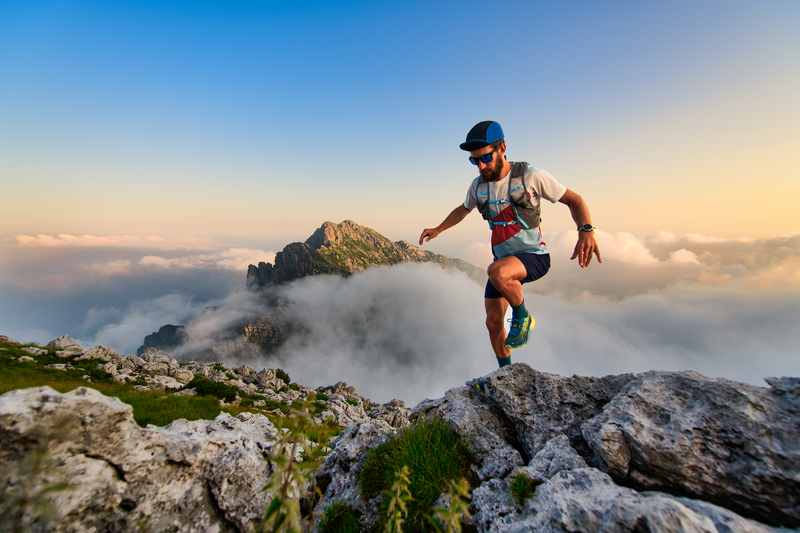
95% of researchers rate our articles as excellent or good
Learn more about the work of our research integrity team to safeguard the quality of each article we publish.
Find out more
ORIGINAL RESEARCH article
Front. Microbiol. , 24 January 2017
Sec. Microbial Symbioses
Volume 8 - 2017 | https://doi.org/10.3389/fmicb.2017.00059
This article is part of the Research Topic Optics and Ecophysiology of Coral Reef Organisms View all 15 articles
Climate change-related coral bleaching, i.e., the visible loss of zooxanthellae from the coral host, is increasing in frequency and extent and presents a major threat to coral reefs globally. Coral bleaching has been proposed to involve accelerating light stress of their microalgal endosymbionts via a positive feedback loop of photodamage, symbiont expulsion and excess in vivo light exposure. To test this hypothesis, we used light and O2 microsensors to characterize in vivo light exposure and photosynthesis of Symbiodinium during a thermal stress experiment. We created tissue areas with different densities of Symbiodinium cells in order to understand the optical properties and light microenvironment of corals during bleaching. Our results showed that in bleached Pocillopora damicornis corals, Symbiodinium light exposure was up to fivefold enhanced relative to healthy corals, and the relationship between symbiont loss and light enhancement was well-described by a power-law function. Cell-specific rates of Symbiodinium gross photosynthesis and light respiration were enhanced in bleached P. damicornis compared to healthy corals, while areal rates of net photosynthesis decreased. Symbiodinium light exposure in Favites sp. revealed the presence of low light microniches in bleached coral tissues, suggesting that light scattering in thick coral tissues can enable photoprotection of cryptic symbionts. Our study provides evidence for the acceleration of in vivo light exposure during coral bleaching but this optical feedback mechanism differs between coral hosts. Enhanced photosynthesis in relation to accelerating light exposure shows that coral microscale optics exerts a key role on coral photophysiology and the subsequent degree of radiative stress during coral bleaching.
Solar radiation governs coral photophysiology and ultimately drives the productivity and growth of coral reefs (Falkowski et al., 1990). Light stimulates photosynthesis of coral microalgal endosymbionts (Symbiodinium spp.), generating O2 and carbohydrates that are exported to the host fueling coral animal metabolism (Muscatine et al., 1981). However, excess light enhances Symbiodinium photodamage (Warner et al., 1999; Takahashi et al., 2004) and, in combination with anomalous seawater temperatures, can induce the breakdown of the coral-algal symbiosis known as coral bleaching (Brown, 1997; Hoegh-Guldberg, 1999). Coral bleaching events are regarded as a major threat to the future of coral reefs (Ainsworth et al., 2016) and hence the physiological mechanisms triggering coral bleaching have been a major research focus for decades (Weis, 2008). Coral bleaching susceptibility is affected by a combination of factors that act on different spatial and temporal scales, including coral thermal history (Brown et al., 2002; Hughes et al., 2003), Symbiodinium genotype (Sampayo et al., 2008), as well as biochemical pathways and tissue properties of the coral host species (Baird et al., 2009). At the cellular scale, coral bleaching involves enhanced thermal and radiative exposure of Symbiodinium cells, resulting in photodamage and the subsequent generation of reactive oxygen species (ROS) that induce the breakdown of the symbiosis (Lesser, 1996; Hoegh-Guldberg, 1999; Weis, 2008). The in vivo light and temperature exposure of Symbiodinium within the host tissue ultimately controls whether Symbiodinium undergoes photodamage, and it is thus important to resolve the optical and thermal microenvironment of coral hosts (Enriquez et al., 2005; Jimenez et al., 2008; Wangpraseurt et al., 2012; Swain et al., 2016).
Application of light microsensors has shown that the in vivo light exposure within coral tissues can be enhanced over the incident downwelling irradiance (Kühl et al., 1995; Wangpraseurt et al., 2012, 2014a,b; Brodersen et al., 2014). Such irradiance enhancement is modulated by the unique optical properties of coral tissue and skeleton (Enriquez et al., 2005; Teran et al., 2010; Kahng et al., 2012; Marcelino et al., 2013; Wangpraseurt et al., 2016a) and can improve photosynthesis under low light conditions (Brodersen et al., 2014; Wangpraseurt et al., 2014a) or lead to light stress under high irradiance (Marcelino et al., 2013; Swain et al., 2016). The loss of Symbiodinium spp. cells from corals under environmental stress has been hypothesized to further increase irradiance exposure in hospite due to decreased shading by photopigments and increased backscattered light from the coral skeleton (Enriquez et al., 2005; Teran et al., 2010; Swain et al., 2016). According to this so-called optical feedback hypothesis (Enriquez et al., 2005; Swain et al., 2016), skeleton backscattering can further stimulate symbiont loss inducing an accelerating cycle, where symbiont loss promotes light enhancement and vice versa. Several studies have speculated on the relevance of such a feedback loop exacerbating coral bleaching, arguing that the light microenvironment during a stress event could serve as a key factor in determining the severity of a bleaching event (Hoegh-Guldberg, 1999; Franklin et al., 2006; Hoogenboom et al., 2006; Abrego et al., 2008; Weis, 2008; Baird et al., 2009). However, experimental proof of such a mechanism has been lacking.
Here, we provide the first direct measurements of the in vivo light environment of Symbiodinium during coral bleaching. We performed a thermal stress experiment and monitored changes in the in vivo light environment using light microsensors in two coral species with contrasting optical properties in concert with O2 microsensor-based measurements of gross photosynthesis, net photosynthesis and light respiration. We provide evidence for the acceleration of in vivo light exposure upon coral bleaching and show that such light enhancement differs between coral hosts. This highlights the importance of skeleton and tissue optics for coral photophysiology and stress responses.
Light microenvironments were investigated in two shallow-water corals: the branching coral Pocillopora damicornis, and the massive coral Favites sp. The coral P. damicornis is generally a bleaching-susceptible species (Loya et al., 2001; Swain et al., 2016) with thin tissues (maximum thickness ∼150 and 300 μm for coenosarc and polyp tissues, respectively) and low tissue light attenuation (Szabó et al., 2014). P. damicornis from Heron Island is known to harbor different Symbiodinium spp. including symbiont type C42ab and C33a (Tonk et al., 2013). Favites sp. is a more bleaching-resistant species (Swain et al., 2016) with a polyp tissue thickness of ∼2 mm (Wangpraseurt et al., 2014a) and is known to associate with symbionts of type C1, C21, and C33 (Tonk et al., 2013). Corals were collected at low tide from shallow waters (<2 m depth) on the reef flat off Heron Island, Great Barrier Reef, Australia (152°06′ E, 20°29′ S) in August–September 2014. On a cloudless day during mid-day sun, the incident downwelling photon irradiance integrated over 400–700 nm, i.e., PAR, reached in situ values at the level of the sampled colonies of Ed(PAR) > 2000 μmol photons m-2 s-1 (Wangpraseurt et al., 2014b). Corals were selected from the same small sample area in order to ensure that they were adapted to a comparable light and flow regime. Coral fragments from at least 10 different daughter colonies of P. damicornis and Favites sp. were collected and sub-fragmented (P. damicornis ca. 3–10 cm in length and Favites ca. 3 cm in diameter).
Collected corals were allowed to recover from potential translocation stress for a minimum of 1 week in a shaded outdoor aquarium at Heron Island Research Station before transfer to the experimental incubation set-up consisting of an outdoor aquarium tank (300 L) that was exposed to natural sunlight (13 h of daylight) and continuously supplied with seawater from the reef flat at an exchange rate of about 0.2 L s-1. The set-up mimicked conditions on the Heron Island reef flat for a water depth of 20–30 cm with high solar irradiance exposure. A water heater connected to a thermistor (accuracy: ±1.5°C, Sensor type: KTY81-121, Sentien Electronics, Australia) was placed in one corner of the tank and two submersible water pumps directed the water flow (3–5 cm s-1) toward the heater, while four additional water pumps recirculated the water in the tank at a comparable flow speed in order to avoid the build-up of temperature gradients within the tank. Ed(PAR) during noon was ∼2000 μmol photons m-2 s-1 as measured at the water surface with a planar quantum irradiance sensor connected to a light meter (LI-190 and LI-1400, Li-Cor, Lincoln, NE, USA). Temperature was monitored using data loggers (Onset, USA) randomly distributed within the tank and logging at a time interval of 1 min. Coral fragments were randomly distributed within the tank. In order to induce coral bleaching, we followed the protocol of Middlebrook et al. (2010), whereby the water temperature was step-wise increased by about 1°C per day. In total the experiment ran for 13 days during September 2014, and included 3 days of baseline measurements at ambient water temperature (22°C) followed by 10 days ramping of temperature from 22 to 33°C.
Often experimental measurements on coral bleaching use a balanced experimental design where temperature treated corals are assessed on par with healthy corals during the course of the experiment. In the microenvironmental approach used here, the total number of samples was limited by the experimental challenges involving intra-tissue microsensor measurements with a high chance of sensor breakage. As our main aim was to assess the optical mechanisms underlying coral bleaching, we prioritized measuring coral tissues with reduced algal cell densities over a larger number of control measurements on healthy corals. It is important to note that our experimental design does thus not allow for excluding potential experimental treatment effects (e.g., coral disease) on the physiology of the algae. However, all corals were visually expected for tissue necrosis and sloughing, and corals appeared visually free of disease. During the temperature ramping experiment, visual bleaching of Favites sp. occurred much slower than in P. damicornis and we thus accelerated the bleaching process for Favites sp. through a combined temperature and light stress treatment (see Supplementary Methods). Our approach did not allow us to compare differences in photophysiology or the rate of symbiont loss between P. damicornis and Favites sp.
Spectral scalar irradiance E0(λ) and reflectance RH(λ) measurements were performed in a flow chamber that was flushed at a flow rate of 0.5–1 cm s-1 with seawater at the same temperature as in the experimental bleaching treatment tank. Fiber-optic scalar irradiance microprobes with a spherical tip diameter of 80 μm (Rickelt et al., 2016) were used to measure the spectral light microenvironment on and within coral coenosarc and polyp tissues as described previously (Wangpraseurt et al., 2012; see Supplementary Methods for details).
Spectral reflectance measurements on intact corals (RH) were performed with a flat-cut fiber-optic reflectance probe (2 mm diameter, Ocean Optics, USA) positioned at a 45° angle relative to the coral surface at a distance of 1 cm from the sample, using a similar measuring configuration as in Rodriguez-Roman et al. (2006). Vertically incident light [Ed(PAR) = 400 μmol photons m-2 s-1] was provided by a fiber-optic tungsten halogen-lamp equipped with a collimating lens (Schott KL-2500, Germany). The coral reflected light measurements were normalized to the reflected light measured under identical optical configuration from a 99% white reflectance standard (Spectralon, Labsphere, USA). Scalar irradiance and reflectance spectra were also measured on bare skeletons (see Supplementary Figure S1).
Clark-type O2 microsensors (tip size of 25 μm, a 90% response time of <0.5 s and a stirring sensitivity of ∼1%; Unisense A/S, Aarhus, Denmark) were used to measure O2 production and consumption of P. damicornis as described previously (Wangpraseurt et al., 2012; Brodersen et al., 2014). Sensor readings were linearly calibrated from measurements in air saturated water and anoxic water (flushed with N2). Percent air saturation in seawater at experimental temperature and salinity was transformed to O2 concentration (μmol O2 L-1) using gas tables (Ramsing and Gundersen, Unisense, Denmark; www.unisense.com). All O2 microsensor measurements were performed in the same configuration as the scalar irradiance microsensor measurements but with the O2 microsensors approaching the coral surface at an angle of ∼10° relative to the vertically incident light. Measurements of gross photosynthesis at the coral surface were performed using the light–dark shift technique (see Revsbech and Jorgensen, 1983 for detailed description) followed by measurements of steady-state O2 concentration profiles from the coral tissue surface through the diffusive boundary layer (DBL) and into the mixed turbulent water phase above (Brodersen et al., 2014) under an incident photon irradiance of Ed(PAR) = 400 μmol photons m-2 s-1. Steady-state O2 conditions were usually achieved within 5–10 min at a constant light level and each microsensor profile took on average about 5 min. We chose Ed = 400 μmol photons m-2 s-1 as a irradiance level close to the photosynthetic maximum based on previous microsensor measurements under comparable conditions (Ulstrup et al., 2006) and preliminary results from variable chlorophyll fluorimetry-based measurement of relative photosynthetic electron transport rates vs. irradiance (data not shown). The coral was then placed in darkness for >15 min before O2 profiles measured the dark respiration of the coral holobiont (Schrameyer et al., 2014). Additional measurements of the dark-adapted maximum quantum yield of PSII were done with a commercial variable chlorophyll fluorescence imaging system (Imaging-PAM, Walz GmbH, Germany; see supporting information and Supplementary Figure S2).
Symbiodinium cell density was determined for small tissue areas of Favites sp. using a microsampling technique (Kemp et al., 2008), where individual polyps were sampled by careful separation of the coral tissue from the underlying skeleton using a syringe and needle (see supporting information and Supplementary Figure S3). This technique was not used for P. damicornis since the thin tissue of this coral was firmly attached to the skeleton, making it difficult to completely remove the tissue with a syringe. Instead small branch tips (maximum of 0.5–0.8 cm in length) were collected, crushed and centrifuged to separate host tissue, skeleton and Symbiodinium (see supporting information). For both coral species, Symbiodinium density was then estimated using a haemocytometer. Additional tissue samples were collected (as described above) for HPLC (high performance liquid chromatography) analysis using standard protocols (see supporting information).
Measured raw spectra were integrated between 400 and 700 nm (PAR) using the mathematical integration function of Origin Pro 9.1 (Origin, USA). The in vivo light enhancement relative to the incident downwelling irradiance was calculated as E0(PAR)/Ed(PAR). Data was averaged for measurements performed on day 1 (‘healthy,’ water temperature 22°C) and day 10 (‘bleached,’ water temperature 33°C). We also expressed the enhancement of spectral scalar irradiance, E0(λ), of bleached corals relative to healthy corals as E0(λ)bleached/E0(λ)healthy. Areal rates of gross photosynthesis (PG; nmol O2 cm-2 s-1) were calculated by depth integration of the volumetric rates assuming constant rates of photosynthesis over the entire tissue. Tissue thickness of P. damicornis was ca. 300 μm for polyp tissues and ca. 150 μm for coenosarc tissues as determined with scalar irradiance microsensors (data not shown, see Wangpraseurt et al., 2014a). Net photosynthesis (PN) and dark respiration (RD) rates were calculated using Fick’s first law of diffusion (see Chan et al., 2016 for details). Light respiration of the coral holobiont (LR) was then calculated as the difference between gross and net photosynthesis (Schrameyer et al., 2014). In order to compare Symbiodinium dependent gross photosynthetic rates between healthy and bleached corals, we normalized our areal rates to cell density. Values of O2 production in nmol O2 cm-2 s-1 was divided by the average measured cell density (in cells per cm-2) to express gross photosynthesis in fmol O2 cell-1 s-1.
Regression analyses were used to test for the relationship between Symbiodinium cell density and in vivo coral tissue scalar irradiance. A non-linear curve fitting procedure was applied to test the relationship between the in vivo E0 (expressed in % of Ed) measured in polyp surface, polyp aboral and coenosarc surface tissues of P. damicornis and the cell density (cells per cm-2). The fitting used the non-linear Levenberg Marquardt iteration algorithm and applied a power-law function (i.e., y = ax-b) to yield the adjusted R2 and reduced χ2 goodness of fit parameters. Physiological and optical parameters measured for P. damicornis and Favites sp., including Fv/Fm, PG, PN, RD, LR, E0(PAR)/Ed(PAR) were compared between healthy (day = 1) and bleached (day = 10) conditions using a two-sample Student’s t-test (for equal variances) or Welch’s t-test (for unequal variances). Statistical tests were performed in Origin Pro 9.1 (Origin, USA).
Symbiodinium cell density was significantly reduced at the end of the experiment for both P. damicornis and Favites sp. (Figure 1). In P. damicornis, the mean cell density was reduced 4.2-fold from 1.43 × 106 (± SE 2.8 × 105) to 3.42 × 105 (± 7.5 × 104 SE) cells cm-2 [Student’s t-test: t(10) = 3.7, p = 0.003; Supplementary Figure S4]. In Favites sp., there was a comparable fourfold reduction in cell density from 2.30 × 106 (3.6 × 105) to 5.6 × 105 (1.4 × 105 SE) cells cm-2 tissue surface area [Student’s t-test: t(10) = 3.3, p = 0.008]. Chlorophyll a density (per g of host tissue biomass) decreased by about 2.1- and 1.7-fold for P. damicornis [Student’s t-test: t(8) = 1.6, p = 0.146] and Favites sp. [Welch’s t-test: t(7.5) = 2.4, p = 0.047], respectively although this difference was only statistically significant for Favites sp. (Figure 1).
FIGURE 1. Changes in Symbiodinium cell density (cells per cm-2 surface area) and chlorophyll a density (in μg chlorohyll a per g dry host tissue biomass) during the bleaching experiment. Healthy corals (black symbols) and bleached corals (gray symboles) were measured at the beginning (day 1) and the end of the experiment (day 10). Data are mean ± SEM; n = 4–8 for cell density estimates and n = 6–9 for chlorophyll a estimates.
For all corals and measurement locations, in vivo photon scalar irradiance, E0(PAR), increased in bleached compared to healthy corals (Figures 2a–e). In P. damicornis, the greatest increase in E0(PAR) was measured in the aboral polyp tissues, where PAR was about 3.65-fold higher in bleached vs. healthy corals [E0(PAR)/Ed(PAR) = 1.97 ± 0.21 SE and 0.54 ± 0.09 SE, respectively; Student’s t-test: t(10) = -6.1, p < 0.001; Figure 2d]. The E0(PAR) enhancement was less pronounced for oral polyp tissues and coenosarc oral tissues with ratios of E0(PAR)/Ed(PAR) = 1.58 and 1.66, respectively (Figure 2d).
FIGURE 2. Effect of bleaching on in vivo photon scalar irradiance, E0(PAR)-enhancement. Typical measurement locations over the coenosarc and polyp tissues of Pocillopora damicornis (a) and Favites sp. (b,c). Scale bar = 1 mm (a) and 2 mm (b,c). Note the scalar irradiance microprobe (b) and extruded mesenterial filaments (mf) in the stressed Favites sp. coral (c). E0(PAR)-enhancement was expressed as E0(PAR)/Ed(PAR) and was measured in healthy and bleached P. damicornis (d) and Favites sp. (e). Measurements were performed on the surface of coenosarc and polyp tissues as well as in the lowermost aboral tissue layer (vertical depths closest to the skeleton) for healthy (day 1) and bleached corals (day 10). Data are mean ± SE (n = 6 corallite-level replicates).
For Favites sp., E0(PAR) for aboral polyp tissues of bleached corals [E0(PAR)/Ed(PAR) = 0.26 ± 0.03 SE] was ∼3.5 times higher than in respective healthy tissues [E0(PAR)/Ed(PAR) = 0.07 ± 0.02 SE] but this difference was not significant [Welch’s t-test: t(3.1) = -2.3, p = 0.11]. E0(PAR) on the oral polyp tissue increased by 1.13 times upon bleaching [Welch’s t-test: t(3.5) = -5.5, p = 0.007, Figure 2e]. For coenosarc tissues, we similarly observed a proportionally greater E0(PAR) enhancement upon bleaching in aboral tissues E0(PAR)/Ed(PAR) = 2.3 vs. oral tissues E0(PAR)/Ed(PAR) = 1.27 (Figure 2e). The magnitude of E0(PAR) enhancement in bleached relative to healthy coral tissues was similar for Favites sp. and P. damicornis, but the absolute enhancement was different as low light niches remained in Favites sp. [E0(PAR)/Ed(PAR) = 0.25 ± 0.03] (Figures 2d,e).
In vivo E0(λ) of bleached corals was increased relative to healthy corals (λ = 400–700 nm) for both species; however, the relative enhancement of E0(λ), i.e., E0(λ)bleached/E0(λ)healthy, was dependent on coral species and tissue type (Figures 3A–D and 4A,B). In P. damicornis, E0(λ)bleached/E0(λ)healthy in aboral polyp tissues was highest for blue light [E0(λ)bleached/E0(λ)healthy ∼ 5 for λ = 400–500 nm] followed by far red light [E0(λ)bleached/E0(λ)healthy ∼ 4 for λ = 670–690 nm] and least pronounced for green to red light [E0(λ)bleached/E0(λ)healthy ∼ 3 for λ = 550–650 nm] (Figures 3C and 4A). In contrast, tissue surface E0(λ) did not exhibit such pronounced spectral features and scalar irradiance was more evenly enhanced between 400 and 700 nm in both polyp and coenosarc tissues (Figures 3A,C and 4A). A similar trend was observed in Favites sp., where maximal E0(λ) enhancement was measured in aboral polyp tissues in the blue (λ≈500 nm) and far-red (λ≈650–695 nm) (Figures 3B,D and 4B). Note that no light enhancement factors were calculated for aboral polyp tissues for wavelengths less than 500 nm, due to a relatively low signal to noise ratio (a result of the almost completely depleted blue light in healthy corals). E0(λ) was fairly evenly enhanced between 400 and 700 nm for surface tissues of Favites (Figure 4B).
FIGURE 3. Effect of coral bleaching on in vivo spectral scalar irradiance, E0(λ) expressed in % of the incident downwelling irradiance, Ed(λ). Measurements were performed on the coenosarc (A,B) and polyp tissue (C,D) of P. damicornis (left panel), and Favites sp. (right panel). Solid and dashed lines represent measurements performed in aboral tissues and at the tissue surface, respectively. Measurements were performed on healthy corals (day 1; black) and bleached corals (day 10; gray). Data are mean ± SE (n = 6 corallite-level replicates).
FIGURE 4. Spectral light enhancement in bleached relative to healthy corals. The enhancement in E0(λ) and RH(λ) due to bleaching was calculated as E0(λ)bleached/E0(λ)healthy and RH(λ)bleached/RH(λ)healthy. RH(λ) (dotted lines) was measured at a distance of 1 cm from the tissue surface and E0(λ) was measured at the tissue surface (dashed lines) and in the aboral tissue layers closest to the skeleton (solid lines). Data was collected for both polyp (blue) and coenosarc tissues (red) for P. damicornis (A), and Favites sp. (B). Note that P. damicornis RH(λ) measurements include areas covering both polyp and coenosarc tissues, and that E0(λ) measurements were not performed in aboral coenosarc tissues of P. damicornis due to lack of sufficient tissue thickness. Original RH(λ) spectra for bleached and healthy corals are shown in Supplementary Figure S5.
Enhancement of spectral reflectance, RH(λ)bleached/RH(λ)healthy in P. damicornis followed a similar wavelength dependency as the E0(λ) enhancement in aboral polyp tissues, i.e., it peaked for λ = 420–550 nm and λ = 650–690 nm (Figure 4A; Supplementary Figure S5). However, the RH(λ)bleached/RH(λ)healthy ratio was lower than the E0(λ)bleached/E0(λ)healthy ratio, i.e., the increase in reflectance was lower than the increase in in vivo scalar irradiance of bleached tissues relative to healthy tissues (Figure 4A). For polyp tissues of Favites sp., RH(λ)bleached/RH(λ)healthy was ∼1 and ∼1.5 for λ = 400–500 and 550–690 nm, respectively (Figure 4B). For coenosarc tissues, RH(λ)bleached/RH(λ)healthy was similar to polyp tissues for wavelengths >550 nm but was <1 at 450–510 nm (Figure 4B). Thus reflectance measurements were not able to capture tissue depth- and tissue type-dependent dynamics in scalar irradiance, as can be seen by the more moderate increase in reflectance relative to the in vivo scalar irradiance during bleaching (Figure 4).
In vivo E0(PAR) enhancement and Symbiodinium cell density was described by a power-law function (Figures 5A–C) from all data collected throughout the bleaching experiment. The best fit was obtained when Symbiodinium cell density was matched against E0(PAR) enhancement measured in aboral polyp tissues (R2 = 0.45; Figure 5C) (compared to R2 fits of 0.43 and 0.30 for coenosarc surface and polyp surface tissues, respectively; Figures 5A,B).
FIGURE 5. In vivo photon scalar irradiance enhancement E0(PAR)/ Ed(PAR) as a function of Symbiodinium cell density in Pocillopora damicornis for coenosarc surface tissues (A), polyp surface tissues (B), and polyp aboral tissues (C). The best fit (solid red lines) in all cases was a power-law relationship (y = ax-b). Red and light red areas represent 95% confidence and prediction intervals, respectively.
Areal rates of gross photosynthesis in bleached coenosarc and polyp tissues were reduced by 70 and 25%, respectively, when compared to the corresponding healthy tissues (Figure 6A), but these measurements do not account for symbiont loss after bleaching (Figure 1). Instead, cell-specific rates of gross photosynthesis were about 1.3- and 3.2-fold higher in coenosarc and polyp tissues, respectively, for Symbiodinium remaining in the bleached corals as compared to the unbleached corals (Figure 6D). Measurements of the maximum quantum yield of PSII showed that upon bleaching Fv/Fm was reduced by about 50% as compared to healthy corals (0.38 ± 0.03 vs. 0.8 ± 0.01, respectively; mean ± SEM; Supplementary Figure S2).
FIGURE 6. Areal rates (in nmol O2 cm-2 s-1) of gross photosynthesis (A; PG), net photosynthesis (B; PN), and apparent light respiration (C; LR = PG-PN) and dark respiration (E; RD) in coenosarc and polyp tissues of healthy (black bars) and bleached fragments (gray bars) of Pocillopora damicornis. (D,F) same as left panels but normalized to cell density, i.e., cell specific-rates. Data are mean ± SEM (n = 10–12 corallite-level replicates). Significant differences (p < 0.05) between bleached and healthy tissues are marked with an asterisk.
Measurements of areal net photosynthesis generally showed net O2 production except for measurements in bleached coenosarc tissues that exhibited a net O2 consumption of 0.046 (± 0.015 SE) nmol O2 cm-2 s-1 in the light (Figure 6B). There was a trend toward increased holobiont light respiration in bleached vs. healthy coenosarc tissues (Figure 6C), while a decreased light respiration was found in polyp tissues after bleaching (0.40 ± 0.08 vs. 0.33 ± 0.03 nmol O2 cm-2 s-1; mean ± SEM). In contrast, apparent light respiration rates per Symbiodinium cell increased after bleaching by 4.2- and 2.5-fold for coenosarc and polyp tissues, respectively (Figure 6F). Areal dark respiration rates did not change significantly after the bleaching experiment for both coensoarc and polyp tissues (Student’s t-test, p > 0.05; Figure 6E).
Microscale light measurements revealed an average E0(PAR)-enhancement of ∼2 for P. damicornis, with maximal values of up to 2.6 within bleached polyp tissues (Figure 5). Such measured enhancement in P. damicornis can at first approximation be explained by simple light scattering events within the coral skeleton (Enriquez et al., 2005; Wangpraseurt et al., 2014a). As Symbiodinium cell density becomes reduced (Figure 1), more light penetrates toward the skeleton (Figure 3A) and an increased fraction of this light is backscattered (Enriquez et al., 2005). For a flat isotropically scattering skeleton with a reflectance, R, the average path length of upwelling photons is twice that of photons in the incident collimated light beam traversing a thin layer of coral tissue (ignoring tissue scattering) (Kühl and Jørgensen, 1994). Here, the contribution of the coral skeleton reflectance on the scalar irradiance can be calculated as (Kortüm, 2012): Eo = (1 + 2R)Ed, where Ed is the incident downwelling irradiance. The flux absorbed by Symbiodinium can thus be estimated as Φabs = +
= (1 + 2R)
, where
is the absorption of the incident beam, i.e.,
= A × Ed, where A is the absorption cross-section of Symbiodinium (Enriquez et al., 2005). Diffuse reflectance of P. damicornis skeletons in our study was on average R = 0.5 (data not shown), which is comparable to previous measurements (R = 0.36, Swain et al., 2016). As such, our theoretical calculations would predict an E0(PAR)-enhancement of 2, a value consistent with our direct measurements for the thin bleached coenosarc tissues of P. damicornis (Figure 5A).
In contrast to the consistent theoretical and measured E0(PAR)-enhancement values for P. damicornis coenosarc tissues, we observed an E0(PAR) enhancement of >2 for polyp tissues (Figures 5B,C). E0(PAR) enhancement of >2 suggests an additional scattering contribution from the coral tissue (Wangpraseurt et al., 2014a) and/or from the concave corallite architecture (Ow and Todd, 2010). Indeed, measurements for bare skeletons (Supplementary Figure S1) revealed a higher E0 within the corallite than over the coenosteum, suggesting that the small concave corallite for P. damicornis (width and length ∼1 mm; Nothdurft and Webb, 2007) effectively homogenizes and redirects the incident radiation, through multiple reflections from the skeletal walls toward the center of the corallite, whereas light escapes more easily as diffuse reflectance from the rather flat coenosteum. Given the thin tissue in P. damicornis, multiple scattering by such millimeter-sized skeletal structures likely contributes to the observed higher E0(PAR)-enhancement of Symbiodinium in polyp vs. coenosarc tissues in the intact living coral (Figure 4A). However, the E0(PAR)-enhancement observed in intact bleached P. damicornis was still higher than for bare skeletons indicating additional light enhancement through tissue scattering. It is possible that diffusely backscattered photons from the skeleton are trapped by the tissue (see detailed discussion in Wangpraseurt et al., 2014a) and/or that the scattering coefficient is higher for coral tissue than for coral skeleton, as is the case for Favites sp. (Wangpraseurt et al., 2016a).
The optical feedback hypothesis predicts an inverse power-law relationship between Symbiodinium cell density and in vivo light exposure, i.e., the rate of light field ‘amplification’ increases as symbiont cell density decreases (Teran et al., 2010; Swain et al., 2016). For a small cup-shaped polyp, with low tissue absorption and scattering, the greatest effect of corallite architecture on light scattering is expected in close proximity to the skeletal surface at the center of the corallite (Teran et al., 2010; Wangpraseurt et al., 2016a). Our measurements for P. damicornis support these predictions since the highest rate of light enhancement was measured within aboral polyp tissues (R2 = 0.45, Figure 5C). Scattering by (sub)millimeter-sized skeletal surface elements in such a thin-tissued coral with small polyps thus play a key role in the measured light enhancement dynamics upon bleaching.
Coral bleaching also involves a change in the spectral exposure of the remaining symbionts (Figure 4). In a normally pigmented healthy coral, blue light (400–450 nm) is rapidly attenuated vertically within the coral tissue due to peak light absorption by Chl a, leaving symbionts in aboral tissue layers exposed to a green to red shifted light spectrum (Figure 3; Szabó et al., 2014). As coral bleaching progresses, symbionts in aboral tissues are progressively exposed to greater amounts of blue light (Figure 4). It is important to note that such spectral changes could not be captured solely based on surface scalar irradiance or diffuse reflectance measurements (Figure 4), highlighting the relevance of depth resolved measurement of in vivo scalar irradiance. Minor changes in the spectral composition of light can affect Symbiodinium photosynthesis in vivo (Wangpraseurt et al., 2014c) and it will be interesting in the future to study the effect of changing spectral exposure on symbiont photosynthesis along a vertical gradient within the tissue (Lichtenberg et al., 2016).
Our data demonstrated the presence of species-specific patterns of light modulation, whereby light gradients were alleviated in polyp tissues of P. damicornis but remained present in polyp tissues of Favites sp. after bleaching (Figures 3A–D). Favites sp. are characterized by thick, light scattering tissues (Wangpraseurt et al., 2014a, 2016a) with host pigments such as GFP (Salih et al., 2000; Lyndby et al., 2016) and mycosporine-like amino acids (Dunlap and Shick, 1998; Lesser and Farrell, 2004) that often remain present during bleaching (Smith et al., 2013). Although, P. damicornis does also have a GFP-like pigment (Takabayashi and Hoegh-Guldberg, 1995), the GFP-like pigments in Favites sp. are arranged in a chromatophore system that strongly enhances light scattering (Lyndby et al., 2016; Wangpraseurt et al., 2016b) facilitating a steep light attenuation along the enhanced optical path within thick scattering coral tissue (Wangpraseurt et al., 2012; Lyndby et al., 2016). Our finding of low light levels in the aboral polyp tissues therefore suggests that tissue background scattering and absorption effectively attenuate light even in bleached tissues of Favites sp. Additionally, differences between P. damicornis and Favites sp. in skeleton optical properties are likely (Marcelino et al., 2013; Swain et al., 2016; Wangpraseurt et al., 2016a), which would further affect the fraction of light ‘amplification’ by the skeleton (Marcelino et al., 2013). Thus our results indicate that symbionts in P. damicornis will be more severely affected by ‘optical feedback’ than symbionts in Favites sp. during coral bleaching. The moderate light environment in aboral polyp tissues of bleached Favites sp. could facilitate photoprotection of remaining cryptic Symbiodinium sp. (Silverstein et al., 2012), which could be a key determinant for symbiont repopulation and subsequent coral recovery from bleaching (Rowan et al., 1997).
However, it is likely that a variety of structural design solutions exist that provide photoprotection on different spatial scales (see also Yost et al., 2013). For a branching coral, such as P. damicornis, light attenuates along the branch due to shading by neighboring branches (Kaniewska et al., 2011). The magnitude of light attenuation along a single branch is similar to the light attenuation observed within the polyp tissues of Favites sp. (compare Kaniewska et al., 2011 and Wangpraseurt et al., 2012). Thus the presence of moderate light microenvironments is expected for P. damicornis due to its colony-level architectural complexity, which was however, not assessed in the present study. We visually observed that corals bleached first over the coenosarc tissue area, while symbionts remained within the tentacles of P. damicornis despite the enhanced in vivo light environment in polyp tissues. It was not possible to measure the light microenvironment within the highly contractile tentacle tissue and it remains unknown, whether a more moderate light microenvironment remained within the tentacles facilitating resistance to bleaching. Additionally, it is possible that bleaching was alleviated for tentacle tissues because of tentacle movement leading to enhanced gas exchange (Shashar et al., 1993) and possibly reducing the build-up of high O2 levels alleviating oxidative stress.
We observed increased symbiont cell-specific rates of gross O2 evolution and light respiration in bleached relative to healthy P. damicornis, although Fv/Fm values and areal photosynthetic rates (gross and net) decreased (Figure 6). Numerous studies have reported lowered Fv/Fm values (Warner et al., 1999; Jones et al., 2000; Supplementary Figure S2) and reduced net photosynthesis of Symbiodinium in response to thermal and/or light stress (Iglesias-Prieto and Trench, 1994; Warner et al., 1996, 1999), but few studies have measured gross O2 evolution independent of light respiration (Kühl et al., 1995; Abrego et al., 2008; Schrameyer et al., 2014). A lowering of Fv/Fm is often interpreted as a sign of photoinhibition of Symbiodinium and can result from the formation of non-functional PS II reaction centers, i.e., a downregulation of PSII efficiency that still allows for O2 evolution to occur (Hill et al., 2004). The high cell-specific gross photosynthetic rate (Figure 6D) could be explained as a downregulation of PSII efficiency counteracting the rapidly increasing in vivo irradiance (Figure 4). It is also possible that the observed dynamics reflect photoacclimation of Symbiodinium, as high light acclimation involves an increase in the functional absorption cross section of PSII that leads to a lowering of Fv/Fm (Hennige et al., 2008; Suggett et al., 2009). Additionally, it is possible that bleaching affected the symbiont community, potentially favoring more efficient photosynthesisers under high temperature (Roth, 2014; Sampayo et al., 2016).
The relative increase of cell-specific photosynthesis for bleached relative to healthy corals will depend on the quantity of incident solar radiation. Assuming a scenario where incident radiation saturates photosynthesis in all algal cell layers of a healthy coral, the in vivo light enhancement due to bleaching would not allow for a further enhancement of photosynthesis. Likewise, a faster increase in temperature would lead to more severe photoinhibtion and ultimately reduced photosynthetic rates. Additionally, our data showed that cell density (per surface area) declined to a greater extent than chlorophyll content (per g host tissue biomass). The reason for this mismatch remains unknown but could be related to reduced tissue thickness, which would affect chlorophyll but not cell density data, and/or potentially reflect the limited capacity of the HPLC to detect the Chl a signals in our microsamples. Normalization of gross photosynthesis data per chlorophyll a content of the corals showed that gross photosynthesis was only enhanced for polyp tissues (by about 1.6-fold) of bleached vs. healthy P. damicornis corals (data not shown). The enhancement of cell-specific photosynthesis during coral bleaching should thus be interpreted with caution and needs further investigation.
Light respiration significantly increased during the bleaching experiment, accounting for about 35% of gross photosynthesis in healthy tissues up to about 150% of gross photosynthesis in bleached tissues (Figure 6). Enhanced, apparently light-driven, O2 consumption is a sign of increased electron flow through alternative electron pathways, of which the Mehler reaction is especially prevalent in Symbiodinium (Roberty et al., 2014). While an upregulation of the Mehler reaction has been interpreted as a photoprotective mechanism to decrease excitation pressure on PSII under high light (Roberty et al., 2014), the Mehler reaction generates ROS that can readily lead to oxidative stress, if antioxidants such as superoxide dismutase and ascorbate peroxidase are not upregulated as well (Krueger et al., 2014; Roberty et al., 2014). Together, the enhanced metabolic activity likely reflects a response of Symbiodinium to the strongly accelerated in vivo light environment (Figure 5) showing that coral microscale optics exert a key role in coral photophysiology.
Climate change-related coral bleaching is arguably the prime cause of global reef decline and better predictions of coral bleaching susceptibility rest on understanding the mechanisms at play (Hoegh-Guldberg, 1999; Hoegh-Guldberg et al., 2007; Weis, 2008). The optical feedback hypothesis (Enriquez et al., 2005), whereby Symbiodinium undergoes light exposure ‘amplification’ during coral bleaching (Enriquez et al., 2005; Swain et al., 2016) has lacked experimental evidence in terms of direct measures of the tissue light field before and during coral bleaching. Here, we give the first experimental proof that the in vivo scalar irradiance in corals is enhanced during coral bleaching due to changes in the balance between absorption and scattering upon loss of Symbiodinium. Light amplification differs between coral hosts, as tissue light gradients and optical shelter remained in the massive coral Favites sp. but were alleviated in P. damicornis. The finding of optical shelter in bleached Favites sp. tissues implies a photoprotective microenvironment that could sustain coral resilience by facilitating the repopulation from cryptic Symbiodinium after stress. Our study shows that coral bleaching can in fact enhance cell-specific photosynthetic rates of remaining Symbodinium, which is interpreted as a response to the accelerated in vivo light exposure during bleaching. We conclude that coral microscale optics have a fundamental role in shaping the radiative exposure of Symbiodinium and thus photophysiological stress responses during coral bleaching.
All authors designed the experiment. JH and DW analyzed the data. DW, MK, DS, and JH interpreted the data. DW performed microsensor measurements and JH performed symbiont cell density and tissue surface area estimates. DW, JH, AL performed the experimental bleaching treatment. MK and PR provided new reagents, materials and tools: DW, MK, and JH wrote the paper with editorial input and consent from all co-authors.
This study was funded by a Danielle Simmons Award grant from the Australian Coral Reef Society (DW), a Science for Management Award from the Great Barrier Reef Marine Park Authority (DW), a Distinguished Postdoctoral Fellowship from the Carlsberg Foundation (DW), and a Sapere-Aude Advanced grant from the Danish Council for Independent Research | Natural Sciences (MK). The authors declare no competing interests.
The authors declare that the research was conducted in the absence of any commercial or financial relationships that could be construed as a potential conflict of interest.
We thank Unnikrishnan Kuzhiumparambil for performing the HPLC analysis and Christian Evenhuis for initial discussions. Giovanni Bernal and the staff at Heron Island research station are thanked for help with coral sample collection as well as excellent assistance in the field.
The Supplementary Material for this article can be found online at: http://journal.frontiersin.org/article/10.3389/fmicb.2017.00059/full#supplementary-material
Ed(PAR), incident downwelling photon irradiance (μmol photons m-2 s-1); E0(PAR), photon scalar irradiance (μmol photons m-2 s-1); E0(λ), spectral scalar irradiance (μmol photons m-2 s-1 nm-1); E0(PAR)bleached/E0(PAR)healthy, photon scalar irradiance enhancement of a bleached coral relative to a healthy coral; E0(λ)bleached/E0(λ)healthy, spectral scalar irradiance enhancement of a bleached coral relative to a healthy coral; LR, light respiration; NIR, near-infrared radiation; PAR, photosynthetically active radiation (400–700 nm); PN, net photosynthesis; PG, gross photosynthesis; RD, dark respiration; RH(λ), spectral holobiont reflectance; RH(λ)bleached/RH(λ)healthy, holobiont reflectance enhancement of a bleached coral relative to a healthy coral.
Abrego, D., Ulstrup, K. E., Willis, B. L., and Van Oppen, M. J. H. (2008). Species-specific interactions between algal endosymbionts and coral hosts define their bleaching response to heat and light stress. Proc. R. Soc. B 275, 2273–2282. doi: 10.1098/rspb.2008.0180
Ainsworth, T. D., Heron, S. F., Ortiz, J. C., Mumby, P. J., Grech, A., Ogawa, D., et al. (2016). Climate change disables coral bleaching protection on the Great Barrier Reef. Science 352, 338–342. doi: 10.1126/science.aac7125
Baird, A. H., Bhagooli, R., Ralph, P. J., and Takahashi, S. (2009). Coral bleaching: the role of the host. Trends Ecol. Evol. 24, 16–20. doi: 10.1016/j.tree.2008.09.005
Brodersen, K. E., Lichtenberg, M., Ralph, P. J., Kühl, M., and Wangpraseurt, D. (2014). Radiative energy budget reveals high photosynthetic efficiency in symbiont-bearing corals. J. R. Soc. Interface 11:20130997. doi: 10.1098/rsif.2013.0997
Brown, B., Dunne, R., Goodson, M., and Douglas, A. (2002). Experience shapes the susceptibility of a reef coral to bleaching. Coral Reefs 21, 119–126.
Brown, B. E. (1997). Coral bleaching: causes and consequences. Coral Reefs 16, S129–S138. doi: 10.1007/s003380050249
Chan, N., Wangpraseurt, D., Kühl, M., and Connolly, S. R. (2016). Flow and coral morphology control coral surface pH: implications for the effects of ocean acidification. Front. Mar. Sci. 3:10. doi: 10.3389/fmars.2016.00010
Dunlap, W. C., and Shick, J. M. (1998). Ultraviolet radiation-absorbing mycosporine-like amino acids in coral reef organisms: a biochemical and environmental perspective. J. Phycol. 34, 418–430. doi: 10.1046/j.1529-8817.1998.340418.x
Enriquez, S., Mendez, E. R., and Iglesias-Prieto, R. (2005). Multiple scattering on coral skeletons enhances light absorption by symbiotic algae. Limnol. Oceanogr. 50, 1025–1032. doi: 10.1364/AO.49.005032
Falkowski, P. G., Jokiel, P. L., and Kinzie, R. (1990). Irradiance and corals. Ecosyst. World 25, 89–107.
Franklin, D. J., Cedres, C. M. M., and Hoegh-Guldberg, O. (2006). Increased mortality and photoinhibition in the symbiotic dinoflagellates of the Indo–Pacific coral Stylophora pistillata (Esper) after summer bleaching. Mar. Biol. 149, 633–642. doi: 10.1007/s00227-005-0230-z
Hennige, S. J., Smith, D. J., Perkins, R., Consalvey, M., Paterson, D. M., and Suggett, D. J. (2008). Photoacclimation, growth and distribution of massive coral species in clear and turbid waters. Mar. Ecol. Prog. Ser. 369, 77–88. doi: 10.3354/meps07612
Hill, R., Larkum, A. W., Frankart, C., Kühl, M., and Ralph, P. J. (2004). Loss of functional photosystem II reaction centres in zooxanthellae of corals exposed to bleaching conditions: using fluorescence rise kinetics. Photosynth. Res. 82, 59–72. doi: 10.1023/B:PRES.0000040444.41179.09
Hoegh-Guldberg, O. (1999). Climate change, coral bleaching and the future of the world’s coral reefs. Mar. Freshw. Res. 50, 839–866. doi: 10.1071/MF99078
Hoegh-Guldberg, O., Mumby, P. J., Hooten, A. J., Steneck, R. S., Greenfield, P., Gomez, E., et al. (2007). Coral reefs under rapid climate change and ocean acidification. Science 318, 1737–1742. doi: 10.1126/science.1152509
Hoogenboom, M. O., Anthony, K. R. N., and Connolly, S. R. (2006). Energetic cost of photoinhibition in corals. Mar. Ecol. Prog. Ser. 313, 1–12. doi: 10.3354/meps313001
Hughes, T. P., Baird, A. H., Bellwood, D. R., Card, M., Connolly, S. R., Folke, C., et al. (2003). Climate change, human impacts, and the resilience of coral reefs. Science 301, 929–933. doi: 10.1126/science.1085046
Iglesias-Prieto, R., and Trench, R. K. (1994). Acclimation and adaptation to irradiance in symbiotic dinoflagellates. I. Responses of the photosynthetic unit to changes in photon flux density. Mar. Ecol. Prog. Ser. 113, 163–175. doi: 10.3354/meps113163
Jimenez, I. M., Kuhl, M., Larkum, A. W. D., and Ralph, P. J. (2008). Heat budget and thermal microenvironment of shallow-water corals: do massive corals get warmer than branching corals? Limnol. Oceanogr. 53, 1548–1561. doi: 10.4319/lo.2008.53.4.1548
Jones, R. J., Ward, S., Amri, A. Y., and Hoegh-Guldberg, O. (2000). Changes in quantum efficiency of photosystem II of symbiotic dinoflagellates of corals after heat stress, and of bleached corals sampled after the 1998 Great Barrier Reef mass bleaching event. Mar. Freshw. Res. 51, 63–71. doi: 10.1071/MF99100
Kahng, S. E., Hochberg, E. J., Apprill, A., Wagner, D., Luck, D. G., Perez, D., et al. (2012). Efficient light harvesting in deep-water zooxanthellate corals. Mar. Ecol. Prog. Ser. 455, 65–77. doi: 10.3354/meps09657
Kaniewska, P., Magnusson, S. H., Anthony, K. R. N., Reef, R., Kühl, M., and Hoegh-Guldberg, O. (2011). Importance of macro- versus microstructure in modulating light levels inside coral colonies. J. Phycol. 47, 846–860. doi: 10.1111/j.1529-8817.2011.01021.x
Kemp, D., Fitt, W., and Schmidt, G. (2008). A microsampling method for genotyping coral symbionts. Coral Reefs 27, 289–293. doi: 10.1007/s00338-007-0333-8
Kortüm, G. (2012). Reflectance Spectroscopy: Principles, Methods, Applications. New York, NY: Springer Science & Business Media.
Krueger, T., Becker, S., Pontasch, S., Dove, S., Hoegh-Guldberg, O., Leggat, W., et al. (2014). Antioxidant plasticity and thermal sensitivity in four types of Symbiodinium sp. J. Phycol. 50, 1035–1047. doi: 10.1111/jpy.12232
Kühl, M., Cohen, Y., Dalsgaard, T., Jørgensen, B. B., and Revsbech, N. P. (1995). Microenvironment and photosynthesis of zooxanthellae in scleractinian corals studied with microsensors for O2, pH and light. Mar. Ecol. Prog. Ser. 117, 159–172. doi: 10.3354/meps117159
Kühl, M., and Jørgensen, B. B. (1994). The light-field of microbenthic communities – radiance distribution and microscale optics of sandy coastal sediments. Limnol. Oceanogr. 39, 1368–1398. doi: 10.4319/lo.1994.39.6.1368
Lesser, M. P. (1996). Elevated temperatures and ultraviolet radiation cause oxidative stress and inhibit photosynthesis in symbiotic dinoflagellates. Limnol. Oceanogr. 41, 271–283. doi: 10.4319/lo.1996.41.2.0271
Lesser, M. P., and Farrell, J. H. (2004). Exposure to solar radiation increases damage to both host tissues and algal symbionts of corals during thermal stress. Coral Reefs 23, 367–377. doi: 10.1007/s00338-004-0392-z
Lichtenberg, M., Larkum, A. W. D., and Kühl, M. (2016). Photosynthetic acclimation of Symbiodinium in hospite depends on vertical position in the tissue of the scleractinian coral Montastrea curta. Front. Microbiol. 7:230. doi: 10.103389/fmixb.2016.00230
Loya, Y., Sakai, K., Yamazato, K., Nakano, Y., Sambali, H., and Van Woesik, R. (2001). Coral bleaching: the winners and the losers. Ecol. Lett. 4, 122–131. doi: 10.1046/j.1461-0248.2001.00203.x
Lyndby, N. H., Kühl, M., and Wangpraseurt, D. (2016). Heat generation and light scattering of green fluorescent protein-like pigments in coral tissue. Sci. Rep. 6:26599. doi: 10.1038/srep26599
Marcelino, L. A., Westneat, M. W., Stoyneva, V., Henss, J., Rogers, J. D., Radosevich, A., et al. (2013). Modulation of light-enhancement to symbiotic Algae by light scattering in corals and evolutionary trends in bleaching. PLoS ONE 8:e61492. doi: 10.1371/journal.pone.0061492
Middlebrook, R., Anthony, K. R., Hoegh-Guldberg, O., and Dove, S. (2010). Heating rate and symbiont productivity are key factors determining thermal stress in the reef-building coral Acropora formosa. J. Exp. Biol. 213, 1026–1034. doi: 10.1242/jeb.031633
Muscatine, L., Mccloskey, L. R., and Marian, R. E. (1981). Estimating the daily contribution of carbon from zooxanthellae to coral animal respiration. Limnol. Oceanogr. 26, 601–611. doi: 10.4319/lo.1981.26.4.0601
Nothdurft, L. D., and Webb, G. E. (2007). Microstructure of common reef-building coral genera Acropora, Pocillopora, Goniastrea and Porites: constraints on spatial resolution in geochemical sampling. Facies 53, 1–26. doi: 10.1007/s10347-006-0090-0
Ow, Y., and Todd, P. (2010). Light-induced morphological plasticity in the scleractinian coral Goniastrea pectinata and its functional significance. Coral Reefs 29, 797–808. doi: 10.1007/s00338-010-0631-4
Revsbech, N. P., and Jorgensen, B. B. (1983). Photosynthesis of benthic microflora measured with high spatial-resolution by the oxygen microprofile method - capabilities and limitations of the method. Limnol. Oceanogr. 28, 749–756. doi: 10.4319/lo.1983.28.4.0749
Rickelt, L. F., Lichtenberg, M., Trampe, E. C. L., and Kühl, M. (2016). Fiber-optic probes for small-scale measurements of scalar irradiance. Photochem. Photobiol. 92, 331–342. doi: 10.1111/php.12560
Roberty, S., Bailleul, B., Berne, N., Franck, F., and Cardol, P. (2014). PSI Mehler reaction is the main alternative photosynthetic electron pathway in Symbiodinium sp., symbiotic dinoflagellates of cnidarians. New Phytol. 204, 81–91. doi: 10.1111/nph.12903
Rodriguez-Roman, A., Hernandez-Pech, X., Thome, P. E., Enriquez, S., and Iglesias-Prieto, R. (2006). Photosynthesis and light utilization in the Caribbean coral Montastraea faveolata recovering from a bleaching event. Limnol. Oceanogr. 51, 2702–2710. doi: 10.4319/lo.2006.51.6.2702
Roth, M. S. (2014). The engine of the reef: photobiology of the coral–algal symbiosis. Front. Microbiol. 5:422. doi: 10.3389/fmicb.2014.00422
Rowan, R., Knowlton, N., Baker, A., and Jara, J. (1997). Landscape ecology of algal symbionts creates variation in episodes of coral bleaching. Nature 388, 265–269. doi: 10.1038/40843
Salih, A., Larkum, A., Cox, G., Kühl, M., and Hoegh-Guldberg, O. (2000). Fluorescent pigments in corals are photoprotective. Nature 408, 850–853. doi: 10.1038/35048564
Sampayo, E. M., Ridgway, T., Bongaerts, P., and Hoegh-Guldberg, O. (2008). Bleaching susceptibility and mortality of corals are determined by fine-scale differences in symbiont type. Proc. Natl. Acad. Sci. U.S.A. 105, 10444–10449. doi: 10.1073/pnas.0708049105
Sampayo, E. M., Ridgway, T., Franceschinis, L., Roff, G., Hoegh-Guldberg, O., and Dove, S. (2016). Coral symbioses under prolonged environmental change: living near tolerance range limits. Sci. Rep. 6:36271. doi: 10.1038/srep36271
Schrameyer, V., Wangpraseurt, D., Hill, R., Kühl, M., Larkum, A. W., and Ralph, P. J. (2014). Light respiratory processes and gross photosynthesis in two scleractinian corals. PLoS ONE 9:e110814. doi: 10.1371/journal.pone.0110814
Shashar, N., Cohen, Y., and Loya, Y. (1993). Extreme diel fluctuations of oxygen in diffusive boundary layers surrounding stony corals. Biol. Bull. 185, 455–461. doi: 10.2307/1542485
Silverstein, R. N., Correa, A. M. S., and Baker, A. C. (2012). Specificity is rarely absolute in coral–algal symbiosis: implications for coral response to climate change. Proc. R. Soc. B 279, 2609–2618. doi: 10.1098/rspb.2012.0055
Smith, E., D’angelo, C., Salih, A., and Wiedenmann, J. (2013). Screening by coral green fluorescent protein (GFP)-like chromoproteins supports a role in photoprotection of zooxanthellae. Coral Reefs 32, 463–474. doi: 10.1007/s00338-012-0994-9
Suggett, D. J., Moore, C. M., Hickman, A. E., and Geider, R. J. (2009). Interpretation of fast repetition rate (FRR) fluorescence: signatures of phytoplankton community structure versus physiological state. Mar. Ecol. Prog. Ser. 376, 1–19. doi: 10.3354/meps07830
Swain, T. D., Dubois, E., Gomes, A., Stoyneva, V. P., Radosevich, A. J., Henss, J., et al. (2016). Skeletal light-scattering accelerates bleaching response in reef-building corals. BMC Ecol. 16:1. doi: 10.1186/s12898-016-0061-4
Szabó, M., Wangpraseurt, D., Tamburic, B., Larkum, A. W. D., Schreiber, U., Suggett, D. J., et al. (2014). Effective light absorption and absolute electron transport rates in the coral Pocillopora damicornis. Plant Physiol. Bioch. 83, 159–167. doi: 10.1016/j.plaphy.2014.07.015
Takabayashi, M., and Hoegh-Guldberg, O. (1995). Ecological and physiological differences between two color morphs of the coral Pocillopora damicornis. Mar. Biol. 123, 705–714. doi: 10.1007/BF00349113
Takahashi, S., Nakamura, T., Sakamizu, M., Van Woesik, R., and Yamasaki, H. (2004). Repair machinery of symbiotic photosynthesis as the primary target of heat stress for reef-building corals. Plant Cell Physiol. 45, 251–255. doi: 10.1093/pcp/pch028
Teran, E., Mendez, E. R., Enriquez, S., and Iglesias-Prieto, R. (2010). Multiple light scattering and absorption in reef-building corals. Appl. Opt. 49, 5032–5042. doi: 10.1364/AO.49.005032
Tonk, L., Bongaerts, P., Sampayo, E. M., and Hoegh-Guldberg, O. (2013). SymbioGBR: a web-based database of Symbiodinium associated with cnidarian hosts on the Great Barrier Reef. BMC Ecol. 13:7. doi: 10.1186/1472-6785-13-7
Ulstrup, K. E., Ralph, P. J., Larkum, A. W. D., and Kuhl, M. (2006). Intra-colonial variability in light acclimation of zooxanthellae in coral tissues of Pocillopora damicornis. Mar. Biol. 149, 1325–1335. doi: 10.1007/s00227-006-0286-4
Wangpraseurt, D., Jacques, S., Petri, T., and Kuhl, M. (2016a). Monte Carlo modeling of photon propagation reveals highly scattering coral tissue. Front. Plant Sci. 7:1404. doi: 10.3389/fpls.2016.01404
Wangpraseurt, D., Larkum, A. W., Franklin, J., Szabó, M., Ralph, P. J., and Kühl, M. (2014a). Lateral light transfer ensures efficient resource distribution in symbiont-bearing corals. J. Exp. Biol. 217, 489–498. doi: 10.1242/jeb.091116
Wangpraseurt, D., Larkum, A. W., Ralph, P. J., and Kühl, M. (2012). Light gradients and optical microniches in coral tissues. Front. Microbiol. 3:316. doi: 10.3389/fmicb.2012.00316
Wangpraseurt, D., Polerecky, L., Larkum, A. W., Ralph, P. J., Nielsen, D. A., Pernice, M., et al. (2014b). The in situ light microenvironment of corals. Limnol. Oceanogr. 59, 917–926. doi: 10.4319/lo.2014.59.3.0917
Wangpraseurt, D., Tamburic, B., Szabó, M., Suggett, D., Ralph, P. J., and Kühl, M. (2014c). Spectral effects on Symbiodinium photobiology studied with a programmable light engine. PLoS ONE 9:e112809. doi: 10.1371/journal.pone.0112809
Wangpraseurt, D., Wentzel, C., Jacques, S. L., Wagner, M., and Kuhl, M. (2016b). In vivo imaging of coral tissue and skeleton with optical coherence tomography. bioRxiv doi: 10.1101/088682
Warner, M. E., Fitt, W. K., and Schmidt, G. W. (1996). The effects of elevated temperature on the photosynthetic efficiency of zooxanthellae in hospite from four different species of reef coral: a novel approach. Plant Cell Environ. 19, 291–299. doi: 10.1111/j.1365-3040.1996.tb00251.x
Warner, M. E., Fitt, W. K., and Schmidt, G. W. (1999). Damage to photosystem II in symbiotic dinoflagellates: a determinant of coral bleaching. Proc. Natl. Acad. Sci. U.S.A. 96, 8007–8012. doi: 10.1073/pnas.96.14.8007
Weis, V. M. (2008). Cellular mechanisms of Cnidarian bleaching: stress causes the collapse of symbiosis. J. Exp. Biol. 211, 3059–3066. doi: 10.1242/jeb.009597
Keywords: coral bleaching, Symbiodinium, photosynthesis, light scattering, coral optics, ecophysiology
Citation: Wangpraseurt D, Holm JB, Larkum AWD, Pernice M, Ralph PJ, Suggett DJ and Kühl M (2017) In vivo Microscale Measurements of Light and Photosynthesis during Coral Bleaching: Evidence for the Optical Feedback Loop? Front. Microbiol. 8:59. doi: 10.3389/fmicb.2017.00059
Received: 14 October 2016; Accepted: 09 January 2017;
Published: 24 January 2017.
Edited by:
Virginia Weis, Oregon State University, USAReviewed by:
Melissa Susan Roth, University of California, Berkeley, USACopyright © 2017 Wangpraseurt, Holm, Larkum, Pernice, Ralph, Suggett and Kühl. This is an open-access article distributed under the terms of the Creative Commons Attribution License (CC BY). The use, distribution or reproduction in other forums is permitted, provided the original author(s) or licensor are credited and that the original publication in this journal is cited, in accordance with accepted academic practice. No use, distribution or reproduction is permitted which does not comply with these terms.
*Correspondence: Daniel Wangpraseurt, d2FuZ3ByYXNldXJ0QGdtYWlsLmNvbQ== Michael Kühl, bWt1aGxAYmlvLmt1LmRr
†Present address: Daniel Wangpraseurt Department of Chemistry, University of Cambridge, Cambridge, UK
Disclaimer: All claims expressed in this article are solely those of the authors and do not necessarily represent those of their affiliated organizations, or those of the publisher, the editors and the reviewers. Any product that may be evaluated in this article or claim that may be made by its manufacturer is not guaranteed or endorsed by the publisher.
Research integrity at Frontiers
Learn more about the work of our research integrity team to safeguard the quality of each article we publish.