- Department of Biological Sciences, Biological Sciences Building, University of Alberta, Edmonton, AB, Canada
Ammonia-oxidizing bacteria (AOB) have well characterized genes that encode and express nitrite reductases (NIR) and nitric oxide reductases (NOR). However, the connection between presence or absence of these and other genes for nitrogen transformations with the physiological production of nitric oxide (NO) and nitrous oxide (N2O) has not been tested across AOB isolated from various trophic states, with diverse phylogeny, and with closed genomes. It is therefore unclear if genomic content for nitrogen oxide metabolism is predictive of net N2O production. Instantaneous microrespirometry experiments were utilized to measure NO and N2O emitted by AOB during active oxidation of ammonia (NH3) or hydroxylamine (NH2OH) and through a period of anoxia. This data was used in concert with genomic content and phylogeny to assess whether taxonomic factors were predictive of nitrogen oxide metabolism. Results showed that two oligotrophic AOB strains lacking annotated NOR-encoding genes released large quantities of NO and produced N2O abiologically at the onset of anoxia following NH3-oxidation. Furthermore, high concentrations of N2O were measured during active O2-dependent NH2OH oxidation by the two oligotrophic AOB in contrast to non-oligotrophic strains that only produced N2O at the onset of anoxia. Therefore, complete nitrifier denitrification did not occur in the two oligotrophic strains, but did occur in meso- and eutrophic strains, even in Nitrosomonas communis Nm2 that lacks an annotated NIR-encoding gene. Regardless of mechanism, all AOB strains produced measureable N2O under tested conditions. This work further confirms that AOB require NOR activity to enzymatically reduce NO to N2O in the nitrifier denitrification pathway, and also that abiotic reactions play an important role in N2O formation, in oligotrophic AOB lacking NOR activity.
Introduction
Chemolithotrophic ammonia-oxidizing bacteria (AOB) are important players in the global biogeochemical nitrogen cycle and perform the first step in nitrification; the oxidation of ammonia (NH3) to nitrite (). AOB are abundant in a vast array of environments including soils, marine and fresh-water, and wastewater treatment plants (Klotz et al., 2006; Norton et al., 2008; Jia and Conrad, 2009; Ke et al., 2015) and are implicated in production of nitrous oxide (N2O) through enzymatic (Stein, 2011; Kozlowski et al., 2014) and abiotic processes (Jones et al., 2015; Zhu-Barker et al., 2015). AOB have the potential to utilize as an alternate terminal electron acceptor through the process of nitrifier denitrification (Stein, 2011) resulting in net production of N2O (Stein and Yung, 2003; Kool et al., 2011; Zhu et al., 2013). N2O has been measured from pure cultures of AOB from both the Nitrosomonas (Poth and Focht, 1985; Kozlowski et al., 2014) and Nitrosospira (Dundee and Hopkins, 2001; Wrage et al., 2004; Shaw et al., 2006) genera. However, studies on the enzymology and pathways of N2O production by AOB have mostly focused on N. europaea ATCC 19718 (Beaumont et al., 2002, 2004; Cantera and Stein, 2007; Yu and Chandran, 2010; Yu et al., 2010; Kozlowski et al., 2014) leaving open the possibility that not all AOB strains share equivalent pathways and regulatory mechanisms.
The nitrifier denitrification pathway includes a nitrite reductase (NIR) to reduce to nitric oxide (NO) and nitric oxide reductase (NOR) to reduce NO to N2O. All closed AOB genomes, with the exception of N. communis Nm2 (Kozlowski et al., 2016b), have genes encoding the copper-containing NirK (Prosser et al., 2014). Furthermore, all AOB encode NOR genes (norB and/or norY) with the exception of Nitrosomonas sp. Is79A3 (Bollmann et al., 2013) and N. ureae Nm10 (Kozlowski et al., 2016a). Both Nitrosomonas sp. Is79A3 and N. ureae Nm10 are considered oligotrophic, growing optimally in medium containing 1–5 mM ammonium (Prosser et al., 2014). In contrast, N. communis Nm2 is considered eutrophic and prefers higher concentrations of 10–50 mM ammonium (Prosser et al., 2014).
Previous studies on the model organism N. europaea, a eutrophic strain, showed that both hydroxylamine (NH2OH) oxidation and reduction can lead to significant emission of N2O (Cantera and Stein, 2007; Kozlowski et al., 2014). Previous work also revealed that NorB, but not NirK, is required for production of N2O by N. europaea (Kozlowski et al., 2014). This observation, in addition to the lack of annotated NIR or NOR genes in some closed AOB genomes, has brought into question whether all AOB can even perform nitrifier denitrification and emit N2O under similar conditions as N. europaea. There is also a question of whether uncharacterized NIR and/or NOR enzymes are expressed in AOB that can contribute to the process. The production and metabolism of NO and its role in N2O emission is another understudied aspect of nitrogen oxide metabolism in AOB; N. multiformis ATCC 25196 was recently found to emit large quantities of NO during active NH3-oxidation (Kozlowski et al., 2016c).
Due to the lack of comparative information on nitrogen oxide metabolism in AOB, five strains representing different phylogenies and trophic states and with closed genomes were selected for this study. Our main objectives were to: (i) compare NO and N2O production profiles of the five strains during NH3 and NH2OH oxidation and over a period of anoxia when nitrifier denitrification is most active in N. europaea, and (ii) determine whether gene content, trophic state, and/or phylogeny of these diverse AOB were predictive of their capacity to metabolize and/or emit NO or N2O.
Materials and Methods
Strains and Cultivation
AOB strains included N. europaea ATCC 19718T, N. communis strain Nm2T, Nitrosomonas sp. Is79A3, N. ureae Nm10T, and N. multiformis ATCC 25196T. All strains have closed genomes and grow under similar cultivation conditions to allow for proper comparisons across phylotypes and trophic status. Furthermore, an AOB strain was selected from each cluster in the Betaproteobacteria with a cultured representative, 3, 6, 7, and 8 (based on 16S rRNA phylogeny; Norton, 2011), with the exception of the newly cultured cluster 0 N. lacus sp. nov. as its genome is not yet closed (Garcia et al., 2013; Urakawa et al., 2014). AOB cultures were grown and maintained in Wheaton bottles (250 mL) sealed with caps inlayed with butyl rubber stoppers at 28°C in 100 mL HEPES-buffered HK medium (Krümmel and Harms, 1982) and phenol red as pH indicator (pH of 7.5–8) with either 5 mM (NH4)2SO4 for the meso- and eutrophic strains (N. europaea, N. communis, and N. multiformis), or 2.5 mM (NH4)2SO4 for the oligotrophic strains (Nitrosomonas sp. Is79A3 and N. ureae; Prosser et al., 2014). All cultures were transferred (5% v/v inoculum) when ca. 80% of the NH3 substrate was consumed as determined by concentration (Bollmann et al., 2011). The pH of all cultures was adjusted as needed with 10% NaHCO3.
Phylogenetic and Genome Analysis of AOB
PhyloPhlAn (Segata et al., 2013) was used to generate and analyze the genome-wide phylogeny of AOB. Genomes of 14 AOB were obtained from the National Center for Biotechnology Information1. All of the predicted protein-coding sequences for each genome were exported into PhyloPhlAn to identify and align 400 broadly conserved protein sequences between all of the input genomes. PhyML 3.0 (Guindon et al., 2010) was used to construct a maximum likelihood phylogeny using the Gammaproteobacteria as the root and node support was calculated using 500 bootstrap replicates.
Microrespirometry Experiments
Instantaneous microrespirometry (MR) experiments of AOB are described in detail elsewhere (Kozlowski et al., 2016c). Briefly, MR experiments were performed at 28°C in a 10 mL 2-port injection lid glass chamber (Unisense, Aarhus, Denmark). For instantaneous experiments all strains were grown to late-log phase (7–8 mM ), filtered on Supor® 200 0.2 μm filters (Pall, Ann Arbor WI), and rinsed three times with NH3-free HK media (Krümmel and Harms, 1982). Ca. 1 × 1010 total cells were used per experiment for all strains as determined by direct cell count by phase-contrast light microscopy. All cells for instantaneous MR measurements were in a planktonic state, re-suspended in NH3-free HK medium and provided either 2 mM NH4Cl as substrate or pulses of 250 μM or 100 μM NH2OH-HCl (final chamber concentration; 99.999% purity, Sigma–Aldrich, St Louis, MO, USA). Previous testing revealed that all strains could tolerate up to 250 μM NH2OH (final chamber concentration) with the exception of N. communis which was unable to tolerate more than 100 μM NH2OH (final chamber concentration) per injection (data not shown). Chamber O2 was determined by an O2 electrode (OX-MR 500 μm tip diameter MR oxygen electrode; Unisense, Aarhus, DenmarK), N2O concentration was measured using an N2O-500 N2O minisensor electrode with 500 μm tip diameter (Unisense, Aarhus, DenmarK), and NO was measured using an ami-600 NO sensor with 600 μm tip diameter (Innovative Instruments Inc., Tampa, FL, USA). The availability of O2 in the MR chamber, a closed system, was ca. 243 μM O2 based on equilibrium O2 concentration at operating temperatures and medium salinities for experiments performed without N2-sparged medium.
Chemical Controls
Chemical controls were performed to determine the production of N2O from reactivity of NH2OH with media + , or from killed-cells (1 × 1010 total cells) with media + NH2OH. Chemical controls used N2-sparged medium (to achieve 0–3% O2 saturation in liquid phase) containing 250 μM NaNO2 and then adding 250 μM NH2OH (final chamber concentration) to reflect conditions in the chamber when testing for consumption by AOB as an alternate terminal electron acceptor with NH2OH as the electron donor. Cells for control experiments were heat-killed by boiling for 30 min. The heat-killed cell controls involved addition of 250 μM NH2OH to the MR-chamber containing N2-sparged media with 1 × 1010 total heat-killed cells of each AOB strain. N2O was measured as described above.
Results and Discussion
Phylogeny and Comparative Gene Inventory of AOB
A whole-genome analysis utilizing PhyloPhlAn showed that each of the 5 Betaproteobacteria AOB chosen for physiological analysis in the present study separated into individual clades (Figure 1). The separation of each AOB into a unique branch, using 400 core protein markers from available complete genome sequences to form a high-resolution tree, shows a clearer and greater separation than currently available 16S rRNA or amoA single gene sequence phylogenies (Norton, 2011). The results of this multiple-marker, genome-wide, comparison highlight a need to reevaluate and perhaps reclassify some members of Nitrosomonas into different genera.
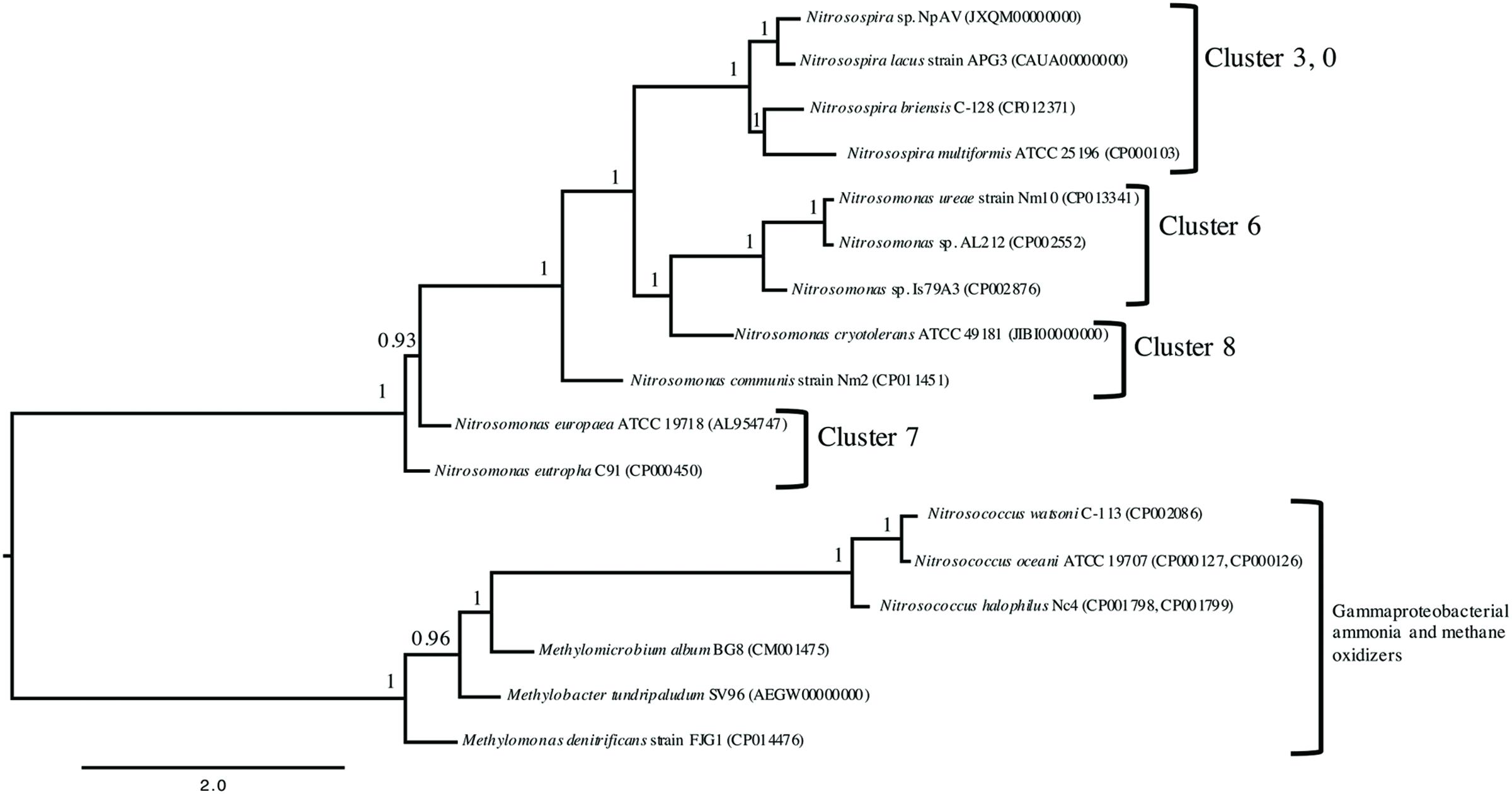
FIGURE 1. Rooted maximum likelihood phylogeny of 14 publically available genomes of ammonia oxidizing bacteria based on 400 broadly conserved amino-acid sequences. PhyloPhlAn (Segata et al., 2013) was used to identify and align the amino-acid sequences in all input genomes. The tree was constructed using PhyML 3.0 (Guindon et al., 2010) with the Gammaproteobacteria as the root. Bootstrap values (as a proportion of 500 replicates) are denoted above the branches and branch lengths correspond to sequence differences as indicated by the scale bar at the bottom.
Comparison of inventory involved in central ammonia-oxidizing metabolism and NOx production revealed differences across the 5 strains (Table 1). In agreement with previous analyses of AMO gene clusters in betaproteobacterial AOB (Klotz and Stein, 2011) all AOB of the current study contain 1–2 copies of the amoCABED cluster encoding ammonia-monooxygenase (Table 1). All strains encoded at least one monocistronic copy of the amoC gene with the exception of N. communis (Table 1), a feature shared in common with the gammaproteobacerial AOB (Klotz et al., 2006; Arp et al., 2007; Campbell et al., 2011). The singleton AmoC is proposed to participate in cellular recovery from stressors such as elevated temperatures and starvation by stabilizing the AMO complex in the membrane of N. europaea (Berube and Stahl, 2012). Also, every strain encoded at least one copy of the amoD gene in tandem with amoE, a common feature of betaproteobacterial AOB still needing biochemical characterization (Klotz and Stein, 2011). It is also common for betaproteobacterial AOB to encode 2–3 complete or incomplete (lacking cycB) copies of the haoAB-cycAB cluster (Arp et al., 2007). However, N. ureae represents the first sequenced AOB to harbor 4 complete copies of the Hydroxylamine dehydrogenase (HAO) gene cluster (Table 1). Knockouts of one or two haoA gene copies from N. europaea did not result in a significant phenotype (Hommes et al., 2002), suggesting that the multiple copies are isofunctional. However, knockouts of individual amoA or amoB gene copies in N. europaea did result in different phenotypes, suggesting that operons encoding AMO are differentially regulated (Stein et al., 2000). For N. ureae and perhaps Nitrosomonas sp. AL212 (Suwa et al., 2011), additional gene clusters encoding AMO and HAO could be a strategy to thrive in oligotrophic environments to gain maximum reductant from available substrate; however, further studies are required to validate whether all of the gene copies are expressed, isofunctional, and/or differentially regulated. As with N. europaea and N. eutropha, one copy of the HAO gene cluster in N. communis lacks cycB (Table 1), encoding cytochrome Cm552 (Arp et al., 2007). All strains, with the exception of Nitrosomonas sp. Is79A3 (Bollmann et al., 2013), encode the AOB-specific red copper protein nitrosocyanin (Table 1) proposed to be involved in the NH3-oxidation pathway as a redox sensitive electron carrier (Arciero et al., 2002; Sayavedra-Soto and Arp, 2011).
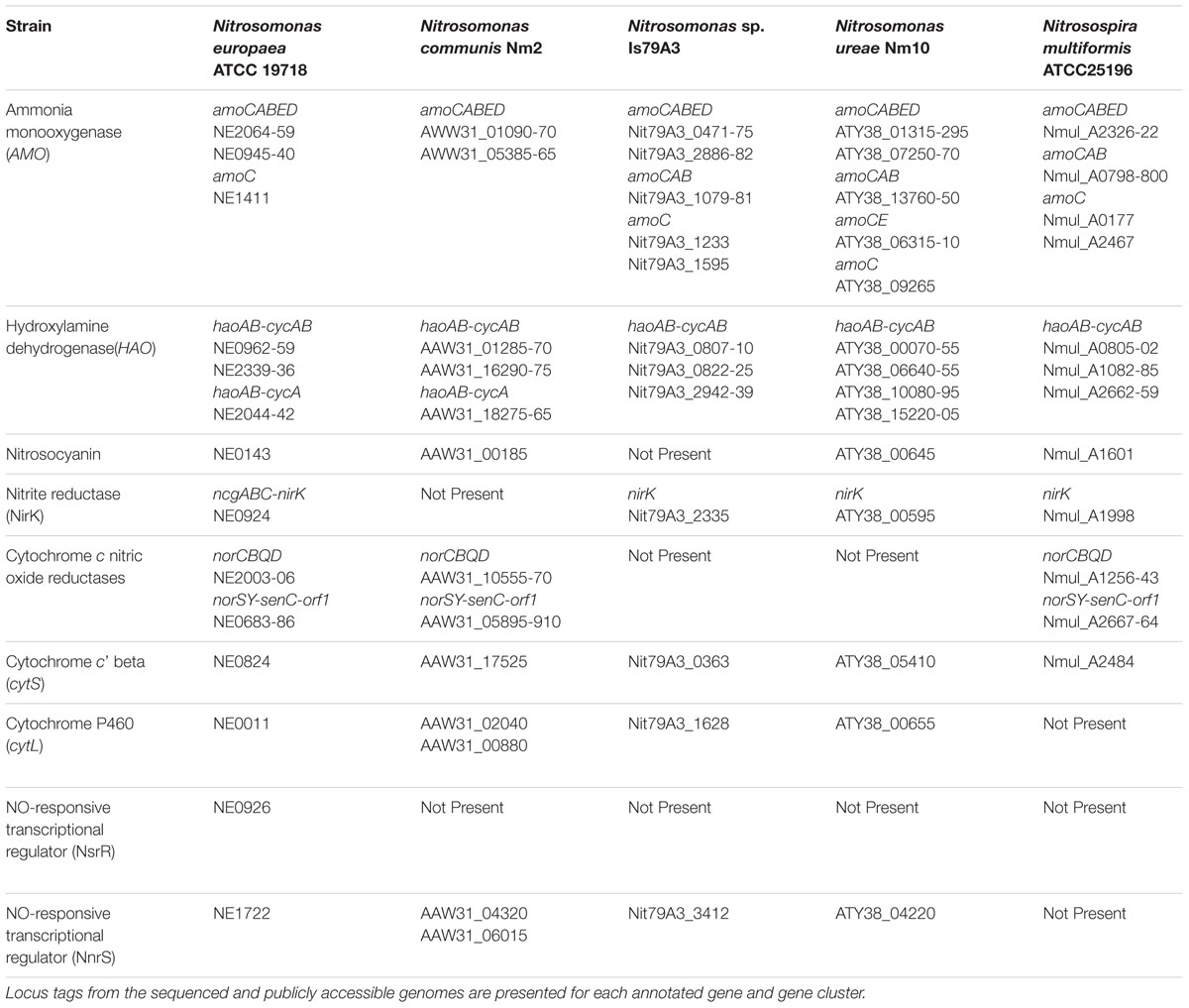
TABLE 1. Annotated gene inventory with implications in ammonia-oxidation or N-oxide metabolism from complete genomes of Betaproteobacteria AOB utilized in the present study.
Analysis of NIR and NOR genes revealed that N. communis is the only sequenced and closed AOB genome without a copper-containing nitrite reductase (nirK; Kozlowski et al., 2016b) (Table 1). This is interesting as nirK is present in all published genomes of ammonia-oxidizing Thaumarchaeota (AOA; Bartossek et al., 2010), is highly expressed in metatranscriptomes (Hollibaugh et al., 2011; Radax et al., 2012), and is important for efficient substrate oxidation in N. europaea (Cantera and Stein, 2007; Kozlowski et al., 2014). Of the 5 strains, only N. europaea contains the operonic nirK and NO-responsive nsrR transcriptional regulator (Chain et al., 2003; Table 1), features shared by the closely related N. eutropha C-91 strain (Stein et al., 2007) (Figure 1). All the Nitrosomonas strains, but not N. multiformis, encode the NO-responsive NnrS transcriptional regulator. Two strains, Nitrosomonas sp. Is79A3 and N. ureae, both within the Cluster 6 AOB, lack annotated operons for cytochrome c nitric oxide reductases (Bollmann et al., 2013; Kozlowski et al., 2016a) (Table 1). The genome of the closely related Nitrosomonas sp. AL212 (Figure 1) does encode norCBQD but lacks genes for the other NOR frequently found in AOB genomes, norSY-senC-orf1 (Suwa et al., 2011). We hypothesize that environments with low substrate availability do not experience oversaturation of NH3 and thus preclude accumulation of N-oxides such as NH2OH and NO (Hooper and Terry, 1979). Thus, NORs may not be not required by some oligotrophic AOB as nitrosative stress should be minimal. However, testing of strains such as Nitrosomonas AL212, an oligotrophic, NOR-encoding strain, must be accomplished to determine whether trophic state or gene content is more predictive of nitrifier denitrification activity. N. multiformis does not have an annotated cytochrome P460 (cytL) whereas N. communis has two copies (Table 1), a feature shared with Nitrosomonas sp. AL212 (Suwa et al., 2011). Cytochrome P460 has a proposed role in detoxification of NOx through the simultaneous oxidation NH2OH and NO to (Elmore et al., 2007; Stein, 2011) and may be important for alleviating nitrosative stress in AOB lacking NORs. All 5 genomes also contain sequences for cytochrome c’ beta, potentially having NOR activity (Elmore et al., 2007; Stein, 2011). Future work with focus on the transcription and activities of cytochromes P460 and c’ beta under conditions of nitrosative stress would better clarify the role of both enzymes as substitutes for lack of annotated NORs.
Comparison of Instantaneous NOx Production from AOB during Oxidation of NH3 or NH2OH
Measurement of NO or N2O production during oxidation of NH3 or NH2OH were compared among the 5 strains and revealed that all AOB produce measureable quantities of NO during active oxidation of NH3 (Figures 2A,C,E,G,I). Although each AOB had a unique and dynamic NO production profile, making comparative rate calculations impractical, all strains produced>50 nM NO (per 1 × 1010 total cells) prior to anoxia in the MR chamber. N. europaea produced the least amount of NO compared to the other strains during active oxidation and prior to anoxia (Figure 2A; Supplementary Table S1). As reported previously (Kozlowski et al., 2016c) N. multiformis began re-consuming NO once ca. 50% O2 was left in the MR-chamber (Figure 2I) and both N. europaea and N. communis re-consumed a small amount of NO following anoxia in the MR-chamber (Figures 2A,C). Interestingly, either immediately upon O2 depletion in the case of Nitrosomonas sp. Is79A3 (Figure 2E) or ca. 5 min. post-anoxia for N. ureae, these two strains released massive quantities of NO outside the limit for measurement by the ami-600 NO microsensor (Figures 2E,G). Unlike the other AOB strains, neither Nitrosomonas sp. Is79A3 nor N. ureae re-consumed NO during active NH3-oxidation or following anoxia in the MR-chamber.
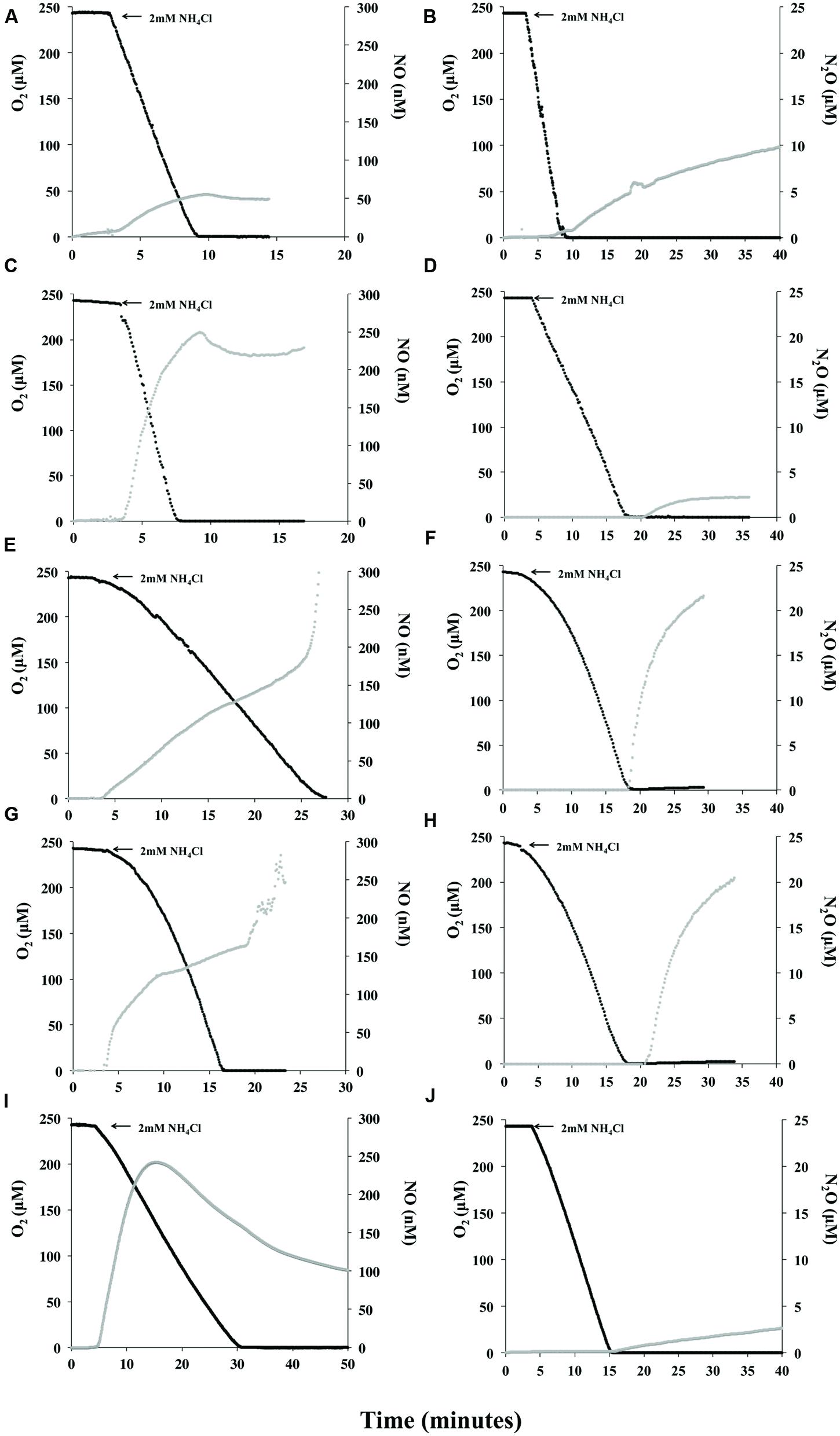
FIGURE 2. Instantaneous measurement of O2 consumption and NO or N2O during oxidation of 2 mM NH4Cl. Nitrosomonas europaea (A,B), N. communis (C,D), Nitrosomonas sp. Is79A3 (E,F), N. ureae (G,H), Nitrosospira multiformis (I,J). Panels are single representative measurements of reproducible results (n = 3). Note differences in scale of x-axis for traces of NO production during NH3-oxidation.
Measurement of NO during active substrate oxidation has so far only been studied in pure cultures of N. europaea (Kester et al., 1997; Yu and Chandran, 2010; Yu et al., 2010) and N. multiformis (Kozlowski et al., 2016c), both of which have annotated nirK, norB, and norY genes (Table 1). It is known, however, that the thaumarchaeotal ammonia-oxidizers (AOA) also produce NO during NH3-oxidation (Martens-Habbena et al., 2015; Kozlowski et al., 2016c); however, they retain very tight control over its production and consumption (Kozlowski et al., 2016c). There are significant similarities in NO profiles of the AOA Nitrososphaera viennensis and the oligotrophic AOB of the present study in that once O2 was depleted in the MR chamber substantial quantities of NO were released (Figures 2E,G; Kozlowski et al., 2016c). This similarity between the AOA and the oligotrophic AOB, both lineages with a low Km and high affinity for ammonium (Martens-Habbena et al., 2009; Stahl and de la Torre, 2012; Prosser et al., 2014), could be explained by a lack of NOR genes to combat high intracellular NO experienced during anoxia either due to release of NO directly from the NH3-oxidation pathway, in the case of AOA (Kozlowski et al., 2016c), or perhaps from reduction in the case of the AOB (Stein, 2011). Importantly, the N2O measured from N. viennensis following NH3-oxidation and over an extended period of anoxia was a result of NO release and abiotic media-dependent conversion to N2O (Kozlowski et al., 2016c). Also, in the nitrifier-denitrification pathway of N. europaea, it should be noted that NorB is required for reduction to N2O (Kozlowski et al., 2014). This suggests that the lack of annotated NOR precludes a complete nitrifier-denitrification pathway in ammonia-oxidizers.
Following O2 depletion and in the presence of some AOB can perform nitrifier denitrification (Stein, 2011; Kozlowski et al., 2014). This was tested in the present study by measurement of N2O during active NH3- or NH2OH-oxidation and through a period of anoxia (Figures 2 and 3). It should be noted that the Km for the copper-containing nitrite reductase, NirK, has not been tested for AOB and therefore it is not known whether ca. 162 or 243 μM following NH3 or NH2OH oxidation, respectively, in the chamber is at saturation for NirK.
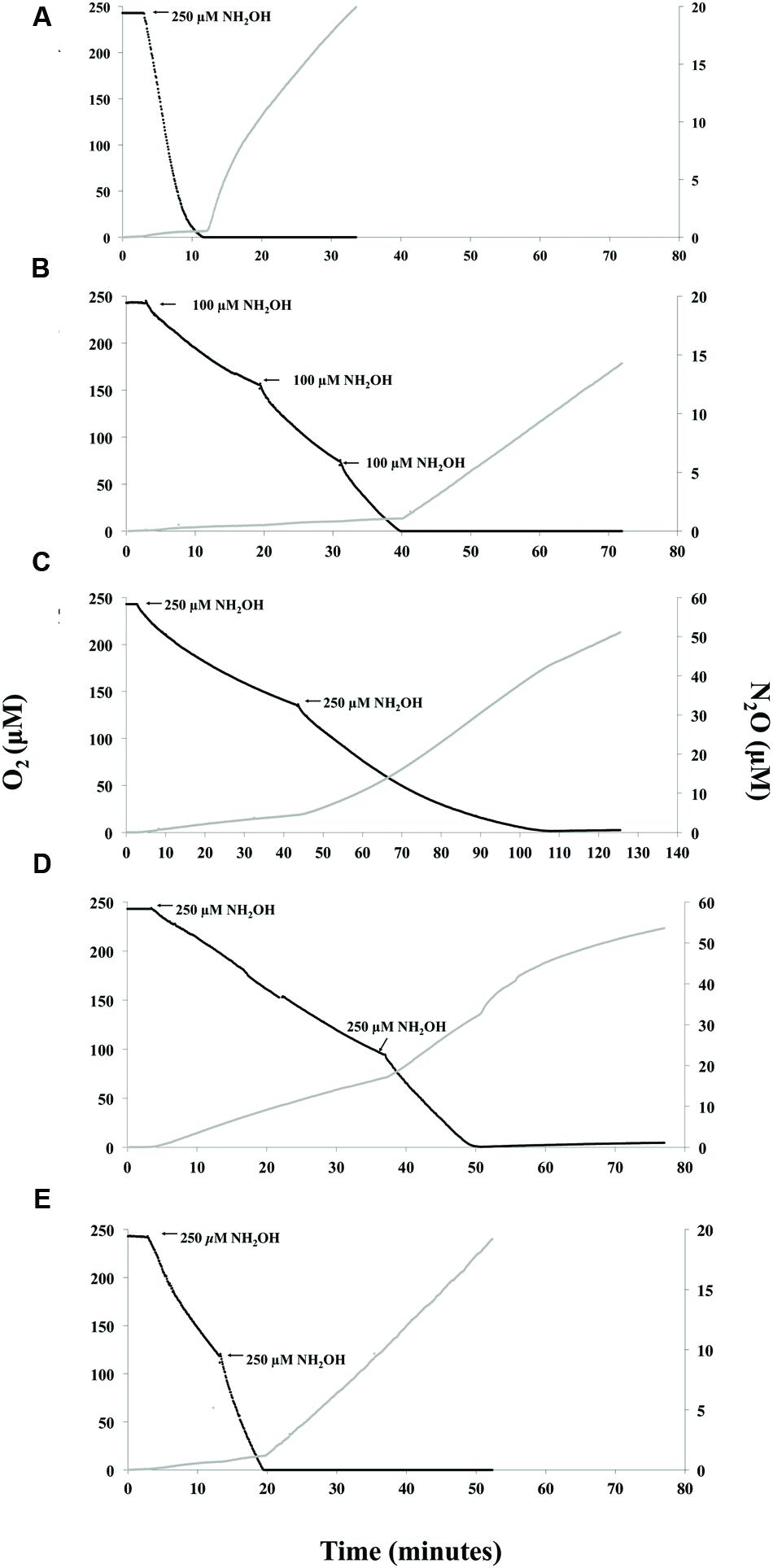
FIGURE 3. Instantaneous measurement of O2 consumption and N2O production during oxidation of NH2OH. N. europaea (A), N. communis (B), Nitrosomonas sp. Is79A3 (C), N. ureae (D), or N. multiformis (E). Note the x-axis for Nitrosomonas sp. Is79A3 differs from the other traces. Nitrosomonas sp. Is79A3 and N. ureae have different y-axes for N2O production.
Following NH3-oxidation, N2O was produced by all strains in the MR-chamber (Figures 2B,D,F,H,J). A greater delay of ca. 3 min in measureable N2O was seen from traces with both N. communis (Figure 2D) and N. ureae (Figure 2H). The lowest concentrations and slowest rates of N2O came from N. communis and N. multiformis (Figures 2D,J; Supplementary Table S1). N. europaea N2O production in the MR-chamber began immediately following O2-depletion and was produced at a rate of 0.47 μM N2O per 1010 cells1 per minute (Figure 2B; Supplementary Table S1). As with NO production, both Nitrosomonas sp. Is79A3 and N. ureae had similar N2O traces with similarly fast rates for N2O production following anoxia (Figures 2F,H; Supplementary Table S1).
With NH2OH as substrate, the majority of N2O in the MR-chamber from N. europaea (Figure 3A), N. communis (Figure 3B), and N. multiformis (Figure 3E) was produced in a linear fashion directly following anoxia suggesting enzymatic reduction of available to N2O and thus nitrifier denitrification. However, in the case of both Nitrosomonas sp. Is79A3 (Figure 3C) and N. ureae (Figure 3D) the majority of N2O was measured during active NH2OH-oxidation with production in both traces slowing upon complete O2-depletion. Furthermore, the quantity of N2O measured from both Nitrosomonas sp. Is79A3 and N. ureae during active NH2OH-oxidation was much greater overall than that produced from any other AOB, suggesting a greater overall release of NO, or other reactive intermediates, during this process (Law et al., 2012) (Figure 3).
It is interesting that N. communis, the only AOB lacking NirK, had very weak non-linear N2O production from NH3, yet strong linear production when NH2OH was provided (Figures 2D and 3B). The linearity of N2O formation with NH2OH as substrate suggests that there is an enzymatic pathway for N2O formation under anoxic conditions, but this pathway is not active when NH3 is provided as substrate. This observation provides insight into the function of unidentified enzymology that links direct NH2OH oxidation to N2O production in N. communis that requires further investigation. Similarly, an N. europaea NirK deficient mutant was also able to reduce to N2O (Cantera and Stein, 2007; Kozlowski et al., 2014), further supporting the presence of alternate, as yet unidentified, NIRs in AOB.
Contribution of AOB to Abiotic N2O
The N2O profiles of both Nitrosomonas sp. Is79A3 and N. ureae post-anoxia (Figures 2F,H) are congruent with a rapid and abundant release of NO (Figures 2E,G) being abiotically reduced to N2O, a characteristic trait observed in the AOA N. viennensis (Kozlowski et al., 2016c). Also in support of an abiotic origin of N2O for both Nitrosomonas sp. Is79 and N. ureae in comparison to the other AOB strains (Figure 3) is the observation that the majority of N2O was measured during active oxidation of NH2OH. Accumulation of NH2OH can lead to NO and N2O production at the active site of the HAO (Hooper and Terry, 1979; Stein, 2011). A high enough concentration of NO will react with components of the HK medium to form N2O as well (Kozlowski et al., 2016c). Interestingly, the lack of NirK did not cause significant production of N2O during active NH2OH-oxidation by N. communis, as shown previously for NirK-deficient N. europaea (Cantera and Stein, 2007), suggesting a different configuration of the ammonia-oxidation pathway among AOB that lack NirK.
In previous control experiments the intermediate NH2OH reacted with heat-killed cell moieties of the AOA, N. viennensis EN76, to produce abiological N2O (Kozlowski et al., 2016c). In the present study, abiotic and heat-killed cell controls were performed to demonstrate if NH2OH could react with either media components or heat-killed cells to produce N2O in the absence of active cellular functioning (Supplementary Figure S1). NH4+-free HK medium + NaNO2 or with heat-killed AOB and addition of 250 μM NH2OH showed that medium + NaNO2 or medium with heat-killed N. europaea, N. communis, and N. multiformis + NH2OH did not facilitate significant measureable N2O (Supplementary Figure S1). However, heat-killed cells of both Nitrosomonas sp. Is79A3 and N. ureae both produced measureable N2O following addition of 250 μM NH2OH. The reactivity of cellular moieties with NH2OH is further evidence of similarities among these oligotrophic AOB and the AOA as heat-killed controls of N. viennensis cells showed similar reactivity with NH2OH in growth medium (Kozlowski et al., 2016c). Taken altogether, the data support that N2O is produced abiotically from Nitrosomonas sp. Is79 and N. ureae similarly to that of the AOA, N. viennensis, likely due to their massive release of NO at anoxia and also the reactivity of their cellular moieties with NH2OH and other medium constituents.
Conclusion
The present study highlights many new findings in the comparative phylogeny and nitrogen oxide metabolism of betaproteobaterial AOB. First, the data support the previous study of N. europaea that a cytochrome c-dependent NOR is required for nitrifier denitrification activity (Kozlowski et al., 2014). Second, the release of NO by the two oligotrophic strains in Cluster 6 of the AOB likely contributes to abiotic N2O production (chemo-denitrification), especially under environmental conditions that facilitate NO or NH2OH release (Jones et al., 2015; Zhu-Barker et al., 2015). This observation is congruent with the physiology of the oligotrophic AOA that lack NOR (Kozlowski et al., 2016c). Third, this study showcases the utility of comparative physiological studies on pure cultures of ammonia-oxidizers to characterize the diversity of mechanisms for NOx production and ultimately for N2O release to the environment.
Author Contributions
JK and LS conceived the project; JK designed and performed all experiments, KK performed a PhyloPhlAn analysis and created the phylogenetic tree; JK, KK, and LS analyzed the data, JK and LS wrote and edited the manuscript.
Funding
Support for this research was provided by Alberta Innovates Technology Futures (JK and KK) and by a Discovery Grant from the Natural Sciences and Engineering Research Council of Canada (LS).
Conflict of Interest Statement
The authors declare that the research was conducted in the absence of any commercial or financial relationships that could be construed as a potential conflict of interest.
Acknowledgment
The authors would like to thank Dr. Annette Bollmann at Miami University, Oxford OH for providing cultures of Nitrosomonas sp. Is79A3.
Supplementary Material
The Supplementary Material for this article can be found online at: http://journal.frontiersin.org/article/10.3389/fmicb.2016.01090
Footnotes
References
Arciero, D. M., Pierce, B. S., Hendrich, M. P., and Hooper, A. B. (2002). Nitrosocyanin, a red cupredoxin-like protein from Nitrosomonas europaea. Biochemistry 41, 1703–1709. doi: 10.1021/bi015908w
Arp, D. J., Chain, P. S. G., and Klotz, M. G. (2007). The impact of genome analyses on our understanding of ammonia-oxidizing bacteria. Annu. Rev. Microbiol. 61, 503–528. doi: 10.1146/annurev.micro.61.080706.093449
Bartossek, R., Nicol, G. W., Lanzen, A., Klenk, H.-P., and Schleper, C. (2010). Homologues of nitrite reductases in ammonia-oxidizing archaea: diversity and genomic context. Environ. Microbiol. 12, 1075–1088. doi: 10.1111/j.1462-2920.2010.02153.x
Beaumont, H. J. E., Hommes, N. G., Sayavedra-Soto, L. A., Arp, D. J., Arciero, D. M., Hooper, A. B., et al. (2002). Nitrite reductase of Nitrosomonas europaea is not essential for production of gaseous nitrogen oxides and confers tolerance to nitrite. J. Bacteriol. 184, 2557–2560. doi: 10.1128/JB.184.9.2557-2560.2002
Beaumont, H. J. E., van Schooten, B., Lens, S. I., Westerhoff, H. V., and van Spanning, R. J. M. (2004). Nitrosomonas europaea expresses a nitric oxide reductase during nitrification. J. Bacteriol. 186, 4417–4421. doi: 10.1128/JB.186.13.4417-4421.2004
Berube, P. M., and Stahl, D. A. (2012). The divergent AmoC3 subunit of ammonia monooxygenase functions as part of a stress response system in Nitrosomonas europaea. J. Bacteriol. 194, 3448–3456. doi: 10.1128/JB.00133-12
Bollmann, A., French, E., and Laanbroek, H. J. (2011). “Isolation, cultivation, and characterization of ammonia-oxidizing bacteria and archaea adapted to low ammonium concentrations,” in Methods in Enzymology, ed. M. G. Klotz (San Diego, CA: Academic Press), 55–88. doi: 10.1016/B978-0-12-381294-0.00003-1
Bollmann, A., Sedlacek, C., Norton, J., Norton, J. M., Laanbroek, H. J., Suwa, Y., et al. (2013). Complete genome sequence of Nitrosomonas sp. Is79, an ammonia oxidizing bacterium adapted to low ammonium concentrations. Stand. Genom. Sci. 7, 469–482. doi: 10.4056/sigs.3517166
Campbell, M. A., Chain, P. S. G., Dang, H., Sheikh El, A. F., Norton, J. M., Ward, N. L., et al. (2011). Nitrosococcus watsonii sp. nov., a new species of marine obligate ammonia-oxidizing bacteria that is not omnipresent in the world’s oceans: calls to validate the names ‘Nitrosococcus halophilus’ and “Nitrosomonas mobilis.” FEMS Microbiol. Ecol. 76, 39–48. doi: 10.1111/j.1574-6941.2010.01027.x
Cantera, J. J. L., and Stein, L. Y. (2007). Role of nitrite reductase in the ammonia-oxidizing pathway of Nitrosomonas europaea. Arch. Microbiol. 188, 349–354. doi: 10.1007/s00203-007-0255-4
Chain, P., Lamerdin, J., Larimer, F., Regala, W., Lao, V., Land, M., et al. (2003). Complete genome sequence of the ammonia-oxidizing bacterium and obligate chemolithoautotroph Nitrosomonas europaea. J. Bacteriol. 185, 2759–2773. doi: 10.1128/JB.185.9.2759-2773.2003
Dundee, L., and Hopkins, D. W. (2001). Different sensitivities to oxygen of nitrous oxide production by Nitrosomonas europaea and Nitrosolobus multiformis. Soil Biol. Biochem. 33, 1563–1565. doi: 10.1016/S0038-0717(01)00059-1
Elmore, B. O., Bergmann, D. J., Klotz, M. G., and Hooper, A. B. (2007). Cytochromes P460 and c’-beta; A new family of high-spin cytochromes c. FEBS Lett. 581, 911–916. doi: 10.1016/j.febslet.2007.01.068
Garcia, J. C., Urakawa, H., Le, V. Q., Stein, L. Y., Klotz, M. G., and Nielsen, J. L. (2013). Draft genome sequence of Nitrosospira sp. Strain APG3, a psychrotolerant ammonia-oxidizing bacterium isolated from sandy lake sediment. Genome Announc. 1, e00930-13. doi: 10.1128/genomeA.00930-13
Guindon, S., Dufayard, J.-F., Lefort, V., Anisimova, M., Hordijk, W., and Gascuel, O. (2010). New algorithms and methods to estimate maximum-likelihood phylogenies: assessing the performance of PhyML 3.0. Syst. Biol. 59, 307–321. doi: 10.1093/sysbio/syq010
Hollibaugh, J. T., Gifford, S., Sharma, S., Bano, N., and Moran, M. A. (2011). Metatranscriptomic analysis of ammonia-oxidizing organisms in an estuarine bacterioplankton assemblage. ISME J. 5, 866–878. doi: 10.1038/ismej.2010.172
Hommes, N. G., Sayavedra-Soto, L. A., and Arp, D. J. (2002). The roles of the three gene copies encoding hydroxylamine oxidoreductase in Nitrosomonas europaea. Arch. Microbiol. 178, 471–476. doi: 10.1007/s00203-002-0477-4
Hooper, A. B., and Terry, K. R. (1979). Hydroxylamine oxidoreductase of Nitrosomonas: production of nitric oxide from hydroxylamine. Biochim. Biophys. Acta 571, 12–20. doi: 10.1016/0005-2744(79)90220-1
Jia, Z., and Conrad, R. (2009). Bacteria rather than Archaea dominate microbial ammonia oxidation in an agricultural soil. Environ. Microbiol. 11, 1658–1671. doi: 10.1111/j.1462-2920.2009.01891.x
Jones, L. C., Peters, B., Pacheco, J. S. L., Casciotti, K. L., and Fendorf, S. (2015). Stable isotopes and iron oxide mineral products as markers of chemodenitrification. Environ. Sci. Technol. 49, 3444–3452. doi: 10.1021/es504862x
Ke, X., Lu, W., and Conrad, R. (2015). High oxygen concentration increases the abundance and activity of bacterial rather than archaeal nitrifiers in rice field soil. Microb. Ecol. 70, 961–970. doi: 10.1007/s00248-015-0633-4
Kester, R. A., De Boer, W., and Laanbroek, H. J. (1997). Production of NO and N2O by pure cultures of nitrifying and denitrifying bacteria during changes in aeration. Appl. Environ. Microbiol. 63, 3872–3877.
Klotz, M. G., Arp, D. J., Chain, P. S. G., El-Sheikh, A. F., Hauser, L. J., Hommes, N. G., et al. (2006). Complete genome sequence of the marine, chemolithoautotrophic, ammonia-oxidizing bacterium Nitrosococcus oceani ATCC 19707. Appl. Environ. Microbiol. 72, 6299–6315. doi: 10.1128/AEM.00463-06
Klotz, M. G., and Stein, L. Y. (2011). “Genomics of ammonia-oxidizing bacteria and insights to their evolution,” in Nitrification, eds B. B. Ward, D. J. Arp, and M. G. Klotz (Washington, DC: ASM Press), 57–93.
Kool, D. M., Dolfing, J., Wrage, N., and Van Groenigen, J. W. (2011). Nitrifier denitrification as a distinct and significant source of nitrous oxide from soil. Soil Biol. Biochem. 43, 174–178. doi: 10.1016/j.soilbio.2010.09.030
Kozlowski, J. A., Kits, K. D., and Stein, L. Y. (2016a). Complete genome sequence of Nitrosomonas ureae Strain Nm10, an oligotrophic group 6a Nitrosomonad. Genome Announc. 4:e00094-16.
Kozlowski, J. A., Kits, K. D., and Stein, L. Y. (2016b). Genome sequence of Nitrosomonas communis Strain Nm2, a mesophilic ammonia-oxidizing bacterium isolated from mediterranean soil. Genome Announc. 4, e1541–e1515. doi: 10.1128/genomeA.01541-15
Kozlowski, J. A., Price, J., and Stein, L. Y. (2014). Revision of N2O-producing pathways in the ammonia-oxidizing bacterium. Nitrosomonas europaea ATCC 19718. Appl. Environ. Microbiol. 80, 4930–4935. doi: 10.1128/AEM.01061-14
Kozlowski, J. A., Stieglmeier, M., Schleper, C., Klotz, M. G., and Stein, L. Y. (2016c). Pathways and key intermediates required for obligate aerobic ammonia-dependent chemolithotrophy in bacteria and Thaumarchaeota. ISME J. doi: 10.1038/ismej.2016.2 [Epub ahead of print].
Krümmel, A., and Harms, H. (1982). Effect of organic matter on growth and cell yield of ammonia-oxidizing bacteria. Arch. Microbiol. 133, 50–54. doi: 10.1007/BF00943769
Law, Y., Ye, L., Pan, Y., and Yuan, Z. (2012). Nitrous oxide emissions from wastewater treatment processes. Philos. Trans. R. Soc. Lond. B. Biol. Sci. 367, 1265–1277. doi: 10.1098/rstb.2011.0317
Martens-Habbena, W., Berube, P. M., Urakawa, H., de la Torre, J. R., and Stahl, D. A. (2009). Ammonia oxidation kinetics determine niche separation of nitrifying Archaea and Bacteria. Nature 461, 976–979. doi: 10.1038/nature08465
Martens-Habbena, W., Qin, W., Horak, R. E. A., Urakawa, H., Schauer, A. J., Moffett, J. W., et al. (2015). The production of nitric oxide by marine ammonia-oxidizing archaea and inhibition of archaeal ammonia oxidation by a nitric oxide scavenger. Environ. Microbiol. 17, 2261–2274. doi: 10.1111/1462-2920.12677
Norton, J. M. (2011). “Diversity and environmental distribution of ammonia-oxidizing bacteria,” in Nitrification, eds B. B. Ward, D. J. Arp, and M. G. Klotz (Washington, DC: ASM Press), 39–56. doi: 10.1128/9781555817145
Norton, J. M., Klotz, M. G., Stein, L. Y., Arp, D. J., Bottomley, P. J., Chain, P. S. G., et al. (2008). Complete genome sequence of Nitrosospira multiformis, an ammonia-oxidizing bacterium from the soil environment. Appl. Environ. Microbiol. 74, 3559–3572. doi: 10.1128/AEM.02722-07
Poth, M., and Focht, D. D. (1985). 15N kinetic analysis of N2O production by Nitrosomonas europaea: an examination of nitrifier denitrification. Appl. Environ. Microbiol. 49, 1134–1141.
Prosser, J. I., Head, I. M., and Stein, L. Y. (2014). “The family nitrosomonadaceae,” in The Prokaryotes, eds M. Dworkinm, S. Falkow, E. Rosenberg, K.-H. Schleifer, and E. Stackebrandt (Berlin: Springer), 901–918.
Radax, R., Rattei, T., Lanzen, A., Bayer, C., Rapp, H. T., Urich, T., et al. (2012). Metatranscriptomics of the marine sponge Geodia barretti: tackling phylogeny and function of its microbial community. Environ. Microbiol. 14, 1308–1324. doi: 10.1111/j.1462-2920.2012.02714.x
Sayavedra-Soto, L. A., and Arp, D. J. (2011). “Ammonia-oxidizing bacteria: their biochemistry and molecular biology,” in Nitrification, eds B. B. Ward, D. J. Arp, and M. G. Klotz (Washington, DC: ASM Press), 11–38.
Segata, N., Börnigen, D., Morgan, X. C., and Huttenhower, C. (2013). PhyloPhlAn is a new method for improved phylogenetic and taxonomic placement of microbes. Nat. Commun. 4:2304. doi: 10.1038/ncomms3304
Shaw, L. J., Nicol, G. W., Smith, Z., Fear, J., Prosser, J. I., and Baggs, E. M. (2006). Nitrosospira spp. can produce nitrous oxide via a nitrifier denitrification pathway. Environ. Microbiol. 8, 214–222. doi: 10.1111/j.1462-2920.2005.00882.x
Stahl, D. A., and de la Torre, J. R. (2012). Physiology and diversity of ammonia-oxidizing Archaea. Annu. Rev. Microbiol. 66, 83–101. doi: 10.1146/annurev-micro-092611-150128
Stein, L. Y. (2011). “Surveying N2O-producing pathways in bacteria,” in Methods in Enzymology, Vol. 486, ed. M. G. Klotz (San Diego, CA: Academic Press), 131–152.
Stein, L. Y., Arp, D. J., Berube, P. M., Chain, P. S. G., Hauser, L., Jetten, M. S. M., et al. (2007). Whole-genome analysis of the ammonia-oxidizing bacterium, Nitrosomonas eutropha C91: implications for niche adaptation. Environ. Microbiol. 9, 2993–3007. doi: 10.1111/j.1462-2920.2007.01409.x
Stein, L. Y., Sayavedra-Soto, L. A., Hommes, N. G., and Arp, D. J. (2000). Differential regulation of amoA and amoB gene copies in Nitrosomonas europaea. FEMS Microbiol. Lett. 192, 163–168. doi: 10.1111/j.1574-6968.2000.tb09376.x
Stein, L. Y., and Yung, Y. L. (2003). Production, isotopic composition, and atmospheric fate of biologically produced nitrous oxide. Annu. Rev. Earth Planet. Sci. 31, 329–356. doi: 10.1146/annurev.earth.31.110502.080901
Suwa, Y., Yuichi, S., Norton, J. M., Bollmann, A., Klotz, M. G., Stein, L. Y., et al. (2011). Genome sequence of Nitrosomonas sp. strain AL212, an ammonia-oxidizing bacterium sensitive to high levels of ammonia. J. Bacteriol. 193, 5047–5048. doi: 10.1128/JB.05521-11
Urakawa, H., Garcia, J. C., Nielsen, J. L., Le, V. Q., Kozlowski, J. A., Stein, L. Y., et al. (2014). Nitrosospira lacus sp. nov., a psychrotolerant ammonia-oxidizing bacterium from sandy lake sediment. Int. J. Syst. Evol. Microbiol. 65, 242–250. doi: 10.1099/ijs.0.070789-0
Wrage, N., Velthof, G. L., Oenema, O., and Laanbroek, H. J. (2004). Acetylene and oxygen as inhibitors of nitrous oxide production in Nitrosomonas europaea and Nitrosospira briensis: a cautionary tale. FEMS Microbiol. Ecol. 47, 13–18. doi: 10.1016/S0168-6496(03)00220-4
Yu, R., and Chandran, K. (2010). Strategies of Nitrosomonas europaea 19718 to counter low dissolved oxygen and high nitrite concentrations. BMC Microbiol. 10:70. doi: 10.1186/1471-2180-10-70
Yu, R., Kampschreur, M. J., Loosdrecht, M. C. M. V., and Chandran, K. (2010). Mechanisms and specific directionality of autotrophic nitrous oxide and nitric oxide generation during transient anoxia. Environ. Sci. Technol. 44, 1313–1319. doi: 10.1021/es902794a
Zhu, X., Burger, M., Doane, T. A., and Horwath, W. R. (2013). Ammonia oxidation pathways and nitrifier denitrification are significant sources of N2O and NO under low oxygen availability. Proc. Natl. Acad. Sci. U.S.A. 110, 6328–6333. doi: 10.1073/pnas.1219993110
Keywords: nitrogen oxides, nitrifier denitrification, ammonia-oxidizers, Nitrosomonas, Nitrosospira, nitrous oxide, nitric oxide, chemodenitrification
Citation: Kozlowski JA, Kits KD and Stein LY (2016) Comparison of Nitrogen Oxide Metabolism among Diverse Ammonia-Oxidizing Bacteria. Front. Microbiol. 7:1090. doi: 10.3389/fmicb.2016.01090
Received: 24 May 2016; Accepted: 29 June 2016;
Published: 12 July 2016.
Edited by:
Manuel Kleiner, University of Calgary, CanadaReviewed by:
James Moir, University of York, UKJulio Perez, Delft University of Technology, Netherlands
Copyright © 2016 Kozlowski, Kits and Stein. This is an open-access article distributed under the terms of the Creative Commons Attribution License (CC BY). The use, distribution or reproduction in other forums is permitted, provided the original author(s) or licensor are credited and that the original publication in this journal is cited, in accordance with accepted academic practice. No use, distribution or reproduction is permitted which does not comply with these terms.
*Correspondence: Lisa Y. Stein, bGlzYS5zdGVpbkB1YWxiZXJ0YS5jYQ==