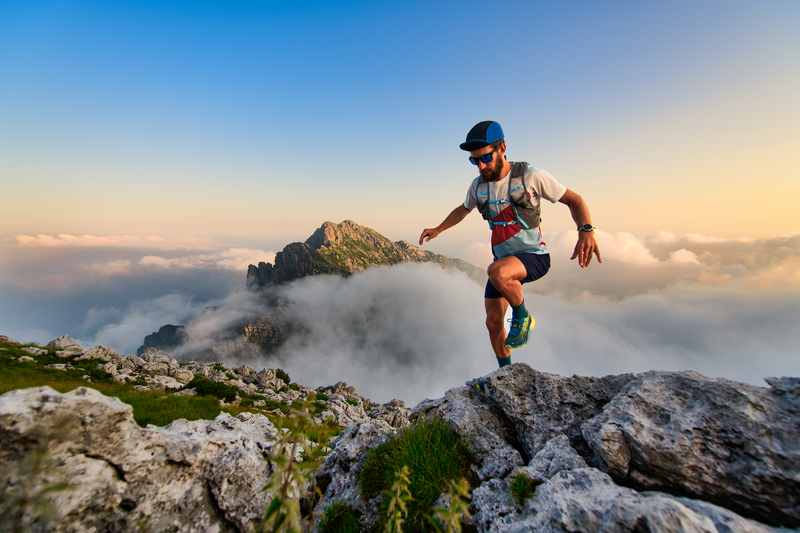
95% of researchers rate our articles as excellent or good
Learn more about the work of our research integrity team to safeguard the quality of each article we publish.
Find out more
HYPOTHESIS AND THEORY article
Front. Microbiol. , 15 November 2016
Sec. Microbiological Chemistry and Geomicrobiology
Volume 7 - 2016 | https://doi.org/10.3389/fmicb.2016.01784
This article is part of the Research Topic Origin and Evolution of Photosynthesis View all 7 articles
Stromatolites are solid, laminar structures of biological origin. Living examples are sparsely distributed and formed by cyanobacteria, which are oxygenic phototrophs. However, stromatolites were abundant between 3.4 and 2.4 Gyr, prior to the advent of cyanobacteria and oxygenic photosynthesis. Here I propose that many Archaean stromatolites were seeded at points of efflux of hydrogen sulfide from hydrothermal fields into shallow water, while their laminar composition arose from alternating modes of strictly anoxygenic photosynthetic metabolism. These changes were a redox regulatory response of gene expression to changing hydrogen sulfide concentration, which fluctuated with intermittent dilution by tidal action or by rainfall into surface waters. The proposed redox switch between modes of metabolism deposited sequential microbial mats. These mats gave rise to alternating carbonate sediments predicted to retain evidence of their origin in differing ratios of isotopes of carbon and sulfur and in organic content. The mats may have arisen either by replacement of microbial populations or by continuous lineages of protocyanobacteria in which a redox genetic switch selected between Types I and II photosynthetic reaction centers, and thus between photolithoautotrophic and photoorganoheterotrophic metabolism. In the latter case, and by 2.4 Gyr at the latest, a mutation had disabled the redox genetic switch to give simultaneous constitutive expression of both Types I and II reaction centers, and thus to the ability to extract electrons from manganese and then water. By this simple step, the first cyanobacterium had the dramatic advantage of emancipation from limiting supplies of inorganic electron donors, produced free molecular oxygen as a waste product, and initiated the Great Oxidation Event in Earth’s history at the transition from the Archaean to the Paleoproterozoic.
Stromatolites today are unusual features of shorelines. They grow typically in anoxic, shallow, hypersaline waters (Figure 1) and are constructed by microbial communities whose primary producers are cyanobacteria. In the pre-Cambrian Palaeozoic and Archaean eons, stromatolites were much more abundant than today, took on a wide variety of sizes and morphologies, and built massive reefs of sediments whose organic and inorganic carbon isotopic signatures suggest large-scale solar energy conversion with primary productivity comparable with that seen today in coral reefs (Bosak et al., 2013).
FIGURE 1. Stromatolites at Hamelin Pool, Western Australia, 4 October 2007. Photograph kindly provided by Dr. Catherine Colas des Francs-Small.
Archaean stromatolites are an anomaly. If cyanobacteria had yet to appear, what made them? There is clear and multiple, independent lines of evidence that the Earth’s oceans and atmosphere were anoxic for roughly half its history, that is, until 2.4 Gyr (Knoll et al., 2016). Oxygen-evolving photosynthesis then emerged from anoxygenic photosynthesis with the advent of cyanobacteria – the only oxygenic phototrophic prokaryotes (Schopf, 2011). Nevertheless stromatolites comprise exposed sediments, notably in South Africa and Western Australia, that are dated unambiguously up to at least a billion years before the start of the rise in atmospheric oxygen concentration (Hofmann et al., 1999; Allwood et al., 2006; Nutman et al., 2016). It has been considered that cyanobacteria may have first emerged during the early or mid-Archaean, giving “whiffs of oxygen” (Holland, 2006; Summons et al., 2006). However, the abundance of Archaean stromatolite coastal reefs (Schopf et al., 2007; Bosak et al., 2013) is likely to reflect abundant photosynthetic microbial activity that could be expected to have created a much earlier Great Oxidation Event if cyanobacteria alone had been responsible.
Oxygenic photosynthesis appeared with the first cyanobacterium (Fischer et al., 2016). Photosynthetic oxygen production always requires two, connected photosystems (Nelson and Junge, 2015). Photosystem I uses light energy at its primary photochemical reaction center to oxidize a chlorophyll molecule that donates its electron to a series of iron–sulfur proteins (Fromme et al., 2001; Amunts et al., 2007), and then on to coupled assimilatory metabolism such as the Benson-Calvin cycle of CO2 fixation. In contrast, the oxidized chlorophyll of the photosystem II reaction center passes its electron to a pair of quinone molecules (Nitschke and Rutherford, 1991; Brinkert et al., 2016). The photooxidised chlorophyll of photosystem II is reduced by electrons from water (Umena et al., 2011; Shen, 2015; Ho et al., 2016). Oxidation of two water molecules by transfer of four electrons releases one molecule of oxygen. Photosystem I and photosystem II have homologs in the single, separate photosystems of anoxygenic photosynthetic bacteria, each with either a Type I or a Type II photochemical reaction center (Figure 2), while it is clear that Types I and reaction centers are themselves homologous (Schubert et al., 1998) – related by descent from a common ancestor. For the origin of oxygenic photosynthesis, the reaction centers of two independent photosystems must have become connected, electrically in series, to allow two separate light reactions (Hill and Bendall, 1960) to transfer electrons from water to the iron–sulfur acceptors that supply electrons at low redox potentials to assimilatory reactions (Hohmann-Marriott and Blankenship, 2011) (Figure 3).
FIGURE 2. Electron transport chains incorporating either Type II (left) or Type I (right) reaction centers of anoxygenic photosynthesis.
FIGURE 3. The electron transport chain of oxygenic photosynthesis, where Types II and I reaction centers are linked, in series, as photosystem II (PS II) and photosystem I (PS I), respectively.
One proposal for the way in which this close coupling began is that genes for each of the two reaction centers, Types I and II, were present as alternatives in a versatile, anoxygenic photosynthetic bacterium termed the “protocyanobacterium” (Allen, 2005; Allen and Martin, 2007).
Photosynthesis is a light-driven redox reaction that can be supported by any of a variety of electron donors (sources) and acceptors (sinks). Archaean electron donors included hydrogen, ferrous iron, and hydrogen sulfide, each varying in availability with time and location (Bosak et al., 2013). Major Archean electron acceptors were carbon dioxide and nitrogen gas, as today, but not oxygen.
The overall process of photosynthesis as light-driven electron or hydrogen transfer to CO2 is summarized in the van Niel equation (1) (Van Niel, 1954):
Where H2A is an electron donor, A is its oxidation product, and CH2O represents sugars, organic products of CO2 fixation. H2A can be inorganic, giving photolithotrophy, or organic, giving photoorganotrophy. Oxygenic photosynthesis is the special case of photolithotrophy where H2A is H2O and 2A is O2. Anoxygenic photosynthesis today typically relies either on inorganic donors, as seen in green and purple sulfur bacteria, which are obligate anaerobes, or on organic donors, as seen in green and purple non-sulfur bacteria, which today can often tolerate oxygen, but not while remaining phototrophic.
It should be noted that CO2 is an assimilatory substrate and not a direct electron acceptor for photosynthesis, and the van Niel equation (1) can be regarded as the sum of two half reactions, (2) and (3):
Together with coupled synthesis of ATP, photosynthetic reduction of ferredoxin drives eventual assimilation of CO2 and N2.
N2 fixation, reaction (4), is a “dark reaction” analogous to reaction (3):
The overall equation for photosynthetic N2 fixation is then the sum of (2) and (4), giving (5):
I suggest that, in addition to CO2 fixation, light-driven N2 fixation with an unlimited supply of reductant may have been an immediate benefit of the use of water as an electron donor by the first cyanobacterium. Phylogenetic analysis suggests that the earliest cyanobacteria were indeed filamentous nitrogen-fixers (Dagan et al., 2013). While inhibition of nitrogen fixation by O2 would have been an immediate consequence of water oxidation, this penalty could have been offset by dilution of free O2, into the anoxic environment. Environmental O2 concentration may then have risen slowly, eventually to reach a steady state where a low maximal rate of nitrogen fixation sustained a correspondingly low rate of photosynthetic water oxidation. An atmospheric oxygen concentration of about 10% of that seen today is a maximum beyond which nitrogen fixation cannot proceed (Berman-Frank et al., 2003; Falkowski, 2007). It is possible to imagine that atmospheric oxygen was maintained by this dynamic equilibrium at about 10% of its present concentration during the “boring billion” (Holland, 2006; Johnston et al., 2009; Lyons et al., 2014) of the Proterozoic.
Major differences between oxygenic and anoxygenic photosynthesis are listed in Table 1.
Anaerobic, anoxygenic photosynthesis is likely to have been be the only option for Archaean primary production using the energy of sunlight. Where H2S is available as an electron donor, Type I photosynthetic reaction centers transport electrons to iron–sulfur electron acceptors – ferredoxins – in a linear or non-cyclic electron transport pathway. In contrast, where organic electron donors such as succinate or pyruvate are used, Type II photosynthetic reaction centers use quinone acceptors that pass electrons back to the reaction center, giving proton-motive cyclic electron flow and coupled ATP synthesis Figure 2.
Hydrothermal activity would have produced marked variation in concentration of dissolved inorganic electron donors in Archaean euphotic zones such as littoral, coastal, and river environments where microbial mats were formed (Homann et al., 2015). Environments replete with ferrous iron or hydrogen sulfide would have changed repeatedly and reversibly to ones where the only available electron donors were organic – these locations would therefore have alternated in their provision for photosynthesis by Types I and II reaction centers.
A versatile, anoxygenic bacterium with inducible Types I and II photochemical reaction centers has been proposed as the immediate forerunner of oxygenic cyanobacteria (Allen, 2005) (Figure 4). Such a protocyanobacterium would have had a minimum quantum requirement for four-electron transport of 4, which conferred, at limiting light intensities, an energetic advantage over oxygenic photosynthesis with its equivalent quantum requirement of at least 8. This advantage suggests that protocyanobacteria may persist today, albeit at low abundance in specialized environments where light is a limiting factor for growth and there is a fluctuating availability of inorganic and organic electron donors. In the late Archaean, however, CO2 concentration was much higher than it is today, solar luminosity was lower, and facultative Type I–Type II photosynthesis can be envisaged as an optimal growth strategy where supplies of an inorganic electron donor varied. One reason for this variation may have been the cyclic depletion of Fe2+ when insoluble Fe3+ salts were formed, thus giving rise to the banded iron formations seen throughout the Archaean and well into the Proterozoic. Fe2+ depletion could also have arisen where H2S was in excess of Fe, giving FeS and FeS2 (pyrite). However, when Fe2+ ran out, and, in any case, in shallow waters above hydrothermal fields, hydrogen sulfide derived ultimately from magma would have been introduced at specific points, and along fissures, in the bedrock, and would have been available an electron donor both by sulfur bacteria and by the protocyanobacteria. The competitive advantage of the protocyanobacteria would have been maintenance of populations of cells with their core metabolisms and genetic systems always in place. It is thus possible that protocyanobacteria contributed to the massive stromatolite and microbialite reefs laid down before, after, and across the Archaean-Proterozoic boundary.
FIGURE 4. The hypothetical protocyanobacterium. Expression of Type I center genes in the presence of H2S gives a Type I reaction center (RC I) and is accompanied by repression of Type II genes. In the absence of H2S, Type II genes are induced, giving a Type II reaction center (RC II), and Type I genes become repressed. Subsequent impairment of redox regulatory control allows expression and co-existence of both Type I and Type II reaction centers, with complementary functions. In place of H2S, the Type II center, as PS II, oxidizes water, liberating oxygen, and donates electrons to the Type I center, as PS I. The proposed loss of the redox regulatory switch replaces the logical (Boolean) relation “Type I XOR Type II” (each type excluding the other) with “Type I OR Type II” (either is, and both are, allowed). This in turn leads to “Type I AND Type II” when interdependency of PSs I and II is established in the non-cyclic electron transport chain of oxygenic photosynthesis.
Observations on the Strelley Pool formation of the Pilbara Craton in Eastern Australia shows that stromatolites were abundant at 3.4 Gyr (Wacey, 2010), and their distribution coincides spatially with hydrothermal venting as judged by lithography and with the presence of sulfur isotopes in both organic (kerogen) and inorganic (carbonate) stromatolite composition (Sugitani et al., 2015). The proposed process of stromatolite building as H2S supply fluctuated is outlined schematically in Figure 5. Figure 6 provides a context for hydrothermally derived columnar stromatolites built by influx of H2S.
FIGURE 5. A model for seeding of stromatolites. Left (green box): H2S is exhaled from a hydrothermal vent or fissure, accumulates, and serves as an electron donor to Type I photosynthesis in photoautotrophic growth. Right (purple box): H2S is depleted by dilution through tidal action or rainfall, leaving Type II, photoheterotrophic growth, using organic compounds (gray) that accumulated as products of photosynthesis during the previous, photoautotrophic stage.
FIGURE 6. A model for formation and growth of stromatolites. Sequential concentration and dilution of hydrothermally derived H2S results in alternation of Types I and II photosynthesis, and in alternation of photoautotrophy and photoheterotrophy. Sequential deposition of layers of cellular material from each mode of metabolism produces a laminar substrate that raises the growing microbial mat incrementally nearer to the surface of the water and source of light.
In contrast to the first bacteria and archaea, and to microbiota growing in the vicinity of submarine hydrothermal vents, all of which were chemoautotrophic, by the Archaean extensive microbial growth had become dependent on photosynthesis, and required sunlight. Depending on solutes and turbidity, water transmits light only to limited depths at wavelengths and flux densities that can be used by photosynthesis (Falkowski and Raven, 2007). Thus stromatolites grew in shallow water, and became abundant where large surface areas were exposed to sunlight, typically adjacent to shorelines or in river estuaries.
Where H2S was the photosynthetic electron donor, photolithoautotrophic growth would have been favored at precise locations where H2S flux gave rise to optimal steady-state concentrations, that is, in the vicinity of sources of H2S from hydrothermal activity. Such coastal hydrothermal fields supported microbial communities and stromatolite formation at 3.4 Gya as seen in the Strelley Pool Formation, Pilbara Craton, Western Australia (Sugitani et al., 2015). Fractured rock in hydrothermal fields is predicted to have given a specific spatial pattern of seeding or initiation of stromatolite growth, corresponding to the pattern of entry to dissolved H2S into the water. It is therefore proposed that each individual Archaean stromatolite column can be assigned to an individual channel or fissure in the field’s bedrock.
Enzyme-catalyzed reactions discriminate in favor of lighter isotopes of elements in substrates. 13C is about one percent of total carbon on Earth, the rest being 12C. Photoautotrophic growth thus preferentially assimilates 12CO2 into organic products. The greater the number of enzymatic steps involved, the greater the depletion of 13C in the photosynthate, as illustrated today by the differing isotopic compositions of C3 and C4 crop plants (von Caemmerer et al., 2014). If stromatolite laminae were formed by alternating phases of autotrophic and heterotrophic growth, then the delta-13C composition of the organic material should fluctuate and be expected to be lowest in the heterotrophic layers, reliant on metabolic reactions additional to those of the preceding, primary carboxylation step of CO2 assimilation. Photoautotrophically obtained carbon in alternate laminae will still show a lower delta-13C than that of the surrounding environment. A clear pattern is also to be expected of increased 13C in carbonates precipitated from the surrounding medium. These would be richest in 13C where they had selectively been depleted of 12C by CO2 assimilation, while showing evidence of re-enrichment with 12C from the environment during the heterotrophic phase of growth.
The same logic applies to predicted sulfur isotopic signatures, where negative relative values of the ratio 34S/32S are taken to indicate a biological origin. Large negative 34S/32S values are reported for bulk barite (barium sulfate) deposits in chert of the Dresser formation (Philippot et al., 2007). These values could be predicted to have arisen from an H2S-oxidizing, Type I photosynthesis, where the initial enzyme-catalyzed reaction is that of sulfide-quinone oxidoreductase (Arieli et al., 1994). If the biological mass fractionation of sulfur isotopes arose from alternating photolithotrophy and photoautotrophy, giving stromatolite laminae by a process as depicted in Figures 5 and 6, then, given sufficient spatial resolution, negative delta 34S excursions in products of lithotrophic growth should be coincident with negative delta 12C excursions reporting autotrophy.
For both predictions 3 and 4, above, mass spectroscopy of Archaean stromatolite laminae nanometer-scale resolution (Lepot et al., 2008) may be required. Laminae can be as thin as a millimeter (Bosak et al., 2009), arguing for relatively transient deposition of sequential layers of microbial biomass.
Each of the predicted observations 1–4, above, might be explained by cyclical replacement of anoxygenic microbial mats each composed of either sulfur (Type I) or non-sulfur (Type II) photosynthetic bacteria. However, another possibility is that one type of protocyanobacterium predominated and left signatures of alternating modes of metabolism supported by one genome containing genes for both Types I and II reaction centers. The advantage of this special case of the hypothesis is that it allows us to envisage simple steps from anoxygenic to oxygenic photosynthesis, a profound evolutionary transition otherwise lacking clear explanation supported by observation. The late Archaean abundance of the protocyanobacterium and its proposed role in the onset of the GOE makes further predictions 5–7, as follows.
Photosystem II abstracts four electrons from two water molecules, yielding one oxygen molecule. It does this by accumulating four positive changes in an inorganic prosthetic group of four manganese atoms, one calcium, and five oxygen atoms (Umena et al., 2011). A Type II reaction center oxidizing manganese seems likely to have been a precursor of the oxygen-evolving photosystem II reaction center (Khorobrykh et al., 2013) and might itself have evolved from a Type II reaction center capable of oxidizing chlorophyll a to chlorophyll f (Ho et al., 2016). A sequence of manganese carbonate from the Koegas Subgroup, Kaapvaal Craton, South Africa is reported at 2.41 Gyr, when free oxygen is reported to have been absent (Johnson et al., 2013). The bacterium that deposited manganese may have done so as a result of the first coupling of Types II and I reaction centers, and only later could higher oxidation states of manganese become re-reduced by water (Allen, 2014; Fischer et al., 2016). When this happened, environmental manganese would have ceases to be a substrate for lithotrophy, and manganese became obligatory as a catalyst of water oxidation, to be sequestered and conserved. It is possible that minerals such as hollandite with a unit cell resembling the water-oxidizing cluster of photosystem II accumulated from such biological concentration and deposition (Russell et al., 2008).
The redox switch hypothesis (Allen, 2005) rests on the assumption of an anoxygenic phototroph as an immediate precursor of the first true two-light reaction phototroph, whether the latter oxidized environmental manganese directly, or water by means of a derived manganese catalyst (Allen and Martin, 2007; Russell et al., 2008). It could be argued that the protocyanobacterium was out-competed and displaced by the cyanobacteria to which it gave rise, and eventually became extinct. There are, however, specialized environments today where a fluctuating supply of hydrogen sulfide into an anoxic, photic water column would confer a selective advantage upon growth by means of a single photosystem, where the quantum yield is twice that of growth requiring two light reactions. Facultatively oxygenic Oscillatoria species, including Oscillatoria limnetica, are today oxygenic in the absence of H2S but switch in the presence of H2S to a Type I-only photosynthesis (Oren and Padan, 1978). If an Oscillatoria species were to retain the capacity for photoheterotrophic growth using a photosystem II depleted of water-oxidizing complex then its quantum requirement (of four) would be lower in both of its modes metabolism than a that (of eight) of a regular cyanobacterium. The protocyanobacterium would be better adapted than cyanobacteria to growth at low light intensity. It seems worth looking for Type I–Type II versatile phototrophs in anoxic, low-light environments with variable concentration of dissolved H2S. Stromatolite formation by anoxygenic photosynthesis may have continued through the Proterozoic (Johnston et al., 2009), gradually becoming displaced by facultative, oxygenic photosynthesis in cyanobacteria resembling extant Oscillatoria species.
Cyanobacteria (Ashby and Houmard, 2006) and bacteria in general (Stock et al., 2000) respond to environmental change by means of two-component regulatory systems. These systems consist of a histidine sensor kinase that transfers a phosphoryl group to a response regulator if, and only if, a specific environmental change occurs. The response regulator controls gene expression, usually though not exclusively at the level of transcription, in such a way as to bring about a change that better adapts the organism to its changed environment. Redox state of electron carriers responds rapidly to relevant environmental changes, and redox sensor kinases (Allen, 1993) are known to regulate photosynthetic gene transcription in cyanobacteria (Li and Sherman, 2000) and chloroplasts (Puthiyaveetil et al., 2008). A two-component redox genetic switch has been characterized that selects between transcription of photosystems I and II reaction center apoproteins in both chloroplasts (Pfannschmidt et al., 1999) and cyanobacteria (Ibrahim et al., 2016a). A protocyanobacterial version of this system, centered on the conserved Histidine Kinase 2 (Ibrahim et al., 2016b), could govern transcription of Types I and II reaction center genes and underlie the metabolic adaptability of the proposed protocyanobacterium. A simple mutation that impairs the switch could have been the beginning to two photosystems functioning simultaneously and in series (Allen, 2005, 2014).
Throughout Earth’s history, its crust, lithosphere, hydrosphere and atmosphere have undergone immense changes in overall composition (Nisbet and Sleep, 2001), while environments for life have been dynamic on a wide range of time scales (Allen, 1998). Life’s capacity to adapt is one of its defining features (Allen, 2010) making regulation at the boundary of internal and external environments coincident with mechanisms of nutrient exchange and energy transduction; a requirement for life’s origin (Mitchell, 1957; Sousa et al., 2013). By the Archaean the planet’s surface had separated into solid, liquid, and gaseous phases – into land and sea sharing a turbulent atmosphere. Tidal action, evaporation and precipitation would have created varying concentrations of solutes, notably at shore-lines and in rock pools, lakes, and rivers. When photosynthesis began to supply an input of free energy, itself varying diurnally and seasonally, then shallow waters, the euphotic zones, are most likely to have become locations of the greatest biological activity and primary productivity.
Here I suggest that alternating Types I and type II photosynthesis is a plausible mechanism for the seeding (Figure 5) and growth (Figure 6) of stromatolites, such as those recorded in Archaean stratigraphy, in the absence of cyanobacteria. It is further suggested that this type of versatile phototrophic growth occurred in single lineages of a protocyanobacterium that was a natural precursor of the first oxygenic cyanobacteria. The single step from Type I-OR-Type II photosynthesis to Type I-AND-Type II photosynthesis gave rise to two-light reactions, water-oxidizing, oxygenic photosynthesis (Allen, 2005; Allen and Martin, 2007; Russell et al., 2008), proliferation of genes for oxidation-reduction enzymes (Baymann et al., 2003; David and Alm, 2011), and to emancipation of biology from fleeting and potentially rate-limiting supplies of inorganic electron donors. The evolutionary success of this transition at 2.4 Gyr was the basis of the Great Oxidation Event, one of the greatest transitions in the evolution of life on our planet (Lyons et al., 2014).
The author confirms being the sole contributor of this work and approved it for publication.
The authors declare that the research was conducted in the absence of any commercial or financial relationships that could be construed as a potential conflict of interest.
I thank Michael J. Russell, William F. Martin, John A. Raven, Sujith Puthiyaveetil, Nick Lane, Wolfgang Nitschke, and Brenda Thake for discussion, Catherine Colas des Francs-Small for the photograph in Figure 1, two referees for comments on the manuscript, and the Leverhulme Trust for Emeritus Research Fellowship EM-2015-068.
Allen, J. F. (1993). Redox control of transcription: sensors, response regulators, activators and repressors. FEBS Lett. 332, 203–207. doi: 10.1016/0014-5793(93)80631-4
Allen, J. F. (1998). “Light, time and micro-organisms,” in Proceedings of the Symposium of the Society for General Microbiology: Microbial Responses to Light and Time, eds M. X. Caddick, S. Baumberg, D. A. Hodgson, and M. K. Phillips-Jones (Cambridge: Cambridge University Press), 1–31.
Allen, J. F. (2005). A redox switch hypothesis for the origin of two light reactions in photosynthesis. FEBS Lett. 579, 963–968. doi: 10.1016/j.febslet.2005.01.015
Allen, J. F. (2010). Redox homeostasis in the emergence of life. On the constant internal environment of nascent living cells. J. Cosmol. 10, 3362–3373.
Allen, J. F. (2014). “Origin of oxygenic photosynthesis from anoxygenic type I and Type II reaction centers,” in The Biophysics of Photosynthesis, eds J. H. Golbeck and A. Van Der Est (New York, NY: Springer), 422–450.
Allen, J. F., and Martin, W. (2007). Evolutionary biology – Out of thin air. Nature 445, 610–612. doi: 10.1038/445610a
Allwood, A. C., Walter, M. R., Kamber, B. S., Marshall, C. P., and Burch, I. W. (2006). Stromatolite reef from the Early Archaean era of Australia. Nature 441, 714–718. doi: 10.1038/nature04764
Amunts, A., Drory, O., and Nelson, N. (2007). The structure of a plant photosystem I supercomplex at 3.4 resolution. Nature 447, 58–63. doi: 10.1038/nature05687
Arieli, B., Shahak, Y., Taglicht, D., Hauska, G., and Padan, E. (1994). Purification and characterization of sulfide-quinone reductase, a novel enzyme driving anoxygenic photosynthesis in Oscillatoria limnetica. J. Biol. Chem. 269, 5705–5711.
Ashby, M. K., and Houmard, J. (2006). Cyanobacterial two-component proteins: structure, diversity, distribution, and evolution. Microbiol. Mol. Biol. Rev. 70, 472–509. doi: 10.1128/MMBR.00046-05
Baymann, F., Lebrun, E., Brugna, M., Schoepp–Cothenet, B., Giudici–Orticoni, M.-T., and Nitschke, W. (2003). The redox protein construction kit: pre-last universal common ancestor evolution of energy-conserving enzymes. Philos. Trans. R. Soc. Lond. B Biol. Sci. 358, 267–274. doi: 10.1098/rstb.2002.1184
Berman-Frank, I., Lundgren, P., and Falkowski, P. (2003). Nitrogen fixation and photosynthetic oxygen evolution in cyanobacteria. Res. Microbiol. 154, 157–164. doi: 10.1016/s0923-2508(03)00029-9
Bosak, T., Knoll, A. H., and Petroff, A. P. (2013). “The meaning of stromatolites,” in Annual Review of Earth and Planetary Sciences, Vol. 41, ed. R. Jeanloz (Palo Alto, CA: Annual Reviews), 21–44.
Bosak, T., Liang, B., Sim, M. S., and Petroff, A. P. (2009). Morphological record of oxygenic photosynthesis in conical stromatolites. Proc. Natl. Acad. Sci. U.S.A. 106, 10939–10943. doi: 10.1073/pnas.0900885106
Brinkert, K., De Causmaecker, S., Krieger-Liszkay, A., Fantuzzi, A., and Rutherford, A. W. (2016). Bicarbonate-induced redox tuning in Photosystem II for regulation and protection. Proc. Natl. Acad. Sci. U.S.A. 113, 12144–12149. doi: 10.1073/pnas.1608862113
Dagan, T., Roettger, M., Stucken, K., Landan, G., Koch, R., Major, P., et al. (2013). Genomes of stigonematalean cyanobacteria (subsection v) and the evolution of oxygenic photosynthesis from prokaryotes to plastids. Genome Biol. Evol. 5, 31–44. doi: 10.1093/Gbe/Evs117
David, L. A., and Alm, E. J. (2011). Rapid evolutionary innovation during an Archaean genetic expansion. Nature 469, 93–96. doi: 10.1038/nature09649
Falkowski, P. G. (2007). “On the evolution of the carbon cycle,” in Phytoplankton Productivity: Carbon Assimilation in Marine and Freshwater Ecosystems, eds P. J. L. B. Williams, D. N. Thomas, and C. S. Reynolds (Oxford: Blackwell Science Ltd), 318–349.
Falkowski, P. G., and Raven, J. A. (2007). Aquatic Photosynthesis. Princeton, NJ: Princeton University Press.
Fischer, W. W., Hemp, J., and Johnson, J. E. (2016). “Evolution of oxygenic photosynthesis,” in Annual Review of Earth and Planetary Sciences, Vol. 44, eds R. Jeanloz and K. H. Freeman (Palo Alto: Annual Reviews), 647–683.
Fromme, P., Jordan, P., and Krauss, N. (2001). Structure of photosystem I. Biochim. Biophys. Acta Bioenerg. 1507, 5–31. doi: 10.1016/S0005-2728(01)001955
Hill, R., and Bendall, F. (1960). Function of the two cytochrome components in chloroplasts: a working hypothesis. Nature 186, 136–137. doi: 10.1038/186136a0
Ho, M.-Y., Shen, G., Canniffe, D. P., Zhao, C., and Bryant, D. A. (2016). Light-dependent chlorophyll f synthase is a highly divergent paralog of PsbA of photosystem II. Science 353:aaf9178. doi: 10.1126/science.aaf9178
Hofmann, H. J., Grey, K., Hickman, A. H., and Thorpe, R. I. (1999). Origin of 3.45 Ga coniform stromatolites in Warrawoona Group, Western Australia. Geol. Soc. Am. Bull. 111, 1256–1262. doi: 10.1130/0016-7606(1999)111<1256:oogcsi>2.3.co;2
Hohmann-Marriott, M. F., and Blankenship, R. E. (2011). Evolution of photosynthesis. Annu. Rev. Plant Biol. 62, 515–548. doi: 10.1146/Annurev-Arplant-042110-103811
Holland, H. D. (2006). The oxygenation of the atmosphere and oceans. Philos. Trans. R. Soc. Lond. B Biol. Sci. 361, 903–915. doi: 10.1098/rstb.2006.1838
Homann, M., Heubeck, C., Airo, A., and Tice, M. M. (2015). Morphological adaptations of 3.22 Ga-old tufted microbial mats to Archean coastal habitats (Moodies Group, Barberton Greenstone Belt, South Africa). Precambrian Res. 266, 47–64. doi: 10.1016/j.precamres.2015.04.018
Ibrahim, I. M., Puthiyaveetil, S., and Allen, J. F. (2016a). A two-component regulatory system in transcriptional control of photosystem stoichiometry: redox-dependent and sodium ion-dependent phosphoryl transfer from cyanobacterial histidine kinase Hik2 to response regulators rrel and RppA. Front. Plant Sci. 7:137. doi: 10.3389/fpls.2016.00137
Ibrahim, I. M., Puthiyaveetil, S., Khan, C., and Allen, J. F. (2016b). Probing the nucleotide-binding activity of a redox sensor: two-component regulatory control in chloroplasts. Photosynth. Res. 130, 93–101. doi: 10.1007/s11120-016-0229-y
Johnson, J. E., Webb, S. M., Thomas, K., Ono, S., Kirschvink, J. L., and Fischer, W. W. (2013). Manganese-oxidizing photosynthesis before the rise of cyanobacteria. Proc. Natl. Acad. Sci. U.S.A. 110, 11238–11243. doi: 10.1073/pnas.1305530110
Johnston, D. T., Wolfe-Simon, F., Pearson, A., and Knoll, A. H. (2009). Anoxygenic photosynthesis modulated Proterozoic oxygen and sustained Earth’s middle age. Proc. Natl. Acad. Sci. U.S.A. 106, 16925–16929. doi: 10.1073/pnas.0909248106
Khorobrykh, A., Dasgupta, J., Kolling, D. R. J., Terentyev, V., Klimov, V. V., and Dismukes, G. C. (2013). Evolutionary origins of the photosynthetic water oxidation cluster: bicarbonate permits Mn2+ photo-oxidation by anoxygenic bacterial reaction centers. Chembiochem 14, 1725–1731. doi: 10.1002/cbic.201300355
Knoll, A. H., Bergmann, K. D., and Strauss, J. V. (2016). Life: the first two billion years. Philos. Trans. R. Soc. B. Biol. Sci. 371. doi: 10.1098/rstb.2015.0493
Lepot, K., Benzerara, K., Brown, G. E., and Philippot, P. (2008). Microbially influenced formation of 2,724-million-year-old stromatolites. Nat. Geosci. 1, 118–121. doi: 10.1038/ngeo107
Li, H., and Sherman, L. A. (2000). A redox-responsive regulator of photosynthesis gene expression in the cyanobacterium Synechocystis sp strain PCC 6803. J. Bacteriol. 182, 4268–4277. doi: 10.1128/JB.182.15.4268-4277.2000
Lyons, T. W., Reinhard, C. T., and Planavsky, N. J. (2014). The rise of oxygen in Earth’s early ocean and atmosphere. Nature 506, 307–315. doi: 10.1038/nature13068
Mitchell, P. (1957). “The origin of life and the formation and organising functions of natural membranes,” in Proceedings of the First International Symposium on the Origin of Life on the Earth, eds A. I. Oparin, A. G. Pasynski, A. E. Braunstein, and T. E. Pavlovskaya (Moscow: USSR Academy of Sciences), 229–234.
Nelson, N., and Junge, W. (2015). Structure and energy transfer in photosystems of oxygenic photosynthesis. Annu. Rev. Biochem. 84, 659–683. doi: 10.1146/annurev-biochem-092914-041942
Nisbet, E. G., and Sleep, N. H. (2001). The habitat and nature of early life. Nature 409, 1083–1091. doi: 10.1038/35059210
Nitschke, W., and Rutherford, A. W. (1991). Photosynthetic reaction centers - variations on a common structural theme. Trends Biochem. Sci. 16, 241–245. doi: 10.1016/0968-0004(91)90095-D
Nutman, A. P., Bennett, V. C., Friend, C. R. L., Van Kranendonk, M. J., and Chivas, A. R. (2016). Rapid emergence of life shown by discovery of 3,700-million-year-old microbial structures. Nature 537, 535–538. doi: 10.1038/nature19355
Oren, A., and Padan, E. (1978). Induction of anaerobic, photoautotrophic growth in the cyanobacterium Oscillatoria limnetica. J. Bacteriol. 133, 558–563.
Pfannschmidt, T., Nilsson, A., and Allen, J. F. (1999). Photosynthetic control of chloroplast gene expression. Nature 397, 625–628. doi: 10.1038/17624
Philippot, P., Van Zuilen, M., Lepot, K., Thomazo, C., Farquhar, J., and Van Kranendonk, M. J. (2007). Early Archaean microorganisms preferred elemental sulfur, not sulfate. Science 317, 1534–1537. doi: 10.1126/science.1145861
Puthiyaveetil, S., Kavanagh, T. A., Cain, P., Sullivan, J. A., Newell, C. A., Gray, J. C., et al. (2008). The ancestral symbiont sensor kinase CSK links photosynthesis with gene expression in chloroplasts. Proc. Natl. Acad. Sci. U.S.A. 105, 10061–10066. doi: 10.1073/pnas.0803928105
Russell, M. J., Allen, J. F., and Milner-White, E. J. (2008). “Inorganic complexes enabled the onset of life and oxygenic photosynthesis,” in Energy from the Sun: Proceedings of the 14th International Congress on Photosynthesis, eds J. F. Allen, E. Gantt, J. H. Golbeck, and B. Osmond (Heidelberg: Springer), 1187–1192.
Schopf, J. W. (2011). The paleobiological record of photosynthesis. Photosynth. Res. 107, 87–101. doi: 10.1007/s11120-010-9577-1
Schopf, J. W., Kudryavtsev, A. B., Czaja, A. D., and Tripathi, A. B. (2007). Evidence of archean life: stromatolites and microfossils. Precambrian Res. 158, 141–155. doi: 10.1016/j.precamres.2007.04.009
Schubert, W. D., Klukas, O., Saenger, W., Witt, H. T., Fromme, P., and Krauss, N. (1998). A common ancestor for oxygenic and anoxygenic photosynthetic systems: a comparison based on the structural model of photosystem I. J. Mol. Biol. 280, 297–314. doi: 10.1006/Jmbi.1998.1824
Shen, J.-R. (2015). The structure of photosystem II and the mechanism of water oxidation in photosynthesis. Annu. Rev. Plant Biol. 66, 23–48. doi: 10.1146/annurev-arplant-050312-120129
Sousa, F. L., Thiergart, T., Landan, G., Nelson-Sathi, S., Pereira, I. A. C., Allen, J. F., et al. (2013). Early bioenergetic evolution. Philos. Trans. R. Soc. Lond. B Biol. Sci. 368:20130088. doi: 10.1098/rstb.2013.0088
Stock, A. M., Robinson, V. L., and Goudreau, P. N. (2000). Two-component signal transduction. Annu. Rev. Biochem. 69, 183–215. doi: 10.1146/annurev.biochem.69.1.183
Sugitani, K., Mimura, K., Takeuchi, M., Yamaguchi, T., Suzuki, K., Senda, R., et al. (2015). A Paleoarchean coastal hydrothermal field inhabited by diverse microbial communities: the Strelley Pool Formation, Pilbara Craton, Western Australia. Geobiology 13, 522–545. doi: 10.1111/gbi.12150
Summons, R. E., Bradley, A. S., Jahnke, L. L., and Waldbauer, J. R. (2006). Steroids, triterpenoids and molecular oxygen. Philos. Trans. R. Soc. Lond. B Biol. Sci. 361, 951–968. doi: 10.1098/rstb.2006.1837
Umena, Y., Kawakami, K., Shen, J. R., and Kamiya, N. (2011). Crystal structure of oxygen-evolving photosystem II at a resolution of 1.9 angstrom. Nature 473, 55–60. doi: 10.1038/Nature09913
Van Niel, C. B. (1954). The chemoautotrophic and photosynthetic bacteria. Annu. Rev. Microbiol. 8, 105–132. doi: 10.1146/annurev.mi.08.100154.000541
von Caemmerer, S., Ghannoum, O., Pengelly, J. J. L., and Cousins, A. B. (2014). Carbon isotope discrimination as a tool to explore C-4 photosynthesis. J. Exp. Bot. 65, 3459–3470. doi: 10.1093/jxb/eru127
Keywords: cyanobacteria, redox regulation, Great Oxidation Event, boring billion, protoerozoic, protocyanobacterium, redox switch hypothesis, two-component regulatory systems
Citation: Allen JF (2016) A Proposal for Formation of Archaean Stromatolites before the Advent of Oxygenic Photosynthesis. Front. Microbiol. 7:1784. doi: 10.3389/fmicb.2016.01784
Received: 24 June 2016; Accepted: 24 October 2016;
Published: 15 November 2016.
Edited by:
Trinity L. Hamilton, University of Cincinnati, USAReviewed by:
Mustafa Yucel, Middle East Technical University, TurkeyCopyright © 2016 Allen. This is an open-access article distributed under the terms of the Creative Commons Attribution License (CC BY). The use, distribution or reproduction in other forums is permitted, provided the original author(s) or licensor are credited and that the original publication in this journal is cited, in accordance with accepted academic practice. No use, distribution or reproduction is permitted which does not comply with these terms.
*Correspondence: John F. Allen, ai5mLmFsbGVuQHVjbC5hYy51aw==
Disclaimer: All claims expressed in this article are solely those of the authors and do not necessarily represent those of their affiliated organizations, or those of the publisher, the editors and the reviewers. Any product that may be evaluated in this article or claim that may be made by its manufacturer is not guaranteed or endorsed by the publisher.
Research integrity at Frontiers
Learn more about the work of our research integrity team to safeguard the quality of each article we publish.