- 1Division of Geological and Planetary Sciences, California Institute of Technology, Pasadena, CA, USA
- 2Division of Biology and Bioengineering, California Institute of Technology, Pasadena, CA, USA
Hydrothermal vents are an important contributor to marine biogeochemistry, producing large volumes of reduced fluids, gasses, and metals and housing unique, productive microbial and animal communities fueled by chemosynthesis. Methane is a common constituent of hydrothermal vent fluid and is frequently consumed at vent sites by methanotrophic bacteria that serve to control escape of this greenhouse gas into the atmosphere. Despite their ecological and geochemical importance, little is known about the ecophysiology of uncultured hydrothermal vent-associated methanotrophic bacteria. Using metagenomic binning techniques, we recovered and analyzed a near-complete genome from a novel gammaproteobacterial methanotroph (B42) associated with a white smoker chimney in the Southern Lau basin. B42 was the dominant methanotroph in the community, at ∼80x coverage, with only four others detected in the metagenome, all on low coverage contigs (7x–12x). Phylogenetic placement of B42 showed it is a member of the Methylothermaceae, a family currently represented by only one sequenced genome. Metabolic inferences based on the presence of known pathways in the genome showed that B42 possesses a branched respiratory chain with A- and B-family heme copper oxidases, cytochrome bd oxidase and a partial denitrification pathway. These genes could allow B42 to respire over a wide range of oxygen concentrations within the highly dynamic vent environment. Phylogenies of the denitrification genes revealed they are the result of separate horizontal gene transfer from other Proteobacteria and suggest that denitrification is a selective advantage in conditions where extremely low oxygen concentrations require all oxygen to be used for methane activation.
Introduction
Deep-sea hydrothermal vent systems are a significant contributor to the marine methane cycle, considered both a global source and sink of this potent greenhouse gas. Hydrothermal vent systems are common along mid-ocean ridges, back-arc spreading centers, and other subaqueous divergent plate boundaries (Beaulieu et al., 2013) where concentration of methane in vent fluids is ∼100 times higher than the surrounding ocean waters (Welhan and Craig, 1979, 1983). Vents vary in chemical composition, but plumes are often enriched in H2S, CH4, H2, Fe2+, and Mn2+, which chemosynthetic microbes utilize as energy resources, and also emit trace elements such as copper, zinc, iron, cobalt, and chromium (Dick et al., 2013; Sylvan et al., 2013). As these reduced compounds mix with the open ocean, they oxidize to form chimney structures, or alternatively disperse throughout the water column to provide an important source of trace elements and nutrients for the broader marine system (Elderfield and Schultz, 1996; Tagliabue et al., 2010). Methane emitted from vents provides a significant source of energy for microbial communities (Dick et al., 2013) and is consumed almost entirely by methanotrophic microbes living both near the seafloor and in the water column (Lesniewski et al., 2012). Despite their importance in the global methane efflux, the diversity, distribution, and detailed characterization of methane-oxidizing microorganisms from hydrothermal vent environments have not been significantly characterized.
Methanotrophic bacteria utilize methane to provide cellular carbon and energy through oxidation via methane monooxygenase (MMO). Carbon assimilation proceeds by either the ribulose monophosphate (RuMP) pathway found in Type I methanotrophs, or the serine pathway found in Type II methanotrophs (Trotsenko and Murrell, 2008). To date all vent-associated methanotrophs are type I, consistent with reports that type II methanotrophs are not identified in marine systems (Lidstrom, 1988). Using metagenomic and transcriptome sequencing, as well as 16S rRNA or MMO gene assays (Hirayama et al., 2007; Lesniewski et al., 2012; Dick et al., 2013; Li et al., 2014; Anantharaman et al., 2015), Type I methanotrophs belonging to the Gammaproteobacteria have been detected at hydrothermal vent sites such as the Lau and Guayams Basin (Tavormina et al., 2010; Dick et al., 2013; Sylvan et al., 2013), off of the Islands of Japan (Hirayama et al., 2007, 2013, 2014) at the Mid-Okinawa Trough, the Trans-Atlantic Geotraverse (Elsaied et al., 2004), and the Rainbow Vent fields (Nercessian et al., 2005). Though these experiments have provided estimates of the abundance and diversity of aerobic methane-oxidizing organisms at hydrothermal vent sites, complete methanotroph genomes from deep-sea hydrothermal vents have yet to be sequenced and interpreted. Such information can contribute to a more complete understanding of the functional capacity of these chemosynthesizers at hydrothermal vent sites.
The role of aerobic methanotrophs in global nitrogen cycling is also of growing interest. Some methanotrophs have been shown to contribute to nitrogen fixation (Auman et al., 2001) and non-specific oxidation of ammonium by MMO is also known to occur, resulting in inadvertent nitrification by methanotrophs (Bédard and Knowles, 1989; Hommes et al., 2001). This oxidative metabolism produces hydroxylamine, a toxic intermediate that can be converted to nitrite via hydroxylamine oxidoreductase (Campbell et al., 2011). Many aerobic methylotrophs possess pathways for assimilatory nitrate reduction, while dissimilatory nitrate reduction pathways are often absent (Stein and Klotz, 2011).
Recently, additional overlap between the methane and nitrogen cycling has been demonstrated. Denitrifying microbes have been implicated in nitrate reduction coupled to methane oxidation using either the reverse methanogenesis pathway in Candidatus Methanoperedens nitroreducens (Haroon et al., 2013), a novel intra-aerobic methanotrophic pathway in Candidatus Methylomirabilis oxyfera (Ettwig et al., 2010), as well as denitrification-coupled aerobic methanotrophy in bacteria in dysoxic conditions (Kits et al., 2015). Denitrification-coupled aerobic methanotrophy in dysoxic conditions has been demonstrated in the gammaproteobacterium Methylomonas denitrificans, which requires trace amounts of molecular oxygen to activate methane but is capable of using oxidized nitrogen as a terminal electron acceptor (Kits et al., 2015).
In this study, metagenomic sequencing was used to sample the microbial community and associated metabolic potential with a white smoker chimney from the Tu’i Malila vent field in the Lau Basin. From this metagenomic dataset, we successfully reconstructed a draft genome from an aerobic methanotroph that, like M. denitrificans, is capable of denitirfication-coupled aerobic methanotrophy.
Materials and Methods
Site Description and Sample Collection
The Lau Basin in the western Pacific is a back-arc basin that consists of several ridge segments arranged approximately North-South, aligned subparallel to the convergent Pacific-Australian plate margin to the east. A large (∼15 cm) piece of a white smoker chimney, sample #2044C, was collected during cruise tuim06mv on Dive J2_144 (May 21, 2005) from the Tu’i Malila vent field (176° 34.060′ W, 21°59.350′ S; depth 1876 m), located on the Valu Fa Ridge at the southern end of the Lau Basin in the western Pacific Ocean. Fluids emanating from this white smoker were measured at 260°C, with 10–12°C temperatures measured on the exterior surface of the chimney where #2044C was sampled. Upon recovery shipboard, a sterile chisel and hammer were used to subsample the exterior chimney material, followed by storage at -80°C until genomic DNA extraction in 2015.
Mineralogical analysis of sample #2044C by XRD (B. Harrison personal communication) indicates a composition dominated by sphalerite, anhydrite, chalcopyrite (copper iron sulfide), and galena (lead sulfide) with lower contributions of wurtzite (zinc iron sulfide), barite (barium sulfate), pyrite (iron sulfide), and molybdenum.
Metagenomic Sequencing, Assembly, Binning and Annotation
DNA from sample #2044C was extracted using the MO BIO PowerSoil® DNA isolation kit, following the manufacturer’s instructions, and sequenced using the Illumina HiSeq2500 platform. Raw metagenomic sequencing reads were assembled using megahit 0.1.2 (Li et al., 2015) using default parameters. Contig binning was performed using emergent self-organizing maps using the tetranucleotide frequencies of contigs greater than 2 kbp in size. Tetranucleotide frequencies for contigs were generated using bioruby-kmer_counter 0.1.2 (https://github.com/wwood/bioruby-kmer_counter) using the default parameters. ESOM maps were generated using the databionics-ESOM package (Ultsch and Moerchen, 2005) using the parameters reported by Dick et al. (2009); genome bins were classified manually on the ESOM map. Extracted genome bins were validated using checkM 0.9.7 (Parks et al., 2015). The B42 draft genome was improved by reassembly using spades 3.5.0 (Bankevich et al., 2012). Reads from contigs of the original assembly of the bin and reads that were found in contigs that linked through paired read information were extracted and used as the input to spades. Manual improvement to correct mis-assemblies and join contigs was performed on the spades scaffolds. Annotation of the B42 genome was performed with RAST (Aziz et al., 2008; Overbeek et al., 2014).
Phylogenetic Analysis
Gene phylogenies for NirK and NarG were constructed by identifying genes in the IMG database (Markowitz et al., 2014) that contained sequence similarity using the BLASTp algorithm using a cutoff score of 1e-5. Up to 500 of the best hits were used to construct each phylogenetic tree. The PmoA tree was constructed by selected sequences that were above a cutoff score of 1e-105 against the NCBI NR database. Four additional sequences obtained from the metagenomic assembly were also included in the phylogeny of the PmoA tree. Sequences were aligned with Muscle 3.8.31 (Edgar, 2004), using default parameters. Trees were calculated using RAxML 8.1.7 (Stamatakis, 2014) using the following parameters: -f a -k -x 483735 -p 54927 -N 100 -T 16 -m PROTGAMMAWAG.
Data Availability
The draft genome sequence is available in the Integrated Microbial Genomes (IMG) database under the accession 2623620619.
Results and Discussion
Genome Reconstruction and Phylogeny
Metagenomic sequencing, assembly and binning resulted in a number of high quality genome bins from the hydrothermal vent metagenome. Analysis of the metabolisms of these genome bins identified only a single draft genome that contained the enzymes for aerobic methane oxidation. This genome bin, referred to hereafter as B42, was assembled into 39 scaffolds containing 3.04 Mbp of total sequence at an average coverage of 80x. B42 was estimated to be 97% complete and 1% contaminated based on the presence of single-copy marker genes. The genome bin contained a single complete rRNA operon that allowed for phylogenetic classification as a member of the Methylothermaceae, with the closest cultured isolate being Methylohalobius crimeensis (Figure 1). Phylogenetic placement of the pmoA gene is consistent with the 16S rDNA tree indicating phylogenetic affiliation within the Methylothermaceae family (Figure 2). A search for pmoA in the whole metagenome returned four additional copies, three of which were phylogenetically related to the Methylothermaceae, while the fourth copy was most closely related to Methylocaldum (Figure 2). These additional pmoA genes were on low coverage contigs (7x – 12x) that indicates that while other methanotrophs are present, B42 is the most abundant and presumably the most important for methane oxidation at this site. The average amino acid identity (AAI) between B42 and all other genome sequences of gammaproteobacterial methylotrophs was below 70%, with the exception of Methylohalobius crimeensis (Figure 3). This value is lower than the AAI of genomes from other genera such as Methylobacter (AAI: 70–95%) or Methylomonas (AAI: 75–90%) and suggests that although B42 is most related to Methylohalobius crimeensis it may not be a member of the same genus (Figure 3).
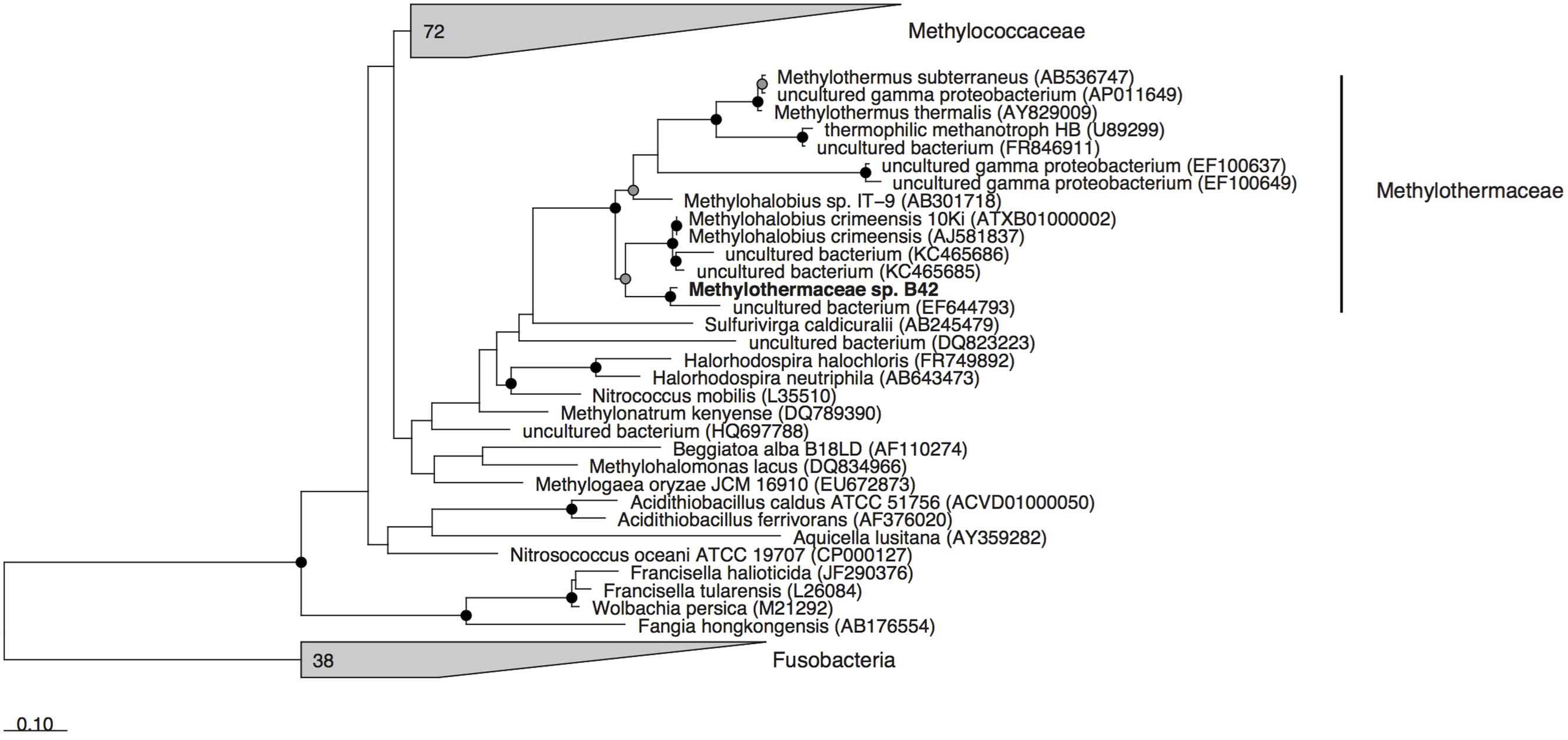
FIGURE 1. Maximum likelihood phylogenetic tree of the 16S rRNA gene from cultured Methanococcales and associated non-methanotrophic Gammaproteobacteria, and some related environmental clones. Gray wedges indicate monophyletic groups of sequences. Cultured isolated from the Fusobacteria were used as the outgroup. Nodes with greater than 70 or 90% bootstrap support are indicated with a gray or black circle, respectively. Scale bar indicates substitutions per site.
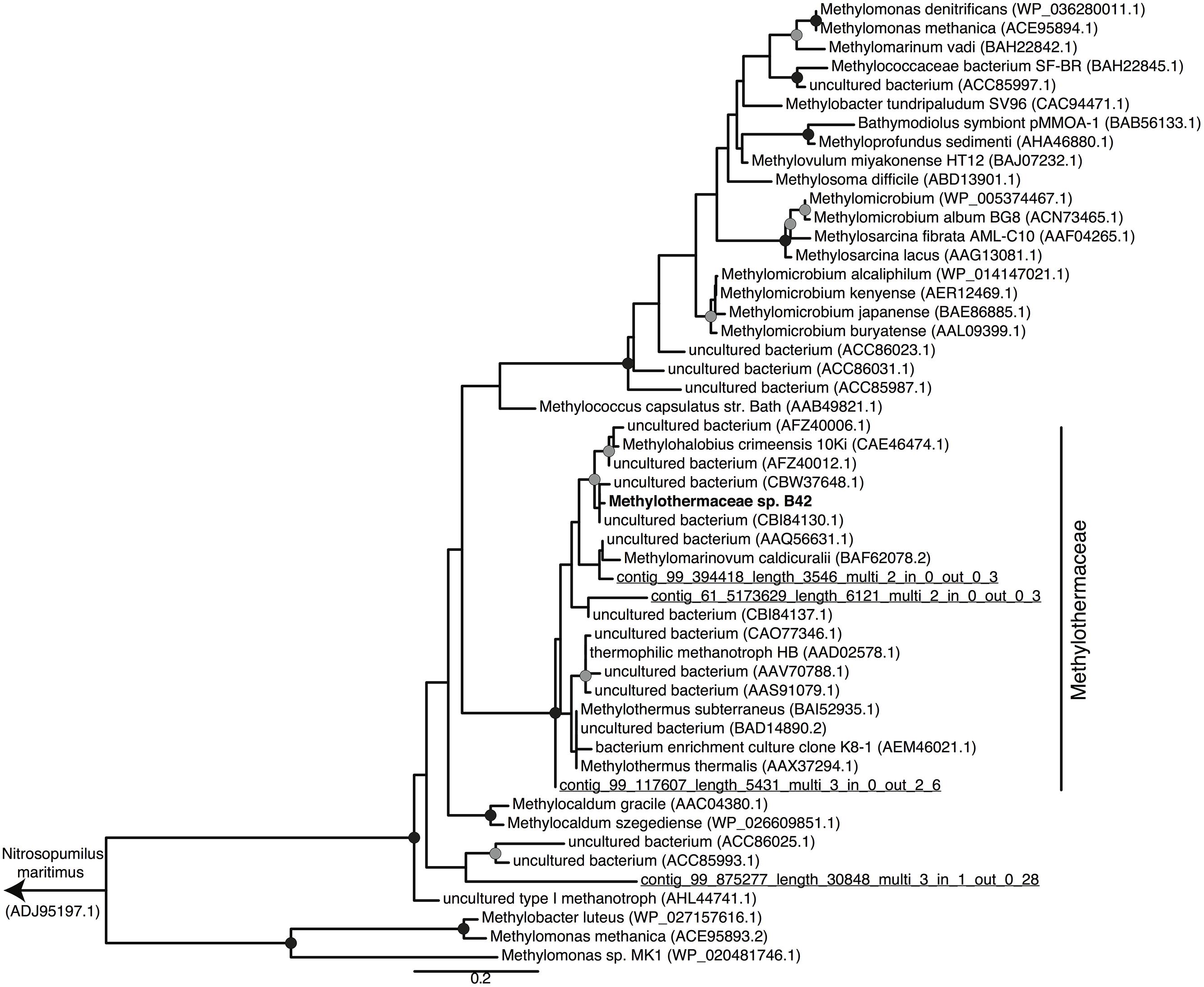
FIGURE 2. Maximum likelihood phylogenetic tree of PmoA from cultured Methanococcales, and some related environmental clones. PmoA sequences recovered from the metagenome are underlined and labeled with their contig names. The Nitrosopumilus maritimus ammonia monooxygenase subunit A protein was used as the outgroup of the tree. Nodes with greater than 70 or 90% bootstrap support are indicated with a gray or black circle, respectively. Scale bar indicates substitutions per site.
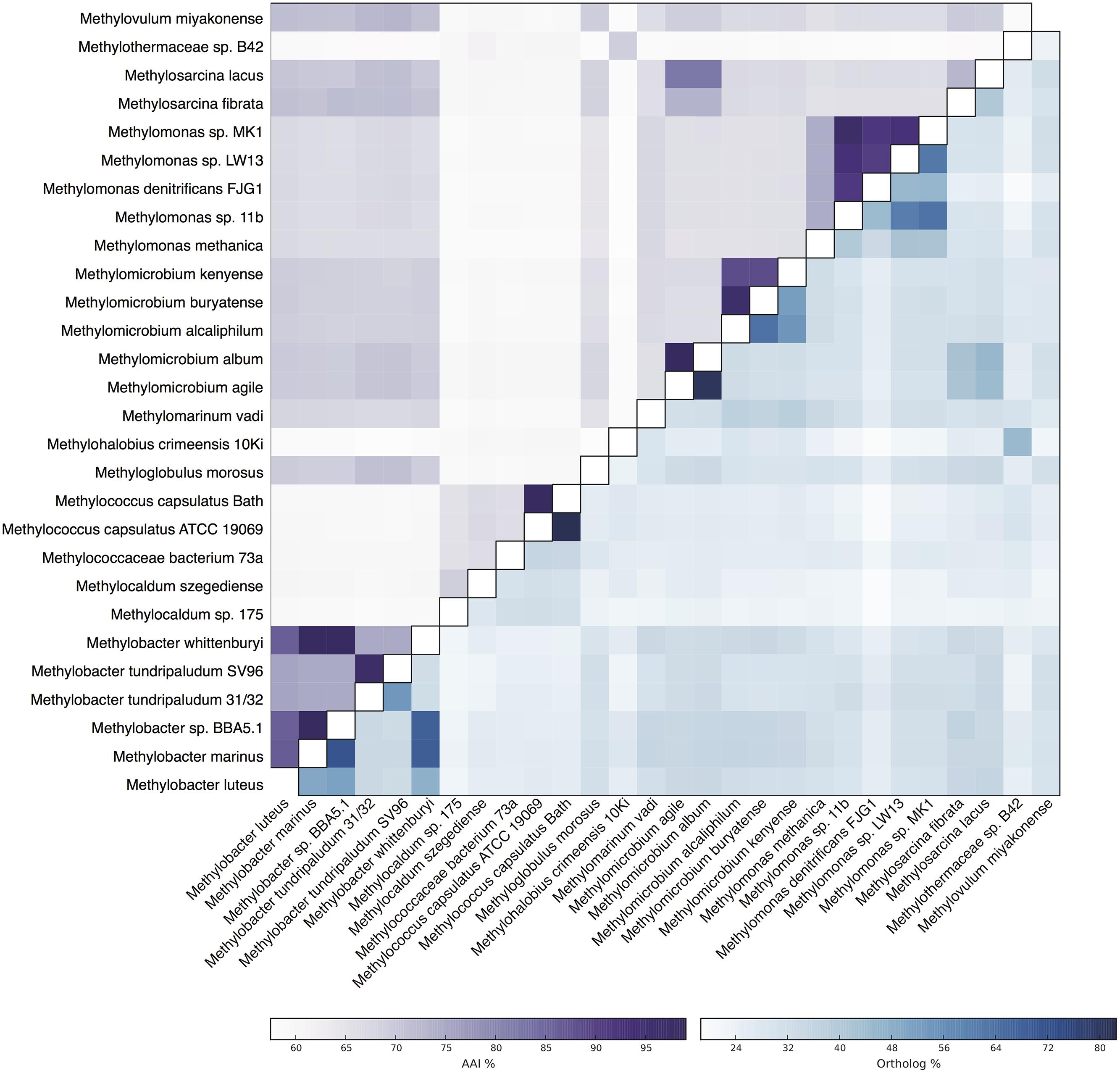
FIGURE 3. Heatmap of genome relatedness between B42 and other gammaproteobacterial methanotrophs. The average amino acid identity (AAI) between homologous proteins between pairs of genomes is shown in the upper triangle. The percentage of total proteins that are homologous is shown in the lower triangle.
Carbon Metabolism
B42 contained all of the necessary RuMP pathway genes (type I methanotroph) for assimilation of methane-derived carbon that has been found in all previously sequenced gammaproteobacterial methanotrophs (Hanson and Hanson, 1996). Notably, this includes all three subunits of the particulate methane monoxygenase (pMMO) that were organized in an operon pmoCAB, which is a consistent synteny to other type I methanotrophs (Trotsenko and Murrell, 2008). The B42 genome contained an extra pmoC subunit separate from the pMMO operon. Previous hypotheses have suggested that additional pmoC genes play a distinct role in the pMMO, possibly to activate pMMO (Stolyar et al., 1999), it may be a neutral duplication, or it may be involved in ammonia oxidation, which is closely related to methane oxidation (Hanson and Hanson, 1996). Since the two copies of pmoC are more closely related to each other than to pmoC from other Methylothermaceae, it likely originated from a recent duplication event. B42 does not appear to possess a homolog of the soluble methane monoxygenase, sMMO, which is found in only some of the gammaproteobacterial methanotrophs (Hanson and Hanson, 1996).
The pMMO catalyzes the production of methanol, which is further oxidized to formaldehyde by a periplasmic methanol dehydrogenase (MDH; Figure 4). MDH transfers electrons through cytochrome cL and cytochrome cH to the terminal heme copper oxidase. B42 utilizes the methenyltetrahydromethanopterin-linked pathway to oxidize formaldehyde to formate. Formate is oxidized to carbon dioxide by NAD-dependent formate dehydrogenase (FDH), with four isoenzymes present in B42 that have high sequence similarity to other members of the Methylococcaceae. Formaldehyde can then be assimilated into biomass using the RuMP pathway (Figure 4).
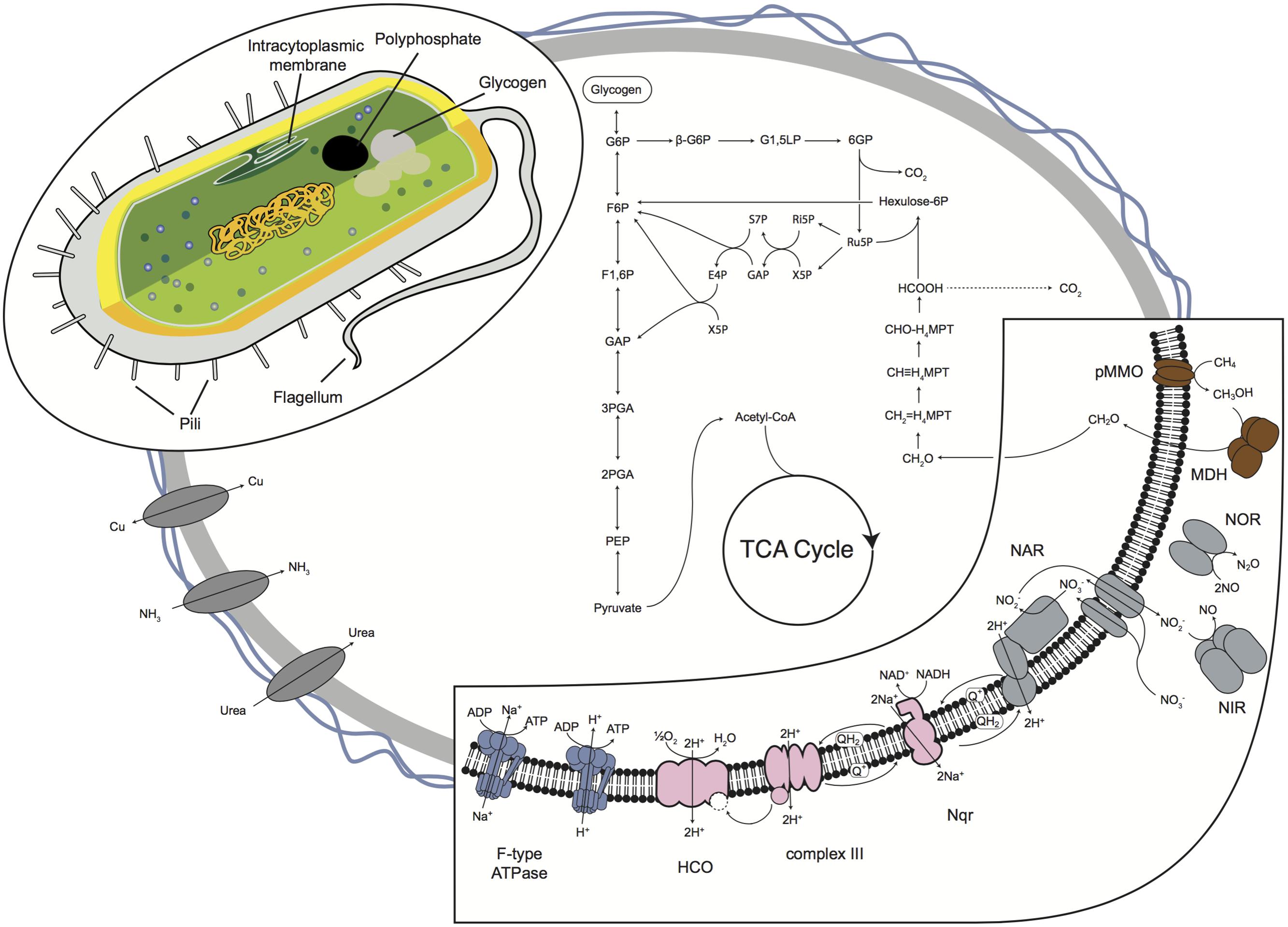
FIGURE 4. Model predictions of the central metabolism inferred from the B42 genome sequence. Important energy generating reactions are shown expanded on the lower left corner. Carbon derived from methane is either completely oxidized to CO2 (dashed line) or is assimilated through the RuMP pathway into the glycolysis and TCA cycle. A proposed cellular ultrastructure is shown in the top right. The genome also encodes genes to the construction of pili, flagella and for the accumulation of polyphosphate and glycogen granules. Abbreviations: G6P, glucose 6-phosphate; G1,5LP, 6-phospho D-glucono-1,5-lactone; 6GP, gluconate 6-phosphate; Ru5P, ribulose 5-phosphate; Ri5P, ribose 5-phosphate; X5P, xylulose 5-phosphate; GAP, glyceraldehyde 3-phosphate; S7P, sedoheptulose 7-phosphate; F6P, fructofuranose 6-phosphate; E4P, erythrose 4-phosphate; F1,6P, fructose 1,6-bisphosphate; 3PGA, 3-phospho-D-glycerate; 2PGA, 2-phospho-D-glycerate; PEP, phosphoenolpyruvate; CH2 = H4MPT, 5,10-methylene-tetrahydromethanopterin; CH2≡H4MPT, 5,10-methenyltetrahydromethanopterin; CHO-H4MPT, 5-formyl-tetrahydromethanopterin.
B42 has the ability to store excess carbon in the form of glycogen. The genome contains genes involved in glyconeogenesis (glycogen formation) including glycogen synthase, and branching enzymes and glycogen utilizing enzymes such as debranching enzymes, glycogen phosphorylase, and alpha-amylase. Methanotrophs have been observed to use either glycogen or PHB, with a preference for type I methanotrophs to produce glycogen exclusively (Pieja et al., 2011). Interestingly, some cultured members of the Methylothermaceae have been observed to contain intracellular granules, reported to be PHB (Heyer et al., 2005; Hirayama et al., 2011, 2014). While there were no genes for PHB production identified in the partial B42 genome, M. crimeensis was found to contain some of the genes required for PHB synthesis. Further confirmation of intracellular granules in cultured Methylothermaceae is required, however, PHB production may be possible in certain strains of type I methanotrophs.
Copper Acquisition, Use, and Expulsion
Copper is the metal cofactor for pMMO, and as such, the copper requirement of methanotrophs is estimated to be 10 times that of other organisms (Semrau et al., 2013). B42 appears to lack copper uptake genes for the synthesis of methanobactin; and does not contain the mmoB gene, usually associated with the soluble MMO, which acts in copper sensing and regulation (Semrau et al., 2013). However, methanobactin may be confined to a few type II methanotrophs (Kenney and Rosenzweig, 2013), which may mean that B42 uses a novel chalkophore or perhaps the high copper concentrations found at the Tu’i Malila hydrothermal vents (Sylvan et al., 2013) abrogate the need for a copper concentrating mechanism. Copper homeostasis may be achieved in B42 using the cop operon (copABCD) that has been shown to be involved in copper import and efflux in non-methanotroph gammaproteobacterial model organisms. For example, CopC and CopD have been shown to increase copper uptake in Pseudomonas (Cha and Cooksey, 1993), while CopA and CopB are P-type ATPases that can import or export copper across the outer membrane (Cooksey, 1994; Solioz and Stoyanov, 2003).
Respiration Using a Branched Electron Transport Chain
As an aerobic methanotroph, B42 requires oxygen for the oxidation of methane. Perhaps to survive oxygen limitation, B42 encodes a number of respiratory complexes that allow for respiration over a wide range of oxygen concentrations (Figure 4). The B42 genome encodes a complete electron transport chain, including a sodium-translocating NADH:quinone oxidoreductase, Complex III, and genes for utilizing both oxygen and oxidized nitrogen as terminal electron acceptors. These pathways have a variety of evolutionary histories and reflect both vertical inheritance of pathways and horizontal gene transfer.
The genome of B42 contains all of the subunits of two members of the heme-copper oxidase (HCO) superfamily that encode an A-family and a B-family O2 reductase. The B-family O2 reductase enzymes are adapted to lower concentrations of oxygen than the A-family, having converted a conserved proton channel into an O2 channel. This result in a higher affinity for O2 but fewer protons pumped per electron (Han et al., 2011). Possessing both A- and B-family HCO genes may allow B42 to respire over a wide range of oxygen concentrations.
B42 also possesses a cytochrome bd oxidase, a respiratory quinol:O2 oxidoreductase with a very high affinity for O2 (Borisov et al., 2011). The bd oxidase family is not homologous to the HCO superfamily, and conserves less energy than HCOs as bd oxidase donates electrons to O2 directly from quinol, bypassing energy conservation at Complex III, and lacks conserved channels for pumping protons (Borisov et al., 2011; Han et al., 2011). However, bd oxidase has an extremely high affinity for O2, allowing it to be used for respiration at vanishingly low oxygen concentrations, with a Km for O2 consumption of 3–8 nM (D’mello et al., 1996; Stolper et al., 2010).
Multiple pathways suggest that B42 is capable of generating or consuming either a H+ or Na+ gradient for energy. Two separate and complete ATP synthase operons were detected in the genome, one of which was annotated as Na+-translocating. Precise understanding of the sequence-level differences between proton and sodium translocating ATP synthases is lacking in most organisms other than Acetobacterium woodii, which has a distinct evolutionary history (Müller et al., 2001; Müller and Grüber, 2003). Conclusive evidence for sodium-driven ATP generation in B42 is therefore difficult, however, the closest homolog is found the cultured organism, M. crimeensis. Further testing of M. crimeensis’ membrane kinetics may therefore aid in determining B42′s activity. To generate the Na+ gradient, B42 possesses a complete Na+-pumping NADH:quinone oxidoreductase operon (nqrABCDEF) similar to those described for Vibrio cholerae species. These proteins are functionally similar to the proton-translocating protein of the same name, but are evolutionarily distinct, and are composed of non-homologous subunits (Steuber et al., 2014).
B42 may be able to utilize nitrate as an alternative electron acceptor under extreme oxygen limitation (Figure 4). As pMMO has an absolute O2 requirement to convert methane to methanol, this metabolism could not continue when oxygen is entirely absent. However, denitrification in B42 could be coupled to aerobic methanotrophy under oxygen limitation by utilizing available O2 for activating methane with pMMO while using oxidized nitrogen as an electron acceptor, as has been shown recently for Methylomonas denitrificans (Kits et al., 2015). Like M. denitrificans, B42 appears to contain a cyanoglobin homolog, which in M. denitrificans is upregulated under hypoxic conditions and has been hypothesized to bind oxygen for delivery to pMMO (Kits et al., 2015).
An incomplete denitrification pathway is present in the B42 genome to convert nitrate to nitrous oxide (Figure 4). Two respiratory nitrate reductase operons (narGHIJ) are present and both are adjacent to the nitrate/nitrite transporter (narK). Phylogenetic analysis of the NarG gene revealed that the two copies have distinct evolutionary histories (Figure 5), suggesting they are the result of independent horizontal transfer events and not due to duplication within the B42 genome. One copy of NarG was most related to sequences from Alphaproteobacteria, while the other copy is most related to sequences from the Gammaproteobacteria (Figure 5). Nitrite reduction occurs using the copper-containing nitrite reductase, nirK, and appears to have been inherited from the common ancestor of B42 and M. crimeensis (Figure 6). Nitrite is a toxic intermediate formed not only from nitrate reduction but also ammonium oxidation. The pMMO enzyme can oxidize small amounts of ammonium, creating hydroxylamine that is converted to nitrite by hydroxylamine dehydrogenase (hao), also found in the B42 genome (Nyerges and Stein, 2009). As NirK is found in a number of non-denitrifying members of the Methylococcaceae, it may be conserved in methanotrophs, along with hydroxylamine oxidoreductase and nitric oxide reductase, as part of a conserved detoxification pathway for the byproducts of non-specific ammonia oxidation by pMMO. Nitric oxide produced from nitrite reduction can be reduced using the cytochrome c dependent nitric oxide reductase cNOR, a member of the heme copper oxidase superfamily. The final step in denitrification, reduction of nitrous oxide to nitrogen gas, does not appear to be encoded by the genome. Nitrous oxide reduction is often absent from denitrifier genomes, as nitrous oxide is largely non-toxic (Zumft, 1997) and this step conserves less energy, hence when oxidized nitrogen is not limiting it is more energy efficient to allow nitrous oxide to escape (Chen and Strous, 2013).
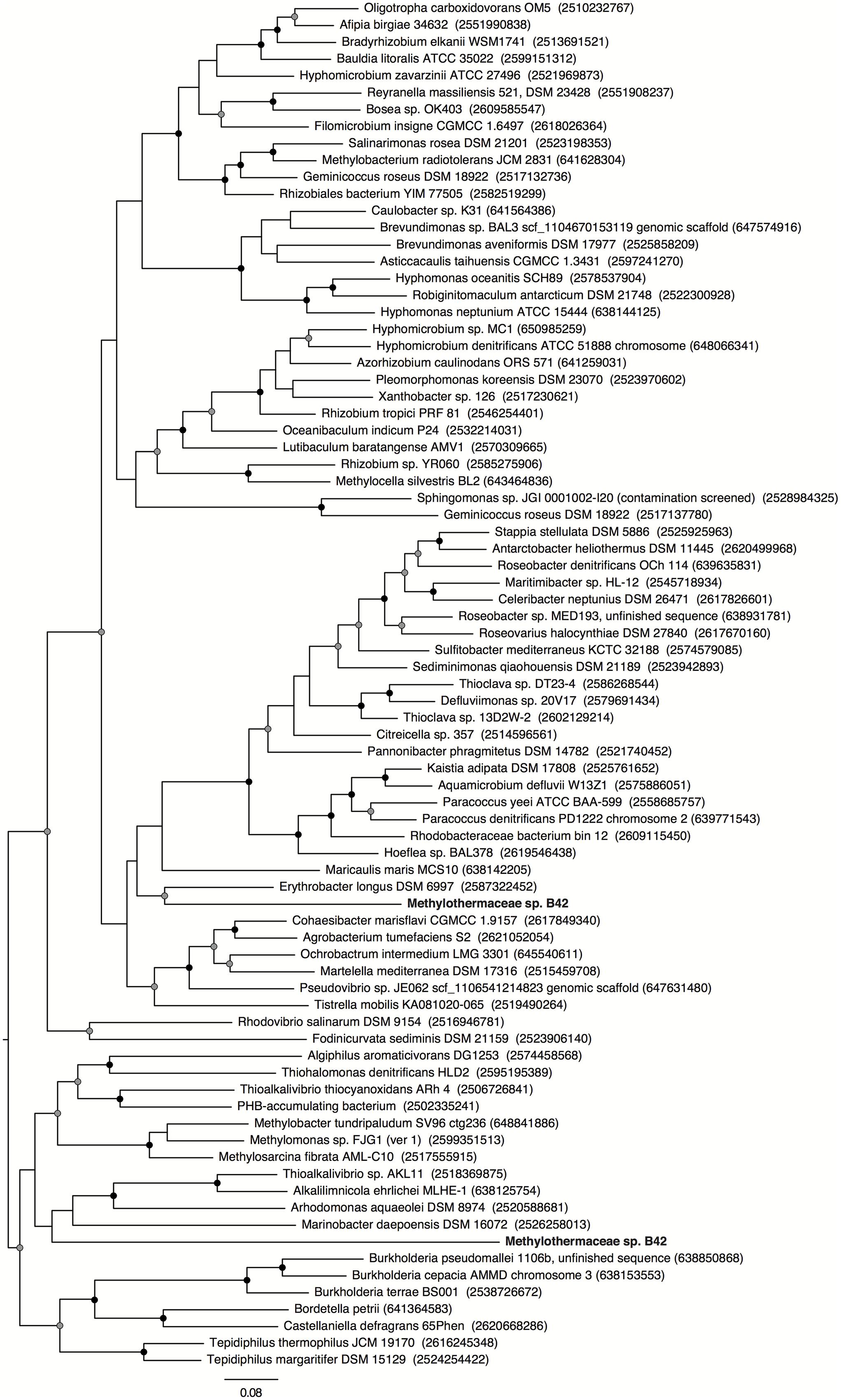
FIGURE 5. Maximum likelihood phylogenetic tree of NarG from reference genomes and B42. Tip labels show the genome name and the IMG gene id in brackets. The tree was rooted at the mid-point node, no outgroup was included. Nodes with greater than 70 or 90% bootstrap support are indicated with a gray or black circle, respectively. Scale bar indicates substitutions per site.
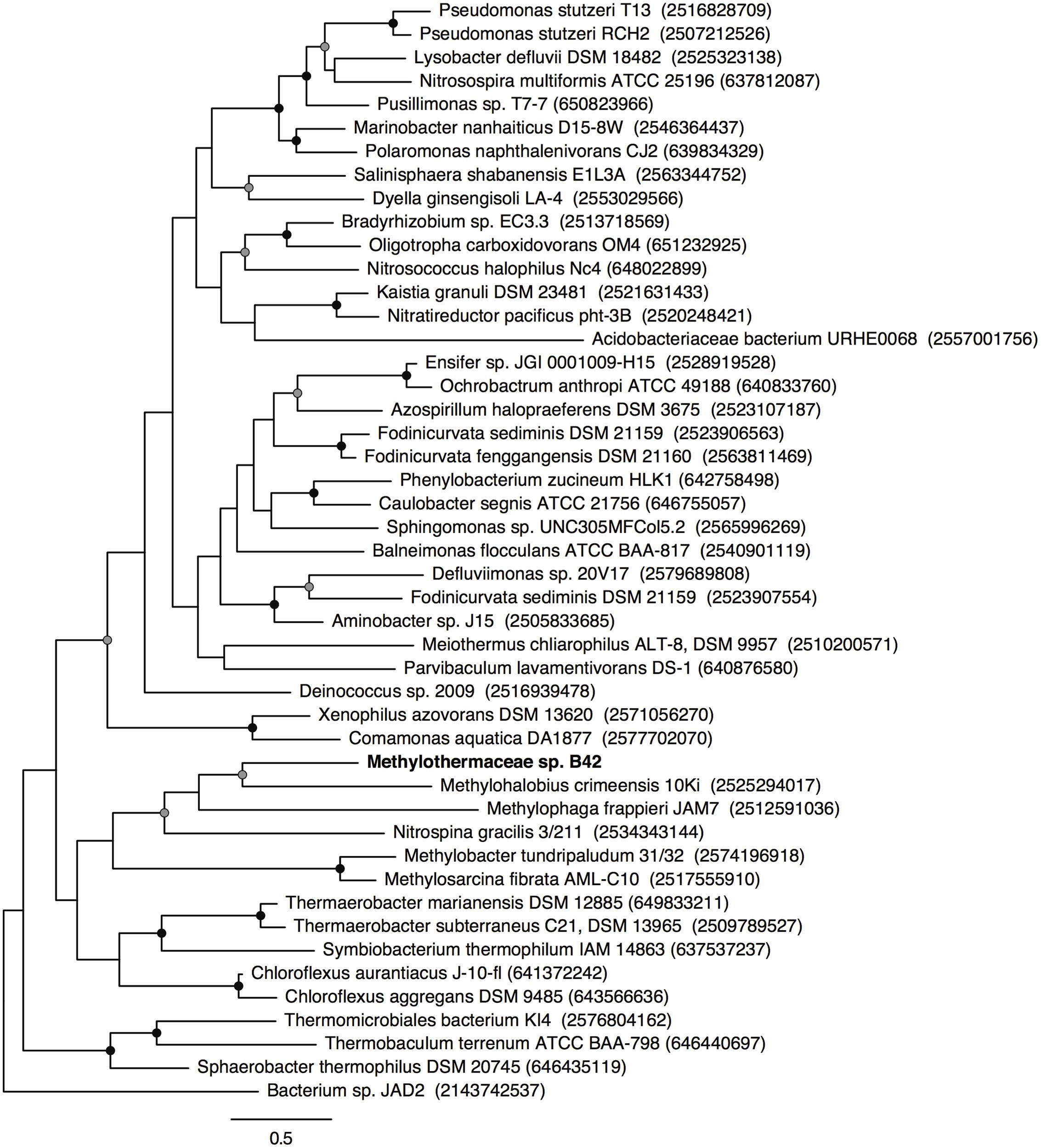
FIGURE 6. Maximum likelihood phylogenetic tree of NirK from reference genomes and B42. Nodes with greater than 70 or 90% bootstrap support are indicated with a gray or black circle, respectively. Scale bar indicates substitutions per site.
The complement of terminal electron accepting reactions possessed by B42 implies an organism capable of thriving under a range of oxygen concentrations. While it would conserve the most energy utilizing its A-family HCO under high oxygen conditions, B42 appears to be capable of continuing to respire under low-O2 conditions using the B-family HCO or the cytochrome bd oxidase, and to be capable of transitioning to incomplete denitrification when oxygen is nearly depleted. Respiratory nitrate reduction by aerobic methanotrophic bacteria has previously been demonstrated in M. denitrificans and its presence in the B42 genome may indicate it is also capable of nitrate respiration. Nitrate is generally non-toxic, and its dissimilatory reduction is adaptive as an electron acceptor. The acquisition of denitrification genes could greatly expand the niche of B42 and also suggests an adaptive advantage to using nitrate as an electron acceptor. Fluctuating oxygen concentrations could severely limit growth by aerobic methanotrophs, however, denitrification allows all oxygen to be utilized for activating methane, thus enabling B42 and other denitrifying aerobic methanotrophs to outcompete others for methane in these environments.
It remains unclear how a transition of oxygen to nitrate utilization would be accomplished, and what electron donors would be utilized under denitrifying conditions. An alternative, though perhaps not mutually exclusive hypothesis, is that denitrification and aerobic respiration are run simultaneously. As aerobic respiration and denitrification share many components of the electron transport chain, differing only in the terminal electron acceptor, it has been proposed that a “hybrid” of the two pathways can be run to maximize energy conservation under low-O2 conditions and to minimize response times when oxygen levels fluctuate (Chen and Strous, 2013). A final alternative is that B42 utilizes denitrification under anoxic conditions coupled to heterotrophy rather than methanotrophy. Resolution of these possibilities may be possible via isolation of B42 or in situ transcriptomic analyses under a range of oxygen concentrations.
Conclusion
Metagenomic sequencing of a deep-sea hydrothermal vent has recovered the genome of a novel methanotroph, B42, from the family Methylothermaceae. B42 was the dominant methanotroph recovered from this white smoker chimney and its genome contained multiple adaptations to varying oxygen concentrations. The presence of denitrification genes has only been identified in three other gammaproteobacterial methanotrophs and suggests that oxygen limitation generates evolutionary pressure for B42 at hydrothermal vents. Coupling of methanotrophy and denitrification suggests that links between the methane and nitrogen cycles are more common than previously recognized. However, the nature of denitrification-coupled methanotrophy is currently unresolved, and requires physiological experiments to determine oxygen concentrations for and dynamics of the transition from oxygen to nitrate as an electron acceptor.
Author Contributions
VO and VG conceived the initial study of the hydrothermal vent systems. KC performed laboratory work to prepare samples for sequencing. CS performed initial assembly and binning. VO and CS conceived the analysis of the metagenome bin. CS, LW, AM, KM, CV, SM performed analysis of the metagenome bin. CS, LW, AM, KM, CV, SM, VO wrote and revised the manuscript.
Funding
This research was supported in part by a grant from the NASA Astrobiology Institute (Award # NNA13AA92A) and the Gordon and Betty Moore Foundation Marine Microbiology Initiative (GBMF3780) to VO. This is NAI-Life Underground Publication Number 070. Part of this work was supported by grants to VG: NIH 1R21MH103824-01; the Gordon and Betty Moore Foundation through Grant GBMF2809 to the Caltech Programmable Molecular Technology Initiative and by the Beckman Institute for Optogenetics and CLARITY. KC is supported by the NIH Predoctoral Training in Biology and Chemistry grant (2T32GM007616-36). LW was supported by an NSF Graduate Research Fellowship. Sample collection from the Tu’i Malila vent field was funded by the National Science Foundation (NSF), grant number NSF0CE-0241613, awarded to Robert Vrijenhoek.
Conflict of Interest Statement
The authors declare that the research was conducted in the absence of any commercial or financial relationships that could be construed as a potential conflict of interest.
Acknowledgments
The metagenomic analysis and annotation for B42 was done in part by the students of the GeBI 246 Molecular Geobiology course at Caltech. We thank Ben Harrison for XRD analysis on sample #2044C and Patty Tavormina for assistance during the course and critical reading of this manuscript. We also thank Chief Scientist Robert Vrijenhoek (Monterey Bay Aquarium Research Institute) for providing the opportunity to collect samples during the 2005 tuim06mv cruise. We thank Igor Antoshechkin of the Millard and Muriel Jacobs Genetics and Genomics Laboratory at Caltech for his services and input during sequencing of the genomic library.
References
Anantharaman, K., Breier, J. A., and Dick, G. J. (2015). Metagenomic resolution of microbial functions in deep-sea hydrothermal plumes across the Eastern Lau Spreading Center. ISME J. doi: 10.1038/ismej.2015.81 [Epub ahead of print].
Auman, A. J., Speake, C. C., and Lidstrom, M. E. (2001). nifH sequences and nitrogen fixation in type I and type II methanotrophs. Appl. Environ. Microbiol. 67, 4009–4016. doi: 10.1128/AEM.67.9.4009-4016.2001
Aziz, R., Bartels, D., Best, A., DeJongh, M., Disz, T., Edwards, R., et al. (2008). The RAST Server: rapid annotations using subsystems technology. BMC Genomics 9:75. doi: 10.1186/1471-2164-9-75
Bankevich, A., Nurk, S., Antipov, D., Gurevich, A. A., Dvorkin, M., Kulikov, A. S., et al. (2012). SPAdes: a new genome assembly algorithm and its applications to single-cell sequencing. J. Comput. Biol. 19, 455–477. doi: 10.1089/cmb.2012.0021
Beaulieu, S. E., Baker, E. T., German, C. R., and Maffei, A. (2013). An authoritative global database for active submarine hydrothermal vent fields. Geochem. Geophys. Geosyst. 14, 4892–4905. doi: 10.1002/2013GC004998
Bédard, C., and Knowles, R. (1989). Physiology, biochemistry, and specific inhibitors of CH4, NH4+, and CO oxidation by methanotrophs and nitrifiers. Microbiol. Rev. 53, 68–84.
Borisov, V. B., Gennis, R. B., Hemp, J., and Verkhovsky, M. I. (2011). The cytochrome bd respiratory oxygen reductases. Biochim. Biophys. Acta 1807, 1398–1413. doi: 10.1016/j.bbabio.2011.06.016
Campbell, M. A., Nyerges, G., Kozlowski, J. A., Poret-Peterson, A. T., Stein, L. Y., and Klotz, M. G. (2011). Model of the molecular basis for hydroxylamine oxidation and nitrous oxide production in methanotrophic bacteria. FEMS Microbiol. Lett. 322, 82–89. doi: 10.1111/j.1574-6968.2011.02340.x
Cha, J. S., and Cooksey, D. A. (1993). Copper hypersensitivity and uptake in Pseudomonas syringae containing cloned components of the copper resistance operon. Appl. Environ. Microbiol. 59, 1671–1674.
Chen, J., and Strous, M. (2013). Denitrification and aerobic respiration, hybrid electron transport chains and co-evolution. Biochim. Biophys. Acta 1827, 136–144. doi: 10.1016/j.bbabio.2012.10.002
Cooksey, D. A. (1994). Molecular mechanisms of copper resistance and accumulation in bacteria. FEMS Microbiol. Rev. 14, 381–386. doi: 10.1111/j.1574-6976.1994.tb00112.x
Dick, G., Andersson, A., Baker, B., Simmons, S., Thomas, B., Yelton, A. P., et al. (2009). Community-wide analysis of microbial genome sequence signatures. Genome Biol. 10, R85. doi: 10.1186/gb-2009-10-8-r85
Dick, G. J., Anantharaman, K., Baker, B. J., Li, M., Reed, D. C., and Sheik, C. S. (2013). The microbiology of deep-sea hydrothermal vent plumes: ecological and biogeographic linkages to seafloor and water column habitats. Front. Microbiol. 4:124. doi: 10.3389/fmicb.2013.00124
D’mello, R., Hill, S., and Poole, R. K. (1996). The cytochrome bd quinol oxidase in Escherichia coli has an extremely high oxygen affinity and two oxygen-binding haems: implications for regulation of activity in vivo by oxygen inhibition. Microbiology 142(Pt 4), 755–763. doi: 10.1099/00221287-142-4-755
Edgar, R. C. (2004). MUSCLE: multiple sequence alignment with high accuracy and high throughput. Nucleic Acids Res. 32, 1792–1797. doi: 10.1093/nar/gkh340
Elderfield, H., and Schultz, A. (1996). Mid-ocean ridge hydrothermal fluxes and the chemical composition of the ocean. Annu. Rev. Earth Planet. Sci. 24, 191–224. doi: 10.1146/annurev.earth.24.1.191
Elsaied, H. E., Hayashi, T., and Naganuma, T. (2004). Molecular analysis of deep-sea hydrothermal vent aerobic methanotrophs by targeting genes of 16S rRNA and particulate methane monooxygenase. Mar. Biotechnol. 6, 503–509. doi: 10.1007/s10126-004-3042-0
Ettwig, K. F., Butler, M. K., Le Paslier, D., Pelletier, E., Mangenot, S., Kuypers, M. M. M., et al. (2010). Nitrite-driven anaerobic methane oxidation by oxygenic bacteria. Nature 464, 543–548. doi: 10.1038/nature08883
Han, H., Hemp, J., Pace, L. A., Ouyang, H., Ganesan, K., Roh, J. H., et al. (2011). Adaptation of aerobic respiration to low O2 environments. Proc. Natl. Acad. Sci. U.S.A. 108, 14109–14114. doi: 10.1073/pnas.1018958108
Haroon, M. F., Hu, S., Shi, Y., Imelfort, M., Keller, J., Hugenholtz, P., et al. (2013). Anaerobic oxidation of methane coupled to nitrate reduction in a novel archaeal lineage. Nature 500, 567–570. doi: 10.1038/nature12375
Heyer, J., Berger, U., Hardt, M., and Dunfield, P. F. (2005). Methylohalobius crimeensis gen. nov., sp. nov., a moderately halophilic, methanotrophic bacterium isolated from hypersaline lakes of Crimea. Int. J. Syst. Evol. Microbiol. 55, 1817–1826. doi: 10.1099/ijs.0.63213-0
Hirayama, H., Abe, M., Miyazaki, M., Nunoura, T., Furushima, Y., Yamamoto, H., et al. (2014). Methylomarinovum caldicuralii gen. nov., sp. nov., a moderately thermophilic methanotroph isolated from a shallow submarine hydrothermal system, and proposal of the family Methylothermaceae fam. nov. Int. J. Syst. Evol. Microbiol. 64, 989–999. doi: 10.1099/ijs.0.058172-0
Hirayama, H., Fuse, H., Abe, M., Miyazaki, M., Nakamura, T., Nunoura, T., et al. (2013). Methylomarinum vadi gen. nov., sp. nov., a methanotroph isolated from two distinct marine environments. Int. J. Syst. Evol. Microbiol. 63, 1073–1082. doi: 10.1099/ijs.0.040568-0
Hirayama, H., Sunamura, M., Takai, K., Nunoura, T., Noguchi, T., Oida, H., et al. (2007). Culture-dependent and-independent characterization of microbial communities associated with a shallow submarine hydrothermal system occurring within a coral reef off Taketomi Island, Japan. Appl. Environ. Microbiol. 73, 7642–7656. doi: 10.1128/AEM.01258-07
Hirayama, H., Suzuki, Y., Abe, M., Miyazaki, M., Makita, H., Inagaki, F., et al. (2011). Methylothermus subterraneus sp. nov., a moderately thermophilic methanotroph isolated from a terrestrial subsurface hot aquifer. Int. J. Syst. Evol. Microbiol. 61, 2646–2653. doi: 10.1099/ijs.0.028092-0
Hommes, N. G., Sayavedra-Soto, L. A., and Arp, D. J. (2001). Transcript analysis of multiple copies of amo (encoding ammonia monooxygenase) and hao (encoding hydroxylamine oxidoreductase) in Nitrosomonas europaea. J. Bacteriol. 183, 1096–1100. doi: 10.1128/JB.183.3.1096-1100.2001
Kenney, G. E., and Rosenzweig, A. C. (2013). Genome mining for methanobactins. BMC Biol. 11:17. doi: 10.1186/1741-7007-11-17
Kits, K. D., Klotz, M. G., and Stein, L. Y. (2015). Methane oxidation coupled to nitrate reduction under hypoxia by the Gammaproteobacterium Methylomonas denitrificans, sp. nov. type strain FJG1. Environ. Microbiol. 17, 3219–3232. doi: 10.1111/1462-2920.12772
Lesniewski, R. A., Jain, S., Anantharaman, K., Schloss, P. D., and Dick, G. J. (2012). The metatranscriptome of a deep-sea hydrothermal plume is dominated by water column methanotrophs and lithotrophs. ISME J. 6, 2257–2268. doi: 10.1038/ismej.2012.63
Li, D., Liu, C.-M., Luo, R., Sadakane, K., and Lam, T.-W. (2015). MEGAHIT: an ultra-fast single-node solution for large and complex metagenomics assembly via succinct de Bruijn graph. Bioinformatics 31, 1674–1676. doi: 10.1093/bioinformatics/btv033
Li, M., Toner, B. M., Baker, B. J., Breier, J. A., Sheik, C. S., and Dick, G. J. (2014). Microbial iron uptake as a mechanism for dispersing iron from deep-sea hydrothermal vents. Nat. Commun. 5, 3192. doi: 10.1038/ncomms4192
Lidstrom, M. E. (1988). Isolation and characterization of marine methanotrophs. Antonie Van Leeuwenhoek 54, 189–199. doi: 10.1007/BF00443577
Markowitz, V. M., Chen, I.-M. A., Chu, K., Szeto, E., Palaniappan, K., Pillay, M., et al. (2014). IMG/M 4 version of the integrated metagenome comparative analysis system. Nucleic Acids Res. 42, D568–D573. doi: 10.1093/nar/gkt919
Mottl, M. J., Seewald, J. S., Wheat, C. G., Tivey, M. K., Michael, P. J., Proskurowski, G., et al. (2011). Chemistry of hot springs along the Eastern Lau Spreading Center. Geochim. Cosmochim. Acta 75, 1013–1038. doi: 10.1016/j.gca.2010.12.008
Müller, V., Aufurth, S., and Rahlfs, S. (2001). The Na(+) cycle in Acetobacterium woodii: identification and characterization of a Na(+) translocating F(1)F(0)-ATPase with a mixed oligomer of 8 and 16 kDa proteolipids. Biochim. Biophys. Acta 1505, 108–120. doi: 10.1016/S0005-2728(00)00281-4
Müller, V., and Grüber, G. (2003). ATP synthases: structure, function and evolution of unique energy converters. Cell. Mol. Life Sci. 60, 474–494. doi: 10.1007/s000180300040
Nercessian, O., Bienvenu, N., Moreira, D., Prieur, D., and Jeanthon, C. (2005). Diversity of functional genes of methanogens, methanotrophs and sulfate reducers in deep-sea hydrothermal environments. Environ. Microbiol. 7, 118–132. doi: 10.1111/j.1462-2920.2004.00672.x
Nyerges, G., and Stein, L. Y. (2009). Ammonia cometabolism and product inhibition vary considerably among species of methanotrophic bacteria. FEMS Microbiol. Lett. 297, 131–136. doi: 10.1111/j.1574-6968.2009.01674.x
Overbeek, R., Olson, R., Pusch, G. D., Olsen, G. J., Davis, J. J., Disz, T., et al. (2014). The SEED and the Rapid Annotation of microbial genomes using Subsystems Technology (RAST). Nucleic Acids Res. 42, D206–D214. doi: 10.1093/nar/gkt1226
Parks, D. H., Imelfort, M., Skennerton, C. T., Hugenholtz, P., and Tyson, G. W. (2015). CheckM: assessing the quality of microbial genomes recovered from isolates, single cells, and metagenomes. Genome Res. 25, 1043–1055. doi: 10.1101/gr.186072.114
Pieja, A. J., Rostkowski, K. H., and Criddle, C. S. (2011). Distribution and selection of poly-3-hydroxybutyrate production capacity in methanotrophic proteobacteria. Microb. Ecol. 62, 564–573. doi: 10.1007/s00248-011-9873-0
Semrau, J. D., Jagadevan, S., DiSpirito, A. A., Khalifa, A., Scanlan, J., Bergman, B. H., et al. (2013). Methanobactin and MmoD work in concert to act as the “copper-switch” in methanotrophs. Environ. Microbiol. 15, 3077–3086. doi: 10.1111/1462-2920.12150
Sieburth, J. N., Johnson, P. W., Eberhardt, M. A., Sieracki, M. E., Lidstrom, M., and Laux, D. (1987). The first methane-oxidizing bacterium from the upper mixing layer of the deep ocean: Methylomonas pelagica sp. nov. Curr. Microbiol. 14, 285–293. doi: 10.1007/BF01568138
Solioz, M., and Stoyanov, J. V. (2003). Copper homeostasis in Enterococcus hirae. FEMS Microbiol. Rev. 27, 183–195. doi: 10.1016/S0168-6445(03)00053-6
Stamatakis, A. (2014). RAxML version 8: a tool for phylogenetic analysis and post-analysis of large phylogenies. Bioinformatics 30, 1312–1313. doi: 10.1093/bioinformatics/btu033
Stein, L. Y., and Klotz, M. G. (2011). Nitrifying and denitrifying pathways of methanotrophic bacteria. Biochem. Soc. Trans. 39, 1826–1831. doi: 10.1042/BST20110712
Steuber, J., Vohl, G., Casutt, M. S., Vorburger, T., Diederichs, K., and Fritz, G. (2014). Structure of the V. cholerae Na+-pumping NADH: quinone oxidoreductase. Nature 516, 62–67. doi: 10.1038/nature14003
Stolper, D. A., Revsbech, N. P., and Canfield, D. E. (2010). Aerobic growth at nanomolar oxygen concentrations. Proc. Natl. Acad. Sci. U.S.A. 107, 18755–18760. doi: 10.1073/pnas.1013435107
Stolyar, S., Costello, A. M., Peeples, T. L., and Lidstrom, M. E. (1999). Role of multiple gene copies in particulate methane monooxygenase activity in the methane-oxidizing bacterium Methylococcus capsulatus Bath. Microbiology 145(Pt 5), 1235–1244. doi: 10.1099/13500872-145-5-1235
Sylvan, J. B., Sia, T. Y., Haddad, A. G., Briscoe, L. J., Toner, B. M., Girguis, P. R., et al. (2013). Low temperature geomicrobiology follows host rock composition along a geochemical gradient in lau basin. Front. Microbiol. 4:61. doi: 10.3389/fmicb.2013.00061
Tagliabue, A., Bopp, L., Dutay, J.-C., Bowie, A. R., Chever, F., Jean-Baptiste, P., et al. (2010). Hydrothermal contribution to the oceanic dissolved iron inventory. Nat. Geosci. 3, 252–256. doi: 10.1038/ngeo818
Tavormina, P. L., Ussler, W. III, Joye, S. B., Harrison, B. K., and Orphan, V. J. (2010). Distributions of putative aerobic methanotrophs in diverse pelagic marine environments. ISME J. 4, 700–710. doi: 10.1038/ismej.2009.155
Trotsenko, Y. A., and Murrell, J. C. (2008). Metabolic aspects of aerobic obligate methanotrophy. Adv. Appl. Microbiol. 63, 183–229. doi: 10.1016/S0065-2164(07)00005-6
Ultsch, A., and Moerchen, F. (2005). ESOM-Maps: Tools for Clustering, Visualization, and Classification with Emergent SOM. Technical Report No. 46. Marburg: University of Marburg.
Welhan, J. A., and Craig, H. (1979). Methane and hydrogen in East Pacific Rise hydrothermal fluids. Geophys. Res. Lett. 6, 829–831. doi: 10.1029/GL006i011p00829
Welhan, J. A., and Craig, H. (1983). “Methane, hydrogen and helium in hydrothermal fluids at 21°N on the East Pacific Rise,” in Hydrothermal Processes at Seafloor Spreading Centers NATO Conference Series, eds P. A. Rona, K. Boström, L. Laubier, and K. L. Smith Jr. (New York, NY: Springer), 391–409. doi: 10.1007/978-1-4899-0402-7_17
Keywords: methane, nitrate, denitrification, deep sea, thermophile, hydrothermal vent, Lau Basin, methane oxidation
Citation: Skennerton CT, Ward LM, Michel A, Metcalfe K, Valiente C, Mullin S, Chan KY, Gradinaru V and Orphan VJ (2015) Genomic Reconstruction of an Uncultured Hydrothermal Vent Gammaproteobacterial Methanotroph (Family Methylothermaceae) Indicates Multiple Adaptations to Oxygen Limitation. Front. Microbiol. 6:1425. doi: 10.3389/fmicb.2015.01425
Received: 02 October 2015; Accepted: 30 November 2015;
Published: 23 December 2015.
Edited by:
Marc Strous, University of Calgary, CanadaReviewed by:
Ludmila Chistoserdova, University of Washington, USABiswarup Sen, Amity University Haryana, India
Copyright © 2015 Skennerton, Ward, Michel, Metcalfe, Valiente, Mullin, Chan, Gradinaru and Orphan. This is an open-access article distributed under the terms of the Creative Commons Attribution License (CC BY). The use, distribution or reproduction in other forums is permitted, provided the original author(s) or licensor are credited and that the original publication in this journal is cited, in accordance with accepted academic practice. No use, distribution or reproduction is permitted which does not comply with these terms.
*Correspondence: Connor T. Skennerton, Yy5za2VubmVydG9uQGdtYWlsLmNvbQ==; Victoria J. Orphan, dm9ycGhhbkBnbWFpbC5jb20=