materials. Taken together, it is likely that oral absorption of Fe or Zn fortification can be enhanced by surface coating to some extent, but highly dependent on the nature of fortified minerals and surface coating materials. High oral absorption efficacy of SunActive FeTM is likely to be attributed to its increased intestinal transport through M cells and Caco-2 monolayers, which might be related to the size, dispersibility, and coating stability. Interestingly, we found that surface coating did not affect tissue distribution patterns, showing no accumulation in all organs analyzed after a single-dose oral administration of both SunActiveTM products. It can be strongly suggested that enhanced oral Fe absorption by surface coating did not influence on tissue accumulation, and surface modified Fe or Zn fortification has low toxicity potential.
Conclusion
Surface modified Fe or Zn fortification with low-molecular-carbohydrates and glycerides had stable and high dispersibility as well as small hydrodynamic radius in various aqueous media. However, cytotoxicity, uptake behaviors, and oral absorption were highly dependent on the nature of fortified minerals and surface coating materials; SunActive FeTM with dextrin coating enhanced cellular uptake, intestinal transport efficacy, and bioavailability, but did not cause cytotoxicity, which was not found for SunActive ZnTM with maltodextrin coating. The discrepancy can be attributed to the stability of coating materials under physiological condition. On the other hand, surface modification of Fe or Zn fortification did not affect intestinal transport mechanism and tissue distribution pattern. Taken together, bioavailability of mineral fortification can be enhanced by surface modification to some extent, without affecting potential toxicity. These findings will provide a useful strategy to enhance oral absorption efficacy of food fortification at safe levels.
Author Contributions
Conceived and designed the experiments and wrote the manuscript: S-JC and J-MO, performed biological experiments: H-JiK, S-HB, performed physicochemical experiments: H-JuK, K-MK, JHS, performed statistical and data analysis: M-RG and JY.
Conflict of Interest Statement
The authors declare that the research was conducted in the absence of any commercial or financial relationships that could be construed as a potential conflict of interest.
Acknowledgment
This research was supported by a grant (14162MFDS135) from Ministry of Food and Drug Safety in 2014 and by a research grant from Seoul Women’s University (2017).
Supplementary Material
The Supplementary Material for this article can be found online at: http://journal.frontiersin.org/article/10.3389/fmicb.2017.00749/full#supplementary-material
References
Beard, J., and Stoltzfus, R. J. (2001). Iron deficiency anemia: reexamining the nature and magnitude of the public health problem. J. Nutr. 131, 563S–703S.
Brown, K. H., Rivera, J. A., Bhutta, Z., Gibson, R. S., King, J. C., Lönnerdal, B., et al. (2004). International Zinc Nutrition Consultative Group (IZiNCG) technical document #1. Assessment of the risk of zinc deficiency in populations and options for its control. Food Nutr. Bull. 25, S91–S204.
Brown, K. H., and Wuehler, S. E. (2000). Zinc and Human Health: Results of Recent Trials and Implications for Program Interventions and Research. Ottawa, ON: International Development Research Centre.
Chronakis, I. S. (1998). On the molecular characteristics, compositional properties, and structural-functional mechanisms of maltodextrins: a review. Crit. Rev. Food Sci. 38, 599–637. doi: 10.1080/10408699891274327
des Rieux, A., Fievez, V., Théate, I., Mast, J., Préat, V., and Schneider, Y. J. (2007). An improved in vitro model of human intestinal follicle-associated epithelium to study nanoparticle transport by M cells. Eur. J. Pharm. Sci. 30, 380–391. doi: 10.1016/j.ejps.2006.12.006
Faiz, H., Zuberi, A., Nazir, S., Rauf, M., and Younus, N. (2015). Zinc oxide, zinc sulfate and zinc oxide nanoparticles as source of dietary zinc: comparative effects on growth and hematological indices of juvenile grass carp (Ctenopharyngodon idella). Int. J. Agric. Biol. 17, 568–574. doi: 10.17957/IJAB/17.3.14.446
Fidler, M. C., Walczyk, T., Davidsson, L., Zeder, C., Sakaguchi, N., Juneja, L. R., et al. (2004). A micronised, dispersible ferric pyrophosphate with high relative bioavailability in man. Br. J. Nutr. 91, 107–112. doi: 10.1079/BJN20041018
Food and Drug Administration [FDA] (2014). Guidance for Industry Assessing the Effects of Significant Manufacturing Process Changes, Including Emerging Technologies, on the Safety and Regulatory Status of Food Ingredients and Food Contact Substances, Including Food Ingredients that are Color Additives. Silver Spring, MD: Food and Drug Administration.
Hider, R. C., and Kong, X. (2013). “Effect of overload and deficiency,” in Interrelations between Essential Metal Ions and Human Diseases, ed. A. Sigel (Dordrecht: Springer), 229–294.
Honda, N., Sakaguchi, K., Nakata, K., and Nanbu, H. (2010). Mineral Composition E.P. Patent No 1,649,762. Munich: European Patent Office.
Hubatsch, I., Ragnarsson, E. G. E., and Artursson, P. (2007). Determination of drug permeability and prediction of drug absorption in Caco-2 monolayers. Nat. Protoc. 2, 2111–2119. doi: 10.1038/nprot.2007.303
Hurrell, R. F. (1997). Preventing iron deficiency through food fortification. Nutr. Rev. 55, 210–222. doi: 10.1111/j.1753-4887.1997.tb01608.x
Hurrell, R. F. (2002). Fortification: overcoming technical and practical barriers. J. Nutr. 132, 806S–812S.
Ishihara, K., Yamanami, K., Takano, M., Suzumura, A., Mita, Y., Oka, T., et al. (2008). Zinc bioavailability is improved by the micronised dispersion of zinc oxide with the addition of L-histidine in zinc-deficient rats. J. Nutr. Sci. Vitaminol. 54, 54–60.
Jang, M. H., Kweon, M. N., Iwatania, K., Yamamoto, M., Terahara, K., Sasakawa, C., et al. (2004). Intestinal villous M cells: an antigen entry site in the mucosal epithelium. Proc. Natl. Acad. Sci. U.S.A. 101, 6110–6115. doi: 10.1073/pnas.0400969101
Jo, M. R., Chung, H. E., Kim, H. J., Bae, S. H., Go, M. R., Yu, J., et al. (2016). Effects of zinc oxide nanoparticle dispersants on cytotoxicity and cellular uptake. Mol. Cell. Toxicol. 12, 281–288. doi: 10.1007/s13273-016-0033-y
Joseph, T., and Morrison, M. (2006). Nanoforum Report: Nanotechnology in Agriculture and Food. Wolverhampton: Institute of Nanotechnology.
Kernéis, S., Bogdanova, A., Kraehenbuhl, J. P., and Pringault, E. (1997). Conversion by Peyer’s patch lymphocytes of human enterocytes into M cells that transport bacteria. Science 277, 949–952. doi: 10.1126/science.277.5328.949
Kloots, W., den Kamp, D. O., and Abrahamse, L. (2004). In vitro iron availability from iron-fortified whole-grain wheat flour. J. Agric. Food Chem. 52, 8132–8136. doi: 10.1021/jf040010+
Ma, T. Y., Boivin, M. A., Ye, D., Pedram, A., and Said, H. M. (2005). Mechanism of TNF-{alpha} modulation of Caco-2 intestinal epithelial tight junction barrier: role of myosin light-chain kinase protein expression. Am. J. Physiol. Gastrointest. Liver Physiol. 288, G422–G430. doi: 10.1152/ajpgi.00412.2004
Manke, A., Wang, L., and Rojanasakul, Y. (2013). Mechanisms of nanoparticle-induced oxidative stress and toxicity. Biomed. Res. Int. 2013:942916. doi: 10.1155/2013/942916
Neutra, M. R., Mantis, N. J., and Kraehenbuhl, J. P. (2001). Collaboration of epithelial cells with organized mucosal lymphoid tissues. Nat. Immunol. 2, 1004–1009. doi: 10.1038/ni1101-1004
Prasad, A. S. (2012). Discovery of human zinc deficiency: 50 years later. J. Trace Elem. Med. Biol. 26, 66–69. doi: 10.1016/j.jtemb.2012.04.004
Raya, S. D. H. A., Hassan, M. I., Farroh, K. Y., Hashim, S. A., and Salaheldin, T. A. (2016). Zinc oxide nanoparticles fortified biscuits as a nutritional supplement for zinc deficient rats. J. Nanomed. Res. 4:00081. doi: 10.15406/jnmr.2016.04.00081
Roe, M. A., Collings, R., Hoogewerff, J., and Fairweather-Tait, S. J. (2009). Relative bioavailability of micronized, dispersible ferric pyrophosphate added to an apple juice drink. Eur. J. Nutr. 48, 115–119. doi: 10.1007/s00394-008-0770-3
Rossi, L., Velikov, K. P., and Philipse, A. P. (2014). Colloidal iron(III) pyrophosphate particles. Food Chem. 151, 243–247. doi: 10.1016/j.foodchem.2013.11.050
Sakaguchi, N., Rao, T. P., Nakata, K., Nanbu, H., and Juneja, L. R. (2004). Iron absorption and bioavailability in rats of micronized dispersible ferric pyrophosphate. Int. J. Vitam. Nutr. Res. 74, 3–9. doi: 10.1024/03009831.74.1.3
Song, W., Zhang, J., Guo, J., Zhang, J., Ding, F., Li, L., et al. (2010). Role of the dissolved zinc ion and reactive oxygen species in cytotoxicity of ZnO nanoparticles. Toxicol. Lett. 199, 389–397. doi: 10.1016/j.toxlet.2010.10.003
Srinivas, P. R., Philbert, M., Vu, T. Q., Huang, Q., Kokini, J. L., Saltos, E., et al. (2010). Nanotechnology research: applications in nutritional sciences. J. Nutr. 140, 119–124. doi: 10.3945/jn.109.115048
Torabi, M., Kesmati, M., Harooni, H. E., and Varzi, H. N. (2013). Effects of nano and conventional zinc oxide on anxiety-like behavior in male rats. Indian J. Pharmacol. 45, 508–512. doi: 10.4103/0253-7613.117784
Wang, B., Zhang, Y., Mao, Z., Yu, D., and Gao, C. (2014). Toxicity of ZnO nanoparticles to macrophages due to cell uptake and intracellular release of zinc ions. J. Nanosci. Nanotechnol. 14, 5688–5696. doi: 10.1166/jnn.2014.8876
Yin, Y., Lin, Q., Sun, H., Chen, D., Wu, Q., Chen, X., et al. (2012). Cytotoxic effects of ZnO hierarchical architectures on RSC96 Schwann cells. Nanoscale Res. Lett. 7:439. doi: 10.1186/1556-276X-7-439
Zariwala, M. G., Somavarapu, S., Farnaud, S., and Renshaw, D. (2013). Comparison study of oral iron preparations using a human intestinal model. Sci. Pharm. 81, 1123–1139. doi: 10.3797/scipharm.1304-03
Keywords: surface modification, SunActiveTM, toxicity, uptake, oral absorption, dispersibility
Citation: Kim H-J, Bae S-H, Kim H-J, Kim K-M, Song JH, Go M-R, Yu J, Oh J-M and Choi S-J (2017) Cytotoxicity, Intestinal Transport, and Bioavailability of Dispersible Iron and Zinc Supplements. Front. Microbiol. 8:749. doi: 10.3389/fmicb.2017.00749
Received: 13 March 2017; Accepted: 11 April 2017;
Published: 28 April 2017.
Edited by:
Jayanta Kumar Patra, Dongguk University, South KoreaReviewed by:
Yong-Ro Kim, Seoul National University, South KoreaDae-Hwan Park, University of South Australia, Australia
Copyright © 2017 Kim, Bae, Kim, Kim, Song, Go, Yu, Oh and Choi. This is an open-access article distributed under the terms of the Creative Commons Attribution License (CC BY). The use, distribution or reproduction in other forums is permitted, provided the original author(s) or licensor are credited and that the original publication in this journal is cited, in accordance with accepted academic practice. No use, distribution or reproduction is permitted which does not comply with these terms.
*Correspondence: Jae-Min Oh, amFlbWluLm9oQHlvbnNlaS5hYy5rcg== Soo-Jin Choi, c2pjaG9pQHN3dS5hYy5rcg==
†These authors have contributed equally to this work.