- 1Department of Chemistry and Biomolecular Sciences, Macquarie University, Sydney, NSW, Australia
- 2Astbury Centre for Structural Molecular Biology, School of Biomedical Sciences, University of Leeds, Leeds, UK
- 3Laboratory for Microbial Dynamics, Department of Pharmaceutical Biosciences, School of Pharmacy, University of Oslo, Oslo, Norway
The era of antibiotics as a cure-all for bacterial infections appears to be coming to an end. The emergence of multidrug resistance in many hospital-associated pathogens has resulted in “superbugs” that are effectively untreatable. Multidrug efflux pumps are well known mediators of bacterial drug resistance. Genome sequencing efforts have highlighted an abundance of putative efflux pump genes in bacteria. However, it is not clear how many of these pumps play a role in antimicrobial resistance. Efflux pump genes that participate in drug resistance can be under tight regulatory control and expressed only in response to substrates. Consequently, changes in gene expression following antimicrobial shock may be used to identify efflux pumps that mediate antimicrobial resistance. Using this approach we have characterized several novel efflux pumps in bacteria. In one example we recently identified the Acinetobacter chlorhexidine efflux protein (AceI) efflux pump in Acinetobacter. AceI is a prototype for a novel family of multidrug efflux pumps conserved in many proteobacterial lineages. The discovery of this family raises the possibility that additional undiscovered intrinsic resistance proteins may be encoded in the core genomes of pathogenic bacteria.
Introduction
Multidrug efflux pumps are a significant obstacle preventing the control of infections caused by pathogenic bacteria. Genes encoding these transporters have been found in all bacterial genomes sequenced, and the overexpression of just one can lead to the reduced efficacy of a range of structurally and mechanistically unrelated antimicrobials (Ren and Paulsen, 2007; Brzoska et al., 2013). Five families of transporters that include multidrug efflux systems have been studied extensively, and include representative proteins that have been characterized biochemically and by tertiary structural analyses. These include the ATP-binding cassette (ABC) superfamily, the major facilitator superfamily (MFS), the resistance/nodulation/division (RND) superfamily, the small multidrug resistance (SMR) family, and the multidrug and toxic compound extrusion (MATE) family (Figure 1).
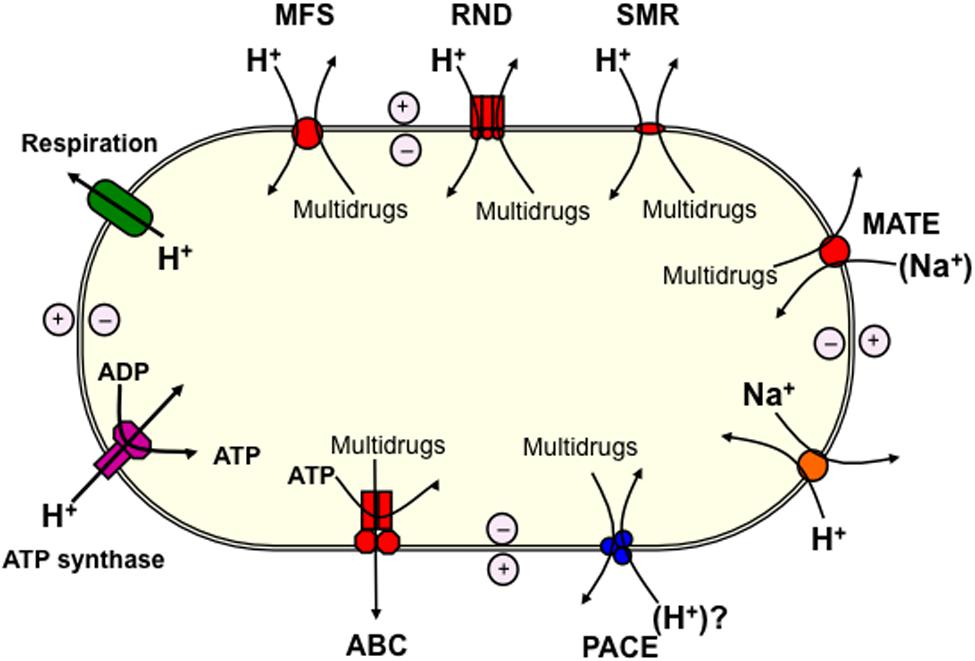
FIGURE 1. Schematic diagram showing the basis for the energisation of multidrug efflux pumps operating in bacteria. The large oval represents the bacterial cell. An electrochemical H+ gradient (proton-motive-force) across the cytoplasmic membrane is generated as a result of respiration (green). Energy from the proton-motive-force is used to power transport in secondary active transport systems, such as those within the major facilitator superfamily (MFS), resistance/nodulation/division (RND), and small multidrug resistance (SMR) (super) families. Sodium/proton antiporters (orange) harness the proton-motive-force to generate a Na+ gradient that powers transport by other multidrug efflux pumps, including those in the multidrug and toxic compound extrusion (MATE) family. ATP production by ATP-synthase (pink) is also powered by the proton-motive-force, and ATP is used to power transport by the primary active transporters of the ATP-binding cassette (ABC) superfamily. Previously characterized efflux pump (super) families are shown in red, whereas the proteobacterial antimicrobial compound efflux (PACE) family is shown in blue. The PACE family transport proteins are likely to be powered by the H+ gradient.
Significant longstanding difficulties surround identifying the physiological functions of these multidrug efflux transport proteins and determining which of the many pumps encoded by bacterial strains actually contribute to antimicrobial resistance (Piddock, 2006; Schindler et al., 2015). Studies have shown that these efflux pumps often have overlapping substrate recognition profiles (Tal and Schuldiner, 2009). Furthermore, it is not uncommon for a bacterial genome to encode a large number of efflux pumps that have predicted drug substrates, e.g., strains of Bacillus cereus encode more than 100 of these pumps accounting for more than 2% or their predicted protein coding potential (Ren and Paulsen, 2007; Simm et al., 2012). It is unlikely that all these pumps share the primary function of protection against toxic compounds, highlighting a need for higher throughput approaches to assess the physiological roles of individual proteins, be they in drug resistance, native housekeeping functions, or other cellular roles.
Efflux Pumps Participate in Intrinsic, Adaptive, and Acquired Resistance
Bacterial drug resistance can be divided into three general categories, intrinsic, adaptive, and acquired (Fernandez and Hancock, 2012). Depending on their mode(s) of regulation and their local genetic context, bacterial multidrug efflux pumps can be geared to participate in any of these three resistance categories. Intrinsic resistance stems from inherent properties of a bacterial cell and can occur as a result of high constitutive expression and activity of some multidrug efflux pumps. Adaptive resistance is related to physiological alterations that are induced by environmental changes and can occur when multidrug efflux pumps are expressed in response to antimicrobial substrates. Finally, acquired resistance can result from mutations promoting constitutive expression of an ordinarily tightly controlled endogenous multidrug efflux system, or when efflux pump genes are acquired on a mobile genetic element, such as a plasmid or phage.
Adaptive Resistance Responses Identify Efflux Pumps that Mediate Drug Resistance
High-level expression of efflux pumps can have a negative impact on cell growth (Brzoska et al., 2013), resulting in a need to control the timing of efflux system expression to coincide with specific physiological requirements. As such, efflux pumps with physiological resistance functions may be characteristically expressed in response to drug substrates. These pumps may be part of an adaptive drug resistance response or part of general stress response regulons. For example, expression of the adeAB and adeIJK efflux pump genes in Acinetobacter baumannii (Hassan et al., 2013; our unpublished data), the acrAB and acrF genes in Escherichia coli (Shaw et al., 2003; Bailey et al., 2009), the mexXY and mexCD genes in Pseudomonas aeruginosa (Morita et al., 2014), the norA gene in Staphylococcus aureus (Kaatz and Seo, 2004), and the bmr gene in Bacillus subtilis (Ahmed et al., 1994), is induced in response to antimicrobial shock treatments.
The mode of regulation and regulatory cues of most efflux pumps are typically only investigated after their functional characterisation. However, global gene expression profiles that show heightened expression of putative efflux pump genes following drug or toxin shocks have provided the impetus to assess the drug resistance functions of these pumps. For example, members of our team recognized that an uncharacterised MFS exporter, BC4707, was expressed in response to bile salt shock in the human food-poisoning associated pathogen Bacillus cereus, and went on to characterize its role in drug resistance (Kristoffersen et al., 2007). The gene encoding BC4707 is conserved in the core genome of B. cereus and its deletion from B. cereus ATCC 14579 resulted in increased susceptibility to norfloxacin (Simm et al., 2012). Overexpression of BC4707 in E. coli BL21 ΔacrAB resulted in increased resistance to norfloxacin, ciprofloxacin, and kanamyacin and fluorescence transport assays showed that accumulation of norfloxacin is reduced by BC4707 in an energy dependent manner (Simm et al., 2012).
Adaptive Resistance Responses Identify a New Class of Drug Efflux Pump
Extending from this work, we have exploited adaptive resistance responses to identify efflux pumps that may mediate drug resistance in hospital-acquired bacterial pathogens, with a focus on biocide resistance. For example, in recent work we conducted a transcriptomic study to examine the regulatory response of A. baumannii to a shock treatment with the synthetic biocide chlorhexidine (Hassan et al., 2013). Chlorhexidine is commonly applied in antibacterial soaps, mouthwashes and antiseptics, and is listed as an “Essential Medicine” by the World Health Organization. Chlorhexidine is a membrane active biocide and as such, multidrug efflux pumps are commonly associated with reduced levels of susceptibility (Russell, 1986; McDonnell and Russell, 1999). In line with the discussion above, the most highly overexpressed genes in our chlorhexidine shock treatment encoded AdeAB, components of a major tripartite RND multidrug efflux system in A. baumannii (Hassan et al., 2013). This efflux system has previously been shown to mediate resistance to a very broad range of antimicrobials and biocides, including chlorhexidine (Rajamohan et al., 2009). The overexpression of genes encoding AdeAB in response to chlorhexidine confirmed the role of this efflux system in adaptive resistance to chlorhexidine in A. baumannii. Apart from the genes encoding AdeAB, only one gene was highly (>10-fold) overexpressed in response to chlorhexidine. This gene was originally annotated as encoding a hypothetical membrane protein. Using biochemical approaches we showed that this protein is in fact a chlorhexidine resistance protein that functions via an active efflux mechanism (Hassan et al., 2013). We named this protein the Acinetobacter chlorhexidine efflux protein I (AceI).
The AceI Transporter is a Prototype for a New Family of Bacterial Multidrug Efflux Systems
The AceI transport protein contains two tandem “Bacterial Transmembrane Pair” (BTP) protein domains defined within the Pfam database (Finn et al., 2014). There are more than 750 protein sequences containing this domain architecture listed in the Pfam database (version 27.0). Genes encoding these proteins are particularly common among proteobacterial lineages, but can also be found in the genomes of unrelated bacterial genera, including the Firmicutes and Actinobacteria. We have not yet identified these genes in the genomes of any archaeal or eukaryotic organisms.
We have recently characterized more than 20 homologs of the AceI transporter by heterologous expression in E. coli (Hassan et al., 2015). These studies have demonstrated that many AceI homologs are able to provide resistance to an array of biocides in addition to chlorhexidine. For example, the VP1155 protein from Vibrio parahaemolyticus and Bcen2424_2356 protein from Burkholderia cenocepacia each conferred increased resistance to chlorhexidine, benzalkonium, acriflavine, and proflavine, when expressed in E. coli (Hassan et al., 2015). Fluorescence transport assays conducted on cells expressing these and other AceI homologs that conferred resistance to acriflavine and proflavine, demonstrated that these compounds are actively exported from the cell by these transporters (Hassan et al., 2015). These results corroborate our earlier findings that chlorhexidine is actively transported by AceI (Hassan et al., 2013), and indicate that efflux is the mechanism of resistance operating in this group of proteins. Taken together all the observations suggest that these proteins comprise a new family of multidrug efflux pumps common amongst Proteobacterial lineages. We have named this family the Proteobacterial Antimicrobial Compound Efflux (PACE) family (Figure 1; Hassan et al., 2015).
PACE Family Proteins are Encoded Within the Core Genome
Given that the PACE family represents a new class of resistance determinants, we were interested in gathering basic information regarding the mode of inheritance of these genes in bacteria. To this end, we examined their level of conservation within representative bacterial lineages following the basic premise that highly conserved genes within core bacterial genomes are expected to have been inherited vertically, whereas those in the accessory genome are likely to have been horizontally acquired.
We examined PACE family protein conservation in four γ-Proteobacterial species (A. baumannii, P. aeruginosa, V. parahaemolyticus, and E. coli) a β-Proteobacterial species (B. cenocepacia) and a member of the Firmicutes (Veillonella parvula). Annotated protein sequences from all complete and draft genomes of these species were downloaded from the NCBI Genbank database (October, 2014) and were queried using the BTP PfamHMM (Finn et al., 2014) in HMMER3 searches (Eddy, 2011). These searches determined that PACE family proteins are encoded in the pan-genomes of all six species examined. To determine the number of distinct orthologous groups of PACE family proteins in each species we performed a clustering analysis based on sequence identity (cluster stringency >90%) using cd-hit v4.6.1 (Fu et al., 2012). This analysis demonstrated that A. baumannii had three clusters (100, 96.7, and 0.3% conservation in 623 strains); P. aeruginosa had three clusters (99.5, 99.5, and 0.5% conservation in 197 strains); V. parahaemolyticus had one cluster (90.1% conservation in 101 strains); E. coli had 4 clusters (0.2, 0.1, 0.1, and 0.1% conservation in 1986 strains); B. cenocepacia had three clusters (100, 88.9, and 88.9% conservation in nine strains); and V. parvula had one cluster (100% conservation in four strains).
These data demonstrate that the pattern of PACE family protein conservation is variable between the species. For example, both A. baumannii and P. aeruginosa each encode two highly conserved PACE family proteins present in virtually all sequenced strains, and one additional PACE protein encoded in one or two specific strains. Whereas, V. parahaemolyticus and V. parvula each encode only one highly conserved PACE protein, and B. cenocepacia encodes three highly conserved PACE proteins. Most E. coli strains do not encode a PACE family protein, although a small handful of strains encode one of four PACE protein variants. The highly conserved PACE family proteins encoded by A. baumannii, P. aeruginosa, V. parahaemolyticus, B. cenocepacia, and V. parvula are likely to constitute part of the core genome in these species and to have been inherited vertically rather than on mobile genetic elements. The almost complete lack of genes encoding PACE family proteins in E. coli strains suggests that these genes were lost early in the development of the E. coli lineage, but after its divergence from other γ-proteobacteria. In the few cases where E. coli strains were found to encode a PACE family protein, it was sometimes associated with mobile genetic elements suggesting that it had been acquired by horizontal gene transfer. The paucity of PACE genes in E. coli strains confirms our previous conclusion that E. coli is an excellent host to study the function of these proteins (Hassan et al., 2013).
Physiological Substrates for PACE Family Transporters
To date, the substrates identified for PACE family transport proteins include synthetic biocides only, such as chlorhexidine, dequalinium, benzalkonium, proflavine, and acriflavine. The presence of these toxic biocides in the environments occupied by Proteobacteria is likely to have been negligible across evolutionary time, until perhaps the last 50–100 years when these compounds were applied in various industries. Given that the organisms encoding PACE family genes are likely to have diverged long before the development of this potential selective pressure, it is seems unlikely that biocides are the native physiological substrates of PACE efflux pumps. Nonetheless, these genes are transcriptionally responsive to at least one biocide, chlorhexidine in four species, A. baumannii, A. baylyi, P. aeruginosa, and B. cenocepacia (Nde et al., 2009; Coenye et al., 2011; Hassan et al., 2013), suggesting that chlorhexidine can serve as a mimic of their natural physiological substrate for inducing efflux pump expression.
Regulatory Proteins Acting on PACE Efflux Pumps
In addition to antimicrobial resistance, the promiscuous substrate recognition profiles of multidrug efflux pumps allow them to participate in diverse physiological processes. For example, efflux systems in Gram-negative bacteria function in cell adherence, invasion, biofilm formation, virulence in animals and plants, and resistance to host encoded factors (Piddock, 2006). Consequently, the regulation of bacterial drug efflux systems can be highly complex and responsive to a range of cellular and extracellular conditions. Complex regulation may be particularly apparent in efflux pumps, such as AceI and its homologs, which are encoded within core bacterial genomes. These genes are likely to have been present in these species for significant periods of evolutionary time, allowing fine-tuning of their expression in response to a range of environmental cues. A case in point, as summarized within the EcoCyc database (Keseler et al., 2013), transcription of the acrAB efflux system genes, within the core genome of E. coli, is controlled by at least seven distinct regulatory proteins, which are themselves subject to a range of regulatory pressures. These regulatory proteins are likely to integrate efflux pumps into the adaptive resistance responses observed in bacteria, as well as other pathways controlling their alternative physiological functions.
Regulators mediating the most direct control of genes encoding efflux pumps are often encoded locally – adjacent to and divergently transcribed from the gene(s) encoding the efflux system. These regulators, either activators or repressors, typically bind a similar spectrum of compounds to their cognate efflux pump with high affinity as a signal for transcriptional activation or relief of transcriptional repression. Some well characterized examples include AcrR, which controls transcription of the E. coli acrAB efflux pump genes (Li et al., 2007), and QacR, which controls qacA/qacB expression in S. aureus (Grkovic et al., 1998; Schumacher et al., 2001).
The PACE family transporters that we have studied to date are each encoded adjacent to a divergently transcribed LysR family regulator. To determine whether these regulators control the expression of their cognate PACE family gene, we used our established methods (Brzoska et al., 2013) to construct a deletion mutant of the regulator gene ACIAD1979 in A. baylyi ADP1, which is encoded adjacent to the PACE family chlorhexidine resistance gene ACIAD1978. We examined the expression of ACIAD1978 in both the wild-type and the ΔACIAD1979 regulatory mutant in response to chlorhexidine shock treatments using quantitative real-time PCR analysis (Brzoska and Hassan, 2014). In the absence of chlorhexidine the expression of ACIAD1978 was similar in both strains. However, whereas increasing concentrations of chlorhexidine induced ACIAD1978 gene expression in the wild-type strain, chlorhexidine addition failed to induce ACIAD1978 expression in the ΔACIAD1979 mutant (Figure 2). These results suggest that the ACIAD1979 LysR family regulator functions as an activator of the PACE family gene ACIAD1978. We are currently investigating the role of LysR family proteins in controlling expression of PACE family pumps in other species and are determining whether the spectrum of ligands recognized by these regulators is closely linked to the substrate recognition profile of their cognate PACE family pump. It also remains to be determined whether the PACE-associated regulators control expression of other genes, or if there are distally encoded regulators that also modulate expression of PACE transporter genes.
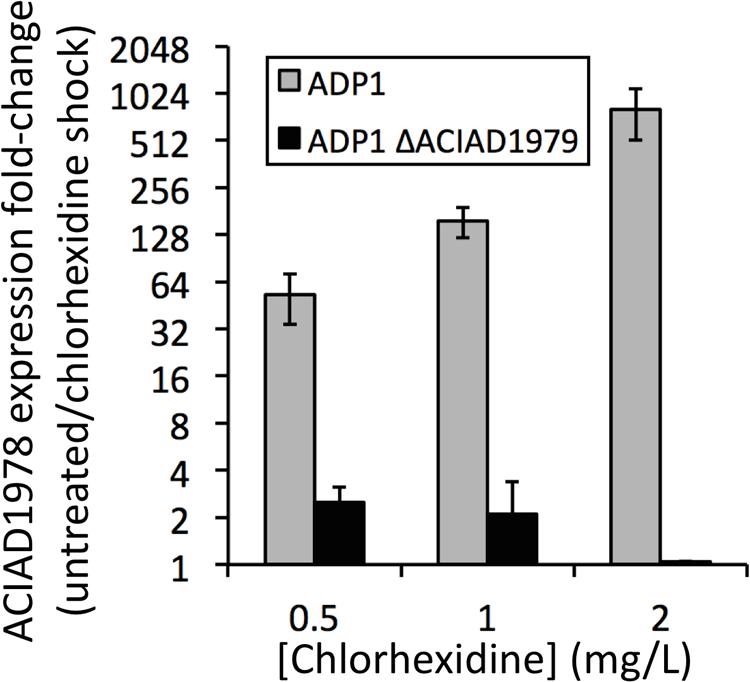
FIGURE 2. Expression of the PACE family gene, ACIAD1978, in wild-type and mutant (ΔACIAD1979) A. baylyi ADP1 in response to chlorhexidine shock treatments. Cells were grown in LB broth to OD600 = 0.6, then split, with one half of each culture treated with chlorhexidine and the other half receiving no treatment. RNA was isolated and assessed by qRT-PCR following our established protocols (Brzoska and Hassan, 2014) to determine relative expression levels of ACIAD17978 in chlorhexidine treated and untreated samples. Error bars show the SEM. Changes in expression of ACIAD1978 were negligible in the ACIAD1979 mutant treated with 2 mg/L chlorhexidine, and thus the bar is difficult to see.
Conclusion and Future Directions
Transcriptomic analyses of antimicrobial shock treatments are valuable in identifying the potential resistance mechanisms operating in bacteria, including multidrug efflux pumps participating in the adaptive resistance response. Using transcriptomic analyses, we have defined roles for new efflux pumps and identified the PACE family of multidrug transport proteins, the first new family of drug efflux proteins discovered in over a decade.
Transporters within the PACE family are currently enigmas. We have identified drug substrates, such as chlorhexidine that are common to many of these pumps. Furthermore, PACE family gene expression is induced by chlorhexidine, a response that is mediated via locally encoded regulators. This highlights a close relationship between the function of these pumps and their regulatory control. Since PACE family genes are encoded in the core genomes of bacterial lineages that diverged long ago, this functional-regulatory relationship is likely to have arisen early in the evolution of these proteins. However, the substrates/inducers that have been identified for PACE proteins are synthetic biocides that are likely to have been absent from the environment until the last 50–100 years. Therefore, it is unlikely that these biocides would have provided the selective pressure required to drive the functional or regulatory evolution of PACE family pumps. Consequently, a deeper understanding of these novel resistance proteins requires future investigations aimed at identifying their physiological substrate(s) and primary functional roles in bacteria.
The discovery of the PACE family opens up the possibility that there may be more novel efflux proteins waiting to be discovered. There are many hypothetical membrane proteins of unknown function encoded in all bacterial genomes. For example, even in the best-studied bacterial genome, E. coli K12, there are 409 membrane proteins of unknown function. At least some of these may represent entirely novel types of efflux pumps.
Conflict of Interest Statement
The authors declare that the research was conducted in the absence of any commercial or financial relationships that could be construed as a potential conflict of interest.
Acknowledgments
This work was supported by National Health and Medical Research Council (Australia) Project Grant (1060895) to IP, KH, and PH and a Macquarie University Research Development Grant (9201401563) to KH and LE. Collaboration between the Australian and UK laboratories is supported by an EU International Research Staff Exchange Scheme BacMT 247634 (AK, PH, and IP). Additional travel support was provided by the Australian Academy of Science (IP). Work in the PH laboratory was supported by EU European Drug Initiative for Channels and Transporters Grant 201924, and also by a Leverhulme Trust Emeritus Award to PH.
References
Ahmed, M., Borsch, C. M., Taylor, S. S., Vázquez-Laslop, N., and Neyfakh, A. A. (1994). A protein that activates expression of a multidrug efflux transporter upon binding the transporter substrates. J. Biol. Chem. 269, 28506–28513.
Bailey, A. M., Constantinidou, C., Ivens, A., Garvey, M. I., Webber, M. A., Coldham, N., et al. (2009). Exposure of Escherichia coli and Salmonella enterica serovar typhimurium to triclosan induces a species-specific response, including drug detoxification. J. Antimicrob. Chemother. 64, 973–985. doi: 10.1093/jac/dkp320
PubMed Abstract | Full Text | CrossRef Full Text | Google Scholar
Brzoska, A. J., and Hassan, K. A. (2014). Quantitative PCR for detection of mRNA and gDNA in environmental isolates. Methods Mol. Biol. 1096, 25–42. doi: 10.1007/978-1-62703-712-9_3
PubMed Abstract | Full Text | CrossRef Full Text | Google Scholar
Brzoska, A. J., Hassan, K. A., De Leon, E. J., Paulsen, I. T., and Lewis, P. J. (2013). Single-step selection of drug resistant Acinetobacter baylyi ADP1 mutants reveals a functional redundancy in the recruitment of multidrug efflux systems. PLoS ONE 8:e56090. doi: 10.1371/journal.pone.0056090
PubMed Abstract | Full Text | CrossRef Full Text | Google Scholar
Coenye, T., Van Acker, H., Peeters, E., Sass, A., Buroni, S., Riccardi, G., et al. (2011). Molecular mechanisms of chlorhexidine tolerance in Burkholderia cenocepacia biofilms. Antimicrob. Agents Chemother. 55, 1912–1919. doi: 10.1128/AAC.01571-10
PubMed Abstract | Full Text | CrossRef Full Text | Google Scholar
Eddy, S. R. (2011). Accelerated profile HMM searches. PLoS Comput. Biol. 7:e1002195. doi: 10.1371/journal.pcbi.1002195
PubMed Abstract | Full Text | CrossRef Full Text | Google Scholar
Fernandez, L., and Hancock, R. E. (2012). Adaptive and mutational resistance: role of porins and efflux pumps in drug resistance. Clin. Microbiol. Rev. 25, 661–681. doi: 10.1128/CMR.00043-12
PubMed Abstract | Full Text | CrossRef Full Text | Google Scholar
Finn, R. D., Bateman, A., Clements, J., Coggill, P., Eberhardt, R. Y., Eddy, S. R., et al. (2014). Pfam: the protein families database. Nucleic. Acids Res. 42, D222–D230. doi: 10.1093/nar/gkt1223
PubMed Abstract | Full Text | CrossRef Full Text | Google Scholar
Fu, L., Niu, B., Zhu, Z., Wu, S., and Li, W. (2012). CD-HIT: accelerated for clustering the next-generation sequencing data. Bioinformatics 28, 3150–3152. doi: 10.1093/bioinformatics/bts565
PubMed Abstract | Full Text | CrossRef Full Text | Google Scholar
Grkovic, S., Brown, M. H., Roberts, N. J., Paulsen, I. T., and Skurray, R. A. (1998). QacR is a repressor protein that regulates expression of the Staphylococcus aureus multidrug efflux pump QacA. J. Biol. Chem. 273, 18665–18673. doi: 10.1074/jbc.273.29.18665
Hassan, K. A., Jackson, S. M., Penesyan, A., Patching, S. G., Tetu, S. G., Eijkelkamp, B. A., et al. (2013). Transcriptomic and biochemical analyses identify a family of chlorhexidine efflux proteins. Proc. Natl. Acad. Sci. U.S.A. 110, 20254–20259. doi: 10.1073/pnas.1317052110
PubMed Abstract | Full Text | CrossRef Full Text | Google Scholar
Hassan, K. A., Li, Q., Henderson, P. J. F., and Paulsen, I. T. (2015). Homologs of the Acinetobacter baumannii aceI transporter represent a new family of bacterial multidrug efflux systems. mBio 6, e01982–e01914. doi: 10.1128/mBio.01982-14
PubMed Abstract | Full Text | CrossRef Full Text | Google Scholar
Kaatz, G. W., and Seo, S. M. (2004). Effect of substrate exposure and other growth condition manipulations on norA expression. J. Antimicrob. Chemother. 54, 364–369. doi: 10.1093/jac/dkh341
PubMed Abstract | Full Text | CrossRef Full Text | Google Scholar
Keseler, I. M., Mackie, A., Peralta-Gil, M., Santos-Zavaleta, A., Gama-Castro, S., Bonavides-Martinez, C., et al. (2013). EcoCyc: fusing model organism databases with systems biology. Nucleic. Acids Res. 41, D605–D612. doi: 10.1093/nar/gks1027
PubMed Abstract | Full Text | CrossRef Full Text | Google Scholar
Kristoffersen, S. M., Ravnum, S., Tourasse, N. J., Okstad, O. A., Kolsto, A. B., and Davies, W. (2007). Low concentrations of bile salts induce stress responses and reduce motility in Bacillus cereus ATCC 14579. J. Bacteriol. 189, 5302–5313. doi: 10.1128/JB.00239-07
PubMed Abstract | Full Text | CrossRef Full Text | Google Scholar
Li, M., Gu, R., Su, C. C., Routh, M. D., Harris, K. C., Jewell, E. S., et al. (2007). Crystal structure of the transcriptional regulator AcrR from Escherichia coli. J. Mol. Biol. 374, 591–603. doi: 10.1016/j.jmb.2007.09.064
PubMed Abstract | Full Text | CrossRef Full Text | Google Scholar
McDonnell, G., and Russell, A. D. (1999). Anitseptics and disinfectants: activity, action and resistance. Clin. Microbiol. Rev. 12, 147–179.
Morita, Y., Tomida, J., and Kawamura, Y. (2014). Responses of Pseudomonas aeruginosa to antimicrobials. Front. Microbiol. 4:422. doi: 10.3389/fmicb.2013.00422
PubMed Abstract | Full Text | CrossRef Full Text | Google Scholar
Nde, C. W., Jang, H. J., Toghrol, F., and Bentley, W. E. (2009). Global transcriptomic response of Pseudomonas aeruginosa to chlorhexidine diacetate. Environ. Sci. Technol. 43, 8406–8415. doi: 10.1021/es9015475
PubMed Abstract | Full Text | CrossRef Full Text | Google Scholar
Piddock, L. J. (2006). Multidrug-resistance efflux pumps - not just for resistance. Nat. Rev. Microbiol. 4, 629–636. doi: 10.1038/nrmicro1464
PubMed Abstract | Full Text | CrossRef Full Text | Google Scholar
Rajamohan, G., Srinivasan, V. B., and Gebreyes, W. A. (2009). Novel role of Acinetobacter baumannii RND efflux transporters in mediating decreased susceptibility to biocides. J. Antimicrob. Chemother. 65, 228–232. doi: 10.1093/jac/dkp427
Ren, Q., and Paulsen, I. T. (2007). Large-scale comparative genomic analyses of cytoplasmic membrane transport systems in prokaryotes. J. Mol. Microbiol. Biotechnol. 12, 165–179. doi: 10.1159/000099639
PubMed Abstract | Full Text | CrossRef Full Text | Google Scholar
Russell, A. D. (1986). Chlorhexidine: antibacterial action and bacterial resistance. Infection 14, 212–215. doi: 10.1007/BF01644264
Schindler, B. D., Frempong-Manso, E., Demarco, C. E., Kosmidis, C., Matta, V., Seo, S. M., et al. (2015). Analyses of multidrug efflux pump-like proteins encoded on the Staphylococcus aureus chromosome. Antimicrob. Agents Chemother. 59, 747–748. doi: 10.1128/AAC.04678-14
PubMed Abstract | Full Text | CrossRef Full Text | Google Scholar
Schumacher, M. A., Miller, M. C., Grkovic, S., Brown, M. H., Skurray, R. A., and Brennan, R. G. (2001). Structural mechanisms of QacR induction and multidrug recognition. Science 294, 2158–2163. doi: 10.1126/science.1066020
PubMed Abstract | Full Text | CrossRef Full Text | Google Scholar
Shaw, K. J., Miller, N., Liu, X., Lerner, D., Wan, J., Bittner, A., et al. (2003). Comparison of the changes in global gene expression of Escherichia coli induced by four bactericidal agents. J. Mol. Microbiol. Biotechnol. 5, 105–122. doi: 10.1159/000069981
PubMed Abstract | Full Text | CrossRef Full Text | Google Scholar
Simm, R., Voros, A., Ekman, J. V., Sodring, M., Nes, I., Kroeger, J. K., et al. (2012). BC4707 is a major facilitator superfamily multidrug resistance transport protein from Bacillus cereus implicated in fluoroquinolone tolerance. PLoS ONE 7:e36720. doi: 10.1371/journal.pone.0036720
PubMed Abstract | Full Text | CrossRef Full Text | Google Scholar
Tal, N., and Schuldiner, S. (2009). A coordinated network of transporters with overlapping specificities provides a robust survival strategy. Proc. Natl. Acad. Sci. U.S.A. 106, 9051–9056. doi: 10.1073/pnas.0902400106
PubMed Abstract | Full Text | CrossRef Full Text | Google Scholar
Keywords: multidrug efflux systems, bacterial transmembrane pair, adaptive resistance, bacterial drug resistance transcriptomics
Citation: Hassan KA, Elbourne LDH, Li L, Gamage HKAH, Liu Q, Jackson SM, Sharples D, Kolstø A-B, Henderson PJF and Paulsen IT (2015) An ace up their sleeve: a transcriptomic approach exposes the AceI efflux protein of Acinetobacter baumannii and reveals the drug efflux potential hidden in many microbial pathogens. Front. Microbiol. 6:333. doi: 10.3389/fmicb.2015.00333
Received: 12 February 2015; Accepted: 02 April 2015;
Published online: 22 April 2015
Edited by:
Keith Poole, Queen’s University, CanadaReviewed by:
Govindan Rajamohan, Council of Scientific and Industrial Research – Institute of Microbial Technology, IndiaAyush Kumar, University of Manitoba, Canada
Copyright © 2015 Hassan, Elbourne, Li, Gamage, Liu, Jackson, Sharples, Kolstø, Henderson and Paulsen. This is an open-access article distributed under the terms of the Creative Commons Attribution License (CC BY). The use, distribution or reproduction in other forums is permitted, provided the original author(s) or licensor are credited and that the original publication in this journal is cited, in accordance with accepted academic practice. No use, distribution or reproduction is permitted which does not comply with these terms.
*Correspondence: Ian T. Paulsen and Karl A. Hassan, Department of Chemistry and Biomolecular Sciences, Macquarie University, Eastern Road, Sydney, NSW 2109, AustraliaaWFuLnBhdWxzZW5AbXEuZWR1LmF1;a2FybC5oYXNzYW5AbXEuZWR1LmF1
†Present address: Scott M. Jackson, Institute of Molecular Biology and Biophysics, ETH Zurich, Zurich, Switzerland