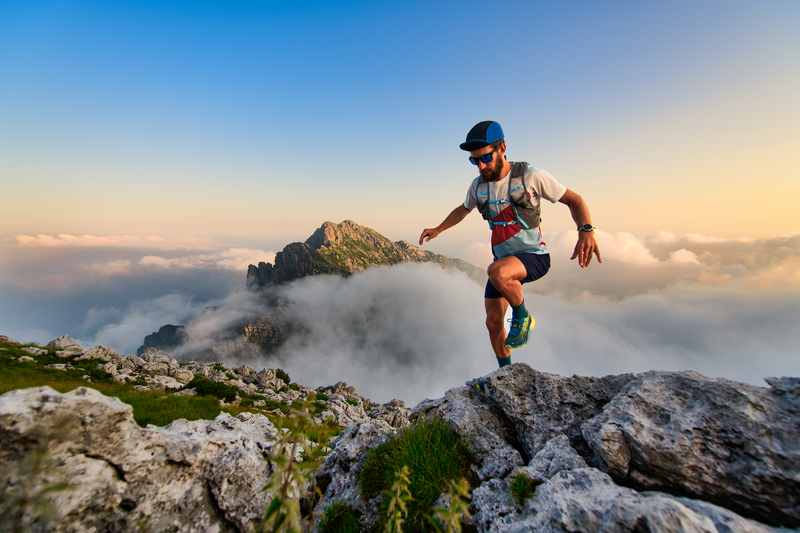
95% of researchers rate our articles as excellent or good
Learn more about the work of our research integrity team to safeguard the quality of each article we publish.
Find out more
ORIGINAL RESEARCH article
Front. Microbiol. , 19 January 2017
Sec. Microbiotechnology
Volume 8 - 2017 | https://doi.org/10.3389/fmicb.2017.00009
This article is part of the Research Topic Electrochemically active microorganisms View all 20 articles
Microbial fuel cells (MFCs) have attracted much attention due to their ability to generate electricity while treating wastewater. The performance of a double-chamber MFC with simultaneous nitrification and denitrification (SND) in the cathode for treating synthetic high concentration ammonia wastewater was investigated at different dissolved oxygen (DO) concentrations and high temperatures. The results showed that electrode denitrification and traditional heterotrophic denitrification co-existed in the cathode chamber. Electrode denitrification by aerobic denitrification bacterium (ADB) is beneficial for achieving a higher voltage of the MFC at high DO concentrations (3.0–4.2 mg/L), while traditional heterotrophic denitrification is conducive to higher total nitrogen (TN) removal at low DO (0.5–1.0 mg/L) concentrations. Under high DO conditions, the nitrous oxide production and TN removal efficiency were higher with a 50 Ω external resistance than with a 100 Ω resistance, which demonstrated that electrode denitrification by ADB occurred in the cathode of the MFC. Sufficient electrons were inferred to be provided by the electrode to allow ADB survival at low carbon:nitrogen ratios (≤0.3). Polymerase chain reaction-denaturing gradient gel electrophoresis (PCR-DGGE) results showed that increasing the DO resulted in a change of the predominant species from thermophilic autotrophic nitrifiers and facultative heterotrophic denitrifiers at low DO concentrations to thermophilic ADB at high DO concentrations. The predominant phylum changed from Firmicutes to Proteobacteria, and the predominant class changed from Bacilli to Alpha, Beta, and Gamma Proteobacteria.
Microbial fuel cells (MFCs) have gained widespread attention as an innovative wastewater treatment and energy recovery technology that combines sewage purification and electricity production (Janicek et al., 2014; Li et al., 2014). Recent studies have shown that nitrate and nitrite can be removed from wastewater as electron acceptors in the cathode of an MFC through electrochemical reduction or autotrophic denitrification (Zhang and Angelidaki, 2012). Several developments of nitrogen removal with MFCs have been achieved with various designs and configurations (He et al., 2009; Virdis et al., 2010; Zhang and Angelidaki, 2013). In the studies of Bernardino Virdis et al., the cathodic process with in situ nitrification through specific aeration attained simultaneous nitrification and denitrification (SND) in one half-cell (Virdis et al., 2010). Although, nitrogen recovery with MFCs through NH3 stripping has been successfully developed to simultaneously produce energy and recover ammonium (Kuntke et al., 2012; Zhang and Angelidaki, 2015), SND in cathode of MFCs and its some new biochemical mechanisms still remain valuable to explore. Studies of simultaneous phenol removal, nitrification and denitrification using MFCs have indicated that phenol-degrading bacteria, nitrifiers, and denitrifiers in the aerobic cathode chamber are responsible for phenol oxidation, aerobic nitrification and aerobic denitrification, respectively (Feng et al., 2015). The impact of dissolved oxygen (DO) on the SND process in the cathode of an MFC has also been investigated comprehensively (Virdis et al., 2010). Because the bacteria may evolve during long-term operation, the impact of DO on the performance of cathode denitrification is different from that over shorttime periods. The SND mechanism in the aerobic cathode chamber is complex and remains unclear.
In the traditional theory of biological nitrogen removal, ammonia is first oxidized to nitrate by autotrophic nitrifiers, and the nitrate is then reduced to nitrogen by heterotrophic denitrifiers (Robertson and Kuenen, 1984). Based on the different growth conditions of nitrifiers and denitrifiers, the traditional theory of biological nitrogen removal makes a strict distinction between the nitrification and denitrification processes. The former is carried out under aerobic conditions, while the latter requires anaerobic conditions. Therefore, it is impossible for the two reactions to occur simultaneously in the same reactor. However, the discovery of heterotrophic nitrifiers and aerobic denitrifiers has made it possible for nitrification and denitrification to occur simultaneously (Huang et al., 2013; Li et al., 2015). Heterotrophic nitrifying bacteria can produce hydroxylamine, nitrite and nitrate by nitrification using organic carbon as a source for growth, and most of these bacteria can also directly convert nitrifying products to nitrogen gas through the process of aerobic denitrification (Papen and Von Berg, 1998). Aerobic denitrification bacterium (ADB) can use aerobic denitrifying enzymes for denitrification under aerobic conditions (Robertson et al., 1988; Bell and Ferguson, 1991).
In the 1980s, Robertson and Kuenen (1984) isolated the aerobic denitrifiers Thiosphaera pantotropha, Pseudomonas spp. and Alcaligenes faecalis for the first time and reported the existence of the aerobic denitrifying enzyme system (Robertson et al., 1988). They also confirmed that the growth rate of Paracoccus denitrificans will be higher in the presence of O2 and . Bell and Ferguson (1991) demonstrated that aerobic denitrifying enzymes were more active in the presence of O2, and Meiberg et al. (Ferguson, 1994) reported that denitrification could be carried out by Hyphomicrobium X under aerobic conditions. Many studies have proved the existence of ADB (Chen et al., 2003; Kim et al., 2005) and found that some denitrifiers survive under high O2 concentration conditions (Takaya et al., 2003). Certain groups of bacteria, such as Bacillus, P. putida, P. stutzeri, Hydrogenophaga, and Achromobacter, have been shown to have heterotrophic nitrification and aerobic denitrification abilities and to convert ammonium to nitrogen aerobically in the cathode chamber of an MFC (Feng et al., 2015). Nevertheless, few attempts have been made to attain SND at high temperatures. Because some wastewater, similar to sludge digestion solutions and effluents of anaerobic reactors that treat landfill leachate, contains high concentrations of ammonia at high temperatures, studies of SND and the performance of ADB in the cathode of an MFC at high temperatures are important.
This study investigated the performance of a double-chamber MFC with SND in the cathode at fluctuating high temperatures (36–48°C). Synthetic wastewater that contained organics and high concentrations of ammonia was fed into the anode chamber and then turned into the cathode chamber. The denitrification characteristics were studied by comparing scenarios with two ranges of DO concentrations (0.5–1.0 and 3.0–4.2 mg/L) and scenarios with two external resistances (50 and 100 Ω) at high DO concentrations. The microbial communities at the two DO concentrations in the cathode of the MFC were identified with polymerase chain reaction-denaturing gradient gel electrophoresis (PCR-DGGE) to explore the evolution of the dominant bacteria.
The MFC device was constructed with cathode and anode chambers. The anode and cathode chambers were both made of organic glass tube 8 cm high and 9 cm in diameter and had an effective volume of 0.452 L (Figure 1). Each chamber used a carbon brush as the electrode. The two chambers were separated by a proton exchange membrane (Nafion 117) and placed in a water bath. The temperature was initially set to 31 ± 1°C and then changed to a dynamic temperature (36–48°C) later in the operation. The cathode and anode chambers were connected with a manual variable resistor (0–9999 Ω) to close the circuit. The cathode chamber was exposed to air, and blast aeration was used. The influent was injected into the anode chamber using a peristaltic pump (YZ1515X, Lange), and the effluent from the anode was fed into the cathode chamber.
The influent for the MFC reactor was an artificially simulated high strength ammonia sludge digestion solution with components of 0.38 g/L CH3COONa, 2.708 g/L NH4HCO3, 0.33 g/L KH2PO4, 1 g/L K2HPO4·3H2O, 1 g/L KCl, 1.5 g/L NaHCO3, 0.016 g/L CaCl2, and 1 ml/L trace nutrient solution. CH3COONa and NH4HCO3 were added to maintain the chemical oxygen demand (COD) and the ammonia nitrogen concentrations in the influent at 300 and 480 mg/L, respectively.
The anode and cathode chambers of the MFC were inoculated with aerobic sludge from the aeration tank of the Fourth Wastewater Treatment Plant in Xi'an, China. Before operating the MFC, the anode and cathode were soaked in the inoculation sludge for 24 h. Once the reactor was filled with synthetic wastewater, the MFC entered the stage of static culture without the influent while keeping the cathode aerated and the inner circuit open. The concentrations of DO, -N, -N, and -N and the pH of the cathode electrolyte were measured daily. After more than half of the original -N was converted to -N in the cathode electrolyte, the aeration mode was changed from continuous to intermittent (2 h aeration and 2 h static), and the wastewater was pumped continuously into the anode chamber with hydraulic retention times (HRTs) of 10.4 h for both the cathode and the anode chambers. After a period of continuous operation, stable partial nitrification was obtained in the cathode chamber of the MFC. An external resistance of 100 Ω was then connected, following which the operation of MFC with SND started.
The anode potential was monitored with a saturated calomel electrode (SCE, +0.242 V standard hydrogen electrode; Type 232, Leici Instrument Factory, Shanghai, China). -N, -N and -N were measured according to Standard Methods for the Examination of Water and Wastewater (Clesceri et al., 1998). DO was determined using a Hach-HQ30d DO analyzer (HACH, America). The voltage and anode potential were monitored and recorded using a PCI1717 voltage collector (Yanhua Company, Shenzhen, China). An N2O microsensor (Unisense, Denmark) was used for the N2O analysis.
Samples from the biofilm of the cathode were collected on day 27 and day 83 to investigate the microbial community with DGGE, and DNA was extracted using a fast DNA spin kit (SK8233) for soil according to the manufacturer's instructions. The bacterial 16S rRNA genes were amplified by PCR with the universal primers F357-GC (5′-CGCCCGCCGCGCCCCGCGCCCGGCCCGCCGCCCCG
CCCCCCTACGGGAGGCAGCAG-3′) and R518 (5′-ATTACCGCGGCTGCTGG-3′). A polyacrylamide gel (8%) with a 30–60% denaturing gradient was used to separate the PCR products (7 mol L-1 urea and 40% formamide comprising 100% denaturant), which were analyzed using DGGE technology and washed with ultrapure water to flush the gel and dye. Eight representative DGGE strips were selected by a clean scalpel and transferred to a 1.5 mL centrifuge tube. The target DNA fragments were then excised and reamplified using the primer sets F357 (5′-CCTACGGGAGGCAGCAG-3′) and R518 (5′-ATTACCGCGGCTGCTGG-3′), and the obtained sequence was matched with the Seqmatch database for sequence alignment. The homology information of each strip was obtained by Sangon Biotech Co., Ltd. (Shanghai, China).
The results of the continuous operation test are shown in Figure 2.
In stage 1 (first 5 days of the test), the MFC with SND was operated at 31 ± 1°C, 100 Ω resistance and intermittent aeration (2 h aeration and 2 h static), and the DO of the catholyte was 0.5–1.0 mg/L. The concentrations of -N and -N decreased with a gradual increase in the release voltage of the MFC. However, the removal of total nitrogen (TN) was much greater than that with the electrical current of the MFC. This finding implies that the traditional heterotrophic microbial denitrification with COD was more efficient at removing nitrogen than the electrode denitrification in the cathode chamber. The moderate temperature (31 ± 1°C) and low DO (0.5–1.0 mg/L) in the cathode may be beneficial for SND with COD still present in the anode effluent.
In stage 2 (days 6–34), the temperature of the reactor increased and fluctuated over the range of 36–48°C. The removal of TN began to increase sharply, and the concentration of -N in the cathode effluent began to decrease correspondingly from the 6th day, while the voltage of the MFC increased slightly and then stabilized. This might have been due to the high temperature in the cathode (36–48°C), which was harmful for the growth of normal ammonia oxidizing bacteria (AOB). Because the suitable range of temperatures for AOB metabolism is 20–30°C, the nitrification rate decreased, and the -N concentration decreased to approximately 30 mg/L. The increase in the TN removal was mainly caused by the volatilization of ammonium at high temperature. The TN removal from the denitrification in the electrode during stage 2 was lower and similar to that at the end of stage 1 (not greater than 7 mg/L·d based on the MFC voltage). The biofilm was sampled, and the bacteria were identified with PCR-DGGE. The predominant species was found to be Ureibacillus thermosphaericus of the genus Ureibacillus, which grows at temperatures of 37° to 60°C (Fortina et al., 2001).
In stage 3 (days 35–77), the reactor was set to continuous aeration instead of intermittent aeration. As a result, the DO increased to 3 ± 0.6 mg/L. The amount of TN removed per day began to decrease, but the voltage continued to increase. This might have occurred because the heterotrophic denitrification with COD as the electron donor was inhibited by the increase of DO, and the partial oxygen accepted electrons from the electrode. In stage 3, the release voltage initially increased, then dropped and finally steadied at approximately 100 mV, which was much higher than that in stage 2. The following strain sampling clearly demonstrated that the predominant species changed to aerobic denitrifiers (a detailed analysis is provided later). The curves in Figure 2 suggest that the aerobic denitrifiers might have replaced the anaerobic denitrifiers in the latter phase of stage 3. The aerobic denitrifiers appeared to be much more receptive to the electrons from the electrode than the anaerobic denitrifiers, which was determined by comparing the voltage of stage 2 with that of stage 3, in which the effects of electron acceptance by oxygen was taken into account. In contrast, TN removal by anaerobic denitrifiers was much greater than that by aerobic denitrifiers. The heterotrophic denitrification with COD might have mainly caused TN removal, excluding the effect of volatilization in the cathode. Depending on the voltage, the TN removal by electrode denitrification was 8.5–9.0 mg/(L·d), which represented only a small part of the TN removal in stage 3.
The conditions in stage 4 (days 78–88) remained the same as those in stage 3 except for the change in the resistance of the MFC from 100 to 50 Ω. The results showed that the concentrations of -N and -N in the cathode effluent decreased, and the amount of TN removed increased in the latter part of this stage (Figure 2). The voltages were greater than those in stage 3, but the TN removal by electrode denitrification was 13.5–14.6 mg/(L·d), which was greater than that in stage 3.
The electricity production performance of the MFC under high DO conditions and different external resistances was investigated as a case study (Figures 3–5, which correspond to days 75, 82, and 97 in Figure 2, respectively). The voltage was positively related to the increase in temperature under external resistances of 50 Ω and 100 Ω, while it was independent of the temperature under open circuit conditions. Regardless of whether the resistances were applied or an open circuit was used, the N2O emissions were always positively related to the increase in temperature, while the DO was always negatively related to the increase in temperature. The characteristics of denitrification and electricity production of the MFC can be evaluated using these factors.
Figure 3. Profiles of temperature, DO, N2O, voltage, and anode potential of the MFC with 100 Ω external resistance at temperatures of 36–48°C (day 75 in Figure 2). (A) Temperature profile; (B) DO and N2O profiles; (C) voltage profile; (D) anode potential profile.
The fluctuations in the concentrations of DO and N2O caused by the temperature (Figures 3, 5) show that the DO fluctuation amplitude was approximately 1.2 mg/L, whereas the corresponding N2O fluctuation was approximately 0.4 mg/L in the open circuit. With a 100 Ω resistance, the DO and N2O fluctuation amplitudes were 1.5 mg/L and 0.3 mg/L, respectively. This demonstrated that the reduction in oxygen caused by the voltage fluctuation was approximately 0.3 mg O2/L, and no N2O was produced by electrode denitrification. The concentrations of N2O were similar in both scenarios (0.7–1.1 mg/L), whereas the concentrations of DO with a 100 Ω resistance were 0.7–1.0 mg/L, which was lower than that in the open circuit (Figures 3, 5). This indicated that the decrease in DO was dependent on the electrode reaction, while the production of N2O was independent of the electrode reaction. Using Coulomb's law, with a 100 Ω resistance, the electrode reduction rate of oxygen was calculated to be 1.6–2.3 mg O2/(L·d), whereas the rate of electrode reduction of nitrite to nitrogen gas was 8.5–9.0 mg N/(L·d) with no production of N2O.
The fluctuations in the concentrations of DO and N2O caused by temperature (Figures 4, 5) show that the decrease of DO and the increase of N2O were both dependent on the electrode reaction. Using Coulomb's law, with a 50 Ω resistance, the electrode reduction rate of oxygen was calculated to be 2.8–4.4 mg O2/(L·d), whereas the rate of electrode denitrification was 13.5–14.6 mg N/(L·d), in which approximately 10% of the nitrogen removed was converted to N2O and 90% was converted to nitrogen gas.
Figure 4. Profiles of temperature, DO, N2O, voltage, and anode potential of the MFC with 50 Ω external resistance at temperatures of 36–48°C (day 82 in Figure 2). (A) Temperature profile; (B) DO and N2O profiles; (C) voltage profile; (D) anode potential profile.
Figure 5. Profiles of temperature, DO, N2O, voltage, and anode potential of the MFC in open circuit at temperatures of 36–48°C (day 97 in Figure 2). (A) Temperature profile; (B) DO and N2O profiles; (C) voltage profile; (D) anode potential profile.
The N2O production and TN removal efficiencies with a 50 Ω external resistance were higher than those with a 100 Ω resistance, which illustrates that electrode denitrification occurred in the cathode of the MFC. These analyses indicate that both oxygen and nitrite can obtain electrons simultaneously from the electrode in the cathode of the MFC.
Biofilm samples were collected from the cathode chamber of the MFC on day 27 in stage 2 (Figure 2) and day 83 in stage 4 (Figure 2). The representative DGGE strips are shown in Figure 6. The closest species and classification of each representative band in the DGGE profile were deposited in the GenBank database (Tables 1, 2). Sequences from DGGE bands are shown in Supplementary Material.
Figure 6. Representative DGGE strips of samples. (A) Sampling at low DO levels (first sampling); (B) sampling at high DO levels (second sampling).
Table 1. Identification of DGGE bands under low DO conditions (the first sampling; Figure 6A).
Table 2. Identification of DGGE bands under high DO conditions (second sampling; Figure 6B).
In the first microbial identification (27th day), which corresponded to the low DO and high temperature MFC operating conditions (stage 2 in Figure 2), 12 bands were identified (Table 1). The results showed that the microbial community could be divided into 4 phyla, 4 classes and 8 genera. The phylum Firmicutes (bands 2, 3, 4, 5, 7, 8, 9, 10, and 13) was the predominant bacteria, while the phyla Ignavibacteriae (band 1), Chloroflexi (band 6) and Proteobacteria (band 14) were the subdominant groups.
Within the Firmicutes phylum, bands 2, 4, 5, 7, 8, and 9 belonged to the genus Ureibacillus, related to a species of U. thermosphaericus (similarity 91.2–100%), which was reported to be a thermophilic bacteria (37–60°C, optimum 50–60°C) with heterotrophic growth in aerobic environments and no ability for nitrate reduction (Fortina et al., 2001). This species was inferred to dominate the nitrification in the cathode of the MFC under low DO and high temperature conditions.
Bands 1 and 6 belonged to the genera Ignavibacterium and Unclassified Anaerolineaceae, respectively, which grow under anaerobic conditions, are heterotrophic and cannot utilize nitrate as electron acceptors (Yamada et al., 2006; Iino et al., 2010). These species were inferred to dominate the anaerobic degradation of COD.
Bands 3 (1) and 13 belonged to the genera Geobacillus and Anoxybacillus, respectively, which grow under aerobic conditions, are heterotrophic and can reduce nitrate (Poli et al., 2006; Inan et al., 2013). These species were inferred to dominate the aerobic denitrification.
Bands 3 (2) and 10 belonged to the genera Anoxybacillus and Bacillus, respectively, which grow under anaerobic conditions, are heterotrophic and can reduce nitrate (Kim et al., 1998; Cihan et al., 2014). These two species were inferred to be responsible for the anaerobic denitrification in stage 2 (Figure 2).
Band 14 belonged to the genus Cucumibacter, which grows under aerobic conditions, is heterotrophic and cannot utilize nitrate as electron acceptors (Hwang and Cho, 2008). This species was inferred to be responsible for the aerobic degradation of COD in stage 2 (Figure 2).
In the second microbial identification (83th day), which corresponded to the high DO and high temperature MFC operating conditions (stage 4 in Figure 2), 7 bands were identified (Table 2). The results showed that the microbial community could be divided into 1 phylum, 7 classes and 8 genera. The phylum Proteobacteria (bands 15, 18, 24, 25, 26, 27, and 28) was the predominant and unique phylum. This result agrees with other studies that indicated that Proteobacteria dominates some cathodic denitrifying biofilms (Wrighton et al., 2010).
In the phylum Proteobacteria, bands 15, 24 and 27 belonged to the genera Aquamicrobium, Alishewanella, and Brachymonas or Comamonas, respectively. These three species can grow under aerobic conditions, are heterotrophic, can utilize nitrate as electron acceptors (Hiraishi et al., 1995; Roh et al., 2009; Jin et al., 2013)and were inferred to dominate the aerobic denitrification under the high DO conditions of stage 4 (Figure 2). In particular, Comamonas denitrificans (band 27) has been reported to switch the metabolic pathway for extracellular electron transfer (Xing et al., 2010). A species in the Comamonas genus is known to be an aerobic denitrifier, and C. denitrificans is the only species in the Comamonas genus that can reduce nitrate to nitrogen gas and contains cd1-type nitrite reductase (Gumaelius et al., 2001). Therefore, we propose that C. denitrificans be considered a species of ADB. The other two species were inferred to belong to ADB.
Bands 18, 26 and 28 belonged to the genera Pelomonas, Altererythrobacter and Acinetobacter, respectively. These three species can grow under aerobic conditions, are heterotrophic, cannot utilize nitrate as electron acceptors (Xie and Yokota, 2005; Kwon et al., 2007; Nemec et al., 2009) and might have dominated the aerobic degradation of COD in stage 4 (Figure 2).
Band 25 belonged to the genus Brevundimonas, which grows under aerobic conditions and cannot reduce nitrate, and 90% of the strains are autotrophic (Segers et al., 1994). This species was inferred to be a nitrifier.
Based on this analysis, at high temperatures, the increase in DO resulted in a change in the predominant species from thermophilic autotrophic nitrifiers and facultative heterotrophic denitrifiers at low DO concentrations to thermophilic ADB at high DO concentrations during the operation of the MFC. Three species from the genera Aquamicrobium, Brachymonas or Comamonas, and Alishewanella were inferred to belong to ADB and dominate the aerobic denitrification at high levels of DO. Some aerobic denitrifiers were known to be heterotrophic nitrifiers, which might benefit SND under aerobic conditions. Therefore, autotrophic nitrifiers were replaced, and ADB evolved to be the predominant bacteria at high DO concentrations in stage 4 of the operation. This result is similar to the study by Feng et al., who indicted that aerobic denitrification in the cathode chamber is an important pathway for nitrite and nitrate removal (Feng et al., 2015).
Based on the analysis of the composition of the microbial community and the experimental results, we speculated on the possible reactions in the cathode of the MFC (Figure 7).
Figure 7. Mechanisms in the cathode chamber of the MFC. The numbers in the circles represent the quantities of DGGE bands that correspond to the identified bacteria.
The mechanism of aerobic denitrification was determined by studying the aerobic denitrifier T. pantotropha. The cooperative breathing theory, which was proposed by Robertson et al. (1988), is widely recognized as the aerobic denitrification mechanism. Cooperative breathing theory means that both and O2 can be used as the final electron receptors. Therefore, the denitrifiers can transfer electrons from the reduced substance to or O2, and the denitrification can occur in an aerobic environment. According to the electron transport model proposed by Willson and Bouwer (1997), both and O2 can be used as the final electron receptors, while the denitrifiers can transfer electrons from the reduced substance to O2 or through nitrate reductase. Moreover, a carbon source is required for ADB. The higher the concentration of the carbon source is, the faster the aerobic denitrification rate will be (Robertson and Kuenen, 1983). Huang and Tseng (2001) indicated that the denitrification rate was highest when C/N was 5 and decreased with increasing C/N when C/N was greater than 5 and acetate was used as the carbon source. However, ADB did not appear to be required at such a high C/N ratio in our study. The influent C/N for the cathode chamber of the MFC was less than 0.3, possibly because the electrons provided by the electrode of the MFC were sufficient for the growth of aerobic bacteria. The aeration cathode chamber of the MFC may be beneficial for the growth of ADB.
Huang et al. indicated that DO was a key factor for aerobic denitrification (Xing et al., 2010). DO concentrations of 2–6 mg/L were beneficial for the growth of aerobic bacteria and the denitrification performance. As a result of the coexistence of aerobic respiration and the denitrifying reductase in one system, both O2 and were indispensable for the growth of ADB and were used as electron acceptors. Therefore, at the high DO levels in this study (3.0–4.2 mg/L), the activity of ADB improved, and the denitrification performance of ADB was enhanced. The results that showed that the production of N2O and the TN removal efficiency were higher with a 50 Ω external resistance than with a 100 Ω resistance at high levels of DO demonstrated that electrode denitrification with ADB occurred in the cathode of the MFC.
The increase of DO resulted in a change in the predominant species from thermophilic autotrophic nitrifiers and facultative heterotrophic denitrifiers at low DO levels to thermophilic ADB at high DO levels in the cathode of the MFC. The predominant phylum changed from Firmicutes to Proteobacteria, and the predominant class changed from Bacilli to Alpha, Beta, and Gamma Proteobacteria.
ADB is beneficial for achieving higher MFC voltages under high DO conditions, while traditional heterotrophic denitrification is conducive to higher TN removal under low DO conditions.
SND in the aeration cathode of the MFC may be beneficial for the growth of ADB.
JZ conceived and designed the experiments, and JW and SW performed the experiments. JW analyzed the data and wrote the paper, and JZ, SW, XL, BH, and XD reviewed and edited the manuscript. All of the authors approved the manuscript to be published and agreed to be accountable for all aspects of the work and for questions related to the accuracy of the results.
The authors declare that the research was conducted in the absence of any commercial or financial relationships that could be construed as a potential conflict of interest.
This study was supported by the Shaanxi Province Science and Technology Development Program (2014K15-03-02).
The Supplementary Material for this article can be found online at: http://journal.frontiersin.org/article/10.3389/fmicb.2017.00009/full#supplementary-material
Bell, L. C., and Ferguson, S. J. (1991). Nitric and nitrous oxide reductases are active under aerobic conditions in cells of Thiosphaera pantotropha. Biochem. J. 273, 423–427. doi: 10.1042/bj2730423
Chen, F., Xia, Q., and Ju, L. K. (2003). Aerobic denitrification of Pseudomonas aeruginosa monitored by online NAD(P)H fluorescence. Appl. Environ. Microbiol. 69, 6715–6722. doi: 10.1128/AEM.69.11.6715-6722.2003
Cihan, A. C., Cokmus, C., Koc, M., and Ozcan, B. (2014). Anoxybacillus calidus sp. nov., a thermophilic bacterium isolated from soil near a thermal power plant. Int. J. Syst. Evol. Microbiol. 64(Pt 1), 211–219. doi: 10.1099/ijs.0.056549-0
Clesceri, L., Greenberg, A. E., and Eaton, A. D. (1998). Standard Methods for the Examination of Water and Wastewater, 20th Edn. Washington, DC: American Public Health Association.
Feng, C., Huang, L., Yu, H., Yi, X., and Wei, C. (2015). Simultaneous phenol removal, nitrification and denitrification using microbial fuel cell technology. Water Res. 76, 160–170. doi: 10.1016/j.watres.2015.03.001
Ferguson, S. J. (1994). Denitrification and its control. Antonie Van Leeuwenhoek. 66, 89–110. doi: 10.1007/BF00871634
Fortina, M. G., Pukall, R., Schumann, P., Mora, D., Parini, C., Manachini, P. L., et al. (2001). Ureibacillus gen. nov., a new genus to accommodate Bacillus thermosphaericus (Andersson et al. 1995), emendation of Ureibacillus thermosphaericus and description of Ureibacillus terrenus sp. nov. Int. J. Syst. Evol. Microbiol. 51, 447–455. doi: 10.1099/00207713-51-2-447
Gumaelius, L., Magnusson, G., Pettersson, B., and Dalhammar, G. (2001). Comamonas denitrificans sp. nov., an efficient denitrifying bacterium isolated from activated sludge. Int. J. Syst. Evol. Microbiol. 51, 999–1006. doi: 10.1099/00207713-51-3-999
He, Z., Kan, J., Wang, Y., Huang, Y., Mansfeld, F., and Nealson, K. H. (2009). Electricity production coupled to ammonium in a microbial fuel cell. Environ. Sci. Technol. 43, 3391–3397. doi: 10.1021/es803492c
Hiraishi, A., Sugiyama, J., and Shin, Y. K. (1995). Brachymonas denitrificans gen. nov., sp. nov., an aerobic chemoorganotrophic bacterium which contains rhodoquinones, and evolutionary relationships of rhodoquinone producers to bacterial species with various quinone classes. J. Gen. Appl. Microbiol. 41, 99–117. doi: 10.2323/jgam.41.99
Huang, H. K., and Tseng, S. K. (2001). Nitrate reduction by Citrobacter diversus under aerobic environment. Appl. Microbiol. Biotechnol. 55, 90–94. doi: 10.1007/s002530000363
Huang, X., Li, W., Zhang, D., and Qin, W. (2013). Ammonium removal by a novel oligotrophic Acinetbacter sp. Y16 capable of heterotrophic nitrification-aerobic denitrification at low temperature. Bioresour. Technol. 146, 44–50. doi: 10.1016/j.biortech.2013.07.046
Hwang, C. Y., and Cho, B. C. (2008). Cucumibacter marinus gen. nov., sp. nov., a marine bacterium in the family Hyphomicrobiaceae. Int. J. Syst. Evol. Microbiol. 58, 1591–1597. doi: 10.1099/ijs.0.65587-0
Iino, T., Mori, K., Uchino, Y., Nakagawa, T., Harayama, S., and Suzuki, K. (2010). Ignavibacterium album gen. nov., sp. nov., a moderately thermophilic anaerobic bacterium isolated from microbial mats at a terrestrial hot spring and proposal of Ignavibacteria classis nov., for a novel lineage at the periphery of green sulfur bacteria. Int. J. Syst. Evol. Microbiol. 60, 1376–1382. doi: 10.1099/ijs.0.012484-0
Inan, K., Belduz, A. O., and Canakci, S. (2013). Anoxybacillus kaynarcensis sp. nov., a moderately thermophilic, xylanase producing bacterium. J. Basic Microbiol. 53, 410–419. doi: 10.1002/jobm.201100638
Janicek, A., Fan, Y., and Liu, H. (2014). Design of microbial fuel cells for practical application: a review and analysis of scale-up studies. Biofuels 5, 79–92. doi: 10.4155/bfs.13.69
Jin, H. M., Kim, J. M., and Jeon, C. O. (2013). Aquamicrobium aestuarii sp. nov., a marine bacterium isolated from a tidal flat. Int. J. Syst. Evol. Microbiol. 63(Pt 11), 4012–4017. doi: 10.1099/ijs.0.048561-0
Kim, J. K., Park, K. J., Cho, K. S., Nam, S. W., Park, T. J., and Bajpai, R. (2005). Aerobic nitrification-denitrification by heterotrophic Bacillus strains. Bioresour. Technol. 96, 1897–1906. doi: 10.1016/j.biortech.2005.01.040
Kim, Y. O., Kim, H. K., Bae, K. S., Yu, J. H., and Oh, T. K. (1998). Purification and properties of a thermostable phytase from Bacillus sp. DSll. Enzyme Microb. Technol. 22, 2–7. doi: 10.1016/S0141-0229(97)00096-3
Kuntke, P., Smiech, K. M., Bruning, H., Zeeman, G., Saakes, M., Sleutels, T. H., et al. (2012). Ammonium recovery and energy production from urine by a microbial fuel cell. Water Res. 46, 2627–2636. doi: 10.1016/j.watres.2012.02.025
Kwon, K. K., Woo, J. H., Yang, S. H., Kang, J. H., Kang, S. G., Kim, S. J., et al. (2007). Altererythrobacter epoxidivorans gen. nov., sp. nov., an epoxide hydrolase-active, mesophilic marine bacterium isolated from cold-seep sediment, and reclassification of Erythrobacter luteolus Yoon et al. 2005 as Altererythrobacter luteolus comb. nov. Int. J. Syst. Evol. Microbiol. 57, 2207–2211. doi: 10.1099/ijs.0.64863-0
Li, C., Yang, J., Wang, X., Wang, E., Li, B., He, R., et al. (2015). Removal of nitrogen by heterotrophic nitrification–aerobic denitrification of a phosphate accumulating bacterium Pseudomonas stutzeri YG-24. Bioresour. Technol. 182, 18–25. doi: 10.1016/j.biortech.2015.01.100
Li, W. W., Yu, H. Q., and He, Z. (2014). Towards sustainable wastewater treatment by using microbial fuel cells-centered technologies. Energy Environ. Sci. 7, 911–924. doi: 10.1039/c3ee43106a
Nemec, A., Musílek, M., Maixnerová, M., De Baere, T., van der Reijden, T. J., Vaneechoutte, M., et al. (2009). Acinetobacter beijerinckii sp. nov. and Acinetobacter gyllenbergii sp. nov., haemolytic organisms isolated from humans. Int. J. Syst. Evol. Microbiol. 59, 118–124. doi: 10.1099/ijs.0.001230-0
Papen, H., and Von Berg, R. (1998). A most probable number method (MPN) for the estimation of cell numbers of heterotrophic nitrifying bacteria in soil. Plant Soil 199, 123–130. doi: 10.1023/A:1004243810473
Poli, A., Romano, I., Caliendo, G., Nicolaus, G., Orlando, P., Falco, A. D., et al. (2006). Geobacillus toebii subsp. decanicus subsp. nov., a hydrocarbon-degrading, heavy metal resistant bacterium from hot compost. J. Gen. Appl. Microbiol. 52, 223–234. doi: 10.2323/jgam.52.223
Robertson, L. A., and Kuenen, J. G. (1983). Thiosphaera pantotropha gen. nov. sp. nov. a facultatively anaerobic facultatively autotrophic sulphur bacterium. J. Gen. Microbiol. 129, 2847–2855. doi: 10.1099/00221287-129-9-2847
Robertson, L. A., and Kuenen, J. G. (1984). Aerobic denitrification: a controversy revived. Arch. Microbiol. 139, 351–354. doi: 10.1007/BF00408378
Robertson, L. A., van Niel, E. W. J., Torremans, R. A. M., and Kuenen, J. G. (1988). Simultaneous nitrification and denitrification in aerobic chemostat cultures of Thiosphaera pantotropha. Appl. Environ. Microbiol. 54, 2812–2818.
Roh, S. W., Nam, Y. D., Chang, H. W., Kim, K. H., Kim, M. S., Oh, H. M., et al. (2009). Alishewanella aestuarii sp. nov., isolated from tidal flat sediment, and emended description of the genus Alishewanella. Int. J. Syst. Evol. Microbiol. 59(Pt 2), 421–424. doi: 10.1099/ijs.0.65643-0
Segers, P., Vancanneyt, M., Pot, B., Torck, U., Hoste, B., Dewettinck, D., et al. (1994). Classification of Pseudomonas diminuta Leifson and Hugh 1954 and Pseudomonas vesicularis Büsing, Döll, and Freytag 1953 in Brevundimonas gen. nov. as Brevundimonas diminuta comb. nov. and Brevundimonas vesicularis comb. nov., respectively. Int. J. Syst. Bacteriol. 44, 499–510. doi: 10.1099/00207713-44-3-499
Takaya, N., Catalan-Sakairi, M. A. B., Sakaguchi, Y., Kato, I., Zhou, Z., and Shoun, H. (2003). Aerobic denitrification bacteria that produce low levels of nitrous oxide. Appl. Environ. Microbiol. 69, 3152–3157. doi: 10.1128/AEM.69.6.3152-3157.2003
Virdis, B., Rabaey, K., Rozendal, R. A., Yuan, Z., and Keller, J. (2010). Simultaneous nitrification, denitrification and carbon removal in microbial fuel cells. Water Res. 44, 2970–2980. doi: 10.1016/j.watres.2010.02.022
Willson, L. P., and Bouwer, E. J. (1997). Biodegradation of aromatic compounds under mixed oxygen /denitrifying conditions: a review. J. Ind. Microbiol. Biotechnol. 18, 116–130. doi: 10.1038/sj.jim.2900288
Wrighton, K. C., Virdis, B., Clauwaert, P., Read, S. T., Daly, R. A., Boon, N., et al. (2010). Bacterial community structure corresponds to performance during cathodic nitrate reduction. ISME J. 4, 1443–1455. doi: 10.1038/ismej.2010.66
Xie, C. H., and Yokota, A. (2005). Reclassification of Alcaligenes latus strains IAM 12599T and IAM 12664 and Pseudomonas saccharophila as Azohydromonas lata gen. nov., comb. nov., Azohydromonas australica sp. nov. and Pelomonas saccharophila gen. nov., comb. nov., respectively. Int. J. Syst. Evol. Microbiol. 55, 2419–2425. doi: 10.1099/ijs.0.63733-0
Xing, D., Cheng, S., Logan, B. E., and Regan, J. M. (2010). Isolation of the exoelectrogenic denitrifying bacterium Comamonas denitrificans based on dilution to extinction. Appl. Microbiol. Biotechnol. 85, 1575–1587. doi: 10.1007/s00253-009-2240-0
Yamada, T., Sekiguchi, Y., Hanada, S., Imachi, H., Ohashi, A., Harada, H., et al. (2006). Anaerolinea thermolimosa sp. nov., Levilinea saccharolytica gen. nov., sp. nov. and Leptolinea tardivitalis gen. nov., sp. nov., novel filamentous anaerobes, and description of the new classes Anaerolineae classis nov. and Caldilineae classis nov. in the bacterial phylum Chloroflex. Int. J. Syst. Evol. Microbiol. 56, 1331–1340. doi: 10.1099/ijs.0.64169-0
Zhang, Y., and Angelidaki, I. (2012). Bioelectrode-based approach for enhancing nitrate and nitrite removal and electricity generation from eutrophic lakes. Water Res. 46, 6445–6453. doi: 10.1016/j.watres.2012.09.022
Zhang, Y., and Angelidaki, I. (2013). A new method for in situ nitrate removal from groundwater using submerged microbial desalination denitrification cell (SMDDC). Water Res. 47, 1827–1836. doi: 10.1016/j.watres.2013.01.005
Keywords: aerobic denitrifying bacteria, dissolved oxygen, microbial fuel cell, predominant species, simultaneous nitrification and denitrification
Citation: Zhao J, Wu J, Li X, Wang S, Hu B and Ding X (2017) The Denitrification Characteristics and Microbial Community in the Cathode of an MFC with Aerobic Denitrification at High Temperatures. Front. Microbiol. 8:9. doi: 10.3389/fmicb.2017.00009
Received: 09 September 2016; Accepted: 03 January 2017;
Published: 19 January 2017.
Edited by:
Yong Xiao, Institute of Urban Environment (CAS), ChinaReviewed by:
Xianhua Liu, Tianjin University, ChinaCopyright © 2017 Zhao, Wu, Li, Wang, Hu and Ding. This is an open-access article distributed under the terms of the Creative Commons Attribution License (CC BY). The use, distribution or reproduction in other forums is permitted, provided the original author(s) or licensor are credited and that the original publication in this journal is cited, in accordance with accepted academic practice. No use, distribution or reproduction is permitted which does not comply with these terms.
*Correspondence: Jianqiang Zhao, NjI2NzEwMjg3QHFxLmNvbQ==
Disclaimer: All claims expressed in this article are solely those of the authors and do not necessarily represent those of their affiliated organizations, or those of the publisher, the editors and the reviewers. Any product that may be evaluated in this article or claim that may be made by its manufacturer is not guaranteed or endorsed by the publisher.
Research integrity at Frontiers
Learn more about the work of our research integrity team to safeguard the quality of each article we publish.