- 1Institute for Molecular Microbiology and Biotechnology, University of Münster, Münster, Germany
- 2Center for Biotechnology (CeBiTec), Bielefeld, Germany
- 3Research Center Borstel, Sülfeld, Germany
- 4German Center for Infection Research, Borstel, Germany
- 5Institute for Medical Microbiology and Hospital Epidemiology, Hannover Medical School, Hannover, Germany
Pseudomonas aeruginosa employs 2-heptyl-3-hydroxy-4(1H)-quinolone (the Pseudomonas quinolone signal, PQS) and 2-heptyl-4(1H)-quinolone (HHQ) as quorum sensing signal molecules, which contribute to a sophisticated regulatory network controlling the production of virulence factors and antimicrobials. We demonstrate that Mycobacterium abscessusT and clinical M. abscessus isolates are capable of degrading these alkylquinolone signals. Genome sequences of 50 clinical M. abscessus isolates indicated the presence of aqdRABC genes, contributing to fast degradation of HHQ and PQS, in M. abscessus subsp. abscessus strains, but not in M. abscessus subsp. bolletii and M. abscessus subsp. massiliense isolates. A subset of 18 M. a. subsp. abscessus isolates contained the same five single nucleotide polymorphisms (SNPs) compared to the aqd region of the type strain. Interestingly, representatives of these isolates showed faster PQS degradation kinetics than the M. abscessus type strain. One of the SNPs is located in the predicted promoter region of the aqdR gene encoding a putative transcriptional regulator, and two others lead to a variant of the AqdC protein termed AqdCII, which differs in two amino acids from AqdCI of the type strain. AqdC, the key enzyme of the degradation pathway, is a PQS dioxygenase catalyzing quinolone ring cleavage. While transcription of aqdR and aqdC is induced by PQS, transcript levels in a representative of the subset of 18 isolates were not significantly altered despite the detected SNP in the promoter region. However, purified recombinant AqdCII and AqdCI exhibit different kinetic properties, with approximate apparent Km values for PQS of 14 μM and 37 μM, and kcat values of 61 s-1 and 98 s-1, respectively, which may (at least in part) account for the observed differences in PQS degradation rates of the strains. In co-culture experiments of P. aeruginosa PAO1 and M. abscessus, strains harboring the aqd genes reduced the PQS levels, whereas mycobacteria lacking the aqd gene cluster even boosted PQS production. The results suggest that the presence and expression of the aqd genes in M. abscessus lead to a competitive advantage against P. aeruginosa.
Introduction
Pseudomonas aeruginosa is an opportunistic pathogen regulating its virulence via a complex quorum sensing (QS) network. Besides N-acyl homoserine lactone-mediated las and rhl systems, it possesses an alkylquinolone (AQ) dependent QS system which uses PQS [the Pseudomonas quinolone signal, 2-heptyl-3-hydroxy-4(1H)-quinolone] and its biosynthetic precursor HHQ [2-heptyl-4(1H)-quinolone] as signal molecules (reviewed in Heeb et al., 2011; Huse and Whiteley, 2011). AQ signaling is involved in the regulation of a number of virulence factors such as the siderophore pyoverdine, the redox-active phenazine pigment pyocyanin, rhamnolipid biosurfactants, and the cytotoxic lectin and adhesin LecA (Deziel et al., 2005; Heeb et al., 2011; Huse and Whiteley, 2011).
Several QS-regulated exoproducts of P. aeruginosa do not only contribute to establishing infections of the host, but also act as antimicrobials. These may affect other species coexisting with P. aeruginosa in mixed microbial communities such as those infecting the lung of cystic fibrosis (CF) patients. Studies using laboratory co-cultures of P. aeruginosa with other bacteria support the hypothesis that QS-controlled exoproducts are important for competition. For example, hydrogen cyanide, rhamnolipids, and phenazines together promoted P. aeruginosa competitiveness in co-culture with Burkholderia multivorans, with hydrogen cyanide contributing the greatest effect (Smalley et al., 2015). Pyoverdine was found to contribute to growth inhibition of B. cenocepacia by P. aeruginosa (Costello et al., 2014), and another study showed that pyoverdine-mediated iron acquisition was responsible for growth suppression of Corynebacterium glutamicum, Bacillus subtilis, and Staphylococcus aureus by cell-free culture supernatants of P. aeruginosa (Lee et al., 2016). Thus, in polymicrobial communities, bacteria capable of quenching the production of P. aeruginosa antimicrobials by interference with its QS systems should have some advantage for survival and growth.
Besides acting as QS signals contributing to the regulation of P. aeruginosa exoproducts, PQS and HHQ have antagonistic effects on other microorganisms. PQS acts as an iron-trap (Bredenbruch et al., 2006; Diggle et al., 2007), HHQ exhibits bacteriostatic activity against several Gram-negative bacteria, and both PQS and HHQ repress motility in a range of bacteria (Reen et al., 2011). Moreover, the AQ biosynthetic pathway of P. aeruginosa besides 2-alkyl-4(1H)-quinolones and their 3-hydroxylated congeners also yields 2-alkyl-4-hydroxyquinoline-N-oxides (AQNOs), which act as antibiotics, inhibiting respiratory electron transfer at the cytochrome bc1 complex (Lightbown and Jackson, 1956; Cooley et al., 2005). 2-Heptyl-4-hydroxyquinoline-N-oxide (HQNO), along with siderophores produced by P. aeruginosa, drives S. aureus from aerobic respiration to fermentative metabolism. This is not only detrimental to S. aureus growth rates and fitness, but also results in the production of lactate that is preferentially utilized by P. aeruginosa (Filkins et al., 2015).
Rhodococcus erythropolis BG43, an isolate from soil, is the first bacterium described to be able to degrade HHQ and PQS (Müller et al., 2014). Two gene clusters aqdA1B1C1 and aqdRA2B2C2, both inducible by PQS or a metabolite thereof, were shown to be involved in PQS and HHQ degradation (Müller et al., 2015). Considering their multiple biological functions, degradation of HHQ and PQS could serve for QS interference, or detoxification, or both. Interestingly, homologs to the entire aqdRA2B2C2 cluster from R. erythropolis BG43 (locus tags XU06_RS29725 to XU06_RS29740; NZ_CP011296.1) are conserved in the genomes of some other actinobacteria, especially in representatives of the rapidly growing mycobacteria (RGM) such as strains of M. fortuitum, M. mageritense, and M. abscessus (locus tags MAB_0300c to MAB_0303; NC_010397.1; Müller et al., 2015). M. abscessus, one of the most pathogenic and antibiotic-resistant RGM, is considered an emerging pathogen, causing a pseudotuberculosis lung disease to which patients with CF are particularly susceptible (Griffith et al., 2007; Roux et al., 2009). A recent study revealed that dominant clones of M. abscessus, which emerged a few decades ago and show increased virulence, have spread globally, emerging as a major threat to individuals with CF (Bryant et al., 2016).
The identification of genes potentially coding for an AQ conversion pathway in the genomes of M. abscessus raises the question of whether the strains are indeed able to transform AQ compounds. In this study, we analyzed the degradation of HHQ and PQS by M. abscessusT DSM 44196 and by M. abscessus isolates from CF patients. Having identified three groups of strains that differ in their kinetics of PQS degradation and in the presence and type of aqd genes, we determined their effect on PQS levels in co-cultures with P. aeruginosa. To find out whether the differences in PQS degradation kinetics observed for the two groups of clinical isolates harboring aqd genes are due to distinct catalytic properties of their PQS dioxygenases, or due to differences in gene expression, we compared the kinetic properties of the purified enzymes and determined aqd transcript levels.
Materials and Methods
Mycobacterium abscessus Strains
The nomenclature of Mycobacterium abscessus is complicated by the non-uniform use in the literature of species and subspecies designations. Currently, two subspecies are recognized: M. abscessus subsp. abscessus, and M. abscessus subsp. bolletii which unites the previous subspecies massiliense and bolletii (Leao et al., 2011). However, for the sake of clarity and because recent publications support the previous classification (Cho et al., 2013; Tan et al., 2015; Bryant et al., 2016; Tortoli et al., 2016), we use the three-subspecies designations. The M. abscessus isolates from CF patients used in this study were characterized previously (Rüger et al., 2014; Kehrmann et al., 2016). The type strain of M. abscessus subsp. abscessus (DSM 44196) was obtained from DSMZ, Braunschweig, Germany.
Chemicals
Pseudomonas quinolone signal and HHQ were purchased from Sigma Aldrich and dissolved in methanol.
Genome Sequencing
Sequencing libraries were constructed from extracted genomic DNA with the Nextera XT kit (Illumina) and sequenced on the Illumina MiSeq instrument in a 2 × 300 bp paired end run or in a HiSeq 2 × 150 bp paired end Rapid Run.
Reverse Transcription PCR
For isolation of RNA, M. abscessusT (DSM 44196) was grown in DSM219 medium. To possibly induce the expression of aqd genes, 20 μM PQS was added 2 h before harvesting the cells by centrifugation. Cells were frozen in liquid nitrogen and stored at –80°C. Cells were then resuspended in TE buffer (10 mM Tris, 1 mM EDTA, pH 8.0) and disrupted using the Mikro-Dismembrator S (Sartorius, 3000 rpm, 2 min) after addition of glass beads (150–212 μm diameter) to the cell suspension. Subsequently, RNA purification was performed with the innuPREP RNA Mini kit (Analytik Jena) according to the manufacturer’s instructions. RNA concentration was determined using the Nanophotometer N60 (IMPLEN). Removal of DNA contamination was executed with DNAse I (Thermo Scientific) at 37°C and checked via PCR (GoTaq Polymerase, Promega) after renewed RNA purification. For analysis of operon structures, cDNA synthesis was carried out using the RevertAid H Minus First Strand cDNA Synthesis kit (Thermo Scientific) according to the manufacturer’s instructions. Primers were designed to amplify 500 bps of regions spanning aqdAB and aqdBC.
For relative mRNA quantification of single genes, RT-qPCR was used. Primers were designed to amplify 75–150 bps of the analyzed genes (sequences shown in Table 1). All measurements were performed in a LightCycler 96 System (Roche) with a SensiFast SYBR No-Rox One-Step Kit (Bioline, London, UK) and 96 well lightcycler plates (Sarstedt) as reaction vessels, sealed with qPCR seals (Sarstedt). The concentrations of all template RNA samples were adjusted to 200 ng/μL for normalization on total RNA. One microlitre of the RNA samples was used as template and mixed with 19 μL master mix containing 1 μL of specific primers (10 μM each), 0.2 μL reverse transcriptase, 0.4 μL RNase inhibitor, 10 μL reaction mix and 7.4 μL 5 M glycine betaine. All measurements were carried out with a minimum of three biological replicates in two technical duplicates each. For each primer pair two negative controls with 1 μL H2O as template were included. Reverse transcription was carried out at 45°C for 20 min, followed by 2 min polymerase activation at 95°C, a three step amplification (95°C 5 s, 60°C 10 s, 72°C 10 s, 60 cycles) and a melting profile analysis. Evaluation of control measurements and analysis of the melting curves as well as Cq calculation was carried out with the LightCycler 96 V1.1 software. The relative transcript amount was normalized on total RNA (200 ng) and calculated as 2-ΔCq where ΔCq corresponds to the difference of the mean Cq values.
Expression of aqd Genes in E. coli for Biotransformation Experiments
The aqdB and aqdC genes were amplified by PCR from a colony of M. abscessusT (DSM 44196). Both AqdB and AqdC were produced as His8-MBP fusion proteins in recombinant E. coli strains, obtained by restriction-free cloning of the corresponding genes into the pET28b(+) expression vector (van den Ent and Löwe, 2006). E. coli Rosetta(DE3) was transformed with pET28b(+)::his8-mbp-aqdB due to many rare codons in the aqdB sequence. E. coli BL21(DE3) was transformed with pET28b(+)::his8-mbp-aqdC.
Growth Conditions and Biotransformation Assays
Mycobacterium strains were cultivated in DSM219 medium, and recombinant E. coli BL21(DE3) and Rosetta(DE3) strains were grown in LB medium supplemented with 50 μg/mL kanamycin, at 37°C. For AQ biotransformation by mycobacteria, cells from pre-cultures were suspended at an optical density at 600 nm of 3.5 in fresh DSM219 medium, supplemented with 20 μM of HHQ or PQS, and incubated at 37°C. AQs were extracted at different time points as described previously (Müller et al., 2014). Samples of extracted cell suspensions were solubilized in methanol and analyzed via HPLC. For biotransformations of AQs by recombinant E. coli strains harboring pET28b(+)::his8-mbp-aqdB or pET28b(+)::his8-mbp-aqdC, cells were grown overnight at 30°C in the presence of 0.1 mM IPTG, harvested by centrifugation, and resuspended in fresh LB medium with 0.1 mM IPTG, adjusting an OD600nm of 3.5. Biotransformation assays were performed as described above.
Preparation of Mycobacterium Cell Extracts
Cell extracts were prepared from M. abscessusT (DSM 44196) grown in DSM219 medium. To possibly induce the expression of AQ-converting enzymes, 20 μM PQS was added 2 h before harvesting the cells by centrifugation. Cells resuspended in 50 mM potassium phosphate buffer pH 7.5 were disrupted by sonication, and cell debris was removed by centrifugation (20.000 × g, 45 min, 4°C). The supernatant (crude extract) was desalted using ZebaTM Spin Desalting columns (10 K molecular weight cut-off, Thermo Scientific). Total protein amount in cell extract supernatants was determined using the Bradford method as modified by Zor and Selinger (1996).
Purification of AqdC Proteins
The sequence of aqdC of M. abscessusT was optimized for codon usage of E. coli using OPTIMIZER (Puigbò et al., 2007) and synthesized by MWG Eurofins. For the purification of AqdC proteins, codon-optimized synthetic genes were cloned in pET28b(+) using restriction-free cloning (van den Ent and Löwe, 2006). The sequence coding for TEV protease cleavage site was introduced between the coding sequences for his8-tag and AqdC protein. In the following, AqdCI refers to the protein of the type strain, and the protein which differs from AqdCI by the two amino acid substitutions R129P and A133T is termed AqdCII. E. coli BL21(DE3) harboring pET28b(+)::his8-aqdCI or pET28b(+)::his8-aqdCII were grown at 37°C in Terrific Broth. At an OD600nm of 1.0, cultures were supplemented with 0.2 mM IPTG and incubated at 16°C overnight (for approximately 16 h). Cells harvested by centrifugation were resuspended in washing buffer (300 mM NaCl, 20 mM Tris and 10 mM imidazole, pH 8.0), disrupted by sonication, and the AqdC proteins were purified by Ni-NTA affinity chromatography and stored in buffer containing 20 mM Tris, 10% (v/v) glycerol (pH 8.0) at -80°C.
Enzyme Assay
The catalytic activity of AqdC proteins was determined spectrophotometrically at 30°C by measuring PQS consumption at 337 nm. The assays contained 20 μM PQS in assay buffer (50 mM Tris, 2 mM EDTA, 10% PEG 1500, 4% (v/v) DMSO, pH 8.0). The extinction coefficient of PQS in assay buffer is 10169 M-1 cm-1 at 337 nm. Apparent steady-state kinetic constants of AqdC proteins (two biological replicates with three technical replicates each) were estimated by fitting the initial velocities measured at different substrate concentrations with the Michaelis Menten equation.
Cocultivation of P. aeruginosa and M. abscessus Clinical Isolates
Overnight cultures of P. aeruginosa PAO1 and M. abscessus strains were used as inocula for co-cultivation experiments in 10% LB medium. To account for differences in growth rates (generation times of M. abscessus and P. aeruginosa in 10% LB are 38.5 and 16.5 h, respectively), P. aeruginosa was adjusted to an initial OD600nm of 0.05, and the mycobacterial strain to an OD600nm of 0.15, as described by Costa et al. (2015). Cells were incubated at 37°C under vigorous shaking. PQS was extracted after 8 and 24 h as described previously (Müller et al., 2014) and quantified by HPLC analysis. Colony forming units (CFUs) of P. aeruginosa PAO1 were determined by dropping 10 μL of a diluted culture onto LB agar and counting colonies after incubation at 37°C overnight. M. abscessus formed colonies only after 36 to 48 h, so selective medium was not necessary.
HPLC Analysis
For the identification and quantification of PQS and other AQs, compounds were separated on a 250 × 4 mm Eurospher II RP-18 column using a Hitachi EZchrom Elite HPLC system with diode array detector model 2450, or an Agilent 1100 series system with diode array detector model G1315B. Methanol with 0.1% (w/v) citric acid and 0.1% (w/v) citric acid in water were used as solvents. Separation of PQS and HHQ was carried out via a linear gradient from 80 to 100% methanol (v/v) over 20 min at a flow rate of 0.5 mL min-1.
Results
AQ Degradation in M. abscessusT
The presence of an aqdRABC gene cluster in the genome of M. abscessusT suggested that it might degrade HHQ and PQS via reactions analogous to those identified in R. erythropolis BG43 (Figure 1). The aqdB2 gene of R. erythropolis BG43 codes for an NADH-dependent HHQ monooxygenase, whereas the gene product of aqdC2 is a PQS-cleaving dioxygenase which requires O2 as only co-substrate (Müller et al., 2015). AqdB and AqdC activity was present in cell extract supernatant suggesting cytoplasmic localization. Desalted cell extract supernatants of M. abscessusT supplemented with NADH transformed HHQ (Figure 2A), and HHQ consumption was accompanied by transient formation of 2.0 ± 0.3 μM PQS, as identified by HPLC. Interestingly, extracts from cells pre-incubated with PQS converted HHQ and especially PQS faster than extracts from non-induced cells (Figure 2). To analyze whether transformation of HHQ and PQS is indeed catalyzed by the mycobacterial AqdB and AqdC protein, respectively, recombinant E. coli strains expressing aqdB or aqdC of M. abscessusT were constructed and tested for AQ biotransformation. E. coli cells producing the His8-MBP-AqdB protein converted HHQ to PQS, and E. coli cells expressing the M. abscessusT His8-MBP-AqdC fusion protein were able to cleave PQS to N-octanoylanthranilic acid, as identified by HPLC and comparison of UV/Vis spectra and fluorescent properties with reference compounds (Figure 3).
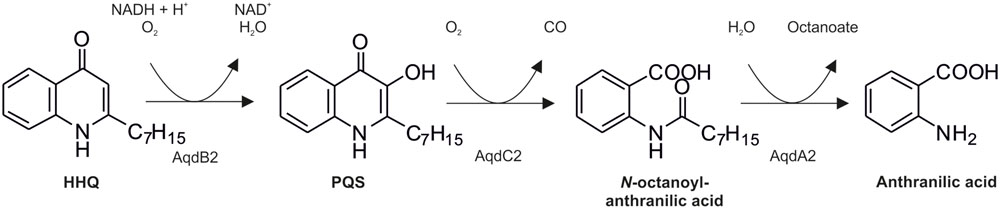
FIGURE 1. Pathway of 2-heptyl-4(1H)-quinolone (HHQ) and Pseudomonas quinolone signal (PQS) conversion in R. erythropolis BG43 (Müller et al., 2015). AqdB2, HHQ 3-monooxygenase; AqdC2, PQS 2,4-dioxygenase; AqdA2, N-octanoylanthranilate amide hydrolase.
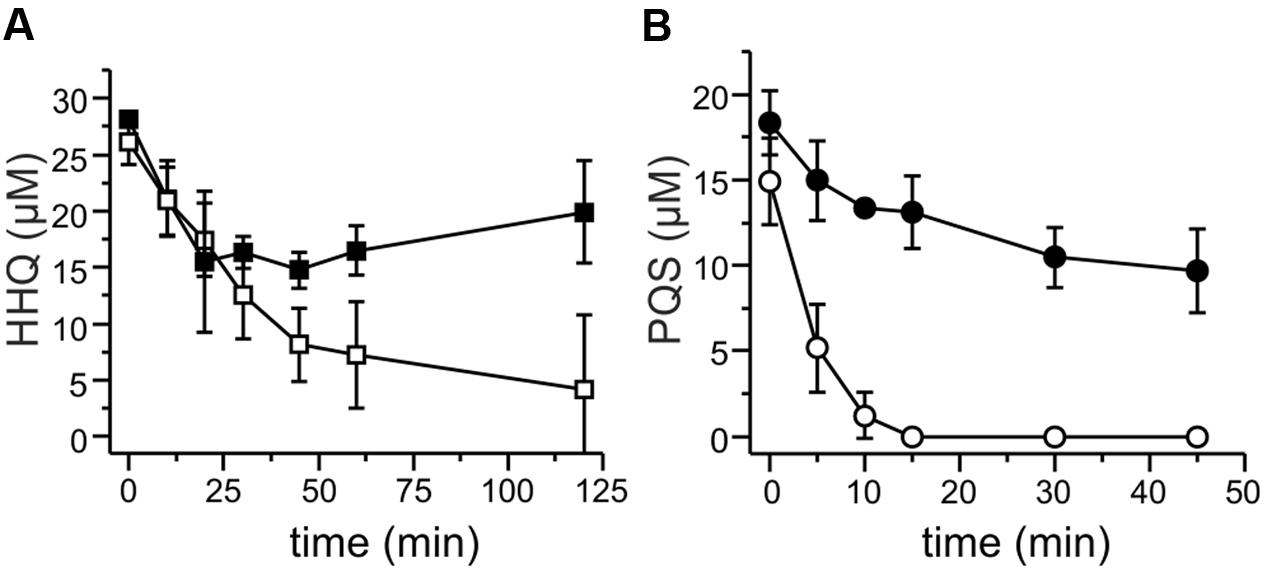
FIGURE 2. HHQ and PQS conversion by cell extracts of M. abscessusT. Extracts were obtained from cells grown in DSM219 medium (filled symbols), and from cells grown in the same medium but supplemented with PQS 2 h prior to harvesting (open symbols). Extracts obtained by sonication of cell suspensions were centrifuged, the resulting supernatants were desalted, set to a protein concentration of 1 mg/mL in 50 mM potassium phosphate buffer pH 7.5, and incubated with 20 μM HHQ and 500 μM NADH (A), or with 20 μM PQS (B). Samples were withdrawn at the given time points, extracted, and analyzed by HPLC. Means ± SD of three biological replicates are shown.
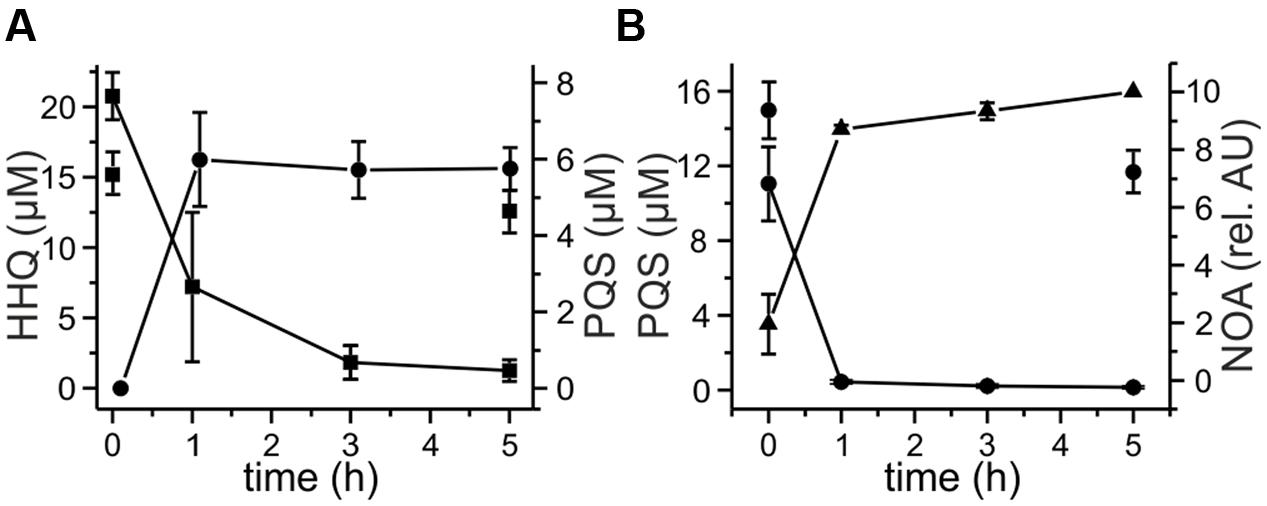
FIGURE 3. Degradation of 20 μM HHQ (A) and PQS (B) by recombinant E. coli strains. Cells suspended in LB medium were induced with 0.5 mM IPTG and incubated at 30°C overnight. Then they were supplemented with 20 μM HHQ or PQS and incubated at 30°C. Extracts of culture samples were analyzed by HPLC. (A) HHQ conversion by E. coli Rosetta pET28b::mbp-aqdB, squares: HHQ, circles: PQS. (B) PQS conversion by E. coli BL21 pET28b::mbp-aqdC; circles: PQS, triangles: N-octanoylanthranilic acid (NOA). E. coli Rosetta and E. coli BL21 did not convert HHQ (discrete squares in A) and PQS (discrete circles in B).
Taken together, the observations strongly support the hypothesis that the mycobacterial aqdRABC gene cluster (locus tags MAB_0300c to MAB_0303 in NC_010397.1) codes for an inducible AQ degradation pathway which proceeds analogous to that identified in R. erythropolis BG43 (Figure 1). N-Octanoylanthranilic acid formed by the PQS dioxygenase AqdC presumably is hydrolyzed by AqdA, a member of the carboxylesterase type B family, to anthranilic acid and octanoate, which both can be channeled into the central metabolism. The aqdR gene codes for a putative transcriptional regulator of the TetR family.
Presence of aqd Genes in M. abscessus Strains Isolated from CF Patients
The genomes of M. abscessus strains, previously isolated from respiratory samples of CF patients (Rüger et al., 2014), were analyzed for the presence and sequence of the aqd gene cluster with BLASTN analyses (Altschul et al., 1997). Among these 50 strains, 22 (44%) lack the aqd gene cluster. Interestingly, absence of the aqd genes correlates with the assignment of the strains to the subspecies bolletii (3 strains) and massiliense (19 strains), whereas all 28 strains of the subspecies abscessus harbor the aqd gene cluster. In all cases where two isolates were obtained from the same patient, the nucleotide sequences of the aqd gene clusters did not differ between the first and second isolate.
The nucleotide sequences of the aqdRABC genes of strains P5a, P5n, P23a, P23n, P28a, and P28n are identical to those of M. abscessus subsp. abscessusT (DSM 44196, ATCC 19977). Compared to the aqd gene region of the type strain, those of strains P13, P24 and another 16 isolates contain the same five single nucleotide variations (SNPs). Two of these SNPs within the protein coding regions of AqdA (triplet encoding L173) and AqdC (triplet encoding I187) are silent, and one is a transition within the predicted promoter region of aqdR. The two other SNPs lead to differences in the amino acids at position 129 and 133 of the predicted PQS dioxygenase AqdC (Table 2 and Supplementary Figure 1). Two other aqd gene modifications observed in the genome of individual strains lead to single amino acid deviations in AqdA (G394S) and AqdB (G15S), respectively (Table 2 and Supplementary Figure 1). However, with respect to AqdC, the key enzyme in PQS degradation, the strains can be divided into two groups: Nine isolates produce the same protein as the type strain (AqdCI), and 18 strains form a protein (AqdCII) which differs in two amino acids (Table 2). Supplementary Figure 1 shows the translated nucleotide sequence of the aqdRABC region and indicates the changes in nucleotide and amino acid sequences.
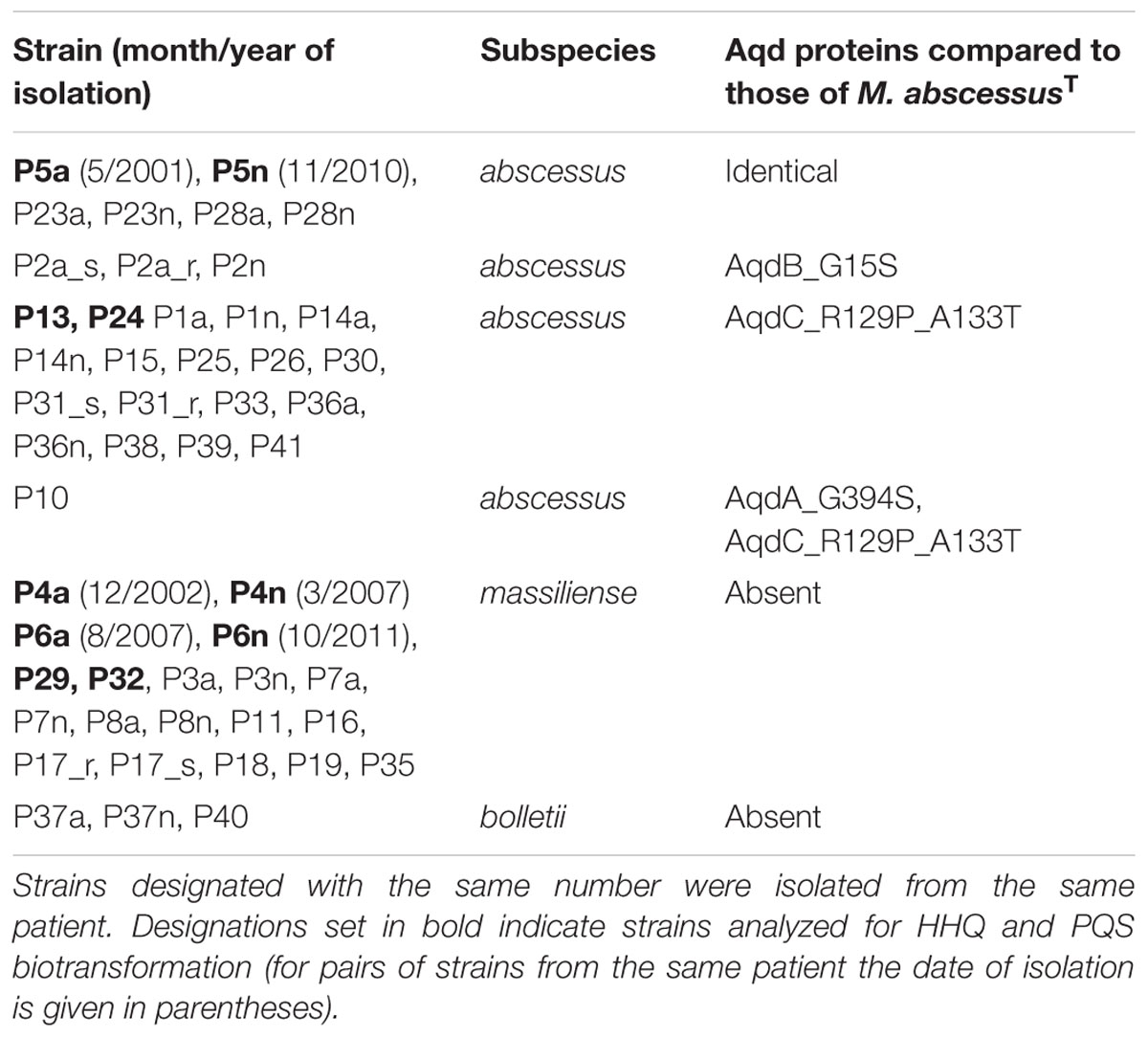
TABLE 2. Prediction of aqd gene products from the genomes of clinical isolates of M. abscessus (Rüger et al., 2014; Kehrmann et al., 2016).
AQ Degradation by Clinical M. abscessus Isolates
Ten of the clinical M. abscessus isolates (Table 2) were analyzed for their ability to degrade PQS and HHQ. Interestingly, members of the subset of 18 strains carrying the aqd gene cluster with the five SNPs (as compared to that of M. abscessusT) appeared to be the more potent PQS degraders. Surprisingly, even those strains that do not harbor aqd genes reduced the amount of PQS to different extents within 24 h (Figure 4).
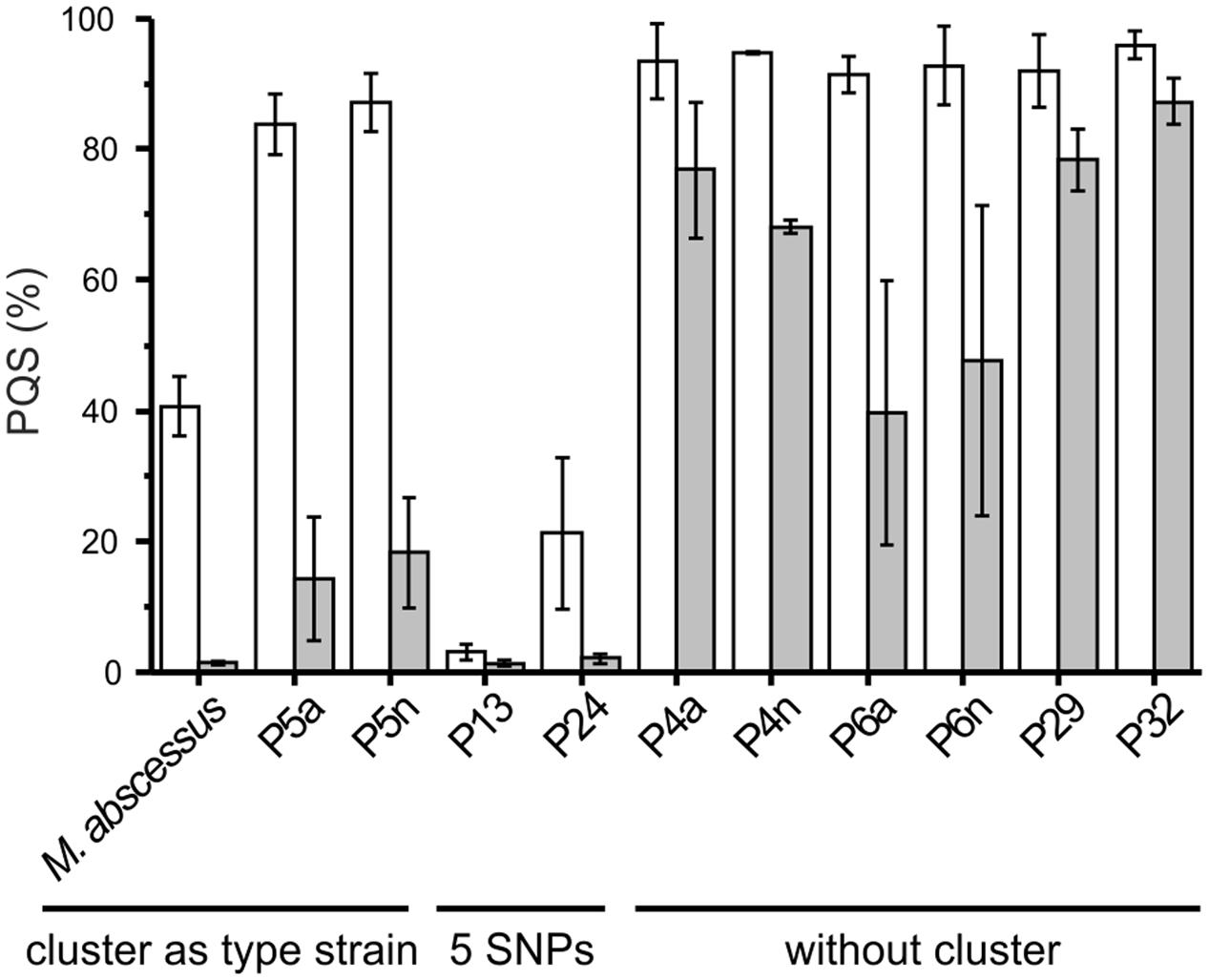
FIGURE 4. Pseudomonas quinolone signal consumption by the type strain and clinical isolates of Mycobacterium abscessus. Cells suspended at an OD600 nm of 3.5 in DSM219 medium were supplemented with 20 μM PQS and incubated at 37°C. Extracts of culture samples withdrawn after 0, 4 and 24 h were analyzed by HPLC. The PQS concentrations in samples taken at t = 0 h were set as 100%. Means ± SE of three independent biological replicates are shown. Decrease in PQS concentrations in sterile DSM219 medium due to abiotic oxidation was not observed within 24 h.
We selected a representative of each of the groups of strains – strain P5a with an aqd gene cluster identical to that of M. a. subsp. abscessusT, strain P13 with the five SNPs in the aqd region, and strain P4a lacking aqd genes – to determine the time course of HHQ and PQS conversion by cell suspensions. HHQ consumption by strains P5a and P13 followed similar kinetics. However, as already suggested by the preliminary data shown in Figure 4, strain P13 converted PQS faster than strain P5a, and strain P4a lacking the gene cluster very slowly converted HHQ as well as PQS (Figures 5A,B).
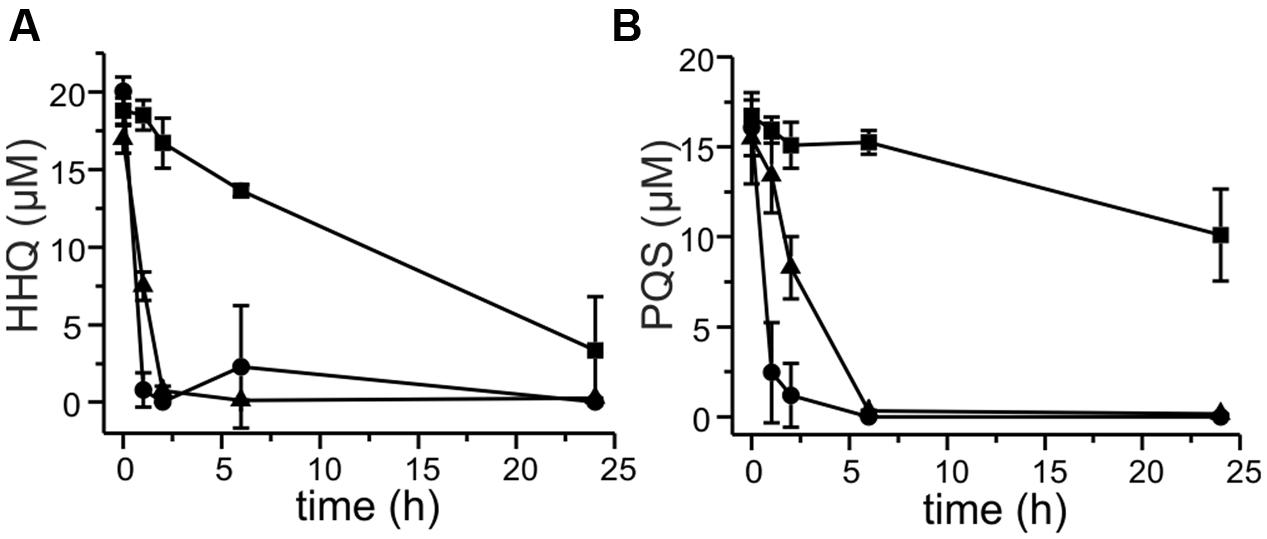
FIGURE 5. Degradation of 20 μM HHQ (A) and PQS (B) by M. abscessus strains. Cells suspended at an OD600 nm of 3.5 in DSM219 medium were supplemented with 20 μM HHQ or PQS, and incubated at 37°C. Extracts of culture samples were analyzed by HPLC. Squares: M. abscessus P4a, circles: M. abscessus P13, triangles: M. abscessus P5a. Means ± SD of three biological replicates are shown.
Strains without the aqd-cluster which slowly consume PQS and HHQ must use alternative enzymes to modify or even degrade these AQs. HPLC analyses of culture extracts revealed transient formation of 4.9 ± 3.9 μM PQS after 2 h of incubation with 20 μM HHQ, besides an arsenal of other metabolites. Thus, it appears that other monooxygenases besides AqdB can mediate HHQ hydroxylation to PQS. Due to the complexity of metabolites formed, incomplete peak separations, and the low concentrations of intermediates (expected to be produced by the cultures at the nM range), elucidation of their structures will require the establishment of improved extraction and separation protocols, as well as considerable upscaling, to enable NMR analyses for structural identification. However, because the UV spectra of many of the peaks of the HPLC elution profile resemble that of PQS, we assume that the corresponding metabolites are quinolones, with modifications introduced mainly to the alkyl chain.
Transcription of aqd Genes in Strains P13 and P5a
To analyze whether the aqdABC genes are co-transcribed, RT-PCR was performed with primer pairs addressing adjacent gene transcripts (for primer sequences see Table 1). Formation of PCR products, as verified by gel electrophoresis (not shown), indicate that aqdAB and aqdBC are co-transcribed, suggesting organization of the aqdABC genes in an operon.
Cells induced with 20 μM PQS have higher transcript levels of aqdR (fold change between 4.0 and 6.8), indicating autoregulation of aqdR transcription. Transcript levels of aqdC were also increased when the cells were induced with PQS (fold change between 5.4 and 5.7) (Figure 6A). In strains harboring the aqd genes with five SNPs compared to the aqd region of the type strain, the transition within the predicted promoter region of aqdR changes the inverted repeat sequence TTGTCGCATCGACAA to TCGTCGCATCGACAA. To determine whether the SNP results in different expression levels, transcript levels of aqdR and aqdC were compared for strains P5a and P13. RT-qPCR analyses revealed only slight reduction of transcription amounts in strain P13 (Figure 6B). Thus, it seems unlikely that differential expression of the aqdC gene coding for the key enzyme, a PQS dioxygenase, accounts for the observed differences in PQS degradation rates (Figure 5).
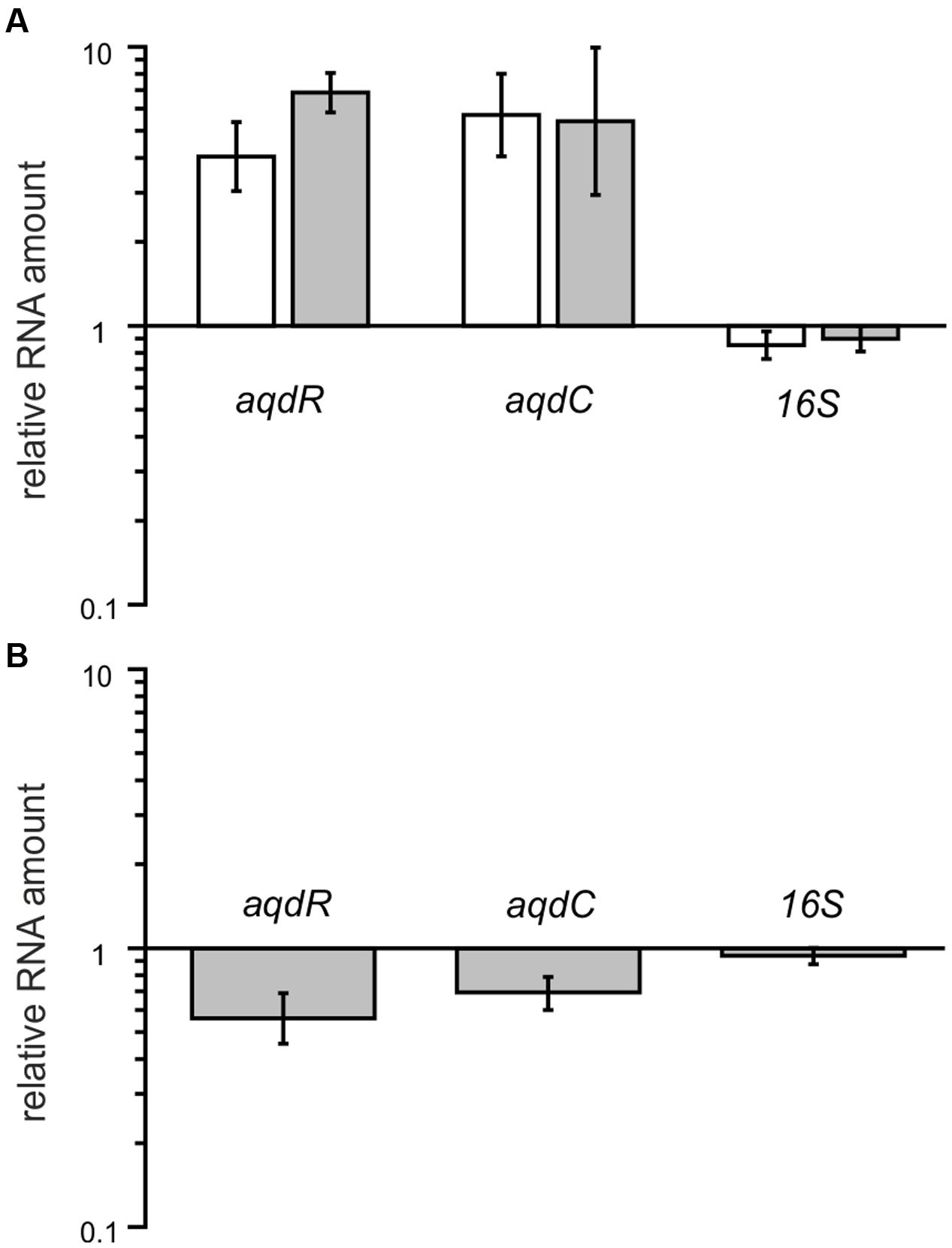
FIGURE 6. Relative transcript levels of aqdR, aqdC, and 16S rRNA gene as a control in strains P5a and P13. (A) Fold change in gene transcription due to PQS induction. White bars: strain P5a, gray bars: strain P13. Transcript amounts in non-induced cells were set as 1. (B) Transcript amounts of induced P13 cells normalized to levels of induced P5a cells. Means ± SD of at least three biological replicates are shown.
Catalytic Activity of AqdC Proteins
To analyze the possibility that the AqdCI and AqdCII proteins differ in their catalytic efficiency, recombinant proteins were purified to electrophoretic homogeneity, and their steady-state kinetic parameters were determined. Under the conditions of the assay, a specific activity of 53.2 U mg-1, an apparent kcat of 97.8 ± 19.1 s-1 and an apparent Km value for PQS of 37.2 ± 9.8 μM were observed for AqdCI, the PQS dioxygenase form of the M. a. subsp abscessus type strain and the group of strains represented by the isolate P5a. For AqdCII, the enzyme of the group of isolates represented by strain P13,a specific activity of 50.5 U mg-1, an apparent kcat of 61.0 ± 4.3 s-1 and apparent Km value of 13.6 ± 1.6 μM were determined.
PQS Concentrations in Co-cultures of P. aeruginosa and M. abscessus
Representatives of each M. abscessus group were co-cultivated with P. aeruginosa PAO1 to test whether PQS produced by P. aeruginosa, which is packaged into membrane vesicles for trafficking between cells (Mashburn-Warren et al., 2008), is amenable to degradation by the mycobacteria. Indeed, the clinical isolates P5a and P13, which degraded synthetic PQS, also were able to reduce the PQS concentration in co-culture with P. aeruginosa PAO1 (Figure 7). Most interestingly, however, the PQS concentration increased significantly when P. aeruginosa PAO1 was cultivated with strain P4a, a representative of the group lacking the aqd genes. Compared with the P. aeruginosa PAO1 cultures, the amount of PQS in P. aeruginosa PAO1 – M. abscessus P4a co-cultures was fivefold higher after 24 h of cultivation. P. aeruginosa PAO1 cultivated with dead (autoclaved) M. abscessus strain P13 cells produced increased amounts of PQS as well. After 24 h of incubation, the PQS concentration was almost eightfold higher than in the PAO1 solo cultures. CFUs of P. aeruginosa PAO1 cultures were similar to those of PAO1 in co-culture with M. abscessus strain P13, or PAO1 cultivated in the presence of autoclaved M. abscessus P13 cells. Therefore, the increase in PQS production especially in presence of autoclaved cells is not due to increased population density of P. aeruginosa. The boosting of PQS production may rely on the recognition of cell components by PAO1. It makes the reduced levels of PQS in PAO1-P5a and PAO1-P13 co-cultures even more remarkable.
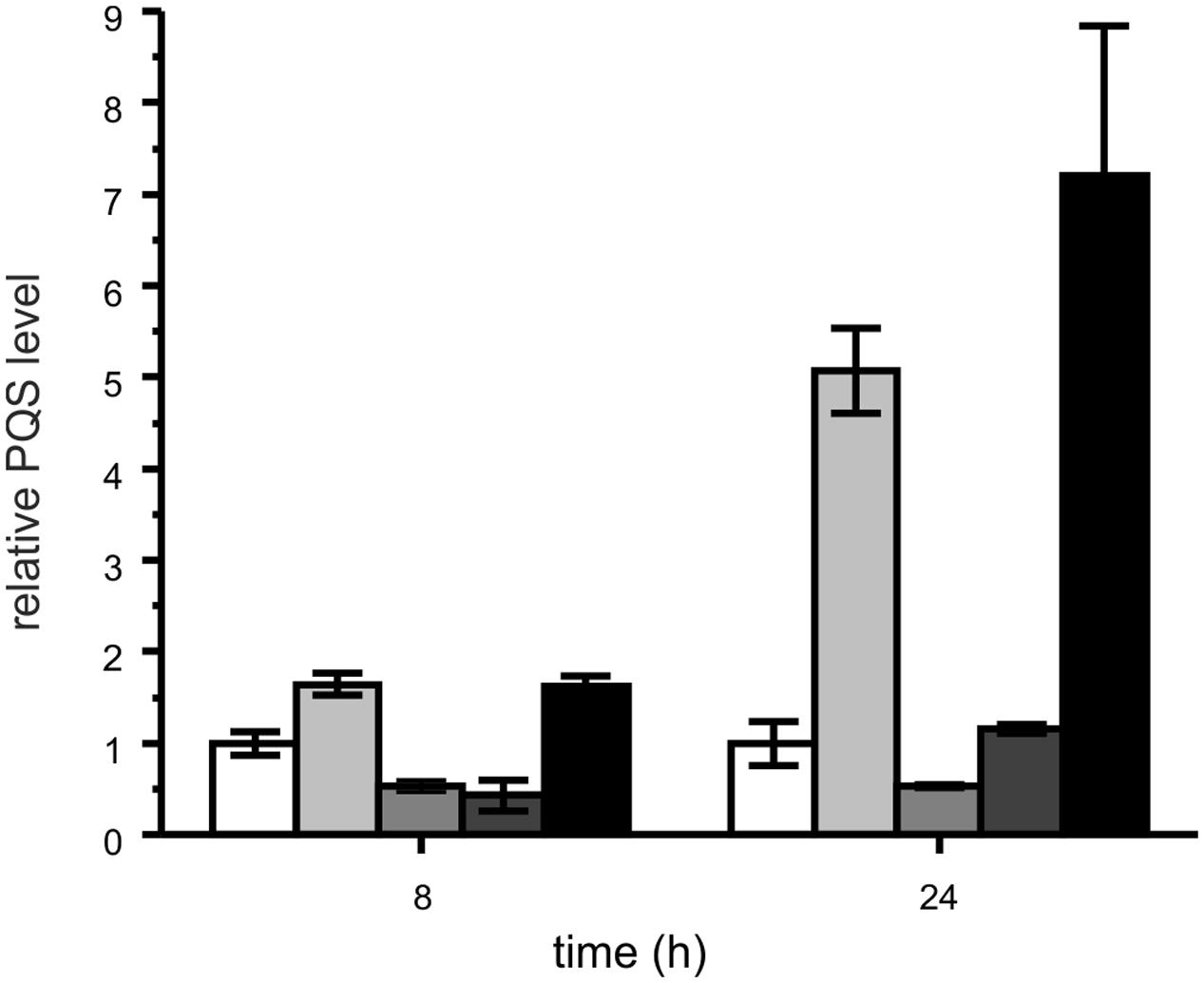
FIGURE 7. Relative PQS levels in cultures of P. aeruginosa PAO1 (white bars) and co-cultures of P. aeruginosa PAO1 with M. abscessus P4a (light gray), M. abscessus P5a (gray), M. abscessus P13 (dark gray), and autoclaved M. abscessus P13 (black), incubated under shaking at 37°C. Extracts of cultures were analyzed by HPLC. PQS concentrations in P. aeruginosa PAO1 cultures were set as 1 (8 h: 1.79 μM; 24 h: 0.76 μM PQS). Means ± SD of three biological replicates are shown. CFUs of P. aeruginosa PAO1 after 24 h of incubation: PAO1 culture: 1.81 × 1011; PAO1 – P13 co-culture: 1.21 × 1011; PAO1 cultured with autoclaved P13: 1.32 × 1011.
Discussion
Pseudomonas aeruginosa often dominates the microbiome of the lungs of adult CF patients, however, the CF lung is usually colonized with multiple pathogens. CF-related lung disease also is a risk factor for chronic pulmonary infection with RGM, and RGM are actually detected with increasing prevalence in the CF population. Especially M. abscessus is considered an emerging threat to individuals with CF (Bar-On et al., 2015; Bryant et al., 2016).
Alkylquinolones produced by P. aeruginosa, acting as QS signals and antimicrobials, have been detected in the sputum of CF patients (Collier et al., 2002; Barr et al., 2015). Thus, M. abscessus, when co-colonizing the CF lung, may well encounter these secondary metabolites. In this study, we demonstrate that M. abscessus is capable of degrading the P. aeruginosa signal molecules HHQ and PQS. Interestingly, clinical M. abscessus strains isolated from patients with CF showed different kinetics in PQS degradation, correlating with the presence and type of aqdRABC genes coding for an inducible HHQ and PQS degradation pathway. Differences in transcript levels, possibly related with the SNP in the promoter of the aqdR gene, differences in the kinetic properties of the PQS dioxygenases AqdCI and AqdCII, and additional factors such as mRNA or protein stability might influence the degradation rates. While RT-qPCRs showed no significant differences in expression levels of the aqdR and aqdC genes of strains P13 and P5a, the catalytic efficiency (kcat/Km) of the PQS dioxygenase AqdCII was found to be about 1.7-fold higher than that of AqdCI. Strain P13 and the majority of the isolates harboring the aqd gene cluster produce the AqdCII variant. Considering that PQS levels in P. aeruginosa cultures are about 16 and 2 μM when cultivated in LB and artificial sputum medium, respectively (Collier et al., 2002; Lépine et al., 2003), and those in CF sputum can reach high nM ranges (Barr et al., 2015), the AqdCII protein with its lower Km value should perform better under physiological conditions.
Co-cultivation of M. abscessus isolates P13 and P5a with P. aeruginosa PAO1 reduced PQS concentrations in these cultures, whereas the presence of strain P4a (which lacks the aqd genes), or presence of dead mycobacterial cells significantly enhanced PQS production by P. aeruginosa PAO1. Thus, P. aeruginosa seems to recognize and respond to the presence of M. abscessus cells, cellular components, or exoproducts. Upregulation of PQS production by a mycobacterial effector should have broad implications on virulence as well as competitiveness of P. aeruginosa, because the pqs system controls a diverse array of virulence factors, such as the redox-active pigment pyocyanin, rhamnolipid surfactants, the siderophore pyoverdine, and the antimicrobial 2-alkyl-4-hydroxyquinoline N-oxides (Heeb et al., 2011). Especially the latter compounds not only affect competing microorganisms but also the fitness of P. aeruginosa itself, even promoting cell autolysis and DNA release (Hazan et al., 2016). As regards a possible mycobacterial effector, it is interesting that P. aeruginosa has been reported to enhance production of PQS and phenazine antimicrobials in response to N-acetylglucosamine, a peptidoglycan turnover product shed by Gram-positive bacteria (Korgaonkar and Whiteley, 2011; Korgaonkar et al., 2013). However, since mycobacteria have a complex cell envelope dominated by arabinogalactan and mycolic acids besides peptidoglycan, P. aeruginosa may sense additional or other components.
Remarkably, among the M. abscessus isolates tested, the presence of aqd genes strictly correlated with the subspecies abscessus, which is the subspecies most frequently isolated from CF patients worldwide (Bryant et al., 2016). It will be interesting to analyze whether the ability to rapidly inactivate P. aeruginosa QS signals, which not only control the production of virulence factors and antimicrobials but act as antimicrobials themselves, contributes to co-colonization competitiveness of the subspecies abscessus. However, AQ degradation by M. abscessus likely is only one aspect in a multi-faceted interaction between the two pathogens and our observation of increased PQS production in response to mycobacterial cell material opens up new questions of how P. aeruginosa monitors and responds to its biotic environment.
Author Contributions
SF and FSB conceived the experiments. TK performed genome sequencing, TW performed and analyzed RT-qPCRs, and FSB performed all other experiments. JK analyzed genome sequences. KR and FB provided mycobacterial strains. SF and FSB analyzed data and wrote the paper. All authors contributed to the final version of the manuscript.
Funding
This work was supported by the Deutsche Forschungsgemeinschaft (grant no. FE 383/25-1 to SF). FSB thanks the Studienstiftung des deutschen Volkes for funding and support. Parts of the work were supported by the European Union PathoNgenTrace project (FP7- 278864-2) and the German Center for Infection Research (DZIF).
Conflict of Interest Statement
The authors declare that the research was conducted in the absence of any commercial or financial relationships that could be construed as a potential conflict of interest.
Acknowledgments
SF and FSB gratefully acknowledge Karina Kleinlosen for cloning and expression of the mycobacterial aqdB gene, Hannah Schmitz for preparation and first biochemical experiments with AqdC dioxygenases, and Almut Kappius for excellent technical assistance.
Supplementary Material
The Supplementary Material for this article can be found online at: http://journal.frontiersin.org/article/10.3389/fmicb.2017.00339/full#supplementary-material
References
Altschul, S. F., Madden, T. L., Schäffer, A. A., Zhang, J., Zhang, Z., Miller, W., et al. (1997). Gapped BLAST and PSI-BLAST: a new generation of protein database search programs. Nucleic Acids Res. 25, 3389–3402. doi: 10.1093/nar/25.17.3389
Bar-On, O., Mussaffi, H., Mei-Zahav, M., Prais, D., Steuer, G., Stafler, P., et al. (2015). Increasing nontuberculous mycobacteria infection in cystic fibrosis. J. Cyst. Fibros. 14, 53–62. doi: 10.1016/j.jcf.2014.05.008
Barr, H. L., Halliday, N., Cámara, M., Barrett, D. A., Williams, P., Forrester, D. L., et al. (2015). Pseudomonas aeruginosa quorum sensing molecules correlate with clinical status in cystic fibrosis. Eur. Respir. J. 46, 1046–1054. doi: 10.1183/09031936.00225214
Bredenbruch, F., Geffers, R., Nimtz, M., Buer, J., and Häussler, S. (2006). The Pseudomonas aeruginosa quinolone signal (PQS) has iron chelating activity. Environ. Microbiol. 8, 1318–1329. doi: 10.1111/j.1462-2920.2006.01025.x
Bryant, J. M., Grogono, D. M., Rodriguez-Rincon, D., Everall, I., Brown, K. P., Moreno, P., et al. (2016). Emergence and spread of a human-transmissible multidrug-resistant nontuberculous mycobacterium. Science 353, 751–757. doi: 10.1126/science.aaf8156
Cho, Y. J., Yi, H., Chun, J., Cho, S. N., Daley, C. L., Koh, W. J., et al. (2013). The genome sequence of ‘Mycobacterium massiliense’ strain CIP108297 suggests the independent taxonomic status of the Mycobacterium abscessus complex at the subspecies level. PLoS ONE 8:e81560. doi: 10.1371/journal.pone.0081560
Collier, D. N., Anderson, L., McKnight, S. L., Noah, T. L., Knowles, M., Boucher, R., et al. (2002). A bacterial cell to cell signal in the lungs of cystic fibrosis patients. FEMS Microbiol. Lett. 215, 41–46. doi: 10.1111/j.1574-6968.2002.tb11367.x
Cooley, J. W., Ohnishi, T., and Daldal, F. (2005). Binding dynamics at the quinone reduction (Qi) site influence the equilibrium interactions of the iron sulfur protein and hydroquinone oxidation (Qo) site of the cytochrome bc1 complex. Biochemistry 44, 10520–10532.
Costa, K. C., Bergkessel, M., Saunders, S., Korlach, J., and Newman, D. K. (2015). Enzymatic degradation of phenazines can generate energy and protect sensitive organisms from toxicity. mBio 6, 6 e01520-15. doi: 10.1128/mBio.01520-15
Costello, A., Reen, F. J., O’Gara, F., Callaghan, M., and McClean, S. (2014). Inhibition of co-colonizing cystic fibrosis-associated pathogens by Pseudomonas aeruginosa and Burkholderia multivorans. Microbiology 160, 1474–1487. doi: 10.1099/mic.0.074203-0
Deziel, E., Gopalan, S., Tampakaki, A. P., Lépine, F., Padfield, K. E., Saucier, M., et al. (2005). The contribution of MvfR to Pseudomonas aeruginosa pathogenesis and quorum sensing circuitry regulation: multiple quorum sensing-regulated genes are modulated without affecting lasRI, rhlRI or the production of N-acyl-L-homoserine lactones. Mol. Microbiol. 55, 998–1014. doi: 10.1111/j.1365-2958.2004.04448.x
Diggle, S. P., Matthijs, S., Wright, V. J., Fletcher, M. P., Chhabra, S. R., Lamont, I. L., et al. (2007). The Pseudomonas aeruginosa 4-quinolone signal molecules HHQ and PQS play multifunctional roles in quorum sensing and iron entrapment. Chem. Biol. 14, 87–96. doi: 10.1016/j.chembiol.2006.11.014
Filkins, L. M., Graber, J. A., Olson, D. G., Dolben, E. L., Lynd, L. R., Bhuju, S., et al. (2015). Co-culture of Staphylococcus aureus with Pseudomonas aeruginosa drives S. aureus towards fermentative metabolism and reduced viability in a cystic fibrosis model. J. Bacteriol. 197, 2252–2264. doi: 10.1128/JB.00059-15
Griffith, D. E., Aksamit, T., Brown-Elliott, B. A., Catanzaro, A., Daley, C., Gordin, F., et al. (2007). An official ATS/IDSA statement: diagnosis, treatment, and prevention of nontuberculous mycobacterial diseases. J. Am. Respir. Crit. Care Med. 175, 367–416. doi: 10.1164/rccm.200604-571ST
Hazan, R., Que, Y. A., Maura, D., Strobel, B., Majcherczyk, P. A., Hopper, L. R., et al. (2016). Auto poisoning of the respiratory chain by a quorum-sensing-regulated molecule favors biofilm formation and antibiotic tolerance. Curr. Biol. 26, 195–206. doi: 10.1016/j.cub.2015.11.056
Heeb, S., Fletcher, M. P., Chhabra, S. R., Diggle, S. P., Williams, P., and Cámara, M. (2011). Quinolones: from antibiotics to autoinducers. FEMS Microbiol. Rev. 35, 247–274. doi: 10.1111/j.1574-6976.2010.00247.x
Huse, H., and Whiteley, M. (2011). 4-Quinolones: smart phones of the microbial world. Chem. Rev. 111, 152–159. doi: 10.1021/cr100063u
Kehrmann, J., Wessel, S., Murali, R., Hampel, A., Bange, F.-C., Buer, J., et al. (2016). Principal component analysis of MALDI TOF MS mass spectra separates M. abscessus (sensu strictu) from M. massiliense isolates. BMC Microbiol. 16:24. doi: 10.1186/s12866-016-0636-4
Korgaonkar, A. K., Trivedi, U., Rumbaugh, K. P., and Whitley, M. (2013). Community surveillance enhances Pseudomonas aeruginosa virulence during polymicrobial infection. Proc. Natl. Acad. Sci. U.S.A. 110, 1059–1064. doi: 10.1073/pnas.1214550110
Korgaonkar, A. K., and Whiteley, M. (2011). Pseudomonas aeruginosa enhances production of an antimicrobial in response to N-acetylglucosamine and peptidoglycan. J. Bacteriol. 193, 909–917. doi: 10.1128/JB.01175-10
Leao, S. C., Tortoli, E., Euzeby, J. P., and Garcia, M. J. (2011). Proposal that Mycobacterium massiliense and Mycobacterium bolletii be untied and reclassified as Mycobacterium abscessus subsp. bolletii comb. nov., designation of Mycobacterium abscessus subsp. abscessus subsp. nov. and emended description of Mycobacterium abscessus. Int. J. Syst. Evol. Microbiol. 61, 2311–2313. doi: 10.1099/ijs.0.023770-0
Lee, Y., Kim, Y. J., Lee, J. H., Yu, H. E., Lee, K., Jin, S., et al. (2016). TatC-dependent translocation of pyoverdine is responsible for the microbial growth suppression. J. Microbiol. 54, 122–130. doi: 10.1007/s12275-016-5542-9
Lépine, F., Déziel, E., Milot, S., and Rahme, L. G. (2003). A stable isotope dilution assay for the quantification of the Pseudomonas quinolone signal in Pseudomonas aeruginosa cultures. Biochim. Biophys. Acta 1622, 335–342. doi: 10.1016/S0304-4165(03)00103-X
Lightbown, J. W., and Jackson, F. L. (1956). Inhibition of cytochrome systems of heart muscle and certain bacteria by the antagonists of dihydrostreptomycin: 2-alkyl-4-hydroxyquinoline N-oxides. Biochem. J. 63, 130–137.
Mashburn-Warren, L., Howe, J., Garidel, P., Richter, W., Steiniger, F., Roessle, M., et al. (2008). Interaction of quorum signals with outer membrane lipids: insights into prokaryotic membrane vesicle formation. Mol. Microbiol. 69, 491–502. doi: 10.1111/j.1365-2958.2008.06302.x
Müller, C., Birmes, F. S., Niewerth, H., and Fetzner, S. (2014). Conversion of the Pseudomonas aeruginosa quinolone signal (PQS) and related alkylhydroxyquinolines by Rhodococcus sp. strain BG43. Appl. Environ. Microbiol. 80, 7266–7274. doi: 10.1128/AEM.02342-14
Müller, C., Birmes, F. S., Rückert, C., Kalinowski, J., and Fetzner, S. (2015). Rhodococcus erythropolis BG43 genes mediating Pseudomonas aeruginosa quinolone signal degradation and virulence factor attenuation. Appl. Environ. Microbiol. 81, 7720–7729. doi: 10.1128/AEM.02145-15
Puigbò, P., Guzmán, E., Romeu, A., and Garcia-Vallvé, S. (2007). OPTIMIZER: a web server for optimizing the codon usage of DNA sequences. Nucleic Acids Res. 35, W126–W131. doi: 10.1093/nar/gkm219
Reen, F. J., Mooij, M. J., Holcombe, L. J., McSweeney, C. M., McGlacken, G. P., Morrissey, J. P., et al. (2011). The Pseudomonas quinolone signal (PQS), and its precursor HHQ, modulate interspecies and interkingdom behavior. FEMS Microbiol. Ecol. 77, 413–428. doi: 10.1111/j.1574-6941.2011.01121.x
Roux, A. L., Catherinot, E., Ripoll, F., Soismier, N., Macheras, E., Ravilly, S., et al. (2009). Multicenter study of prevalence of nontuberculous mycobacteria in patients with cystic fibrosis in France. J. Clin. Microbiol. 47, 4124–4128. doi: 10.1128/JCM.01257-09
Rüger, K., Hampel, A., Billig, S., Rücker, N., Suerbaum, S., and Bange, F.-C. (2014). Characterization of rough and smooth morphotypes of Mycobacterium abscessus isolates from clinical specimens. J. Clin. Microbiol. 52, 244–250. doi: 10.1128/JCM.01249-13
Smalley, N. E., An, D., Parsek, M. R., Chandler, J. R., and Dandekar, A. A. (2015). Quorum sensing protects Pseudomonas aeruginosa against cheating by other species in a laboratory co-culture model. J. Bacteriol. 197, 3154–3159. doi: 10.1128/JB.00482-15
Tan, J. L., Ngeow, Y. F., and Choo, S. W. (2015). Support from phylogenomic networks and subspecies signatures for separation of Mycobacterium massiliense form Mycobacterium bolletii. J. Clin. Microbiol. 53, 3042–3046. doi: 10.1128/JCM.00541-15
Tortoli, E., Kohl, T. A., Brown-Elliot, B. A., Trovato, A., Cardoso Leao, S., Garcia, M. J., et al. (2016). Emended description of Mycobacterium abscessus, Mycobacterium abscessus subs. abscessus, Mycobacterium abscessus subsp. bolletii and designation of Mycobacterium abscessus subsp. massiliense comb. nov. Int. J. Syst. Evol. Microbiol. 66, 4471–4479. doi: 10.1099/ijsem.0.001376
van den Ent, F., and Löwe, J. (2006). RF cloning: a restriction-free method for inserting target genes into plasmids. J. Biochem. Biophys. Methods 67, 67–74. doi: 10.1016/j.jbbm.2005.12.008
Keywords: Mycobacterium abscessus, Pseudomonas aeruginosa, quorum sensing, quorum quenching, Pseudomonas quinolone signal, alkylquinolone degradation
Citation: Birmes FS, Wolf T, Kohl TA, Rüger K, Bange F, Kalinowski J and Fetzner S (2017) Mycobacterium abscessus subsp. abscessus Is Capable of Degrading Pseudomonas aeruginosa Quinolone Signals. Front. Microbiol. 8:339. doi: 10.3389/fmicb.2017.00339
Received: 06 December 2016; Accepted: 17 February 2017;
Published: 02 March 2017.
Edited by:
Frank Schmidt, University of Greifswald, GermanyReviewed by:
Mika Tapio Tarkka, Helmholtz Centre for Environmental Research, GermanyDave Siak-Wei Ow, Bioprocessing Technology Institute, Singapore
Copyright © 2017 Birmes, Wolf, Kohl, Rüger, Bange, Kalinowski and Fetzner. This is an open-access article distributed under the terms of the Creative Commons Attribution License (CC BY). The use, distribution or reproduction in other forums is permitted, provided the original author(s) or licensor are credited and that the original publication in this journal is cited, in accordance with accepted academic practice. No use, distribution or reproduction is permitted which does not comply with these terms.
*Correspondence: Susanne Fetzner, ZmV0em5lckB1bmktbXVlbnN0ZXIuZGU=