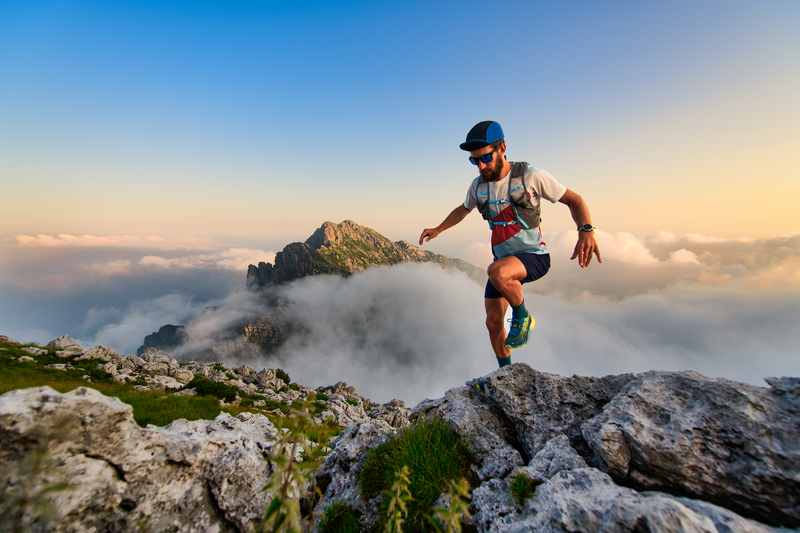
95% of researchers rate our articles as excellent or good
Learn more about the work of our research integrity team to safeguard the quality of each article we publish.
Find out more
ORIGINAL RESEARCH article
Front. Microbiol. , 28 November 2016
Sec. Evolutionary and Genomic Microbiology
Volume 7 - 2016 | https://doi.org/10.3389/fmicb.2016.01901
Non-homologous end-joining (NHEJ) is a double strand break (DSB) repair pathway which does not require any homologous template and can ligate two DNA ends together. The basic bacterial NHEJ machinery involves two partners: the Ku protein, a DNA end binding protein for DSB recognition and the multifunctional LigD protein composed a ligase, a nuclease and a polymerase domain, for end processing and ligation of the broken ends. In silico analyses performed in the 38 sequenced genomes of Streptomyces species revealed the existence of a large panel of NHEJ-like genes. Indeed, ku genes or ligD domain homologues are scattered throughout the genome in multiple copies and can be distinguished in two categories: the “core” NHEJ gene set constituted of conserved loci and the “variable” NHEJ gene set constituted of NHEJ-like genes present in only a part of the species. In Streptomyces ambofaciens ATCC23877, not only the deletion of “core” genes but also that of “variable” genes led to an increased sensitivity to DNA damage induced by electron beam irradiation. Multiple mutants of ku, ligase or polymerase encoding genes showed an aggravated phenotype compared to single mutants. Biochemical assays revealed the ability of Ku-like proteins to protect and to stimulate ligation of DNA ends. RT-qPCR and GFP fusion experiments suggested that ku-like genes show a growth phase dependent expression profile consistent with their involvement in DNA repair during spores formation and/or germination.
Double strand breaks (DSB) are the most detrimental genomic lesions and their repair constitutes a major challenge for cell viability. In eukaryotes and prokaryotes, two main repair machineries have evolved to repair these DSBs, namely homologous recombination (HR) and non-homologous end joining (NHEJ) (Alonso et al., 2013; Deriano and Roth, 2013; Glickman, 2014). In contrast to HR which needs an intact template to ensure repair of the break, NHEJ can bind DNA ends together without any template after a facultative DNA ends remodeling step. In multicellular eukaryotes, the NHEJ mechanism represents the predominant DSB repair pathway and requires the intervention of the end tethering heterodimer Ku70/80 as well as diverse end processing proteins (for review: Deriano and Roth, 2013). In prokaryotes, NHEJ is not ubiquitous and the minimal NHEJ machinery first described in Mycobacterium consists of two actors: a Ku homolog and an ATP dependent ligase LigD (Weller et al., 2002; Della et al., 2004; Gong et al., 2005). The latter one is a multifunctional protein that carries three distinct enzymatic domains conferring nuclease, polymerase and ligase activities (Pitcher et al., 2005). Unlike DSB repair through HR, DSB repair carried out by NHEJ is mutagenic. Nucleotide addition and/or deletion are scars frequently left after DNA ends ligation (Stephanou et al., 2007; Aniukwu et al., 2008; Gupta et al., 2011). The initial model involving two unique actors is now renewed by the discovery of alternative actors. When the ligase domain of LigD (LigDom) is defective, a back-up ligation activity is provided by LigC, another ATP dependent ligase (Della et al., 2004; Bhattarai et al., 2014). In the same way, Mycobacterium smegmatis possesses two additional polymerases which share the same in vitro activity than the polymerase domain of LigD (PolDom) (Zhu et al., 2012).
Streptomyces are filamentous bacteria mainly found in soils, sediments and seawater as well as symbionts with plants, fungi and animals (Seipke et al., 2012). Soil is a complex and changing environment where Streptomyces have to adapt to the heterogeneousness of the niche (moisture, nutrients, temperature…) and to cope with biotic competition. Their rich and varied secondary metabolism such as signaling molecules or chemical weapons is assumed to mediate their relationships with other organisms (Davies and Davies, 2010; Abrudan et al., 2015). Under limited nutritive conditions, their hyphae differentiate into long chains of exospores providing an efficient way for resistance and dissemination until suitable conditions for another growth cycle are met (Chater, 1993). While hyphae are large multi-genomic compartments, spores are known to include a single genome (Hopwood, 2006). In contrast to the vast majority of bacteria, Streptomyces possess a linear chromosome whose size is ranging from 5.96 to 11.94 Mb1 with a central replication origin and terminally inverted repeats that are covalently bound to specific telomere proteins (Lin et al., 1993). The Streptomyces genome presents a high plasticity and their chromosome is highly compartmented, with a conserved central region including mostly housekeeping genes whereas the dispensable genes are predominantly located in the chromosomal arms (Bentley et al., 2002; Ikeda et al., 2003; Kim et al., 2015). The loss of synteny at the ends of the chromosome results from horizontally acquired DNA insertion or deletion events (Choulet et al., 2006; Thibessard and Leblond, 2014). This terminal variability reveals the strong recombination activity taking place at the ends of the genome which is reminiscent of the DNA rearrangements reported several decades ago (Leblond et al., 1990; Volff and Altenbuchner, 1998). Hence, Streptomyces are also subject to a high level of genetic instability which is directly linked to major genome rearrangements. Characterization of some of these rearrangements revealed large deletions, chromosome circularization, (Fischer et al., 1997; Inoue et al., 2003), arm replacement (Fischer et al., 1998; Uchida et al., 2003), chromosome end-to-end fusion (Wenner et al., 2003) or large DNA amplifications (Yanai et al., 2006). Molecular analyses showed that chromosome circularization and end-to-end fusion events especially rely on illegitimate recombination whereas chromosome arm replacement or DNA amplifications is dependent on HR. This terminal recombination pattern may result from higher recombination frequencies triggered by the preferential formation of DSBs in these regions. On the other hand, genome rearrangements could also be more tolerated if formed in these dispensable regions.
Nevertheless, little is known about DSB repair in Streptomyces. Like the other bacteria, Streptomyces possess a RecA homologue involved in HR (Aigle et al., 1997; Muth et al., 1997; Vierling et al., 2001; Huang and Chen, 2006). However, recent studies that we performed suggest that Streptomyces has an atypical HR pathway. In fact, in contrast to bacterial models like Escherichia coli, Bacillus subtilis or Mycobacterium, deletion of genes encoding a potential helicase-nuclease complex supposed to be involved in the initial end resection step during HR is not viable in S. ambofaciens (Zhang et al., 2014). On the other hand, deletion of ruvABC and recG, two loci encoding factors that have a redundant role in migration and resolution of Holliday junction during late steps of HR, has only a mild effect on recombination efficiency (Hoff et al., 2016). Early bioinformatics studies showed that the two model species, S. coelicolor and S. avermitilis, both possess a ku ortholog suggesting also the existence of a NHEJ pathway in Streptomyces (Rocha et al., 2005). Here, we performed in silico analyses in the fully sequenced Streptomyces genomes leading to the identification of a large panel of conserved and variable genes suspected to be involved in a NHEJ pathway. Construction of single and multiple NHEJ-like genes mutant strains in S. ambofaciens allowed us to study the impact of these different genes in DNA damage repair. All the results we obtained suggest the existence of a complex and atypical bacterial NHEJ pathway in Streptomyces.
A total of 38 sequenced Streptomyces genomes, including that of S. ambofaciens ATCC23877 was scanned to identify genes putatively encoding NHEJ Ku-like or LigD-like proteins based on BLAST similarity search against the non-redundant database. A preliminary BLASTP search (e-value cut-off 10-30; identity threshold 30%) using M. tuberculosis Rv0937c and Rv0938 (Ku and LigD respectively) protein sequences as a query allow to identify and numerate the candidates. Secondly, BLASTP were performed using S. ambofaciens ATCC23877 NHEJ-like genes and reciprocal best hits allowed to organize them into groups of orthologs.
For the phylogeny of Ku-like proteins, the tree was built using a maximum-likelihood method based on a GTR + G + I model. Phylogenetic trees were built and edited with Mega6 (Tamura et al., 2013). Support of the tree branches was estimated with 100 bootstrap replicates and all positions with <80% site coverage were eliminated. A total of 295 and 309 positions were respectively used for the Ku-like and the LigC/LigD protein phylogenetic trees.
All strains used in this study are listed in Table 1. The DH5α E. coli strain is used as conservation host for BACs and plasmids. For biochemical analyses, plasmid constructions were done in E.coli MC1061 (Casadaban and Cohen, 1980). ER2566 (NEB) was used for kuB gene expression and Rosetta (DE3) PLysS (Stratagene) for kuA and kuC gene expression. E.coli strains were grown in Luria-Bertani (LB) medium at 37°C except for the BW25113 pKD20 thermosensitive strain used for PCR targeting, which was grown at 30°C. S. ambofaciens strains were grown at 30°C on Soya flour mannitol (SFM) plates except for phenotypical analyses and for liquid cultures which were performed on solid Hickey Tresner (HT) medium. All Streptomyces mutants derive from our reference strain S. ambofaciens ATCC23877 (Pinnert-Sindico, 1954). Mutant strains were constructed by PCR targeting as described by Gust et al. (2003). To summarize, a recombinant BAC containing the locus of interest was transformed in the highly recombinogenic E. coli BW25113/pKD20 strain in order to replace the target gene by an oriT- aac(3)IV resistance disruption cassette (Raynal et al., 2006). The ET12567 non-methylating strain containing the mobilizing pUZ8002 plasmid was used as donor for intergeneric conjugation of the modified BAC between E. coli and S. ambofaciens Double-crossover (CO) events leading to the replacement of the target gene by the cassette were selected by the sensitivity to kanamycin (loss of the BAC vector part) along with the resistance to apramycin (insertion of the resistance cassette). The disruption cassette flanked by attL and attR sites was excised in the newly obtained mutant strains thanks to the introduction of pOSV508 a plasmid allowing the expression of int and xis genes from pSAM2 (Raynal et al., 2006). Strains expressing translational EGFP fusions were also constructed by in vivo recombination. The egfp gene with the transcription terminator from phage λ was cloned from plasmid pIJ8660 (Sun et al., 1999) into an oriT aac(3)IV disruption cassette (Raynal et al., 2006). This cassette was then used as the source to make kuA-egfp, kuB-egfp and kuC-egfp fusions. PCR was performed using egfp-oriT-aac(3)IV as the template with specific oligonucleotides which anneal to the linker sequence at the 5′ end of egfp and downstream of the 3′ end of aac(3)IV and introduce 40 bases of homology to the 3′ ends of kuA, kuB, or kuC, as well as removing the stop codons. PCR products were introduced into E. coli BW25113/pKD20 containing the cognate BACs with either kuA, kuB, or kuC, and the following steps of the procedure are described above. In these recombinant strains, the fusion genes (kuA-egfp, kuB-egfp or kuC-egfp, respectively) are the only copies of kuA, kuB, or kuC.
An amount of 109 spores of different Streptomyces strains was diluted in sterile water in 96 well microplates and exposed to 0, 0.1, 0.3, 0.5, 0.7, and 1 kGy of radiation doses. Processing was carried out in a Van de Graaf® electron accelerator (VIVIRAD, Handschuheim, France) in the technological resource center Aérial (Illkirch-Graffenstaden, France). Energy delivered by the electron accelerator reach 2.2 MeV with an intensity of 10 μA. Dose rate equals approximately 60 kGy/h. After irradiation, several spore dilutions were plated with the easySpiral® apparatus (Interscience, Saint-Nom-la-Bretèche, France) on SFM Petri dishes and the number of colonies was counted after 3 days incubation at 30°C. For each genetic context, at least two independent mutant strains were exposed in two independent experiences.
Strains for microscopic observations were inoculated in the acute-angle junction of standard-sized microscope coverslips inserted with an angle of 45° in SFM agar, and grown for 48 h. The coverslips were then carefully pulled from the medium and directly mounted in a drop of sterile water on a microscope slide. Fluorescent microscopy observations were carried out using a Axiovert 200M MOT device (Carl Zeiss Micro imaging) equipped with a mercury vapor lamp and a FITC filter set (470/40 excitation, 540/50 emission). Both contrast phase and fluorescent observations were made with an X100 objective.
To verify the mutant strains, Streptomyces genomic DNA was extracted by low binding sulfate salt method as described by Kieser et al. (2000). Liquid HT medium was used for growth of mycelium before DNA extraction. For biochemical analyses, the gene coding for KuA was amplified from the BAC 16ZB5. KuB and KuC genes were amplified from the BAC 27ZF1. Primers used for amplification were designed to flank the open reading frames with the coding sequence of a His6-tag followed by a NdeI site on their 5′ side, and by a XhoI site on their 3′ side. The resulting ORFs were cloned under the T7 promoter of the pJ411 vector (DNA2.0) giving the plasmids pSMG258, 259, 260 allowing expression of the proteins 6His-KuA, 6His-KuB, 6His-KuC, respectively (named hereafter KuA, KuB, and KuC). Sequences of the primers used in this study are available upon request.
For RNA extraction from cultures on solid medium, streaks were harvested after growth (from 24 to 60 h depending of the experiment) at 30°C on HT solid medium covered with cellophanes membranes. For RNA extraction after a genotoxic stress, pre-germinated spores of Streptomyces were grown until 0.2 OD600 was reached and exposed to mitomycin C (MMC, 1.5 μg.mL-1, i.e., CMI/4) for 30 min. RNA isolation was performed using the Aurum Total RNA mini-kit (Bio-Rad) according to the manufacturer’s instructions. To optimize cell lysis, an additional sonication step (3 × 30 s) using a Bioruptor apparatus was added. The RNA quality was assessed after migration on 1% agarose gel. In order to eliminate genomic DNA contamination, the RNA samples were treated before reverse transcription with DnaseI (Ambion) in the presence of 10 U of RNase inhibitor per μg of RNA. DnaseI was then inactivated at 75°C during 10 min in the presence of EDTA. Reverse transcription was performed on 2 μg RNA with an iScript Advanced cDNA synthesis kit (Bio-Rad) according to the manufacturer’s instructions. The absence of contamination with genomic DNA was validated by a 30 cycle-PCR on a control aliquot removed before reverse transcription using hrdB-F and hrdB-R primers. Quantitative PCR was undertaken in a CFX96 (Bio-Rad) apparatus in a 10 μL reaction mixture containing respectively 5 μL of SYBR supermix (Bio-Rad), 0.2 μM of each primer and 4 μL of a 10-fold dilution of cDNA. The reaction conditions were as follow: a 3 min initial denaturation step at 95°C and 40 cycles of 10 s at 95°C and 30 s at 60°C. In order to check the absence of secondary products, melting curves were realized from 65 to 95°C with an increase rate of 0.5°C/5 s. The transcription level of interest genes was analyzed using the mathematical model proposed by Pfaffl (2001). The hrdB gene, encoding the principal sigma factor in Streptomyces, was used as the reference gene for the normalization of the expression level as it was the case in former studies (Galet et al., 2015; Martínez-Burgo et al., 2015).
Escherichia coli Rosetta PlysS cells transformed with pSMG258 were grown at 30°C in 2 l of LB medium supplemented with 25 mg/mL thymine, 30 μg/mL kanamycin and 25 μg/mL chloramphenicol to OD600 = 0.7. Production of protein was induced with 0.5 mM IPTG for 15 h at 20°C. Cells were harvested by centrifugation and the pellet was resuspended in 40 mL lysis buffer A (50 mM Tris-HCl pH 8, 0.25 M NaCl). Cells were broken by a freeze/thaw step in liquid N2/37°C and then centrifuged 1 h at 100 000 g at 4°C. The pellet was resuspended with 40 mL of a 20 mM Tris pH 7.5, 2.5 mM MgCl2, 0.5 mM CaCl2, 1 mg/mL DNaseI buffer. After 1 h at 20°C, the mixture was centrifuged 20 min at 100 000 g at 4°C. All subsequent steps were carried at 4°C. The pellet was washed with 40 ml buffer P1 (50 mM Tris-HCl, pH 8, 1 M NaCl), and then solubilized with 40 mL of buffer P2 (50 mM Tris pH 8, 8 M urea). After 1 h the mixture was centrifuged 20 min at 100 000 g and 3 mL of Ni2+ affinity column (Ni-NTA agarose, Qiagen) was added to the supernatant which was transferred onto an Econo-Column (Biorad). The flow through was discarded and the resin was washed with series of 25 mL of buffer P3 (50 mM Tris pH 8, 1% sucrose, 0.5 M NaCl) with urea concentration decreasing from 8 M to 0 M by steps of 0.5 M. The Ni-NTA column was washed with buffer P3, then with buffer W (50 mM Tris pH 8.0, 0.5 M NaCl) supplemented with 20 mM imidazole. KuA protein was eluted in buffer W supplemented with 250 mM imidazole and then dialysed against buffer D (50 mM Tris-HCl pH 8, 0.4 M NaCl, 50% glycerol, 1 mM DTT) prior to storage at -20°C.
The expression of KuC gene was induced in E. coli Rosetta PLysS cells transformed with pSMG260 as described for KuA. Cells were harvested by centrifugation and the pellet was resuspended in 40 mL lysis buffer B (50 mM Tris-HCl pH 8, 0.25 M NaCl, 0.2 mg/mL lysozyme) supplemented with Complete Antiprotease (Roche) and incubated 1 h at 20°C. The mixture was then centrifuged and the pellet was treated with DnaseI, as described for KuA purification. Purification procedure of KuC at this step is the same as the one described for KuA.
ER2566 cells transformed with pSMG259 were grown at 30°C in 2 l of LB medium supplemented with 25 mg/mL thymine and 30 μg/mL kanamycin and kuB gene expression was induced as for kuA and kuC genes. Cells were harvested by centrifugation, the pellet was resuspended in 40 mL of lysis buffer B and incubated 1 h at 20°C. All subsequent steps were carried at 4°C. Cell lysis mixture was supplemented with 0.5 M NaCl, 0.1% Triton and 0.1% Non-idet P40 and incubated 1 h. Two mL of polyethyleneimine (PEI) 10%, pH 8 was added to precipitate nucleic acids; the mixture was stirred for 1 h and then centrifuged 1 h at 100 000 g. The soluble phase was next precipitated by raising the concentration of ammonium sulfate (AS) firstly to 50% of saturation, KuB was mainly found in the supernatant at this step, and secondly to 80% of saturation to precipitate KuB. The corresponding pellet was resuspended in 25 mL of buffer P1 and 3 mL of Ni-NTA agarose, pre-equilibrated in buffer P1, were added. The Ni-NTA agarose was washed with buffer P1, then with buffer W supplemented with 20 mM imidazole and KuB was eluted in buffer W supplemented with 250 mM imidazole. The eluted fraction was dialyzed against buffer D and stored at -20°C.
Final purification step analyzed by SDS PAGE showed that purified KuA, KuB and KuC were obtained at 90% purity and were a mix of full length and truncated forms (Supplementary Figure S1). Their concentration was determined by UV absorption at λ = 280 nm on a NanoDrop 1000 Spectrophotometer (Thermo Scientific).
LigD from Bacillus subtilis fused in C-terminal to a His6 tag was purified as previously described McGovern et al. (2016).
Fifty nanograms (∼75 fmol) of a 1001 bp linear DNA substrate with 5′-phosphorylated blunt-ends were preincubated with raising concentrations of KuA, KuB, or KuC in 19 μL of buffer T5 (50 mM Tris-HCl pH 8, 1 mM DTT, 5 mM MgCl2, 40 mM NaCl and 5% glycerol) for 30 min at 37°C. One μL of T5 exonuclease (0.5 U/μL, Biolabs) was added to the reaction mixture and reactions were incubated for 30 min at 37°C. Reactions were stopped by adding 4 μL of stop buffer (5 mg/mL proteinase K, 2% SDS and 0.1 M EDTA) and incubated at 37°C for 30 min. Four microliter of DNA loading buffer (33% glycerol and 0.25% xylene cyanol) were added, and the reactions were loaded on a 0.7% agarose gel in TBE Buffer. After electrophoresis, DNA was stained by the SYBR Gold reagent (Invitrogen) and the gel was photographed using a Chemidoc apparatus (Bio-Rad).
In a final reaction volume of 20 μL in the T4 DNA ligase ligation buffer (NEB, 50 mM Tris-HCl pH 7.5, 10 mM MgCl2, 10 mM DTT, 1 mM ATP), 50 nanograms (∼75 fmol) of the 1001 bp linear DNA substrate were preincubated with 0.25 or 1 μM of KuA, KuB, or KuC for 30 min at 30°C. Then, raising concentrations of LigDBsub were added to reactions. Ligation reactions were stopped after 2 h at 37°C by adding 4 μL of stop buffer and analyzed as described above.
Genes homologous to the bacterial NHEJ factors ku and ligD were sought in the genome of our model strain Streptomyces ambofaciens ATCC23877 (Thibessard et al., 2015). To do so, the M. tuberculosis Rv0937c and Rv0938 (KuMtub and LigDMtub respectively) protein sequences were used as query in a BLASTP search. The first observation was that the LigDMtub protein had no full length homologue in S. ambofaciens, but that each candidate protein matched only with one single domain at a time. Therefore, the 759 amino acid sequence of LigDMtub was split into 3 slightly overlapping parts (1–300, 271–459, and 451–759) corresponding to the polymerase (PolDom), the nuclease (NucDom) and the ligase (LigDom) domains, respectively. In this way, 3 Ku-like encoding genes (sharing 36, 39, and 40% of protein identity with KuMtub), 3 LigDom-like encoding genes (sharing 30, 32, and 41% of protein identity with the LigDom of LigDMtub) and 3 PolDom-like encoding genes (sharing 29, 34, and 41% of protein identity with the PolDom of LigDMtub) were identified in S. ambofaciens ATCC23877 genome (Figure 1). No NucDom-like encoding gene was detected. The 3 ku-like genes (SAM23877_5082, SAM23877_6929 and SAM23877_6942) were named kuA, kuB, and kuC respectively. Two of the 3 LigDom-like genes (SAM23877_6361 and SAM23877_0862) were named ligC and ligD, respectively. Indeed, SAM23877_6361 appeared to share more amino-acid identity to M. tuberculosis LigC (LigCMtub), i.e., 60% than to the LigDom of LigDMtub i.e., 30% and was therefore considered as LigCMtub ortholog. Similarly, SAM23877_0862 appeared to be the closest relative of the LigDom of LigDMtub. The third ATP-dependent DNA ligase encoding gene detected (SAM23877_1283) would encode a LigB protein known to exhibit a strong nick sealing activity in vitro and whose defect would not alter mycobacterial NHEJ (Gong et al., 2004). Therefore, this third ligase was not included in our further investigations. The 3 PolDom-like genes (SAM23877_5081, SAM23877_6202, and SAM23877_6362) were named polK, polO and polR, respectively. It was noticeable that 2 of the 3 PolDom like genes (polK and polR) colocalized with kuA and ligC.
FIGURE 1. Organization of the NHEJ-like genes in several Streptomyces genomes. The two conserved loci kuA-polK and ligC-polR are framed in dotted lines.
KuA, KuB, and KuC proteins are composed of 365, 383, and 302 amino-acids, respectively. All these proteins harbor the conserved Ku core domain at their N-terminal side, the conserved minimal C-ter domain (red in Supplementary Figure S2), that has been shown to be required for the interaction with LigD in B. subtilis (McGovern et al., 2016), and a basic extended C-terminal domain, which is of 114, 128, and 47 aa, respectively. The pIs of these extended domains (11.64, 10.97, and 10.65) are consistent with those described for the majority of bacterial Ku proteins. This suggests that their roles in DNA binding as well as in the modulation of the ability to thread inward the DNA molecule are conserved in Streptomyces (Kushwaha and Grove, 2013; McGovern et al., 2016). Interestingly, a SAP domain, previously predicted in the S. coelicolor KuB homologue (SCO0601) by Aravind and Koonin (2001) is also detected in S. ambofaciens KuB (at position 337–370, Supplementary Figure S2). This SAP domain is assumed to be also involved in nucleic acid binding (Aravind et al., 2000) and could modify the DNA binding abilities of KuB compare to KuA and KuC. Further experiments are required to highlight the roles and the differences of the extended C-terminal domains of these three Ku proteins.
Once this list of genes potentially involved in a Streptomyces NHEJ pathway established, the question of their conservation in the Streptomyces genus was addressed by seeking their homologues in 37 other Streptomyces complete genome sequences available at http://www.ncbi.nlm.nih.gov/genome. Therefore, S. ambofaciens KuA, KuB, KuC, LigC, LigD, PolK, PolO and PolR were used as query in BLASTP searches. A wide variety of situations were encountered and a few of them are illustrated in Figure 1. The homologues identified in 38 Streptomyces genomes are listed by their locus tag in Supplementary Table S1 and their presence or absence is summarized in Figure 2. The number and position of NHEJ-like genes varied from one species to another. However, two loci are widely shared among Streptomyces: ligC-polR which is present in all the investigated genomes and kuA-polK which is present in all the tested strains but Streptomyces vietnamensis.
FIGURE 2. Distribution of NHEJ-like genes in 38 complete genomes of Streptomyces. The presence of a homolog is mentioned by a black box and the absence of a homolog by an empty box. The number inside the boxes indicates when several homologs were identified; ∗ plasmidic; ∗∗ closed to kuC; ∗∗∗ partial (cover of 35%).
Focusing on the main function expected from a functional NHEJ system, we analyzed further the Ku-like and LigDom-like encoding genes and extended our interest to the PolDom-like genes colocalizing with them.
All but S. vietnamensis possess at least one kuA gene. In contrast, kuB was detected in only 8 out of the 38 analyzed genomes. Regarding kuC, the copy number varies from 0 to 2. Indeed, in the case of S. coelicolor, two identical copies of kuC are included in the terminal inverted repeats of the SCP1 linear plasmid. A few Streptomyces strains carry several copies of kuA genes leading us to make a distinction according to their vicinity of polK: the neighbors of polK were called kuA primary genes while standalone kuA genes were called secondary kuA genes. All the ku-like genes (43 kuA, 8 kuB and 17 kuC) were aligned and submitted to a Maximum Likelihood phylogenetic analysis. The tree topology (Figure 3), together with the fact that the primary kuA gene is almost ubiquitous, suggested that KuA was probably present in the Streptomyces common ancestor and evolved along with Streptomyces. The secondary kuA gene carried by Streptomyces sp. 769, S. albulus ZPM, S. albulus NK660, and S. lydicus shares an average nucleotidic identity ranging from 67 to 72% with their respective primary copy. For these species, the primary and secondary KuA phylogenies are congruent, suggesting that their common ancestor gained a second kuA copy either by gene duplication or by gene transfer. Regarding the kuC gene, it was present in 17 out of 38 analyzed genomes, 7 of which were plasmid-borne. It was closely related to KuA and formed a sister group of KuA within a large KuA-KuC monophyletic group that excluded KuB. In fact kuB genes formed a strong monophyletic group and appeared much more scattered in Streptomyces genera.
FIGURE 3. Phylogeny of Ku-like proteins identified in 38 Streptomyces strains. Streptomyces Ku-like proteins were identified in 38 Streptomyces strains and compared with the closest homologues of M. tuberculosis, M. smegmatis, B. subtilis, B cereus, B halodurans, and P. aeruginosa. The tree was built using a maximum-likelihood method with a 295 aa alignment. Only bootstrap values higher than 70% are reported. Heavy lines indicate branches supported by bootstrap values >90% (100 replicates). The scale represents mutations per amino-acid. Primary KuA are in red, secondary KuA are in orange, KuB are in green and KuC in blue. The asterisks symbolized the proteins encoded by plasmid borne-genes. The locus-tag of each sequence is mentioned in brackets. The suspected event of gene duplication is depicted by the two braces. B, Bacillus; M, Mycobacterium; P, Pseudomonas; S, Streptomyces. NRRL 8057 = DSM 46488; C34 = DSM 42122 = NRRL B-24963; MA-4680 = NBRC 14893.
Regarding the distribution of LigC and LigD ligases, LigC encoding genes were found in all genomes and always in the close vicinity of polR as in M. tuberculosis (Figure 1). In contrast, LigD was detected in 29 out of the 38 cases (Figure 2). In 6 of them the encoding gene colocalized with kuC, and in S. cattleya, it colocalized with kuA-polK (see Figure 1). Phylogenetic analysis by Maximum Likelihood method (Supplementary Figure S3) showed that the LigC proteins branched together with M. tuberculosis LigC with a topology that seemed quite congruent with the species relations, suggesting a vertical inheritance of this conserved locus. LigD proteins also branched together and together with LigDom of M. tuberculosis LigD.
Altogether, two NHEJ-like gene categories were delineated: (i) those that are conserved among the Streptomyces and were considered as “core” putative NHEJ factors (i.e., kuA and the colocalized polK, ligC and the colocalized polR) and (ii) those that are sporadically represented among the Streptomyces and were considered as “variable” putative NHEJ factors (i.e., kuB, kuC and ligD).
After in silico identification of the “core” and “variable” NHEJ putative factors in Streptomyces, we constructed deleted mutant strains by replacing the chromosomal locus with an excisionable antibiotic resistance cassette (Raynal et al., 2006) by the use of PCR targeting (Gust et al., 2003). The whole selection procedure was performed several times simultaneously in order to select at least two independent mutant lineages for each genetic context. Table 1 summarizes the different genotypes constructed for this study. The mutations were checked by PCR amplification of the mutated locus. The maintenance of the chromosomal linearity of the mutants was checked by PCR amplification of the telomeres and of some loci scattered along the chromosome (not shown).
All the mutations, simple or multiple, were obtained with classical frequencies indicating that none of the targeted genes (“core” or “variable”) was essential. In addition, there was no significant difference between the wild-type reference strain and any of mutants in colonial morphology and sporulation under normal growth conditions (not shown).
Electronic beam irradiation (EBI) was shown to induce loss of viability of Bacillus spores by damaging spore coat, by altering membrane permeability or by DNA fragmentation (Fiester et al., 2012). High level energy beam such as those used in this work (2.2 MeV) is likely to trigger DSB as the most significant lesions in the spore embedded DNA. In order to test the involvement of the NHEJ-like factors to DNA damage response, spores of the wild-type S. ambofaciens reference strain as well as spores of at least two independent mutants for each genetic context were irradiated by EBI from 0.1 to 1 kGy and their survival was assessed after growth on SFM medium. The results are represented in Figure 4.
FIGURE 4. Electronic beam irradiation of S. ambofaciens strains deficient in NHEJ-like genes. Spores of mutants of NHEJ-like genes, namely single and multiple ku (A), ligase (B) and polymerase (C) mutants, were exposed to 0.1, 0.3, 0.5, 0.7, and 1 kGy of electronic beam irradiation. (D) In the same way, a Δku-like ΔrecA mutant also affected in HR was exposed to the same irradiation doses. In this latter case, a logarithmic scale was used for survival rate representation. Survival rates were calculated as the number of clones for a given dose relative to the number of clones unexposed to radiation. For each graph, wild-type strain is represented as a control. Each curve corresponds to data obtained from an individual mutant strain. Error bars indicate standard error of a minimum of three replicates.
Depending on the dose, deletion of kuA, which belongs to the “core” NHEJ-like gene set, conferred a 2–4 fold increase of the sensitivity compared to the wild-type (Figure 4A). Since kuA is present in the large majority of the sequenced genome, it appears as the major Ku actor of the putative Streptomyces NHEJ. Surprisingly, mutations of the variable ku-like genes, kuB and kuC, also conferred an increased sensitivity but at a lower level, with a maximal twofold cell viability decrease reached after 0.5 and 0.7 kGy exposition for both contexts (Figure 4A). In addition, the sensitivity was aggravated when kuB and kuC were both deleted in a ΔkuA background to generate a triple ΔkuABC mutant, until reaching a sevenfold cell viability diminution in comparison to the WT for the medium radiation doses (Figure 4A). This result suggests that the three Ku products participate to the response to DNA damaging agents in spores, each of them assuming their share of the DNA repair independently from the two others. Additionally, the triple ΔkuABC mutation assumed to abolish any putative NHEJ repair strongly increased the sensitivity of a HR deficient context (i.e., ΔrecA) (Figure 4D). This last result shows that RecA and Ku-like proteins are both important contributors to the S. ambofaciens spore resistance to EBI.
In Mycobacterium, LigD is the main NHEJ ligase whereas LigC only provides a back-up activity (Della et al., 2004). In contrast to Mycobacterium where a single ligC mutation does not impact survival rate to DNA damage, the defect of both the conserved ligC and the variable ligD of S. ambofaciens conferred a significant sensitivity to EBI. Surprisingly, the deletion of ligD conferred an even higher sensitivity than the mutation of ligC (Figure 4B). Furthermore, the ΔligCD double mutant showed an aggravated phenotype in the same proportions as a ΔkuABC triple mutant (Figure 4B). These data clearly show that the two ligase activities participate to DNA repair in response to EBI.
Single deletion of polK or polR, the two conserved homologues of Mycobacterium PolDom colocalized respectively with kuA and ligC led to a weak sensitivity to electronic beam exposure. For both single mutants, increase of sensitivity in comparison to the wild-type does not exceed 1.5 fold for each dose (Figure 4C). On the other hand, double polRK mutant strains are approximately twice more sensitive than the single mutants (Figure 4C). Both loci are therefore involved in DNA damage response.
In order to investigate the spatial and temporal expression of NHEJ-like genes during development, a time-course of RT-PCR experiments was performed and translational fusions with EGFP were observed by fluorescent microscopy. First of all, the results showed that NHEJ-like genes are weakly expressed throughout the development. For instance, the expression of ligC, ligD, polK and polR was too low to be monitored by qPCR whereas the expression of ku genes was 10 to 50 fold lower than hrdB expression (Figure 5A). Secondly, kuA expression was clearly growth-phase dependent with a 10 fold increase between 24 and 60 h after germination. It is important to indicate that sporulation began to be observable after 48 h of development in these growth conditions. There was no difference throughout the time-course for kuB and kuC expression. Both of them were less expressed than kuA at the late stages of development. The WT strain was also grown to OD600 of 0.2 in HT liquid medium and then exposed to MMC (1.5 μg⋅ml-1) for 30 min. RNAs were extracted and reverse-transcribed to assess by PCR the regulation of NHEJ-like genes during a genotoxic stress. No induction or repression of NHEJ-like genes was noticeable, while the positive induction control (recA) was up-regulated up to 20 fold (not shown).
FIGURE 5. Growth-dependent and cell-type specific expression of NHEJ-like genes. (A) Expression level of S. ambofaciens ATCC23877 ku genes relative to hrdB after 24, 36, 48, and 60 h growth on SFM plates at 30°C. Error bars represent standard error. Expression of kuA after 60 h growth is significantly higher than that at 24 h. Furthermore, kuA expression at 60 h growth is significantly different than that of kuB and kuC (t-test with Bonferroni correction, α = 0.05). (B) Strains expressing EGFP fusion with KuA, KuB, or KuC were inoculated in the acute-angle junction of standard-sized microscope coverslips inserted at 45° in SFM agar, and grown for 48 h before observations by fluorescent microscopy. The control strain does not contain any GFP gene. Representative examples of spores or aerial mycelium are shown as a contrast phase image (upper panels) and as EGFP fluorescent channel (lower panels). The weak fluorescent foci observed mainly in mycelia were readily detected in the control strain and are not likely to correspond to GFP signal. The scale is represented by a 5 μm size bar.
To observe the cellular localization of Ku proteins in physiological conditions, egfp translational fusions were constructed at kuA, kuB, and kuC chromosomal loci. Fusion strains were inoculated on SFM agar plates and grown for 48 h before observations by fluorescent microscopy along with a negative control strain without any egfp gene. A positive control strain constitutively expressing egfp gene exhibited a strong green fluorescence signal in all cells throughout the developmental stages of the bacteria, proving the reporter gene to be functional in S. ambofaciens (not shown). For KuA fusion, the green fluorescence signal was significant in spores and segmenting aerial hyphae but not in vegetative mycelium (Figure 5B), showing a specific accumulation of KuA in spores. This result corroborates the phase dependent expression pattern of kuA observed in the previous qPCR analysis. For KuB and KuC fusions, although the signals were weaker than for KuA fusion, they also revealed the accumulation of KuB and KuC in spore chains. Since no significant modulation of gene expression was detected for kuB and kuC along the growth cycle (Figure 5A), this protein accumulation pattern suggests a translational (and/or a post-translational) regulation level.
To assess which proteins are required in a putative S. ambofaciens NHEJ, we tried to purify all the proteins putatively implicated in this pathway. Among them, we succeeded to express and purified S. ambofaciens KuA, KuB and KuC proteins (39.1, 42.4, and 33.6 KDa respectively). To evaluate the oligomeric state of the three Ku proteins, we performed gel filtration experiments. KuA and KuB were eluted in fractions corresponding to a molecular weight of 160 and 100 kDa, respectively, strongly suggesting that KuA and KuB are multimeric (Supplementary Figure S1 and Supplementary Data Sheet S1). To date, characterized bacterial Ku are dimers. However, the elongated shape of the B. subtilis Ku (KuBsub) dimer (McGovern et al., 2016) led to the elution of this protein with apparent molecular weight corresponding to a tetramer. Then KuA and KuB could also be dimers displaying elongated shape, as for KuBsub. KuC was clearly purified in different oligomeric states ranging from 70 to more than 600 kDa. The main elution peak, corresponding to a protein of apparent molecular weight around 160 kDa, was similar to the one observed for KuA.
KuA, KuB, and KuC were able to protect a linear dsDNA molecule from the T5 exonuclease (Figure 6A), a property shared by the KuBsub protein (McGovern et al., 2016) and the Pseudomonas aeruginosa Ku against different exonucleases (Zhu and Shuman, 2010). This result suggests that these proteins are able to bind DNA ends. Another role of Ku in the bacterial NHEJ is to recruit the LigD at DNA ends and to stimulate its ligase activity. Remarkably, KuA, KuB, and KuC were able to stimulate the ligation activity of the B. subtilis LigD protein (Figure 6B), even if they were less efficient than KuBsub. However, we observed a different concentration-dependent stimulation of the ligase by the S. ambofaciens Ku proteins. Raising the concentration of KuA and KuC from 0.25 to 1 μM in the ligation assay seemed to slightly increase ligation efficiency. At the opposite, the stimulation activity observed with 0.25 μM of KuB appeared to be reduced when its concentration was raised to 1 μM. A similar inhibitory effect was observed with KuBsub.
FIGURE 6. KuA, KuB, and KuC protect linear dsDNA from the T5 exonuclease and stimulate the ligase activity of LigDBsub. (A) A 1001 bp linear dsDNA with 5′-phosphorylated ends (3.8 nM) was incubated 30 min at 37°C with increasing amounts of KuA, KuB, or KuC as indicated. T5 exonuclease (0.5 U) was then added in each reaction mixtures for 30 min at 37°C and digested products were analyzed as described in the Section “Material and Methods.” Control experiments showing the 1001 ds DNA substrate and the nearly complete degradation of the DNA molecule by the T5 exonuclease without other proteins added are shown on the left. (B) A 1001 bp linear dsDNA with 5′-phosphorylated ends (3.8 nM) was incubated with 0.25 or 1 μM of KuA, KuB, or KuC as indicated. Increasing amounts of LigDBsub were then added (allowing final concentrations of LigDBsub indicated above the gels) and ligation products were analyzed as described in Section “Material and Methods.” Control experiments showing the weak activity of LigDBsub alone at different concentration are shown on the left (No Ku). For comparison, KuBsub was assessed for its LigDBsub stimulation activity in the same conditions (right part).
The presence of a NHEJ pathway in prokaryotes appears to be limited to some phyla representing about 20% of the species (estimated on a sample of 2645 complete bacterial genomes) (McGovern et al., 2016). The minimal set of NHEJ gene encodes a two partner system including the homodimeric Ku protein and a ligase polypeptide composed of the ligase enzymatic domain (LigDom) to a nuclease (NucDom) and a polymerase (PolDom) domain. The latter enzymatic activities process DNA ends before ligation. The NHEJ gene repertoire ranges from the simplest in P. aeruginosa with a single Ku and a ligase (ligase D) to the more complex with those described in Agrobacterium (Zhu and Shuman, 2007) or Sinorhizobium (Kobayashi et al., 2008) and Streptomyces as described in this work. Hence, while B. subtilis and Mycobacterium possess a single Ku, a single LigD and one or two LigC, A. tumefaciens possesses three Ku, two LigD and three LigC (Zhu and Shuman, 2007). Sinorhizobium meliloti and other rhizobia possess up to 4 Ku, 5 LigD and one LigC (Kobayashi et al., 2008); the ligase, nuclease and polymerase domains are present in different organizations, sometimes (i) fused together in a single polypeptide or (ii) encoded by distinct genes. This situation is similar in archaea where the putative ligase, nuclease and polymerase domains are encoded by distinct genes, however, kept into an operonic organization assumed to ensure the co-regulation of the activities (Bartlett et al., 2013). However, among actinobacteria, Streptomyces appears as an exception, since other main phyla (Nocardia, Frankia, Rhodococcus, and Corynebacterium) seem to possess a multidomain LigD homologue (not shown).
In Streptomyces, several levels of complexity are also noticeable. First, the activities putatively involved in NHEJ are encoded by standalone genes that are scattered along the genome. Consequently the repertoire is rather expanded: the number of genes ranging from none up to 4 for ku, from one to two LigDom encoding genes (ligC, ligD), from 2 to 3 PolDom encoding genes, from none to one NucDom encoding gene. The total number of NHEJ-like genes ranges from 4 in S. bingchenggensis and S. venezuelae to 8 in S. ambofaciens and S. reticuli: 3 ku (kuA, kuB, kuC), one ligD and one ligC, and 3 polymerase genes (polK, polR, polO). These genes can fall into two groups. On one hand, the “core” set includes kuA, ligC, polK and polR which are present in almost all Streptomyces species and organized in two loci of rather conserved organization (i.e., kuA-polK, ligC-polR). The only exception appears to be in S. vietnamiensis which lacks the couple kuA-polK and the other ku genes. On the other hand, the rest of the genes constitute the “variable” gene set. An interesting feature to highlight is a gene duplication event involving kuA or kuC. The duplicated copies are either located in the variable region of the genome or present within the terminal inverted repeats of the linear replicons. This phenomenon is interesting since duplicated copies can evolve through gene divergence to raise new function. This mechanism is rather rare in bacteria but gene duplication seems frequent in Streptomyces, notably with large terminal duplication probably resulting from DSB repair by a mechanism related to break-induced replication.
The large number of NHEJ-like genes in Streptomyces as well as their variability questions their implication in DNA repair mechanisms. It is tempting to imply the “core” NHEJ gene set in a classical NHEJ pathway with a Ku (i.e., KuA) and an ATP-dependent DNA ligase (i.e., LigC). In addition to being the conserved ku gene, kuA is the more expressed of the 3 ku-like genes in the late growth phases, its defect conferred the most marked sensitivity to EBI and finally, KuA is the most efficient of the 3 Ku-like proteins in stimulating LigDBsub activity in vitro.
It might be surprising that the NHEJ ligase could be LigC rather than LigD which is the canonical NHEJ ligase in other bacterial species. However, it should be reminded that in Streptomyces, LigD carries the only ligase domain, as does LigC. Both ligases are devoid of the PolDom domain known to be involved in its recruitment by Ku. Therefore LigD and LigC may ensure similar functional roles in Streptomyces. One could hypothesize that a gene rearrangement split the ligase domain from the two others (i.e., PolDom and NucDom) in a Streptomyces common ancestor. The two ligase genes would have then provided an equivalent contribution to the bacterium, finally resulting in the replacement of LigD by LigC in the “core” NHEJ set by a founder effect.
In S. ambofaciens, in which both ligC and ligD cohabits, single mutants displayed a sensitive phenotype to EBI and the double mutant displayed an aggravated phenotype. In this context, at least two hypotheses can be drawn. First, both LigC and LigD could participate to the same repair pathway; in that case, the sensitivity of single mutants and the aggravated phenotype of double mutants could be explained by a dose effect that is the need for a certain amount of ligases to cope for the induced DNA damage. In that case, both ligase genes would contribute to achieve the required level of ligase. Alternatively, they could participate to different repair pathways required to take over varied DNA damage (see below).
If the “core” NHEJ gene set indeed establishes a functional NHEJ pathway, the presence of two PolDoms in the “core” NHEJ gene set constitutes another intriguing feature, especially considering that they do not seem to have redundant functions. Hence, the sensitivity of both polK and polR simple mutants was aggravated in the double mutant. As shown in M. tuberculosis NHEJ, the role of the PolDom domain of LigD would be less to provide a polymerase activity than to recruit LigD to Ku (Pitcher et al., 2005). Then PolK and/or PolR may play a role of linker/adapter to recruit a ligase; the more likely being that PolK and PolR recruit LigC, however, on different types of DNA extremities (e.g., blunt, 3′ or 5′ overhanging ends). In the case of the presence of LigD (as in S. ambofaciens for example), the two PolK and PolR may recruit both LigC and LigD.
It is particularly noticeable that the “variable” genes (i.e., ligD but also kuB, kuC) were involved in the response to EBI. The exposure to EBI is assumed to trigger DSBs. However, different types of lesions can result from direct transmission of energy to the DNA structure and from secondary oxidative stress (e.g., oxidized bases). In consequence, damage induced by EBI requires NHEJ but may also require other DNA repair pathways. Thus, these “variable” genes would (i) support the NHEJ encoded by the “core” gene set in an opportunistic way, (ii) constitute an alternative NHEJ pathways or (iii) constitute a repair pathway dedicated to other DNA damage single strand gaps or abasic sites). The first hypothesis would imply a dose effect of the Ku as well as the ligase proteins to explain the aggravated phenotype of the multiple mutants (i.e., ΔkuABC and ΔligCD). In this first hypothesis, considering that prokaryote Ku act as dimers (McGovern et al., 2016), we can speculate that these three Ku proteins could act as homodimers as well as heterodimers. A data in favour of the second hypothesis (an alternative NHEJ pathway) is the colocalization of the kuC and ligD genes in 6 Streptomyces species clustering with S. griseus (Supplementary Table S1; Figure 3). However, no clear trend toward the co-occurrence of these two “variable” genes can be noticed in the 38 analysed genomes. In B. subtilis, the recent involvement of LigDBsub and KuBsub in base excision repair along with their major role in NHEJ supports the third hypothesis (de Ory et al., 2014, 2016).
In order to solve the question of the involvement of these genes in a NHEJ pathway, a forward-looking approach could be to specifically induce DSB formation at targeted chromosomal loci and assessed the sensitivity of the NHEJ-candidate genes to identify those truly involved in DSB repair per se.
The presence of a NHEJ pathway in bacteria is usually assigned to DSB repair in low rate growth phases characterizing bacterial species involved in pathogenic processes or exposed to harsh environmental living conditions. When bacteria replicate such as in laboratory conditions, sister chromosomes providing an intact template are preferentially used to ensure faithful DSB repair by HR. However, when replication is slowing down (i.e., stationary phases) or stopping (e.g., in resistance forms such as spores), DSB repair strongly relies on NHEJ. This is consistent with the fact that NHEJ genes are expressed preferentially when DSB repair cannot be ensured by a faithful process. Hence, in B. subtilis as well as in Streptomyces, the NHEJ genes were shown to be expressed in stationary phase and to accumulate in spores. The forespore-specific sigma factor σG in B. subtilis directly regulates ykoU (coding for LigD) and ykoV (coding for Ku), inducing their expression during the forespore development (Wang et al., 2006). In addition, B. subtilis Ku protein specifically localizes to the nucleoid of germinating spores while it disappears at later stages of germination. In Streptomyces, gene transcription analyses showed in this work an expression of the ku in stationary phase with an increase of kuA expression during sporulation. Moreover, GFP fusions showed a predominant localization of the Ku proteins in spores. Furthermore, mutations in ku and ligase genes confer sensitivity to genotoxic agents to B. subtilis as well as Streptomyces spores. Sensitivity to heat and desiccation which are biotic parameters known to trigger DSB is also associated to the NHEJ mutations in B. subtilis and Mycobacterium smegmatis (Moeller et al., 2007; Pitcher et al., 2007; Stephanou et al., 2007). The presence of multiple ku genes (and of other NHEJ-like genes) in Sinorhizobium meliloti is assumed to correspond to the need for DNA repair at different steps of the cell cycle. Although no induction of the NHEJ pathways was observed under genotoxic conditions (i.e., IR exposure), the expression patterns of the sku genes suggest that each Ku protein has a distinct role under different conditions (free bacterial form, bacteroids). In contrast, NHEJ would not be required during the establishment of symbiosis since a strain lacking all four sku genes was shown to establish functional nodules (Kobayashi et al., 2008). As in S. meliloti, it was shown that each of the S. ambofaciens ku genes is functional since their deletion conferred a phenotype of sensitivity toward genotoxic agents. Mutant strains lacking kuA, kuB, and kuC genes showed an aggravated phenotype suggesting that each participated to the response to DNA damage. We can therefore suppose that like in S. meliloti, intervention of the Streptomyces ku genes is done under specific conditions.
Recently in Pseudomonas putida, the NHEJ was shown to participate to another late growth phase phenomenon (Paris et al., 2015). Hence, bacteria frequently encountered nutrient starvation during late-growth phase. Populations starving display a phenomenon called stationary-phase mutagenesis (also known as adaptive mutations) from which emerge mutants able to overcome the shortage and initiate new vegetative cycles. The underlying mechanisms notably involve unfaithful DNA polymerases during HR events (Shee et al., 2012). Although the involvement of NHEJ in late mutagenesis remains unknown, deficiency of each of the NHEJ activities triggered an altered pattern of mutations (Paris et al., 2015). The authors speculate on ability to bypass DNA lesions of the LigD polymerase domain.
While the origin of the NHEJ “core” gene set is likely to result from vertical inheritance from a common Streptomyces ancestor, the origin of the “variable” set is questionable. The sporadic distribution of the “variable” genes may reflect either the existence of NHEJ in a bacterial common ancestor and its subsequent sporadic loss during genus/species separation. Reciprocally, it may reflect the acquisition of NHEJ genes through horizontal gene transfer in some phyla. Both hypotheses can also be combined and result in the observed complexity. The most explicit argument about their origin is their localization in the variable regions of the chromosome or in plasmids. While plasmids can be mobilized and even self-transmitted, the terminal regions of the Streptomyces chromosome is known to be targeted by recombination phenomena leading to insertions of acquired DNA and further losses (Choulet et al., 2006).
Whatever the evolutionary past of the NHEJ gene sets, the presence of a conserved “core” gene set enriched by additional functional “variable” genes may confer some advantage. In all bacteria studied so far, NHEJ is not essential (Ku/LigD) for growth, at least under laboratory conditions. Mutants of NHEJ in Mycobacterium, Pseudomonas, or Bacillus are not affected although they show sensitivity to environmental stress (Weller et al., 2002; Jacobs et al., 2003; Gong et al., 2004, 2005). We came to the same conclusion in S. ambofaciens although it was particularly interesting to note that a part of the “variable” genes (i.e., kuB, kuC and ligD) was involved in response to genotoxic agents. Hence the aggravated phenotype observed in double or multiple mutant strains (i.e., kuABC, ligCD) revealed the cooperative actions of the multiple alleles. The “variable” genes in Streptomyces may increase the flexibility and/or efficiency of the DSB repair mechanism and influence evolution of the genome. Lessons about the role of NHEJ in genome evolution can be drawn from organisms lacking NHEJ. Hence, the only eukaryote lacking NHEJ is the yeast Lachancea kluyveri (Gordon et al., 2011). Compared to other species of the Saccharomycetaceae family, this species showed a significantly low number of genomic rearrangements and the lack of telomere-to-telomere fusions. The authors suggest that the loss of the NHEJ and an alternative end joining pathway [named microhomology-mediated end joining (MMEJ) or alternative end joining (A-EJ)] is responsible for the low recombination rate assessed in this phylum. In this way, we can hypothesize that in Streptomyces the presence/absence of NHEJ genes in addition to the “core” NHEJ (the “variable” genes participating to a unique NHEJ or other alternative pathways) may modulate the frequency of DNA rearrangements and DNA acquisition through horizontal gene transfer and confer different evolution rates to Streptomyces species. These genes being themselves subject to transfer, the recombinant phenotype (i.e., hyperrecombinant) could be variable within a population and favor genetic diversification and adaptation to the environment. This situation could be reminiscent of mutator strains identified in E. coli (for review: Giraud et al., 2001) but applied to the capacity to recombine DNA including incoming genetic information.
A key question is the relative contribution of HR and NHEJ to the evolution and the remarkable compartmentalization of the linear Streptomyces chromosome. Is there any regulation between the two pathways during the development of the bacteria? In S. avermitilis, the deletion of the ku homologues (ku1 and ku2 corresponding to kuA and kuC respectively) was associated to an increased efficiency of HR (Zhang et al., 2012). This may reveal the existence of a balance resulting from the competition between HR and NHEJ repair mechanisms, and that both repair pathways co-exist at least during replicating phases. Is there any spatial regulation of the DSB repair mechanisms? DSB could be healed by different mechanisms depending of their chromosomal location leading to either faithful repair or possible integration of foreign information.
Investigation: GH, CB, LZ, EP, LC, and SM; Computer analysis: AT and CyB; Methodology: CyB, FC, and FL; Conceptualization: CB, AT, FL, and PL; Writing original draft: GH, CB, AT, and PL; Writing-review and editing: FL, FC, CyB, CB, AT, and PL; Resources: FC, FL, and PL; Funding acquisition: CB, AT, and PL; Supervision: AT and PL.
This work was supported by the French National Research Agency program Streptoflux (ANR Blanc 0096_01), by ANR through the Laboratory of Excellence ARBRE (ANR-12- LABXARBRE-01) and by the Région Lorraine.
The authors declare that the research was conducted in the absence of any commercial or financial relationships that could be construed as a potential conflict of interest.
We would like to thank Sébastien Riegler and Florian Kuntz from the Technological Resource center Aérial (Illkirch-Graffenstaden, France) for their technical support and their warm welcome.
The Supplementary Material for this article can be found online at: http://journal.frontiersin.org/article/10.3389/fmicb.2016.01901/full#supplementary-material
Abrudan, M. I., Smakman, F., Grimbergen, A. J., Westhoff, S., Miller, E. L., van Wezel, G. P., et al. (2015). Socially mediated induction and suppression of antibiosis during bacterial coexistence. Proc. Natl. Acad. Sci. U.S.A. 112, 11054–11059. doi: 10.1073/pnas.1504076112
Aigle, B., Holl, A., Angulo, J. F., Leblond, P., and Decaris, B. (1997). Characterization of two Streptomyces ambofaciens recA mutants: identification of the RecA protein by immunoblotting. FEMS Microbiol. Lett. 149, 181–187. doi: 10.1111/j.1574-6968.1997.tb10326.x
Alonso, J. C., Cardenas, P. P., Sanchez, H., Hejna, J., Suzuki, Y., and Takeyasu, K. (2013). Early steps of double-strand break repair in Bacillus subtilis. DNA Repair(Amst.) 12, 162–176. doi: 10.1016/j.dnarep.2012.12.005
Aniukwu, J., Glickman, M. S., and Shuman, S. (2008). The pathways and outcomes of mycobacterial NHEJ depend on the structure of the broken DNA ends. Genes Dev. 22, 512–527. doi: 10.1101/gad.1631908
Aravind, L., and Koonin, E. V. (2001). Prokaryotic homologs of the eukaryotic DNA-end-binding protein Ku, novel domains in the Ku protein and prediction of a prokaryotic double-strand break repair system. Genome Res. 11, 1365–1374. doi: 10.1101/gr.181001
Aravind, L., Makarova, K. S., and Koonin, E. V. (2000). Holliday junction resolvases and related nucleases: identification of new families, phyletic distribution and evolutionary trajectories. Nucleic Acids Res. 28, 3417–3422. doi: 10.1093/nar/28.18.3417
Bartlett, E. J., Brissett, N. C., and Doherty, A. J. (2013). Ribonucleolytic resection is required for repair of strand displaced nonhomologous end-joining intermediates. Proc. Natl. Acad. Sci. U.S.A. 110, E1984–E1991. doi: 10.1073/pnas.1302616110
Bentley, S. D., Chater, K. F., Cerdeno-Tarraga, A.-M., Challis, G. L., Thomson, N. R., James, K. D., et al. (2002). Complete genome sequence of the model actinomycete Streptomyces coelicolor A3 (2). Nature 417, 141–147. doi: 10.1038/417141a
Bhattarai, H., Gupta, R., and Glickman, M. S. (2014). DNA ligase C1 mediates the LigD-independent nonhomologous end-joining pathway of Mycobacterium smegmatis. J. Bacteriol. 196, 3366–3376. doi: 10.1128/JB.01832-14
Casadaban, M. J., and Cohen, S. N. (1980). Analysis of gene control signals by DNA fusion and cloning in Escherichia coli. J. Mol. Biol. 138, 179–207. doi: 10.1016/0022-2836(80)90283-1
Chater, K. F. (1993). Genetics of differentiation in Streptomyces. Annu. Rev. Microbiol. 47, 685–711. doi: 10.1146/annurev.mi.47.100193.003345
Choulet, F., Aigle, B., Gallois, A., Mangenot, S., Gerbaud, C., Truong, C., et al. (2006). Evolution of the terminal regions of the Streptomyces linear chromosome. Mol. Biol. Evol. 23, 2361–2369. doi: 10.1093/molbev/msl108
Davies, J., and Davies, D. (2010). Origins and evolution of antibiotic resistance. Microbiol. Mol. Biol. Rev. 74, 417–433. doi: 10.1128/MMBR.00016-10
de Ory, A., Nagler, K., Carrasco, B., Raguse, M., Zafra, O., Moeller, R., et al. (2016). Identification of a conserved 5’-dRP lyase activity in bacterial DNA repair ligase D and its potential role in base excision repair. Nucleic Acids Res. 44, 1833–1844. doi: 10.1093/nar/gkw054
de Ory, A., Zafra, O., and de Vega, M. (2014). Efficient processing of abasic sites by bacterial nonhomologous end-joining Ku proteins. Nucleic Acids Res. 42, 13082–13095. doi: 10.1093/nar/gku1029
Della, M., Palmbos, P. L., Tseng, H., Tonkin, L. M., Daley, J. M., Topper, L. M., et al. (2004). Mycobacterial Ku and ligase proteins constitute a two-component NHEJ repair machine. Science 306, 683–685. doi: 10.1126/science.1099824
Deriano, L., and Roth, D. B. (2013). Modernizing the nonhomologous end-joining repertoire: alternative and classical NHEJ share the stage. Annu. Rev. Genet. 47, 433–455. doi: 10.1146/annurev-genet-110711-155540
Fiester, S. E., Helfinstine, S. L., Redfearn, J. C., Uribe, R. M., and Woolverton, C. J. (2012). Electron beam irradiation dose dependently damages the Bacillus spore coat and spore membrane. Int. J. Microbiol. 2012, 1–9. doi: 10.1155/2012/579593
Fischer, G., Decaris, B., and Leblond, P. (1997). Occurrence of deletions, associated with genetic instability in Streptomyces ambofaciens, is independent of the linearity of the chromosomal DNA. J. Bacteriol. 179, 4553–4558. doi: 10.1128/jb.179.14.4553-4558.1997
Fischer, G., Wenner, T., Decaris, B., and Leblond, P. (1998). Chromosomal arm replacement generates a high level of intraspecific polymorphism in the terminal inverted repeats of the linear chromosomal DNA of Streptomyces ambofaciens. Proc. Natl. Acad. Sci. U.S.A. 95, 14296–14301. doi: 10.1073/pnas.95.24.14296
Galet, J., Deveau, A., Hôtel, L., Frey-Klett, P., Leblond, P., and Aigle, B. (2015). Pseudomonas fluorescens pirates both ferrioxamine and ferricoelichelin siderophores from Streptomyces ambofaciens. Appl. Environ. Microbiol. 81, 3132–3141. doi: 10.1128/AEM.03520-14
Giraud, A., Radman, M., Matic, I., and Taddei, F. (2001). The rise and fall of mutator bacteria. Curr. Opin. Microbiol. 4, 582–585. doi: 10.1016/S1369-5274(00)00254-X
Glickman, M. S. (2014). Double-strand DNA break repair in mycobacteria. Microbiol. Spectr. 2:MGM2-0024-2013. doi: 10.1128/microbiolspec.MGM2-0024-2013
Gong, C., Bongiorno, P., Martins, A., Stephanou, N. C., Zhu, H., Shuman, S., et al. (2005). Mechanism of nonhomologous end-joining in mycobacteria: a low-fidelity repair system driven by Ku, ligase D and ligase C. Nat. Struct. Mol. Biol. 12, 304–312. doi: 10.1038/nsmb915
Gong, C., Martins, A., Bongiorno, P., Glickman, M., and Shuman, S. (2004). Biochemical and genetic analysis of the four DNA ligases of mycobacteria. J. Biol. Chem. 279, 20594–20606. doi: 10.1074/jbc.M401841200
Gordon, J. L., Byrne, K. P., and Wolfe, K. H. (2011). Mechanisms of chromosome number evolution in yeast. PLoS Genet. 7:e1002190. doi: 10.1371/journal.pgen.1002190
Gupta, R., Barkan, D., Redelman-Sidi, G., Shuman, S., and Glickman, M. S. (2011). Mycobacteria exploit three genetically distinct DNA double-strand break repair pathways. Mol. Microbiol. 79, 316–330. doi: 10.1111/j.1365-2958.2010.07463.x
Gust, B., Challis, G. L., Fowler, K., Kieser, T., and Chater, K. F. (2003). PCR-targeted Streptomyces gene replacement identifies a protein domain needed for biosynthesis of the sesquiterpene soil odor geosmin. Proc. Natl. Acad. Sci. U.S.A. 100, 1541–1546. doi: 10.1073/pnas.0337542100
Hoff, G., Bertrand, C., Piotrowski, E., Thibessard, A., and Leblond, P. (2016). Implication of RuvABC and RecG in homologous recombination in Streptomyces ambofaciens. Res. Microbiol. doi: 10.1016/j.resmic.2016.07.003 [Epub ahead of print].
Hopwood, D. A. (2006). Soil to genomics: the Streptomyces chromosome. Annu. Rev. Genet. 40, 1–23. doi: 10.1146/annurev.genet.40.110405.090639
Huang, T.-W., and Chen, C. W. (2006). A recA null mutation may be generated in Streptomyces coelicolor. J. Bacteriol. 188, 6771–6779. doi: 10.1128/JB.00951-06
Ikeda, H., Ishikawa, J., Hanamoto, A., Shinose, M., Kikuchi, H., Shiba, T., et al. (2003). Complete genome sequence and comparative analysis of the industrial microorganism Streptomyces avermitilis. Nat. Biotechnol. 21, 526–531. doi: 10.1038/nbt820
Inoue, S., Higashiyama, K., Uchida, T., Hiratsu, K., and Kinashi, H. (2003). Chromosomal circularization in Streptomyces griseus by nonhomologous recombination of deletion ends. Biosci. Biotechnol. Biochem. 67, 1101–1108. doi: 10.1271/bbb.67.1101
Jacobs, M. A., Alwood, A., Thaipisuttikul, I., Spencer, D., Haugen, E., Ernst, S., et al. (2003). Comprehensive transposon mutant library of Pseudomonas aeruginosa. Proc. Natl. Acad. Sci. U.S.A. 100, 14339–14344. doi: 10.1073/pnas.2036282100
Kieser, T., Bibb, M. J., Buttner, M. J., Chater, K. F., and Hopwood, D. A. (2000). Practical Streptomyces Genetics. Norwich: John Innes Foundation.
Kim, J.-N., Kim, Y., Jeong, Y., Roe, J.-H., Kim, B.-G., and Cho, B.-K. (2015). Comparative genomics reveals the core and accessory genomes of Streptomyces species. J. Microbiol. Biotechnol. 25, 1599–1605. doi: 10.4014/jmb.1504.04008
Kobayashi, H., Simmons, L. A., Yuan, D. S., Broughton, W. J., and Walker, G. C. (2008). Multiple Ku orthologues mediate DNA non-homologous end-joining in the free-living form and during chronic infection of Sinorhizobium meliloti. Mol. Microbiol. 67, 350–363. doi: 10.1111/j.1365-2958.2007.06036.x
Kushwaha, A. K., and Grove, A. (2013). C-terminal low-complexity sequence repeats of Mycobacterium smegmatis Ku modulate DNA binding. Biosci. Rep. 33:e00016. doi: 10.1042/BSR20120105
Leblond, P., Demuyter, P., Simonet, J. M., and Decaris, B. (1990). Genetic instability and hypervariability in Streptomyces ambofaciens: towards an understanding of a mechanism of genome plasticity. Mol. Microbiol. 4, 707–714. doi: 10.1111/j.1365-2958.1990.tb00641.x
Lin, Y.-S., Kieser, H. M., Hopwood, D. A., and Chen, C. W. (1993). The chromosomal DNA of Streptomyces lividans 66 is linear. Mol. Microbiol. 10, 923–933. doi: 10.1111/j.1365-2958.1993.tb00964.x
Martínez-Burgo, Y., Álvarez-Álvarez, R., Rodríguez-García, A., and Liras, P. (2015). The pathway-specific regulator ClaR of Streptomyces clavuligerus has a global effect on the expression of genes for secondary metabolism and differentiation. Appl. Environ. Microbiol. 81, 6637–6648. doi: 10.1128/AEM.00916-15
McGovern, S., Baconnais, S., Roblin, P., Nicolas, P., Drevet, P., Simonson, H., et al. (2016). C-terminal region of bacterial Ku controls DNA bridging, DNA threading and recruitment of DNA ligase D for double strand breaks repair. Nucleic Acids Res. 44, 4785–4806. doi: 10.1093/nar/gkw149
Moeller, R., Stackebrandt, E., Reitz, G., Berger, T., Rettberg, P., Doherty, A. J., et al. (2007). Role of DNA repair by nonhomologous-end joining in Bacillus subtilis spore resistance to extreme dryness, mono- and polychromatic UV, and ionizing radiation. J. Bacteriol. 189, 3306–3311. doi: 10.1128/JB.00018-07
Muth, G., Frese, D., Kleber, A., and Wohlleben, W. (1997). Mutational analysis of the Streptomyces lividans recA gene suggests that only mutants with residual activity remain viable. Mol. Gen. Genet. 255, 420–428. doi: 10.1007/s004380050514
Paris,Ü, Mikkel, K., Tavita, K., Saumaa, S., Teras, R., and Kivisaar, M. (2015). NHEJ enzymes LigD and Ku participate in stationary-phase mutagenesis in Pseudomonas putida. DNA Repair (Amst.) 31, 11–18. doi: 10.1016/j.dnarep.2015.04.005
Pfaffl, M. W. (2001). A new mathematical model for relative quantification in real-time RT-PCR. Nucleic Acids Res. 29, 2002–2007. doi: 10.1093/nar/29.9.e45
Pinnert-Sindico, S. (1954). Une nouvelle espèce de Streptomyces productrice d’antibiotiques: Streptomyces ambofaciens n. sp. caractères culturaux. Ann. Inst. Pasteur. 87, 702–707.
Pitcher, R. S., Green, A. J., Brzostek, A., Korycka-Machala, M., Dziadek, J., and Doherty, A. J. (2007). NHEJ protects mycobacteria in stationary phase against the harmful effects of desiccation. DNA Repair (Amst.) 6, 1271–1276. doi: 10.1016/j.dnarep.2007.02.009
Pitcher, R. S., Tonkin, L. M., Green, A. J., and Doherty, A. J. (2005). Domain structure of a NHEJ DNA repair ligase from Mycobacterium tuberculosis. J. Mol. Biol. 351, 531–544. doi: 10.1016/j.jmb.2005.06.038
Raynal, A., Karray, F., Tuphile, K., Darbon-Rongere, E., and Pernodet, J.-L. (2006). Excisable cassettes: new tools for functional analysis of Streptomyces genomes. Appl. Environ. Microbiol. 72, 4839–4844. doi: 10.1128/AEM.00167-06
Rocha, E. P. C., Cornet, E., and Michel, B. (2005). Comparative and evolutionary analysis of the bacterial homologous recombination systems. PLoS Genet. 1:e15. doi: 10.1371/journal.pgen.0010015
Seipke, R. F., Kaltenpoth, M., and Hutchings, M. I. (2012). Streptomyces as symbionts: an emerging and widespread theme? FEMS Microbiol. Rev. 36, 862–876. doi: 10.1111/j.1574-6976.2011.00313.x
Shee, C., Gibson, J. L., and Rosenberg, S. M. (2012). Two mechanisms produce mutation hotspots at DNA breaks in Escherichia coli. Cell Rep. 2, 714–721. doi: 10.1016/j.celrep.2012.08.033
Stephanou, N. C., Gao, F., Bongiorno, P., Ehrt, S., Schnappinger, D., Shuman, S., et al. (2007). Mycobacterial nonhomologous end joining mediates mutagenic repair of chromosomal double-strand DNA breaks. J. Bacteriol. 189, 5237–5246. doi: 10.1128/JB.00332-07
Sun, J., Kelemen, G. H., Fernández-Abalos, J. M., and Bibb, M. J. (1999). Green fluorescent protein as a reporter for spatial and temporal gene expression in Streptomyces coelicolor A3 (2). Microbiology 145, 2221–2227. doi: 10.1099/00221287-145-9-2221
Tamura, K., Stecher, G., Peterson, D., Filipski, A., and Kumar, S. (2013). MEGA6: molecular evolutionary genetics analysis version 6.0. Mol. Biol. Evol. 30, 2725–2729. doi: 10.1093/molbev/mst197
Thibessard, A., Haas, D., Gerbaud, C., Aigle, B., Lautru, S., Pernodet, J.-L., et al. (2015). Complete genome sequence of Streptomyces ambofaciens ATCC 23877, the spiramycin producer. J. Biotechnol. 214, 117–118. doi: 10.1016/j.jbiotec.2015.09.020
Thibessard, A., and Leblond, P. (2014). “Subtelomere plasticity in the bacterium Streptomyces,” in Subtelomeres, eds E. J. Louis and M. M. Becker (Berlin: Springer), 243–258.
Uchida, T., Miyawaki, M., and Kinashi, H. (2003). Chromosomal arm replacement in Streptomyces griseus. J. Bacteriol. 185, 1120–1124. doi: 10.1128/JB.185.3.1120-1124.2003
Vierling, S., Weber, T., Wohlleben, W., and Muth, G. (2001). Evidence that an additional mutation is required to tolerate insertional inactivation of the Streptomyces lividans recA gene. J. Bacteriol. 183, 4374–4381. doi: 10.1128/JB.183.14.4374-4381.2001
Volff, J.-N., and Altenbuchner, J. (1998). Genetic instability of the Streptomyces chromosome. Mol. Microbiol. 27, 239–246. doi: 10.1046/j.1365-2958.1998.00652.x
Wang, S. T., Setlow, B., Conlon, E. M., Lyon, J. L., Imamura, D., Sato, T., et al. (2006). The forespore line of gene expression in Bacillus subtilis. J. Mol. Biol. 358, 16–37. doi: 10.1016/j.jmb.2006.01.059
Weller, G. R., Kysela, B., Roy, R., Tonkin, L. M., Scanlan, E., Della, M., et al. (2002). Identification of a DNA nonhomologous end-joining complex in bacteria. Science 297, 1686–1689. doi: 10.1126/science.1074584
Wenner, T., Roth, V., Fischer, G., Fourrier, C., Aigle, B., Decaris, B., et al. (2003). End-to-end fusion of linear deleted chromosomes initiates a cycle of genome instability in Streptomyces ambofaciens. Mol. Microbiol. 50, 411–425. doi: 10.1046/j.1365-2958.2003.03698.x
Yanai, K., Murakami, T., and Bibb, M. (2006). Amplification of the entire kanamycin biosynthetic gene cluster during empirical strain improvement of Streptomyces kanamyceticus. Proc. Natl. Acad. Sci. U.S.A. 103, 9661–9666. doi: 10.1073/pnas.0603251103
Zhang, L., Nguyen, H. C., Chipot, L., Piotrowski, E., Bertrand, C., Thibessard, A., et al. (2014). The adnAB locus, encoding a putative helicase-nuclease activity, is essential in Streptomyces. J. Bacteriol. 196, 2701–2708. doi: 10.1128/JB.01513-14
Zhang, X., Chen, W., Zhang, Y., Jiang, L., Chen, Z., Wen, Y., et al. (2012). Deletion of ku homologs increases gene targeting frequency in Streptomyces avermitilis. J. Ind. Microbiol. Biotechnol. 39, 917–925. doi: 10.1007/s10295-012-1097-x
Zhu, H., Bhattarai, H., Yan, H.-G., Shuman, S., and Glickman, M. S. (2012). Characterization of Mycobacterium smegmatis PolD2 and PolD1 as RNA/DNA polymerases homologous to the POL domain of bacterial DNA ligase D. Biochemistry 51, 10147–10158. doi: 10.1021/bi301202e
Zhu, H., and Shuman, S. (2007). Characterization of Agrobacterium tumefaciens DNA ligases C and D. Nucleic Acids Res. 35, 3631–3645. doi: 10.1093/nar/gkm145
Keywords: Streptomyces, non-homologous end joining, DNA repair, double strand breaks (DSBs), ligases, Ku protein, DNA damage
Citation: Hoff G, Bertrand C, Zhang L, Piotrowski E, Chipot L, Bontemps C, Confalonieri F, McGovern S, Lecointe F, Thibessard A and Leblond P (2016) Multiple and Variable NHEJ-Like Genes Are Involved in Resistance to DNA Damage in Streptomyces ambofaciens. Front. Microbiol. 7:1901. doi: 10.3389/fmicb.2016.01901
Received: 07 October 2016; Accepted: 14 November 2016;
Published: 28 November 2016.
Edited by:
Frank T. Robb, University of Maryland, Baltimore, USAReviewed by:
Igor Kovalchuk, University of Lethbridge, CanadaCopyright © 2016 Hoff, Bertrand, Zhang, Piotrowski, Chipot, Bontemps, Confalonieri, McGovern, Lecointe, Thibessard and Leblond. This is an open-access article distributed under the terms of the Creative Commons Attribution License (CC BY). The use, distribution or reproduction in other forums is permitted, provided the original author(s) or licensor are credited and that the original publication in this journal is cited, in accordance with accepted academic practice. No use, distribution or reproduction is permitted which does not comply with these terms.
*Correspondence: Pierre Leblond, cGllcnJlLmxlYmxvbmRAdW5pdi1sb3JyYWluZS5mcg==
Disclaimer: All claims expressed in this article are solely those of the authors and do not necessarily represent those of their affiliated organizations, or those of the publisher, the editors and the reviewers. Any product that may be evaluated in this article or claim that may be made by its manufacturer is not guaranteed or endorsed by the publisher.
Research integrity at Frontiers
Learn more about the work of our research integrity team to safeguard the quality of each article we publish.