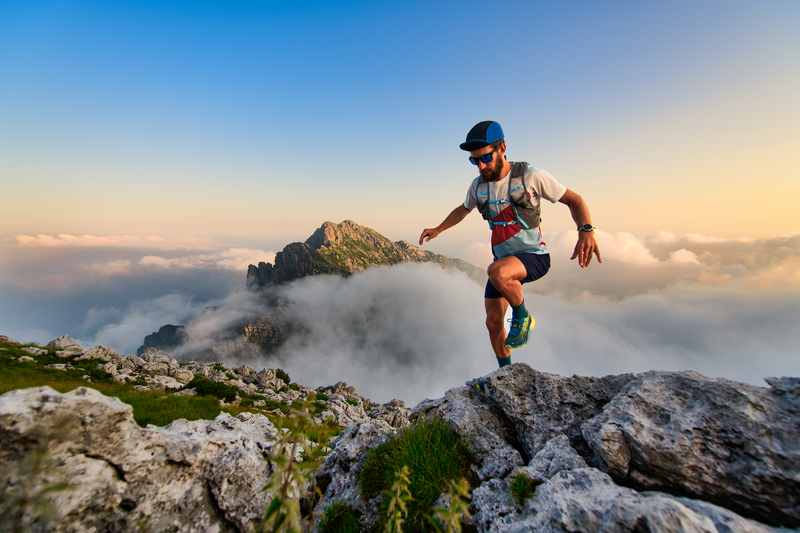
95% of researchers rate our articles as excellent or good
Learn more about the work of our research integrity team to safeguard the quality of each article we publish.
Find out more
ORIGINAL RESEARCH article
Front. Microbiol. , 07 February 2017
Sec. Aquatic Microbiology
Volume 8 - 2017 | https://doi.org/10.3389/fmicb.2017.00176
The hologenome theory of evolution (HTE), which is under fierce debate, presupposes that parts of the microbiome are transmitted from one generation to the next [vertical transmission (VT)], which may also influence the evolution of the holobiont. Even though bacteria have previously been described in early life stages of corals, these early life stages (larvae) could have been inoculated in the water and not inside the parental colony (through gametes) carrying the parental microbiome. How Symbiodinium is transmitted to offspring is also not clear, as only one study has described this mechanism in spawners. All other studies refer to incubators. To explore the VT hypothesis and the key components being transferred, colonies of the broadcast spawner species Mussismilia hispida were kept in nurseries until spawning. Gamete bundles, larvae and adult corals were analyzed to identify their associated microbiota with respect to composition and location. Symbiodinium and bacteria were detected by sequencing in gametes and coral planula larvae. However, no cells were detected using microscopy at the gamete stage, which could be related to the absence of those cells inside the oocytes/dispersed in the mucus or to a low resolution of our approach. A preliminary survey of Symbiodinium diversity indicated that parental colonies harbored Symbiodinium clades B, C and G, whereas only clade B was found in oocytes and planula larvae [5 days after fertilization (a.f.)]. The core bacterial populations found in the bundles, planula larvae and parental colonies were identified as members of the genera Burkholderia, Pseudomonas, Acinetobacter, Ralstonia, Inquilinus and Bacillus, suggesting that these populations could be vertically transferred through the mucus. The collective data suggest that spawner corals, such as M. hispida, can transmit Symbiodinium cells and the bacterial core to their offspring by a coral gamete (and that this gamete, with its bacterial load, is released into the water), supporting the HTE. However, more data are required to indicate the stability of the transmitted populations to indicate whether the holobiont can be considered a unit of natural selection or a symbiotic assemblage of independently evolving organisms.
The hologenome theory of evolution (HTE) considers the host and its associated microbiota genomes combined as one unit of natural selection in evolution (Rosenberg et al., 2007; Zilber-Rosenberg and Rosenberg, 2008). However, different aspects regarding the evolution of the genomes of hosts and their symbionts, as well as complex aspects of the evolutionary processes, remain under discussion (Leggat et al., 2007; Rosenberg et al., 2007; Moran and Sloan, 2015). In fact, assumptions underpinning this theory that the microbiome, or parts thereof, can be transmitted from one generation to the next and may influence the evolution of the coral holobiont have not been completely elucidated and remain under debate.
Corals depend on their associated microbiome (Rosenberg et al., 2007). Previous studies have indicated that the majority of broadcast spawning coral species do not vertically transfer their microalgal symbionts (Symbiodinium spp. Freudenthal, 196) (Freudenthal, 1962; Knowlton and Rohwer, 2003; Fabina et al., 2012) and other microbial symbionts (Sharp et al., 2010) via their gametes. They generally acquire symbionts as larvae or during post-settlement and metamorphosis as a juvenile polyp via horizontal transmission (from seawater) (Weis et al., 2001; Knowlton and Rohwer, 2003; Apprill et al., 2009). However, new data have reported the vertical transmission (VT) (from a parental colony) in broadcast spawning coral Montipora capitata, which transfers its Symbiodinium through its eggs (Padilla-Gamiño et al., 2012). Many studies have also confirmed that brooded coral transmit their Symbiodinium vertically from parent to offspring through their larvae (Fadlallah, 1983; Harrison and Wallace, 1990; Hirose et al., 2001; Thornhill et al., 2006). Less information is available about the transmission of other important coral microbial symbionts, such as bacteria. Sharp et al. (2011) demonstrated that Roseobacter spp. are vertically translocated from the parental colony to newly released larvae in the brooded coral Porites astreoides. On the other hand, some larvae seem to remain free of bacteria after fertilization, being capable of acquiring bacteria directly from the seawater during larval or polypoid stages (Apprill et al., 2009). Moreover, horizontal transmission of coral-associated bacteria in seven broadcast spawning coral species was reported by Sharp et al. (2010). Littman et al. (2009) and Lema et al. (2014) have also reported changes and uptake of bacteria in early stages of corals but not inside the oocytes.
Considering the controversial data regarding Symbiodinium transmission and the absolute lack of information concerning bacterial core diversity, dynamics and transmission in broadcast spawning corals, specifically the lack of data on this transmission through coral gametes, we here investigate and identify the (core) bacteria and Symbiodinium associated with different life stages of the Brazilian endemic coral Mussismilia hispida (Verrill, 1902). M. hispida is a hermaphrodite species that releases gametes in the water column in a seasonal spawning event that occurs over three consecutive months (Pires et al., 1999; Pires et al., 2016). Approximately 1/3 of the stony coral reef fauna of Brazil are endemic, and most are major builders of Brazilian reefs, including the Mussismilia genus.
Permission for sampling was obtained from the Brazilian Institute of Environment and Renewable Natural Resources (IBAMA)/Chico Mendes Institute for Biodiversity Conservation (ICMBio), permanent permission number 16942, in accordance with the Instruction Normative n° 03/2014 of System Authorization and Information on Biodiversity (SISBIO) and from local authorities of the Municipality Environmental Agency (SMMA)/Porto Seguro, Bahia, Brazil.
Approximately, seven M. hispida colonies were collected between latitudes 16°23′30″ S and 16°25′06″ S and longitudes 038°58′30″ W and 038°59′18″ W approximately 1 month (August, 2012) before the spawning event at “Recife de Fora,” Porto Seguro, Bahia, Brazil. These colonies were kept in tanks during the experiment. Seawater was collected directly from the beach and kept in a reservoir that distributed water to the tanks. Tanks had a 1000 L (1.1 m diameter) capacity and were kept in a room at 25°C with aerators to maintain oxygenation and circulation of the water. After this period, approximately 50% of the water was changed daily.
A spawning event occurred naturally on September 2012 (Pires et al., 2016). Some gamete bundles (approximately 15–20) of three coral colonies were collected immediately after spawning and kept at -20°C, while others were transferred to three different aquaria. Gamete fertilization occurred naturally inside each aquarium. All aquaria had an 80 L (40 cm × 40 cm × 50 cm) capacity and were kept in a room at 25°C with aerators to maintain oxygenation and circulation of the water. In the first 24–48 h, the water was not changed to minimize loss of sperm. After this period, approximately 50% of the water in each aquarium was changed daily. Corals, gametes, and coral planula were still exposed to natural day–night cycles. Approximately 25 coral planula larvae (3 and 5 days after fertilization) from the three coral colonies were collected, washed with sterile NaCl (3%) to minimize microbial contamination from seawater (to perform sequencing analysis) and kept at -20°C. Immediately after spawning, M. hispida fragments (approximately 30 mm diameter) from the three different colonies that released the bundles were also collected and kept at -20°C. Surrounding seawater samples were also collected from the tanks and aquaria during the spawning event and 3 and 5 days after spawning. In each case, approximately 1000 mL of seawater was filtered through a 0.22 μm filter. Sampling was performed in triplicate so that each colony was a replica, with a total of 21 samples. All samples were frozen in -80°C for molecular analyses. A summary of the experimental design that was used in this study can be seen in Supplementary Figure S1.
For light and transmission electron microscopy (TEM), embryonic and coral planula larvae stages coral (three samples from each bundle and planula larvae sample) were fixed in 4% formaldehyde and 2.5% glutaraldehyde, respectively, in 0.1 M cacodylate buffer pH 7.3 prepared with deionized water. To preserve the structure of M. hispida bundles, they were immobilized with agarose solution 3.5% (v/v). Then, the samples were post-fixed in buffered 1% OsO4 for 2 h, washed in the same buffer, dehydrated in an acetone series (30, 50, 70, 90, and 100% 3x), embedded in Polybed resin, and polymerized for 72 h at 60°C.
Thin (2 μm) and ultra-thin (70 nm) sections were obtained in a Leica ultramicrotome (Leica Microsystems, Vienna, Austria). Thin sections were stained toluidine blue (1%) and imaged with a Zeiss Axioimager (Carl Zeiss, Jena, Germany) optical light microscope. Ultra-thin sections were placed in a copper grids, stained with uranyl acetate and lead citrate and imaged with an FEI Morgagni (FEI Company, Eindhoven, The Netherlands) TEM operating at 80 kV.
Coral samples were crushed and homogenized (3 polyp fragments from adult corals, approximately 15–20 bundles from each coral colony, and approximately 25 coral planula larvae, at 3 and 5 days a.f, composed samples from the three coral colonies) and were collected from each coral sample. The DNA (water and coral samples) was extracted using the PowerSoil® DNA Isolation Kit (MoBio Laboratories, Carlsbad, CA, USA) following a modification of the method described by Sunagawa et al. (2010).
The amplification of a specific region of the 28S rRNA gene of Symbiodinium was performed using the primers D1D2-F and D1D2-R (Loh et al., 2001) in a solution containing 1X PCR buffer, 0.2 mM dNTPs, 2.5 mM MgCl2, 2.5 U of recombinant Taq DNA polymerase (Promega, Madison, WI, USA), 10–40 ng of total DNA, 0.5 μM of each primer and sterile Milli-Q water in a final volume of 15 μl. The reaction was performed in a thermocycler (Mastercycler Gradient, Eppendorf, Hamburg, Germany) with the following conditions: initial denaturing at 94°C for 5 min; 1 cycle at 94°C for 1 min, 56°C for 45 s and 72°C; 29 cycles at 92°C for 1 min, 56°C for 1 min and 72°C for 45 s; and a final extension at 72°C for 10 min.
Polymerase chain reaction products were cloned using the pJET 1.2 vector (CloneJET PCR Cloning Kit #K1231, Thermo Scientific) and transformed into Escherichia coli competent cells (Single Step – KRX – Competent Cells, Promega) with the TransformAid Bacterial Transformation Kit (#K2710 Thermo Scientific) according to the manufacturer’s instructions. Approximately 50 clones were re-amplified for the 28S rRNA gene fragments and digested using the restriction enzyme Taq I (Promega, cutting site 5′T/CGA3′) to perform a screening analysis using RFLPs (Restriction Fragment Length Polymorphisms). The RFLPs enabled the selection of distinct Symbiodinium lineages for sequencing. Digestions were performed in a solution containing the enzyme buffer 1X, 4 μl of the PCR product, 5 U of Taq I and sterile Milli-Q water in a final volume of 20 μl. Reactions were incubated at 65°C for approximately 16 h. All digestion products were visualized by agarose gel electrophoresis 2% (v/v) in 0.5X TBE buffer, stained with ethidium bromide and visualized under UV light. Symbiodinium clades were identified by comparisons with RFLP patterns of pure cultures of Symbiodinium that commonly occur in the Atlantic (clades A, B, C, and D), obtained by donation from Prof. Mark Warner, University of Delaware, USA.
After selection by RFLP, clones from each clade were amplified and purified by the automatic robot epMotion 5075 (Eppendorf, Hamburg, Germany) and directly sequenced by Sanger method (Sanger et al., 1977) in the automatic sequencer ABI 3500 (Applied Biosystems). Sequences were manually trimmed removing the low quality ends and primers using the SeqMan 7 software (DNAStar, Inc.). Sequences with a final length of 601 bp were obtained. All sequences were deposited in the GenBank database with the accession number (KX459520-KX459562).
Specific regions of the gene encoding the 16S rRNA gene were amplified using the primers U968f GC and L1401r (Heuer and Smalla, 1997) in a solution containing 1X PCR buffer, 0.2 mM dNTPs, 2.5 mM MgCl2, 2.5 U of recombinant Taq DNA polymerase (Promega, Madison, WI, USA), 10–40 ng of total DNA, 200 μmol of each primer and sterile Milli-Q water in a final volume of 50 μl. The reaction was performed in a thermocycler (Mastercycler Gradient, Eppendorf, Hamburg, Germany) with the following conditions: initial denaturing at 94°C for 3 min; 35 cycles at 94°C for 1 min, 55°C for 1 min and 72°C for 1 min; and a final extension at 72°C for 10 min. The denaturing gradient gel elecrtophoresis (DGGE) gels (45–65% urea and formamide) were prepared with a solution of polyacrylamide (6%) in Tris-acetate (pH 8.3). Electrophoresis was performed in Tris-acetate-EDTA buffer at 60°C at a constant voltage of 75 V for 16 h. The DGGE gels were stained with SYBR Green (Invitrogen, Carlsbad, CA, USA) and visualized using a Storm 860 Imaging System (GE Healthcare, Milwaukee, WI, USA).
Based on the obtained bacterial profiles, samples with different profiles were selected to perform the sequencing approach. The 16S rRNA gene V4 variable region PCR primers 515F/806R (Caporaso et al., 2011) were used in a single-step 30 cycle PCR using the HotStarTaq Plus Master Mix Kit (Qiagen, USA) under the following conditions: 94°C for 3 min, followed by 28 cycles (five cycle used on PCR products) of 94°C for 30 s, 53°C for 40 s and 72°C for 1 min, followed by a final elongation step at 72°C for 5 min. Sequencing was performed at MR DNA (www.mrdnalab.com, Shallowater, TX, USA) in an Ion Torrent PGM following the manufacturer’s guidelines.
The QIIME (Caporaso et al., 2010b) software package was used to process the raw sequence data. In brief, sequences were trimmed using the following parameters: quality score > 25, sequence length > 200, maximum length of homopolymer of 6 and 0 mismatched bases in the primers and barcodes.
The remaining high-quality sequences were binned into operational taxonomic units (OTUs) at 97% sequence identity USEARCH 6.1 methodology (version v6.1.544) followed by selection of a representative sequence. Chimeric sequences were also identified using USEARCH 6.1 methodology (version v6.1.544) (Edgar, 2010) and removed. A representative sequence for each phylotype was aligned against the Greengenes database (Desantis et al., 2006) using PyNAST (Caporaso et al., 2010a), with sequences classified using the Greengenes taxonomy via RDP classifier (Wang et al., 2007). Before further analysis, singletons and chloroplast plastid, mitochondrial and archaeal sequences were removed from the dataset. For all OTU-based analyses, the original OTU table was rarified to a depth of 14500 sequences per sample to minimize the effects of sampling effort on the analysis. The QIIME package was also used to generate weighted UniFrac distance matrices (Lozupone et al., 2006) and α-diversity metrics, including richness and diversity indices. All sequences were deposited in the NCBI Sequence Read Archive database with the accession numbers (SRR3740771–SRR3740793).
The core microbiome of gametes, planula larval, and parental coral colony was identified using QIIME and determined by plotting OTU abundance. The coral bacterial core was represented by OTUs that were shared by 100% of the samples.
The DGGE band profiles were digitalized and converted into data matrices using the Bionumerics v6.0 package (Applied Maths) according to the manufacturer’s instructions. The matrices were ordered by NMS (Kruskal, 1964; Mather, 1976) using a Bray–Curtis distance matrix. To analyze the difference between profiles and composition of bacterial communities, we used non-metric scaling (NMS) with Past 3.x Software (Hammer et al., 2001). To assess the variation among different samples (parental colony, bundles and larvae), we used a permutational multivariate analysis of variance (PERMANOVA; Kelly et al., 2015) using Past 3.x Software (Hammer et al., 2001).
Symbiodinium diversity was assessed using the resulting 28S rRNA gene sequences and performing searches using the Basic Local Alignment Search Tool – BLAST tool1 (Altschul et al., 1990). Additionally, phylogenetic reconstruction analysis was performed using the 28S rRNA gene sequences from this study together with representatives of each of the Symbiodinium clade that were found by BLAST searches (downloaded from the NCBI database). Sequence alignments were performed using MEGA 5 software (Tamura et al., 2011) with the ClustalW tool (Larkin et al., 2007), and phylogenetic analysis was conducted with the Maximum-Likelihood method (ML) recovered in the PhyML platform (Guindon et al., 2010). The phylogeny were reconstructed with the substitution model GTR+I+G [General Time Reversible with Invariable sites proportion fixed and Gamma-distributed rates (Buckley et al., 2001)], and phylogenetic clade support was calculated by the 1000 bootstrap resampling method. ML trees were edited using FigTree 1.4.2 software2.
The 16S rRNA gene-based PCR-DGGE and next-generation sequencing analyses of the bacterial assemblages associated with three parental coral colonies, bundles (gametes) and planula larvae stages and seawater samples revealed a clear clustering of patterns according to the sample. Non-metric dimensional scaling (NMDS) of the data clearly indicated a dichotomy in the clustering, with one major cluster being subdivided in two subclusters (Figures 1A,B). The patterns derived from the gametes (bundles) and representatives of the coral planula larvae stages were closely related to those from the parental coral, in contrast to the seawater samples, which clustered apart. PERMANOVA confirmed the results of the NMDS analysis, demonstrating that the bacterial assemblages varied significantly among the parental coral, bundles, coral planula larvae stages and water-derived patterns (p = 0.01; Pseudo-F = 9.46 and 8.94, respectively) (Figures 1A,B). The results from the pairwise PERMANOVA suggested that the microbial communities of the bundles and coral planula larvae stages were significantly related to those from the parental coral microbial communities (Supplementary Table S1). As the microbial community structure of coral planula larvae (3 and 5 days a.f.) were very similar, only coral planula larvae 5 days a.f. were used for sequencing (Figure 1A). Bacterial community richness decreases in coral and water samples between spawning time and after 5 days after spawning (Supplementary Figure S2).
FIGURE 1. NMS (Bray–Curtis) plot of bacterial communities associated to different life stages of Mussismilia hispida and surrounding seawater based on PCR-DGGE (A) and high throughput sequencing (B) data (n = 3). Contours are based on significant pairwise PERMANOVA results (p = 0.01).
The phylogenetic reconstruction analysis of the 28S region detected clades B, C, and G in the parental colony of M. hispida, while only clade B was detected in gamete bundles and representatives of the coral planula larvae stages (Figure 2). Additionally, the parental colony seemed to harbor three distinct lineages of B that differ from the sequences downloaded from the NCBI database. The phylogenetic analysis also showed that the bundle and coral planula larvae harbor only one of the lineages (clade B) found in the parental colony (group 1 in Figure 2).
FIGURE 2. Maximum likelihood phylogenetic reconstruction of Symbiodinium inferred from partial 28S rRNA gene sequences, including sequences obtained from M. hispida parental colony, gametes and planula larvae stages and sequences downloaded from the NCBI database (see GenBank accession numbers in Supplementary Material). Sequences from Prorocentrum spp. was used as outgroup. G0d: bundles at the spawning event, L5d: coral planula larvae, 5 days a.f., and MH: adult colonies of M. hispida. Bootstrap support values are indicated at the base of branches.
The coral bacterial “core” (shared microorganisms among all samples) represented 10.5% of the total microbial diversity in coral adult colonies and 60.7% in bundles stage and increased to 84.7% in coral planula larvae 5 days a.f. (Supplementary Figure S3). The genera Burkholderia, Pseudomonas, Acinetobacter, Ralstonia, Inquilinus, and Bacillus composed the coral bacterial “core” at different life stages of M. hispida (Figure 3; Supplementary Table S2). Burkholderia was the most abundant genus associated with the early stages of this coral life, representing approximately 75% of the gamete microbiome and increasing by 10% in coral planula larvae 5 days a.f. On the other hand, the relative abundance of Acinetobacter spp. decreased from 15% (gamete stage) to less than 5% (coral planula larvae 5 days a.f.). The abundance of the genera Ralstonia and Inquilinus remained stable, both at approximately 10%, from the time of spawning (in the gamete bundles) until the coral planula larvae 5 days a.f.
FIGURE 3. Relative abundance of the core microbiome genera from different M. hispida life stages provided by 16S rRNA gene high throughput (Ion Torrent PGM) sequencing data. G0d: bundles at the spawning event, L5d: coral planula larvae, 5 days a.f., and MH: adult colonies of M. hispida.
The evaluation of the bundle sections (Supplementary Figure S4) did not reveal the presence of Symbiodinium or bacterial cells. However, cells identified as Symbiodinium-like cells but that were smaller than Symbiodinium cells were observed in the peripheral region of the female gametes (eggs) (Figures 4A,B). These cells were found in the endodermis of the coral planula larvae 5 days a.f. No bacteria were observed inside the eggs (Figures 4A,B) or close to the spermatic cysts (Figures 4C,D). In the coral planula larvae stages (Figure 5), some bacterium-like structures were observed in the epidermis.
FIGURE 4. Ultrastructure of male (A,B) and female (C,D) gametes of M. hispida, highlighting the presumptive Symbiodinium-like cells in the oocytes. (D) Is a zoom of (C). White arrow indicate the male gametes, orange arrowheads the Symbiodinium-like cells and white circles indicate mitochondria.
FIGURE 5. Light microscopy micrograph of a thin section of M. hispida coral planula larvae (B) is a zoom of (A), 5 days a.f., highlighting the Symbiodinium-like cells into the endodermis (B). EN: endodermis, EC: ectodermis. White arrows indicate the Symbiodinium-like cells. White circles indicate bacteria-like structures.
To the best of our knowledge, this is the first report that shows the bacterial core composition and dynamics at early stages of coral life, including gametes, as discussed below. We showed that the spawner coral M. hispida can vertically transfer its bacteria and Symbiodinium cells.
Considering the transmission of bacterial symbionts, there is no consensus or pattern on the transmission of these microorganisms. A study on the gametes and planulae larvae of other seven broadcast spawner coral species did not find any bacterial association in these life stages (Sharp et al., 2010). Thus, these corals may acquire their associated microbiota from the seawater (horizontal transmission). On the other hand, Ceh et al. (2012) investigated the microbiome of three different broadcast spawner and brooder corals (Acropora tenuis, Pocillopora damicornis, and Tubastrea faulkneri) before and after spawning and indicated that the bacterial diversity increased after spawning in all coral species. The authors highlighted that Roseobacter spp. was an important genus transmitted during coral reproduction. They also observed a decrease in some key groups that could have evolved through bacterial VT. However, the authors could not prove the occurrence of VT because only adult corals were sampled.
Here, not only adult colonies but also gametes and coral planula larvae at 3 and 5 days a.f. were sampled. The sequencing data showed that the M. hispida microbiome was composed by bacteria and Symbiodinium cells, even in gametes, although we could not verify the presence of bacterial and Symbiodinium cells in association with the (female and male) gametes of M. hispida through microscopy. Sequencing data also showed a stable core bacterial microbiome from the gametes to planula larvae stages that was also present in the parental coral colony. In addition, we verified that the stable microbiome represents a greater proportion of the associated bacteria in gametes and planula larvae stages. In contrast, the core microbiome represents a low proportion in the adult colonies, indicating microbial transmission once these microorganisms have left the parental colony for the bundles. The core microbiome presence could indicate a potential key function of this group in early life stages of coral development. Therefore, the obtained data suggest that this coral could use parallel and different strategies for microbiome acquisition. Considering our sequencing data, the bacterial core is transmitted vertically, potentially via the mucus that visually binds the gametes together in a bundle (observed in vivo). Most likely, the offspring can be partly colonized post-spawning through the uptake of microbial associates released by the parental colony into the surrounding seawater, as previously described (Apprill et al., 2009; Sharp et al., 2011; Ceh et al., 2012, 2013), and partly colonized by parental colonies, which transfer their bacterial core by seeding the mucus with parental-derived bacterial symbionts, as indicated by our data.
Thus, while Symbiodinium cells could be transmitted inside gametes, as shown in literature and suggested by our molecular data, the core bacterial community – being present in abundance within the mucus, which will form the bundle – could be vertically transmitted ‘from outside’ of the gametes, inside the coral. This does not exclude that some bacteria are eventually transmitted inside the gamete or even inside the Symbiodinium cells being transmitted in the eggs; we could not detect these bacteria due to poor resolving power of our methods. The presence of bacterial symbionts living in the Symbiodinium was previously reported by Ainsworth et al. (2015) in tissues of adult corals. On the other hand, Symbiodinium cells, which can be transmitted inside oocytes, can also be acquired from seawater in the planula larvae stage or even by coral adults (Boulotte et al., 2016).
Herein, we propose the model presented in Figure 6 for the transmission of microorganisms in this coral species. M. hispida is a broadcaster spawner and a hermaphroditic species; therefore, its eggs and spermatic cysts develop inside the same mesenteries, but oogenesis and spermatogenesis start at different periods, with oogenesis beginning several months before spermatogenesis (Pires et al., 1999). During gamete development and spawning, the gametes are initially in the gastrodermis, migrating to the mesoglea during the vitellogenesis period. When the eggs and spermatic cysts are mature, the rupture of the mesentery wall occurs, and the bundles that formed (eggs, cysts, and mucus) reach the gastrovascular cavity, passing through the mouth to reach the surface (Pires et al., 1999). This mural contains knowledge already available in the literature (Apprill et al., 2009; Sharp et al., 2011; Ceh et al., 2012, 2013) and includes our new data, providing this mural a very comprehensive view of coral microbiome transmission in M. hispida, potentially applicable to other coral species.
FIGURE 6. Proposed model for the different strategies of microbiome transmission in the broadcast spawning coral M. hispida. This model uses the knowledge already available in literature (Apprill et al., 2009; Sharp et al., 2011; Ceh et al., 2012, 2013) and includes our new information, providing this mural with a comprehensive view of the microbiome transmission (1) Gametes are located inside the mesentery without any symbiotic association (Symbiodinium or bacterial cells); (2) Gametes leave the mesentery, and Symbiodinium cells could be acquired by the oocytes; (3) During the bundle formation the spermatic cysts and eggs containing Symbiodinium cells are surrounded by the mucus full of bacterial cells; (4) Bacterial cells can be also acquired during fertilization. It is also possible that bacterial cells could be transmitted inside oocytes, or even inside Symbiodinium cells.
Regarding the preliminary survey of the dominant Symbiodinium clades diversity, according to Picciani-de-Souza et al. (2016), M. hispida colonies from Bahia, Brazil, shelter only Symbiodinium belonging to clade C, while possessing clades A and B at other locations along the Brazilian coast. In contrast, only clades B, C, and G were found in our parental colonies, which suggests that this coral can acquire new clades or have them in lower abundance under normal field conditions, including the rare association with clade G (commonly found in Foraminifera hosts (Pochon et al., 2001; Coffroth and Santos, 2005). However, when conditions change (i.e., aquarium confinement or pre-spawning event), the abundance of less abundant clades can be enhanced or simply detected by PCR, a technique that detects mostly dominant sequences. Additionally, although Symbiodinium clades are transferred vertically, clade selection seems to occurs early, since only clade B was found in the embryonic and planula larvae stages. Abrego et al. (2009) demonstrated the presence of only Symbiodinium of clade C in parental colonies of acroporid corals, whereas only Symbiodinium of clades A or D were found in the larvae, though the mechanisms behind these associations remain unknown. Our data corroborate the findings of Padilla-Gamiño et al. (2012), demonstrating that Symbiodinium can be transmitted vertically, even in coral species where fertilization of gametes and larval development occur in seawater. Boulotte et al. (2016) demonstrated that Symbiodinium cells can also be acquired from the environment by adult corals, and Hester et al. (2016) indicated the presence of a stable core microbiome comparing different coral species and locations but also demonstrated that coral–microbiome associations are not exclusive and therefore could not be considered heritable as a unit. Altogether, these results reinforce the idea that the mechanisms for coral–microbial symbiosis could be more flexible and dynamic than first thought (Boulotte et al., 2016).
Not only are the symbiotic mechanisms not yet fully understood, but there is also a great paucity of knowledge on the potential beneficial traits of microbial holobiont members that are vertically or horizontally transferred during spawning and this remains an important issue for ongoing studies. Antimicrobial activity of symbiont microbes may serve as a protective function for coral spawned gametes or larvae. As noted by Marquis et al. (2005), Montipora digitata seeds its eggs inside bundles with Symbiodinium, which might suggest a potentially protective role for Symbiodinium-derived metabolites. A recent study of Lin et al. (2015) found biochemical complementarity between the genomes of the symbiont and the host, which is indicative of coevolution. These data seem to underpin the need to guarantee the transfer of the microbiota, even if complementary strategies of transmission are needed. Most studies have indicated Roseobacter and Alteromonas as genera that occur in the early stages of coral life (Sharp et al., 2011; Ceh et al., 2012, 2013). However, we found here that at least six genera are associated with these stages, with Burkholderia sp. being the most abundant genus (approximately 75–85%). Recent studies have suggested that members of this genus are also vertically transmitted in plants (Sphagnum spp.) (Bragina et al., 2013). Several Burkholderia species are capable of fixing nitrogen and degrading aromatic compounds (Suárez-Moreno et al., 2012), whereas others strongly interact with fungi and/or cold-adapted plants. The presence of N-fixing bacteria was previously observed in the initial stages of coral life (Lema et al., 2014). Thus, bacteria can potentially benefit the coral host, since these features seem to be important to coral symbionts (Santos et al., 2014) and to the protection of coral from environmental impact (Santos et al., 2015).
Some studies have described a Pseudomonas sp. isolated from corals with antimicrobial and anti-pathogenic potentials, which could be important to regulate the presence of some microorganism or prevent the establishment of pathogens during early stages of coral life (Radjasa et al., 2007; Bakkiyaraj et al., 2013). Members of the genus Pseudomonas are also genetically very plastic, including different abilities that are important to corals, such us nitrogen fixation, pollutant degradation, use of different carbon sources to provide nutrients and production of antibiotics (Garcia-Valdes et al., 1988; Das and Mukherjee, 2007; Rojo, 2010). These features seem to provide clues about the importance of this genus being transmitted to coral offspring.
Corals from the Southwestern Atlantic are located in waters with higher sedimentation rates compared to those of the Pacific Ocean (Ricardo et al., 2015). Suspended sediment can represent a risk to the reproductive processes of corals because high sediment concentrations in suspension are capable of removing sperm from the seawater surface during reproduction events (Ricardo et al., 2015). This would decrease the contact time between the male and female gametes and might compromise recruitment rates and, in turn, have a negative population effect (Ricardo et al., 2015). We believe that corals from the Brazilian coast have developed specific strategies to live and survive in turbid waters. In its embryonic and planula larvae stages, the coral M. hispida, besides being able to transfer Symbiodinium cells to its offspring, also hosts bacterial genera that have not yet been described as part of the microbiome of other coral species.
The specific microorganisms transmitted by coral from generation to generation are assumed to be key to the sustenance of the coral life. It is likely that such transmission reflects some level of selection and influences host fitness. The HTE hypothesizes that the holobiont is the unit of selection in evolution and that any genetic variation in the holobiont, occurring in the host and/or in the microbial symbiont genomes, in cases of selective advantage is successfully transmitted to the offspring. This theory places as much emphasis on cooperation as it does on competition according to the principle of ‘survival of the fittest’ or as a result of stochastic events (Rosenberg et al., 2009). Even though our data indicate VT in M. hispida, we agree that this is not enough to assume co-evolution, as stated by other authors, including Hester et al. (2016), although it can be considered as additional evidence. More data are required to indicate whether the stable transmitted populations and the hosts are indeed units of natural selection or symbiotic assemblages of independently evolving organisms. The organisms that constitute the holobiont need to fit together in a tight relationship (including the vertical microbiome transmission demonstrated here in M. hispida) so that the holobiont adapts to the environment.
The spawner coral M. hispida can transfer its bacterial and Symbiodinium cells to its offspring through VT. The core bacterial populations found in the bundles, planula larvae and parental colonies were identified as members of the genera Burkholderia, Pseudomonas, Acinetobacter, Ralstonia, Inquilinus, and Bacillus. The abundance of these populations was shown to be dynamic and related to coral life stages. While the stable microbiome represents a greater proportion of the associated bacteria in embryonic and planula larvae stages, the core microbiome represents a low proportion in the adult colonies. This result suggests that M. hispida has parallel and different strategies for its microbiome acquisition. We hypothesized that coral offspring can be partly post-spawning colonized through the uptake of microbial associates released by the parental colony into the surrounding seawater and partly colonized by parental colonies, which transfer their bacterial core and Symbiodinium cells by seeding the mucus and the gametes, respectively. Being dynamic, the coral holobiont may incorporate part of its associated microbiota from the water column every time an adaptation to new environmental conditions is required, and this plasticity and capacity to modulate the microbiome uptake could be an important strategy of corals that could explain their billions of years ancient existence and persistence.
Study conception and design: RP, AR, DL, HS, CC, DP. Acquisition and identification of coral samples: DL, CC, DP. Acquisition of data (experimental development): DL, PL, UL, AG, CZ. Analyses and interpretation of data: RP, AR, DL, PL, UL, AG, JvE, CZ, CC, DP. Drafting of the manuscript: RP, DL. Critical revision: all authors. Financial support: RP, AR, UL, CC, CZ.
This work was supported by the National Council for Scientific and Technological Development (CNPq), the National Council for the Improvement of Higher Education (CAPES) and the Carlos Chagas Filho Foundation for Research Support of Rio de Janeiro State (FAPERJ).
The authors declare that the research was conducted in the absence of any commercial or financial relationships that could be construed as a potential conflict of interest.
The authors would like to thank Prof. Ruth Gates for her valuable comments and suggestions. The authors would also like to thank Coral Vivo and its sponsors (Arraial d’Ajuda Eco Parque and Petrobras) for the logistical support and the use of its research base. We also thank the National Council for Scientific and Technological Development (CNPq), the National Council for the Improvement of Higher Education (CAPES) and the Carlos Chagas Filho Foundation for Research Support of Rio de Janeiro State (FAPERJ) for their support of this work, and the Secretary for the Environment of the Municipality of Porto Seguro for the collection license.
The Supplementary Material for this article can be found online at: http://journal.frontiersin.org/article/10.3389/fmicb.2017.00176/full#supplementary-material
FIGURE S1 | Scheme showing the experimental strategy used in this study. Boxes in gray scale: collected samples are represented from embryonic stage (bundles, spawning time) to coral planula larvae (3 and 5 days a.f.). Blue rectangles: techniques used in this study; bacterial communities profile and composition were evaluated by PCR-DGGE and sequencing, respectively, and zooxanthellae diversity was evaluated by PCR and cloning/sequencing.
FIGURE S2 | Richness indexes for bacterial communities associated with different life stages of Mussismilia hispida and surrounding seawater. (A) Phylogenetic distance, (B) Chao and (C) Observed OTUs. W0d: seawater at the spawning event, W5d: seawater 5 days after the spawning event, G0d: Bundles at the spawning event, L5d: coral planula larvae, 5 days a.f., and MH: adult colonies of M. hispida.
FIGURE S3 | Relative abundance of the variable and core microbiome from different M. hispida life stages, according to 16S rRNA gene high throughput (Ion Torrent PGM) sequencing data. G0d: bundles at the spawning event, L5d: coral planula larvae, 5 days a.f., and MH: adult colonies of M. hispida.
FIGURE S4 | Light microscopy micrograph of a thin section of M. hispida bundles, highlighting male and female gametes. F: female gametes. M: spermatic cysts.
TABLE S1 | Permutational statistical (PERMANOVA) analyses of DGGE and sequencing data to assess the water and Mussismilia hispida microbiome.
TABLE S2 | Bacterial core community of different life stages of M. hispida at OTU level. G0d: bundles at the spawning event, L5d: coral planula larvae, 5 days a.f., and MH: adult colonies of M. hispida.
Abrego, D., Van Oppen, M. J. H., and Willis, B. L. (2009). Highly infectious symbiont dominates initial uptake in coral juveniles. Mol. Ecol. 18, 3518–3531. doi: 10.1111/j.1365-294X.2009.04275.x
Ainsworth, T. D., Krause, L., Bridge, T., Torda, G., Raina, J.-B., Zakrzewski, M., et al. (2015). The coral core microbiome identifies rare bacterial taxa as ubiquitous endosymbionts. ISME J. 9, 2261–2274. doi: 10.1038/ismej.2015.39
Altschul, S. F., Gish, W., Miller, W., Myers, E. W., and Lipman, D. J. (1990). Basic local alignment search tool. J. Mol. Biol. 215, 403–410. doi: 10.1016/S0022-2836(05)80360-2
Apprill, A., Marlow, H. Q., Martindale, M. Q., and Rappé, M. S. (2009). The onset of microbial associations in the coral Pocillopora meandrina. ISME J. 3, 685–699. doi: 10.1038/ismej.2009.3
Bakkiyaraj, D., Sivasankar, C., and Pandian, S. K. (2013). Anti-pathogenic potential of coral associated bacteria isolated from gulf of mannar against Pseudomonas aeruginosa. Indian J. Microbiol. 53, 111–113. doi: 10.1007/s12088-012-0342-3
Boulotte, N. M., Dalton, S. J., Carroll, A. G., Harrison, P. L., Putnam, H. M., Peplow, L. M., et al. (2016). Exploring the Symbiodinium rare biosphere provides evidence for symbiont switching in reef-building corals. ISME J. 10, 2693–2701. doi: 10.1038/ismej.2016.54
Bragina, A., Cardinale, M., Berg, C., and Berg, G. (2013). Vertical transmission explains the specific Burkholderia pattern in Sphagnum mosses at multi-geographic scale. Front. Microbiol. 4:394. doi: 10.3389/fmicb.2013.00394
Buckley, T. R., Simon, C., and Chambers, G. K. (2001). Exploring among-site rate variation models in a maximum likelihood framework using empirical data: effects of model assumptions on estimates of topology, branch lengths, and bootstrap support. Syst. Biol. 50, 67–86. doi: 10.1080/10635150116786
Caporaso, J. G., Bittinger, K., Bushman, F. D., Desantis, T. Z., Andersen, G. L., and Knight, R. (2010a). PyNAST: a flexible tool for aligning sequences to a template alignment. Bioinformatics 26, 266–267. doi: 10.1093/bioinformatics/btp636
Caporaso, J. G., Kuczynski, J., Stombaugh, J., Bittinger, K., Bushman, F. D., Costello, E. K., et al. (2010b). QIIME allows analysis of high-throughput community sequencing data. Nat. Methods 7, 335–336. doi: 10.1038/nmeth.f.303
Caporaso, J. G., Lauber, C. L., Walters, W. A., Berg-Lyons, D., Lozupone, C. A., Turnbaugh, P. J., et al. (2011). Global patterns of 16S rRNA diversity at a depth of millions of sequences per sample. Proc. Natl. Acad. Sci. U.S.A. 108, 4516–4522. doi: 10.1073/pnas.1000080107
Ceh, J., Keulen, M., and Bourne, D. G. (2013). Intergenerational transfer of specific bacteria in corals and possible implications for offspring fitness. Microb. Ecol. 65, 227–231. doi: 10.1007/s00248-012-0105-z
Ceh, J., Raina, J.-B., Soo, R. M., Van Keulen, M., and Bourne, D. G. (2012). Coral-bacterial communities before and after a coral mass spawning event on ningaloo reef. PLoS ONE 7:e36920. doi: 10.1371/journal.pone.0036920
Coffroth, M. A., and Santos, S. R. (2005). Genetic diversity of symbiotic dinoflagellates in the genus Symbiodinium. Protist 156, 19–34. doi: 10.1016/j.protis.2005.02.004
Das, K., and Mukherjee, A. K. (2007). Crude petroleum-oil biodegradation efficiency of Bacillus subtilis and Pseudomonas aeruginosa strains isolated from a petroleum-oil contaminated soil from North-East India. Bioresour. Technol. 98, 1339–1345. doi: 10.1016/j.biortech.2006.05.032
Desantis, T. Z., Hugenholtz, P., Larsen, N., Rojas, M., Brodie, E. L., Keller, K., et al. (2006). Greengenes, a chimera-checked 16S rRNA gene database and workbench compatible with ARB. Appl. Environ. Microbiol. 72, 5069–5072. doi: 10.1128/AEM.03006-05
Edgar, R. C. (2010). Search and clustering orders of magnitude faster than BLAST. Bioinformatics 26, 2460–2461. doi: 10.1093/bioinformatics/btq461
Fabina, N. S., Putnam, H. M., Franklin, E. C., Stat, M., and Gates, R. D. (2012). Transmission mode predicts specificity and interaction patterns in coral-Symbiodinium networks. PLoS ONE 7:e44970. doi: 10.1371/journal.pone.0044970
Fadlallah, Y. H. (1983). Sexual reproduction, development and larval biology in scleractinian corals. Coral Reefs 2, 129–150. doi: 10.1111/j.1365-2656.2008.01387.x
Freudenthal, H. D. (1962). Symbiodinium gen. nov. and Symbiodinium microadriaticum sp. nov., a zooxanthella: taxonomy, life cycle, and morphology. J. Protozool. 9, 45–52. doi: 10.1111/j.1550-7408.1962.tb02579.x
Garcia-Valdes, E., Cozar, E., Rotger, R., Lalucat, J., and Ursing, J. (1988). New naphthalene-degrading marine Pseudomonas strains. Appl. Environ. Microbiol. 54, 2478–2485.
Guindon, S., Dufayard, J.-F., Lefort, V., Anisimova, M., Hordijk, W., and Gascuel, O. (2010). New algorithms and methods to estimate maximum-likelihood phylogenies: assessing the performance of PhyML 3.0. Syst. Biol. 59, 307–321. doi: 10.1093/sysbio/syq010
Hammer,Ø, Harper, D. A. T., and Ryan, P. D. (2001). PAST: paleontological statistics software package for education and data analysis. Palaeontol. Electron. 4:9.
Harrison, P., and Wallace, C. (1990). Reproduction, dispersal and recruitment of scleractinian corals. Ecosyst. world 25, 133–207.
Hester, E. R., Barott, K. L., Nulton, J., Vermeij, M. J. A., and Rohwer, F. L. (2016). Stable and sporadic symbiotic communities of coral and algal holobionts. ISME J. 10, 1157–1169. doi: 10.1038/ismej.2015.190
Heuer, H., and Smalla, K. (1997). “ Application of denaturing gradient gel electrophoresis (DGGE) and temperature gradient gel electrophoresis (TGGE) for studying soil microbial communities,” in Modern Soil Microbiology, eds J. D. Van Elsas, J. T. Trevors, and E. M. H. Wellington (New York, NY: Marcel Dekker, Inc).
Hirose, M., Kinzie, R., and Hidaka, M. (2001). Timing and process of entry of zooxanthellae into oocytes of hermatypic corals. Coral Reefs 20, 273–280. doi: 10.1007/s003380100171
Kelly, B. J., Gross, R., Bittinger, K., Sherrill-Mix, S., Lewis, J. D., Collman, R. G., et al. (2015). Power and sample-size estimation for microbiome studies using pairwise distances and PERMANOVA. Bioinformatics 31, 2461–2468. doi: 10.1093/bioinformatics/btv183
Knowlton, N., and Rohwer, F. (2003). Multispecies microbial mutualisms on coral reefs: the host as a habitat. Am. Nat. 162, 51–62. doi: 10.1086/378684
Kruskal, J. B. (1964). Nonmetric multidimensional scaling: a numerical method. Psychometrika 29, 115–129. doi: 10.1007/BF02289694
Larkin, M. A., Blackshields, G., Brown, N., Chenna, R., Mcgettigan, P. A., Mcwilliam, H., et al. (2007). Clustal W and Clustal X version 2.0. Bioinformatics 23, 2947–2948. doi: 10.1093/bioinformatics/btm404
Leggat, W., Ainsworth, T., Bythell, J., Dove, S., Gates, R., Hoegh-Guldberg, O., et al. (2007). The hologenome theory disregards the coral holobiont. Nat. Rev. Microbiol. 5. doi: 10.1038/nrmicro1635-c2
Lema, K. A., Bourne, D. G., and Willis, B. L. (2014). Onset and establishment of diazotrophs and other bacterial associates in the early life history stages of the coral Acropora millepora. Mol. Ecol. 23, 4682–4695. doi: 10.1111/mec.12899
Lin, S., Cheng, S., Song, B., Zhong, X., Lin, X., Li, W., et al. (2015). The Symbiodinium kawagutii genome illuminates dinoflagellate gene expression and coral symbiosis. Science 350, 691–694. doi: 10.1126/science.aad0408
Littman, R. A., Willis, B. L., and Bourne, D. G. (2009). Bacterial communities of juvenile corals infected with different Symbiodinium (dinoflagellate) clades. Mar. Ecol. Prog. Ser. 389, 45–59. doi: 10.3354/meps08180
Loh, W. K. W., Loi, T., Carter, D., and Hoegh-Guldberg, O. (2001). Genetic variability of the symbiotic dinoflagellates from the wide ranging coral species Seriatopora hystrix and Acropora longicyathus in the Indo-West Pacific. Mar. Ecol. Prog. Ser. 222, 97–107. doi: 10.3354/meps222097
Lozupone, C., Hamady, M., and Knight, R. (2006). UniFrac - An online tool for comparing microbial community diversity in a phylogenetic context. BMC Bioinformatics 7:371. doi: 10.1186/1471-2105-7-371
Marquis, C., Baird, A., De Nys, R., Holmström, C., and Koziumi, N. (2005). An evaluation of the antimicrobial properties of the eggs of 11 species of scleractinian corals. Coral Reefs 24, 248–253. doi: 10.1007/s00338-005-0473-7
Mather, P. M. (1976). Computational Methods of Multivariate Analysis in Physical Geography. London: Wiley.
Moran, N. A., and Sloan, D. B. (2015). The hologenome concept: Helpful or hollow? PLoS Biol. 13:e1002311. doi: 10.1371/journal.pbio.1002311
Padilla-Gamiño, J. L., Pochon, X., Bird, C., Concepcion, G. T., and Gates, R. D. (2012). From parent to gamete: vertical transmission of Symbiodinium (Dinophyceae) ITS2 sequence assemblages in the reef building coral Montipora capitata. PLoS ONE 7:e38440. doi: 10.1371/journal.pone.0038440
Picciani-de-Souza, N., Garrido, A. G., and Zilberberg, C. (2016). “Do Equador aos trópicos: as zooxantelas na dimensão do espaço,” in Conhecendo os Recifes Brasileiros: Rede de Pesquisas Coral Vivo, eds C. Zilberberg, D. P. Abrantes, J. A. Marques, L. F. Machado, and L. F. D. B. Marangoni (Rio de Janeiro: Museus Nacional), 360.
Pires, D. O., Castro, C. B., and Ratto, C. C. (1999). Reef coral reproduction in the abrolhos reef complex, Brazil: the endemic genus Mussismilia. Mar. Biol. 135, 463–471.
Pires, D. O., Castro, C. B., Segal, B., Pereira, C. M., Carmo, E. C., Silva, R. G., et al. (2016). “Reprodução de corais de águas rasas do Brasil,” in Conhecendo os Recifes Brasileiros: Rede de Pesquisas Coral Vivo, eds C. Zilberberg, D. P. Abrantes, J. A. Marques, L. F. Machado, and L. F. D. B. Marangoni (Rio de Janeiro: Museu Nacional).
Pochon, X., Pawlowski, J., Zaninetti, L., and Rowan, R. (2001). High genetic diversity and relative specificity among Symbiodinium-like endosymbiotic dinoflagellates in soritid foraminiferans. Mar. Biol. 139, 1069–1078. doi: 10.1007/s002270100674
Radjasa, O. K., Salasia, S. I. O., Sabdono, A., Weise, J., Imhoff, J. F., Lammler, C., et al. (2007). Antibacterial activity of marine bacterium Pseudomonas sp. associated with soft coral Sinularia polydactyla against Streptococcus equi subsp. zooepidemicus. Int. J. Pharmacol. 3, 170–174. doi: 10.3923/ijp.2007.170.174
Ricardo, G. F., Jones, R. J., Clode, P. L., Humanes, A., and Negri, A. P. (2015). Suspended sediments limit coral sperm availability. Sci. Rep. 5:18084. doi: 10.1038/srep18084
Rojo, F. (2010). Carbon catabolite repression in Pseudomonas: optimizing metabolic versatility and interactions with the environment. FEMS Microbiol. Rev. 34, 658–684. doi: 10.1111/j.1574-6976.2010.00218.x
Rosenberg, E., Koren, O., Reshef, L., Efrony, R., and Zilber-Rosenberg, I. (2007). The role of microorganisms in coral health, disease and evolution. Nat. Rev. Microbiol. 5, 355–362. doi: 10.1038/nrmicro1635
Rosenberg, E., Sharon, G., and Zilber-Rosenberg, I. (2009). The hologenome theory of evolution contains Lamarckian aspects within a Darwinian framework. Environ. Microbiol. 11, 2959–2962. doi: 10.1111/j.1462-2920.2009.01995.x
Sanger, F., Nicklen, S., and Coulson, A. R. (1977). DNA sequencing with chain-terminating inhibitors. Proc. Natl. Acad. Sci. U.S.A. 74, 5463–5467. doi: 10.1073/pnas.74.12.5463
Santos, H. F., Carmo, F. L., Duarte, G., Dini-Andreote, F., Castro, C. B., Rosado, A. S., et al. (2014). Climate change affects key nitrogen-fixing bacterial populations on coral reefs. ISME J. 8, 2272–2279. doi: 10.1038/ismej.2014.70
Santos, H. F., Duarte, G. A. S., Rachid, C. T. C. C., Chaloub, R. M., Calderon, E. N., Marangoni, L. F. B., et al. (2015). Impact of oil spills on coral reefs can be reduced by bioremediation using probiotic microbiota. Sci. Rep. 5:18268. doi: 10.1038/srep18268
Sharp, K. H., Distel, D., and Paul, V. J. (2011). Diversity and dynamics of bacterial communities in early life stages of the Caribbean coral Porites astreoides. ISME J. 6, 790–801. doi: 10.1038/ismej.2011.144
Sharp, K. H., Ritchie, K. B., Schupp, P. J., Ritson-Williams, R., and Paul, V. J. (2010). Bacterial Acquisition in juveniles of several broadcast spawning coral species. PLoS ONE 5:e10898. doi: 10.1371/journal.pone.0010898
Suárez-Moreno, Z., Caballero-Mellado, J., Coutinho, B., Mendonça-Previato, L., James, E., and Venturi, V. (2012). Common features of environmental and potentially beneficial plant-associated Burkholderia. Microb. Ecol. 63, 249–266. doi: 10.1007/s00248-011-9929-1
Sunagawa, S., Woodley, C. M., and Medina, M. (2010). Threatened corals provide underexplored microbial habitats. PLoS ONE 5:e9554. doi: 10.1371/journal.pone.0009554
Tamura, K., Peterson, D., Peterson, N., Stecher, G., Nei, M., and Kumar, S. (2011). MEGA5: molecular evolutionary genetics analysis using maximum likelihood, evolutionary distance, and maximum parsimony methods. Mol. Biol. Evol. 28, 2731–2739. doi: 10.1093/molbev/msr121
Thornhill, D. J., Daniel, M. W., Lajeunesse, T. C., Schmidt, G. W., and Fitt, W. K. (2006). Natural infections of aposymbiotic Cassiopea xamachana scyphistomae from environmental pools of Symbiodinium. J. Exp. Mar. Biol. Ecol. 338, 50–56. doi: 10.1016/j.jembe.2006.06.032
Verrill, A. E. (1902). Variation and Nomenclature of Bermudian, West Indian and Brazilian reef corals, with Notes on Various Indo-Pacific Corals, Vol. 11. New Haven, CT: Connecticut Academy of Arts and Sciences, 63–168.
Wang, H. F., Zhu, W. Y., Yao, W., and Liu, J. X. (2007). DGGE and 16S rRNA gene gene sequencing analysis of bacterial communities in colon content and feces of pigs fed whole crop rice. Anaerobe 13, 127–133. doi: 10.1016/j.anaerobe.2007.03.001
Weis, V. M., Reynolds, W. S., and Krupp, D. A. (2001). Host-symbiont specificity during onset of symbiosis between the dinoflagellates Symbiodinium spp. and planula larvae of the scleractinian coral Fungia scutaria. Coral Reefs 20, 301–308. doi: 10.1007/s003380100179
Keywords: holobiont, microbiota transmission, Symbiodinium, coral core microbiome, bacteria
Citation: Leite DCA, Leão P, Garrido AG, Lins U, Santos HF, Pires DO, Castro CB, van Elsas JD, Zilberberg C, Rosado AS and Peixoto RS (2017) Broadcast Spawning Coral Mussismilia hispida Can Vertically Transfer its Associated Bacterial Core. Front. Microbiol. 8:176. doi: 10.3389/fmicb.2017.00176
Received: 27 October 2016; Accepted: 24 January 2017;
Published: 07 February 2017.
Edited by:
Hongyue Dang, Xiamen University, ChinaReviewed by:
Sebastian Fraune, University of Kiel, GermanyCopyright © 2017 Leite, Leão, Garrido, Lins, Santos, Pires, Castro, van Elsas, Zilberberg, Rosado and Peixoto. This is an open-access article distributed under the terms of the Creative Commons Attribution License (CC BY). The use, distribution or reproduction in other forums is permitted, provided the original author(s) or licensor are credited and that the original publication in this journal is cited, in accordance with accepted academic practice. No use, distribution or reproduction is permitted which does not comply with these terms.
*Correspondence: Raquel S. Peixoto, cmFxdWVscGVpeG90b0BtaWNyby51ZnJqLmJy
Disclaimer: All claims expressed in this article are solely those of the authors and do not necessarily represent those of their affiliated organizations, or those of the publisher, the editors and the reviewers. Any product that may be evaluated in this article or claim that may be made by its manufacturer is not guaranteed or endorsed by the publisher.
Research integrity at Frontiers
Learn more about the work of our research integrity team to safeguard the quality of each article we publish.