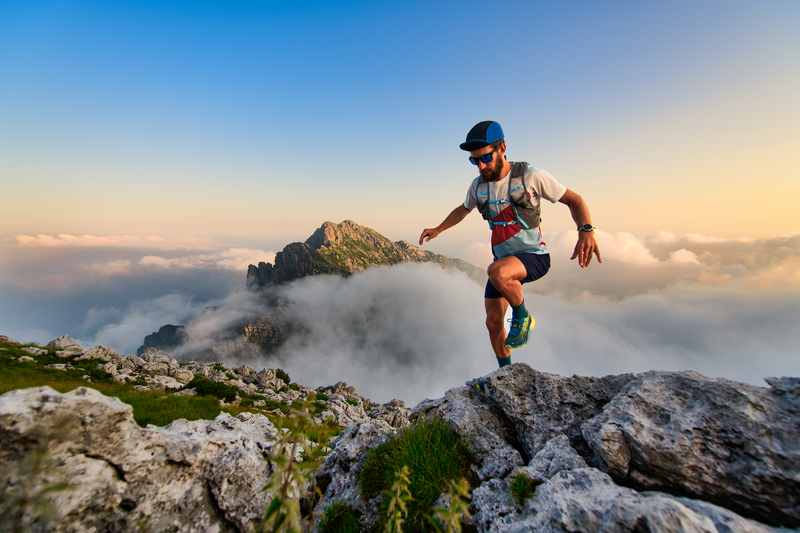
95% of researchers rate our articles as excellent or good
Learn more about the work of our research integrity team to safeguard the quality of each article we publish.
Find out more
PERSPECTIVE article
Front. Microbiol. , 17 July 2015
Sec. Microbiological Chemistry and Geomicrobiology
Volume 6 - 2015 | https://doi.org/10.3389/fmicb.2015.00677
This article is part of the Research Topic Nanomaterial-Biofilm Interactions View all 8 articles
Metal and metal-oxide nanoparticles (NPs) are used in numerous applications and have high likelihood of entering engineered and natural environmental systems. Careful assessment of the interaction of these NPs with bacteria, particularly biofilm bacteria, is necessary. This perspective discusses mechanisms of NP interaction with bacteria and identifies challenges in understanding NP–biofilm interaction, considering fundamental material attributes and inherent complexities of biofilm structure. The current literature is reviewed, both for planktonic bacteria and biofilms; future challenges and complexities are identified, both in light of the literature and a dataset on the toxicity of silver NPs toward planktonic and biofilm bacteria. This perspective aims to highlight the complexities in such studies and emphasizes the need for systematic evaluation of NP–biofilm interaction.
Nanomaterials show unique electrical, optoelectronic, physical, catalytic, and photoactive properties. Metallic nanoparticles (NPs), such as Ag (Chambers et al., 2014) and Cu (Shah et al., 2012; Arijit Kumar et al., 2014), and nano-scale metal-oxides, such as ZnO (Li et al., 2011a,b), TiO2 (Huang et al., 2008; Brunet et al., 2009; Simon-Deckers et al., 2009), and CuO (Heinlaan et al., 2008; Chang et al., 2012; Ivask et al., 2014), exhibit antimicrobial properties, making them useful for water treatment (Theron et al., 2008), odorless textiles (Simoncic and Tomsic, 2010), bandages (Lo et al., 2009; Chaloupka et al., 2010), and biomedical and dental implants (Chen and Schluesener, 2008; Allaker, 2010).
Substantial research has examined the impact of metal and metal-oxide NPs on planktonic (i.e., free-swimming) bacteria. These studies have identified key NP attributes related to NP–cell interaction and associated mechanisms of toxicity for planktonic cells, but the relevance of that literature to NP–biofilm interaction is unknown because the biofilm environment and the biofilm cells themselves are fundamentally different from their planktonic counterparts. This perspective discusses current knowledge on nano-bacterial interaction via underlying antimicrobial mechanisms, notes differences between NP interaction with planktonic and biofilm bacteria, and identifies challenges in NP–biofilm interaction as governed by the complexities of the bacterial biofilm.
Biofilms [i.e., collections of bacteria associated with a surface and surrounded by a matrix of extracellular polymeric substances (EPS)], are common in natural and engineered environments. Planktonic cells attach to a surface (Figure 1Ai,ii); an EPS matrix is produced (Figure 1Aiii) consisting of polysaccharides, proteins, DNA, and lipids (Flemming and Wingender, 2010); the biofilm matures (Figure 1Aiv) during which time the spatial distribution of EPS components can change (Ma et al., 2009); the biofilm acts as a viscoelastic fluid that can undergo detachment events (Stoodley et al., 2002; Figure 1Av).
FIGURE 1. Schematic representing (A) stages in biofilm formation and growth, (B) metal and metal-oxide nanoparticle (NP) interaction with planktonic bacteria and immature biofilm, (C) metal, and metal-oxide NP interaction and translocation in mature biofilm, (D) a close-up of NP interaction with a cell surface, (E) ligand-exchange process of NPs with extracellular polymeric substances (EPS) in a biofilm, and (F) band architecture of a metal-oxide NP showing reactive oxygen species (ROS) generation via electron transfer from the valence band (Ev) to the conduction band (Ec) and eventually to the surrounding fluid.
Planktonic and biofilm cells exhibit key differences. (1) As compared to planktonic cells in a homogeneous environment, biofilm cells are exposed to different microenvironments depending on their location in the biofilm; for instance, biofilms can have gradients of electron donor and acceptor (Tang et al., 2011). (2) Many bacteria can sense surface contact (e.g., by inhibition of flagellar motion) and modulate their transcriptional patterns accordingly (reviewed in Wozniak and Parsek, 2014). For instance, the transcriptional patterns of Campylobacter jejuni shifted more toward iron uptake, oxidative stress combat, and membrane transport in biofilms as compared to planktonic cells (Sampathkumar et al., 2006). (3) Biofilms generally produce substantially more EPS as compared to planktonic cells, especially at low specific growth rates (Evans et al., 1994). Relatedly, distinct differences were found between the transcriptional profiles of Pseudomonas aeruginosa biofilm and planktonic cells, where the expression of EPS-related genes was an important hallmark of the biofilm (Dötsch et al., 2012).
Such differences between planktonic and biofilm cells will influence the impact of antimicrobial agents. As compared to planktonic cells, biofilm cells often show increased tolerance and resistance to traditional antimicrobial agents; such observations are linked with biofilm-specific pumps (Gillis et al., 2005), protection due to biofilm EPS (Billings et al., 2013), and the ability of biofilms to reduce the concentrations of reactive oxygen species (ROS; Nguyen et al., 2011; Khakimova et al., 2013). Thus, the antimicrobiality of NPs (i.e., non-traditional antimicrobial agents) is likely different between planktonic and biofilm cells.
Several major mechanisms have been proposed to explain the stress/toxicity to bacteria exposed to metallic NPs as reviewed by others (Nel et al., 2006; Manke et al., 2013; Reidy et al., 2013; von Moos and Slaveykova, 2014; Djurišić et al., 2015): (a) ROS-mediated oxidative stress, with lipid peroxidation and DNA damage; (b) dissolution of metal ions, which react with cellular components; (c) and physical disruption of the cell envelope. For planktonic bacteria (Figure 1B), these mechanisms are described as follows.
ROS production, under the influence of photo- or chemical-activation, is a common stress/toxicity mechanism for bacteria exposed to metal and metal-oxide NPs [e.g., quantum dots (Lu et al., 2008), Ag (Choi and Hu, 2008), TiO2 (Li et al., 2012), CuO (Zhao et al., 2013; Laha et al., 2014), and ZnO (Li et al., 2012)]. ROS is an aggregate term that encompasses radical and non-radical forms of high energy chemical species, such as singlet oxygen, 1O2; superoxide anion, ; hydroxyl radical, •OH; and hydrogen peroxide, H2O2 (Thannickal and Fanburg, 2000; Finkel, 2001; Apel and Hirt, 2004). ROS can be formed as byproducts of aerobic metabolism (D’Autréaux and Toledano, 2007) and might act as regulatory molecules in prokaryotic cells (Finkel, 2001; Cabiscol et al., 2010). Cells have multiple pathways to limit ROS build-up (D’Autréaux and Toledano, 2007), but loss of cellular function can occur when this capacity is exhausted. As summarized in Figures 1D,F, metal and metal-oxide NPs can induce ROS outside the cell, at the cell membrane, and inside the cell (when NPs are internalized) by direct interaction with biomolecules in the environmental medium, the cell/outer membrane, and organic cytoplasmic components, respectively, or via similar interactions of dissolved metal ions with biomacromolecules (Park et al., 2009; Cabiscol et al., 2010; Dutta et al., 2012); recent studies of metal-oxide NPs have attempted to correlate conduction band-edge positioning with respect to cellular redox potential and the resulting ability to generate ROS (Zhang et al., 2012; Kaweeteerawat et al., 2015).
Extracellular or cell-surface ROS can compromise cellular integrity; membrane-leakage can be incurred via lipid peroxidation or protein modifications (Dutta et al., 2012). Intracellular ROS results in similar lipid peroxidation and protein modification, as well as DNA damage (Sies and Menck, 1992; Cabiscol et al., 2010; Laha et al., 2014).
Ion release from metallic NPs (Figure 1D), such as the release of Ag+, Zn2+, or Cu2+ from nano-scale Ag, ZnO, or CuO, respectively, is an important cause of the antimicrobiality of NPs (Marambio-Jones and Hoek, 2010; Ma et al., 2013; Chambers et al., 2014; Ivask et al., 2014). NP dissolution can occur outside the cell, at the cell surface, or within the cell. Dissolved metals can impact cellular functions, primarily via coordination and non-homeostasis (Chang et al., 2012). Chelation of metal ions with the chemical moieties of intracellular or extracellular ligands, e.g., oxygen, phosphorus, nitrogen, and sulfur functional groups, can alter biomolecule structure or function. For example, Ag+, known to dissolve from silver nanoparticles (AgNPs; Chambers et al., 2014), forms adducts with respiration enzymes, DNA, and membrane-associated proteins via thiol groups, thereby damaging cellular function (Feng et al., 2000; Matsumura et al., 2003; Lok et al., 2006; Wigginton et al., 2010). Relatedly, for the current study, we used live-dead staining with flow cytometry to show loss of membrane integrity in Escherichia coli with increased Ag+ exposure due to AgNP dissolution (Supplementary Figure S1).
As shown in Figure 1D, interaction of metal or metal-oxide NPs with the cell surface can result in chemically and physically induced toxicity (Pal et al., 2007; Okyay et al., 2015). The interaction of NPs with the outer membrane/lipopolysaccharide (LPS) for Gram-negative bacteria and the cell wall/membrane for all bacteria is dependent on local chemistry (e.g., NP coating, LPS composition, pH, and ionic strength). NPs are often coated to stabilize them in aqueous suspension, which typically introduces surface charge and inhibits nanoparticle dissolution/aggregation (Ghosh Chaudhuri and Paria, 2012; Li et al., 2013); Gram-positive and Gram-negative bacteria often are negatively charged in solution (Stendahl et al., 1977; Caputy and Costerton, 1984). The interaction between charged NPs and bacteria can be described via Derjaguin, Landau, Verwey, and Overbeek (DLVO) theory, which includes attractive van der Waals and repulsive electrical double layer forces. The surface functionality of the NPs can induce transport of these particles to and through the cell membrane via favorable electrostatic interaction (Feris et al., 2010; Suresh et al., 2012). Once the NPs undergo an interfacial journey to the inside of a cell, further chemical reactions can take place.
Nanoparticle shape is important in the disruption of the cell envelope. CuO nano-sheets and nano-spheres attached to bacterial cells, but nano-sheets produced more surface damage in Gram-positive bacteria and nano-spheres produced more surface damage in Gram-negative bacteria (Laha et al., 2014). In addition to acting as a localized source of ROS or dissolved metals outside a cell, NPs might cause direct physical stress to the cell membrane [e.g., due to sharp edges of ZnO nanorods (Okyay et al., 2015)]. Such physical disruption is impacted by the presence of dissolved organic matter, which coats NP surfaces and reduces the ability of NPs to injure cells (Zhao et al., 2013).
Many studies have evaluated the interaction between NPs and planktonic bacteria, but few have assessed NP–biofilm interaction. Planktonic cells present a very different interaction environment as compared to mature biofilms (Figures 1B,C). Planktonic cells in a homogenous environment have similar gene expression, metabolic activity, and EPS production (Mikkelsen et al., 2007). Previous studies focusing on traditional antibiotic challenges to bacteria found that the lower metabolic activity of biofilm cells can reduce the effectiveness of certain antibiotics (Walters et al., 2003). Some studies suggest that EPS plays an important role in limiting antibiotic diffusion through the biofilm (Stewart and Costerton, 2001), thus acting as a physical barrier. The well-studied effects of antibiotics on bacteria provide evidence of unique interactions with planktonic versus biofilm cells. NPs as antimicrobial agents present additional complexities due to their unique surface moieties, shape, size, and aggregation propensity. The following section presents current knowledge regarding the NP–biofilm interaction in light of the literature and our laboratory data.
Of the few studies that have evaluated the impact of NPs on biofilms, some have shown that biofilms, as compared to planktonic cells, have reduced susceptibility to NPs (Fabrega et al., 2009; Choi et al., 2010). Choi et al. (2010) found that biofilms were four times less susceptible to AgNP exposure than were planktonic cells. Similarly, as compared to their planktonic counterparts, biofilms of E. coli, P. aeruginosa, and Serratia proteamaculans have been reported to have up to 25 times greater tolerance to AgNPs stabilized with hydrolyzed casein peptides (Radzig et al., 2013). Starch-coated NPs reduced P. aeruginosa and Staphylococcus aureus biofilm growth but completely inactivated planktonic cells at the same AgNP concentrations (Mohanty et al., 2012). Nano-scale titania under UV irradiation has been shown to produce ROS and substantially decrease the growth and development of Pseudomonas fluorescens (Arroyo et al., 2014) and Bacillus subtilis (Dhandapani et al., 2012) biofilms. Dissolved ions from nano ZnO have been shown to suppress biofilm formation of wastewater biofilms (Hou et al., 2014) and of the opportunistic pathogens Rothia dentocariosa and Rothia mucilaginosa (Khan et al., 2014).
Both planktonic and biofilm cells can produce EPS, which has been shown to lower the diffusion rate of NPs (Peulen and Wilkinson, 2011). However, EPS production is much greater in biofilms as compared to planktonic cells (e.g., Hall-Stoodley et al., 2004; Ruas-Madiedo and de los Reyes-Gavilán, 2005; Kives et al., 2006). Although capsular EPS can provide some protection to planktonic cells from NPs (Hessler et al., 2012), its protective capacity is limited in a planktonic environment due to its relatively small quantity as compared to EPS in biofilms.
For the current study, we assessed the tolerance of E. coli and P. aeruginosa biofilm and planktonic cells to mercaptosuccinic-acid-capped AgNPs. AgNP synthesis and tolerance assays were conducted as described previously (Chambers et al., 2014). Biofilm and planktonic cells were grown simultaneously in a spinning-disk reactor either in the absence of AgNPs or in the presence of a subinhibitory concentration (15.6 μg/L) of AgNPs. Approximately equal amounts of biofilm and planktonic cells were retrieved and placed into microtiter plates; after a 5-h exposure to 0-500 μg/L AgNPs, viable cells were enumerated via plate counts.
Biofilm and planktonic cells without previous exposure to AgNPs showed very similar tolerance to AgNPs upon exposure (E. coli in Figure 2A and P. aeruginosa in Figure 2C); thus, at each AgNP concentration, similar numbers of planktonic and biofilm bacteria survived the AgNP exposure. This was contrary to results from previous studies with traditional antimicrobial agents, where biofilms generally show higher tolerance to traditional antimicrobials than do planktonic cells (e.g., as for P. aeruginosa exposed to tobramycin; Kirisits et al., 2005).
FIGURE 2. Silver nanoparticle (AgNP) tolerance assays for planktonic and biofilm cells of Escherichia coli and Pseudomonas aeruginosa. (A) E. coli cells without previous exposure to AgNPs, (B) E. coli cells with previous exposure to 15.6 μg/L AgNPs, (C) P. aeruginosa cells without previous exposure to AgNPs, (D) P. aeruginosa cells with previous exposure to 15.6 μg/L AgNPs. The detection limit (DL) for colony-forming units (CFU) in each sample was calculated by dividing the minimum CFU detectable on a plate (1 CFU) by the fraction of the total original sample volume plated for the planktonic or biofilm assay. Plate counts falling below the DL are plotted as one-half of the DL.
However, the tolerances of biofilm and planktonic cells to AgNPs (for both E. coli and P. aeruginosa) diverged from one another when the cells were originally grown in the presence of a subinhibitory concentration of 15.6 μg/L AgNPs (see no substantial loss of viability at 15.6 μg/L AgNPs in Figures 2A,C). After having been grown previously in the presence of 15.6 μg/L AgNPs, biofilm cells generally showed greater tolerance to the subsequent AgNP exposure as compared to planktonic cells (Figures 2B,D), which is in keeping with what would be expected based on traditional antimicrobial studies. For planktonic and biofilm E. coli cells, the previous exposure to AgNPs did not provide an advantage to the cells when subsequently exposed to higher AgNP concentrations in the tolerance assay (compare Figures 2A,B); the previous exposure to the subinhibitory concentration weakened E. coli’s tolerance to subsequent AgNP exposures. On the other hand, for planktonic and biofilm P. aeruginosa cells, the previous exposure to AgNPs generally provided an advantage to the cells when subsequently exposed to higher AgNP concentrations in the tolerance assay (compare Figures 2C,D); thus, the previous exposure to the subinhibitory concentration improved P. aeruginosa’s tolerance to subsequent AgNP exposures. The differences between the E. coli and P. aeruginosa results suggest that these organisms employ different mechanisms to combat stress from AgNPs. These data also suggest that long-term exposure to low concentrations of NPs could render opportunistic human pathogens (e.g., P. aeruginosa) better able to withstand efforts to eradicate them with NP-based antimicrobial agents. While these conclusions pertain to AgNPs, generalizing these results to other metal NPs and bacteria requires investigation of bacterial stress response to NPs at a fundamental (e.g., transcriptomic) level.
The challenges in understanding NP–biofilm interaction arise from the inherent complexities of NPs and biofilms. NPs not only introduce variability in surface moieties, electronic structure, and chemical identity, but the interaction between NPs (i.e., homoaggregation) and the interaction of NPs with environmental surfaces (i.e., heteroaggregation) also complicate our understanding. The underlying antimicrobial mechanisms are not immune to these variations.
ROS production is correlated with conduction band position with respect to cellular redox potential (Zhang et al., 2012); however, nano-scale defects and metal doping will alter the band structure and shift the conduction band position, likely further complicating ROS-mediated toxicity. EPS on biofilms will likely coat NP surfaces, thereby altering electronic structure and ROS generation. Aggregation governs particle transport to planktonic cells and can influence the kinetics of particle mass delivery to bacteria. However, for biofilms, aggregation not only will influence transport and mass delivery but also will influence NP translocation through the biofilm. The absence of oxygen limits NP surface oxidation (Xiu et al., 2011); thus, the low oxygen microenvironments in deeper biofilm layers will likely limit the rate of dissolution of metal NPs, thereby reducing their antimicrobial activity.
Biofilm complexities will have a profound impact on NP–NP interaction or NP translocation through the biofilm, particularly due to EPS. Metallic NPs can deposit onto EPS polysaccharides (Ikuma et al., 2014). Thus EPS could act as a physical barrier to translocation of metal NPs through the biofilm. Studies have shown a reduction in NP diffusion in biofilms as compared to bulk solution (Habimana et al., 2011; Peulen and Wilkinson, 2011). EPS also might participate in ligand exchange, where the NP coating could be exchanged with EPS molecules, resulting in either alteration of NP aggregation or NP interfacial interaction with biofilm cells (Figure 1E).
Furthermore, effects of the classical mechanisms discussed for the interaction of NPs with planktonic cells might be exacerbated or ameliorated in biofilms. For example, the release of ROS and/or metal ions in biofilms likely is prolonged because of the longer residence time and localized interaction of the NPs with cells in the biofilm, as compared to their momentary interaction with planktonic bacteria (Peulen and Wilkinson, 2011). If metal ion release occurs, chelation of dissolved metals can occur with moieties in EPS (Kaplan et al., 1987; McLean et al., 1990); this would decrease the free metal ions available to attack cellular proteins and DNA. On the other hand, NP surfaces can undergo ligand exchange or passivation (Figure 1E) via chemical reaction with the molecules or moieties in biofilm EPS (Khan et al., 2011), which would decrease the release of metal ions. Further, entrapment of NPs within the biofilm might inhibit UV activation and associated ROS production, thereby reducing the antimicrobial activity of the NPs. Proteins and carbohydrate chains can react with ROS, thereby mitigating the effects of metal and metal-oxide NPs (Hessler et al., 2012).
Beyond the general advantage provided to biofilms by EPS, biofilm cells often have significant transcriptional differences as compared to planktonic cells (Sampathkumar et al., 2006; Dötsch et al., 2012). Numerous stress response elements are up-regulated in biofilms, which are closely associated with metal stress response, DNA repair, and metabolic stress. These systems might relieve stress caused by metal or metal-oxide NPs.
Systematic studies are necessary to understand NP interaction with and translocation through biofilms as well as stress modulation in biofilms exposed to NPs. Such studies need to carefully control NP attributes (e.g., aggregation, surface functionalities, electronic structure, size, and shape) and should assess NP interaction with biofilms at different stages of development. These controlled studies are needed to carefully decipher the mechanisms of interaction of NPs in the complex biofilm environment.
The authors declare that the research was conducted in the absence of any commercial or financial relationships that could be construed as a potential conflict of interest.
The authors thank the National Science Foundation for funding (CBET 0846719 to MJK and CBET 1440261 to NS).
The Supplementary Material for this article can be found online at: http://journal.frontiersin.org/article/10.3389/fmicb.2015.00677
Allaker, R. (2010). The use of nanoparticles to control oral biofilm formation. J. Dent. Res. 89, 1175–1186. doi: 10.1177/0022034510377794
Apel, K., and Hirt, H. (2004). Reactive oxygen species: metabolism, oxidative stress, and signal transduction. Annu. Rev. Plant Biol. 55, 373–399. doi: 10.1146/annurev.arplant.55.031903.141701
Arijit Kumar, C., Ruchira, C., and Tarakdas, B. (2014). Mechanism of antibacterial activity of copper nanoparticles. Nanotechnology 25, 135101. doi: 10.1088/0957-4484/25/13/135101
Arroyo, J., Olmos, D., Orgaz, B., Puga, C., San José, C., and González-Benito, J. (2014). Effect of the presence of titania nanoparticles in the development of Pseudomonas fluorescens biofilms on LDPE. RSC Adv. 4, 51451–51458. doi: 10.1039/C4RA09642H
Billings, N., Ramirez Millan, M., Caldara, M., Rusconi, R., Tarasova, Y., Stocker, R., et al. (2013). The extracellular matrix component Psl provides fast-acting antibiotic defense in Pseudomonas aeruginosa biofilms. PLoS Pathog 9:e1003526. doi: 10.1371/journal.ppat.1003526
Brunet, L., Lyon, D. Y., Hotze, E. M., Alvarez, P. J. J., and Wiesner, M. R. (2009). Comparative photoactivity and antibacterial properties of C60 fullerenes and titanium dioxide nanoparticles. Environ. Sci. Technol. 43, 4355–4360. doi: 10.1021/es803093t
Cabiscol, E., Tamarit, J., and Ros, J. (2010). Oxidative stress in bacteria and protein damage by reactive oxygen species. Int. Microbiol. 3, 3–8.
Caputy, G., and Costerton, J. W. (1984). Immunological examination of the glycocalyces of Staphylococcus aureus strains. Curr. Microbiol. 11, 297–302. doi: 10.1007/BF01567390
Chaloupka, K., Malam, Y., and Seifalian, A. M. (2010). Nanosilver as a new generation of nanoproduct in biomedical applications. Trends Biotechnol. 28, 580–588. doi: 10.1016/j.tibtech.2010.07.006
Chambers, B. A., Afrooz, A. R. M. N., Bae, S., Aich, N., Katz, L., Saleh, N. B., et al. (2014). Effects of chloride and ionic strength on physical morphology, dissolution, and bacterial toxicity of silver nanoparticles. Environ. Sci. Technol. 48, 761–769. doi: 10.1021/es403969x
Chang, Y.-N., Zhang, M., Xia, L., Zhang, J., and Xing, G. (2012). The toxic effects and mechanisms of CuO and ZnO nanoparticles. Materials 5, 2850–2871. doi: 10.3390/ma5122850
Chen, X., and Schluesener, H. J. (2008). Nanosilver: a nanoproduct in medical application. Toxicol. Lett. 176, 1–12. doi: 10.1016/j.toxlet.2007.10.004
Choi, O., and Hu, Z. (2008). Size dependent and reactive oxygen species related nanosilver toxicity to nitrifying bacteria. Environ. Sci. Technol. 42, 4583–4588. doi: 10.1021/es703238h
Choi, O., Yu, C.-P., Esteban Fernández, G., and Hu, Z. (2010). Interactions of nanosilver with Escherichia coli cells in planktonic and biofilm cultures. Water Res. 44, 6095–6103. doi: 10.1016/j.watres.2010.06.069
D’Autréaux, B., and Toledano, M. B. (2007). ROS as signalling molecules: mechanisms that generate specificity in ROS homeostasis. Nat. Rev. Mol. Cell. Biol. 8, 813–824. doi: 10.1038/nrm2256
Dhandapani, P., Maruthamuthu, S., and Rajagopal, G. (2012). Bio-mediated synthesis of TiO2 nanoparticles and its photocatalytic effect on aquatic biofilm. J. Photochem. Photobiol. B Biol. 110, 43–49. doi: 10.1016/j.jphotobiol.2012.03.003
Djurišić, A. B., Leung, Y. H., Ng, A. M. C., Xu, X. Y., Lee, P. K. H., Degger, N., et al. (2015). Toxicity of metal oxide nanoparticles: mechanisms, characterization, and avoiding experimental artefacts. Small 11, 26–44. doi: 10.1002/smll.201303947
Dötsch, A., Eckweiler, D., Schniederjans, M., Zimmermann, A., Jensen, V., Scharfe, M., et al. (2012). The Pseudomonas aeruginosa transcriptome in planktonic cultures and static biofilms using RNA sequencing. PLoS ONE 7:e31092. doi: 10.1371/journal.pone.0031092
Dutta, R. K., Nenavathu, B. P., Gangishetty, M. K., and Reddy, A. V. R. (2012). Studies on antibacterial activity of ZnO nanoparticles by ROS induced lipid peroxidation. Colloids Surf. B Biointerfaces 94, 143–150. doi: 10.1016/j.colsurfb.2012.01.046
Evans, E., Brown, M. R., and Gilbert, P. (1994). Iron chelator, exopolysaccharide and protease production in Staphylococcus epidermidis: a comparative study of the effects of specific growth rate in biofilm and planktonic culture. Microbiology 140, 153–157. doi: 10.1099/13500872-140-1-153
Fabrega, J., Renshaw, J. C., and Lead, J. R. (2009). Interactions of silver nanoparticles with Pseudomonas putida biofilms. Environ. Sci. Technol. 43, 9004–9009. doi: 10.1021/es901706j
Feng, Q. L., Wu, J., Chen, G. Q., Cui, F. Z., Kim, T. N., and Kim, J. O. (2000). A mechanistic study of the antibacterial effect of silver ions on Escherichia coli and Staphylococcus aureus. J. Biomed. Mater. Res. 52, 662–668. doi: 10.1002/1097-4636(20001215)52:4<662::AID-JBM10>3.0.CO;2-3
Feris, K., Otto, C., Tinker, J., Wingett, D., Punnoose, A., Thurber, A., et al. (2010). Electrostatic interactions affect nanoparticle-mediated toxicity to gram-negative bacterium Pseudomonas aeruginosa PAO1. Langmuir 26, 4429–4436. doi: 10.1021/la903491z
Finkel, T. (2001). Reactive oxygen species and signal transduction. IUBMB Life 52, 3–6. doi: 10.1080/15216540252774694
Flemming, H.-C., and Wingender, J. (2010). The biofilm matrix. Nat. Rev. Microbiol. 8, 623–633. doi: 10.1038/nrmicro2415
Ghosh Chaudhuri, R., and Paria, S. (2012). Core/shell nanoparticles: classes, properties, synthesis mechanisms, characterization, and applications. Chem. Rev. 112, 2373–2433. doi: 10.1021/cr100449n
Gillis, R. J., White, K. G., Choi, K.-H., Wagner, V. E., Schweizer, H. P., and Iglewski, B. H. (2005). Molecular basis of azithromycin-resistant Pseudomonas aeruginosa biofilms. Antimicrob. Agents Chemother. 49, 3858–3867. doi: 10.1128/AAC.49.9.3858-3867.2005
Habimana, O., Steenkeste, K., Fontaine-Aupart, M.-P., Bellon-Fontaine, M.-N., Kulakauskas, S., and Briandet, R. (2011). Diffusion of nanoparticles in biofilms is altered by bacterial cell wall hydrophobicity. Appl. Environ. Microbiol. 77, 367–368. doi: 10.1128/AEM.02163-10
Hall-Stoodley, L., Costerton, J. W., and Stoodley, P. (2004). Bacterial biofilms: from the natural environment to infectious diseases. Nat. Rev. Microbiol. 2, 95–108. doi: 10.1038/nrmicro821
Heinlaan, M., Ivask, A., Blinova, I., Dubourguier, H.-C., and Kahru, A. (2008). Toxicity of nanosized and bulk ZnO, CuO and TiO2 to bacteria Vibrio fischeri and crustaceans Daphnia magna and Thamnocephalus platyurus. Chemosphere 71, 1308–1316. doi: 10.1016/j.chemosphere.2007.11.047
Hessler, C. M., Wu, M.-Y., Xue, Z., Choi, H., and Seo, Y. (2012). The influence of capsular extracellular polymeric substances on the interaction between TiO2 nanoparticles and planktonic bacteria. Water Res. 46, 4687–4696. doi: 10.1016/j.watres.2012.06.009
Hou, J., Miao, L., Wang, C., Wang, P., Ao, Y., Qian, J., et al. (2014). Inhibitory effects of ZnO nanoparticles on aerobic wastewater biofilms from oxygen concentration profiles determined by microelectrodes. J. Hazard. Mater. 276, 164–170. doi: 10.1016/j.jhazmat.2014.04.048
Huang, Z., Zheng, X., Yan, D., Yin, G., Liao, X., Kang, Y., et al. (2008). Toxicological effect of ZnO nanoparticles based on bacteria. Langmuir 24, 4140–4144. doi: 10.1021/la7035949
Ikuma, K., Madden, A. S., Decho, A. W., and Lau, B. L. T. (2014). Deposition of nanoparticles onto polysaccharide-coated surfaces: implications for nanoparticle-biofilm interactions. Environ. Sci. Nano 1, 117–122. doi: 10.1039/c3en00075c
Ivask, A., Juganson, K., Bondarenko, O., Mortimer, M., Aruoja, V., Kasemets, K., et al. (2014). Mechanisms of toxic action of Ag, ZnO and CuO nanoparticles to selected ecotoxicological test organisms and mammalian cells in vitro: a comparative review. Nanotoxicology 8, 57–71. doi: 10.3109/17435390.2013.855831
Kaplan, D., Christiaen, D., and Arad, S. (1987). Chelating properties of extracellular polysaccharides from Chlorella spp. Appl. Environ. Microbiol. 53, 2953–2956.
Kaweeteerawat, C., Ivask, A., Liu, R., Zhang, H., Chang, C. H., Low-Kam, C., et al. (2015). Toxicity of metal oxide nanoparticles in Escherichia coli correlates with conduction band and hydration energies. Environ. Sci. Technol. 49, 1105–1112. doi: 10.1021/es504259s
Khakimova, M., Ahlgren, H. G., Harrison, J. J., English, A. M., and Nguyen, D. (2013). The stringent response controls catalases in Pseudomonas aeruginosa and is required for hydrogen peroxide and antibiotic tolerance. J. Bacteriol. 195, 2011–2020. doi: 10.1128/JB.02061-12
Khan, S. T., Ahamed, M., Musarrat, J., and Al-Khedhairy, A. A. (2014). Anti-biofilm and antibacterial activities of zinc oxide nanoparticles against the oral opportunistic pathogens Rothia dentocariosa and Rothia mucilaginosa. Eur. J. Oral Sci. 122, 397–403. doi: 10.1111/eos.12152
Khan, S. S., Mukherjee, A., and Chandrasekaran, N. (2011). Impact of exopolysaccharides on the stability of silver nanoparticles in water. Water Res. 45, 5184–5190. doi: 10.1016/j.watres.2011.07.024
Kirisits, M. J., Prost, L., Starkey, M., and Parsek, M. R. (2005). Characterization of colony morphology variants isolated from Pseudomonas aeruginosa biofilms. Appl. Environ. Microbiol. 71, 4809–4821. doi: 10.1128/AEM.71.8.4809-4821.2005
Kives, J., Orgaz, B., and SanJosé, C. (2006). Polysaccharide differences between planktonic and biofilm-associated EPS from Pseudomonas fluorescens B52. Colloids Surf. B Biointerfaces 52, 123–127. doi: 10.1016/j.colsurfb.2006.04.018
Laha, D., Pramanik, A., Laskar, A., Jana, M., Pramanik, P., and Karmakar, P. (2014). Shape-dependent bactericidal activity of copper oxide nanoparticle mediated by DNA and membrane damage. Mater. Res. Bull. 59, 185–191. doi: 10.1016/j.materresbull.2014.06.024
Li, M., Pokhrel, S., Jin, X., Mädler, L., Damoiseaux, R., and Hoek, E. M. V. (2011a). Stability, bioavailability, and bacterial toxicity of ZnO and iron-doped ZnO nanoparticles in aquatic media. Environ. Sci. Technol. 45, 755–761. doi: 10.1021/es102266g
Li, M., Zhu, L., and Lin, D. (2011b). Toxicity of ZnO nanoparticles to Escherichia coli: mechanism and the influence of medium components. Environ. Sci. Technol. 45, 1977–1983. doi: 10.1021/es102624t
Li, Y., Zhang, W., Niu, J., and Chen, Y. (2012). Mechanism of photogenerated reactive oxygen species and correlation with the antibacterial properties of engineered metal-oxide nanoparticles. ACS Nano 6, 5164–5173. doi: 10.1021/nn300934k
Li, Y., Zhang, W., Niu, J., and Chen, Y. (2013). Surface-coating-dependent dissolution, aggregation, and reactive oxygen species (ROS) generation of silver nanoparticles under different irradiation conditions. Environ. Sci. Technol. 47, 10293–10301. doi: 10.1021/es400945v
Lo, S.-F., Chang, C.-J., Hu, W.-Y., Hayter, M., and Chang, Y.-T. (2009). The effectiveness of silver-releasing dressings in the management of non-healing chronic wounds: a meta-analysis. J. Clin. Nurs. 18, 716–728. doi: 10.1111/j.1365-2702.2008.02534.x
Lok, C.-N., Ho, C.-M., Chen, R., He, Q.-Y., Yu, W.-Y., Sun, H., et al. (2006). Proteomic analysis of the mode of antibacterial action of silver nanoparticles. J. Proteome. Res. 5, 916–924. doi: 10.1021/pr0504079
Lu, Z., Li, C. M., Bao, H., Qiao, Y., Toh, Y., and Yang, X. (2008). Mechanism of antimicrobial activity of CdTe quantum dots. Langmuir 24, 5445–5452. doi: 10.1021/la704075r
Ma, H., Williams, P. L., and Diamond, S. A. (2013). Ecotoxicity of manufactured ZnO nanoparticles – A review. Environ. Pollut. 172, 76–85. doi: 10.1016/j.envpol.2012.08.011
Ma, L., Conover, M., Lu, H., Parsek, M. R., Bayles, K., and Wozniak, D. J. (2009). Assembly and development of the Pseudomonas aeruginosa biofilm matrix. PLoS Pathog 5:e1000354. doi: 10.1371/journal.ppat.1000354
Manke, A., Wang, L., and Rojanasakul, Y. (2013). Mechanisms of nanoparticle-induced oxidative stress and toxicity. BioMed. Res. Int. 2013:15. doi: 10.1155/2013/942916
Marambio-Jones, C., and Hoek, E. V. (2010). A review of the antibacterial effects of silver nanomaterials and potential implications for human health and the environment. J. Nanopart. Res. 12, 1531–1551. doi: 10.1007/s11051-010-9900-y
Matsumura, Y., Yoshikata, K., Kunisaki, S.-i., and Tsuchido, T. (2003). Mode of bactericidal action of silver zeolite and its comparison with that of silver nitrate. Appl. Environ. Microbiol. 69, 4278–4281. doi: 10.1128/AEM.69.7.4278-4281.2003
McLean, R. J. C., Beauchemin, D., Clapham, L., and Beveridge, T. J. (1990). Metal-binding characteristics of the gamma-glutamyl capsular polymer of Bacillus licheniformis ATCC 9945. Appl. Environ. Microbiol. 56, 3671–3677.
Mikkelsen, H., Duck, Z., Lilley, K., and Welch, M. (2007). Interrelationships between colonies, biofilms, and planktonic cells of Pseudomonas aeruginosa. J. Bacteriol. 189, 2411–2416. doi: 10.1128/JB.01687-06
Mohanty, S., Mishra, S., Jena, P., Jacob, B., Sarkar, B., and Sonawane, A. (2012). An investigation on the antibacterial, cytotoxic, and antibiofilm efficacy of starch-stabilized silver nanoparticles. Nanomedicine 8, 916–924. doi: 10.1016/j.nano.2011.11.007
Nel, A., Xia, T., Mädler, L., and Li, N. (2006). Toxic potential of materials at the nanolevel. Science 311, 622–627. doi: 10.1126/science.1114397
Nguyen, D., Joshi-Datar, A., Lepine, F., Bauerle, E., Olakanmi, O., Beer, K., et al. (2011). Active starvation responses mediate antibiotic tolerance in biofilms and nutrient-limited bacteria. Science 334, 982–986. doi: 10.1126/science.1211037
Okyay, T. O., Bala, R. K., Nguyen, H. N., Atalay, R., Bayam, Y., and Rodrigues, D. F. (2015). Antibacterial properties and mechanisms of toxicity of sonochemically grown ZnO nanorods. RSC Adv. 5, 2568–2575. doi: 10.1039/C4RA12539H
Pal, S., Tak, Y. K., and Song, J. M. (2007). Does the antibacterial activity of silver nanoparticles depend on the shape of the nanoparticle? A study of the gram-negative bacterium Escherichia coli. Appl. Environ. Microbiol. 73, 1712–1720. doi: 10.1128/AEM.02218-06
Park, H.-J., Kim, J. Y., Kim, J., Lee, J.-H., Hahn, J.-S., Gu, M. B., et al. (2009). Silver-ion-mediated reactive oxygen species generation affecting bactericidal activity. Water Res. 43, 1027–1032. doi: 10.1016/j.watres.2008.12.002
Peulen, T.-O., and Wilkinson, K. J. (2011). Diffusion of nanoparticles in a biofilm. Environ. Sci. Technol. 45, 3367–3373. doi: 10.1021/es103450g
Radzig, M. A., Nadtochenko, V. A., Koksharova, O. A., Kiwi, J., Lipasova, V. A., and Khmel, I. A. (2013). Antibacterial effects of silver nanoparticles on gram-negative bacteria: influence on the growth and biofilms formation, mechanisms of action. Colloids Surf. B Biointerfaces 102, 300–306. doi: 10.1016/j.colsurfb.2012.07.039
Reidy, B., Haase, A., Luch, A., Dawson, K., and Lynch, I. (2013). Mechanisms of silver nanoparticle release, transformation and toxicity: a critical review of current knowledge and recommendations for future studies and applications. Materials 6, 2295–2350. doi: 10.3390/ma6062295
Ruas-Madiedo, P., and de los Reyes-Gavilán, C. G. (2005). Invited review: methods for the screening, isolation, and characterization of exopolysaccharides produced by lactic acid bacteria. J. Dairy Sci. 88, 843–856. doi: 10.3168/jds.S0022-0302(05)72750-8
Sampathkumar, B., Napper, S., Carrillo, C. D., Willson, P., Taboada, E., Nash, J. H. E., et al. (2006). Transcriptional and translational expression patterns associated with immobilized growth of Campylobacter jejuni. Microbiology 152, 567–577. doi: 10.1099/mic.0.28405-0
Shah, V., Haiduk, B., Collins, D., Afrooz, A., Aich, N., Rispoli, F., et al. (2012). “Aggregation and antimicrobial activity of copper nanoparticle suspension,” in Proceedings of the 243rd National Meeting: American Chemical Society, Washington, DC.
Sies, H., and Menck, C. F. M. (1992). Singlet oxygen induced DNA damage. Mutat. Res. 275, 367–375. doi: 10.1016/0921-8734(92)90039-R
Simon-Deckers, A., Loo, S., Mayne-L’hermite, M., Herlin-Boime, N., Menguy, N., Reynaud, C., et al. (2009). Size-, composition-and shape-dependent toxicological impact of metal oxide nanoparticles and carbon nanotubes toward bacteria. Environ. Sci. Technol. 43, 8423–8429. doi: 10.1021/es9016975
Simoncic, B., and Tomsic, B. (2010). Structures of novel antimicrobial agents for textiles-A review. Text. Res. J. 80, 1721–1737. doi: 10.1177/0040517510363193
Stendahl, O., Edebo, L., Magnusson, K.-E., Tagesson, C., and Hjertén, S. (1977). Surface-charge characteristics of smooth and rough Salmonella typhimurium bacteria determined by aqueous two-phase partitioning and free zone electrophoresis. Acta Pathol. Microbiol. Scand. B 85B, 334–340. doi: 10.1111/j.1699-0463.1977.tb01984.x
Stewart, P. S., and Costerton, J. W. (2001). Antibiotic resistance of bacteria in biofilms. Lancet 358, 135–138. doi: 10.1016/S0140-6736(01)05321-1
Stoodley, P., Cargo, R., Rupp, C. J., Wilson, S., and Klapper, I. (2002). Biofilm material properties as related to shear-induced deformation and detachment phenomena. J. Ind. Microbiol. Biot. 29, 361–367. doi: 10.1038/sj.jim.7000282
Suresh, A. K., Pelletier, D. A., Wang, W., Morrell-Falvey, J. L., Gu, B., and Doktycz, M. J. (2012). Cytotoxicity induced by engineered silver nanocrystallites is dependent on surface coatings and cell types. Langmuir 28, 2727–2735. doi: 10.1021/la2042058
Tang, Y., Zhao, H., Marcus, A. K., Krajmalnik-Brown, R., and Rittmann, B. E. (2011). A steady-state biofilm model for simultaneous reduction of nitrate and perchlorate, Part 1: model development and numerical solution. Environ. Sci. Technol. 46, 1598–1607. doi: 10.1021/es203129s
Thannickal, V. J., and Fanburg, B. L. (2000). Reactive oxygen species in cell signaling. Am. J. Physiol. Lung. Cell Mol. Physiol. 279, L1005–L1028.
Theron, J., Walker, J. A., and Cloete, T. E. (2008). Nanotechnology and water treatment: applications and emerging opportunities. Crit. Rev. Microbiol. 34, 43–69. doi: 10.1080/10408410701710442
von Moos, N., and Slaveykova, V. I. (2014). Oxidative stress induced by inorganic nanoparticles in bacteria and aquatic microalgae – state of the art and knowledge gaps. Nanotoxicology 8, 605–630. doi: 10.3109/17435390.2013.809810
Walters, M. C., Roe, F., Bugnicourt, A., Franklin, M. J., and Stewart, P. S. (2003). Contributions of antibiotic penetration, oxygen limitation, and low metabolic activity to tolerance of Pseudomonas aeruginosa biofilms to ciprofloxacin and tobramycin. Antimicrob. Agents Chemother. 47, 317–323. doi: 10.1128/AAC.47.1.317-323.2003
Wigginton, N. S., de Titta, A., Piccapietra, F., Dobias, J., Nesatyy, V. J., Suter, M. J. F., et al. (2010). Binding of silver nanoparticles to bacterial proteins depends on surface modifications and inhibits enzymatic activity. Environ. Sci. Technol. 44, 2163–2168. doi: 10.1021/es903187s
Wozniak, D. J., and Parsek, M. R. (2014). Surface-associated microbes continue to surprise us in their sophisticated strategies for assembling biofilm communities. F1000Prime Rep. 6:26. doi: 10.12703/P6-26
Xiu, Z.-M., Ma, J., and Alvarez, P. J. J. (2011). Differential effect of common ligands and molecular oxygen on antimicrobial activity of silver nanoparticles versus silver ions. Environ. Sci. Technol. 45, 9003–9008. doi: 10.1021/es201918f
Zhang, H., Ji, Z., Xia, T., Meng, H., Low-Kam, C., Liu, R., et al. (2012). Use of metal oxide nanoparticle band gap to develop a predictive paradigm for oxidative stress and acute pulmonary inflammation. ACS Nano 6, 4349–4368. doi: 10.1021/nn3010087
Keywords: metal-oxide nanoparticles, reactive oxygen species, dissolved ion, physical disruption, EPS
Citation: Saleh NB, Chambers B, Aich N, Plazas-Tuttle J, Phung-Ngoc HN and Kirisits MJ (2015) Mechanistic lessons learned from studies of planktonic bacteria with metallic nanomaterials: implications for interactions between nanomaterials and biofilm bacteria. Front. Microbiol. 6:677. doi: 10.3389/fmicb.2015.00677
Received: 16 January 2015; Accepted: 22 June 2015;
Published: 17 July 2015.
Edited by:
Boris Lau, University of Massachusetts Amherst, USAReviewed by:
Patrick K. H. Lee, City University of Hong Kong, Hong KongCopyright © 2015 Saleh, Chambers, Aich, Plazas-Tuttle, Phung-Ngoc and Kirisits. This is an open-access article distributed under the terms of the Creative Commons Attribution License (CC BY). The use, distribution or reproduction in other forums is permitted, provided the original author(s) or licensor are credited and that the original publication in this journal is cited, in accordance with accepted academic practice. No use, distribution or reproduction is permitted which does not comply with these terms.
*Correspondence: Mary Jo Kirisits, Department of Civil, Architectural and Environmental Engineering, The University of Texas at Austin, 301 East Dean Keeton Street, Stop 1700, Austin, TX 78712, USA,a2lyaXNpdHNAdXRleGFzLmVkdQ==
†These authors have contributed equally to this work.
Disclaimer: All claims expressed in this article are solely those of the authors and do not necessarily represent those of their affiliated organizations, or those of the publisher, the editors and the reviewers. Any product that may be evaluated in this article or claim that may be made by its manufacturer is not guaranteed or endorsed by the publisher.
Research integrity at Frontiers
Learn more about the work of our research integrity team to safeguard the quality of each article we publish.