- 1Department of Infectious Diseases, The Scripps Research Institute, Jupiter, FL, USA
- 2Department of Molecular and Cellular Neurosciences, The Scripps Research Institute, La Jolla, CA, USA
- 3Department of Metabolism and Aging, The Scripps Research Institute, Jupiter, FL, USA
- 4Department of Neuroscience, The Scripps Research Institute, Jupiter, FL, USA
- 5Department of Pharmacodynamics, University of Florida, Gainesville, FL, USA
Antiretroviral therapy has dramatically improved the lives of human immunodeficiency virus 1 (HIV-1) infected individuals. Nonetheless, HIV-associated neurocognitive disorders (HAND), which range from undetectable neurocognitive impairments to severe dementia, still affect approximately 50% of the infected population, hampering their quality of life. The persistence of HAND is promoted by several factors, including longer life expectancies, the residual levels of virus in the central nervous system (CNS) and the continued presence of HIV-1 regulatory proteins such as the transactivator of transcription (Tat) in the brain. Tat is a secreted viral protein that crosses the blood–brain barrier into the CNS, where it has the ability to directly act on neurons and non-neuronal cells alike. These actions result in the release of soluble factors involved in inflammation, oxidative stress and excitotoxicity, ultimately resulting in neuronal damage. The percentage of methamphetamine (MA) abusers is high among the HIV-1-positive population compared to the general population. On the other hand, MA abuse is correlated with increased viral replication, enhanced Tat-mediated neurotoxicity and neurocognitive impairments. Although several strategies have been investigated to reduce HAND and MA use, no clinically approved treatment is currently available. Here, we review the latest findings of the effects of Tat and MA in HAND and discuss a few promising potential therapeutic developments.
Introduction
The human immunodeficiency virus 1 (HIV-1) affects 35.3 million individuals worldwide (UNAIDS). The entry of the virus in the central nervous system (CNS) early during infection, and the relatively low penetration of current antiretroviral therapies (ART) in the brain, results in neurological complications collectively called HIV-associated neurocognitive disorders (HAND; Davis et al., 1992; reviewed in Gray et al., 1993; reviewed in Owen and Khoo, 2004; reviewed in Miller et al., 2008; Valcour et al., 2012; reviewed in Spudich, 2013). HAND can be subdivided into asymptomatic neurocognitive impairment (ANI), mild neurocognitive disorder (MND), and severe forms of HIV-associated encephalitis/dementia (HAD). Patients affected with ANI and MND score poorly in neuropsychological testing, but their everyday functioning is only minimally impacted. In contrast, HAD patients experience severe neurological failure that severely hampers their daily lives. Characteristic HAD symptoms include inflammation of the brain, decline in cognitive functions (memory, concentration, attention, and executive control), reduced motor function (loss of dexterity and coordination) and the mimicking of other dementia symptoms, such as in Alzheimer’s and Parkinson’s diseases. In addition, HAD patients present comorbid behavioral changes such as unipolar depression (apathy and loss of motivation), fatigue and social withdrawal (reviewed in Antinori et al., 2007). ART has considerably decreased the early occurrence of HAD, but the diagnosis of moderate neurocognitive impairments continues to increase (reviewed in Cisneros and Ghorpade, 2012). This increase is primarily attributed to longer life spans, the long-term toxicity of ART, and the development of resistance to therapy (reviewed in Pardo et al., 2001; reviewed in Lv et al., 2015; reviewed in Underwood et al., 2015).
The best accepted model to explain how HIV-1 gains access to the CNS is the “Trojan horse” hypothesis, in which infected monocytes and macrophages cross the blood–brain barrier (BBB) and subsequently infect non-neuronal cells including perivascular macrophages and glial cells (reviewed in Haase, 1986). Macrophages and microglia are the main supporters of viral replication in the brain (reviewed in Bagashev and Sawaya, 2013), whereas astrocytes are susceptible to HIV-1 infection but alone are not thought to support productive infection (reviewed in Bagashev and Sawaya, 2013). Pericytes have also been suggested as cells that may become infected by HIV-1, although at low levels, and be modified as a result of the presence of HIV-1 in the brain, thereby further contributing to BBB disruption (Nakagawa et al., 2012). Even though HIV-1 does not infect neurons, a major aspect of HAND is neuronal damage and apoptosis (reviewed in Kovalevich and Langford, 2012). Two mechanistic models of toxicity have been proposed: direct and indirect (or “bystander”; reviewed in Lindl et al., 2010). The direct model suggests that viral proteins released from infected cells cause neuronal death through a direct interaction with neurons. The indirect model proposes the death of neurons through the activity of soluble factors released as a result of inflammatory responses to viral particles or changes in the brain environment by non-neuronal cells. Regardless of the means, among the consequences of HIV infection is synaptodendritic injury to neurons that closely correlates to the occurrence and severity of neurocognitive disorders (reviewed in Ellis et al., 2007).
This synaptodendritic injury may be driven by viral replication in non-neuronal cells and is defined by structural and chemical changes occurring at neuronal synapses, resulting in a disturbance of communication between neurons responsible for behaviors such as memory, learning, and executive functions (reviewed in Ellis et al., 2007; reviewed in Nath and Steiner, 2014). Experimental studies in mice, monkeys or in human brain tissues confirm that exposure to HIV and the transactivator of transcription (Tat) protein induce alterations in neuronal morphology (reviewed in Ellis et al., 2007; Bertrand et al., 2014; reviewed in Nath and Steiner, 2014). These morphological changes associated with synaptodendritic injury may involve mitochondrial dysfunction (Greenwood et al., 2007). Notably, synaptodendritic injury may be reversible depending on the degree of injury (an example of “brain plasticity”; reviewed in Ellis et al., 2007). Accordingly, the addition of neuroprotective and regenerative factors to ART has been proposed to reduce HAND progression (reviewed in Ellis et al., 2007).
Among the viral proteins that play a role in neuropathogenesis, Tat, is a significant contributor. Although the main function of Tat is to regulate HIV-1 transcription, it has also been implicated in the dysregulation of inflammatory processes, induction of oxidative stress, excessive activation of neurotransmitters, modulation of apoptotic pathways, and irregular neurogenesis (see “Tat Neurotoxicity”, below). Indeed, elevated levels of Tat mRNA are commonly found in post-mortem brain samples from individuals with HAD (Wesselingh et al., 1993; Wiley et al., 1996; Hudson et al., 2000).
Beyond this, drug abuse correlates with an increased risk of exposure to HIV-1. The world drug report of 2014 (UNAIDS) describes that the prevalence of HIV infection among injecting drug users is 22–50 times higher than in the general population, depending on the country. The abuse of drugs largely prevalent in the US and worldwide, particularly the strong psychostimulant methamphetamine (MA), is correlated with increased neurotoxicity and immune system impairments, with a consequential exacerbation of HAND (Potula and Persidsky, 2008). It has been demonstrated in human and animal studies that the neurotoxicity caused by MA and Tat (or HIV-1 infection), results in greater cognitive and locomotor impairments than with either MA or Tat (or HIV-1) alone (see “Tat and Methamphetamine Neurotoxicity”).
This review will focus on the effects of Tat, MA, and their concerted effects in the CNS. Given that no treatment is yet available against the neurotoxic activity of Tat (Mediouni et al., 2012), or the additive detrimental effects of both Tat and MA, we will conclude with a discussion of some promising candidates emerging in the literature.
Tat Neurotoxicity
The early HIV-1 gene product, Tat, is an 86–103 amino acid protein, divided in six functional regions (Kuppuswamy et al., 1989; Figure 1A). Tat binds through its basic domain to the 5′-terminal region of HIV mRNA’s stem-bulge-loop structure transactivation response element (TAR), and recruits the positive transcription elongation factor b (P-TEFb) to promote transcriptional elongation from the viral promoter (Cann et al., 1985). In addition to its key role in HIV-1 transcription amplification, Tat can be transported and secreted by HIV-1 infected cells, and can cross the BBB. Once in the brain, non-infected cells may uptake Tat passively or via interaction with membrane receptors (reviewed in Li et al., 2009). Immunostaining patterns suggest that Tat can be found in the cytoplasm of perivascular macrophages, microglia nodules and in glial cells, but also in the nuclei of some neurons and oligodendrocytes. These data suggest that Tat can be taken up by all CNS cells and potentially exert its effects distally from HIV-1 replication sites (Del Valle et al., 2000; Hudson et al., 2000; Liu et al., 2000). As noted above, the neurotoxic activity of Tat comes from both direct action on neurons and by altering the release of different soluble factors from surrounding non-neuronal cells resulting in neuronal or synaptodendritic injury. Brain histological changes similar to those observed in HAD patients have been observed in different mouse models expressing HIV-1 Tat (reviewed in Rappaport et al., 1999; Bruce-Keller et al., 2003; Chauhan et al., 2003; Kim et al., 2003). A positive correlation has also been shown between the levels of Tat mRNA transcripts and HIV- and simian human immunodeficiency virus (SIV)-induced encephalitis (Hudson et al., 2000).
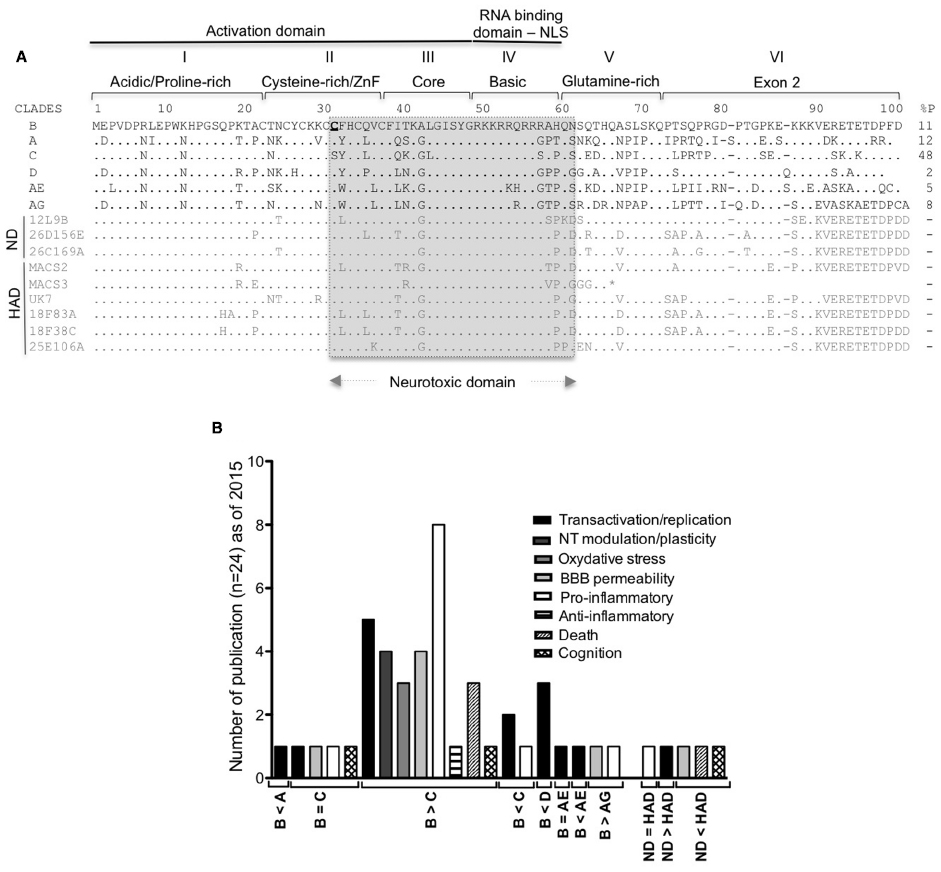
Figure 1. Tat HIV clades and HAND. (A) Conservation of the nucleotide sequences of Tat representative of the main HIV-1 clades (A–D and the circulating recombinant CRF_ AE/AG), brain derived isolates from non-demented HIV/AIDS individuals (ND sequences from Boven et al., 2007) and from individuals with HIV associated dementia [HAD sequences from Boven et al. (2007) and Thomas et al. (2007)]. Tat is encoded by two exons, divided into six functional regions. Region I (residues 1–21) is a proline-rich region, shown to protect Tat from degradation (Campbell and Loret, 2009; Caputo et al., 2009). Region II (residues 22–37) has seven conserved cysteines except for subtype C (which has 31C→31S) and the recombinant CRF_AE and CRF_AG (with more cysteines). Any mutation of these cysteines (except 31C) leads to loss of the transactivation activity (Kuppuswamy et al., 1989; Jeang et al., 1999). Region III (residues 38–48) has a conserved 38F(x)2KxLGISY motif. Mutation of 41K results in loss of transactivation (Kuppuswamy et al., 1989; Peloponese et al., 2000). 38F is conserved in Tat sequences and shown to be involved in binding to tubulin, resulting in apoptosis (Chen et al., 2002). Region I, II, and III constitute the minimal activation domain, which binds to cyclin T1. Region IV (residues 49–59) is rich in basic residues with the conserved sequence 49RKKRRQRRRPP. This domain is responsible for Tat’s interaction with TAR and is also a nuclear and nucleolar signal. Mutations in this domain results in loss of transactivation (Hauber et al., 1989) and delocalization of Tat from the nucleolus (Mousseau et al., 2012). The regions II as well as IV and Tat peptides covering the 31–61 amino acid region (in gray) were demonstrated to be neurotoxic (Mabrouk et al., 1991; Sabatier et al., 1991; Philippon et al., 1994; Weeks et al., 1995; Vives et al., 1997; Jones et al., 1998; Jia et al., 2001; Turchan et al., 2001; Self et al., 2004; Aksenov et al., 2006; Daily et al., 2006; Li et al., 2008; Mishra et al., 2008). Region V (residues 60–72) is the glutamine-rich region and was shown to be involved in apoptosis of lymphocytes T (Campbell et al., 2004). Regions I to V are encoded by exon I. Region VI is encoded by the second exon and contain a conserved RGD motif, found only in subtypes B and D. Predicted stop codon is indicated by an asterisk (*). % P: percentage of HIV-1 infected individuals in 2004–2007 with the indicated type of clade (Hemelaar et al., 2011). (B) Number of publications comparing the activity of HIV-1 clade B to other clades in the indicated neurotoxic activities.
The majority of the studies investigating neurotoxicity mediated by Tat demonstrated that the neurotoxic domain comprised part of the cysteine-rich/ZnF domain, the core and the basic domain of the protein (Figure 1A). Studies on HIV-1 clade B demonstrated the strong contribution of the highly conserved cysteine-rich/ZnF domain to neurotoxicity (Aksenov et al., 2009). In fact, studies carried out using other clades reported lesser neurotoxicity, which was attributed to differences in the sequence of the cysteine-rich/ZnF domain (Mishra et al., 2008). A summary of these findings is shown in Figure 1B. Interestingly, Tat from HIV-1 isolates found in patients with HAD exhibited higher neurotoxic potential than Tat isolated from non-HAD patients (Figure 1B). This suggests a relationship between characteristics of the brain environment and the selection of neurotoxic isolates, and validates the relevance of using Tat from virus present in HAD-positive individuals to study Tat activity, rather than laboratory adapted viruses. Regrettably, most of the studies on Tat to date do not address potential differences between the neurotoxic activity of Tat expressed upon transfection of eukaryotic cells and recombinant Tat produced in bacteria. Of the few studies that have offered such comparisons, either no differences were observed (Zauli et al., 2000; Koller et al., 2001), or higher toxicity was detected with transfected Tat when equivalent concentrations of both were examined (Li et al., 2008). These results could be explained by differences on the folding of Tat protein in vitro, and in vivo, or by differences of post-transcriptional modifications of Tat between eukaryotic and prokaryotic cells. There is also the possibility that the recombinant protein suffers some degradation when added to cell cultures, resulting in reduced activity.
Tat and Neurotransmitters Modulation
The effects of Tat on neurotransmission have been largely studied on the glutamatergic (GLUTergic) and dopaminergic (DAergic) systems. Glutamate is an excitatory neurotransmitter essential for learning, memory, and synaptic plasticity (reviewed in Rahn et al., 2012), and has been shown to regulate drug reward (Campbell et al., 2004; Mishra et al., 2008). However, excessive glutamate causes “glutamate excitotoxicity” leading to neuron death (reviewed in Rahn et al., 2012). Tat protein impairs glutamate reuptake by astrocytes (Zhou et al., 2004) and increases microglial glutamate release (Gupta et al., 2010), elevating extracellular glutamate levels. The down-regulation of the excitatory amino acid transporter-2 (EAAT-2) involved in the reuptake of glutamate and the stimulation of the glutamate release from microglial cells, was also reported in the presence of Tat (Rumbaugh et al., 2007). More directly, Tat further promotes glutamate excitotoxicity by directly binding to or phosphorylating the glutamate N-methyl-D-aspartate (NMDA) receptor (Haughey et al., 2001; Prendergast et al., 2002; Song et al., 2003). The Cys30–31 motif in the clade B Tat protein interacts with the extracellular domain of the NR1 subunit of the NMDA receptor (Li et al., 2008). The mutation of Cys31 into Ser31 in HIV-1 Tat expressed by HIV-1 clade C and/or polymorphisms of the NMDA receptor subunits could explain the lesser neurotoxic activity of clade C Tat (Mishra et al., 2008; Rao et al., 2008; King et al., 2010; Eugenin et al., 2011). Interestingly, a recent study showed that Tat potentiates NMDA-evoked increases in intracellular Ca2+ concentration ([Ca2+]i) via the low-density lipoprotein receptor-related protein (LRP) and activation of Src tyrosine kinase, and mediated by a GTPase RhoA and Rho-associated protein kinase (ROCK; Krogh et al., 2015). However, potentiation is rapidly followed by a gradual adaptation and decrease of [Ca2+]i, resulting in neuronal protection from excessive calcium influx (Krogh et al., 2014, 2015). This suggests that even if Tat activates the NMDA receptor at picomolar concentrations (Li et al., 2008), Tat-mediated neurotoxicity through NMDA may require a certain activation threshold. Consistent with this, Tat inhibits glutamate-induced variation in intracellular Ca2+ in astrocytes (Koller et al., 2001), resulting in protection from death. Several studies using astrocyte cells to study the effects of Tat in HAND and/or the response to drugs of abuse may take advantage of this protection from death (Chauhan et al., 2003; Kim et al., 2003; Thomas et al., 2007; Mediouni et al., 2015).
The neurotransmitter dopamine (DA) plays important roles in motor control, motivation, reward, arousal, cognition, executive functions, and maternal behaviors (reviewed in Nutt et al., 2015). The impairment of DA neuronal functions correlates with early stage of HIV infection (Berger et al., 1994). Tat perturbs the normal signaling of DA transmission by inhibiting the activity of the DA transporter (DAT) through a concentration-dependent allosteric mechanism (Chan and Kantor, 2009; Zhu et al., 2009) without affecting DA release (reviewed in Turowski and Kenny, 2015). This prevents astrocytes from buffering DA and from triggering the release signal for neuroprotective molecules mediated through DAT (Silvers et al., 2007; Zhu et al., 2009, 2011; Perry et al., 2010; Miyazaki et al., 2011; Midde et al., 2012, 2013). Tat also inhibits the vesicular monoamine transporter (VMAT2) and the expression of tyrosine hydroxylase (TH, the rate limiting enzyme in DA biosynthesis), thus amplifying DA neurotoxicity (Zauli et al., 2000; reviewed in Everall et al., 2005; Midde et al., 2012, 2013; Theodore et al., 2012). These findings, associated with disturbances in the DAergic system are observed both in humans and in animals models (reviewed in Koutsilieri et al., 2002a,b; Jenuwein et al., 2004) and may have implications in the development of symptoms commonly observed in both Parkinson’s disease and in HAND (reviewed in Koutsilieri et al., 2002a,b).
Tat and Oxidative Stress
Low- and short-term reactive oxygen species (ROS) such as hydrogen peroxide (H2O2) and nitric oxide (NO) are important to cellular physiology as they participate in cell signaling in several biological processes (reviewed in D’Autreaux and Toledano, 2007; reviewed in Erusalimsky and Moncada, 2007). In the brain, ROS and NO interact in many ways to induce activation of brain cell glial subpopulations (Saha and Pahan, 2006a,b) and promote neuronal activity (Atkins and Sweatt, 1999). However, sustained high levels of ROS induce lipid peroxidation, protein oxidation and impairment of DNA synthesis and fidelity, events collectively called “oxidative stress” (reviewed in Pocernich et al., 2005; reviewed in Schieber and Chandel, 2014). Markers of oxidative stress are detected in brain and cerebrospinal fluid (CSF) of HAD patients (reviewed in Pocernich et al., 2005). Oxidative stress and neurodegeneration are secondary to glial activation and occur when ROS and NO approach inflammatory levels.
Tat has been shown to alter the production of ROS, in particular of reactive nitrogen species, leading to a loss of neuronal activity. Several studies in vivo and in vitro have shown an increase in the expression of intracellular NO synthase (NOS2) in brain cell subsets, resulting in an increase of NO following Tat exposure (Philippon et al., 1994; Bukrinsky et al., 1995; Jones et al., 1998; Liu et al., 2002; Kim et al., 2015). Excess NO may induce glutamate release from astrocytes (Bal-Price et al., 2002), thereby further hyperactivating NMDA receptors on neurons that, in turn, triggers further ROS-associated neuronal death (Capone et al., 2013). Moreover, in astrocytes exposed to Tat, a NOS2-dependent mechanism up-regulates adhesion molecules that induce recruitment of monocytes and viral replication (Song et al., 2011), consistent with the observation that elevated NO levels are associated with increased HIV-1 replication (reviewed in Torre et al., 2002). In parallel, Tat can induce the expression of factors that regulate its own activity over the HIV LTR, such as Nrf2 (nuclear erythroid 2 related factor 2). Nrf2 is a critical regulator of genes involved in the antioxidant response. Although the induction of Nrf2 by Tat is not enough to protect cells from oxidative stress in MAGI cell systems (Zhang et al., 2009), the ROS-induced expression in astrocytes has been reported to show a strong neuroprotective effect (Bell et al., 2011).
Other markers of oxidative stress such as protein carbonyl formation and lipid peroxidation in vitro and in vivo, as well as perturbation of the cellular antioxidant response have also been linked to Tat expression (Flores et al., 1993; Milani et al., 1996; Borgatti et al., 1998; Haughey et al., 1999, 2004; Choi et al., 2000; Aksenov et al., 2001; Richard et al., 2001; Pocernich et al., 2004; Agrawal et al., 2012; Kim et al., 2015).
Tat and Blood–Brain Barrier Disruption
The BBB is composed of brain endothelial cells connected by tight junctions and is supported by astrocytes. Tat and specific Tat peptides can passively cross the BBB in both directions (Banks et al., 2005). Tat can also use different mechanisms to decrease the expression of tight junction proteins and basement membrane extracellular matrix proteins by promoting the expression of inflammatory molecules, oxidative stress and the expression of the matrix metalloproteinase 9 (MMP-9, a zinc dependent endopeptidase; reviewed in Yong et al., 1998; Zidovetzki et al., 1998; Weiss et al., 1999; Pu et al., 2007; Ju et al., 2009; Banerjee et al., 2010; Song et al., 2011; Xu et al., 2012; Huang et al., 2014). Consistent with these effects, agonists of the peroxisome proliferator activated receptors (PPAR), a group of a nuclear receptor proteins mediating expression of anti-inflammatory and anti-oxidative genes, reduce Tat mediated BBB disruption in mice (Huang et al., 2014).
The level of BBB damage may vary depending on the Tat sequence variant found in different clades of the virus. Tat from the HIV-1 B subtype and Tat from isolates recovered from patients diagnosed with advanced HAD proved the most potent in disrupting the BBB (Figure 1B).
Of the molecules present on cells of the BBB that change upon exposure of Tat protein, P-glycoprotein (P-gp) is critical, since it is a pump involved in the transport of small molecule compounds out of the brain. Interestingly, the efflux function, expression and promoter activity of P-gp are up-regulated by Tat (Zhong et al., 2010). This may help explain why HIV-infected individuals maintained on suppressive therapy present low levels of ART in the brain (Gimenez et al., 2004; reviewed in Miller et al., 2008), with up-regulated P-gp removing ART from the CNS. A better understanding of the mechanism of action of Tat on P-gp may offer solutions to improve the efficiency of ART in the brain reservoir of HIV, not only for the enhancement of ART crossing the BBB, but also for improving the anti-viral performance of immune cells that express this protein (reviewed in Owen and Khoo, 2004).
Tat, Glial Cells, and Neuroinflammation
Markers of gliosis have been found in vitro after treatment of cells with either Tat or its neurotoxic domain. The same is true in vivo in the brain and CSF of HAD-affected individuals (Gallo et al., 1989; Tyor et al., 1992; Glass et al., 1993; Wesselingh et al., 1993; Philippon et al., 1994; Jones et al., 1998; Shi et al., 1998; Bruce-Keller et al., 2003; Kim et al., 2003; Turchan-Cholewo et al., 2009a,b; Puccini et al., 2015). Indeed, Tat induces the expression of important pro-inflammatory cytokines such as tumor necrosis factor α (TNF-α), interleukin-1β (IL-1β) and interleukin-6 (IL-6) (Ambrosino et al., 1997; Chen et al., 1997; Zidovetzki et al., 1998; Nath et al., 1999; Buscemi et al., 2007; reviewed in Li et al., 2010; Williams et al., 2010; Yang et al., 2010; Zhao et al., 2014; Mediouni et al., 2015), which make strong contributions to the development of an inflammatory neuropathology. For instance, TNF-α is associated with increased HIV-1 replication, NMDA receptor neurotoxicity and BBB disruption (Wright et al., 1988; Merrill et al., 1989; Stone, 1993; Fine et al., 1996; Heyes et al., 1996; Nuovo and Alfieri, 1996; Bezzi et al., 2001; Smith et al., 2001; reviewed in Brabers and Nottet, 2006; Buscemi et al., 2007; Wang et al., 2008; Zhong et al., 2008; Ju et al., 2009; Kiebala et al., 2010; Kiebala and Maggirwar, 2011). Likewise, IL-1β up-regulation, which leads to the transcription of several pro-inflammatory cytokines and chemokines, has been correlated with the severe neurologic symptoms of HAD patients (Gallo et al., 1989; reviewed in Allan et al., 2005; reviewed in Moynagh, 2005; reviewed in Brabers and Nottet, 2006). In the pathway that leads to Tat up-regulation of these two pro-inflammatory cytokines, targeting of the protein-tyrosine phosphatase (PTP), or CD45, was shown to attenuate microglia activation, suggesting a promising drug target (Jin et al., 2012). Additionally, elevation of IL-6 levels was reportedly associated with increased BBB permeability (Zidovetzki et al., 1998) and HIV replication (reviewed in Li et al., 2010). Tat-induced expression of inflammatory cytokines was also shown to involve other potential targets, such as the Ankyrin-rich membrane spanning protein (ARMS/Kidins220) in microglia cells (Singh et al., 2015) and the regulator of microglial phagocytosis (Leucine-rich repeat kinase 2; Marker et al., 2012).
In addition to the effect on cytokines, Tat modulates chemokine pathways in microglia, inducing neuroinflammation and changes in BBB permeability. Tat may bind to chemokine receptors through its cysteine/ZnF domain (a chemokine like sequence), thereby inhibiting the expression of CX3CR1 by suppressing a NF-kB-YY1 pathway, or inducing the expression of the potent chemoattractant chemokine MCP-1 (Fuentes et al., 1995; Albini et al., 1998; Weiss et al., 1999; Eugenin et al., 2003; Sheng et al., 2003; Bethel-Brown et al., 2011; Duan et al., 2014; Tewari et al., 2015). However, the induction MCP-1 by Tat may result in a complicated mode of action, since it has also been shown that MCP-1 protects both neurons and astrocytes from NMDA or Tat-induced apoptosis (Eugenin et al., 2003).
Tat and Apoptotic Pathways
As Tat influences processes of excitotoxicity, neuroinflammation and oxidative stress, it also modulates intrinsic cellular apoptotic pathways to variably promote both cellular death or protection. Apoptosis induced by Tat may be triggered by different mechanisms such as the disturbance of microtubule functions, changes of mitochondrial activity, reduction of the activity of the tumor suppressor p53 (involved in neuronal differentiation and survival), induction of reticulum stress, or triggering of the complex LRP/postsynaptic density protein-95/NMDA receptor/neuronal nitric oxide synthase (nNOS) at the neuronal membrane (reviewed in Roshal et al., 2001; Chen et al., 2002; Campbell et al., 2004; Pourroy et al., 2004; de Mareuil et al., 2005; Eugenin et al., 2007; Norman et al., 2007; Darbinian et al., 2008; Lecoeur et al., 2012; Liu et al., 2014; Ma et al., 2014b). On the other hand, infected cells from HIV infected patients Tat plays a critical role inducing pathways that inhibit autophagy, phagocytosis, or the expression of MHC molecules, as well as up-regulation of p73 (Chehimi et al., 1996; reviewed in Huigen et al., 2004; Amini et al., 2005; Saunders et al., 2005; reviewed in Li et al., 2010; Van Grol et al., 2010; Hui et al., 2012; Debaisieux et al., 2015). Thus, it is conceivable that Tat may cause the death of cells such as neurons and supportive glial subsets, while perversely preserving host infected cells, thereby contributing to the status of the brain as a reservoir at the cost of neurodegeneration.
Methamphetamine Neurotoxicity
Methamphetamine belongs to the amphetamine-type stimulant family. Used clinically at low doses to improve behavioral and cognitive functions after brain injury (Rau et al., 2012), MA is a strong psychostimulant that is widely abused as a “street drug.” Easily synthesized, relatively long lasting, and generally inexpensive in comparison to cocaine and heroin, MA is the second most commonly abused drug in the world (Figure 2A). Although MA seizure has increased dramatically in the last few years (Figure 2B), these factors have contributed to the increase of drug-associated health problems and social burden.
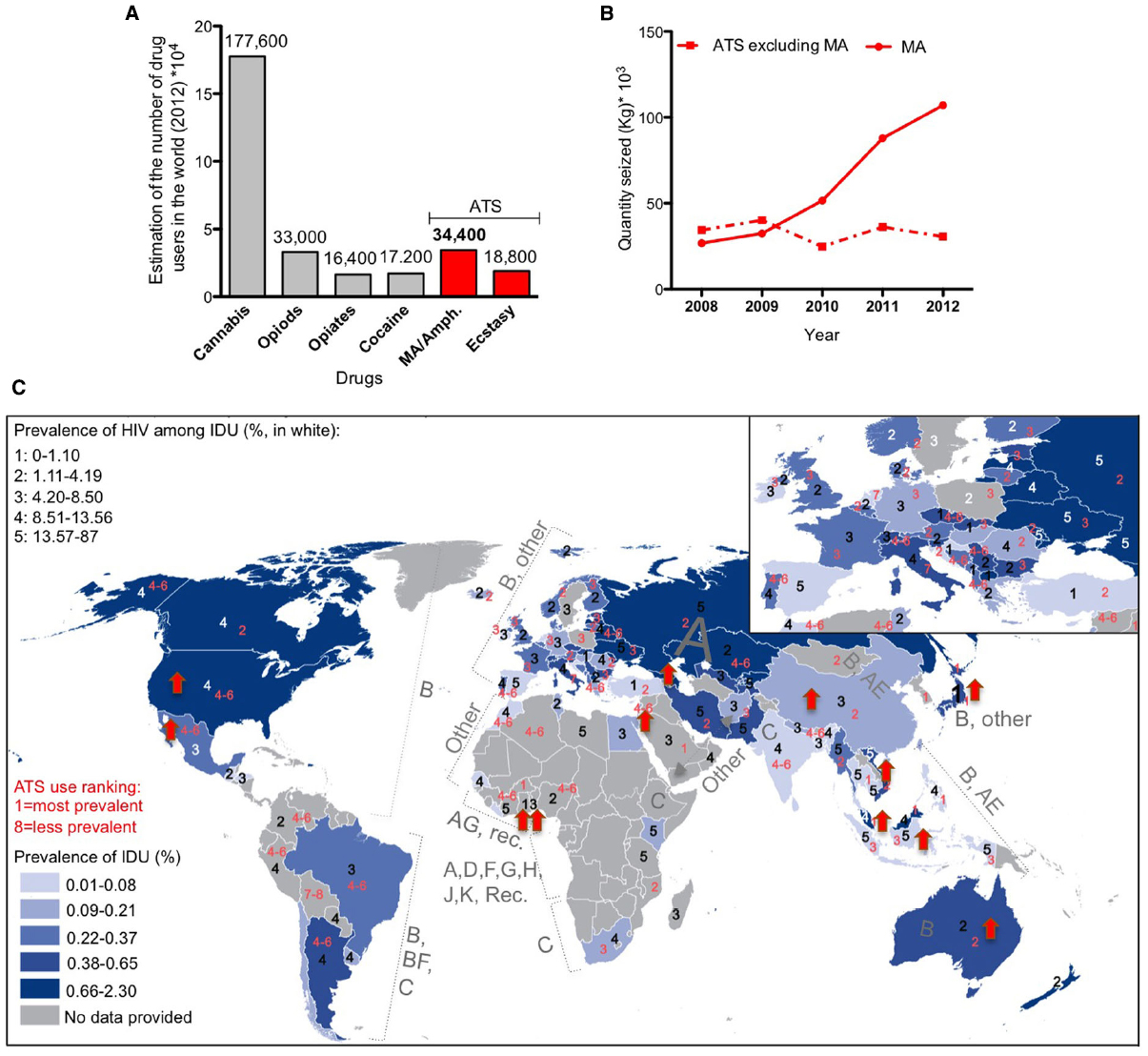
Figure 2. Worldwide distribution of methamphetamine (MA) use and HIV infection. (A) Worldwide estimation of drug use in 2012 (data from the world drug report 2014). ATS: amphetamine-type stimulants. (B) Total amount of seized drugs reported worldwide 2008–2012 (data from UNODC global synthetic drugs assessment 2014). (C) Superimposition of maps of the prevalence of intravenous drug use (IDU, map from the world drug report 2014) in 2012 with the prevalence of HIV infection among IDU in 2012 (numbers in white, data from the world drug report 2014), completed with the world distribution of HIV clades (data from Chan and Kantor, 2009) and the ranking of ATS use in 2012 (numbers in red, data from the world drug report 2014). Red arrow corresponds to an increase of more than 10% in MA/amphetamine drug use in 2011–2012 in the indicated country (data from the drug world report 2014).
Methamphetamine induces acute euphoria, the perception of limitless energy and high libido. Physiologically, it induces potentially lethal hyperthermia, due to both CNS and peripheral effects (Sanchez-Alavez et al., 2014). Chronic MA abuse leads to neuropsychiatric complications including memory impairment, attention deficit and psychomotor decay associated with neurotoxicity and neurological dysfunction, as well as significant dysregulation of neurotransmitters levels. Importantly, MA use correlates with enhanced risk-taking behaviors that may place drug abusers at a higher risk of exposure to infections such as Hepatitis C and HIV, each further contributing to CNS dysfunction. The impressive epidemiological overlap between intravenous drug use (IDU) and the population that is HIV-positive worldwide confirms this trend (Figure 2C).
Methamphetamine and Neurotransmitter Modulation
Although MA modulates a broad assortment of neuro-transmitters, MA-induced toxicity is largely attributed to hyperactivation of the DAergic system. MA increases extracellular DA levels, resulting in long-term degeneration of DAergic neurons, a mechanism also responsible for the motor impairments associated with Parkinson’s disease (reviewed in Riddle et al., 2006). MA exposure also promotes cognitive deficits related to DAergic neurodegeneration (reviewed in Riddle et al., 2006). Considerable neurochemical evidence of this neurotoxicity includes the demonstrated decrease of DAT and the activity of TH, a decrease of degradation of DA by monoamine oxidase (MAO), a reduction of activity and protein levels of VMAT2, and an increase of the reverse transport of DA via the DAT into the extracellular space (Gibb and Kogan, 1979; Zaczek et al., 1991; Giros et al., 1996; reviewed in Riddle et al., 2006; Eyerman and Yamamoto, 2007; Xie and Miller, 2009; reviewed in Silverstein et al., 2011; reviewed in Coller and Hutchinson, 2012). MA acts through the DA receptors (D1 and D2) and the TAAR1 receptor, but recent studies have also posited a role for other DA receptors specially the D3 involved in addiction, hyperthermia and modulation of the immune system (Ricci and Amenta, 1994; Ricci et al., 1997, 1998; Amenta et al., 1999; Ricci et al., 1999; Granado et al., 2011a, Ares-Santos et al., 2012; reviewed in Yu et al., 2015).
Following the increase of extracellular DA, MA causes a rise in striatal glutamate release (Nash and Yamamoto, 1992; Stephans and Yamamoto, 1994; Mark et al., 2004). Glutamate receptors, the EAAT-2 and VGLUT (the vesicular glutamate transporters, which are in charge of transporting glutamate into synaptic vesicles), are also involved in mediating different behaviors attributed to MA, such as reward, hyperlocomotor activity and, to some extent, hyperthermia (Golembiowska et al., 2003; Achat-Mendes et al., 2012a,b; reviewed in Cisneros and Ghorpade, 2012; Crawford et al., 2013; Herrold et al., 2013; He et al., 2014; Hiyoshi et al., 2014; Suryavanshi et al., 2014).
Independent from the DAergic system, MA also directly modulates the presynaptic release of glutamate in rat cultured hippocampal primary neurons (Zhang et al., 2014). Furthermore, serotonergic, γ-amino-butyric acid-ergic (GABAergic) and cholinergic systems are also altered by MA, highlighting the widespread damage caused by this drug (Halpin et al., 2014; reviewed in Northrop and Yamamoto, 2015; reviewed in Yu et al., 2015).
Methamphetamine and Oxidative Stress
Several in vivo and in vitro studies showed the increase of cytoplasmic and mitochondrial oxidative stress markers following acute or chronic exposition to MA (reviewed in Silverstein et al., 2011; Sanchez-Alavez et al., 2013; Halpin et al., 2014; Nam et al., 2014; Sanchez-Alavez et al., 2014; Solhi et al., 2014; Walker et al., 2014; reviewed in Yu et al., 2015). Importantly, several neurotoxicity hallmarks induced by MA are absent in animals that express the human superoxide dismutase 1 (SOD 1) gene, or that can otherwise quickly detoxify free radicals induced by MA (Cadet et al., 1994; Hirata et al., 1995, 1996, 1998; Deng and Cadet, 2000; Deng et al., 2001). In addition, antioxidant treatments have shown neuroprotective effects against MA-induced brain damage, suggesting a significant role for oxidative stress in MA toxicity (reviewed in Chen et al., 2010; Lecoeur et al., 2012; Barayuga et al., 2013; Koriem et al., 2013; reviewed in Liddie et al., 2013; Shin et al., 2014). Antioxidants are also able to prevent and reverse MA-induced hyperthermia, and MA-associated mitochondrial changes in animal models, suggesting that the thermal dysregulation in drug abuse, which is also reported as a trigger of neurotoxicity, involves the capacity of MA to induce ROS (Sanchez-Alavez et al., 2013, 2014).
The effects of MA are associated with cellular energy consumption and increased metabolism, potentially leading to mitochondrial dysfunction. Mitochondria functions are affected by the inhibition of ATP and the complex II of the electron transport chain (Burrows et al., 2000; Brown et al., 2005), mediated both by glutamate and NO (Brown et al., 2005). Moreover, ROS and quinone, a product of DA oxidation, are both known to inhibit mitochondrial enzymes associated with energy production (O’Shea et al., 2014). Therefore, the oxidative stress that results from MA abuse may have dramatic consequences for mitochondria metabolic efficiency.
Interestingly, unlike Tat, MA-induced astrogliosis was associated with the decrease of Nrf2 (Granado et al., 2011b), suggesting that astrocytes may have a low antioxidant capacity when exposed to MA.
Methamphetamine and Blood–Brain Barrier Disruption
Several preclinical and clinical studies reported BBB dysfunction by MA through different mechanisms (reviewed in Kousik et al., 2012; Matsumoto et al., 2014; reviewed in Northrop and Yamamoto, 2015; reviewed in Turowski and Kenny, 2015). MA-induced oxidative stress, hyperthermia, glutamate release and induction of inflammation combine to decrease tight junctions and transendothelial electric resistance via oxidative stress, down-regulate MMP expression mediated by hyperthermia, activate glutamate receptors, and increase the release of inflammatory cytokines as well as the expression of heat shock proteins (Ramirez et al., 2009; reviewed in Silverstein et al., 2011; reviewed in Kousik et al., 2012; Shah et al., 2012; Martins et al., 2013; Urrutia et al., 2013; reviewed in Northrop and Yamamoto, 2015). In addition, MA exposure has been correlated with a lack of the glucose-induced energy required for tight junction assembly and in the attenuation of brain capillary endothelial cell growth (Abdul Muneer et al., 2011; Fisher et al., 2015). Taken together, these mechanisms account for collective insults to the BBB that precedes neurological symptoms associated with chronic MA abuse.
Methamphetamine, Glial Cells, and Neuroinflammation
Modifications to the inflammatory status of CNS glial cells caused by MA exposure have strong implications that vary from biochemical alterations to behavioral changes. For instance, the release of cytokines by activated glial cells following MA exposure is correlated with behavioral effects such as high motor activity, MA self-administration and relapse, as well as hyperthermia (Boven et al., 2007; reviewed in Beardsley and Hauser, 2014; reviewed in Loftis and Janowsky, 2014; reviewed in Salamanca et al., 2014; reviewed in Yu et al., 2015). MA-induced DA and glutamate release can induce the activation of pattern recognition receptors (PRRs) in microglia that were shown to activate glial cells (Kuhn et al., 2006; reviewed in Beardsley and Hauser, 2014; Halpin et al., 2014; reviewed in Loftis and Janowsky, 2014).
Sigma receptors on glia and neurons have been recently implicated in MA-induced neuroinflammation. The sigma receptor is a chaperone protein at the endoplasmic reticulum that controls calcium signaling through the inositol triphosphate receptor. Various sigma receptor antagonists with different characteristics and potency have been characterized, and proven effective against neuroinflammatory activity (Kaushal et al., 2013, 2014; Zhang et al., 2015).
It has been suggested that voltage-gated potassium channels (Kv) are involved in neuronal damage as well as microglial function. Indeed, Kv1.3 is a major K+ channel expressed in microglia that has been previously involved in MA-induced microglia damage. MA significantly increases outward K+ currents and Kv1.3 antagonists increase microglial viability, while also decreasing the expression of neurotoxins and pro-inflammatory cytokines from these cells (Wang et al., 2014).
Interestingly, the pattern of microgliosis in individuals with a history of MA addiction has been shown to persist for at least 2 years into abstinence (Sekine et al., 2008). Likewise, astrocytes remain reactive up to 30 days following MA exposure. It is possible this is initially beneficial, turning maladaptive over time. In the short term, these effects may serve as a protection mechanism against MA-induced neurotoxicity (Hebert and O’Callaghan, 2000; Friend and Keefe, 2013; Robson et al., 2014). The release of neurotrophic factors, anti-inflammatory cytokines, and radical scavengers by glial cells to protect neurons from MA, have also been reported (reviewed in Beardsley and Hauser, 2014).
Methamphetamine and Apoptotic Pathways
The interaction between the excitotoxicity, oxidative stress, neuroinflammation and BBB disruption results in significant neuronal death in DA-rich regions (reviewed in Coller and Hutchinson, 2012; reviewed in Loftis and Janowsky, 2014; reviewed in Salamanca et al., 2014; reviewed in Yu et al., 2015). The mechanisms of apoptosis can be either caspase-dependent or -independent (Jayanthi et al., 2004). Even though MA-mediated neuronal apoptosis is very well documented, the apoptosis of other cells in the brain is less clearly established (Deng et al., 2001; Jayanthi et al., 2004; Halpin et al., 2014). MA induces the death of glial and oligodendrocytes through caspase-dependent pathways (Genc et al., 2003; Jumnongprakhon et al., 2014). Melatonin has been shown to rescue glial cells from death (Jumnongprakhon et al., 2014), an effect that might be anticipated because melatonin serves several immune-related functions such as the modulation of immune cell numbers, inhibition of pro-inflammatory cytokines, increased neurogenesis and a reduction of adhesion molecules (reviewed in Maldonado et al., 2009). As such, melatonin was suggested as a potential candidate to treat mental disorders and to possess potential in MA recovery therapies (reviewed in Maldonado et al., 2009).
On the other hand, MA was also associated with the autophagy process to protect neurons and endothelial cells (Castino et al., 2008; Fornai et al., 2008; Lin et al., 2012; Ma et al., 2014a). Interestingly, caffeine increases MA induced toxicity in neuroblastoma cell lines by inhibiting this autophagy process (Sinchai et al., 2011; Pitaksalee et al., 2015).
Tat and Methamphetamine Neurotoxicity
It is not surprising that a positive correlation exists between worldwide MA drug statistics (World drug report 2014) and the prevalence of HIV among intravenous drug users (Figure 2C). Interestingly, there is an increased tendency to MA/amphetamine consumption in countries that are predominantly affected with HIV-1 B subtype (Figure 2C), reported to be more neurotoxic (Figure 1B). Below we review some of studies that show Tat neurotoxicity enhanced by MA co-incubation.
Tat/Methamphetamine and HIV Replication
One important activity of MA in HIV disease progression is the ability to enhance viral replication. MA was shown to activate the promoter of HIV-1 in microglia cells, which Tat synergistically amplifies (Wires et al., 2012).
Methamphetamine dysregulation of the DA system augments viral production in different immune cells by promoting HIV entry and HIV transcription (Rohr et al., 1999a,b; Reynolds et al., 2007; Liang et al., 2008; Toussi et al., 2009; Marcondes et al., 2010; Nair and Saiyed, 2011; reviewed in Purohit et al., 2011). A review by Purohit et al. (2011) nicely illustrates the effects of DA in HIV transcription caused by oxidative stress and NF-κB activation in the presence of drugs of abuse in the brain (reviewed in Purohit et al., 2011). Inhibition of phagocytosis, disruption of pH and the reduced expression of Toll-like receptor 9 have each been proposed as mechanisms to explain the MA promotion of spreading HIV infection (Talloczy et al., 2008; Cen et al., 2013). In turn, the MA-induced elevation in DA levels seems to induce the migration of activated monocytes into the brain in the context of HIV (reviewed in Gaskill et al., 2013; Coley et al., 2015). This finding may be also correlated to the ability of MA in non-human primate to up-regulate C-C chemokine receptor type 5 (CCR5), the co-receptor for viral entry, in microglia cells, and in brain-derived macrophages which otherwise do not exhibit a classical inflammatory phenotype based on surface markers (Marcondes et al., 2010).
An interesting pathway with ties to the neuroinflammatory process triggered by MA that modulates infection is the Wnt/β-catenin signaling cascade, which regulates the survival process of neurons and astrocytes. In fact, astrocytes are the primary source of Wnt ligands, triggering the Wnt signaling cascade that mediates anti-inflammatory responses, neuroprotection and neurogenesis (Sharma et al., 2011). It has been reported that the Wnt/β-catenin/transcription factor 4 signaling pathway inhibits HIV replication in astrocytes. Co-incubation of primary human astrocytes with Tat and MA leads to an increased down-regulation of the Wnt signaling by more than 50% compared to the activity of MA or Tat alone. Given the additive effect of MA and Tat, it was suggested that the toxins may be engaging distinct pathways (Sharma et al., 2011).
Tat/Methamphetamine and Neurotransmitters Modulation
The effects of Tat and MA on neurotransmission have been described primarily in the context of the DA and GLUTergic systems. Cooperative effects of Tat and MA have been shown to enhance the DA mediated amplification of viral replication in correlation with the enhanced motor-stimulant response to a recurrent exposure to MA, a demonstration of behavioral sensitization (Liu et al., 2009). Moreover, Tat and MA showed in vivo a synergetic effect on the death of striatal DA terminals (Maragos et al., 2002; Cass et al., 2003; Theodore et al., 2006b,d; reviewed in Theodore et al., 2007).
Pre-pulse inhibition (PPI) is a neural mechanism in which a weaker pre-stimulus (pre-pulse) reduces the reaction to a following strong startling stimulus (pulse), and is used to measure adaptive inhibition mechanisms. This is thought to be a measure of sensorimotor gating and deficits have been reported in schizophrenia (Bertrand et al., 2014).
Patients with HAND demonstrate deficits in PPI responses not observed in HIV-positive participants without neurocognitive impairment (Greenwood et al., 2007). Similarly, intra-hippocampal infusion of Tat protein disrupts acoustic startle response and PPI in rats (Nath and Steiner, 2014). Among adult HIV-1 transgenic rats (that express seven of the nine clade B HIV-1 genes), Tat has a synergistic role in the increase of the MA-mediated disruption of PPI, an effect attributed to its ability to decrease TH while increasing MAO (Moran et al., 2012). Together, these findings suggest disruptions of DAergic signaling with behavioral and cognitive consequences.
In the GLUTergic system, the combination of MA and Tat was shown to be additive in enhancing the disruption of EAAT-2 in astrocytic cells. This leads to elevated glutamate levels in the synaptic clefts and a synergistic increase in midbrain neuronal death in vitro, in a process that requires both D1 and NMDA-receptors (Aksenov et al., 2012; reviewed in Cisneros and Ghorpade, 2012).
Tat/Methamphetamine and Oxidative Stress
Co-incubation of Tat and MA in cell culture in vitro has an additive effect promoting oxidative stress (Langford et al., 2004). Likewise, the combination in vivo results in a synergic/additive activation of redox system-regulated transcription factors, the increased expression of the intracellular adhesion molecule-1 (ICAM-1) gene, and of cytokines (TNF-α and IL-1β) in some brain regions (Flora et al., 2003). Tat and MA also have an additive effect in decreasing antioxidant enzyme levels (Banerjee et al., 2010). Other studies have shown that the combination of the HIV-1 envelope glycoprotein 120 (gp120) with Tat/MA also has the capacity of synergistically reducing the expression of tight junction proteins through a mechanism that seems to be dependent on oxidative stress, resulting in an impairment of the BBB (Banerjee et al., 2010). This report also demonstrates the possible synergy between the viral proteins in the induction of oxidative stress (Banerjee et al., 2010). On the other hand, a recent study has shown no significant concerted effects between HIV and substance of abuse in the increase of oxidative stress markers detected in the CSF of HIV-positive subjects, with or without substance use disorders (Panee et al., 2015). The differences between these studies may be due to different levels of Tat and/or MA used in the in vitro experiments and models, particularly in comparison to how much Tat is present in patients and their rate of MA usage. Tat in the serum of HIV-infected patients was detected at about 40 ng/ml (Xiao et al., 2000), and the concentration of MA in postmortem brains of chronic MA abusers is reportedly around 0.8–1 mM (Talloczy et al., 2008). However, relatively little work has been done on this topic, and the magnitude of the progression of HIV infection, the frequency of drug use, the composition of ART and the sensitivity of the assays may all influence the outcome of these studies.
Tat/Methamphetamine and Blood–Brain Barrier Disruption
Tat and MA synergistically and additively alter the BBB through the increase of cell adhesion molecules (ICAM-1), the decrease of the tight junction proteins and induction of MMP (Flora et al., 2003; Conant et al., 2004; Banerjee et al., 2010). Moreover, Kass et al. (2010) reported that MA-induced hyperthermia was enhanced in HIV transgenic rats (Kass et al., 2010), consistent with hyperthermia-induced BBB permeability (reviewed in Northrop and Yamamoto, 2015). It has been proposed that low non-toxic doses of Tat and MA could potentially be helpful in temporarily reducing the BBB permeability to enhance the access of antiretrovirals to the CNS (reviewed in Turowski and Kenny, 2015).
Tat/Methamphetamine, Glial Cells, and Neuroinflammation
As previously mentioned, Flora et al. (2003) showed that co-administration of Tat and MA resulted in the higher expression of the pro-inflammatory cytokines TNF-α and IL-1β, as well as the adhesion molecule ICAM-1 in several mouse brain regions (Flora et al., 2003). Theodore et al. (2006c) confirmed the involvement of TNF-α in depletion of DA levels, using a double KO for TNF-αR1 and TNF-αR2. The induction of TNF-α was also associated with a greater loss of hippocampal neurons (Theodore et al., 2006c,d).
A cytokine array performed on rat striatal tissue treated with a combination of MA and Tat revealed synergistically higher levels of MCP-1, timp metallo-peptidase inhibitor 1 (TIMP-1), and IL-1α. This activity was absent in MCP-1 KO mice, highlighting the importance of MCP-1 in Tat/MA neurotoxicity (Theodore et al., 2006a). These findings strongly suggest a crucial role for cytokines in mediating the interactions between MA and Tat in HIV-associated neurotoxicity.
Sharma et al. (2011) previously described the additive effect of Tat and MA in down-regulating the Wnt/β-catenin survival pathway (Sharma et al., 2011). In that study, the authors found that unlike Tat, MA also down-regulated NF-κB reporter activity, and as expected, decreased MCP-1 and IL-6 mRNAs. When Tat and MA were used in combination, the reduction of NF-κB reporter activity and MCP-1 mRNA persisted, but IL-6 expression was normalized. This seeming discrepancy could be explained by the fact that Tat can transactivate the IL-6 promoter by binding to IL-6 leader RNA (a stem loop structure located at the 5′-terminal region of the IL-6 mRNA) and by interacting and increasing the CAAT enhancer-binding protein (C/EBP) DNA binding activity (NF-IL-6) at the IL-6 promoter (reviewed in Li et al., 2010). Together, this suggests that the effect of both toxins together is not always positively correlated, as each toxin could trigger different pathways and produce unexpected phenotypes.
Tat/Methamphetamine and Apoptotic Pathways
The cooperation between Tat and MA in triggering BBB permeability, oxidative stress and increased viral replication may explain the severity of HAD symptoms in HIV-infected MA abusers, as compared to non-drug abusing patients (Nath et al., 2001; Sporer et al., 2005; reviewed in Ferris et al., 2008; reviewed in Purohit et al., 2011; reviewed in Silverstein et al., 2011; reviewed in Strazza et al., 2011; reviewed in Purohit et al., 2013; reviewed in Hauser and Knapp, 2014). This is confirmed in animal studies using the fully replicating virus in combination with MA, showing greater alteration of cognitive and locomotor behavior when compared with either treatment alone (Liu et al., 2009; Kass et al., 2010; reviewed in Silverstein et al., 2011; Kesby et al., 2015), an effect mediated by inflammation and oxidative stress processes (Maragos et al., 2002; Theodore et al., 2006a,b,c,d; Banerjee et al., 2010). The cognitive and locomotor decay may also be explained by the synergic activity of Tat and MA in triggering autophagy and apoptosis mechanisms. For instance, in presence of Tat and MA an increase of autophagosomes and/or multilamellar bodies was observed upon death of a neuroblastoma cell line (Cai and Cadet, 2008; Qi et al., 2011).
Melatonin has been shown to rescue glial cells from death and it was proposed to have potential in MA recovery therapies (reviewed in Maldonado et al., 2009; Jumnongprakhon et al., 2014). Tat was shown to up-regulate melatonin (Kaushal et al., 2014), with potentially resulting effects that remain to be assessed.
Caveats
Throughout this review, we have discussed different mechanisms by which Tat and MA may mediate neurocognitive and locomotor disorders (summarized in Figure 3). Nevertheless, these may represent just the tip of the proverbial iceberg, as Tat is known to have more than 775 interactions with cellular proteins, and thus may interact with many more aspects of cell regulation (Self et al., 2004). On the other hand, the extent of MA-induced cellular damage is also not fully understood (reviewed in Salamanca et al., 2014). It should be noted that other factors such as gender, nutrition, immunological strength and genetic variation could significantly change the neurologic outcomes of HAND in MA abusers.
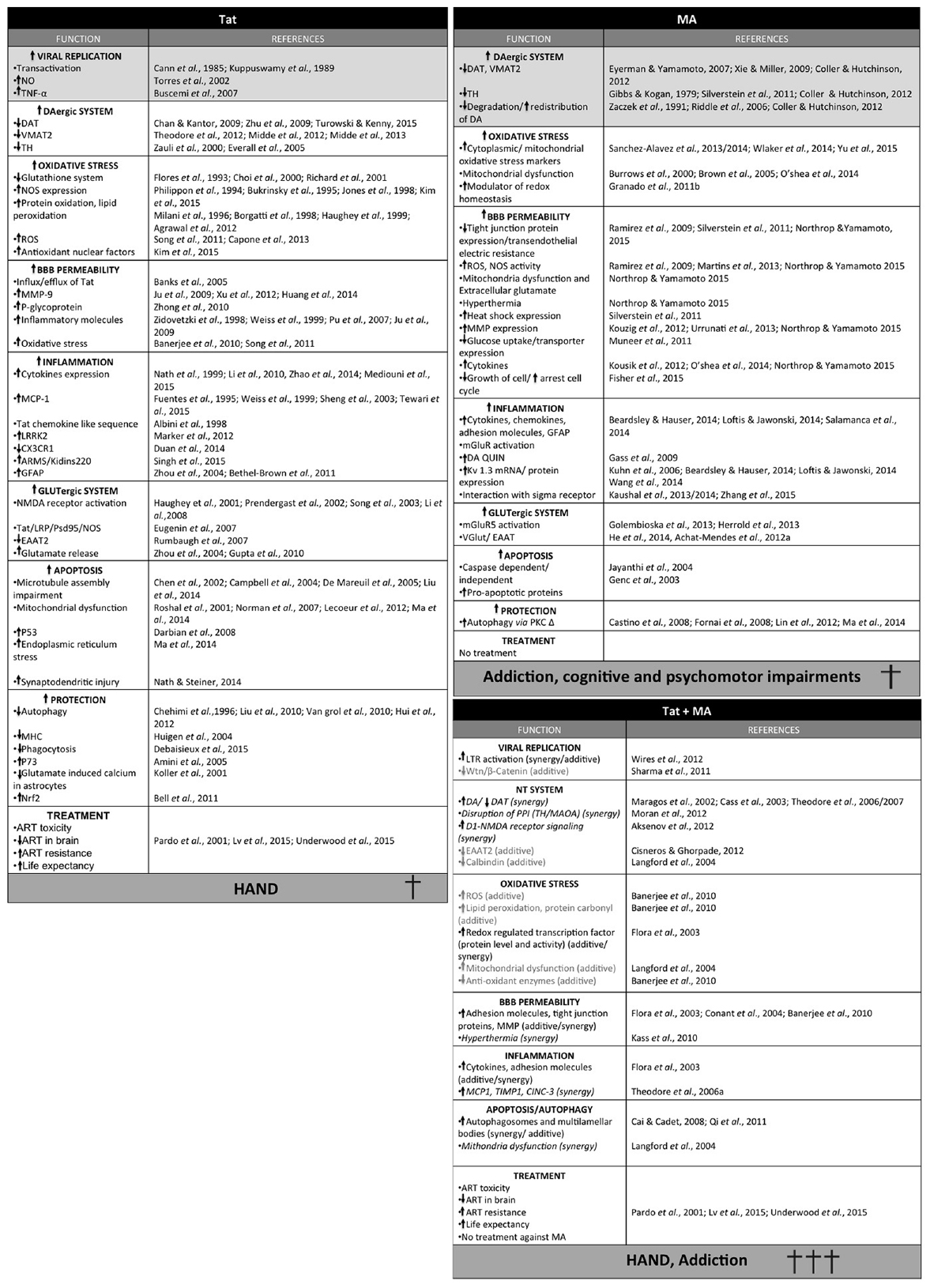
Figure 3. Summary of the different effects of Tat, methamphetamine (MA) or Tat and MA in combination. Synergic activity is shown in italic and additive effects in light gray. Light gray squares correspond to the main activity of each toxin.
Neurotoxicity by Methamphetamine Use and HIV-1 Infection: Potential Therapeutics
According to the CDC, as of 2010, the lifetime treatment cost of an HIV infected patient in the United States was an estimated $379,668. Moreover, the economical cost of substance abuse in the United States in terms of health care, job loss, corruption, imprisonment, and drug enforcement is more than $180 billion dollars a year (2008, NIDA). Unfortunately, the negative impact of HIV infection and drug abuse in our society is anticipated to deepen as the combined incidence of HAND, MA use, and HIV-1 infected MA abusers increases.
Under suppressive ART, HIV-1 patients are living longer, even as current evidence suggests their quality of life will degrade over time. Viral and human genetic variations, presence of latent cells and viral sanctuaries reduce the efficacy of ART (Aksenov et al., 2006). In addition, cofactors such as drug abuse significantly deepen HIV-induced neurological impairments.
As such, improving ART penetration across the BBB remains an important goal. The use of nano-ART (“cell-mediated medication delivery”; reviewed in Gendelman and Gelbard, 2014) or the use of intranasal delivery (reviewed in Hanson and Frey, 2007), have shown improved efficiency in that regard. The addition to ART of adjunctive therapies targeting the excitotoxicity, inflammatory or anti-oxidant systems is also being explored. Individually targeting Tat or MA is also another viable alternative. There are currently several ongoing clinical trials testing these approaches with MA-abusing HIV-1 patients to assess the inhibition of neurocognitive degradation or the decrease of MA use.
Current Clinical Trials in HIV Infected Methamphetamine Users: Adjunctive Therapies
Minocycline, a tetracycline antibiotic currently used to treat bacterial infections, is a potentially exciting therapeutic agent to treat brain disorders including HAND (Chen et al., 2000; Du et al., 2001; Sanchez Mejia et al., 2001; Arvin et al., 2002; Van Den Bosch et al., 2002; Wu et al., 2002; Metz et al., 2004). Minocycline possesses several therapeutic indications including antioxidant, anti-inflammatory, anti-viral, and anti-addictive proprieties, and it crosses the BBB. Using cultured primary macrophages and lymphocytes in vitro, minocycline has been shown to prevent NMDA-induced microglial proliferation and activation (Tikka and Koistinaho, 2001; Tikka et al., 2001) as well as HIV and SIV replication (Zink et al., 2005). Tested in vivo, minocycline prevents inflammation and HIV replication in central and peripheral systems (Zink et al., 2005; Singh et al., 2014). At the behavioral level, mouse studies have demonstrated that minocycline decreases the rewarding proprieties of dextroamphetamine (a stereoisomer of amphetamine), MA and ethanol (Agrawal et al., 2011; Sofuoglu et al., 2011; Fujita et al., 2012). Moreover, minocycline improves cognitive deficits associated with exposure to phencyclidine (NMDA receptor antagonist) or MA (Fujita et al., 2008; Mizoguchi et al., 2008).
Minocycline has been tested in three clinical trials (NCT00855062; NCT01064752; NCT00361257) related to HIV-1-associated cognitive impairments. In the only results available to date, the phase II clinical trial with minocycline (NCT00361257) showed that it was safe and well-tolerated by individuals with HAND. However, no cognitive improvement was observed (Sacktor et al., 2011). Intake of minocycline only resulted in a decrease in lipid markers of oxidative stress (Sacktor et al., 2014), a disappointing result given the promise of preclinical studies.
Ibudilast (AV-411 or MN-166) is a non-specific phosphodiesterase inhibitor, used in Japan to treat ischemic stroke and bronchial asthma (Gibson et al., 2006). Ibudilast inhibits the activation of glial cells and the expression of macrophage migration inhibitor factors (Suzumura et al., 1999, 2003; Cho et al., 2010). It was shown to attenuate microglia activation by inhibiting the expression of pro-inflammatory cytokines, inhibiting markers of oxidative stress and increasing the secretion of anti-inflammatory mediators, which prevent neuronal death and white matter injury in vivo (Wakita et al., 2003; Mizuno et al., 2004).
Interestingly, ibudilast inhibits Tat mediated TNF-α expression by microglial cells (Kiebala et al., 2010; Kiebala and Maggirwar, 2011). It also inhibits Tat induced HIV-neuronal loss in vitro in the presence or absence of drugs such as morphine (Kiebala and Maggirwar, 2011; El-Hage et al., 2014). Moreover, ibudilast was reported to significantly inhibit HIV replication in microglia cells (El-Hage et al., 2014).
Ibudilast also effectively protects against the glial activation responsible for modulating MA-induced behaviors, such as hyperlocomotion activity and MA self-administration, as well as MA relapse caused by stress or re-exposure to MA (reviewed in Caputo et al., 2009; Beardsley et al., 2010; Snider et al., 2012, 2013). Ibudilast and an Ibudilast-analog that is ineffective at inhibiting phosphodiesterase, similarly suppressed MA self-administration in rats (Cho et al., 2010; Snider et al., 2013), raising questions over the mechanism though which ibudilast works.
Currently, there are 10 clinical trials testing ibudilast. Of these, NCT01217970 is a completed phase I clinical trial demonstrating the safety of the compound in combination with MA, whereas NCT01860807 is an ongoing phase II trial on HIV-1 patients.
Other Adjunctive Drugs
Additional FDA approved compounds, currently effective for others brain disorders have been used in clinical trials for reducing MA use in HIV-1 patients such as aripiprazole, mirtazapin, and naltrexone.
Aripiprazole, which is a partial agonist at D2 receptors, is an antipsychotic used in the treatment of schizophrenia, bipolar disorder, depression and autism. It presented high toxicity when used in combination with ritonavir-boosted ART and cytochrome P450 inhibitor, reinforcing the need for caution when choosing the dosage and ART combination (Aung et al., 2010). In addition, depending on the dosage Aripiprazole has also been shown to increase MA-induced reward (Newton et al., 2008). In 2014, a phase II clinical trial (NCT00497055) was completed to test its efficacy to decrease the use of MA by HIV patients. However, the results are not yet available.
Mirtazapine, an antidepressant inhibitor of serotonin receptors, was reported to participate in the reduction of progressive multifocal leukoencephalopathy in one HIV-1 infected male (reviewed in Lasso and Ceron, 2012) and to reduce depression (Elliott and Roy-Byrne, 2000). It was used in a phase II trial in a cohort of HIV-1 men, which were actively using MA. In this study (NCT00497081), mirtazapine reduced MA usage and sexual risk.
Naltrexone, an opioid antagonist, was recently tested in a phase II trial (NCT01822132) in MA-using HIV patients to assess its ability to diminish MA-induced impulsive decision under observation with magnetic resonance imaging to determine functional correlate changes in brain. The results of this trial have not yet been made public.
Phase II clinical trials have been performed with this compound (NCT01449565; NCT00984360) for MA dependence on a cohort of men who have sex with men, due to the high percentage of this population in HIV infected MA abusers (NCT01449565), as well as on a cohort of individuals exhibiting the mutation A118 to G118 on the mu opioid receptor gene (NCT00984360). This mutation was shown to increase MA, alcohol and heroin addiction (Kuppuswamy et al., 1989; reviewed in Jeang et al., 1999; Peloponese et al., 2000; Chen et al., 2002; reviewed in Campbell and Loret, 2009). NCT00984360 was completed but the results are still not available, whereas, the NCT01449565 trial is still recruiting participants at the time of this review. In 2015, a study showed that naltrexone decreased the cue-induced craving and subjective responses to MA (Ray et al., 2015). This activity of the naltrexone had previously been observed with amphetamine and cocaine (Jayaram-Lindstrom et al., 2004, 2008a,b; Comer et al., 2013).
In parallel, several trials based on the management of HIV/MA are still ongoing1.
Promising Candidates
BBB alteration, excitotoxicity, oxidative stress, neuro-inflammation and apoptosis all result from the disruption of different physiological pathways by Tat and/or MA. The review of Rumbaugh et al. (2008) describes different pathways involving Tat in HAND, which are mostly used in MA activities (reviewed in Rumbaugh et al., 2008). Therapeutically targeting cellular pathways requires using caution with dosages, as normally expressed human proteins are involved. Alternatively, one can physically target each toxin individually, which may be a less cytotoxic alternative.
Tat
Triptolide and didehydro-Cortistatin A (dCA), target the N-terminus and the basic domain of Tat respectively. These are currently the most promising Tat-targeting compounds, with an activity in the nanomolar concentration range.
Triptolide
Triptolide, is a diterpenoid epoxide isolated from Tripterygium wilfordii, which inhibits HIV-1 transcription by accelerating Tat degradation. Triptolide was described to have anti-inflammatory, immunosuppressive and anti-tumor properties (Wan and Chen, 2014). It is currently in a phase III clinical trial (NCT02219672), being tested in combination with ART on HIV-1 infected Chinese individuals for its ability to reduce the HIV-1 reservoir size.
Didehydro-Cortistatin A
Didehydro-Cortistatin A, is an analog of a natural steroidal alkaloid isolated from a marine sponge, Corticium simplex. It inhibits Tat-mediated transactivation of the HIV integrated provirus by binding specifically to the TAR-binding domain of Tat (basic region; Mousseau et al., 2012; reviewed in Mousseau et al., 2015). dCA promotes sustained silencing of the HIV promoter without viral rebound even in the absence of ART in vitro. dCA was also shown to inhibit Tat induced neuroinflammation of astrocytes in vitro, as well as Tat-induced cocaine potentiation in vivo (Mediouni et al., 2015).
For currently ongoing clinical trials for Tat vaccines2.
Methamphetamine
Passive and active immunizations
Passive and active immunizations against drugs of abuse are expected to slow down entry of the drug of abuse inside the brain thus preventing drug-associated behaviors. MA vaccines are still in preclinical development and promote low and variable level of antibodies production with moderate affinity for MA (Hauber et al., 1989; Mabrouk et al., 1991; Sabatier et al., 1991; Mousseau et al., 2012; Hambuchen et al., 2015). Research based on conjugate vaccine and adjuvant optimization should enhance the efficiency of these vaccines. Passive immunization with the human-mouse chimeric monoclonal antibody, mAb7F9, is already in more advanced stages of development (Hambuchen et al., 2015). Mab7F9 was reported to specifically bind to MA in the nanomolar range and mediate MA clearance (Laurenzana et al., 2014; Stevens et al., 2014). mAb7F9 attenuated MA induced hyper-locomotion even after irregular treatment and challenge with higher MA doses (Hambuchen et al., 2014; Laurenzana et al., 2014). It also partially reduced addiction-related behavior (Harris et al., 2015). Combination of MA hapten-conjugate vaccine to this antibody for 4 months reduced the presence of MA in the brain (Hambuchen et al., 2015). In 2014, this mAb was the first MA mAb to be used in a phase I clinical study in MA abusers (NCT01603147). The study was completed and confirmed the safety of the antibody. One potential caveat of this approach is that patients could be expected to increase their drug intake to overcome the effects of the antibody.
Sigma receptors
Methamphetamine and cocaine have been shown to directly interact with sigma receptors at physiological relevant concent-rations (Nguyen et al., 2005; reviewed in Robson et al., 2012). Sigma receptors have been shown to be involved in MA induced neurotoxicity through a variety of mechanisms such as neuroinflammation, hyperthermia, apoptosis, modulation of neurotransmitters and oxidative stress (reviewed in Kaushal and Matsumoto, 2011). In addition, sigma receptors show no homology to other human proteins, supporting the development of sigma receptor antagonists therapeutics to treat MA abuse (reviewed in Matsumoto, 2009). Several antagonists with nanomolar affinity have been developed. For instance, pretreatment with CM156 prevents hyperthermia and hyper-locomotor effects of MA as well as monoamine depletion in mice (Kaushal et al., 2011b), whereas AZ66 has been shown to prevent both MA-induced locomotor stimulation and the behavioral sensitization resulting from repeated MA administration in mice (Seminerio et al., 2012). Recently, SN79, which has been shown to have good “drugable” proprieties (Kaushal et al., 2011a), has mitigated MA-induced oxidative stress (Kaushal et al., 2014), apoptosis (Kaushal et al., 2011a), neuroinflammation (astrogliosis and microgliosis; Robson et al., 2013, 2014), hyperthermia (Kaushal et al., 2013, 2014), and neurotransmitters modulation (Kaushal et al., 2013). Moreover, SN79 also reverses cocaine-induced convulsions, locomotor activity and prevents sensitization to cocaine (Kaushal et al., 2011a). However, at higher doses, SN79 induces sedation and motor incoordination (Kaushal et al., 2011a). Currently, none of the sigma receptors antagonists are clinically tested in HIV-infected MA abusers or MA abusers cohorts.
Caveats
Finding the ideal therapy to treat HAND on the increasingly aging population of HIV-infected individuals, drug abusers in particular, is a significant challenge, especially with restricted animal models that fully reflect both HAND and drug abuse toxicity. The ideal therapy should block the pleiotropic activities of the toxic agent(s) without cell-associated toxicity, penetrate the brain or retain the toxic agent in the blood stream (e.g., antibodies), have low posology, present a high threshold for resistance development, and importantly, be inexpensive. Personalized therapeutic strategies may also be envisaged, given the human genetic variation (e.g., the mu opioid mutation A118 to G118, the HIV-1 co-receptor CCR5 Δ32 deletion), drug of abuse-induced epigenetic variations and HIV-1 genome variation (Kuppuswamy et al., 1989; reviewed in Jeang et al., 1999; Peloponese et al., 2000; Chen et al., 2002; Daily et al., 2006; Li et al., 2008; reviewed in Campbell and Loret, 2009). However, the cost of these strategies may be a hurdle. The combination of potent specific compounds (and/or immunizations) to ART could constitute a first step in the treatment of HAND (see Figure 4, for an overview of pathways and potential therapeutic tools).
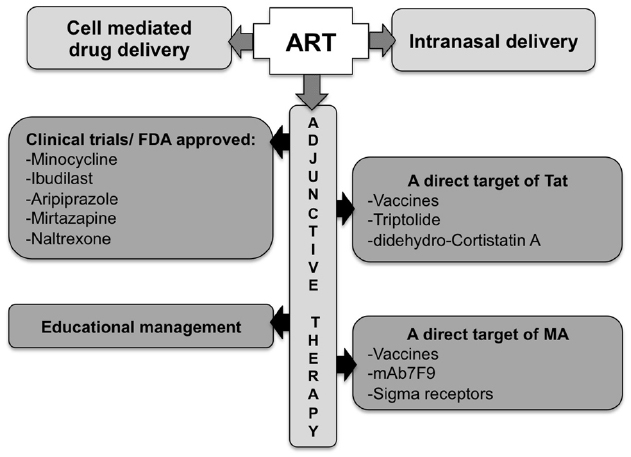
Figure 4. Potential approaches for the treatment of Tat/methamphetamine toxicity. The optimization of ART blood–brain barrier penetration may be achieved though a better route of administration, using carriers, or by including adjunctive therapy.
Conclusion
Although the biological effects of MA abuse and Tat protein have been extensively studied in the past, the result of their interaction is still not fully understood.
Methamphetamine abusers exhibit drug-related risk behaviors, such as needle sharing and unsafe sexual practices that increase the risk of acquiring an infectious disease. MA was shown to enhance HIV-1 replication, which may explain the rapid progression to AIDS of HIV infected MA users. On the other hand, Tat increases drug reward, which may partially explain the higher frequency of drug users in the HIV population.
Tat and MA act on homologous pathways, synergistically or additively, disturbing the same biological processes, including neurotransmitter pathways, inflammation, oxidative stress, and apoptosis. The outcome of this cross-interaction results in varying degrees of neurocognitive and locomotor disorders, that depend on patient complexity (reviewed in Valderas et al., 2009), HIV clade and drug use frequency. Compounds that target cellular pathways used for either Tat or MA-mediated cellular and behavioral activities, have the potential to reduce damage from both agents. Addition of these specific adjunctive compounds to ART is the most accepted treatment approach for HIV infected MA users. In addition, efforts on drug and HIV education and prevention, should be continuously provided.
Conflict of Interest Statement
The authors declare that the research was conducted in the absence of any commercial or financial relationships that could be construed as a potential conflict of interest.
Acknowledgments
SM and SV are supported by NIH grants #NIAID RO1 AI097012-01A1and R21AI116226-01. MM is supported by NIDA 5R01DA036164. MM would like to thank Floriska Chizer for administrative assistance.
Footnotes
- ^ https://clinicaltrials.gov/ct2/results?term=hiv+methamphetamine&Search+Search
- ^ https://clinicaltrials.gov/ct2/results?term=tat+vaccine&Search=Search
References
Abdul Muneer, P. M., Alikunju, S., Szlachetka, A. M., Murrin, L. C., and Haorah, J. (2011). Impairment of brain endothelial glucose transporter by methamphetamine causes blood-brain barrier dysfunction. Mol. Neurodegener 6, 23. doi: 10.1186/1750-1326-6-23
Achat-Mendes, C., Lynch, L. J., Sullivan, K. A., Vallender, E. J., and Miller, G. M. (2012a). Augmentation of methamphetamine-induced behaviors in transgenic mice lacking the trace amine-associated receptor 1. Pharmacol. Biochem. Behav. 101, 201–207. doi: 10.1016/j.pbb.2011.10.025
Achat-Mendes, C., Platt, D. M., and Spealman, R. D. (2012b). Antagonism of metabotropic glutamate 1 receptors attenuates behavioral effects of cocaine and methamphetamine in squirrel monkeys. J. Pharmacol. Exp. Ther. 343, 214–224. doi: 10.1124/jpet.112.196295
Agrawal, L., Louboutin, J. P., Reyes, B. A., Van Bockstaele, E. J., and Strayer, D. S. (2012). HIV-1 Tat neurotoxicity: a model of acute and chronic exposure, and neuroprotection by gene delivery of antioxidant enzymes. Neurobiol. Dis. 45, 657–670. doi: 10.1016/j.nbd.2011.10.005
Agrawal, R. G., Hewetson, A., George, C. M., Syapin, P. J., and Bergeson, S. E. (2011). Minocycline reduces ethanol drinking. Brain Behav. Immun. 25(Suppl. 1), S165–S169. doi: 10.1016/j.bbi.2011.03.002
Aksenov, M. Y., Aksenova, M. V., Mactutus, C. F., and Booze, R. M. (2009). Attenuated neurotoxicity of the transactivation-defective HIV-1 Tat protein in hippocampal cell cultures. Exp. Neurol. 219, 586–590. doi: 10.1016/j.expneurol.2009.07.005
Aksenov, M. Y., Aksenova, M. V., Mactutus, C. F., and Booze, R. M. (2012). D1/NMDA receptors and concurrent methamphetamine+ HIV-1 Tat neurotoxicity. J. Neuroimmune Pharmacol. 7, 599–608. doi: 10.1007/s11481-012-9362-3
Aksenov, M. Y., Aksenova, M. V., Nath, A., Ray, P. D., Mactutus, C. F., and Booze, R. M. (2006). Cocaine-mediated enhancement of Tat toxicity in rat hippocampal cell cultures: the role of oxidative stress and D1 dopamine receptor. Neurotoxicology 27, 217–228. doi: 10.1016/j.neuro.2005.10.003
Aksenov, M. Y., Hasselrot, U., Bansal, A. K., Wu, G., Nath, A., Anderson, C., et al. (2001). Oxidative damage induced by the injection of HIV-1 Tat protein in the rat striatum. Neurosci. Lett. 305, 5–8. doi: 10.1016/S0304-3940(01)01786-4
Albini, A., Ferrini, S., Benelli, R., Sforzini, S., Giunciuglio, D., Aluigi, M. G., et al. (1998). HIV-1 Tat protein mimicry of chemokines. Proc. Natl. Acad. Sci. U.S.A. 95, 13153–13158.
Allan, S. M., Tyrrell, P. J., and Rothwell, N. J. (2005). Interleukin-1 and neuronal injury. Nat. Rev. Immunol. 5, 629–640. doi: 10.1038/nri1664
Ambrosino, C., Ruocco, M. R., Chen, X., Mallardo, M., Baudi, F., Trematerra, S., et al. (1997). HIV-1 Tat induces the expression of the interleukin-6 (IL6) gene by binding to the IL6 leader RNA and by interacting with CAAT enhancer-binding protein beta (NF-IL6) transcription factors. J. Biol. Chem. 272, 14883–14892.
Amenta, F., Bronzetti, E., Felici, L., Ricci, A., and Tayebati, S. K. (1999). Dopamine D2-like receptors on human peripheral blood lymphocytes: a radioligand binding assay and immunocytochemical study. J. Auton Pharmacol. 19, 151–159.
Amini, S., Mameli, G., Del Valle, L., Skowronska, A., Reiss, K., Gelman, B. B., et al. (2005). p73 Interacts with human immunodeficiency virus type 1 Tat in astrocytic cells and prevents its acetylation on lysine 28. Mol. Cell. Biol. 25, 8126–8138. doi: 10.1128/mcb.25.18.8126-8138.2005
Antinori, A., Arendt, G., Becker, J. T., Brew, B. J., Byrd, D. A., Cherner, M., et al. (2007). Updated research nosology for HIV-associated neurocognitive disorders. Neurology 69, 1789–1799. doi: 10.1212/01.WNL.0000287431.88658.8b
Ares-Santos, S., Granado, N., Oliva, I., O’Shea, E., Martin, E. D., Colado, M. I., et al. (2012). Dopamine D(1) receptor deletion strongly reduces neurotoxic effects of methamphetamine. Neurobiol. Dis. 45, 810–820. doi: 10.1016/j.nbd.2011.11.005
Arvin, K. L., Han, B. H., Du, Y., Lin, S. Z., Paul, S. M., and Holtzman, D. M. (2002). Minocycline markedly protects the neonatal brain against hypoxic-ischemic injury. Ann. Neurol. 52, 54–61. doi: 10.1002/ana.10242
Atkins, C. M., and Sweatt, J. D. (1999). Reactive oxygen species mediate activity-dependent neuron-glia signaling in output fibers of the hippocampus. J. Neurosci. 19, 7241–7248.
Aung, G. L., O’brien, J. G., Tien, P. G., and Kawamoto, L. S. (2010). Increased aripiprazole concentrations in an HIV-positive male concurrently taking duloxetine, darunavir, and ritonavir. Ann. Pharmacother. 44, 1850–1854. doi: 10.1345/aph.1P139
Bagashev, A., and Sawaya, B. E. (2013). Roles and functions of HIV-1 Tat protein in the CNS: an overview. Virol. J. 10, 358. doi: 10.1186/1743-422x-10-358
Bal-Price, A., Moneer, Z., and Brown, G. C. (2002). Nitric oxide induces rapid, calcium-dependent release of vesicular glutamate and ATP from cultured rat astrocytes. Glia 40, 312–323. doi: 10.1002/glia.10124
Banerjee, A., Zhang, X., Manda, K. R., Banks, W. A., and Ercal, N. (2010). HIV proteins (gp120 and Tat) and methamphetamine in oxidative stress-induced damage in the brain: potential role of the thiol antioxidant N-acetylcysteine amide. Free Radic Biol. Med. 48, 1388–1398. doi: 10.1016/j.freeradbiomed.2010.02.023
Banks, W. A., Robinson, S. M., and Nath, A. (2005). Permeability of the blood-brain barrier to HIV-1 Tat. Exp. Neurol. 193, 218–227. doi: 10.1016/j.expneurol.2004.11.019
Barayuga, S. M., Pang, X., Andres, M. A., Panee, J., and Bellinger, F. P. (2013). Methamphetamine decreases levels of glutathione peroxidases 1 and 4 in SH-SY5Y neuronal cells: protective effects of selenium. Neurotoxicology 37, 240–246. doi: 10.1016/j.neuro.2013.05.009
Beardsley, P. M., and Hauser, K. F. (2014). Glial modulators as potential treatments of psychostimulant abuse. Adv. Pharmacol. 69, 1–69. doi: 10.1016/b978-0-12-420118-7.00001-9
Beardsley, P. M., Shelton, K. L., Hendrick, E., and Johnson, K. W. (2010). The glial cell modulator and phosphodiesterase inhibitor, AV411 (ibudilast), attenuates prime- and stress-induced methamphetamine relapse. Eur. J. Pharmacol. 637, 102–108. doi: 10.1016/j.ejphar.2010.04.010
Bell, K. F., Al-Mubarak, B., Fowler, J. H., Baxter, P. S., Gupta, K., Tsujita, T., et al. (2011). Mild oxidative stress activates Nrf2 in astrocytes, which contributes to neuroprotective ischemic preconditioning. Proc. Natl. Acad. Sci. U.S.A. 108, E1–E2; author reply E3–E4. doi: 10.1073/pnas.1015229108
Berger, J. R., Kumar, M., Kumar, A., Fernandez, J. B., and Levin, B. (1994). Cerebrospinal fluid dopamine in HIV-1 infection. AIDS 8, 67–71.
Bertrand, S. J., Mactutus, C. F., Aksenova, M. V., Espensen-Sturges, T. D., and Booze, R. M. (2014). Synaptodendritic recovery following HIV Tat exposure: neurorestoration by phytoestrogens. J. Neurochem. 128, 140–151. doi: 10.1111/jnc.12375
Bethel-Brown, C., Yao, H., Callen, S., Lee, Y. H., Dash, P. K., Kumar, A., et al. (2011). HIV-1 Tat-mediated induction of platelet-derived growth factor in astrocytes: role of early growth response gene 1. J. Immunol. 186, 4119–4129. doi: 10.4049/jimmunol.1002235
Bezzi, P., Domercq, M., Brambilla, L., Galli, R., Schols, D., De Clercq, E., et al. (2001). CXCR4-activated astrocyte glutamate release via TNFalpha: amplification by microglia triggers neurotoxicity. Nat. Neurosci. 4, 702–710. doi: 10.1038/89490
Borgatti, P., Zauli, G., Cantley, L. C., and Capitani, S. (1998). Extracellular HIV-1 Tat protein induces a rapid and selective activation of protein kinase C (PKC)-alpha, and -epsilon and -zeta isoforms in PC12 cells. Biochem. Biophys. Res. Commun. 242, 332–337.
Boven, L. A., Noorbakhsh, F., Bouma, G., Van Der Zee, R., Vargas, D. L., Pardo, C., et al. (2007). Brain-derived human immunodeficiency virus-1 Tat exerts differential effects on LTR transactivation and neuroimmune activation. J. Neurovirol. 13, 173–184. doi: 10.1080/13550280701258399
Brabers, N. A., and Nottet, H. S. (2006). Role of the pro-inflammatory cytokines TNF-alpha and IL-1beta in HIV-associated dementia. Eur. J. Clin. Invest. 36, 447–458. doi: 10.1111/j.1365-2362.2006.01657.x
Brown, J. M., Quinton, M. S., and Yamamoto, B. K. (2005). Methamphetamine-induced inhibition of mitochondrial complex II: roles of glutamate and peroxynitrite. J. Neurochem. 95, 429–436. doi: 10.1111/j.1471-4159.2005.03379.x
Bruce-Keller, A. J., Chauhan, A., Dimayuga, F. O., Gee, J., Keller, J. N., and Nath, A. (2003). Synaptic transport of human immunodeficiency virus-Tat protein causes neurotoxicity and gliosis in rat brain. J. Neurosci. 23, 8417–8422.
Bukrinsky, M. I., Nottet, H. S., Schmidtmayerova, H., Dubrovsky, L., Flanagan, C. R., Mullins, M. E., et al. (1995). Regulation of nitric oxide synthase activity in human immunodeficiency virus type 1 (HIV-1)-infected monocytes: implications for HIV-associated neurological disease. J. Exp. Med. 181, 735–745.
Burrows, K. B., Gudelsky, G., and Yamamoto, B. K. (2000). Rapid and transient inhibition of mitochondrial function following methamphetamine or 3,4-methylenedioxymethamphetamine administration. Eur. J. Pharmacol. 398, 11–18. doi: 10.1016/S0014-2999(00)00264-8
Buscemi, L., Ramonet, D., and Geiger, J. D. (2007). Human immunodeficiency virus type-1 protein Tat induces tumor necrosis factor-alpha-mediated neurotoxicity. Neurobiol. Dis. 26, 661–670. doi: 10.1016/j.nbd.2007.03.004
Cadet, J. L., Sheng, P., Ali, S., Rothman, R., Carlson, E., and Epstein, C. (1994). Attenuation of methamphetamine-induced neurotoxicity in copper/zinc superoxide dismutase transgenic mice. J. Neurochem. 62, 380–383.
Cai, N. S., and Cadet, J. L. (2008). The combination of methamphetamine and of the HIV protein, Tat, induces death of the human neuroblastoma cell line, SH-SY5Y. Synapse 62, 551–552. doi: 10.1002/syn.20512
Campbell, G. R., and Loret, E. P. (2009). What does the structure-function relationship of the HIV-1 Tat protein teach us about developing an AIDS vaccine? Retrovirology 6, 50. doi: 10.1186/1742-4690-6-50
Campbell, G. R., Pasquier, E., Watkins, J., Bourgarel-Rey, V., Peyrot, V., Esquieu, D., et al. (2004). The glutamine-rich region of the HIV-1 Tat protein is involved in T-cell apoptosis. J. Biol. Chem. 279, 48197–48204. doi: 10.1074/jbc.M406195200
Cann, A. J., Rosenblatt, J. D., Wachsman, W., Shah, N. P., and Chen, I. S. (1985). Identification of the gene responsible for human T-cell leukaemia virus transcriptional regulation. Nature 318, 571–574.
Capone, C., Cervelli, M., Angelucci, E., Colasanti, M., Macone, A., Mariottini, P., et al. (2013). A role for spermine oxidase as a mediator of reactive oxygen species production in HIV-Tat-induced neuronal toxicity. Free Radic Biol. Med. 63, 99–107. doi: 10.1016/j.freeradbiomed.2013.05.007
Caputo, A., Gavioli, R., Bellino, S., Longo, O., Tripiciano, A., Francavilla, V., et al. (2009). HIV-1 Tat-based vaccines: an overview and perspectives in the field of HIV/AIDS vaccine development. Int. Rev. Immunol. 28, 285–334. doi: 10.1080/08830180903013026
Cass, W. A., Harned, M. E., Peters, L. E., Nath, A., and Maragos, W. F. (2003). HIV-1 protein Tat potentiation of methamphetamine-induced decreases in evoked overflow of dopamine in the striatum of the rat. Brain Res. 984, 133–142. doi: 10.1016/S0006-8993(03)03122-6
Castino, R., Lazzeri, G., Lenzi, P., Bellio, N., Follo, C., Ferrucci, M., et al. (2008). Suppression of autophagy precipitates neuronal cell death following low doses of methamphetamine. J. Neurochem. 106, 1426–1439. doi: 10.1111/j.1471-4159.2008.05488.x
Cen, P., Ye, L., Su, Q. J., Wang, X., Li, J. L., Lin, X. Q., et al. (2013). Methamphetamine inhibits Toll-like receptor 9-mediated anti-HIV activity in macrophages. AIDS Res. Hum. Retrovir. 29, 1129–1137. doi: 10.1089/aid.2012.0264
Chan, P. A., and Kantor, R. (2009). Transmitted drug resistance in nonsubtype B HIV-1 infection. HIV Ther. 3, 447–465. doi: 10.2217/hiv.09.30
Chauhan, A., Turchan, J., Pocernich, C., Bruce-Keller, A., Roth, S., Butterfield, D. A., et al. (2003). Intracellular human immunodeficiency virus Tat expression in astrocytes promotes astrocyte survival but induces potent neurotoxicity at distant sites via axonal transport. J. Biol. Chem. 278, 13512–13519. doi: 10.1074/jbc.M209381200
Chehimi, J., Ma, X., Chouaib, S., Zyad, A., Nagashunmugam, T., Wojcik, L., et al. (1996). Differential production of interleukin 10 during human immunodeficiency virus infection. AIDS Res. Hum. Retrovir. 12, 1141–1149.
Chen, C. H., Howng, S. L., Hwang, S. L., Chou, C. K., Liao, C. H., and Hong, Y. R. (2000). Differential expression of four human dynamin-like protein variants in brain tumors. DNA Cell Biol. 19, 189–194. doi: 10.1089/104454900314573
Chen, D., Wang, M., Zhou, S., and Zhou, Q. (2002). HIV-1 Tat targets microtubules to induce apoptosis, a process promoted by the pro-apoptotic Bcl-2 relative Bim. EMBO J. 21, 6801–6810. doi: 10.1093/emboj/cdf683
Chen, H., Wu, J., Zhang, J., and Hashimoto, K. (2010). Recent topics on pharmacotherapy for amphetamine-type stimulants abuse and dependence. Curr. Drug Abuse Rev. 3, 222–238. doi: 10.2174/1874473711003040222
Chen, P., Mayne, M., Power, C., and Nath, A. (1997). The Tat protein of HIV-1 induces tumor necrosis factor-alpha production. Implications for HIV-1-associated neurological diseases. J. Biol. Chem. 272, 22385–22388.
Cho, Y., Crichlow, G. V., Vermeire, J. J., Leng, L., Du, X., Hodsdon, M. E., et al. (2010). Allosteric inhibition of macrophage migration inhibitory factor revealed by ibudilast. Proc. Natl. Acad. Sci. U.S.A. 107, 11313–11318. doi: 10.1073/pnas.1002716107
Choi, J., Liu, R. M., Kundu, R. K., Sangiorgi, F., Wu, W., Maxson, R., et al. (2000). Molecular mechanism of decreased glutathione content in human immunodeficiency virus type 1 Tat-transgenic mice. J. Biol. Chem. 275, 3693–3698. doi: 10.1074/jbc.275.5.3693
Cisneros, I. E., and Ghorpade, A. (2012). HIV-1, methamphetamine and astrocyte glutamate regulation: combined excitotoxic implications for neuro-AIDS. Curr. HIV Res. 10, 392–406. doi: 10.2174/157016212802138832
Coley, J. S., Calderon, T. M., Gaskill, P. J., Eugenin, E. A., and Berman, J. W. (2015). Dopamine increases CD14+CD16+ monocyte migration and adhesion in the context of substance abuse and HIV neuropathogenesis. PLoS ONE 10:e0117450. doi: 10.1371/journal.pone.0117450
Coller, J. K., and Hutchinson, M. R. (2012). Implications of central immune signaling caused by drugs of abuse: mechanisms, mediators and new therapeutic approaches for prediction and treatment of drug dependence. Pharmacol. Ther. 134, 219–245. doi: 10.1016/j.pharmthera.2012.01.008
Comer, S. D., Mogali, S., Saccone, P. A., Askalsky, P., Martinez, D., Walker, E. A., et al. (2013). Effects of acute oral naltrexone on the subjective and physiological effects of oral D-amphetamine and smoked cocaine in cocaine abusers. Neuropsychopharmacology 38, 2427–2438. doi: 10.1038/npp.2013.143
Conant, K., St Hillaire, C., Anderson, C., Galey, D., Wang, J., and Nath, A. (2004). Human immunodeficiency virus type 1 Tat and methamphetamine affect the release and activation of matrix-degrading proteinases. J. Neurovirol. 10, 21–28. doi: 10.1080/13550280490261699
Crawford, J. T., Roberts, D. C., and Beveridge, T. J. (2013). The group II metabotropic glutamate receptor agonist, LY379268, decreases methamphetamine self-administration in rats. Drug Alcohol. Depend. 132, 414–419. doi: 10.1016/j.drugalcdep.2013.07.024
D’Autreaux, B., and Toledano, M. B. (2007). ROS as signalling molecules: mechanisms that generate specificity in ROS homeostasis. Nat. Rev. Mol. Cell Biol. 8, 813–824. doi: 10.1038/nrm2256
Daily, A., Nath, A., and Hersh, L. B. (2006). Tat peptides inhibit neprilysin. J. Neurovirol. 12, 153–160. doi: 10.1080/13550280600760677
Darbinian, N., Darbinyan, A., Czernik, M., Peruzzi, F., Khalili, K., Reiss, K., et al. (2008). HIV-1 Tat inhibits NGF-induced Egr-1 transcriptional activity and consequent p35 expression in neural cells. J. Cell. Physiol. 216, 128–134. doi: 10.1002/jcp.21382
Davis, L. E., Hjelle, B. L., Miller, V. E., Palmer, D. L., Llewellyn, A. L., Merlin, T. L., et al. (1992). Early viral brain invasion in iatrogenic human immunodeficiency virus infection. Neurology 42, 1736–1739.
Debaisieux, S., Lachambre, S., Gross, A., Mettling, C., Besteiro, S., Yezid, H., et al. (2015). HIV-1 Tat inhibits phagocytosis by preventing the recruitment of Cdc42 to the phagocytic cup. Nat. Commun. 6, 6211. doi: 10.1038/ncomms7211
Del Valle, L., Croul, S., Morgello, S., Amini, S., Rappaport, J., and Khalili, K. (2000). Detection of HIV-1 Tat and JCV capsid protein, VP1, in AIDS brain with progressive multifocal leukoencephalopathy. J. Neurovirol. 6, 221–228. doi: 10.3109/13550280009015824
de Mareuil, J., Carre, M., Barbier, P., Campbell, G. R., Lancelot, S., Opi, S., et al. (2005). HIV-1 Tat protein enhances microtubule polymerization. Retrovirology 2, 5. doi: 10.1186/1742-4690-2-5
Deng, X., and Cadet, J. L. (2000). Methamphetamine-induced apoptosis is attenuated in the striata of copper-zinc superoxide dismutase transgenic mice. Brain Res. Mol. Brain Res. 83, 121–124. doi: 10.1016/S0169-328X(00)00169-8
Deng, X., Wang, Y., Chou, J., and Cadet, J. L. (2001). Methamphetamine causes widespread apoptosis in the mouse brain: evidence from using an improved TUNEL histochemical method. Brain Res. Mol. Brain Res. 93, 64–69. doi: 10.1016/S0169-328X(01)00184-X
Duan, M., Yao, H., Cai, Y., Liao, K., Seth, P., and Buch, S. (2014). HIV-1 Tat disrupts CX3CL1-CX3CR1 axis in microglia via the NF-kappaBYY1 pathway. Curr. HIV Res. 12, 189–200. doi: 10.2174/1570162X12666140526123119
Du, Y., Ma, Z., Lin, S., Dodel, R. C., Gao, F., Bales, K. R., et al. (2001). Minocycline prevents nigrostriatal dopaminergic neurodegeneration in the MPTP model of Parkinson’s disease. Proc. Natl. Acad. Sci. U.S.A. 98, 14669–14674. doi: 10.1073/pnas.251341998
El-Hage, N., Rodriguez, M., Podhaizer, E. M., Zou, S., Dever, S. M., Snider, S. E., et al. (2014). Ibudilast (AV411), and its AV1013 analog, reduce HIV-1 replication and neuronal death induced by HIV-1 and morphine. AIDS 28, 1409–1419. doi: 10.1097/qad.0000000000000291
Elliott, A. J., and Roy-Byrne, P. P. (2000). Mirtazapine for depression in patients with human immunodeficiency virus. J. Clin. Psychopharmacol. 20, 265–267. doi: 10.1097/00004714-200004000-00023
Ellis, R., Langford, D., and Masliah, E. (2007). HIV and antiretroviral therapy in the brain: neuronal injury and repair. Nat. Rev. Neurosci. 8, 33–44. doi: 10.1038/nrn2040
Erusalimsky, J. D., and Moncada, S. (2007). Nitric oxide and mitochondrial signaling: from physiology to pathophysiology. Arterioscler. Thromb. Vasc. Biol. 27, 2524–2531. doi: 10.1161/ATVBAHA.107.151167
Eugenin, E. A., D’aversa, T. G., Lopez, L., Calderon, T. M., and Berman, J. W. (2003). MCP-1 (CCL2) protects human neurons and astrocytes from NMDA or HIV-tat-induced apoptosis. J. Neurochem. 85, 1299–1311. doi: 10.1046/j.1471-4159.2003.01775.x
Eugenin, E. A., King, J. E., Hazleton, J. E., Major, E. O., Bennett, M. V., Zukin, R. S., et al. (2011). Differences in NMDA receptor expression during human development determine the response of neurons to HIV-tat-mediated neurotoxicity. Neurotox. Res. 19, 138–148. doi: 10.1007/s12640-010-9150-x
Eugenin, E. A., King, J. E., Nath, A., Calderon, T. M., Zukin, R. S., Bennett, M. V., et al. (2007). HIV-tat induces formation of an LRP-PSD-95- NMDAR-nNOS complex that promotes apoptosis in neurons and astrocytes. Proc. Natl. Acad. Sci. U.S.A. 104, 3438–3443. doi: 10.1073/pnas.0611699104
Everall, I. P., Hansen, L. A., and Masliah, E. (2005). The shifting patterns of HIV encephalitis neuropathology. Neurotox. Res. 8, 51–61. doi: 10.1007/BF03033819
Eyerman, D. J., and Yamamoto, B. K. (2007). A rapid oxidation and persistent decrease in the vesicular monoamine transporter 2 after methamphetamine. J. Neurochem. 103, 1219–1227. doi: 10.1111/j.1471-4159.2007.04837.x
Ferris, M. J., Mactutus, C. F., and Booze, R. M. (2008). Neurotoxic profiles of HIV, psychostimulant drugs of abuse, and their concerted effect on the brain: current status of dopamine system vulnerability in NeuroAIDS. Neurosci. Biobehav. Rev. 32, 883–909. doi: 10.1016/j.neubiorev.2008.01.004
Fine, S. M., Angel, R. A., Perry, S. W., Epstein, L. G., Rothstein, J. D., Dewhurst, S., et al. (1996). Tumor necrosis factor alpha inhibits glutamate uptake by primary human astrocytes. Implications for pathogenesis of HIV-1 dementia. J. Biol. Chem. 271, 15303–15306.
Fisher, D., Gamieldien, K., and Mafunda, P. S. (2015). Methamphetamine is not toxic but disrupts the cell cycle of blood-brain barrier endothelial cells. Neurotox. Res. 28, 8–17. doi: 10.1007/s12640-015-9520-5
Flora, G., Lee, Y. W., Nath, A., Hennig, B., Maragos, W., and Toborek, M. (2003). Methamphetamine potentiates HIV-1 Tat protein-mediated activation of redox-sensitive pathways in discrete regions of the brain. Exp. Neurol. 179, 60–70. doi: 10.1006/exnr.2002.8048
Flores, S. C., Marecki, J. C., Harper, K. P., Bose, S. K., Nelson, S. K., and Mccord, J. M. (1993). Tat protein of human immunodeficiency virus type 1 represses expression of manganese superoxide dismutase in HeLa cells. Proc. Natl. Acad. Sci. U.S.A. 90, 7632–7636.
Fornai, F., Longone, P., Ferrucci, M., Lenzi, P., Isidoro, C., Ruggieri, S., et al. (2008). Autophagy and amyotrophic lateral sclerosis: the multiple roles of lithium. Autophagy 4, 527–530. doi: 10.4161/auto.5923
Friend, D. M., and Keefe, K. A. (2013). Glial reactivity in resistance to methamphetamine-induced neurotoxicity. J. Neurochem. 125, 566–574. doi: 10.1111/jnc.12201
Fuentes, M. E., Durham, S. K., Swerdel, M. R., Lewin, A. C., Barton, D. S., Megill, J. R., et al. (1995). Controlled recruitment of monocytes and macrophages to specific organs through transgenic expression of monocyte chemoattractant protein-1. J. Immunol. 155, 5769–5776.
Fujita, Y., Ishima, T., Kunitachi, S., Hagiwara, H., Zhang, L., Iyo, M., et al. (2008). Phencyclidine-induced cognitive deficits in mice are improved by subsequent subchronic administration of the antibiotic drug minocycline. Prog. Neuropsychopharmacol. Biol. Psychiatry 32, 336–339. doi: 10.1016/j.pnpbp.2007.08.031
Fujita, Y., Kunitachi, S., Iyo, M., and Hashimoto, K. (2012). The antibiotic minocycline prevents methamphetamine-induced rewarding effects in mice. Pharmacol. Biochem. Behav. 101, 303–306. doi: 10.1016/j.pbb.2012.01.005
Gallo, P., Frei, K., Rordorf, C., Lazdins, J., Tavolato, B., and Fontana, A. (1989). Human immunodeficiency virus type 1 (HIV-1) infection of the central nervous system: an evaluation of cytokines in cerebrospinal fluid. J. Neuroimmunol. 23, 109–116.
Gaskill, P. J., Calderon, T. M., Coley, J. S., and Berman, J. W. (2013). Drug induced increases in CNS dopamine alter monocyte, macrophage and T cell functions: implications for HAND. J. Neuroimmune Pharmacol. 8, 621–642. doi: 10.1007/s11481-013-9443-y
Genc, K., Genc, S., Kizildag, S., Sonmez, U., Yilmaz, O., Tugyan, K., et al. (2003). Methamphetamine induces oligodendroglial cell death in vitro. Brain Res. 982, 125–130. doi: 10.1016/S0006-8993(03)02890-7
Gendelman, H. E., and Gelbard, H. A. (2014). Adjunctive and long-acting nanoformulated antiretroviral therapies for HIV-associated neurocognitive disorders. Curr. Opin. HIV AIDS 9, 585–590. doi: 10.1097/coh.0000000000000111
Gibb, J. W., and Kogan, F. J. (1979). Influence of dopamine synthesis on methamphetamine-induced changes in striatal and adrenal tyrosine hydroxylase activity. Naunyn Schmiedebergs Arch. Pharmacol. 310, 185–187.
Gibson, L. C., Hastings, S. F., Mcphee, I., Clayton, R. A., Darroch, C. E., Mackenzie, A., et al. (2006). The inhibitory profile of Ibudilast against the human phosphodiesterase enzyme family. Eur. J. Pharmacol. 538, 39–42. doi: 10.1016/j.ejphar.2006.02.053
Gimenez, F., Fernandez, C., and Mabondzo, A. (2004). Transport of HIV protease inhibitors through the blood-brain barrier and interactions with the efflux proteins, P-glycoprotein and multidrug resistance proteins. J. Acquir. Immune Defic. Syndr. 36, 649–658. doi: 10.1097/00126334-200406010-00001
Giros, B., Jaber, M., Jones, S. R., Wightman, R. M., and Caron, M. G. (1996). Hyperlocomotion and indifference to cocaine and amphetamine in mice lacking the dopamine transporter. Nature 379, 606–612. doi: 10.1038/379606a0
Glass, J. D., Wesselingh, S. L., Selnes, O. A., and Mcarthur, J. C. (1993). Clinical-neuropathologic correlation in HIV-associated dementia. Neurology 43, 2230–2237.
Golembiowska, K., Konieczny, J., Wolfarth, S., and Ossowska, K. (2003). Neuroprotective action of MPEP, a selective mGluR5 antagonist, in methamphetamine-induced dopaminergic neurotoxicity is associated with a decrease in dopamine outflow and inhibition of hyperthermia in rats. Neuropharmacology 45, 484–492. doi: 10.1016/S0028-3908(03)00209-0
Granado, N., Ares-Santos, S., Oliva, I., O’Shea, E., Martin, E. D., Colado, M. I., et al. (2011a). Dopamine D2-receptor knockout mice are protected against dopaminergic neurotoxicity induced by methamphetamine or MDMA. Neurobiol. Dis. 42, 391–403. doi: 10.1016/j.nbd.2011.01.033
Granado, N., Lastres-Becker, I., Ares-Santos, S., Oliva, I., Martin, E., Cuadrado, A., et al. (2011b). Nrf2 deficiency potentiates methamphetamine-induced dopaminergic axonal damage and gliosis in the striatum. Glia 59, 1850–1863. doi: 10.1002/glia.21229
Gray, F., Hurtrel, M., and Hurtrel, B. (1993). Early central nervous system changes in human immunodeficiency virus (HIV)-infection. Neuropathol. Appl. Neurobiol. 19, 3–9.
Greenwood, S. M., Mizielinska, S. M., Frenguelli, B. G., Harvey, J., and Connolly, C. N. (2007). Mitochondrial dysfunction and dendritic beading during neuronal toxicity. J. Biol. Chem. 282, 26235–26244. doi: 10.1074/jbc.M704488200
Gupta, S., Knight, A. G., Gupta, S., Knapp, P. E., Hauser, K. F., Keller, J. N., et al. (2010). HIV-Tat elicits microglial glutamate release: role of NAPDH oxidase and the cystine-glutamate antiporter. Neurosci. Lett. 485, 233–236. doi: 10.1016/j.neulet.2010.09.019
Halpin, L. E., Collins, S. A., and Yamamoto, B. K. (2014). Neurotoxicity of methamphetamine and 3,4-methylenedioxymethamphetamine. Life Sci. 97, 37–44. doi: 10.1016/j.lfs.2013.07.014
Hambuchen, M. D., Carroll, F. I., Ruedi-Bettschen, D., Hendrickson, H., Hennings, L., Blough, B. E., et al. (2015). Combining active immunization with monoclonal antibody therapy to facilitate early initiation of a long-acting anti-methamphetamine antibody response. J. Med. Chem. 58, 4665–4677. doi: 10.1021/acs.jmedchem.5b00220
Hambuchen, M. D., Ruedi-Bettschen, D., Williams, D. K., Hendrickson, H., and Owens, S. M. (2014). Treatment of rats with an anti-(+)-methamphetamine monoclonal antibody shortens the duration of action of repeated (+)-methamphetamine challenges over a one month period. Vaccine 32, 6213–6219. doi: 10.1016/j.vaccine.2014.09.025
Hanson, L. R., and Frey, W. H. II. (2007). Strategies for intranasal delivery of therapeutics for the prevention and treatment of neuroAIDS. J. Neuroimmune Pharmacol. 2, 81–86. doi: 10.1007/s11481-006-9039-x
Harris, A. C., Lesage, M. G., Shelley, D., Perry, J. L., Pentel, P. R., and Owens, S. M. (2015). The anti-(+)-methamphetamine monoclonal antibody mAb7F9 attenuates acute (+)-methamphetamine effects on intracranial self-stimulation in rats. PLoS ONE 10:e0118787. doi: 10.1371/journal.pone.0118787
Hauber, J., Malim, M. H., and Cullen, B. R. (1989). Mutational analysis of the conserved basic domain of human immunodeficiency virus tat protein. J. Virol. 63, 1181–1187.
Haughey, N. J., Cutler, R. G., Tamara, A., Mcarthur, J. C., Vargas, D. L., Pardo, C. A., et al. (2004). Perturbation of sphingolipid metabolism and ceramide production in HIV-dementia. Ann. Neurol. 55, 257–267. doi: 10.1002/ana.10828
Haughey, N. J., Holden, C. P., Nath, A., and Geiger, J. D. (1999). Involvement of inositol 1,4,5-trisphosphate-regulated stores of intracellular calcium in calcium dysregulation and neuron cell death caused by HIV-1 protein tat. J. Neurochem. 73, 1363–1374.
Haughey, N. J., Nath, A., Mattson, M. P., Slevin, J. T., and Geiger, J. D. (2001). HIV-1 Tat through phosphorylation of NMDA receptors potentiates glutamate excitotoxicity. J. Neurochem. 78, 457–467. doi: 10.1046/j.1471-4159.2001.00396.x
Hauser, K. F., and Knapp, P. E. (2014). Interactions of HIV and drugs of abuse: the importance of glia, neural progenitors, and host genetic factors. Int. Rev. Neurobiol. 118, 231–313. doi: 10.1016/b978-0-12-801284-0.00009-9
Hebert, M. A., and O’Callaghan, J. P. (2000). Protein phosphorylation cascades associated with methamphetamine-induced glial activation. Ann. N. Y. Acad. Sci. 914, 238–262. doi: 10.1111/j.1749-6632.2000.tb05200.x
Hemelaar, J., Gouws, E., Ghys, P. D., and Osmanov, S. (2011). Global trends in molecular epidemiology of HIV-1 during 2000–2007. AIDS 25, 679–689. doi: 10.1097/QAD.0b013e328342ff93
Herrold, A. A., Voigt, R. M., and Napier, T. C. (2013). mGluR5 is necessary for maintenance of methamphetamine-induced associative learning. Eur. Neuropsychopharmacol. 23, 691–696. doi: 10.1016/j.euroneuro.2012.05.014
Heyes, M. P., Achim, C. L., Wiley, C. A., Major, E. O., Saito, K., and Markey, S. P. (1996). Human microglia convert l-tryptophan into the neurotoxin quinolinic acid. Biochem. J. 320, 595–597.
He, Z., Chen, Y., Dong, H., Su, R., Gong, Z., and Yan, L. (2014). Inhibition of vesicular glutamate transporters contributes to attenuate methamphetamine-induced conditioned place preference in rats. Behav. Brain Res. 267, 1–5. doi: 10.1016/j.bbr.2014.02.047
Hirata, H., Asanuma, M., and Cadet, J. L. (1998). Superoxide radicals are mediators of the effects of methamphetamine on Zif268 (Egr-1, NGFI-A) in the brain: evidence from using CuZn superoxide dismutase transgenic mice. Brain Res. Mol. Brain Res. 58, 209–216.
Hirata, H., Ladenheim, B., Carlson, E., Epstein, C., and Cadet, J. L. (1996). Autoradiographic evidence for methamphetamine-induced striatal dopaminergic loss in mouse brain: attenuation in CuZn-superoxide dismutase transgenic mice. Brain Res. 714, 95–103.
Hirata, H., Ladenheim, B., Rothman, R. B., Epstein, C., and Cadet, J. L. (1995). Methamphetamine-induced serotonin neurotoxicity is mediated by superoxide radicals. Brain Res. 677, 345–347.
Hiyoshi, T., Marumo, T., Hikichi, H., Tomishima, Y., Urabe, H., Tamita, T., et al. (2014). Neurophysiologic and antipsychotic profiles of TASP0433864, a novel positive allosteric modulator of metabotropic glutamate 2 receptor. J. Pharmacol. Exp. Ther. 351, 642–653. doi: 10.1124/jpet.114.218651
Huang, W., Chen, L., Zhang, B., Park, M., and Toborek, M. (2014). PPAR agonist-mediated protection against HIV Tat-induced cerebrovascular toxicity is enhanced in MMP-9-deficient mice. J. Cereb. Blood Flow Metab. 34, 646–653. doi: 10.1038/jcbfm.2013.240
Hudson, L., Liu, J., Nath, A., Jones, M., Raghavan, R., Narayan, O., et al. (2000). Detection of the human immunodeficiency virus regulatory protein tat in CNS tissues. J. Neurovirol. 6, 145–155. doi: 10.3109/13550280009013158
Hui, L., Chen, X., Haughey, N. J., and Geiger, J. D. (2012). Role of endolysosomes in HIV-1 Tat-induced neurotoxicity. ASN Neuro 4, 243–252. doi: 10.1042/an20120017
Huigen, M. C., Kamp, W., and Nottet, H. S. (2004). Multiple effects of HIV-1 trans-activator protein on the pathogenesis of HIV-1 infection. Eur. J. Clin. Invest. 34, 57–66. doi: 10.1111/j.1365-2362.2004.01282.x
Jayanthi, S., Deng, X., Noailles, P. A., Ladenheim, B., and Cadet, J. L. (2004). Methamphetamine induces neuronal apoptosis via cross-talks between endoplasmic reticulum and mitochondria-dependent death cascades. FASEB J. 18, 238–251. doi: 10.1096/fj.03-0295com
Jayaram-Lindstrom, N., Hammarberg, A., Beck, O., and Franck, J. (2008a). Naltrexone for the treatment of amphetamine dependence: a randomized, placebo-controlled trial. Am. J. Psychiatry 165, 1442–1448. doi: 10.1176/appi.ajp.2008.08020304
Jayaram-Lindstrom, N., Konstenius, M., Eksborg, S., Beck, O., Hammarberg, A., and Franck, J. (2008b). Naltrexone attenuates the subjective effects of amphetamine in patients with amphetamine dependence. Neuropsychopharmacology 33, 1856–1863. doi: 10.1038/sj.npp.1301572
Jayaram-Lindstrom, N., Wennberg, P., Hurd, Y. L., and Franck, J. (2004). Effects of naltrexone on the subjective response to amphetamine in healthy volunteers. J. Clin. Psychopharmacol. 24, 665–669. doi: 10.1097/01.jcp.0000144893.29987.e5
Jeang, K. T., Xiao, H., and Rich, E. A. (1999). Multifaceted activities of the HIV-1 transactivator of transcription, Tat. J. Biol. Chem. 274, 28837–28840.
Jenuwein, M., Scheller, C., Neuen-Jacob, E., Sopper, S., Tatschner, T., Ter Meulen, V., et al. (2004). Dopamine deficits and regulation of the cAMP second messenger system in brains of simian immunodeficiency virus-infected rhesus monkeys. J. Neurovirol. 10, 163–170. doi: 10.1080/13550280490448016
Jia, H., Lohr, M., Jezequel, S., Davis, D., Shaikh, S., Selwood, D., et al. (2001). Cysteine-rich and basic domain HIV-1 Tat peptides inhibit angiogenesis and induce endothelial cell apoptosis. Biochem. Biophys. Res. Commun. 283, 469–479. doi: 10.1006/bbrc.2001.4790
Jin, C., Wu, L., Li, J., Fang, M., Cheng, L., and Wu, N. (2012). Multiple signaling pathways are involved in the interleukine-4 regulated expression of DC-SIGN in THP-1 cell line. J. Biomed. Biotechnol. 2012, 357060. doi: 10.1155/2012/357060
Jones, M., Olafson, K., Del Bigio, M. R., Peeling, J., and Nath, A. (1998). Intraventricular injection of human immunodeficiency virus type 1 (HIV-1) tat protein causes inflammation, gliosis, apoptosis, and ventricular enlargement. J. Neuropathol. Exp. Neurol. 57, 563–570.
Ju, S. M., Song, H. Y., Lee, J. A., Lee, S. J., Choi, S. Y., and Park, J. (2009). Extracellular HIV-1 Tat up-regulates expression of matrix metalloproteinase-9 via a MAPK-NF-kappaB dependent pathway in human astrocytes. Exp. Mol. Med. 41, 86–93. doi: 10.3858/emm.2009.41.2.011
Jumnongprakhon, P., Govitrapong, P., Tocharus, C., Tungkum, W., and Tocharus, J. (2014). Protective effect of melatonin on methamphetamine-induced apoptosis in glioma cell line. Neurotox. Res. 25, 286–294. doi: 10.1007/s12640-013-9419-y
Kass, M. D., Liu, X., Vigorito, M., Chang, L., and Chang, S. L. (2010). Methamphetamine-induced behavioral and physiological effects in adolescent and adult HIV-1 transgenic rats. J. Neuroimmune Pharmacol. 5, 566–573. doi: 10.1007/s11481-010-9221-z
Kaushal, N., and Matsumoto, R. R. (2011). Role of sigma receptors in methamphetamine-induced neurotoxicity. Curr. Neuropharmacol. 9, 54–57. doi: 10.2174/157015911795016930
Kaushal, N., Robson, M. J., Rosen, A., Mccurdy, C. R., and Matsumoto, R. R. (2014). Neuroprotective targets through which 6-acetyl-3-(4-(4-(4-fluorophenyl)piperazin-1-yl)butyl)benzo[d]oxazol-2(3H)-one (SN79), a sigma receptor ligand, mitigates the effects of methamphetamine in vitro. Eur. J. Pharmacol. 724, 193–203. doi: 10.1016/j.ejphar.2013.12.039
Kaushal, N., Robson, M. J., Vinnakota, H., Narayanan, S., Avery, B. A., Mccurdy, C. R., et al. (2011a). Synthesis and pharmacological evaluation of 6-acetyl-3-(4-(4-(4-fluorophenyl)piperazin-1-yl)butyl)benzo[d]oxazol-2(3H)-one (SN79), a cocaine antagonist, in rodents. AAPS J. 13, 336–346. doi: 10.1208/s12248-011-9274-9
Kaushal, N., Seminerio, M. J., Shaikh, J., Medina, M. A., Mesangeau, C., Wilson, L. L., et al. (2011b). CM156, a high affinity sigma ligand, attenuates the stimulant and neurotoxic effects of methamphetamine in mice. Neuropharmacology 61, 992–1000. doi: 10.1016/j.neuropharm.2011.06.028
Kaushal, N., Seminerio, M. J., Robson, M. J., Mccurdy, C. R., and Matsumoto, R. R. (2013). Pharmacological evaluation of SN79, a sigma (sigma) receptor ligand, against methamphetamine-induced neurotoxicity in vivo. Eur. Neuropsychopharmacol. 23, 960–971. doi: 10.1016/j.euroneuro.2012.08.005
Kesby, J. P., Heaton, R. K., Young, J. W., Umlauf, A., Woods, S. P., Letendre, S. L., et al. (2015). Methamphetamine exposure combined with HIV-1 disease or gp120 expression: comparison of learning and executive functions in humans and mice. Neuropsychopharmacology 40, 1899–909. doi: 10.1038/npp.2015.39
Kiebala, M., and Maggirwar, S. B. (2011). Ibudilast, a pharmacologic phosphodiesterase inhibitor, prevents human immunodeficiency virus-1 Tat-mediated activation of microglial cells. PLoS ONE 6:e18633. doi: 10.1371/journal.pone.0018633
Kiebala, M., Polesskaya, O., Yao, Z., Perry, S. W., and Maggirwar, S. B. (2010). Nuclear factor-kappa B family member RelB inhibits human immunodeficiency virus-1 Tat-induced tumor necrosis factor-alpha production. PLoS ONE 5:e11875. doi: 10.1371/journal.pone.0011875
Kim, B. O., Liu, Y., Ruan, Y., Xu, Z. C., Schantz, L., and He, J. J. (2003). Neuropathologies in transgenic mice expressing human immunodeficiency virus type 1 Tat protein under the regulation of the astrocyte-specific glial fibrillary acidic protein promoter and doxycycline. Am. J. Pathol. 162, 1693–1707. doi: 10.1016/s0002-9440(10)64304-0
Kim, S. H., Smith, A. J., Tan, J., Shytle, R. D., and Giunta, B. (2015). MSM ameliorates HIV-1 Tat induced neuronal oxidative stress via rebalance of the glutathione cycle. Am. J. Transl. Res. 7, 328–338.
King, J. E., Eugenin, E. A., Hazleton, J. E., Morgello, S., and Berman, J. W. (2010). Mechanisms of HIV-tat-induced phosphorylation of N-methyl-D-aspartate receptor subunit 2A in human primary neurons: implications for neuroAIDS pathogenesis. Am. J. Pathol. 176, 2819–2830. doi: 10.2353/ajpath.2010.090642
Koller, H., Schaal, H., Freund, M., Garrido, S. R., Von Giesen, H. J., Ott, M., et al. (2001). HIV-1 protein Tat reduces the glutamate-induced intracellular Ca2+ increase in cultured cortical astrocytes. Eur. J. Neurosci. 14, 1793–1799. doi: 10.1046/j.0953-816x.2001.01808.x
Koriem, K. M., Abdelhamid, A. Z., and Younes, H. F. (2013). Caffeic acid protects tissue antioxidants and DNA content in methamphetamine induced tissue toxicity in Sprague Dawley rats. Toxicol. Mech. Methods 23, 134–143. doi: 10.3109/15376516.2012.730561
Kousik, S. M., Napier, T. C., and Carvey, P. M. (2012). The effects of psychostimulant drugs on blood brain barrier function and neuroinflammation. Front. Pharmacol. 3:121. doi: 10.3389/fphar.2012.00121
Koutsilieri, E., Sopper, S., Scheller, C., Ter Meulen, V., and Riederer, P. (2002a). Involvement of dopamine in the progression of AIDS Dementia Complex. J. Neural. Transm. 109, 399–410. doi: 10.1007/s007020200032
Koutsilieri, E., Sopper, S., Scheller, C., Ter Meulen, V., and Riederer, P. (2002b). Parkinsonism in HIV dementia. J. Neural. Transm. 109, 767–775. doi: 10.1007/s007020200063
Kovalevich, J., and Langford, D. (2012). Neuronal toxicity in HIV CNS disease. Future Virol. 7, 687–698. doi: 10.2217/fvl.12.57
Krogh, K. A., Lyddon, E., and Thayer, S. A. (2015). HIV-1 Tat activates a RhoA signaling pathway to reduce NMDA-evoked calcium responses in hippocampal neurons via an actin-dependent mechanism. J. Neurochem. 132, 354–366. doi: 10.1111/jnc.12936
Krogh, K. A., Wydeven, N., Wickman, K., and Thayer, S. A. (2014). HIV-1 protein Tat produces biphasic changes in NMDA-evoked increases in intracellular Ca2+ concentration via activation of Src kinase and nitric oxide signaling pathways. J. Neurochem. 130, 642–656. doi: 10.1111/jnc.12724
Kuhn, D. M., Francescutti-Verbeem, D. M., and Thomas, D. M. (2006). Dopamine quinones activate microglia and induce a neurotoxic gene expression profile: relationship to methamphetamine-induced nerve ending damage. Ann. N. Y. Acad. Sci. 1074, 31–41. doi: 10.1196/annals.1369.003
Kuppuswamy, M., Subramanian, T., Srinivasan, A., and Chinnadurai, G. (1989). Multiple functional domains of Tat, the trans-activator of HIV-1, defined by mutational analysis. Nucleic Acids Res. 17, 3551–3561.
Langford, D., Grigorian, A., Hurford, R., Adame, A., Crews, L., and Masliah, E. (2004). The role of mitochondrial alterations in the combined toxic effects of human immunodeficiency virus Tat protein and methamphetamine on calbindin positive-neurons. J. Neurovirol. 10, 327–337. doi: 10.1080/13550280490520961
Lasso, M., and Ceron, I. (2012). Mirtazapine and antiretroviral therapy in the treatment of progressive multifocal leukoencephalopathy associated with HIV-1 infection: report of a case and review of literature. Rev. Chilena Infectol. 29, 217–220. doi: 10.4067/s0716-10182012000200016
Laurenzana, E. M., Stevens, M. W., Frank, J. C., Hambuchen, M. D., Hendrickson, H. P., White, S. J., et al. (2014). Pharmacological effects of two anti-methamphetamine monoclonal antibodies. Supporting data for lead candidate selection for clinical development. Hum. Vaccin. Immunother. 10, 2638–2647. doi: 10.4161/hv.29707
Lecoeur, H., Borgne-Sanchez, A., Chaloin, O., El-Khoury, R., Brabant, M., Langonne, A., et al. (2012). HIV-1 Tat protein directly induces mitochondrial membrane permeabilization and inactivates cytochrome c oxidase. Cell Death Dis. 3, e282. doi: 10.1038/cddis.2012.21
Li, J. C., Yim, H. C., and Lau, A. S. (2010). Role of HIV-1 Tat in AIDS pathogenesis: its effects on cytokine dysregulation and contributions to the pathogenesis of opportunistic infection. AIDS 24, 1609–1623. doi: 10.1097/QAD.0b013e32833ac6a0
Li, W., Huang, Y., Reid, R., Steiner, J., Malpica-Llanos, T., Darden, T. A., et al. (2008). NMDA receptor activation by HIV-Tat protein is clade dependent. J. Neurosci. 28, 12190–12198. doi: 10.1523/jneurosci.3019-08.2008
Li, W., Li, G., Steiner, J., and Nath, A. (2009). Role of Tat protein in HIV neuropathogenesis. Neurotox. Res. 16, 205–220. doi: 10.1007/s12640-009-9047-8
Liang, H., Wang, X., Chen, H., Song, L., Ye, L., Wang, S. H., et al. (2008). Methamphetamine enhances HIV infection of macrophages. Am. J. Pathol. 172, 1617–1624. doi: 10.2353/ajpath.2008.070971
Liddie, S., Balda, M. A., and Itzhak, Y. (2013). Nitric oxide (NO) signaling as a potential therapeutic modality against psychostimulants. Curr. Pharm. Des. 19, 7092–7102. doi: 10.2174/138161281940131209144527
Lin, M., Chandramani-Shivalingappa, P., Jin, H., Ghosh, A., Anantharam, V., Ali, S., et al. (2012). Methamphetamine-induced neurotoxicity linked to ubiquitin-proteasome system dysfunction and autophagy-related changes that can be modulated by protein kinase C delta in dopaminergic neuronal cells. Neuroscience 210, 308–332. doi: 10.1016/j.neuroscience.2012.03.004
Lindl, K. A., Marks, D. R., Kolson, D. L., and Jordan-Sciutto, K. L. (2010). HIV-associated neurocognitive disorder: pathogenesis and therapeutic opportunities. J. Neuroimmune Pharmacol. 5, 294–309. doi: 10.1007/s11481-010-9205-z
Liu, M., Li, D., Sun, L., Chen, J., Sun, X., Zhang, L., et al. (2014). Modulation of Eg5 activity contributes to mitotic spindle checkpoint activation and Tat-mediated apoptosis in CD4-positive T-lymphocytes. J. Pathol. 233, 138–147. doi: 10.1002/path.4333
Liu, X., Chang, L., Vigorito, M., Kass, M., Li, H., and Chang, S. L. (2009). Methamphetamine-induced behavioral sensitization is enhanced in the HIV-1 transgenic rat. J. Neuroimmune Pharmacol. 4, 309–316. doi: 10.1007/s11481-009-9160-8
Liu, X., Jana, M., Dasgupta, S., Koka, S., He, J., Wood, C., et al. (2002). Human immunodeficiency virus type 1 (HIV-1) tat induces nitric-oxide synthase in human astroglia. J. Biol. Chem. 277, 39312–39319. doi: 10.1074/jbc.M205107200
Liu, Y., Jones, M., Hingtgen, C. M., Bu, G., Laribee, N., Tanzi, R. E., et al. (2000). Uptake of HIV-1 tat protein mediated by low-density lipoprotein receptor-related protein disrupts the neuronal metabolic balance of the receptor ligands. Nat. Med. 6, 1380–1387. doi: 10.1038/82199
Loftis, J. M., and Janowsky, A. (2014). Neuroimmune basis of methamphetamine toxicity. Int. Rev. Neurobiol. 118, 165–197. doi: 10.1016/b978-0-12-801284-0.00007-5
Lv, Z., Chu, Y., and Wang, Y. (2015). HIV protease inhibitors: a review of molecular selectivity and toxicity. HIV AIDS (Auckl) 7, 95–104. doi: 10.2147/hiv.s79956
Ma, J., Wan, J., Meng, J., Banerjee, S., Ramakrishnan, S., and Roy, S. (2014a). Methamphetamine induces autophagy as a pro-survival response against apoptotic endothelial cell death through the Kappa opioid receptor. Cell Death Dis. 5, e1099. doi: 10.1038/cddis.2014.64
Ma, R., Yang, L., Niu, F., and Buch, S. (2014b). HIV Tat-mediated induction of human brain microvascular endothelial cell apoptosis involves endoplasmic reticulum stress and mitochondrial dysfunction. Mol. Neurobiol. doi: 10.1007/s12035-014-8991-3 [Epub ahead of print].
Mabrouk, K., Van Rietschoten, J., Vives, E., Darbon, H., Rochat, H., and Sabatier, J. M. (1991). Lethal neurotoxicity in mice of the basic domains of HIV and SIV Rev proteins. Study of these regions by circular dichroism. FEBS Lett. 289, 13–17.
Maldonado, M. D., Reiter, R. J., and Perez-San-Gregorio, M. A. (2009). Melatonin as a potential therapeutic agent in psychiatric illness. Hum. Psychopharmacol. 24, 391–400. doi: 10.1002/hup.1032
Maragos, W. F., Young, K. L., Turchan, J. T., Guseva, M., Pauly, J. R., Nath, A., et al. (2002). Human immunodeficiency virus-1 Tat protein and methamphetamine interact synergistically to impair striatal dopaminergic function. J. Neurochem. 83, 955–963. doi: 10.1046/j.1471-4159.2002.01212.x
Marcondes, M. C., Flynn, C., Watry, D. D., Zandonatti, M., and Fox, H. S. (2010). Methamphetamine increases brain viral load and activates natural killer cells in simian immunodeficiency virus-infected monkeys. Am. J. Pathol. 177, 355–361. doi: 10.2353/ajpath.2010.090953
Mark, K. A., Soghomonian, J. J., and Yamamoto, B. K. (2004). High-dose methamphetamine acutely activates the striatonigral pathway to increase striatal glutamate and mediate long-term dopamine toxicity. J. Neurosci. 24, 11449–11456. doi: 10.1523/jneurosci.3597-04.2004
Marker, D. F., Puccini, J. M., Mockus, T. E., Barbieri, J., Lu, S. M., and Gelbard, H. A. (2012). LRRK2 kinase inhibition prevents pathological microglial phagocytosis in response to HIV-1 Tat protein. J. Neuroinflamm. 9, 261. doi: 10.1186/1742-2094-9-261
Martins, T., Burgoyne, T., Kenny, B. A., Hudson, N., Futter, C. E., Ambrosio, A. F., et al. (2013). Methamphetamine-induced nitric oxide promotes vesicular transport in blood-brain barrier endothelial cells. Neuropharmacology 65, 74–82. doi: 10.1016/j.neuropharm.2012.08.021
Matsumoto, R. R. (2009). Targeting sigma receptors: novel medication development for drug abuse and addiction. Expert. Rev. Clin. Pharmacol. 2, 351–358. doi: 10.1586/ecp.09.18
Matsumoto, R. R., Nguyen, L., Kaushal, N., and Robson, M. J. (2014). Sigma (sigma) receptors as potential therapeutic targets to mitigate psychostimulant effects. Adv. Pharmacol. 69, 323–386. doi: 10.1016/b978-0-12-420118-7.00009-3
Mediouni, S., Darque, A., Baillat, G., Ravaux, I., Dhiver, C., Tissot-Dupont, H., et al. (2012). Antiretroviral therapy does not block the secretion of the human immunodeficiency virus tat protein. Infect. Disord. Drug. Targets 12, 81–86. doi: 10.2174/187152612798994939
Mediouni, S., Jablonski, J., Paris, J. J., Clementz, M. A., Thenin-Houssier, S., Mclaughlin, J. P., et al. (2015). Didehydro-cortistatin a inhibits HIV-1 Tat mediated neuroinflammation and prevents potentiation of cocaine reward in tat transgenic mice. Curr. HIV Res. 13, 64–79. doi: 10.2174/1570162X13666150121111548
Merrill, J. E., Koyanagi, Y., and Chen, I. S. (1989). Interleukin-1 and tumor necrosis factor alpha can be induced from mononuclear phagocytes by human immunodeficiency virus type 1 binding to the CD4 receptor. J. Virol. 63, 4404–4408.
Metz, L. M., Zhang, Y., Yeung, M., Patry, D. G., Bell, R. B., Stoian, C. A., et al. (2004). Minocycline reduces gadolinium-enhancing magnetic resonance imaging lesions in multiple sclerosis. Ann. Neurol. 55, 756. doi: 10.1002/ana.20111
Midde, N. M., Gomez, A. M., and Zhu, J. (2012). HIV-1 Tat protein decreases dopamine transporter cell surface expression and vesicular monoamine transporter-2 function in rat striatal synaptosomes. J. Neuroimmune Pharmacol. 7, 629–639. doi: 10.1007/s11481-012-9369-9
Midde, N. M., Huang, X., Gomez, A. M., Booze, R. M., Zhan, C. G., and Zhu, J. (2013). Mutation of tyrosine 470 of human dopamine transporter is critical for HIV-1 Tat-induced inhibition of dopamine transport and transporter conformational transitions. J. Neuroimmune Pharmacol. 8, 975–987. doi: 10.1007/s11481-013-9464-6
Milani, D., Mazzoni, M., Borgatti, P., Zauli, G., Cantley, L., and Capitani, S. (1996). Extracellular human immunodeficiency virus type-1 Tat protein activates phosphatidylinositol 3-kinase in PC12 neuronal cells. J. Biol. Chem. 271, 22961–22964.
Miller, D. S., Bauer, B., and Hartz, A. M. (2008). Modulation of P-glycoprotein at the blood-brain barrier: opportunities to improve central nervous system pharmacotherapy. Pharmacol. Rev. 60, 196–209. doi: 10.1124/pr.107.07109
Mishra, M., Vetrivel, S., Siddappa, N. B., Ranga, U., and Seth, P. (2008). Clade-specific differences in neurotoxicity of human immunodeficiency virus-1 B and C Tat of human neurons: significance of dicysteine C30C31 motif. Ann. Neurol. 63, 366–376. doi: 10.1002/ana.21292
Miyazaki, I., Asanuma, M., Kikkawa, Y., Takeshima, M., Murakami, S., Miyoshi, K., et al. (2011). Astrocyte-derived metallothionein protects dopaminergic neurons from dopamine quinone toxicity. Glia 59, 435–451. doi: 10.1002/glia.21112
Mizoguchi, H., Takuma, K., Fukakusa, A., Ito, Y., Nakatani, A., Ibi, D., et al. (2008). Improvement by minocycline of methamphetamine-induced impairment of recognition memory in mice. Psychopharmacology (Berl.) 196, 233–241. doi: 10.1007/s00213-007-0955-0
Mizuno, T., Kurotani, T., Komatsu, Y., Kawanokuchi, J., Kato, H., Mitsuma, N., et al. (2004). Neuroprotective role of phosphodiesterase inhibitor ibudilast on neuronal cell death induced by activated microglia. Neuropharmacology 46, 404–411. doi: 10.1016/j.neuropharm.2003.09.009
Moran, L. M., Aksenov, M. Y., Booze, R. M., Webb, K. M., and Mactutus, C. F. (2012). Adolescent HIV-1 transgenic rats: evidence for dopaminergic alterations in behavior and neurochemistry revealed by methamphetamine challenge. Curr. HIV Res. 10, 415–424. doi: 10.2174/157016212802138788
Mousseau, G., Clementz, M. A., Bakeman, W. N., Nagarsheth, N., Cameron, M., Shi, J., et al. (2012). An analog of the natural steroidal alkaloid cortistatin A potently suppresses Tat-dependent HIV transcription. Cell Host Microbe 12, 97–108. doi: 10.1016/j.chom.2012.05.016
Mousseau, G., Mediouni, S., and Valente, S. T. (2015). Targeting HIV transcription: the quest for a functional cure. Curr. Top. Microbiol. Immunol. 389, 121–145. doi: 10.1007/82_2015_435
Moynagh, P. N. (2005). The interleukin-1 signalling pathway in astrocytes: a key contributor to inflammation in the brain. J. Anat. 207, 265–269. doi: 10.1111/j.1469-7580.2005.00445.x
Nair, M. P., and Saiyed, Z. M. (2011). Effect of methamphetamine on expression of HIV coreceptors and CC-chemokines by dendritic cells. Life Sci. 88, 987–994. doi: 10.1016/j.lfs.2010.09.019
Nakagawa, S., Castro, V., and Toborek, M. (2012). Infection of human pericytes by HIV-1 disrupts the integrity of the blood-brain barrier. J. Cell Mol. Med. 16, 2950–2957. doi: 10.1111/j.1582-4934.2012.01622.x
Nam, Y., Shin, E. J., Shin, S. W., Lim, Y. K., Jung, J. H., Lee, J. H., et al. (2014). YY162 prevents ADHD-like behavioral side effects and cytotoxicity induced by Aroclor1254 via interactive signaling between antioxidant potential, BDNF/TrkB, DAT and NET. Food Chem. Toxicol. 65, 280–292. doi: 10.1016/j.fct.2013.12.046
Nash, J. F., and Yamamoto, B. K. (1992). Methamphetamine neurotoxicity and striatal glutamate release: comparison to 3,4-methylenedioxymethamphetamine. Brain Res. 581, 237–243.
Nath, A., Conant, K., Chen, P., Scott, C., and Major, E. O. (1999). Transient exposure to HIV-1 Tat protein results in cytokine production in macrophages and astrocytes. A hit and run phenomenon. J. Biol. Chem. 274, 17098–17102.
Nath, A., Maragos, W. F., Avison, M. J., Schmitt, F. A., and Berger, J. R. (2001). Acceleration of HIV dementia with methamphetamine and cocaine. J. Neurovirol. 7, 66–71. doi: 10.1080/135502801300069737
Nath, A., and Steiner, J. (2014). Synaptodendritic injury with HIV-Tat protein: what is the therapeutic target? Exp. Neurol. 251, 112–114. doi: 10.1016/j.expneurol.2013.11.004
Newton, T. F., Reid, M. S., De La Garza, R., Mahoney, J. J., Abad, A., Condos, R., et al. (2008). Evaluation of subjective effects of aripiprazole and methamphetamine in methamphetamine-dependent volunteers. Int. J. Neuropsychopharmacol. 11, 1037–1045. doi: 10.1017/s1461145708009097
Nguyen, E. C., Mccracken, K. A., Liu, Y., Pouw, B., and Matsumoto, R. R. (2005). Involvement of sigma (sigma) receptors in the acute actions of methamphetamine: receptor binding and behavioral studies. Neuropharmacology 49, 638–645. doi: 10.1016/j.neuropharm.2005.04.016
Norman, J. P., Perry, S. W., Kasischke, K. A., Volsky, D. J., and Gelbard, H. A. (2007). HIV-1 trans activator of transcription protein elicits mitochondrial hyperpolarization and respiratory deficit, with dysregulation of complex IV and nicotinamide adenine dinucleotide homeostasis in cortical neurons. J. Immunol. 178, 869–876. doi: 10.4049/jimmunol.178.2.869
Northrop, N. A., and Yamamoto, B. K. (2015). Methamphetamine effects on blood-brain barrier structure and function. Front. Neurosci 9:69. doi: 10.3389/fnins.2015.00069
Nuovo, G. J., and Alfieri, M. L. (1996). AIDS dementia is associated with massive, activated HIV-1 infection and concomitant expression of several cytokines. Mol. Med. 2, 358–366.
Nutt, D. J., Lingford-Hughes, A., Erritzoe, D., and Stokes, P. R. (2015). The dopamine theory of addiction: 40 years of highs and lows. Nat. Rev. Neurosci. 16, 305–312. doi: 10.1038/nrn3939
O’Shea, E., Urrutia, A., Green, A. R., and Colado, M. I. (2014). Current preclinical studies on neuroinflammation and changes in blood-brain barrier integrity by MDMA and methamphetamine. Neuropharmacology 87, 125–134. doi: 10.1016/j.neuropharm.2014.02.015
Owen, A., and Khoo, S. H. (2004). Intracellular pharmacokinetics of antiretroviral agents. J. HIV Ther. 9, 97–101.
Panee, J., Pang, X., Munsaka, S., Berry, M. J., and Chang, L. (2015). Independent and co-morbid HIV infection and Meth use disorders on oxidative stress markers in the cerebrospinal fluid and depressive symptoms. J. Neuroimmune Pharmacol. 10, 111–121. doi: 10.1007/s11481-014-9581-x
Pardo, C. A., Mcarthur, J. C., and Griffin, J. W. (2001). HIV neuropathy: insights in the pathology of HIV peripheral nerve disease. J. Peripher. Nerv. Syst. 6, 21–27. doi: 10.1046/j.1529-8027.2001.006001021.x
Peloponese, J. M. Jr., Gregoire, C., Opi, S., Esquieu, D., Sturgis, J., Lebrun, E., et al. (2000). 1H-13C nuclear magnetic resonance assignment and structural characterization of HIV-1 Tat protein. C. R. Acad. Sci. 323, 883–894. doi: 10.1016/S0764-4469(00)01228-2
Perry, S. W., Barbieri, J., Tong, N., Polesskaya, O., Pudasaini, S., Stout, A., et al. (2010). Human immunodeficiency virus-1 Tat activates calpain proteases via the ryanodine receptor to enhance surface dopamine transporter levels and increase transporter-specific uptake and Vmax. J. Neurosci. 30, 14153–14164. doi: 10.1523/jneurosci.1042-10.2010
Philippon, V., Vellutini, C., Gambarelli, D., Harkiss, G., Arbuthnott, G., Metzger, D., et al. (1994). The basic domain of the lentiviral Tat protein is responsible for damages in mouse brain: involvement of cytokines. Virology 205, 519–529. doi: 10.1006/viro.1994.1673
Pitaksalee, R., Sanvarinda, Y., Sinchai, T., Sanvarinda, P., Thampithak, A., Jantaratnotai, N., et al. (2015). Autophagy inhibition by caffeine increases toxicity of methamphetamine in SH-SY5Y neuroblastoma cell line. Neurotox. Res. 27, 421–429. doi: 10.1007/s12640-014-9513-9
Pocernich, C. B., Sultana, R., Hone, E., Turchan, J., Martins, R. N., Calabrese, V., et al. (2004). Effects of apolipoprotein E on the human immunodeficiency virus protein Tat in neuronal cultures and synaptosomes. J. Neurosci. Res. 77, 532–539. doi: 10.1002/jnr.20182
Pocernich, C. B., Sultana, R., Mohmmad-Abdul, H., Nath, A., and Butterfield, D. A. (2005). HIV-dementia, Tat-induced oxidative stress, and antioxidant therapeutic considerations. Brain Res. Brain Res. Rev. 50, 14–26. doi: 10.1016/j.brainresrev.2005.04.002
Potula, R., and Persidsky, Y. (2008). Adding fuel to the fire: methamphetamine enhances HIV infection. Am. J. Pathol. 172, 1467–1470. doi: 10.2353/ajpath.2008.080130
Pourroy, B., Carre, M., Honore, S., Bourgarel-Rey, V., Kruczynski, A., Briand, C., et al. (2004). Low concentrations of vinflunine induce apoptosis in human SK-N-SH neuroblastoma cells through a postmitotic G1 arrest and a mitochondrial pathway. Mol. Pharmacol. 66, 580–591.
Prendergast, M. A., Rogers, D. T., Mulholland, P. J., Littleton, J. M., Wilkins, L. H. Jr., Self, R. L., et al. (2002). Neurotoxic effects of the human immunodeficiency virus type-1 transcription factor Tat require function of a polyamine sensitive-site on the N-methyl-D-aspartate receptor. Brain Res. 954, 300–307. doi: 10.1016/S0006-8993(02)03360-7
Pu, H., Hayashi, K., Andras, I. E., Eum, S. Y., Hennig, B., and Toborek, M. (2007). Limited role of COX-2 in HIV Tat-induced alterations of tight junction protein expression and disruption of the blood-brain barrier. Brain Res. 1184, 333–344. doi: 10.1016/j.brainres.2007.09.063
Puccini, J. M., Marker, D. F., Fitzgerald, T., Barbieri, J., Kim, C. S., Miller-Rhodes, P., et al. (2015). Leucine-rich repeat kinase 2 modulates neuroinflammation and neurotoxicity in models of human immunodeficiency virus 1-associated neurocognitive disorders. J. Neurosci. 35, 5271–5283. doi: 10.1523/jneurosci.0650-14.2015
Purohit, V., Rapaka, R., Frankenheim, J., Avila, A., Sorensen, R., and Rutter, J. (2013). National Institute on Drug Abuse symposium report: drugs of abuse, dopamine, and HIV-associated neurocognitive disorders/HIV-associated dementia. J. Neurovirol. 19, 119–122. doi: 10.1007/s13365-013-0153-2
Purohit, V., Rapaka, R., and Shurtleff, D. (2011). Drugs of abuse, dopamine, and HIV-associated neurocognitive disorders/HIV-associated dementia. Mol. Neurobiol. 44, 102–110. doi: 10.1007/s12035-011-8195-z
Qi, L., Gang, L., Hang, K. W., Ling, C. H., Xiaofeng, Z., Zhen, L., et al. (2011). Programmed neuronal cell death induced by HIV-1 tat and methamphetamine. Microsc. Res. Tech. 74, 1139–1144. doi: 10.1002/jemt.21006
Rahn, K. A., Slusher, B. S., and Kaplin, A. I. (2012). Glutamate in CNS neurodegeneration and cognition and its regulation by GCPII inhibition. Curr. Med. Chem. 19, 1335–1345. doi: 10.2174/092986712799462649
Ramirez, S. H., Potula, R., Fan, S., Eidem, T., Papugani, A., Reichenbach, N., et al. (2009). Methamphetamine disrupts blood-brain barrier function by induction of oxidative stress in brain endothelial cells. J. Cereb. Blood Flow Metab. 29, 1933–1945. doi: 10.1038/jcbfm.2009.112
Rao, V. R., Sas, A. R., Eugenin, E. A., Siddappa, N. B., Bimonte-Nelson, H., Berman, J. W., et al. (2008). HIV-1 clade-specific differences in the induction of neuropathogenesis. J. Neurosci. 28, 10010–10016. doi: 10.1523/jneurosci.2955-08.2008
Rappaport, J., Joseph, J., Croul, S., Alexander, G., Del Valle, L., Amini, S., et al. (1999). Molecular pathway involved in HIV-1-induced CNS pathology: role of viral regulatory protein, Tat. J. Leukoc. Biol. 65, 458–465.
Rau, T. F., Kothiwal, A. S., Rova, A. R., Brooks, D. M., and Poulsen, D. J. (2012). Treatment with low-dose methamphetamine improves behavioral and cognitive function after severe traumatic brain injury. J. Trauma. Acute Care Surg 73, S165–S172. doi: 10.1097/TA.0b013e318260896a
Ray, L. A., Bujarski, S., Courtney, K. E., Moallem, N. R., Lunny, K., Roche, D., et al. (2015). The effects of naltrexone on subjective response to methamphetamine in a clinical sample: a double-blind, placebo-controlled laboratory study. Neuropsychopharmacology 40, 2347–2356. doi: 10.1038/npp.2015.83
Reynolds, J. L., Mahajan, S. D., Sykes, D. E., Schwartz, S. A., and Nair, M. P. (2007). Proteomic analyses of methamphetamine (METH)-induced differential protein expression by immature dendritic cells (IDC). Biochim. Biophys. Acta 1774, 433–442. doi: 10.1016/j.bbapap.2007.02.001
Ricci, A., and Amenta, F. (1994). Dopamine D5 receptors in human peripheral blood lymphocytes: a radioligand binding study. J. Neuroimmunol. 53, 1–7.
Ricci, A., Bronzetti, E., Felici, L., Greco, S., and Amenta, F. (1998). Labeling of dopamine D3 and D4 receptor subtypes in human peripheral blood lymphocytes with [3H]7-OH-DPAT: a combined radioligand binding assay and immunochemical study. J. Neuroimmunol. 92, 191–195.
Ricci, A., Bronzetti, E., Felici, L., Tayebati, S. K., and Amenta, F. (1997). Dopamine D4 receptor in human peripheral blood lymphocytes: a radioligand binding assay study. Neurosci. Lett. 229, 130–134.
Ricci, A., Bronzetti, E., Mignini, F., Tayebati, S. K., Zaccheo, D., and Amenta, F. (1999). Dopamine D1-like receptor subtypes in human peripheral blood lymphocytes. J. Neuroimmunol. 96, 234–240.
Richard, M. J., Guiraud, P., Didier, C., Seve, M., Flores, S. C., and Favier, A. (2001). Human immunodeficiency virus type 1 Tat protein impairs selenoglutathione peroxidase expression and activity by a mechanism independent of cellular selenium uptake: consequences on cellular resistance to UV-A radiation. Arch. Biochem. Biophys. 386, 213–220. doi: 10.1006/abbi.2000.2197
Riddle, E. L., Fleckenstein, A. E., and Hanson, G. R. (2006). Mechanisms of methamphetamine-induced dopaminergic neurotoxicity. AAPS J. 8, E413–E418. doi: 10.1007/BF02854914
Robson, M. J., Noorbakhsh, B., Seminerio, M. J., and Matsumoto, R. R. (2012). Sigma-1 receptors: potential targets for the treatment of substance abuse. Curr. Pharm. Des. 18, 902–919. doi: 10.2174/138161212799436601
Robson, M. J., Turner, R. C., Naser, Z. J., Mccurdy, C. R., Huber, J. D., and Matsumoto, R. R. (2013). SN79, a sigma receptor ligand, blocks methamphetamine-induced microglial activation and cytokine upregulation. Exp. Neurol. 247, 134–142. doi: 10.1016/j.expneurol.2013.04.009
Robson, M. J., Turner, R. C., Naser, Z. J., Mccurdy, C. R., O’callaghan, J. P., Huber, J. D., et al. (2014). SN79, a sigma receptor antagonist, attenuates methamphetamine-induced astrogliosis through a blockade of OSMR/gp130 signaling and STAT3 phosphorylation. Exp. Neurol. 254, 180–189. doi: 10.1016/j.expneurol.2014.01.020
Rohr, O., Sawaya, B. E., Lecestre, D., Aunis, D., and Schaeffer, E. (1999a). Dopamine stimulates expression of the human immunodeficiency virus type 1 via NF-kappaB in cells of the immune system. Nucleic Acids Res. 27, 3291–3299.
Rohr, O., Schwartz, C., Aunis, D., and Schaeffer, E. (1999b). CREB and COUP-TF mediate transcriptional activation of the human immunodeficiency virus type 1 genome in Jurkat T cells in response to cyclic AMP and dopamine. J. Cell. Biochem. 75, 404–413.
Roshal, M., Zhu, Y., and Planelles, V. (2001). Apoptosis in AIDS. Apoptosis 6, 103–116. doi: 10.1023/A:1009636530839
Rumbaugh, J. A., Li, G., Rothstein, J., and Nath, A. (2007). Ceftriaxone protects against the neurotoxicity of human immunodeficiency virus proteins. J. Neurovirol. 13, 168–172. doi: 10.1080/13550280601178218
Rumbaugh, J. A., Steiner, J., Sacktor, N., and Nath, A. (2008). Developing neuroprotective strategies for treatment of HIV-associated neurocognitive dysfunction. Futur HIV Ther. 2, 271–280. doi: 10.2217/17469600.2.3.271
Sabatier, J. M., Vives, E., Mabrouk, K., Benjouad, A., Rochat, H., Duval, A., et al. (1991). Evidence for neurotoxic activity of tat from human immunodeficiency virus type 1. J. Virol. 65, 961–967.
Sacktor, N., Miyahara, S., Deng, L., Evans, S., Schifitto, G., Cohen, B. A., et al. (2011). Minocycline treatment for HIV-associated cognitive impairment: results from a randomized trial. Neurology 77, 1135–1142. doi: 10.1212/WNL.0b013e31822f0412
Sacktor, N., Miyahara, S., Evans, S., Schifitto, G., Cohen, B., Haughey, N., et al. (2014). Impact of minocycline on cerebrospinal fluid markers of oxidative stress, neuronal injury, and inflammation in HIV-seropositive individuals with cognitive impairment. J. Neurovirol. 20, 620–626. doi: 10.1007/s13365-014-0292-0
Saha, R. N., and Pahan, K. (2006a). Regulation of inducible nitric oxide synthase gene in glial cells. Antioxid. Redox Signal. 8, 929–947. doi: 10.1089/ars.2006.8.929
Saha, R. N., and Pahan, K. (2006b). Signals for the induction of nitric oxide synthase in astrocytes. Neurochem. Int. 49, 154–163. doi: 10.1016/j.neuint.2006.04.007
Salamanca, S. A., Sorrentino, E. E., Nosanchuk, J. D., and Martinez, L. R. (2014). Impact of methamphetamine on infection and immunity. Front. Neurosci. 8:445. doi: 10.3389/fnins.2014.00445
Sanchez Mejia, R. O., Ona, V. O., Li, M., and Friedlander, R. M. (2001). Minocycline reduces traumatic brain injury-mediated caspase-1 activation, tissue damage, and neurological dysfunction. Neurosurgery 48, 1393–1399; discussion 1399–1401.
Sanchez-Alavez, M., Conti, B., Wood, M. R., Bortell, N., Bustamante, E., Saez, E., et al. (2013). ROS and sympathetically mediated mitochondria activation in brown adipose tissue contribute to methamphetamine-induced hyperthermia. Front. Endocrinol. (Lausanne) 4:44. doi: 10.3389/fendo.2013.00044
Sanchez-Alavez, M., Bortell, N., Galmozzi, A., Conti, B., and Marcondes, M. C. (2014). Reactive Oxygen species scavenger N-acetyl cysteine reduces methamphetamine-induced hyperthermia without affecting motor activity in mice. Temperature 1, 227–241. doi: 10.4161/23328940.2014.984556
Saunders, M., Eldeen, M. B., Del Valle, L., Reiss, K., Peruzzi, F., Mameli, G., et al. (2005). p73 modulates HIV-1 Tat transcriptional and apoptotic activities in human astrocytes. Apoptosis 10, 1419–1431. doi: 10.1007/s10495-005-2467-x
Schieber, M., and Chandel, N. S. (2014). ROS function in redox signaling and oxidative stress. Curr. Biol. 24, R453–R462. doi: 10.1016/j.cub.2014.03.034
Sekine, Y., Ouchi, Y., Sugihara, G., Takei, N., Yoshikawa, E., Nakamura, K., et al. (2008). Methamphetamine causes microglial activation in the brains of human abusers. J. Neurosci. 28, 5756–5761. doi: 10.1523/jneurosci.1179-08.2008
Self, R. L., Mulholland, P. J., Harris, B. R., Nath, A., and Prendergast, M. A. (2004). Cytotoxic effects of exposure to the human immunodeficiency virus type 1 protein Tat in the hippocampus are enhanced by prior ethanol treatment. Alcohol. Clin. Exp. Res. 28, 1916–1924. doi: 10.1097/01.ALC.0000148108.93782.05
Seminerio, M. J., Robson, M. J., Mccurdy, C. R., and Matsumoto, R. R. (2012). Sigma receptor antagonists attenuate acute methamphetamine-induced hyperthermia by a mechanism independent of IL-1beta mRNA expression in the hypothalamus. Eur. J. Pharmacol. 691, 103–109. doi: 10.1016/j.ejphar.2012.07.029
Shah, A., Silverstein, P. S., Singh, D. P., and Kumar, A. (2012). Involvement of metabotropic glutamate receptor 5, AKT/PI3K signaling and NF-kappaB pathway in methamphetamine-mediated increase in IL-6 and IL-8 expression in astrocytes. J. Neuroinflamm. 9, 52. doi: 10.1186/1742-2094-9-52
Sharma, A., Hu, X. T., Napier, T. C., and Al-Harthi, L. (2011). Methamphetamine and HIV-1 Tat down regulate beta-catenin signaling: implications for methampetamine abuse and HIV-1 co-morbidity. J. Neuroimmune Pharmacol. 6, 597–607. doi: 10.1007/s11481-011-9295-2
Sheng, W. S., Hu, S., Lokensgard, J. R., and Peterson, P. K. (2003). U50,488 inhibits HIV-1 Tat-induced monocyte chemoattractant protein-1 (CCL2) production by human astrocytes. Biochem. Pharmacol. 65, 9–14. doi: 10.1016/S0006-2952(02)01480-6
Shi, B., Raina, J., Lorenzo, A., Busciglio, J., and Gabuzda, D. (1998). Neuronal apoptosis induced by HIV-1 Tat protein and TNF-alpha: potentiation of neurotoxicity mediated by oxidative stress and implications for HIV-1 dementia. J. Neurovirol. 4, 281–290.
Shin, E. J., Shin, S. W., Nguyen, T. T., Park, D. H., Wie, M. B., Jang, C. G., et al. (2014). Ginsenoside Re rescues methamphetamine-induced oxidative damage, mitochondrial dysfunction, microglial activation, and dopaminergic degeneration by inhibiting the protein kinase Cdelta gene. Mol. Neurobiol. 49, 1400–1421. doi: 10.1007/s12035-013-8617-1
Silvers, J. M., Aksenova, M. V., Aksenov, M. Y., Mactutus, C. F., and Booze, R. M. (2007). Neurotoxicity of HIV-1 Tat protein: involvement of D1 dopamine receptor. Neurotoxicology 28, 1184–1190. doi: 10.1016/j.neuro.2007.07.005
Silverstein, P. S., Shah, A., Gupte, R., Liu, X., Piepho, R. W., Kumar, S., et al. (2011). Methamphetamine toxicity and its implications during HIV-1 infection. J. Neurovirol. 17, 401–415. doi: 10.1007/s13365-011-0043-4
Sinchai, T., Plasen, S., Sanvarinda, Y., Jaisin, Y., Govitrapong, P., Morales, N. P., et al. (2011). Caffeine potentiates methamphetamine-induced toxicity both in vitro and in vivo. Neurosci. Lett. 502, 65–69. doi: 10.1016/j.neulet.2011.07.026
Singh, M., Singh, P., Vaira, D., Amand, M., Rahmouni, S., and Moutschen, M. (2014). Minocycline attenuates HIV-1 infection and suppresses chronic immune activation in humanized NOD/LtsZ-scidIL-2Rgamma(null) mice. Immunology 142, 562–572. doi: 10.1111/imm.12246
Singh, V. B., Wooten, A. K., Jackson, J. W., Maggirwar, S. B., and Kiebala, M. (2015). Investigating the role of ankyrin-rich membrane spanning protein in human immunodeficiency virus type-1 Tat-induced microglia activation. J. Neurovirol. 21, 186–198. doi: 10.1007/s13365-015-0318-2
Smith, D. G., Guillemin, G. J., Pemberton, L., Kerr, S., Nath, A., Smythe, G. A., et al. (2001). Quinolinic acid is produced by macrophages stimulated by platelet activating factor, Nef and Tat. J. Neurovirol. 7, 56–60. doi: 10.1080/135502801300069692
Snider, S. E., Hendrick, E. S., and Beardsley, P. M. (2013). Glial cell modulators attenuate methamphetamine self-administration in the rat. Eur. J. Pharmacol. 701, 124–130. doi: 10.1016/j.ejphar.2013.01.016
Snider, S. E., Vunck, S. A., Van Den Oord, E. J., Adkins, D. E., Mcclay, J. L., and Beardsley, P. M. (2012). The glial cell modulators, ibudilast and its amino analog, AV1013, attenuate methamphetamine locomotor activity and its sensitization in mice. Eur. J. Pharmacol. 679, 75–80. doi: 10.1016/j.ejphar.2012.01.013
Sofuoglu, M., Mooney, M., Kosten, T., Waters, A., and Hashimoto, K. (2011). Minocycline attenuates subjective rewarding effects of dextroamphetamine in humans. Psychopharmacology (Berl.) 213, 61–68. doi: 10.1007/s00213-010-2014-5
Solhi, H., Malekirad, A., Kazemifar, A. M., and Sharifi, F. (2014). Oxidative stress and lipid peroxidation in prolonged users of methamphetamine. Drug. Metab. Lett. 7, 79–82.
Song, H. Y., Ju, S. M., Seo, W. Y., Goh, A. R., Lee, J. K., Bae, Y. S., et al. (2011). Nox2-based NADPH oxidase mediates HIV-1 Tat-induced up-regulation of VCAM-1/ICAM-1 and subsequent monocyte adhesion in human astrocytes. Free Radic Biol. Med. 50, 576–584. doi: 10.1016/j.freeradbiomed.2010.12.019
Song, L., Nath, A., Geiger, J. D., Moore, A., and Hochman, S. (2003). Human immunodeficiency virus type 1 Tat protein directly activates neuronal N-methyl-D-aspartate receptors at an allosteric zinc-sensitive site. J. Neurovirol. 9, 399–403. doi: 10.1080/13550280390201704
Sporer, B., Linke, R., Seelos, K., Paul, R., Klopstock, T., and Pfister, H. W. (2005). HIV-induced chorea: evidence for basal ganglia dysregulation by SPECT. J. Neurol. 252, 356–358. doi: 10.1007/s00415-005-0626-1
Spudich, S. (2013). HIV and neurocognitive dysfunction. Curr. HIV/AIDS Rep. 10, 235–243. doi: 10.1007/s11904-013-0171-y
Stephans, S. E., and Yamamoto, B. K. (1994). Methamphetamine-induced neurotoxicity: roles for glutamate and dopamine efflux. Synapse 17, 203–209. doi: 10.1002/syn.890170310
Stevens, M. W., Tawney, R. L., West, C. M., Kight, A. D., Henry, R. L., Owens, S. M., et al. (2014). Preclinical characterization of an anti-methamphetamine monoclonal antibody for human use. MAbs 6, 547–555. doi: 10.4161/mabs.27620
Stone, T. W. (1993). Neuropharmacology of quinolinic and kynurenic acids. Pharmacol. Rev. 45, 309–379.
Strazza, M., Pirrone, V., Wigdahl, B., and Nonnemacher, M. R. (2011). Breaking down the barrier: the effects of HIV-1 on the blood-brain barrier. Brain Res. 1399, 96–115. doi: 10.1016/j.brainres.2011.05.015
Suryavanshi, P. S., Ugale, R. R., Yilmazer-Hanke, D., Stairs, D. J., and Dravid, S. M. (2014). GluN2C/GluN2D subunit-selective NMDA receptor potentiator CIQ reverses MK-801-induced impairment in prepulse inhibition and working memory in Y-maze test in mice. Br. J. Pharmacol. 171, 799–809. doi: 10.1111/bph.12518
Suzumura, A., Ito, A., and Mizuno, T. (2003). Phosphodiesterase inhibitors suppress IL-12 production with microglia and T helper 1 development. Mult. Scler. 9, 574–578. doi: 10.1191/1352458503ms970oa
Suzumura, A., Ito, A., Yoshikawa, M., and Sawada, M. (1999). Ibudilast suppresses TNFalpha production by glial cells functioning mainly as type III phosphodiesterase inhibitor in the CNS. Brain Res. 837, 203–212.
Talloczy, Z., Martinez, J., Joset, D., Ray, Y., Gacser, A., Toussi, S., et al. (2008). Methamphetamine inhibits antigen processing, presentation, and phagocytosis. PLoS Pathog. 4:e28. doi: 10.1371/journal.ppat.0040028
Tewari, M., Monika, Varghse, R. K., Menon, M., and Seth, P. (2015). Astrocytes mediate HIV-1 Tat-induced neuronal damage via ligand-gated ion channel P2X7R. J. Neurochem. 132, 464–476. doi: 10.1111/jnc.12953
Theodore, S., Cass, W. A., Dwoskin, L. P., and Maragos, W. F. (2012). HIV-1 protein Tat inhibits vesicular monoamine transporter-2 activity in rat striatum. Synapse 66, 755–757. doi: 10.1002/syn.21564
Theodore, S., Cass, W. A., and Maragos, W. F. (2006a). Involvement of cytokines in human immunodeficiency virus-1 protein Tat and methamphetamine interactions in the striatum. Exp. Neurol. 199, 490–498. doi: 10.1016/j.expneurol.2006.01.009
Theodore, S., Cass, W. A., and Maragos, W. F. (2006b). Methamphetamine and human immunodeficiency virus protein Tat synergize to destroy dopaminergic terminals in the rat striatum. Neuroscience 137, 925–935. doi: 10.1016/j.neuroscience.2005.10.056
Theodore, S., Cass, W. A., Nath, A., Steiner, J., Young, K., and Maragos, W. F. (2006c). Inhibition of tumor necrosis factor-alpha signaling prevents human immunodeficiency virus-1 protein Tat and methamphetamine interaction. Neurobiol. Dis. 23, 663–668. doi: 10.1016/j.nbd.2006.05.005
Theodore, S., Stolberg, S., Cass, W. A., and Maragos, W. F. (2006d). Human immunodeficiency virus-1 protein tat and methamphetamine interactions. Ann. N. Y. Acad. Sci. 1074, 178–190. doi: 10.1196/annals.1369.018
Theodore, S., Cass, W. A., Nath, A., and Maragos, W. F. (2007). Progress in understanding basal ganglia dysfunction as a common target for methamphetamine abuse and HIV-1 neurodegeneration. Curr. HIV Res. 5, 301–313. doi: 10.2174/157016207780636515
Thomas, E. R., Dunfee, R. L., Stanton, J., Bogdan, D., Kunstman, K., Wolinsky, S. M., et al. (2007). High frequency of defective vpu compared with tat and rev genes in brain from patients with HIV type 1-associated dementia. AIDS Res. Hum. Retrovir. 23, 575–580. doi: 10.1089/aid.2006.0246
Tikka, T., Fiebich, B. L., Goldsteins, G., Keinanen, R., and Koistinaho, J. (2001). Minocycline, a tetracycline derivative, is neuroprotective against excitotoxicity by inhibiting activation and proliferation of microglia. J. Neurosci. 21, 2580–2588.
Tikka, T. M., and Koistinaho, J. E. (2001). Minocycline provides neuroprotection against N-methyl-D-aspartate neurotoxicity by inhibiting microglia. J. Immunol. 166, 7527–7533. doi: 10.4049/jimmunol.166.12.7527
Torre, D., Pugliese, A., and Speranza, F. (2002). Role of nitric oxide in HIV-1 infection: friend or foe? Lancet Infect. Dis. 2, 273–280. doi: 10.1016/S1473-3099(02)00262-1
Toussi, S. S., Joseph, A., Zheng, J. H., Dutta, M., Santambrogio, L., and Goldstein, H. (2009). Short communication: methamphetamine treatment increases in vitro and in vivo HIV replication. AIDS Res. Hum. Retrovir. 25, 1117–1121. doi: 10.1089/aid.2008.0282
Turchan, J., Anderson, C., Hauser, K. F., Sun, Q., Zhang, J., Liu, Y., et al. (2001). Estrogen protects against the synergistic toxicity by HIV proteins, methamphetamine and cocaine. BMC Neurosci. 2:3. doi: 10.1186/1471-2202-2-3
Turchan-Cholewo, J., Dimayuga, F. O., Gupta, S., Keller, J. N., Knapp, P. E., Hauser, K. F., et al. (2009a). Morphine and HIV-Tat increase microglial-free radical production and oxidative stress: possible role in cytokine regulation. J. Neurochem. 108, 202–215. doi: 10.1111/j.1471-4159.2008.05756.x
Turchan-Cholewo, J., Dimayuga, V. M., Gupta, S., Gorospe, R. M., Keller, J. N., and Bruce-Keller, A. J. (2009b). NADPH oxidase drives cytokine and neurotoxin release from microglia and macrophages in response to HIV-Tat. Antioxid. Redox Signal. 11, 193–204. doi: 10.1089/ars.2008.2097
Turowski, P., and Kenny, B. A. (2015). The blood-brain barrier and methamphetamine: open sesame? Front. Neurosci. 9:156. doi: 10.3389/fnins.2015.00156
Tyor, W. R., Glass, J. D., Griffin, J. W., Becker, P. S., Mcarthur, J. C., Bezman, L., et al. (1992). Cytokine expression in the brain during the acquired immunodeficiency syndrome. Ann. Neurol. 31, 349–360. doi: 10.1002/ana.410310402
Underwood, J., Robertson, K. R., and Winston, A. (2015). Could antiretroviral neurotoxicity play a role in the pathogenesis of cognitive impairment in treated HIV disease? AIDS 29, 253–261. doi: 10.1097/qad.0000000000000538
Urrutia, A., Rubio-Araiz, A., Gutierrez-Lopez, M. D., Elali, A., Hermann, D. M., O’Shea, E., et al. (2013). A study on the effect of JNK inhibitor, SP600125, on the disruption of blood-brain barrier induced by methamphetamine. Neurobiol. Dis. 50, 49–58. doi: 10.1016/j.nbd.2012.10.006
Valcour, V., Chalermchai, T., Sailasuta, N., Marovich, M., Lerdlum, S., Suttichom, D., et al. (2012). Central nervous system viral invasion and inflammation during acute HIV infection. J. Infect. Dis. 206, 275–282. doi: 10.1093/infdis/jis326
Valderas, J. M., Starfield, B., Sibbald, B., Salisbury, C., and Roland, M. (2009). Defining comorbidity: implications for understanding health and health services. Ann. Fam. Med. 7, 357–363. doi: 10.1370/afm.983
Van Den Bosch, L., Tilkin, P., Lemmens, G., and Robberecht, W. (2002). Minocycline delays disease onset and mortality in a transgenic model of ALS. Neuroimage 13, 1067–1070. doi: 10.1097/00001756-200206120-00018
Van Grol, J., Subauste, C., Andrade, R. M., Fujinaga, K., Nelson, J., and Subauste, C. S. (2010). HIV-1 inhibits autophagy in bystander macrophage/monocytic cells through Src-Akt and STAT3. PLoS ONE 5:e11733. doi: 10.1371/journal.pone.0011733
Vives, E., Brodin, P., and Lebleu, B. (1997). A truncated HIV-1 Tat protein basic domain rapidly translocates through the plasma membrane and accumulates in the cell nucleus. J. Biol. Chem. 272, 16010–16017.
Wakita, H., Tomimoto, H., Akiguchi, I., Lin, J. X., Ihara, M., Ohtani, R., et al. (2003). Ibudilast, a phosphodiesterase inhibitor, protects against white matter damage under chronic cerebral hypoperfusion in the rat. Brain Res. 992, 53–59. doi: 10.1016/j.brainres.2003.08.028
Walker, J., Winhusen, T., Storkson, J. M., Lewis, D., Pariza, M. W., Somoza, E., et al. (2014). Total antioxidant capacity is significantly lower in cocaine-dependent and methamphetamine-dependent patients relative to normal controls: results from a preliminary study. Hum. Psychopharmacol. 29, 537–543. doi: 10.1002/hup.2430
Wan, Z., and Chen, X. (2014). Triptolide inhibits human immunodeficiency virus type 1 replication by promoting proteasomal degradation of Tat protein. Retrovirology 11, 88. doi: 10.1186/s12977-014-0088-6
Wang, J., Qian, W., Liu, J., Zhao, J., Yu, P., Jiang, L., et al. (2014). Effect of methamphetamine on the microglial damage: role of potassium channel Kv1.3. PLoS ONE 9:e88642. doi: 10.1371/journal.pone.0088642
Wang, L., Flannery, P. J., Rosenberg, P. B., Fields, T. A., and Spurney, R. F. (2008). Gq-dependent signaling upregulates COX2 in glomerular podocytes. J. Am. Soc. Nephrol. 19, 2108–2118. doi: 10.1681/asn.2008010113
Weeks, B. S., Lieberman, D. M., Johnson, B., Roque, E., Green, M., Loewenstein, P., et al. (1995). Neurotoxicity of the human immunodeficiency virus type 1 tat transactivator to PC12 cells requires the Tat amino acid 49–58 basic domain. J. Neurosci. Res. 42, 34–40. doi: 10.1002/jnr.490420105
Weiss, J. M., Nath, A., Major, E. O., and Berman, J. W. (1999). HIV-1 Tat induces monocyte chemoattractant protein-1-mediated monocyte transmigration across a model of the human blood-brain barrier and up-regulates CCR5 expression on human monocytes. J. Immunol. 163, 2953–2959.
Wesselingh, S. L., Power, C., Glass, J. D., Tyor, W. R., Mcarthur, J. C., Farber, J. M., et al. (1993). Intracerebral cytokine messenger RNA expression in acquired immunodeficiency syndrome dementia. Ann. Neurol. 33, 576–582. doi: 10.1002/ana.410330604
Wiley, C. A., Baldwin, M., and Achim, C. L. (1996). Expression of HIV regulatory and structural mRNA in the central nervous system. AIDS 10, 843–847.
Williams, R., Yao, H., Peng, F., Yang, Y., Bethel-Brown, C., and Buch, S. (2010). Cooperative induction of CXCL10 involves NADPH oxidase: implications for HIV dementia. Glia 58, 611–621. doi: 10.1002/glia.20949
Wires, E. S., Alvarez, D., Dobrowolski, C., Wang, Y., Morales, M., Karn, J., et al. (2012). Methamphetamine activates nuclear factor kappa-light-chain-enhancer of activated B cells (NF-kappaB) and induces human immunodeficiency virus (HIV) transcription in human microglial cells. J. Neurovirol. 18, 400–410. doi: 10.1007/s13365-012-0103-4
Wright, S. C., Jewett, A., Mitsuyasu, R., and Bonavida, B. (1988). Spontaneous cytotoxicity and tumor necrosis factor production by peripheral blood monocytes from AIDS patients. J. Immunol. 141, 99–104.
Wu, D. C., Jackson-Lewis, V., Vila, M., Tieu, K., Teismann, P., Vadseth, C., et al. (2002). Blockade of microglial activation is neuroprotective in the 1-methyl-4-phenyl-1,2,3,6-tetrahydropyridine mouse model of Parkinson disease. J. Neurosci. 22, 1763–1771.
Xiao, H., Neuveut, C., Tiffany, H. L., Benkirane, M., Rich, E. A., Murphy, P. M., et al. (2000). Selective CXCR4 antagonism by Tat: implications for in vivo expansion of coreceptor use by HIV-1. Proc. Natl. Acad. Sci. U.S.A. 97, 11466–11471. doi: 10.1073/pnas.97.21.11466
Xie, Z., and Miller, G. M. (2009). A receptor mechanism for methamphetamine action in dopamine transporter regulation in brain. J. Pharmacol. Exp. Ther. 330, 316–325. doi: 10.1124/jpet.109.153775
Xu, R., Feng, X., Xie, X., Zhang, J., Wu, D., and Xu, L. (2012). HIV-1 Tat protein increases the permeability of brain endothelial cells by both inhibiting occludin expression and cleaving occludin via matrix metalloproteinase-9. Brain Res. 1436, 13–19. doi: 10.1016/j.brainres.2011.11.052
Yang, Y., Wu, J., and Lu, Y. (2010). Mechanism of HIV-1-TAT induction of interleukin-1beta from human monocytes: involvement of the phospholipase C/protein kinase C signaling cascade. J. Med. Virol. 82, 735–746. doi: 10.1002/jmv.21720
Yong, V. W., Krekoski, C. A., Forsyth, P. A., Bell, R., and Edwards, D. R. (1998). Matrix metalloproteinases and diseases of the CNS. Trends Neurosci. 21, 75–80.
Yu, S., Zhu, L., Shen, Q., Bai, X., and Di, X. (2015). Recent advances in methamphetamine neurotoxicity mechanisms and its molecular pathophysiology. Behav. Neurol. 2015, 103969. doi: 10.1155/2015/103969
Zaczek, R., Culp, S., and De Souza, E. B. (1991). Interactions of [3H]amphetamine with rat brain synaptosomes. II. Active transport. J. Pharmacol. Exp. Ther. 257, 830–835.
Zauli, G., Secchiero, P., Rodella, L., Gibellini, D., Mirandola, P., Mazzoni, M., et al. (2000). HIV-1 Tat-mediated inhibition of the tyrosine hydroxylase gene expression in dopaminergic neuronal cells. J. Biol. Chem. 275, 4159–4165. doi: 10.1074/jbc.275.6.4159
Zhang, H. S., Li, H. Y., Zhou, Y., Wu, M. R., and Zhou, H. S. (2009). Nrf2 is involved in inhibiting Tat-induced HIV-1 long terminal repeat transactivation. Free Radic Biol. Med. 47, 261–268. doi: 10.1016/j.freeradbiomed.2009.04.028
Zhang, S., Jin, Y., Liu, X., Yang, L., Ge, Z., Wang, H., et al. (2014). Methamphetamine modulates glutamatergic synaptic transmission in rat primary cultured hippocampal neurons. Brain Res. 1582, 1–11. doi: 10.1016/j.brainres.2014.07.040
Zhang, Y., Lv, X., Bai, Y., Zhu, X., Wu, X., Chao, J., et al. (2015). Involvement of sigma-1 receptor in astrocyte activation induced by methamphetamine via up-regulation of its own expression. J. Neuroinflamm. 12, 29. doi: 10.1186/s12974-015-0250-7
Zhao, L., Pu, S. S., Gao, W. H., Chi, Y. Y., Wen, H. L., Wang, Z. Y., et al. (2014). Effects of HIV-1 tat on secretion of TNF-alpha and IL-1beta by U87 cells in AIDS patients with or without AIDS dementia complex. Biomed. Environ. Sci. 27, 111–117. doi: 10.3967/bes2014.024
Zhong, Y., Hennig, B., and Toborek, M. (2010). Intact lipid rafts regulate HIV-1 Tat protein-induced activation of the Rho signaling and upregulation of P-glycoprotein in brain endothelial cells. J. Cereb. Blood Flow Metab. 30, 522–533. doi: 10.1038/jcbfm.2009.214
Zhong, Y., Smart, E. J., Weksler, B., Couraud, P. O., Hennig, B., and Toborek, M. (2008). Caveolin-1 regulates human immunodeficiency virus-1 Tat-induced alterations of tight junction protein expression via modulation of the Ras signaling. J. Neurosci. 28, 7788–7796. doi: 10.1523/jneurosci.0061-08.2008
Zhou, B. Y., Liu, Y., Kim, B., Xiao, Y., and He, J. J. (2004). Astrocyte activation and dysfunction and neuron death by HIV-1 Tat expression in astrocytes. Mol. Cell. Neurosci. 27, 296–305. doi: 10.1016/j.mcn.2004.07.003
Zhu, J., Ananthan, S., Mactutus, C. F., and Booze, R. M. (2011). Recombinant human immunodeficiency virus-1 transactivator of transcription1-86 allosterically modulates dopamine transporter activity. Synapse 65, 1251–1254. doi: 10.1002/syn.20949
Zhu, J., Mactutus, C. F., Wallace, D. R., and Booze, R. M. (2009). HIV-1 Tat protein-induced rapid and reversible decrease in [3H]dopamine uptake: dissociation of [3H]dopamine uptake and [3H]2beta-carbomethoxy-3-beta-(4-fluorophenyl)tropane (WIN 35,428) binding in rat striatal synaptosomes. J. Pharmacol. Exp. Ther. 329, 1071–1083. doi: 10.1124/jpet.108.150144
Zidovetzki, R., Wang, J. L., Chen, P., Jeyaseelan, R., and Hofman, F. (1998). Human immunodeficiency virus Tat protein induces interleukin 6 mRNA expression in human brain endothelial cells via protein kinase C- and cAMP-dependent protein kinase pathways. AIDS Res. Hum. Retrovir. 14, 825–833.
Keywords: methamphetamine, HAND, HIV-1 Tat, antiretroviral therapy, neurotoxicity
Citation: Mediouni S, Marcondes MCG, Miller C, McLaughlin JP and Valente ST (2015) The cross-talk of HIV-1 Tat and methamphetamine in HIV-associated neurocognitive disorders. Front. Microbiol. 6:1164. doi: 10.3389/fmicb.2015.01164
Received: 30 June 2015; Accepted: 07 October 2015;
Published: 23 October 2015.
Edited by:
Venkata S. R. Atluri, Florida International University, USAReviewed by:
Masanori Baba, Kagoshima University, JapanShilpa J. Buch, University of Nebraska Medical Center, USA
Peter S. Silverstein, University of Missouri-Kansas City, USA
Copyright © 2015 Valente, Mediouni, Miller, Marcondes and McLaughlin. This is an open-access article distributed under the terms of the Creative Commons Attribution License (CC BY). The use, distribution or reproduction in other forums is permitted, provided the original author(s) or licensor are credited and that the original publication in this journal is cited, in accordance with accepted academic practice. No use, distribution or reproduction is permitted which does not comply with these terms.
*Correspondence: Susana T. Valente, c3ZhbGVudGVAc2NyaXBwcy5lZHU=