- 1Center for AIDS Health Disparities Research, Meharry Medical College, Nashville, TN, USA
- 2School of Graduate Studies and Research, Meharry Medical College, Nashville, TN, USA
- 3Department of Biochemistry and Cancer Biology, Meharry Medical College, Nashville, TN, USA
- 4Department of Microbiology and Immunology, Meharry Medical College, Nashville, TN, USA
Over 1.2 million people in the United States are infected with the human immunodeficiency virus type 1 (HIV-1). Tremendous progress has been made over the past three decades on many fronts in the prevention and treatment of HIV-1 disease. However, HIV-1 infection is incurable and antiretroviral drugs continue to remain the only effective treatment option for HIV infected patients. Unfortunately, only three out of ten HIV-1 infected individuals in the US have the virus under control. Thus, majority of HIV-1 infected individuals in the US are either unaware of their infection status or not connected/retained to care or are non-adherent to antiretroviral therapy (ART). This national public health crisis, as well as the ongoing global HIV/AIDS pandemic, is further exacerbated by substance abuse, which serves as a powerful cofactor at every stage of HIV/AIDS including transmission, diagnosis, pathogenesis, and treatment. Clinical studies indicate that substance abuse may increase viral load, accelerate disease progression and worsen AIDS-related mortality even among ART-adherent patients. However, confirming a direct causal link between substance abuse and HIV/AIDS in human patients remains a highly challenging endeavor. In this review we will discuss the recent and past developments in clinical and basic science research on the effects of cocaine abuse on HIV-1 pathogenesis.
Introduction
The ongoing HIV/AIDS pandemic has claimed around 39 million human lives, and over 35 million individuals are currently living with HIV-11 infection (Centers for Disease Control and Prevention, 2012). HIV/AIDS research, despite making tremendous strides in the last three decades, is yet to yield a preventative vaccine. Antiretroviral therapy (ART) continues to remain the sole treatment option and the current standard of care for HIV-infected individuals (Walensky et al., 2006). ART has dramatically reduced HIV/AIDS-related mortality and has been highly effective in controlling the virus in ART-adherent patients (Walensky et al., 2006). Recent studies also suggest that ART prophylaxis may help reduce transmission of HIV-1 among high-risk groups (Grant et al., 2010; Cohen et al., 2011; Marrazzo et al., 2015; Siemieniuk and Bogoch, 2015). Despite such advances in managing the HIV/AIDS disease, latest report from the Centers of Disease Control and Prevention (CDC) indicate that over two thirds of the 1.2 million living with HIV-1 infection in the US do not have their virus under control (Centers for Disease Control and Prevention, 2012). Perhaps, a more troubling statistic is that, around 20% of those individuals are unaware of their status (Centers for Disease Control and Prevention, 2012), which also highlights the challenges in controlling the HIV epidemic even in a resource rich setting. The HIV/AIDS crisis is further exacerbated by substance abuse that serves as a powerful cofactor at every stage of HIV epidemic including transmission, diagnosis, pathogenesis, and adherence to therapy (Friedman et al., 2006; Sohler et al., 2007; Cofrancesco et al., 2008; Kipp et al., 2011). Clinical studies implicate substance abuse in increased viral load, accelerated disease progression and worsening of AIDS-related mortality—even among ART-adherent patients (Anthony et al., 1991; Arnsten et al., 2001, 2002; Friedman et al., 2006; Sohler et al., 2007; Cofrancesco et al., 2008; Kipp et al., 2011). Therefore, substance abuse continues to be a major obstacle in combating this global pandemic. It is increasingly becoming evident that drugs such as methamphetamine, cocaine, opiates, marijuana, and others regulate HIV-1 infection/replication in vitro and in animal models (Wang and Ho, 2011). Furthermore, new research is shedding light on the negative effects of substance abuse on immune system that controls viral infections (Wang and Ho, 2011). Overall, these studies are paving ways to better understand the comorbid condition of drug use and HIV/AIDS. In this review we will focus on the effects of cocaine abuse on HIV-1 pathogenesis, with a special emphasis on the implications of the interplay between cocaine and the virus in the brain.
Cocaine Abuse and Mechanism of Action
Cocaine, a commonly used illicit drug, is an alkaloid derived from the leaves of the coca plant Erythroxylum coca. According to the National Survey on Drug Use and Health (NSDUH), in 2013, around 1.5 million Americans aged 12 and older had abused cocaine in some form2. Cocaine is a potent vasoconstrictor and a powerful stimulant of the brain, and abuse of this compound leads to severe medical complications including neurological effects (headaches, fainting attacks, hemorrhagic brain strokes, central nervous system (CNS) vasculitis and encephalopathies), cardiovascular effects (cardiac arrhythmia and heart attacks), and gastrointestinal complications (Daras, 1996; Riezzo et al., 2012; Marasco et al., 2014). Delirium and seizures significantly contribute to the cocaine-induced morbidity and mortality, while movement disorders and cognitive motor dysfunction are also observed in long-term cocaine users (Nnadi et al., 2005). Cocaine primarily enhances the dopamine neurotransmission in the brain, thereby causing amplification and reactivity of sensory cues (Venton et al., 2006; Little et al., 2009). Cocaine directly elevates the synaptic dopamine levels in the mesocorticolimbic system by binding to the presynaptic dopamine transporters (DATs) and inhibiting dopamine reuptake (Ritz et al., 1987; Kalivas and Volkow, 2005; Marasco et al., 2014). This inhibition is considered to be the main contributor to the reinforcement and behavioral properties of cocaine. The elevated dopamine levels in the synaptic cleft result in increased activation of type 1 and type 2 dopamine receptors, while the inhibition of dopamine reuptake significantly contributes to the reinforcement and behavioral properties of cocaine (Anderson and Pierce, 2005; Schmidt and Pierce, 2006; Graham et al., 2007). While acute cocaine exposure promotes dopamine activity, chronic cocaine abuse has been shown to deregulate striatal dopamine signaling (Willuhn et al., 2014). Cocaine is also known to block the reuptake of other monoamine neurotransmitters including norepinephrine and serotonin, and also impact other excitatory neurotransmitters like glutamate and its receptors (Howell and Cunningham, 2015).
Effects of Cocaine Abuse on HIV pathogenesis
Substance abuse is associated with increased HIV transmission, delayed diagnosis, delayed initiation of therapy, and poor adherence to therapy, which all constitute important barriers for combating the HIV pandemic (Khalsa and Royal, 2004; Cabral, 2006; Friedman et al., 2006; Kipp et al., 2011). Substance abuse also negatively affects the management of HIV/AIDS as roughly one-third of HIV/AIDS patients are intravenous drug users in the United States (Donahoe, 1990). Several studies have reported associations between drug use and HIV-associated clinical outcomes, including the Women’s Interagency HIV study that found substance abuse to be an independent predictor of progression to AIDS and AIDS-related mortality (Cook et al., 2008), and the report by Baum et al., suggesting that substance abuse accelerates HIV disease progression independent of adherence to ART (Baum et al., 2009). Along with clinical evidence, experimental studies have shown that illicit drugs such as amphetamine, marijuana, opiates, nicotine, and cocaine are associated with immunomodulation and the pathogenesis of AIDS (Friedman et al., 2003; Pandhare and Dash, 2011). These drugs cause immunosuppression, modulate the expression of chemokine receptors on CD4+ T lymphocytes, and influence the outcome of HIV-1 infection (Battjes et al., 1988; Donahoe and Falek, 1988; Nair et al., 2000). It is important to point out that there are also several studies that could not find an association between substance use and HIV/AIDS (Junghans et al., 1999; Pezzotti et al., 1999; Chao et al., 2008). Nevertheless, the high prevalence of substance abuse among HIV-1 infected individuals (Pence et al., 2008) and the lack of molecular studies warrant further research to decipher the effects of substance abuse on HIV pathogenesis.
Cocaine Abuse Accentuates HIV-1 Pathogenesis
Cocaine is a commonly abused drug among HIV-1 infected individuals (Pence et al., 2008) and several studies suggest a link between cocaine use and worsening of HIV disease (Chaisson et al., 1989; Anthony et al., 1991; Siddiqui et al., 1993; Larrat et al., 1996; Cofrancesco et al., 2008; Cook et al., 2008; Baum et al., 2009; Cook, 2011). There is evidence that cocaine use results in lack of virologic suppression and accelerated decline of CD4+ T cells even among ART-adherent patients (Carrico et al., 2008; Cofrancesco et al., 2008; Cook et al., 2008; Cook, 2011; Rasbach et al., 2013). For instance, frequent cocaine users exhibit increased risk of worsening HIV-1 disease progression (Webber et al., 1999; Vittinghoff et al., 2001), active cocaine and alcohol use is a strong predictor of failure to maintain viral suppression (Webber et al., 1999; Arnsten et al., 2001, 2002), and cocaine use may result in inferior virologic and immunologic responses to ART (Lucas et al., 2001, 2002, 2006). However, as mentioned previously, some studies had found no significant association between cocaine abuse and HIV-1 disease progression (Pezzotti et al., 1999; Chao et al., 2008). One potential explanation for this discrepancy could be that the effects of cocaine on disease progression may depend in part to non-adherence to ART, as substance abuse is often associated with reduced adherence and/or access to ART (Hinkin et al., 2007). However, it does not appear to be the case as evidenced from several studies, wherein, even when controlled for ART, cocaine users showed higher viral load and were twice as likely to progress to AIDS (Baum et al., 2009), were found less likely to suppress their viral load while taking ART (Palepu et al., 2003), and active cocaine use was associated with lack of virologic suppression independent of ART adherence (Rasbach et al., 2013). Although the mechanism remains largely unclear, increased viral replication and accelerated CD4+ T cell decline has been suggested to play key roles for the accelerated disease seen in cocaine abusing HIV-1 patients.
Cocaine Enhances HIV-1 Replication
In vivo studies on the interactions between cocaine and HIV-1 under physiologic conditions have been conducted in a human-mouse hybrid model (huPBL-SCID mouse; Roth et al., 2002, 2005; Kim et al., 2015). In this model, cocaine administration led to enhanced HIV-1 infection and a significant rise in circulating virus load (Roth et al., 2002, 2005; Kim et al., 2015). Several in vitro studies have also demonstrated the potentiating effects of cocaine on HIV-1 infection/replication in monocyte derived macrophages (MDMs; Dhillon et al., 2007), dendritic cells (Napuri et al., 2013), astrocytes (Reynolds et al., 2006), and peripheral blood mononuclear cells (PBMCs) (Peterson et al., 1991, 1992; Bagasra and Pomerantz, 1993). We and others have shown that cocaine also promotes HIV-1 infection/replication in CD4+ T cells (Mantri et al., 2012; Kim et al., 2013; Addai et al., 2015). Further, compared to non-drug users, cells from cocaine addicts readily supported HIV-1 replication and AIDS-related opportunistic infections in contrast to cells from non-users (Baldwin et al., 1998). Studies have reported that immunomodulatory functions of cocaine may positively contribute to HIV-1 infection and replication. For example, cocaine has been proposed to negatively modulate the expression of HIV-suppressing chemokines and their receptors (Nair et al., 2000; Roth et al., 2005; Reynolds et al., 2009) in target cells. These include RANTES, MIP-1a and MIP-1b, which are all known to inhibit binding/fusion/entry of the virus (Nair et al., 2000, 2001). Furthermore, cocaine has been reported to upregulate the expression of the viral entry co-receptors CXCR4 and CCR5 (Nair et al., 2000, 2001), and, as a result, enhance HIV-1 replication by increasing viral entry. However, accumulating evidence indicate that cocaine enhances HIV-1 infection by targeting both entry and post-entry steps of HIV-1 life cycle (Nair et al., 2000, 2001; Mantri et al., 2012; Addai et al., 2015).
HIV-1 entry into the target cells is facilitated by the binding of viral glycoproteins (gp120 and gp41) to the CD4 receptor and the chemokine co-receptors (CCR5 and CXCR4; Telesnitsky and Goff, 1997). The ensuing fusion of the viral and cell membranes leads to the release of the viral capsid core into the cytoplasm of the target cells (Telesnitsky and Goff, 1997). The subsequent post-entry events of HIV-1 replication can be broadly categorized into reverse transcription, integration, transcription, translation, assembly, virus release and maturation (Telesnitsky and Goff, 1997). We and other research groups have reported that cocaine can modulate post-entry steps of HIV-1 life cycle (Mantri et al., 2012; Kim et al., 2013; Addai et al., 2015). Cocaine has been shown to downregulate the cellular miRNA “miR-125b” in CD4+ T cells (Mantri et al., 2012). The miR-125b is a member of the of anti-HIV miRNA family that suppresses HIV-1 replication by binding to the viral transcripts and inhibiting their translation (Huang et al., 2007). Cocaine was also recently shown to downregulate miR-155 that negatively regulates DC-SIGN (Napuri et al., 2013), which plays a critical role in HIV-1 infection of dendritic cells. A proteomic study of cocaine-treated human astrocytes revealed that cocaine differentially regulates an array of cellular proteins, some or many of which were postulated to potentially modulate HIV-1 replication (Reynolds et al., 2006). Cocaine’s potentiating effect on HIV-1 has been partly attributed to the drug-induced upregulation of sigma-1 receptor, an endoplasmic reticulum-resident molecular chaperone known to bind cocaine. Cocaine’s potentiating effect on HIV-1 has been partly attributed to the drug-induced upregulation of sigma-1 receptor, an endoplasmic reticulum-resident molecular chaperone known to bind cocaine (Ramamoorthy et al., 1995; Roth et al., 2005). More recently, another receptor for cocaine, dopamine D4 receptor (D4R), was shown to mediate the drug-induced enhancement of HIV-1 infection in quiescent CD4+ cells, as evidenced by increased reverse transcription kinetics (Kim et al., 2013). Recently, our group demonstrated that cocaine increases HIV-1 proviral integration in CD4+ T cells (Addai et al., 2015). Increased HIV-1 integration could enhance viral transcription and viral protein production. Cumulatively, these studies provide strong evidence that cocaine can modulate both entry and post-entry steps of HIV-1 infection, thus contributing to increased viral load.
Cocaine’s Effect on CD4+ T cell Decline
CD4+ T cell decline is a powerful diagnostic marker for HIV-1 disease progression (Embretson et al., 1993; Heinkelein et al., 1995; Lane, 2010). Studies suggest that cocaine abusing HIV patients have lower CD4+ T cell counts and accelerated decline in CD4+ T cell number (Chaisson et al., 1989; Anthony et al., 1991; Ruiz et al., 1994; Friedman et al., 2006; Cook, 2011). Compared to non-addicts, individuals addicted to cocaine had significantly reduced T cell population (Ruiz et al., 1994). Further supportive evidence comes from animal studies demonstrating that cocaine decreases the number of circulating lymphocytes (Evans et al., 1996; Pellegrino and Bayer, 1998; Colombo et al., 1999). There is also evidence that cocaine modulates the proliferation of human T cells (Klein et al., 1993; Matsui et al., 1993). Results from in vitro experiments by our laboratory indicate that when CD4+ T cells were treated with both cocaine and HIV-1 virions, the extent of CD4+ T cell apoptosis is significantly higher compared to that of cells treated with cocaine or HIV-1 virions alone (Pandhare et al., 2014). This suggests that, albeit in cell culture system, cocaine and HIV-1 act in a synergistic fashion in inducing CD4+ T cell death (Pandhare et al., 2014). While the precise mechanism by which cocaine synergizes with HIV-1 in the CD4+ T cell decline remains unclear, work done in our laboratory suggest that increase in reactive oxygen species (ROS) may play an important role (Pandhare et al., 2014). Furthermore, it was shown that cocaine downregulates the anti-apoptotic microRNA “miR-125b” in uninfected and infected CD4+ T cells (Mantri et al., 2012). miR-125b is a known negative regulator of the pro-apoptotic gene p53 (Le et al., 2009, 2011), and hence the cocaine-induced downregulation of this miRNA could potentially play an important role in the underlying synergy between cocaine and HIV-1 in CD4+ T cell death. More recently, we also reported that cocaine directly enhances HIV-1 integration in CD4+ T cells (Addai et al., 2015). This conceivably could account for the deleterious effects of cocaine abuse on accelerated CD4+ T cell apoptosis in HIV-1 patients, because increased viral integration has been proposed to induce CD4+ T cell apoptosis (Cooper et al., 2013). Given that both viral load and CD4+ T cell death are important predictors of HIV-1 disease progression, findings from our laboratory and others highlight the potentiating effects of cocaine on HIV-1 disease progression in drug abusing patients.
HIV-1 Infection in the Brain
HIV-1, besides its well-characterized negative effects on the host immune system through depletion of infected CD4+ lymphocytes and immune activation, also broadly impacts the nervous system (Gonzalez-Scarano and Martin-Garcia, 2005; Spudich and Gonzalez-Scarano, 2012). During the early phase of infection, HIV-1 enters the brain and persists in the CNS (Gonzalez-Scarano and Martin-Garcia, 2005; Spudich and Gonzalez-Scarano, 2012). Macrophages and microglia are the primary cell types in the central CNS that can be infected by HIV-1 as well as support viral replication (Koppensteiner et al., 2012). Although neurons are refractory to HIV-1 infection, progressive neuronal damage has been observed in HIV-1 infected patients (Berger and Nath, 1997; Clifford, 2000; Kaul et al., 2001; McArthur et al., 2010). HIV-1 infected individuals suffer from a wide range of neurological disorders. The invasion of the brain by the, leads to a battery of deficits including reduction in motor speed, decline in memory and concentration, CNS injury and neuropathy, which are collectively termed as HIV-associated neurocognitive disorders (HAND; Woods et al., 2009; Patel et al., 2013; Sanmarti et al., 2014). From a clinical perspective, HAND is strongly linked to neurological disorders namely asymptomatic neurocognitive impairment (ANI), mild neurocognitive disorder (MND), and HIV- associated Dementia (HAD)- the most severe form of HAND (Woods et al., 2009; Patel et al., 2013; Sanmarti et al., 2014). The introduction and the widespread use of ART has managed to keep the prevalence of HAD in check. However, the incidences of other milder forms of HAND still persist, are widespread, and are increasing globally (Gray et al., 2003; Tozzi et al., 2007; Joska et al., 2010). HAND affects 20–30% patients in the late stages of AIDS and is believed to be the most common cause of dementia among people aged 40 or less worldwide (Albright et al., 2003; Vivithanaporn et al., 2010). Cognitive, motor, and behavioral dysfunctions are the key phenotypes accompanying neuronal cell injury and death that result from HIV-1 infection of the nervous system in individuals across various age groups (Woods et al., 2009; Patel et al., 2013; Sanmarti et al., 2014). In adult patients, the virus-induced disruption of the nervous system includes targeted damage to regions beneath the cortex, which significantly depletes basal ganglia levels (Aylward et al., 1993; Berger and Nath, 1997). Pathological features of HAND are characterized by monocyte infiltration into brain, formation of macrophage-derived multinucleated giant cells, microglial nodules, gliosis, myelin pallor, and neuronal loss (Gelman, 2015). Both a person’s age and length of HIV-1 infection are key factors for developing HAND because prolonged infection accompanied by a decline in CD4+ T cell count indicates an increased persistence of the virus in the brain (Tozzi et al., 2007; Brew and Letendre, 2008). Further, co-morbidities such as hepatitis C virus (HCV) infection and abuse of drugs such as cocaine also increase the incidence of HAND (Gill and Kolson, 2014; Tedaldi et al., 2015).
Blood–Brain Barrier
The blood–brain barrier (BBB) is a very dynamic structure critical for maintaining brain homeostasis and protecting the brain from pathogens and xenobiotics (Ballabh et al., 2004). The BBB separates the CNS from the periphery and acts as the interface between blood capillaries and the brain parenchyma (Ballabh et al., 2004; Figure 1). The BBB is made up of a continuous layer of tightly linked brain microvascular endothelial cells (BMVECs) that is supported by the basal lamina, followed by the brain parenchyma that consists of astrocytes, pericytes, perivascular macrophages, microglia, and neurons (Ballabh et al., 2004). Pericytes are cells of connective tissue that sustain the BBB by regulating its permeability, capillary blood flow, and phagocytosis of cellular debris (Ivey et al., 2009). The BMVECs, in combination with tight junction proteins, form an unperforated endothelium that confers BBB the selective permeability to peripheral proteins and other external molecules (Stamatovic et al., 2008; Lv et al., 2010). Specifically, BMVECs maintain selective permeability via the luminal membrane-resident transport systems, which modulate the efflux and influx of biomolecules through the BBB (Stamatovic et al., 2008; Lv et al., 2010).
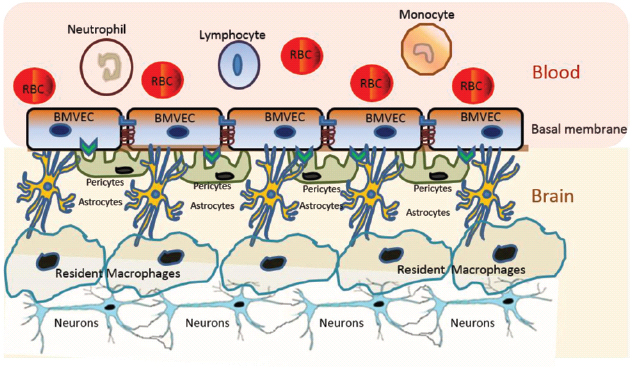
Figure 1. Schematic representation of the BBB. The BBB is made up of a continuous layer of tightly linked BMVECs. The BMVECs are supported by the basal lamina, followed by the brain parenchyma that consists of astrocytes, pericytes, perivascular macrophages, microglia, and neurons. Each component of BBB plays critical roles in maintaining the selective permeability of molecules for neuronal homeostasis and protecting the brain from pathogens and xenobiotics.
Alterations in the Brain and the BBB by HIV-1 Infection
The CNS is a major target for HIV-1 infection and, because many antiretroviral drugs display high variability in penetrating the BBB and reaching therapeutic concentrations, also serves as a reservoir for the virus (Ivey et al., 2009; Stamatovic et al., 2008; Lv et al., 2010). The precise mechanism by which HIV-1 crosses the BBB and causes neuronal dysfunction remains poorly understood. Findings from animal models and in vitro studies provide evidence supporting the “Trojan Horse” model for HIV entry into the brain (Gonzalez-Scarano and Martin-Garcia, 2005; Ivey et al., 2009; Hazleton et al., 2010). This model suggests that HIV-1 virions cross the BBB via trafficking of infected CD4+ cells and monocytes into the CNS (Gonzalez-Scarano and Martin-Garcia, 2005; Ivey et al., 2009; Hazleton et al., 2010). Accordingly, infected monocytes cross the BBB more efficiently than uninfected monocytes (Persidsky, 1999; Persidsky et al., 1999). Further, prevalence of increased numbers of non-proliferating monocytes and brain macrophages in the CNS of HIV-infected patients is observed as a clinical outcome (Fischer-Smith et al., 2004). Infected monocytes that have traversed into the CNS do possess the ability to repopulate as the resident macrophages (Koppensteiner et al., 2012). Despite some evidence pointing to HIV-1 entering the brain by direct migration or transcytosis of endothelial cells through the BBB, the current prevailing model of CNS infection focuses on infected circulating monocytes as viral cargos crossing the BBB into the CNS throughout infection. Once in the brain, HIV-1 can infect perivascular macrophages and microglia, both of which express the CD4+ receptor. Astrocytes can be infected but are not a site of active HIV replication. Neurons do not express the CD4+ receptor and thus are not capable of productive infection; therefore, the HIV-associated neurological complications causing neuronal damage mainly result from inflammatory factors and neurotoxic substances released by infected as well as activated, uninfected cells.
One of the hallmarks of HAND pathogenesis is the breach of the BBB by infected cells. HIV-1 infection has been shown to compromise the BBB integrity (Atluri et al., 2015; Figure 2). Specifically, multiple studies suggest that several HIV-1 proteins can regulate the permeability of the BBB (Toborek et al., 2005). For example, administration of the HIV-1 Trans Activator (Tat) protein, which traverses the BBB potentially by adsorptive endocytosis (Banks, 2005), in mice led to decreased expression of occludin and ZO-1 proteins in the tight junction of BBB (Pu et al., 2007). Further analysis revealed activation of cyclooxygenase 2 (Cox-2) as the mechanistic basis behind the diminished expression of these tight junction proteins (Pu et al., 2007). In vitro treatment of various cell types, including BMVEC’S, with Tat led to increased oxidative stress, which could be attributed to disruption of tight junctions of the BBB (Shiu et al., 2007). In astrocytes, Tat reportedly elevates the expression levels of matrix metalloproteinase-9 (MMP-9; Ju et al., 2009), a member of the zinc-dependent endopeptidases known to cleave the extracellular matrix proteins. Accordingly, MMPs such as MMP-9 have been reported to disrupt the BBB by degrading the extracellular matrix proteins such as laminin and type IV collagen (Yong et al., 1998). Moreover, Tat has been shown to act as a potential chemo attractant in the BBB milieu, which potentially could alter BBB integrity. There is also some evidence that Tat acts as a potential chemo attractant in the BBB milieu that may alter BBB integrity.
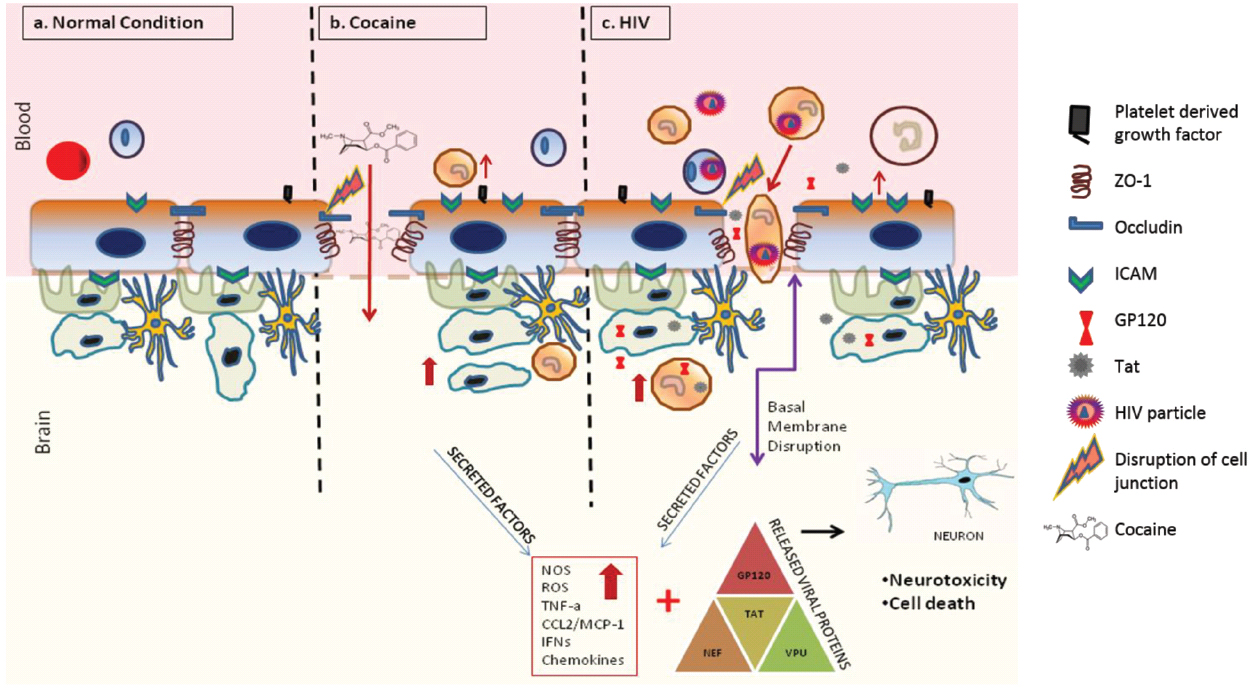
Figure 2. Effects of cocaine and HIV-1 on BBB integrity and neuronal toxicity. Cocaine and HIV-1 infection has been known to disrupt the integrity of BBB. This breach in BBB can increase trafficking of infected cells, viral particles and/or viral proteins into the brain causing systemic neuro-inflammation. Ongoing neuro-inflammation and low level of HIV-1 replication causes neuronal dysfunction and neurotoxicity. Furthermore, drugs of abuse such as cocaine can synergistically accentuate HIV associated neuronal dysfunction and neurotoxicity leading to worsening of HIV-1 neuropathogenesis.
The HIV-1 gp120, which mediates viral entry by binding the CD4 receptor and CXCR4 or CCR5 co-receptor on host cell surface, has also been reported to breach the BBB. For instance, treatment of cultured human BMVECs with gp120 increased monocyte migration and caused a dose-dependent decrease in transendothelial electrical resistance (TEER; Kanmogne et al., 2007). These alterations were postulated to be consequences of increased permeability of BBB caused by the activation of myosin light chain kinase and/or protein kinase C and increased Ca2+ flux (Kanmogne et al., 2007). Furthermore, in vitro treatment of human BMVEC’s with gp120 also decreased BBB tight junction proteins such as ZO-1, ZO-2 and occludin (Kanmogne et al., 2005; Nakamuta et al., 2008). Exposure of THP-1 monocytes to gp120 has also been shown to increase secretion of neurotoxins (Pietraforte et al., 1994). Cytokines could also play a major role in the gp120-induced alteration of the integrity of BBB, as evidenced from studies reporting enhanced expression of pro-inflammatory cytokines due to gp120 treatment in vitro (Ronaldson and Bendayan, 2006). Besides Tat and gp120, other HIV-1 proteins have also been implicated in modulating BBB function (Albright et al., 2003).
Cocaine Abuse and HIV-1 Neuropathogenesis
The complexity of HAND is further exacerbated by co-morbid conditions such as drug abuse. Cocaine has been suggested to increase HAND pathogenesis which continues to be a major challenge in the post ART era (Pakesch et al., 1992; Larrat and Zierler, 1993; Goodwin et al., 1996; Nath et al., 2001; Ferris et al., 2008; Norman et al., 2009). HIV-positive cocaine users are cognitively more impaired and poorly adhere to ART than their respective non-drug-abusing counterparts (Meade et al., 2011). Basal ganglia damage that may lead to basal ganglia dysfunction has been reported in HIV-infected cocaine users (Newsome et al., 2011). Cocaine is also known to regulate the rapid activation of the hypothalamic-pituitary-adrenal axis due to enhanced norepinephrine activity (Torres and Rivier, 1992; Sarnyai et al., 1996; Manetti et al., 2014). Neurodegeneration and neuro inflammation are the hallmark features of HAND (Gill and Kolson, 2014; Rao et al., 2014), and are often exacerbated in the presence of drugs of abuse such as cocaine (Gannon et al., 2011; Buch et al., 2012). Brain autopsy studies revealed significant prevalence of microglia activation, BBB disruption and infiltration of multinucleated giant cells in drug-abusing HIV-positive individuals over non-abusing HIV-positive controls (Gannon et al., 2011). Besides its positive effect on HIV-1 replication in macrophages (Dhillon et al., 2007) and microglia (Gekker et al., 2006), cocaine use has also been shown to enhance HIV-1 replication in the normal human astrocytes (NHAs; Reynolds et al., 2006). Cocaine-exposed, HIV-infected NHAs exhibited upregulated p24 antigen levels, indicative of high viral protein content (Reynolds et al., 2006). Though neurons are generally refractory to HIV-1, persistent and low level viral infection in the brain triggers production of neurotoxic factors and release of proinflammatory cytokines and chemokines from HIV-1 infected macrophages/microglia as well as astrocytes, thus causing neuroinflammation and neurotoxicity that culminates in HAND (Gonzalez-Scarano and Martin-Garcia, 2005; Rao et al., 2014). The plasma and post-mortem brain tissue of HIV-1 infected cocaine users, when compared to that of non-drug users, displayed elevated levels of the lysosomal protease cathepsin B, a neuronal pro-apoptotic factor secreted by activated macrophages (Zenon et al., 2014). This response could be recapitulated when HIV-infected MDM were exposed to cocaine, thereby demonstrating that cocaine potentiates cathepsin B secretion and increases neuronal apoptosis (Zenon et al., 2014). Cocaine abuse also increases expression of acute phase proteins such as C-reactive protein (CRP) and serum amyloid A (SAA), which cause chronic inflammatory effects, including in the brain (Samikkannu et al., 2013). Thus, cocaine abuse not only facilitates HIV-1 neuro-invasion via its effect on the BBB but also enhances neuroinflammation and neurotoxicity observed in HIV-1 infected individuals. In summary, the interplay between cocaine and HIV-1 in the CNS mediated via a myriad of pathways, causes deleterious effects leading to worsening of HAND.
Cocaine Alters Integrity of the BBB
Cocaine, besides being able to passively diffuse through the BBB, has also been shown to breach the integrity of the BBB by modulating the structural components of the barrier endothelium (Sharma et al., 2009). Though the biochemical mechanism behind this effect awaits delineation, cocaine’s ability to alter the expression of proteins such as intracellular adhesion molecule 1 (ICAM-1), vascular cell adhesion molecule 1 (VCAM-1), and endothelial-leukocyte adhesion molecule (ELAM or selectin-1) has been postulated as a key contributory factor (Fiala et al., 1998, 2005; Kousik et al., 2012). This has been shown, both in vitro and in rodents, to trigger increased leukocyte migration across endothelial monolayers, accompanied by elevated levels of pro-inflammatory cytokines and chemokines such as tumor necrosis factor alpha (TNF-α), nuclear factor kappa B (NFkB), interleukin 6 (IL-6), and others, ultimately resulting in neuro-inflammation (Fiala et al., 1998; Chang et al., 2000). In addition, cocaine up-regulates the pro-migratory CCL2/CCR2 ligand-receptor system, thereby enabling the HIV-infected monocytes cross the BBB (Dhillon et al., 2008). In vitro, cocaine binds to its cognate receptor σ-1-R in BMVECs and induces the expression of platelet-derived growth factor (PDGF), both at the transcript and protein levels, thereby enhancing endothelial permeability (Yao et al., 2011). Importantly, treatment with PDGF neutralizing antibodies abrogated this effect in mice (Hata et al., 2010). Cocaine exposure also leads to BBB deregulation via the loss or modulation of tight junction proteins. For example, cocaine downregulates the expression of the tight junction protein ZO-1 (Dhillon et al., 2008). Furthermore, cocaine is also known to upregulate matrix metalloproteinase (MMP) expression which triggers reorganization of the basement membrane (Nair et al., 2005). The ensuing damage to the integrity of the BBB has been suggested to facilitate the entry of the virus into the brain. Thus, cocaine-induced alterations in the BBB integrity can be hypothesized to potentiate HAND pathogenesis by enhancing HIV-1 neuroinvasion, an outcome facilitated by increased monocyte entry and neuroinflammation.
Cocaine Exacerbates the Toxicity of HIV-1 Proteins in the Brain
Cocaine, has been reported to amplify the neurotoxic effects of HIV-1 proteins such as Tat and gp120 released from the infected cells, with a magnitude that exceeds that of cocaine or HIV alone. Both in vitro and in vivo studies have shown that mitochondrial dysfunction and oxidative stress leading to neuronal cell damage and death are key factors in exacerbating the effects of cocaine on the pathophysiology of neurodegenerative disorders. Treatment of primary rat hippocampal neurons with Tat protein in the presence of cocaine induced a synergistic effect that enhanced neurotoxicity (Aksenov et al., 2006). Cocaine also enhanced the Tat-induced mitochondrial depolarization and generation of intracellular ROS; Aksenov et al. (2006). Pre-treatment with a specific dopamine receptor antagonist blocked the cocaine-induced potentiation of Tat neurotoxicity, thereby underpinning the role of dopamine receptor-mediated signaling (Aksenov et al., 2006). Likewise, combinatorial toxicity studies have demonstrated that exposure of rat primary neurons to both cocaine and gp120 led to increased oxidative stress that was accompanied by an increase in pro-apoptotic markers (Yao et al., 2009). In another in vivo study, administering rats with a combination of cocaine and ventricular injection of HIV-1 gp120 protein resulted in increased iNOS (nitric oxide synthase) levels accompanied by neuronal apoptosis in the neocortex region (Bagetta et al., 2004). Importantly, this effect was ameliorated in animals pretreated with the iNOS inhibitor, thus substantiating the involvement of iNOS in HIV-1 gp120 and cocaine-mediated neuronal apoptosis (Bagetta et al., 2004). Furthermore, treatment of hippocampal neurons with both HIV-1 gp120 and cocaine resulted in enhanced loss of neuronal dendrites (Bagetta et al., 2004). Together, these studies demonstrate that cocaine augments the effect of HIV-1 Tat and gp120 on neurotoxicity that may contribute to enhanced HIV-associated neuropathogenesis.
Cocaine Elicits Immuno-modulatory Effects on Brain
Cocaine has multiple physiologic and pathological effects on various organ systems including the CNS. In general, CNS comprises of the brain and the spinal cord. Brain is composed of two major cell types (Glial cells and Neurons) contributing to its conserved uniqueness in terms of memory, learning and motor activity. Neurons carry and transmit signals across specialized synaptic junctions, whereas glial cells maintain the structural and metabolic homeostasis of the CNS. There is evidence that cocaine disrupts the homeostasis in the CNS by exerting toxic and immunomodulatory effects. Persistent inflammation in the brain due to cocaine abuse has been shown to involve neuroendocrine interactions. For example, cocaine modulates secretion of pituitary-adrenocortical hormones that are immunomodulatory (Rivier and Vale, 1987; Mendelson et al., 1992). Cocaine can also directly modulate cellular immune responses by either interacting with specific membrane-associated binding sites or by non-specific interaction with the lymphocyte plasma membrane (Van Dyke et al., 1976; Klein et al., 1993; Matsui et al., 1993). Cocaine’s addictive effects are dependent on the blockage of the DAT and intensification of dopamine neurotransmission (Ritz et al., 1987; Kuhar, 1992; Woolverton and Johnson, 1992). Furthermore, dopamine treatment increased entry of HIV-1 virions into macrophages invoking the possibility that CNS dopamine may be a common mechanism by which cocaine may exacerbate neuroinflammation (Gaskill et al., 2014). In addition to the dopamine system, sigma receptors are also involved in cocaine mediated alterations in immune and neuronal function (Maurice et al., 2002). The sigma1 (σ1) receptor, in particular, has been associated with altered immune functionality because it is expressed in many cells in the CNS and PBMCs (Wolfe et al., 1989; Maurice et al., 2002).
Despite advances in understanding the addictive effects of cocaine, the molecular details behind the immunomodulatory effects of cocaine in the brain are unclear. Cocaine has been shown to modulate immune cell activity and secretion of pro-inflammatory cytokines and chemokines, which together lead to hypersensitive and perturbed immune responses (Baldwin et al., 1998; Pellegrino and Bayer, 1998; Clark et al., 2013). Cocaine users reportedly harbor significantly high numbers of activated microglia and macrophages (Bowers and Kalivas, 2003; Little et al., 2009), and recent evidence indicates that cocaine can activate innate immune signaling within the brain. The innate immune system in brain is comprised primarily of microglial cells, and cocaine has been shown to activate the pattern-recognition receptor Toll-like receptor 4 (TLR4) present on the microglial cells, thereby causing the release of proinflammatory cytokine interleukin-1β (IL-1β; Northcutt et al., 2015). These proinflammatory signals contribute to cocaine-induced changes in the mesolimbic dopamine system and cocaine reward (Cearley et al., 2011). In addition, cocaine has been shown to activate astrocytes directly or indirectly through the microglial proinflammatory response (Bowers and Kalivas, 2003; Coller and Hutchinson, 2012). Upon activation, astrocytes also release proinflammatory cytokines and glutamate, thus altering the glutamatergic signaling and neuronal excitability (Kalivas and Hu, 2006). Chronic cocaine use can cause sustained activation of microglia and astrocytes, and the ensuing excessive extracellular glutamate can cause neurotoxicity via glutamatergic dysregulation. In summary, the immune-modulatory effect of cocaine can elicit neurotoxic and addictive effects in the brain that contribute to enhanced HIV-1 induced neuropathogenesis.
Conclusions and Future Prospective
An in-depth understanding of the synergy between HIV-1 and drugs of abuse such as cocaine is essential to address and effectively combat the HIV/AIDS global pandemic. Even as ART has significantly reduced AIDS-related mortalities, averting the neuro-pathological effects of HIV-1 infection in the brain remains a daunting challenge. This is all the more urgent for drug users, as accumulating evidence suggest that drug use accentuates HIV-1 neuropathogenesis. One major contributor for this exacerbation is the low-level persistent viral replication in the brain that drives neuroinflammation. This complex interplay between the virus and the interfering drugs is further aggravated by the latter’s inherent excitotoxic potential in targeting the CNS at cellular and genetic level. Strategies focused on priority areas of action are indeed required to bolster native brain defense mechanisms toward nullifying the effects of drugs of abuse on HIV-1 pathogenesis. However, our limited mechanistic understanding highlights the need of systematic in vivo model systems to elucidate the cellular circuitries involved in cocaine-mediated HIV-1 toxicity. On that note, an in-depth analysis of potential roles of miRNAs in this complex nexus between the virus and cocaine may yield valuable insights. In addition, whether cocaine interacts with the wild type and drug resistant HIV-1 differently that may accentuate disease progression is yet to be determined. Together, identification of pathways and regulatory molecules behind the effect of cocaine on HIV-1 pathogenesis could greatly assist in target selection for therapeutic intervention, in the days to come.
Conflict of Interest Statement
The authors declare that the research was conducted in the absence of any commercial or financial relationships that could be construed as a potential conflict of interest.
Acknowledgments
This work is partly supported by grants DA037779 to JP and DA024558, DA30896, DA033892, DA021471 to CD. We also acknowledge the RCMI Grant G12MD007586, the Vanderbilt CTSA grant UL1RR024975, the Meharry Translational Research Center (MeTRC) CTSA grant (U54 RR026140 from NCRR/NIH, the U54 grant MD007593 from NIMHD/NIH).
Footnotes
- ^ http://www.who.int/mediacentre/factsheets/fs360/en/index.html
- ^ http://nsduhweb.rti.org/respweb/homepage.cfm
References
Addai, A. B., Pandhare, J., Paromov, V., Mantri, C. K., Pratap, S., and Dash, C. (2015). Cocaine modulates HIV-1 integration in primary CD4+ T cells: implications in HIV-1 pathogenesis in drug-abusing patients. J. Leukoc. Biol. 97, 779–790. doi: 10.1189/jlb.4A0714-356R
Aksenov, M. Y., Aksenova, M. V., Nath, A., Ray, P. D., Mactutus, C. F., and Booze, R. M. (2006). Cocaine-mediated enhancement of Tat toxicity in rat hippocampal cell cultures: the role of oxidative stress and D1 dopamine receptor. Neurotoxicology 27, 217–228. doi: 10.1016/j.neuro.2005.10.003
Albright, A. V., Soldan, S. S., and Gonzalez-Scarano, F. (2003). Pathogenesis of human immunodeficiency virus-nduced neurological disease. J. Neurovirol. 9, 222–227. doi: 10.1080/13550280390194073
Anderson, S. M., and Pierce, R. C. (2005). Cocaine-induced alterations in dopamine receptor signaling: implications for reinforcement and reinstatement. Pharmacol. Ther. 106, 389–403. doi: 10.1016/j.pharmthera.2004.12.004
Anthony, J. C., Vlahov, D., Nelson, K. E., Cohn, S., Astemborski, J., and Solomon, L. (1991). New evidence on intravenous cocaine use and the risk of infection with human immunodeficiency virus type 1. Am. J. Epidemiol. 134, 1175–1189.
Arnsten, J. H., Demas, P. A., Farzadegan, H., Grant, R. W., Gourevitch, M. N., Chang, C. J., et al. (2001). Antiretroviral therapy adherence and viral suppression in HIV-infected drug users: comparison of self-report and electronic monitoring. Clin. Infect. Dis. 33, 1417–1423. doi: 10.1086/323201
Arnsten, J. H., Demas, P. A., Grant, R. W., Gourevitch, M. N., Farzadegan, H., Howard, A. A., et al. (2002). Impact of active drug use on antiretroviral therapy adherence and viral suppression in HIV-infected drug users. J. Gen. Intern. Med. 17, 377–381. doi: 10.1007/s11606-002-0044-3
Atluri, V. S., Hidalgo, M., Samikkannu, T., Kurapati, K. R., Jayant, R. D., Sagar, V., et al. (2015). Effect of human immunodeficiency virus on blood–brain barrier integrity and function: an update. Front. Cell Neurosci. 9:212. doi: 10.3389/fncel.2015.00212
Aylward, E. H., Henderer, J. D., McArthur, J. C., Brettschneider, P. D., Harris, G. J., Barta, P. E., et al. (1993). Reduced basal ganglia volume in HIV-1-associated dementia: results from quantitative neuroimaging. Neurology 43, 2099–2104. doi: 10.1212/WNL.43.10.2099
Bagasra, O., and Pomerantz, R. J. (1993). Human immunodeficiency virus type 1 replication in peripheral blood mononuclear cells in the presence of cocaine. J. Infect. Dis. 168, 1157–1164. doi: 10.1093/infdis/168.5.1157
Bagetta, G., Piccirilli, S., Del Duca, C., Morrone, L. A., Rombola, L., Nappi, G., et al. (2004). Inducible nitric oxide synthase is involved in the mechanisms of cocaine enhanced neuronal apoptosis induced by HIV-1 gp120 in the neocortex of rat. Neurosci. Lett. 356, 183–186. doi: 10.1016/j.neulet.2003.11.065
Baldwin, G. C., Roth, M. D., and Tashkin, D. P. (1998). Acute and chronic effects of cocaine on the immune system and the possible link to AIDS. J. Neuroimmunol. 83, 133–138. doi: 10.1016/S0165-5728(97)00229-4
Ballabh, P., Braun, A., and Nedergaard, M. (2004). The blood–brain barrier: an overview: structure, regulation, and clinical implications. Neurobiol. Dis. 16, 1–13. doi: 10.1016/j.nbd.2003.12.016
Banks, W. A. (2005). Blood–brain barrier transport of cytokines: a mechanism for neuropathology. Curr. Pharm. Des. 11, 973–984. doi: 10.2174/1381612053381684
Battjes, R. J., Leukefeld, C. G., Pickens, R. W., and Haverkos, H. W. (1988). The acquired immunodeficiency syndrome and intravenous drug abuse. Bull. Narc. 40, 21–34.
Baum, M. K., Rafie, C., Lai, S., Sales, S., Page, B., and Campa, A. (2009). Crack-cocaine use accelerates HIV disease progression in a cohort of HIV-positive drug users. J. Acquir. Immune Defic. Syndr. 50, 93–99. doi: 10.1097/QAI.0b013e3181900129
Berger, J. R., and Nath, A. (1997). HIV dementia and the basal ganglia. Intervirology 40, 122–131. doi: 10.1159/000150539
Bowers, M. S., and Kalivas, P. W. (2003). Forebrain astroglial plasticity is induced following withdrawal from repeated cocaine administration. Eur. J. Neurosci. 17, 1273–1278. doi: 10.1046/j.1460-9568.2003.02537.x
Brew, B. J., and Letendre, S. L. (2008). Biomarkers of HIV related central nervous system disease. Int. Rev. Psychiatry 20, 73–88. doi: 10.1080/09540260701878082
Buch, S., Yao, H., Guo, M., Mori, T., Mathias-Costa, B., Singh, V., et al. (2012). Cocaine and HIV-1 interplay in CNS: cellular and molecular mechanisms. Curr. HIV Res. 10, 425–428. doi: 10.2174/157016212802138823
Cabral, G. A. (2006). Drugs of abuse, immune modulation, and AIDS. J. Neuroimmune Pharmacol. 1, 280–295. doi: 10.1007/s11481-006-9023-5
Carrico, A. W., Johnson, M. O., Morin, S. F., Remien, R. H., Riley, E. D., Hecht, F. M., et al. (2008). Stimulant use is associated with immune activation and depleted tryptophan among HIV-positive persons on anti-retroviral therapy. Brain Behav. Immun. 22, 1257–1262. doi: 10.1016/j.bbi.2008.07.010
Centers for Disease Control and Prevention. (2012). HIV Surveillance Report, Vol. 24. Available at: http://www.cdc.gov/hiv/library/reports/surveillance/ (accessed November 22, 2014).
Cearley, C. N., Blindheim, K., Sorg, B. A., Krueger, J. M., and Churchill, L. (2011). Acute cocaine increases interleukin-1β mRNA and immunoreactive cells in the cortex and nucleus accumbens. Neurochem. Res. 36, 686–692. doi: 10.1007/s11064-011-0410-9
Chaisson, R. E., Bacchetti, P., Osmond, D., Brodie, B., Sande, M. A., and Moss, A. R. (1989). Cocaine use and HIV infection in intravenous drug users in San Francisco. JAMA 261, 561–565. doi: 10.1001/jama.1989.03420040099027
Chang, S. L., Bersig, J., Felix, B., Fiala, M., and House, S. D. (2000). Chronic cocaine alters hemodynamics and leukocyte-endothelial interactions in rat mesenteric venules. Life Sci. 66, 2357–2369. doi: 10.1016/S0024-3205(00)00566-X
Chao, C., Jacobson, L. P., Tashkin, D., Martinez-Maza, O., Roth, M. D., Margolick, J. B., et al. (2008). Recreational drug use and T lymphocyte subpopulations in HIV-uninfected and HIV-infected men. Drug Alcohol. Depend. 94, 165–171. doi: 10.1016/j.drugalcdep.2007.11.010
Clark, K. H., Wiley, C. A., and Bradberry, C. W. (2013). Psychostimulant abuse and neuroinflammation: emerging evidence of their interconnection. Neurotox. Res. 23, 174–188. doi: 10.1007/s12640-012-9334-7
Clifford, D. B. (2000). Human immunodeficiency virus-associated dementia. Arch. Neurol. 57, 321–324. doi: 10.1001/archneur.57.3.321
Cofrancesco, J. Jr., Scherzer, R., Tien, P. C., Gibert, C. L., Southwell, H., Sidney, S., et al. (2008). Illicit drug use and HIV treatment outcomes in a US cohort. AIDS 22, 357–365. doi: 10.1097/QAD.0b013e3282f3cc21
Cohen, M. S., Chen, Y. Q., McCauley, M., Gamble, T., Hosseinipour, M. C., Kumarasamy, N., et al. (2011). Prevention of HIV-1 infection with early antiretroviral therapy. N. Engl. J. Med. 365, 493–505. doi: 10.1056/NEJMoa1105243
Coller, J. K., and Hutchinson, M. R. (2012). Implications of central immune signaling caused by drugs of abuse: mechanisms, mediators and new therapeutic approaches for prediction and treatment of drug dependence. Pharmacol. Ther. 134, 219–245. doi: 10.1016/j.pharmthera.2012.01.008
Colombo, L. L., Lopez, M. C., Chen, G. J., and Watson, R. R. (1999). Effect of short-term cocaine administration on the immune system of young and old C57BL/6 female mice. Immunopharmacol. Immunotoxicol. 21, 755–769. doi: 10.3109/08923979909007140
Cook, J. A. (2011). Associations between use of crack cocaine and HIV-1 disease progression: research findings and implications for mother-to-infant transmission. Life Sci. 88, 931–939. doi: 10.1016/j.lfs.2011.01.003
Cook, J. A., Burke-Miller, J. K., Cohen, M. H., Cook, R. L., Vlahov, D., Wilson, T. E., et al. (2008). Crack cocaine, disease progression, and mortality in a multicenter cohort of HIV-1 positive women. AIDS 22, 1355–1363. doi: 10.1097/QAD.0b013e32830507f2
Cooper, A., Garcia, M., Petrovas, C., Yamamoto, T., Koup, R. A., and Nabel, G. J. (2013). HIV-1 causes CD4 cell death through DNA-dependent protein kinase during viral integration. Nature 498, 376–379. doi: 10.1038/nature12274
Dhillon, N. K., Peng, F., Bokhari, S., Callen, S., Shin, S. H., Zhu, X., et al. (2008). Cocaine-mediated alteration in tight junction protein expression and modulation of CCL2/CCR2 axis across the blood–brain barrier: implications for HIV-dementia. J. Neuroimmune Pharmacol. 3, 52–56. doi: 10.1007/s11481-007-9091-1
Dhillon, N. K., Williams, R., Peng, F., Tsai, Y. J., Dhillon, S., Nicolay, B., et al. (2007). Cocaine-mediated enhancement of virus replication in macrophages: implications for human immunodeficiency virus-associated dementia. J. Neurovirol. 13, 483–495. doi: 10.1080/13550280701528684
Donahoe, R. M. (1990). Drug abuse and AIDS: causes for the connection. NIDA Res. Monogr. 96, 181–191.
Donahoe, R. M., and Falek, A. (1988). Neuroimmunomodulation by opiates and other drugs of abuse: relationship to HIV infection and AIDS. Adv. Biochem. Psychopharmacol. 44, 145–158.
Embretson, J., Zupancic, M., Ribas, J. L., Burke, A., Racz, P., Tenner-Racz, K., et al. (1993). Massive covert infection of helper T lymphocytes and macrophages by HIV during the incubation period of AIDS. Nature 362, 359–362. doi: 10.1038/362359a0
Evans, S. M., Cone, E. J., and Henningfield, J. E. (1996). Arterial and venous cocaine plasma concentrations in humans: relationship to route of administration, cardiovascular effects and subjective effects. J. Pharmacol. Exp. Ther. 279, 1345–1356.
Ferris, M. J., Mactutus, C. F., and Booze, R. M. (2008). Neurotoxic profiles of HIV, psychostimulant drugs of abuse, and their concerted effect on the brain: current status of dopamine system vulnerability in NeuroAIDS. Neurosci. Biobehav. Rev. 32, 883–909. doi: 10.1016/j.neubiorev.2008.01.004
Fiala, M., Eshleman, A. J., Cashman, J., Lin, J., Lossinsky, A. S., Suarez, V., et al. (2005). Cocaine increases human immunodeficiency virus type 1 neuroinvasion through remodeling brain microvascular endothelial cells. J. Neurovirol. 11, 281–291. doi: 10.1080/13550280590952835
Fiala, M., Gan, X. H., Zhang, L., House, S. D., Newton, T., Graves, M. C., et al. (1998). Cocaine enhances monocyte migration across the blood–brain barrier. Cocaine’s connection to AIDS dementia and vasculitis? Adv. Exp. Med. Biol. 437, 199–205. doi: 10.1007/978-1-4615-5347-2_22
Fischer-Smith, T., Croul, S., Adeniyi, A., Rybicka, K., Morgello, S., Khalili, K., et al. (2004). Macrophage/microglial accumulation and proliferating cell nuclear antigen expression in the central nervous system in human immunodeficiency virus encephalopathy. Am. J. Pathol. 164, 2089–2099. doi: 10.1016/S0002-9440(10)63767-4
Friedman, H., Newton, C., and Klein, T. W. (2003). Microbial infections, immunomodulation, and drugs of abuse. Clin. Microbiol. Rev. 16, 209–219. doi: 10.1128/CMR.16.2.209-219.2003
Friedman, H., Pross, S., and Klein, T. W. (2006). Addictive drugs and their relationship with infectious diseases. FEMS Immunol. Med. Microbiol. 47, 330–342. doi: 10.1111/j.1574-695X.2006.00097.x
Gannon, P., Khan, M. Z., and Kolson, D. L. (2011). Current understanding of HIV-associated neurocognitive disorders pathogenesis. Curr. Opin. Neurol. 24, 275–283. doi: 10.1097/WCO.0b013e32834695fb
Gaskill, P. J., Yano, H. H., Kalpana, G. V., Javitch, J. A., and Berman, J. W. (2014). Dopamine receptor activation increases HIV entry into primary human macrophages. PLoS ONE 9:e108232. doi: 10.1371/journal.pone.0108232
Gekker, G., Hu, S., Sheng, W. S., Rock, R. B., Lokensgard, J. R., and Peterson, P. K. (2006). Cocaine-induced HIV-1 expression in microglia involves sigma-1 receptors and transforming growth factor-β1. Int. Immunopharmacol. 6, 1029–1033. doi: 10.1016/j.intimp.2005.12.005
Gelman, B. B. (2015). Neuropathology of HAND with suppressive antiretroviral therapy: encephalitis and neurodegeneration reconsidered. Curr. HIV/AIDS Rep. 12, 272–279. doi: 10.1007/s11904-015-0266-8
Gill, A. J., and Kolson, D. L. (2014). Chronic inflammation and the role for cofactors (hepatitis C, drug abuse, antiretroviral drug toxicity, aging) in HAND persistence. Curr. HIV/AIDS Rep. 11, 325–335. doi: 10.1007/s11904-014-0210-3
Gonzalez-Scarano, F., and Martin-Garcia, J. (2005). The neuropathogenesis of AIDS. Nat. Rev. Immunol. 5, 69–81. doi: 10.1038/nri1527
Goodwin, G. M., Pretsell, D. O., Chiswick, A., Egan, V., and Brettle, R. P. (1996). The Edinburgh cohort of HIV-positive injecting drug users at 10 years after infection: a case-control study of the evolution of dementia. AIDS 10, 431–440. doi: 10.1097/00002030-199604000-00012
Graham, D. L., Hoppenot, R., Hendryx, A., and Self, D. W. (2007). Differential ability of D1 and D2 dopamine receptor agonists to induce and modulate expression and reinstatement of cocaine place preference in rats. Psychopharmacology (Berl.) 191, 719–730. doi: 10.1007/s00213-006-0473-5
Grant, R. M., Lama, J. R., Anderson, P. L., McMahan, V., Liu, A. Y., Vargas, L., et al. (2010). Preexposure chemoprophylaxis for HIV prevention in men who have sex with men. N. Engl. J. Med. 363, 2587–2599. doi: 10.1056/NEJMoa1011205
Gray, F., Chretien, F., Vallat-Decouvelaere, A. V., and Scaravilli, F. (2003). The changing pattern of HIV neuropathology in the HAART era. J. Neuropathol. Exp. Neurol. 62, 429–440.
Hata, N., Shinojima, N., Gumin, J., Yong, R., Marini, F., Andreeff, M., et al. (2010). Platelet-derived growth factor BB mediates the tropism of human mesenchymal stem cells for malignant gliomas. Neurosurgery 66, 144–156; discussion 156–147. doi: 10.1227/01.NEU.0000363149.58885.2E
Hazleton, J. E., Berman, J. W., and Eugenin, E. A. (2010). Novel mechanisms of central nervous system damage in HIV infection. HIV AIDS (Auckl) 2, 39–49.
Heinkelein, M., Sopper, S., and Jassoy, C. (1995). Contact of human immunodeficiency virus type 1-infected and uninfected CD4+ T lymphocytes is highly cytolytic for both cells. J. Virol. 69, 6925–6931.
Hinkin, C. H., Barclay, T. R., Castellon, S. A., Levine, A. J., Durvasula, R. S., Marion, S. D., et al. (2007). Drug use and medication adherence among HIV-1 infected individuals. AIDS Behav. 11, 185–194. doi: 10.1007/s10461-006-9152-0
Howell, L. L., and Cunningham, K. A. (2015). Serotonin 5-HT2 receptor interactions with dopamine function: implications for therapeutics in cocaine use disorder. Pharmacol. Rev. 67, 176–197. doi: 10.1124/pr.114.009514
Huang, J., Wang, F., Argyris, E., Chen, K., Liang, Z., Tian, H., et al. (2007). Cellular microRNAs contribute to HIV-1 latency in resting primary CD4+ T lymphocytes. Nat. Med. 13, 1241–1247. doi: 10.1038/nm1639
Ivey, N. S., MacLean, A. G., and Lackner, A. A. (2009). Acquired immunodeficiency syndrome and the blood–brain barrier. J. Neurovirol. 15, 111–122. doi: 10.1080/13550280902769764
Joska, J. A., Gouse, H., Paul, R. H., Stein, D. J., and Flisher, A. J. (2010). Does highly active antiretroviral therapy improve neurocognitive function? A systematic review. J. Neurovirol. 16, 101–114. doi: 10.3109/13550281003682513
Ju, S. M., Song, H. Y., Lee, J. A., Lee, S. J., Choi, S. Y., and Park, J. (2009). Extracellular HIV-1 Tat up-regulates expression of matrix metalloproteinase-9 via a MAPK-NF-kappaB dependent pathway in human astrocytes. Exp. Mol. Med. 41, 86–93. doi: 10.3858/emm.2009.41.2.011
Junghans, C., Low, N., Chan, P., Witschi, A., Vernazza, P., and Egger, M. (1999). Uniform risk of clinical progression despite differences in utilization of highly active antiretroviral therapy: swiss HIV Cohort Study. AIDS 13, 2547–2554. doi: 10.1097/00002030-199912240-00008
Kalivas, P. W., and Hu, X. T. (2006). Exciting inhibition in psychostimulant addiction. Trends Neurosci. 29, 610–616. doi: 10.1016/j.tins.2006.08.008
Kalivas, P. W., and Volkow, N. D. (2005). The neural basis of addiction: a pathology of motivation and choice. Am. J. Psychiatry 162, 1403–1413. doi: 10.1176/appi.ajp.162.8.1403
Kanmogne, G. D., Primeaux, C., and Grammas, P. (2005). HIV-1 gp120 proteins alter tight junction protein expression and brain endothelial cell permeability: implications for the pathogenesis of HIV-associated dementia. J. Neuropathol. Exp. Neurol. 64, 498–505.
Kanmogne, G. D., Schall, K., Leibhart, J., Knipe, B., Gendelman, H. E., and Persidsky, Y. (2007). HIV-1 gp120 compromises blood–brain barrier integrity and enhances monocyte migration across blood–brain barrier: implication for viral neuropathogenesis. J. Cereb. Blood Flow Metab. 27, 123–134. doi: 10.1038/sj.jcbfm.9600330
Kaul, M., Garden, G. A., and Lipton, S. A. (2001). Pathways to neuronal injury and apoptosis in HIV-associated dementia. Nature 410, 988–994. doi: 10.1038/35073667
Khalsa, J. H., and Royal, W. (2004). Do drugs of abuse impact on HIV disease? J. Neuroimmunol. 147, 6–8. doi: 10.1016/j.jneuroim.2003.10.007
Kim, S. G., Jung, J. B., Dixit, D., Rovner, R. Jr., Zack, J. A., Baldwin, G. C., et al. (2013). Cocaine exposure enhances permissiveness of quiescent T cells to HIV infection. J. Leukoc. Biol. 94, 835–843. doi: 10.1189/jlb.1112566
Kim, S. G., Lowe, E. L., Dixit, D., Seyeon Youn, C., Kim, I. J., Jung, J. B., et al. (2015). Cocaine-mediated impact on HIV infection in humanized BLT mice. Sci. Rep. 5, 10010. doi: 10.1038/srep10010
Kipp, A. M., Desruisseau, A. J., and Qian, H. Z. (2011). Non-injection drug use and HIV disease progression in the era of combination antiretroviral therapy. J. Subst. Abuse Treat. 40, 386–396. doi: 10.1016/j.jsat.2011.01.001
Klein, T. W., Matsui, K., Newton, C. A., Young, J., Widen, R. E., and Friedman, H. (1993). Cocaine suppresses proliferation of phytohemagglutinin-activated human peripheral blood T-cells. Int. J. Immunopharmacol. 15, 77–86. doi: 10.1016/0192-0561(93)90033-U
Koppensteiner, H., Brack-Werner, R., and Schindler, M. (2012). Macrophages and their relevance in Human Immunodeficiency Virus Type I infection. Retrovirology 9, 82. doi: 10.1186/1742-4690-9-82
Kousik, S. M., Napier, T. C., and Carvey, P. M. (2012). The effects of psychostimulant drugs on blood–brain barrier function and neuroinflammation. Front. Pharmacol. 3, 121. doi: 10.3389/fphar.2012.00121
Kuhar, M. J. (1992). Molecular pharmacology of cocaine: a dopamine hypothesis and its implications. Ciba Found Symp. 166, 81–89; discussion 89–95.
Lane, H. C. (2010). Pathogenesis of HIV infection: total CD4+ T-cell pool, immune activation, and inflammation. Top HIV Med. 18, 2–6.
Larrat, E. P., and Zierler, S. (1993). Entangled epidemics: cocaine use and HIV disease. J. Psychoactive Drugs 25, 207–221. doi: 10.1080/02791072.1993.10472272
Larrat, E. P., Zierler, S., and Mayer, K. (1996). Cocaine use and HIV disease progression among heterosexuals. Pharmacoepidemiol. Drug Saf. 5, 229–236.
Le, M. T., Shyh-Chang, N., Khaw, S. L., Chin, L., Teh, C., Tay, J., et al. (2011). Conserved regulation of p53 network dosage by microRNA-125b occurs through evolving miRNA-target gene pairs. PLoS Genet. 7:e1002242. doi: 10.1371/journal.pgen.1002242
Le, M. T., Teh, C., Shyh-Chang, N., Xie, H., Zhou, B., Korzh, V., et al. (2009). MicroRNA-125b is a novel negative regulator of p53. Genes Dev. 23, 862–876. doi: 10.1101/gad.1767609
Little, K. Y., Ramssen, E., Welchko, R., Volberg, V., Roland, C. J., and Cassin, B. (2009). Decreased brain dopamine cell numbers in human cocaine users. Psychiatry Res. 168, 173–180. doi: 10.1016/j.psychres.2008.10.034
Lucas, G. M., Cheever, L. W., Chaisson, R. E., and Moore, R. D. (2001). Detrimental effects of continued illicit drug use on the treatment of HIV-1 infection. J. Acquir. Immune Defic. Syndr. 27, 251–259. doi: 10.1097/00126334-200107010-00006
Lucas, G. M., Gebo, K. A., Chaisson, R. E., and Moore, R. D. (2002). Longitudinal assessment of the effects of drug and alcohol abuse on HIV-1 treatment outcomes in an urban clinic. AIDS 16, 767–774. doi: 10.1097/00002030-200203290-00012
Lucas, G. M., Griswold, M., Gebo, K. A., Keruly, J., Chaisson, R. E., and Moore, R. D. (2006). Illicit drug use and HIV-1 disease progression: a longitudinal study in the era of highly active antiretroviral therapy. Am. J. Epidemiol. 163, 412–420. doi: 10.1093/aje/kwj059
Lv, S., Song, H. L., Zhou, Y., Li, L. X., Cui, W., Wang, W., et al. (2010). Tumour necrosis factor-alpha affects blood–brain barrier permeability and tight junction-associated occludin in acute liver failure. Liver Int. 30, 1198–1210. doi: 10.1111/j.1478-3231.2010.02211.x
Manetti, L., Cavagnini, F., Martino, E., and Ambrogio, A. (2014). Effects of cocaine on the hypothalamic-pituitary-adrenal axis. J. Endocrinol. Invest. 37, 701–708. doi: 10.1007/s40618-014-0091-8
Mantri, C. K., Pandhare Dash, J., Mantri, J. V., and Dash, C. C. (2012). Cocaine enhances HIV-1 replication in CD4+ T cells by down-regulating MiR-125b. PLoS ONE 7:e51387. doi: 10.1371/journal.pone.0051387
Marasco, C. C., Goodwin, C. R., Winder, D. G., Schramm-Sapyta, N. L., McLean, J. A., and Wikswo, J. P. (2014). Systems-level view of cocaine addiction: the interconnection of the immune and nervous systems. Exp. Biol. Med. (Maywood) 239, 1433–1442. doi: 10.1177/1535370214537747
Marrazzo, J. M., Ramjee, G., Richardson, B. A., Gomez, K., Mgodi, N., Nair, G., et al. (2015). Tenofovir-based preexposure prophylaxis for HIV infection among African women. N. Engl. J. Med. 372, 509–518. doi: 10.1056/NEJMoa1402269
Matsui, K., Friedman, H., and Klein, T. W. (1993). Molecular mechanisms associated with cocaine-induced modulation of human T lymphocytes proliferation. Adv. Exp. Med. Biol. 335, 127–134. doi: 10.1007/978-1-4615-2980-4_18
Maurice, T., Martin-Fardon, R., Romieu, P., and Matsumoto, R. R. (2002). Sigma(1) (sigma(1)) receptor antagonists represent a new strategy against cocaine addiction and toxicity. Neurosci. Biobehav. Rev. 26, 499–527. doi: 10.1016/S0149-7634(02)00017-9
McArthur, J. C., Steiner, J., Sacktor, N., and Nath, A. (2010). Human immunodeficiency virus-associated neurocognitive disorders: mind the gap. Ann. Neurol. 67, 699–714. doi: 10.1002/ana.22053
Meade, C. S., Conn, N. A., Skalski, L. M., and Safren, S. A. (2011). Neurocognitive impairment and medication adherence in HIV patients with and without cocaine dependence. J. Behav. Med. 34, 128–138. doi: 10.1007/s10865-010-9293-5
Mendelson, J. H., Teoh, S. K., Mello, N. K., Ellingboe, J., and Rhoades, E. (1992). Acute effects of cocaine on plasma adrenocorticotropic hormone, luteinizing hormone and prolactin levels in cocaine-dependent men. J. Pharmacol. Exp. Ther. 263, 505–509.
Nair, M. P., Chadha, K. C., Hewitt, R. G., Mahajan, S., Sweet, A., and Schwartz, S. A. (2000). Cocaine differentially modulates chemokine production by mononuclear cells from normal donors and human immunodeficiency virus type 1-infected patients. Clin. Diagn. Lab. Immunol. 7, 96–100. doi: 10.1128/cdli.7.1.96-100.2000
Nair, M. P., Mahajan, S., Chadha, K. C., Nair, N. M., Hewitt, R. G., Pillai, S. K., et al. (2001). Effect of cocaine on chemokine and CCR-5 gene expression by mononuclear cells from normal donors and HIV-1 infected patients. Adv. Exp. Med. Biol. 493, 235–240. doi: 10.1007/0-306-47611-8_28
Nair, M. P., Mahajan, S. D., Schwartz, S. A., Reynolds, J., Whitney, R., Bernstein, Z., et al. (2005). Cocaine modulates dendritic cell-specific C type intercellular adhesion molecule-3-grabbing nonintegrin expression by dendritic cells in HIV-1 patients. J. Immunol. 174, 6617–6626. doi: 10.4049/jimmunol.174.11.6617
Nakamuta, S., Endo, H., Higashi, Y., Kousaka, A., Yamada, H., Yano, M., et al. (2008). Human immunodeficiency virus type 1 gp120-mediated disruption of tight junction proteins by induction of proteasome-mediated degradation of zonula occludens-1 and -2 in human brain microvascular endothelial cells. J. Neurovirol. 14, 186–195. doi: 10.1080/13550280801993630
Napuri, J., Pilakka-Kanthikeel, S., Raymond, A., Agudelo, M., Yndart-Arias, A., Saxena, S. K., et al. (2013). Cocaine enhances HIV-1 infectivity in monocyte derived dendritic cells by suppressing microRNA-155. PLoS ONE 8:e83682. doi: 10.1371/journal.pone.0083682
Nath, A., Maragos, W. F., Avison, M. J., Schmitt, F. A., and Berger, J. R. (2001). Acceleration of HIV dementia with methamphetamine and cocaine. J. Neurovirol. 7, 66–71. doi: 10.1080/135502801300069737
Newsome, S. D., Johnson, E., Pardo, C., McArthur, J. C., and Nath, A. (2011). Fulminant encephalopathy with basal ganglia hyperintensities in HIV-infected drug users. Neurology 76, 787–794. doi: 10.1212/WNL.0b013e31820e7b4e
Nnadi, C. U., Mimiko, O. A., McCurtis, H. L., and Cadet, J. L. (2005). Neuropsychiatric effects of cocaine use disorders. J. Natl. Med. Assoc. 97, 1504–1515.
Norman, L. R., Basso, M., Kumar, A., and Malow, R. (2009). Neuropsychological consequences of HIV and substance abuse: a literature review and implications for treatment and future research. Curr. Drug Abuse Rev. 2, 143–156. doi: 10.2174/1874473710902020143
Northcutt, A. L., Hutchinson, M. R., Wang, X., Baratta, M. V., Hiranita, T., Cochran, T. A., et al. (2015). DAT isn’t all that: cocaine reward and reinforcement require Toll-like receptor 4 signaling. Mol. Psychiatry doi: 10.1038/mp.2014.177 [Epub ahead of print].
Pakesch, G., Loimer, N., Grunberger, J., Pfersmann, D., Linzmayer, L., and Mayerhofer, S. (1992). Neuropsychological findings and psychiatric symptoms in HIV-1 infected and noninfected drug users. Psychiatry Res. 41, 163–177. doi: 10.1016/0165-1781(92)90108-F
Palepu, A., Tyndall, M., Yip, B., O’Shaughnessy, M. V., Hogg, R. S., and Montaner, J. S. (2003). Impaired virologic response to highly active antiretroviral therapy associated with ongoing injection drug use. J. Acquir. Immune Defic. Syndr. 32, 522–526. doi: 10.1097/00126334-200304150-00009
Pandhare, J., Addai, A. B., Mantri, C. K., Hager, C., Smith, R. M., Barnett, L., et al. (2014). Cocaine enhances HIV-1-Induced CD4 T-cell apoptosis: implications in disease progression in cocaine-abusing HIV-1 patients. Am J Pathol. 184, 927–936. doi: 10.1016/j.ajpath.2013.12.004
Pandhare, J., and Dash, C. (2011). A prospective on drug abuse-associated epigenetics and HIV-1 replication. Life Sci. 88, 995–999. doi: 10.1016/j.lfs.2010.10.005
Patel, S. M., Thames, A. D., Arbid, N., Panos, S. E., Castellon, S., and Hinkin, C. H. (2013). The aggregate effects of multiple comorbid risk factors on cognition among HIV-infected individuals. J. Clin. Exp. Neuropsychol. 35, 421–434. doi: 10.1080/13803395.2013.783000
Pellegrino, T., and Bayer, B. M. (1998). In vivo effects of cocaine on immune cell function. J. Neuroimmunol. 83, 139–147. doi: 10.1016/S0165-5728(97)00230-0
Pence, B. W., Thielman, N. M., Whetten, K., Ostermann, J., Kumar, V., and Mugavero, M. J. (2008). Coping strategies and patterns of alcohol and drug use among HIV-infected patients in the United States Southeast. AIDS Patient Care STDS. 22, 869–877. doi: 10.1089/apc.2008.0022
Persidsky, Y. (1999). Model systems for studies of leukocyte migration across the blood–brain barrier. J. Neurovirol. 5, 579–590. doi: 10.3109/13550289909021287
Persidsky, Y., Ghorpade, A., Rasmussen, J., Limoges, J., Liu, X. J., Stins, M., et al. (1999). Microglial and astrocyte chemokines regulate monocyte migration through the blood–brain barrier in human immunodeficiency virus-1 encephalitis. Am. J. Pathol. 155, 1599–1611. doi: 10.1016/S0002-9440(10)65476-4
Peterson, P. K., Gekker, G., Chao, C. C., Schut, R., Molitor, T. W., and Balfour, H. H. Jr. (1991). Cocaine potentiates HIV-1 replication in human peripheral blood mononuclear cell cocultures. Involvement of transforming growth factor-beta. J. Immunol. 146, 81–84.
Peterson, P. K., Gekker, G., Chao, C. C., Schut, R., Verhoef, J., Edelman, C. K., et al. (1992). Cocaine amplifies HIV-1 replication in cytomegalovirus-stimulated peripheral blood mononuclear cell cocultures. J. Immunol. 149, 676–680.
Pezzotti, P., Galai, N., Vlahov, D., Rezza, G., Lyles, C. M., and Astemborski, J. (1999). Direct comparison of time to AIDS and infectious disease death between HIV seroconverter injection drug users in Italy and the United States: results from the ALIVE and ISS studies. AIDS Link to Intravenous Experiences. Italian Seroconversion Study. J. Acquir. Immune Defic. Syndr. Hum. Retrovirol. 20, 275–282. doi: 10.1097/00042560-199903010-00010
Pietraforte, D., Tritarelli, E., Testa, U., and Minetti, M. (1994). gp120 HIV envelope glycoprotein increases the production of nitric oxide in human monocyte-derived macrophages. J. Leukoc. Biol. 55, 175–182.
Pu, H., Hayashi, K., Andras, I. E., Eum, S. Y., Hennig, B., and Toborek, M. (2007). Limited role of COX-2 in HIV Tat-induced alterations of tight junction protein expression and disruption of the blood–brain barrier. Brain Res. 1184, 333–344. doi: 10.1016/j.brainres.2007.09.063
Ramamoorthy, J. D., Ramamoorthy, S., Mahesh, V. B., Leibach, F. H., and Ganapathy, V. (1995). Cocaine-sensitive sigma-receptor and its interaction with steroid hormones in the human placental syncytiotrophoblast and in choriocarcinoma cells. Endocrinology 136, 924–932.
Rao, V. R., Ruiz, A. P., and Prasad, V. R. (2014). Viral and cellular factors underlying neuropathogenesis in HIV associated neurocognitive disorders (HAND). AIDS Res Ther. 11, 13. doi: 10.1186/1742-6405-11-13
Rasbach, D. A., Desruisseau, A. J., Kipp, A. M., Stinnette, S., Kheshti, A., Shepherd, B. E., et al. (2013). Active cocaine use is associated with lack of HIV-1 virologic suppression independent of nonadherence to antiretroviral therapy: use of a rapid screening tool during routine clinic visits. AIDS Care 25, 109–117. doi: 10.1080/09540121.2012.687814
Reynolds, J. L., Mahajan, S. D., Aalinkeel, R., Nair, B., Sykes, D. E., Agosto-Mujica, A., et al. (2009). Modulation of the proteome of peripheral blood mononuclear cells from HIV-1-infected patients by drugs of abuse. J. Clin. Immunol. 29, 646–656. doi: 10.1007/s10875-009-9309-5
Reynolds, J. L., Mahajan, S. D., Bindukumar, B., Sykes, D., Schwartz, S. A., and Nair, M. P. (2006). Proteomic analysis of the effects of cocaine on the enhancement of HIV-1 replication in normal human astrocytes (NHA). Brain Res. 1123, 226–236. doi: 10.1016/j.brainres.2006.09.034
Riezzo, I., Fiore, C., De Carlo, D., Pascale, N., Neri, M., Turillazzi, E., et al. (2012). Side effects of cocaine abuse: multiorgan toxicity and pathological consequences. Curr. Med. Chem. 19, 5624–5646. doi: 10.2174/092986712803988893
Ritz, M. C., Lamb, R. J., Goldberg, S. R., and Kuhar, M. J. (1987). Cocaine receptors on dopamine transporters are related to self-administration of cocaine. Science 237, 1219–1223. doi: 10.1126/science.2820058
Rivier, C., and Vale, W. (1987). Cocaine stimulates adrenocorticotropin (ACTH) secretion through a corticotropin-releasing factor (CRF)-mediated mechanism. Brain Res. 422, 403–406. doi: 10.1016/0006-8993(87)90953-X
Ronaldson, P. T., and Bendayan, R. (2006). HIV-1 viral envelope glycoprotein gp120 triggers an inflammatory response in cultured rat astrocytes and regulates the functional expression of P-glycoprotein. Mol. Pharmacol. 70, 1087–1098. doi: 10.1124/mol.106.025973
Roth, M. D., Tashkin, D. P., Choi, R., Jamieson, B. D., Zack, J. A., and Baldwin, G. C. (2002). Cocaine enhances human immunodeficiency virus replication in a model of severe combined immunodeficient mice implanted with human peripheral blood leukocytes. J. Infect. Dis. 185, 701–705. doi: 10.1086/339012
Roth, M. D., Whittaker, K. M., Choi, R., Tashkin, D. P., and Baldwin, G. C. (2005). Cocaine and sigma-1 receptors modulate HIV infection, chemokine receptors, and the HPA axis in the huPBL-SCID model. J. Leukoc. Biol. 78, 1198–1203. doi: 10.1189/jlb.0405219
Ruiz, P., Cleary, T., Nassiri, M., and Steele, B. (1994). Human T lymphocyte subpopulation and NK cell alterations in persons exposed to cocaine. Clin. Immunol. Immunopathol. 70, 245–250. doi: 10.1006/clin.1994.1036
Samikkannu, T., Rao, K. V., Arias, A. Y., Kalaichezian, A., Sagar, V., Yoo, C., et al. (2013). HIV infection and drugs of abuse: role of acute phase proteins. J. Neuroinflamm. 10, 113. doi: 10.1186/1742-2094-10-113
Sanmarti, M., Ibanez, L., Huertas, S., Badenes, D., Dalmau, D., Slevin, M., et al. (2014). HIV-associated neurocognitive disorders. J. Mol. Psychiatry 2, 2. doi: 10.1186/2049-9256-2-2
Sarnyai, Z., Mello, N. K., Mendelson, J. H., Eros-Sarnyai, M., and Mercer, G. (1996). Effects of cocaine on pulsatile activity of hypothalamic-pituitary-adrenal axis in male rhesus monkeys: neuroendocrine and behavioral correlates. J. Pharmacol. Exp. Ther. 277, 225–234.
Schmidt, H. D., and Pierce, R. C. (2006). Cooperative activation of D1-like and D2-like dopamine receptors in the nucleus accumbens shell is required for the reinstatement of cocaine-seeking behavior in the rat. Neuroscience 142, 451–461. doi: 10.1016/j.neuroscience.2006.06.004
Sharma, H. S., Muresanu, D., Sharma, A., and Patnaik, R. (2009). Cocaine-induced breakdown of the blood–brain barrier and neurotoxicity. Int. Rev. Neurobiol. 88, 297–334. doi: 10.1016/S0074-7742(09)88011-2
Shiu, C., Barbier, E., Di Cello, F., Choi, H. J., and Stins, M. (2007). HIV-1 gp120 as well as alcohol affect blood–brain barrier permeability and stress fiber formation: involvement of reactive oxygen species. Alcohol. Clin. Exp. Res. 31, 130–137. doi: 10.1111/j.1530-0277.2006.00271.x
Siddiqui, N. S., Brown, L. S. Jr., and Makuch, R. W. (1993). Short-term declines in CD4 levels associated with cocaine use in HIV-1 seropositive, minority injecting drug users. J. Natl. Med. Assoc. 85, 293–296.
Siemieniuk, R. A., and Bogoch, I. I. (2015). Preexposure prophylaxis for HIV infection. N. Engl. J. Med. 372, 1767–1768. doi: 10.1056/NEJMc1502749
Sohler, N. L., Wong, M. D., Cunningham, W. E., Cabral, H., Drainoni, M. L., and Cunningham, C. O. (2007). Type and pattern of illicit drug use and access to health care services for HIV-infected people. AIDS Patient Care STDS 21(Suppl. 1), S68–S76. doi: 10.1089/apc.2007.9985
Spudich, S., and Gonzalez-Scarano, F. (2012). HIV-1-related central nervous system disease: current issues in pathogenesis, diagnosis, and treatment. Cold Spring Harb. Perspect. Med. 2, a007120. doi: 10.1101/cshperspect.a007120
Stamatovic, S. M., Keep, R. F., and Andjelkovic, A. V. (2008). Brain endothelial cell-cell junctions: how to “open” the blood–brain barrier. Curr. Neuropharmacol. 6, 179–192. doi: 10.2174/157015908785777210
Tedaldi, E. M., Minniti, N. L., and Fischer, T. (2015). HIV-associated neurocognitive disorders: the relationship of HIV infection with physical and social comorbidities. Biomed Res. Int. 2015, 641913. doi: 10.1155/2015/641913
Telesnitsky, A., and Goff, S. P. (1997). “Reverse transcriptase and the generation of retroviral DNA,” in Source Retroviruses, eds J. M. Coffin, S. H. Hughes, and H. E. Varmus (New York: Cold Spring Harbor Laboratory Press), 121–160.
Toborek, M., Lee, Y. W., Flora, G., Pu, H., Andras, I. E., Wylegala, E., et al. (2005). Mechanisms of the blood–brain barrier disruption in HIV-1 infection. Cell Mol. Neurobiol. 25, 181–199. doi: 10.1007/s10571-004-1383-x
Torres, G., and Rivier, C. (1992). Differential effects of intermittent or continuous exposure to cocaine on the hypothalamic-pituitary-adrenal axis and c-fos expression. Brain Res. 571, 204–211. doi: 10.1016/0006-8993(92)90656-T
Tozzi, V., Balestra, P., Bellagamba, R., Corpolongo, A., Salvatori, M. F., Visco-Comandini, U., et al. (2007). Persistence of neuropsychologic deficits despite long-term highly active antiretroviral therapy in patients with HIV-related neurocognitive impairment: prevalence and risk factors. J. Acquir. Immune Defic. Syndr. 45, 174–182. doi: 10.1097/QAI.0b013e318042e1ee
Van Dyke, C., Barash, P. G., Jatlow, P., and Byck, R. (1976). Cocaine: plasma concentrations after intranasal application in man. Science 191, 859–861. doi: 10.1126/science.56036
Venton, B. J., Seipel, A. T., Phillips, P. E., Wetsel, W. C., Gitler, D., Greengard, P., et al. (2006). Cocaine increases dopamine release by mobilization of a synapsin-dependent reserve pool. J. Neurosci. 26, 3206–3209. doi: 10.1523/JNEUROSCI.4901-04.2006
Vittinghoff, E., Hessol, N. A., Bacchetti, P., Fusaro, R. E., Holmberg, S. D., and Buchbinder, S. P. (2001). Cofactors for HIV disease progression in a cohort of homosexual and bisexual men. J. Acquir. Immune Defic. Syndr. 27, 308–314. doi: 10.1097/00126334-200107010-00015
Vivithanaporn, P., Heo, G., Gamble, J., Krentz, H. B., Hoke, A., Gill, M. J., et al. (2010). Neurologic disease burden in treated HIV/AIDS predicts survival: a population-based study. Neurology 75, 1150–1158. doi: 10.1212/WNL.0b013e3181f4d5bb
Walensky, R. P., Paltiel, A. D., Losina, E., Mercincavage, L. M., Schackman, B. R., Sax, P. E., et al. (2006). The survival benefits of AIDS treatment in the United States. J. Infect. Dis. 194, 11–19. doi: 10.1086/505147
Wang, X., and Ho, W. Z. (2011). Drugs of abuse and HIV infection/replication: implications for mother-fetus transmission. Life Sci. 88, 972–979. doi: 10.1016/j.lfs.2010.10.029
Webber, M. P., Schoenbaum, E. E., Gourevitch, M. N., Buono, D., and Klein, R. S. (1999). A prospective study of HIV disease progression in female and male drug users. AIDS 13, 257–262. doi: 10.1097/00002030-199902040-00014
Willuhn, I., Burgeno, L. M., Groblewski, P. A., and Phillips, P. E. (2014). Excessive cocaine use results from decreased phasic dopamine signaling in the striatum. Nat. Neurosci. 17, 704–709. doi: 10.1038/nn.3694
Wolfe, S. A., Jr., Culp, S. G., and De Souza, E. B. (1989). Sigma-receptors in endocrine organs: identification, characterization, and autoradiographic localization in rat pituitary, adrenal, testis, and ovary. Endocrinology 124, 1160–1172. doi: 10.1210/endo-124-3-1160
Woods, S. P., Moore, D. J., Weber, E., and Grant, I. (2009). Cognitive neuropsychology of HIV-associated neurocognitive disorders. Neuropsychol. Rev. 19, 152–168. doi: 10.1007/s11065-009-9102-5
Woolverton, W. L., and Johnson, K. M. (1992). Neurobiology of cocaine abuse. Trends Pharmacol. Sci. 13, 193–200. doi: 10.1016/0165-6147(92)90063-C
Yao, H., Allen, J. E., Zhu, X., Callen, S., and Buch, S. (2009). Cocaine and human immunodeficiency virus type 1 gp120 mediate neurotoxicity through overlapping signaling pathways. J. Neurovirol. 15, 164–175. doi: 10.1080/13550280902755375
Yao, H., Duan, M., and Buch, S. (2011). Cocaine-mediated induction of platelet-derived growth factor: implication for increased vascular permeability. Blood 117, 2538–2547. doi: 10.1182/blood-2010-10-313593
Yong, V. W., Krekoski, C. A., Forsyth, P. A., Bell, R., and Edwards, D. R. (1998). Matrix metalloproteinases and diseases of the CNS. Trends Neurosci. 21, 75–80. doi: 10.1016/S0166-2236(97)01169-7
Keywords: drug use, cocaine, HIV, AIDS, pathogenesis
Citation: Dash S, Balasubramaniam M, Villalta F, Dash C and Pandhare J (2015) Impact of cocaine abuse on HIV pathogenesis. Front. Microbiol. 6:1111. doi: 10.3389/fmicb.2015.01111
Received: 09 August 2015; Accepted: 25 September 2015;
Published: 20 October 2015.
Edited by:
Venkata S. R. Atluri, Florida International University, USAReviewed by:
Nirupama Chandel, Feinstein Institute for Medical Research, USAPrem L. Shama, Emory University School of Medicine, USA
Varghese George, University of Miami, USA
Copyright © 2015 Dash, Balasubramaniam, Villalta, Dash and Pandhare. This is an open-access article distributed under the terms of the Creative Commons Attribution License (CC BY). The use, distribution or reproduction in other forums is permitted, provided the original author(s) or licensor are credited and that the original publication in this journal is cited, in accordance with accepted academic practice. No use, distribution or reproduction is permitted which does not comply with these terms.
*Correspondence: Chandravanu Dash, Y2Rhc2hAbW1jLmVkdQ==; Jui Pandhare, anBhbmRoYXJlQG1tYy5lZHU=