- 1Department of Applied Chemistry and Biochemical Engineering, Graduate School of Engineering, Shizuoka University, Shizuoka, Japan
- 2Department of Bioscience, Graduate School of Science and Technology, Shizuoka University, Shizuoka, Japan
Plasmids are important “vehicles” for the communication of genetic information between bacteria. The exchange of plasmids transmits pathogenically and environmentally relevant traits to the host bacteria, promoting their rapid evolution and adaptation to various environments. Over the past six decades, a large number of plasmids have been identified and isolated from different microbes. With the revolution of sequencing technology, more than 4600 complete sequences of plasmids found in bacteria, archaea, and eukaryotes have been determined. The classification of a wide variety of plasmids is not only important to understand their features, host ranges, and microbial evolution but is also necessary to effectively use them as genetic tools for microbial engineering. This review summarizes the current situation of the classification of fully sequenced plasmids based on their host taxonomy and their features of replication and conjugative transfer. The majority of the fully sequenced plasmids are found in bacteria in the Proteobacteria, Firmicutes, Spirochaetes, Actinobacteria, Cyanobacteria and Euryarcheota phyla, and key features of each phylum are included. Recent advances in the identification of novel types of plasmids and plasmid transfer by culture-independent methods using samples from natural environments are also discussed.
Introduction
Plasmids are circular or linear extrachromosomal replicons that are found in many microorganisms in the domains Bacteria, Archaea, and Eukaryota (Funnell and Phillips, 2004). Plasmids are transmissible by conjugation (Frost et al., 2005; Sota and Top, 2008; Frost and Koraimann, 2010). Smillie et al. (2010) reported that about 14% of the full-sequenced plasmids were predicted to be conjugative. Conjugation is one of the most effective mechanisms to spread genetic elements among bacteria (Guglielmini et al., 2011). It is therefore one of the most important “vehicles” for bacterial communication of genetic information, facilitating the rapid evolution and adaptation abilities seen in bacteria (Aminov, 2011).
Plasmids are also important genetic tools used to manipulate and analyze microorganisms through the introduction, modification or removal of target genes (Frost et al., 2005; Sota and Top, 2008). New plasmids have been reported with the recent revolution in nucleotide sequencing technology. Currently, there are 4602 complete sequences of plasmids in the NCBI Plasmid Genome database: 4418 are from bacteria, 137 are from archaea, and the remaining 47 are from eukaryota (Figure 1A; based on the NCBI database, http://ftp.ncbi.nih.gov/genomes/Plasmids, Aug. 2014).
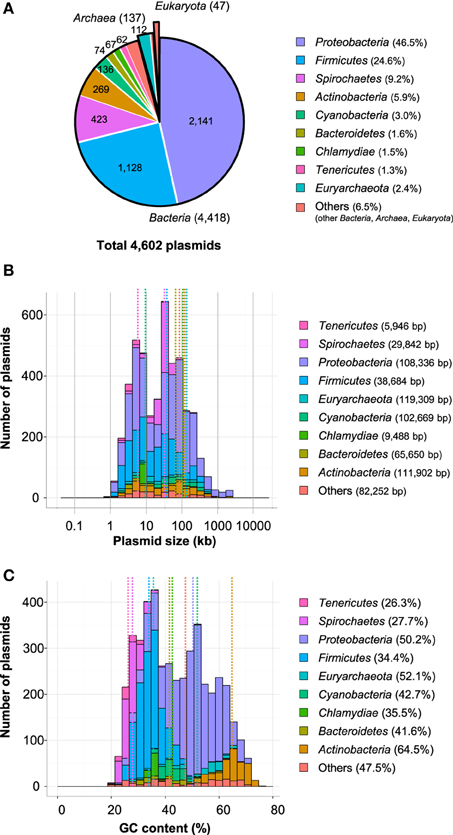
Figure 1. The phylogenetic distribution of fully sequenced plasmids (A), histograms of plasmid size (B), and GC content (%) (C). The ratios of each phylum (A), the average size of plasmids (B), and the average GC contents (C) are shown in parentheses and as dotted lines.
Knowledge of the relationships between plasmid features and host taxonomy is important in order to understand how the plasmids have been spread among microbes. The classification of known plasmids is necessary to identify newly isolated plasmids in the future. Information about the host range of these plasmids is critical to effectively use them as genetic tools for microbial engineering. This information is also important to detect, isolate and identify novel types of plasmids in environmental samples.
In this review, known plasmids for which the complete sequence was available were classified by their host and their (putative) replication or transfer systems. The classification may help to predict which types of plasmids could be replicated in or be transferred to the host microbes. Several insights into the mechanisms of replication and conjugative transfer used by plasmids are summarized first, followed by a description of the features of representative plasmids in several phyla. Recent advances in the identification of novel types of plasmids and plasmid transfers by culture-independent methods using samples from natural environments are also described.
Replication and Transfer Mechanisms
In plasmids, replication occurs at a specific site known as the origin of vegetative replication (oriV). Well-known replication systems of circular plasmids include theta-type replication, rolling-circle replication, and strand displacement-type replication (del Solar et al., 1998). Many theta-type replicating plasmids contain repeated DNA sequences, or iterons, which bind the replication initiation protein, Rep (reviewed in Espinosa et al., 2000; Krüger et al., 2004). The mechanisms of replication control have been extensively studied in two iteron-containing plasmids, pPS10 and R6K (Giraldo and Fernandez-Tresguerres, 2004; Rakowski and Filutowicz, 2013). ColE1-family plasmids are another group of theta-type replicating plasmids whose replication is strictly controlled by an antisense RNA (Polisky, 1988; Cesareni et al., 1991; Espinosa et al., 2000; Brantl, 2004). The rolling-circle replication mechanism is found in many small multi-copy plasmids (Espinosa et al., 2000; Khan, 2005). The representative plasmids using rolling-circle replication are pT181 (Inc14; see below for the discussion of Incompatibility groups), pC194 (Inc8), pMV158 (Inc11), and pUB110 (Inc13), all identified in staphylococci (Khan, 2005). Similar plasmids are also found in Bacillus (Guglielmetti et al., 2007) and several genera in Actinobacteria (Ventura et al., 2007).
Ravin recently reviewed the replication mechanisms of a prophage of Escherichia coli, N15, which was the first linear plasmid identified with covalently closed ends (Ravin, 2011). Most linear plasmids have conserved “telomeres” containing inverted repeat sequences (Ravin, 2011). The 5′ telomeric ends are blocked by covalently attached telomere terminal proteins (Ravin, 2011). Linear plasmids have sets of conserved telomere replication genes known as tpg and tap (Bao and Cohen, 2001, 2003), or tpc and tac (Huang et al., 2007). Many linear type plasmids have been found in Actinobacteria, especially in the genera Mycobacteria, Rhodococcus, and Streptomyces (Ventura et al., 2007).
Conjugative transfer is another important mechanism by which plasmids spread DNA among different bacteria. Self-transmissible plasmids in Gram-negative bacteria generally carry complete sets of genes required for transfer, the origin of transfer (oriT), the relaxase protein, the type IV coupling protein (T4CP), and the type IV secretion system (T4SS). Garcillán-Barcia et al. (2009, 2011) and Smillie et al. (2010) classified the conjugative, or mobilizable, plasmids in the GenBank database into six mobility (MOB) types (MOBC, MOBF, MOBH, MOBP, MOBQ, and MOBV) according to the amino acid sequences of their relaxase proteins. An additional classification was performed based on the plasmids' T4SS involved in mating pair formation (MPF) during conjugation. Smillie et al. proposed four classes of MPF (MPFF, MPFG, MPFI, and MPFT) according to the T4SS amino acid sequences (Smillie et al., 2010). They also investigated the presence of two key elements of plasmid mobility, type IV pili coupling protein (T4CP) and the ATPase VirB4 (Smillie et al., 2010). During conjugation, double-stranded plasmid DNA is cleaved at the oriT site by a relaxase protein, which then covalently binds to the oriT DNA. The resultant DNA-protein complex is transported to the recipient cell by T4SS. This single-stranded DNA is transferred into the recipient cell by the T4CP. The mobilizable plasmids only have oriT, relaxase, and sometimes T4CP (Garcillán-Barcia et al., 2009, 2011).
Gram-positive bacteria transfer plasmids by two methods, although the detailed mechanisms are not well understood. First, a single strand of plasmid DNA is transported via a T4SS, which seems to be widely used as a means for transferring plasmids in Gram positive bacteria (Goessweiner-Mohr et al., 2013). Several plasmids of the order Actinomycetales have conjugative systems that function in a manner similar to the segregation of chromosomal DNA during bacterial cell division and sporulation. The translocation of double-stranded DNA to the recipient cell is mediated by an FtsK-homologous protein (Goessweiner-Mohr et al., 2013). As for archaeal plasmids, only the plasmids in Sulfolobales are known to be transferred (Greve et al., 2004); however, the mechanisms are still not well understood.
Distribution and Classification of Plasmids
Wide variations in both size and GC contents were observed among the 4602 plasmids found in the GenBank database. The average size was 80 kb (range: 744 bp-2.58 Mb), and the average GC content was 44.1% (range: 19.3–75.6%; Table S1). More than 90% of the plasmids in the database were identified in 22 phyla: Proteobacteria (2142 sequences, 47%), Firmicutes (1129 sequences, 25%), Spirochaetes (423 sequences, 9.2%), Actinobacteria (269 sequences, 5.8%), Cyanobacteria (136 sequences, 3.0%) Bacteroidetes (74 sequences, 1.6%), Chlamydiae (67 sequences, 1.5%), and Tenericutes (62 sequences, 1.3%; Figure 1A). The relationships between the phyla of plasmid hosts and plasmid size or GC content are shown in Figures 1B,C. The bimodal distribution of plasmid sizes was plotted as previously shown (Smillie et al., 2010; Garcillán-Barcia et al., 2011) with peaks at approximately 4–8 kb and 32 kb, whereas the number of sequenced plasmids increased from 1730 plasmids to 4602 plasmids. This fact indicates that the distribution of plasmid sizes is conserved if the number of sequenced plasmids increases. The average size of the 4602 plasmids (79.8 kb) was larger than those of 1730 plasmids (63.5 kb). The difference was due to the number of plasmids more than 1 Mb in 4602 plasmids (45 plasmids) was much larger than those in 1730 plasmids (9 plasmids). The average sizes of plasmids in the phyla Actinobacteria, Bacteroidetes, Cyanobacteria, Proteobacteria, and Euryarcheota were similarly distributed (650–1200 kb), while those in Chlamydiae, Firmicutes, Spirocaetes, and Tenericutes were rather small (6–40 kb, Figure 1B). However, the average GC content of plasmids was different in each host phylum (26.3–64.5%, Figure 1C). Nishida reported that the GC contents of the majority of plasmids were lower than those of their host chromosomes, although the difference was less than 10% (Nishida, 2012). Thus, it was reasonable that plasmids found in Actinobacteria had the highest GC content (64.5%; Figure 1C), because their host genomes ranged from 51% to greater than 70% GC (Ventura et al., 2007). It was suggested that the lower GC contents of plasmids than those of host chromosome might be due to the higher energy cost to maintain G and C than A and T (Rocha and Danchin, 2002). On the other hand, the replication of plasmids depending on the host cell's respiration machinery might be expected to have the same GC content as the host (Rocha and Danchin, 2002). Therefore, the GC content of the plasmid is likely to be important for determining its host range. It was suggested that the nucleotide composition of plasmid might be progressively altered toward the average nucleotide composition of the host genome (Lawrence and Ochman, 1998; Rocha and Danchin, 2002). Based on the hypothesis, the relationships of GC contents between the plasmid and the host genome might be also important for predicting when the plasmid was introduced into the host species. Indeed, even in the identical host species, the ranges of GC contents of plasmids were wider than those of the host genome sequence. For example, the GC contents of the whole nucleotide sequences of 10 plasmids in Pseudomonas aeruginosa (Table S1) ranged from 45.8 to 63.8% (the average GC contents were 58.7%). According to the Pseudomonas genome database (http://www.pseudomonas.com/), the GC contents of Pseudomonas aeruginosa ranged from 66.1 to 66.6% (average GC contents = 66.4%). The plasmid with 63.8% GC content might be introduced into strains P. aeruginosa earlier than the one with 45.8%.
Plasmids have been classified based on incompatibility since the 1970s. Incompatibility (Inc) is defined as the inability of plasmids sharing similar replication and partition systems to be propagated stably in the same host cell line. Inc groups have been independently classified in three different genera; there are 27 Inc groups in Enterobacteriaceae, 14 Inc groups of Pseudomonas, and approximately 18 Inc groups in Staphylococcus (Udo and Grubb, 1991; Lawley et al., 2004; Taylor et al., 2004; Thomas and Haines, 2004; Sota and Top, 2008; Carattoli, 2009). Several Inc groups of Pseudomonas are identical to those in enterobacteria, such as IncP-1 (equivalent to IncP), IncP-3 (equivalent to IncA/C), IncP-4 (equivalent to IncQ), and IncP-6(equivalent to IncG/U). Classification into an Inc group is always based on the amino acid sequence of the replication initiation (Rep) protein (replicon typing), and it is not necessarily confirmed by conventional methods whether the plasmid shows incompatibility with the same Inc group plasmid in the same host cell line. The classification based on the replicon typing is also useful for grouping the plasmids in unidentified Inc groups.
As described above, classification of plasmids based on their MOB types and MPF classes has previously been reported (Garcillán-Barcia et al., 2009, 2011; Smillie et al., 2010). Plasmid classification by replicon typing is based on the molecular characteristics of the replicons and has been quite successful (Carattoli et al., 2005). There are difficulties with this method: (i) plasmids frequently carry multiple replicons, and it is therefore difficult to classify the plasmid into single replicon group. (ii) Detailed information about Inc groups or Rep types is limited among several microbial taxonomies especially enterobacteria, and it is difficult to identify replication regions for the other types of plasmids. The classification of plasmids based on their mobility (Garcillán-Barcia et al., 2009; Smillie et al., 2010; Garcillán-Barcia et al., 2011) can overcome these problems because (i) classification by MOB types can cover the whole microbial plasmids, and (ii) plasmids rarely carry more than one relaxase gene (Garcillán-Barcia et al., 2009). However, this classification is not appropriate for non-transmissible plasmids.
In this review, 4602 plasmids with complete sequences listed in the GenBank database were classified based on the genes encoding (putative) Rep proteins, MOB classes, and MPF types. Amino acid sequences of previously identified Rep proteins were used; their accession numbers in the DDBJ/EMBL/GenBank database are listed in Table S2-1. The local TBLASTN program was used for the classification of Rep proteins with the following parameters: e-value <10−5, >50% identity, and >0.5 query coverage. The parameters for identity and coverage were chosen based on variations in the amino acid sequences of the replication initiation protein TrfA from the IncP-1 plasmid (data not shown). The IncP-1 plasmid is one of the best-studied plasmids distributed across many bacterial classes (Adamczyk and Jagura-Burdzy, 2003), and thus, its TrfA protein sequence was used to set the criteria for analysis. For the ColE1 family plasmids, nucleotide sequences of RNA II were used as queries for the local BLASTN program using an e-value <10−5. Notably, the Rep proteins of the IncB, IncFII (RepA1), IncI, and IncK plasmids showed greater than 87% identity with one another. Similarly, the Rep proteins of Inc4, Inc9, Inc10, and Inc14 plasmids have 311–314 amino acid sequences and share a high degree of identity (>75%) with each other. These findings suggest that plasmids from these Inc groups are closely related to one another. In the case that one open reading frame simultaneously showed identity with the Rep proteins of these Inc groups, the one with the smaller e-value was used for the classification. Among the 4602 plasmids analyzed, 1845 plasmids (40.0%) in Proteobacteria, Firmicutes, Actinobacteria, Cyanobacteria, Bacteroidetes, Tenericutes, Euryarchaeota and other phyla were classified into previously known Inc groups or unidentified Inc groups with other known Rep types (Figures 2A,B), although several plasmids were classified into multiple Inc groups (Table S1).
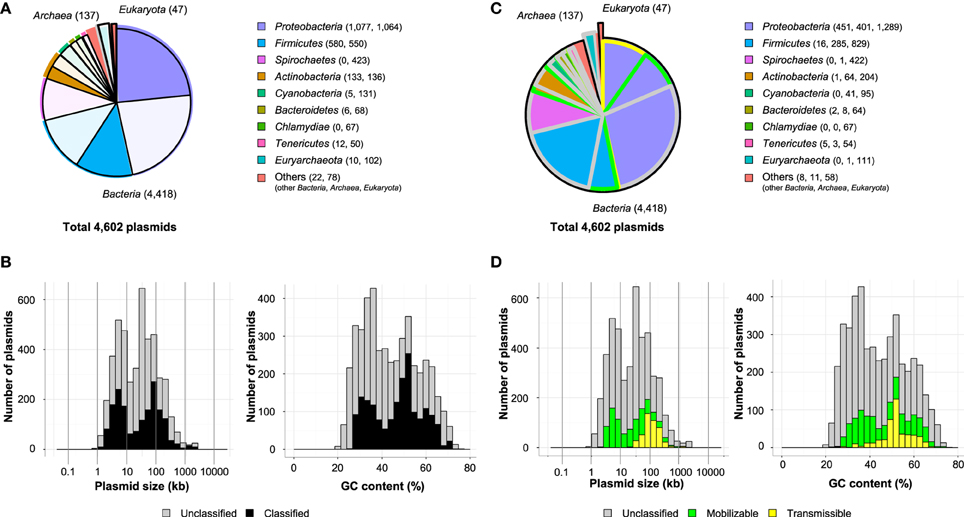
Figure 2. Ratios of classified plasmids in each phylum (A) and histograms of their size (B, left) and GC content (B, right) are shown. The unclassified plasmids are shown as shaded, and the numbers of classified plasmids and unclassified plasmids are shown in parentheses (A). Ratios of putative transmissible plasmids (yellow), putative mobilizable plasmids (green), and others (gray) in each phylum (C) are shown. Histograms of their size (D, left) and GC content (D, right) are shown.
The classification of plasmids into MOB classes and MPF types using T4CPs and VirB4 homologs was performed as proposed by de la Cruz's group (Garcillán-Barcia et al., 2009; Smillie et al., 2010). Instead of using the local PSI-BLAST program (ver. 2.2.24, http://ftp.ncbi.nlm.nih.gov/blast/executables/blast+/LATEST/) as described previously (Garcillán-Barcia et al., 2009), we used the local TBLASTN program with >50% identity and >0.7 query coverage for the alignment of MOB and MPF gene sequences (lists of queries are shown in Table S2-2). The MPF type of a plasmid was determined by the presence of more than two MPF genes on the plasmid. There are five MPF types of plasmids, as defined by de la Cruz's group: (i) non-transmissible plasmids that do not code for a relaxase (“non-mob”); (ii) plasmids that do not contain a relaxase but contain T4CP, VirB4, and MPF, or any two of these three elements (“non-mob, protein export”); (iii) mobilizable plasmids that contain a relaxase gene but lack VirB4 and MPF (“mob”); (iv) conjugative plasmids that contain a known type of T4SS (MPFF, MPFT, MPFI or MPFG), plus relaxase and T4CP (“determined conjugative”); and (v) conjugative plasmids that contain genes for relaxase, T4CP, and VirB4, but whose T4SS does not belong to a specific MPF (“undetermined conjugative”). Because the relationship between the plasmid and each MOB class or MPF type was previously reviewed in detail (Garcillán-Barcia et al., 2009, 2011; Smillie et al., 2010), the distribution of “mob” or putative mobilizable, “determined conjugative” or putative transmissible, and the other plasmids were discussed in this review. As shown in Figure 2C, putative transmissible plasmids were primarily found in the phylum Proteobacteria, with smaller numbers found in Firmicutes, Bacteroidetes, Tenericutes, and others. Many putative mobilizable plasmids were found in the phyla Proteobacteria (401/2141 plasmids, 18.7%), Firmicutes (285/1130 plasmids, 25.2%), Actinobacteria (64/269 plasmids, 23.8%), and Cyanobacteria (41/136 plasmids, 30.1%) (Figure 2C). The mobilizable plasmids had a mean peak at a smaller size (around 5 kb) than that of the transmissible plasmids (around 100 kb; Figure 2D, left). The two major peaks of mobilizable and transmissible plasmids were similarly found as those in previous report (Smillie et al., 2010). This fact indicates that the size distribution of mobilizable and transmissible plasmids is conserved if the number of full-sequenced plasmids increases. As for the distribution of the GC content of the plasmids, both types showed a wide range of values (23–74%; Figure 2D, right), probably because various hosts harbor both mobilizable and conjugative plasmids.
Plasmids in Proteobacteria
Plasmids within Proteobacteria are one of the most extensively studied groups because they include those harboring the pathogenic genes of bacteria known to infect both animals and plants. Indeed, about half of the plasmids in the database were found in Proteobacteria (Figure 1A). Although many catabolic plasmids have also been identified in the phylum Proteobacteria, they are not described in detail here, as they have been reviewed elsewhere (Shintani et al., 2010; Shintani and Nojiri, 2013). The majority of the plasmids within this phylum were found in the classes Gammaproteobacteria (1389; 63%), Alphaproteobacteria (451; 22%), and Betaproteobacteria (187; 8.7%). The size distribution was bimodal, with one group at around 5 kb and the other at 65–131 kb (Figure 3A, left), although the average size of plasmids in each major class was different: 58.7 kb in Gammaproteobacteria, 218 kb in Alphaproteobacteria, and 259 kb in Betaproteobacteria. The average GC contents of plasmids in these groups were 48.0% in Gammaproteobacteria, 56.5% in Alphaproteobacteria, and 58.5% in Betaproteobacteria (Figure 3A, right). Within this phylum, 1077 plasmids were classified using previously known Rep proteins.
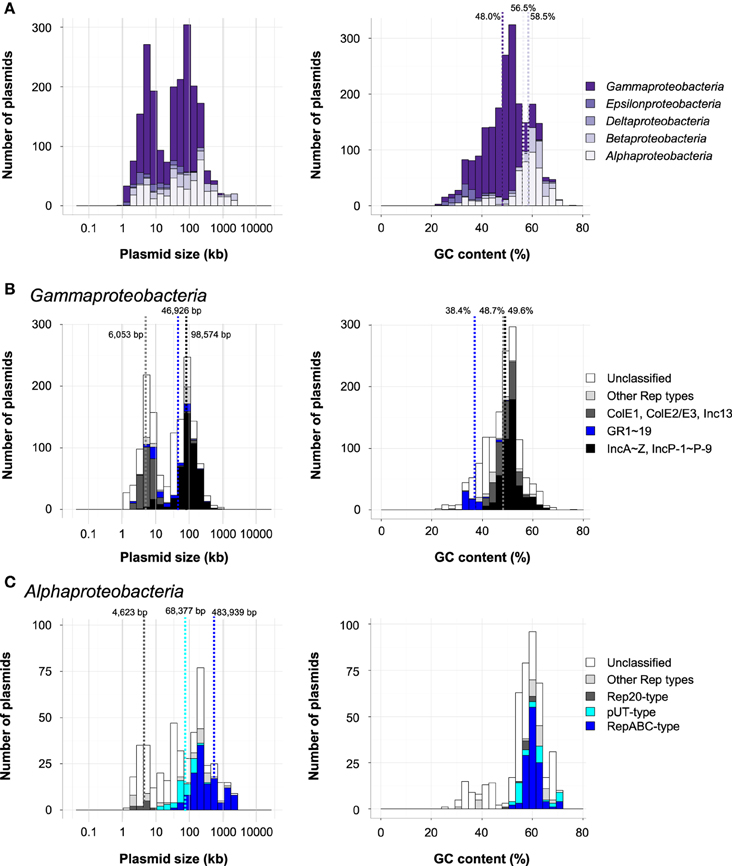
Figure 3. (A) Histograms of plasmid size (left) and GC content (right) of Proteobacteria in each class. (B) Histograms of plasmid size (left) and GC content (right) in Gammaproteobacteria. The distribution of classified plasmids are shown in black (IncA-Z and IncP-1 to IncP-9), blue (GR1 to GR19), dark gray (ColE1, ColE2/E3, and Inc13), light gray (other Rep types), and white (unclassified). (C) Histograms of plasmid size (left) and GC content (right) in Alphaproteobacteria. The distribution of classified plasmids are shown in blue (RepABC-type), light blue (pUT-type), dark gray (Rep20-type), light gray (other Rep types), and white (unclassified). The average sizes of Rep20-type, pUT-type, and RepABC-type plasmids are represented by dotted lines.
Plasmids in Gammaproteobacteria
Among the 1396 plasmids in Gammaproteobacteria, 830 were classified into Inc groups (IncA to IncZ, IncP-1 to IncP-9, and Inc13; 485 plasmids), the ColE1-type plasmid group (210 plasmids), the ColE2/E3-type plasmid group (38 plasmids), and other groups with known Rep (119 plasmids), while several plasmids were classified into multiple Inc or Rep type groups (Table S1). The average sizes of plasmids classified into Inc13, ColE1-type, and ColE2/E3-type plasmids (6053 bp) were smaller than those of other classified plasmids (98,574 bp) (Figure 3B, left), although the average GC contents were similar between the smaller and larger groups (48.7% and 49.6%, respectively; Figure 3B, right). This was probably because the sizes of the plasmids are closely related to their replication systems.
The majority of plasmids in Gammaproteobacteria were from Enterobacteriales (927) and Pseudomonadales (183). The plasmids from Enterobacteriales contain antibiotic resistance plasmids, which cause the rapid development of resistance to antibiotics in bacterial populations. Thus, more than 75% of the plasmids in Enterobacteriales (697/927) were classified into Inc groups or Rep types, including IncF, IncA/C (equivalent to IncP-3), IncL/M, IncI, IncHI2/S, and IncN, containing plasmid families already known to carry resistance genes (Carattoli, 2009; Wang et al., 2013). Carattoli and Partridge have separately reviewed the emergence and spread of antibiotic resistance via plasmids among Enterobacteriaceae (Carattoli, 2009; Partridge, 2011). The spread of plasmids carrying genes for extended-spectrum β-lactamases (ESBLs) has been a particular problem in the treatment of Enterobacteriaceae infections (Paterson, 2006). Plasmid groups found in Enterobacteriales, such as Enterobacter, Escherichia, Salmonella, Shigella, and Yersinia, include a large number of virulence plasmids. It should be noted that virulence-associated traits of E. coli are almost exclusively found on IncF-family plasmids (Johnson and Nolan, 2009). In virulent Yersinia species, extensive research has been carried out on “low calcium ion (Ca2+) response plasmids,” which regulate the growth and expression of several virulence-associated properties by Ca2+ and temperature. This group includes the pCD1, pYVe8081, and pYVe227 series, which encode a set of secreted anti-host proteins and a type III secretion system (Straley et al., 1993). Notably, proteins responsible for replication initiation encoded on pCD-type plasmids showed high similarity with those encoded on IncZ plasmids, and 186 of the 976 plasmids of Enterobacteriales carried genes encoding these types of Rep proteins (Table S1).
Although the IncP-1 to IncP-14 groups have been found in Pseudomonas, only 21 plasmids in the order Pseudomonadales were classified into these groups; the other 93 plasmids classified into these Inc groups were in other orders (including Enterobacteriales and Thiotrichales), other classes (including Alphaproteobacteria and Betaproteobacteria), or even other phyla (including Actinobacteria and Thermotogae; Table S1). Recently, Xiong et al. determined the complete sequence of pOZ176, an IncP-2 plasmid which is resistant to heavy metals (Xiong et al., 2013). Ten additional plasmids have been identified which carry a gene encoding a putative Rep protein with >50% identity and >0.5 query coverage, suggesting that these plasmids may have IncP-2-like replication systems (Table S1). The IncP-2 group contains degradative plasmids whose sizes are typically around 500 kb (Shintani et al., 2010; Shintani and Nojiri, 2013); the average size of the ten plasmids was 425 kb (Table S1). Two plasmids were found to share >83% identity with the sequence of the Rep protein from pOZ176: pPNAP01, a plasmid of Polaromonas naphthalenivorans CJ2, encodes proteins involved in the degradation of naphthalene (Jeon et al., 2003, 2006; Yagi et al., 2009), and pBB1 (GenBank/EMBL/DDBJ accession no. CP002879), a plasmid of Cupriavidus necator N-1, encodes proteins putatively involved in the degradation of catechols (Poehlein et al., 2011). Taken together, these findings suggest that the nucleotide sequences of the replication systems used by previously-known Inc group plasmids could be important tools for classifying other plasmids. It will be therefore helpful for the classification to determine the nucleotide sequences of the other unsequenced Inc group plasmids including IncP-5, IncP-8, IncP-10, IncP-11, IncP-12, IncP-13, and IncP-14 plasmids (Table S2-1).
Acinetobacter baumannii is known to be an important pathogen, and the plasmids in the genus Acinetobacter are considered to be key genetic factors in the spread of multi-drug resistance (Dijkshoorn et al., 2007; Evans and Amyes, 2014). Bertini et al. proposed PCR-based replicon typing of 19 groups (GRs) of resistance plasmids in A. baumannii on the basis of nucleotide sequence similarities between their replication initiation proteins (Bertini et al., 2010). Using the amino acid sequences of Rep from plasmids in the GR1 to GR19 groups, we were able to classify 78 of the 4602 plasmids found in the database, including 60 of the 90 plasmids in genus Acinetobacter (Table S1). The remaining 18 plasmids were also found in Gammaproteobacteria, and, notably, these plasmids were all classified into GR3 (Table S1). The average GC contents of plasmids classified into GR1-19 (38.4%) were lower than those of other plasmids in Gammaproteobacteria (Figure 3B). These findings indicate that the plasmids with GR1- to GR19-type Rep proteins were primarily distributed in Acinetobacter and that GR3 group plasmids may have a broader host range than plasmids in other GR groups.
Plasmids in Alphaproteobacteria
The majority of the 451 plasmids in Alphaproteobacteria were found in Rhizobiales (143 plasmids), Rhodospirillales (122 plasmids), Rhodobacteriales (94 plasmids), and Sphingomonadales (65 plasmids). Many plasmids in Alphaproteobacteria carry genes encoding RepABC proteins (Cevallos et al., 2008). The RepAB proteins are involved in the partitioning of the plasmid, and RepC is associated with replication initiation (Cevallos et al., 2008; Pinto et al., 2012). Indeed, 123 plasmids in Alphaproteobacteria had RepABC type genes (Table S1). Although plasmids containing these types of Rep genes have an average size of 484 kb, their sizes vary widely from 30 to 2430 kb (Figure 3C).
Hosts in the Rhizobiales are known to be symbiotic bacteria, and they can usually fix nitrogen only upon establishing a mutualistic interaction with plants, particularly those of the Leguminosae family (Cevallos et al., 2008; Pinto et al., 2012). Their 143 plasmids are characterized by a highly variable plasmid number (from 0 to 11) and size (from 150 to 1683 kb). Of these, 101 plasmids were classified into the RepABC type group (Table S1). Notably, most of the genes for nodulation and nitrogen fixation are located on a single plasmid, called the symbiotic plasmid (pSym). pSym contains the structural genes for nitrogenase (nifHDK) and/or genes essential to the production of the nodulation factor (notABC). Introduction of pSym into a plasmid-free A. tumefaciens strain leads to nodulation of a specific host and, sometimes, to nitrogen fixation, albeit at modest levels. Conversely, elimination of pSym impairs both the nodulation and nitrogen fixation capacities of the original bacterial strain (Torres et al., 2011). The presence of pSym is common to members of the fast-growing Rhizobium and Sinorhizobium species, including symbionts of many plants of agronomic interest. RepABC plasmids were also found in classes Rhodospirillales and Rhodobacteriales (Table S1) as previously reported (Petersen et al., 2011).
Approximately half of the plasmids in class Rhodosprillales were found in Acetobacter (60 of 122 plasmids; Table S1). The acetic acid bacteria are important for the industrial oxidation of various compounds, and thus, the construction of shuttle vector(s) to facilitate functional gene studies of these bacteria was reported 30 years ago (Fukaya et al., 1985; Okumura et al., 1985). Nevertheless, a well-defined method for the classification of Acetobacter plasmids has not been developed. Two groups have recently reported the characterization of Rep proteins, Rep7 and Rep20, from small plasmids pGR7 (Grones and Grones, 2012) and pAG20 (Babic et al., 2014), respectively, found in Acetobacter (class Rhodospirillales). The Rep20 gene was also found in plasmids in Rhodospirillales and Rhodobacteriales (Figure 3C). The average size of these plasmids was 4.6 kb, while that of RepABC-type plasmids was 483 kb (Figure 3C), suggesting that the newly characterized Rep proteins may be appropriate for the replication of small plasmids.
Several plasmids in Sphingomonadales have been found in bacteria which degrade biphenyl (pNL1 and pNL2 in Novosphingobium aromaticivorans DSM 12444; (Stillwell et al., 1995; Romine et al., 1999), gamma-hexachlorocyclohexane (pCHQ1, pUT1, and pUT2 in Sphingobium japonicum UT26S; (Nagata et al., 2006, 2010, 2011), and dibenzo-p-dioxin (pSWIT01 and pSWIT02 in Sphingomonas wittichii RW1; (Miller et al., 2010). The (putative) Rep proteins of these plasmids (pCHQ1, pUT1, pUT2, pSWIT01, pNL1, and pNL2) were found in 59 other plasmids in Alphaproteobacteria, and 24 plasmids were found in Sphingomonadales (Table S1). Notably, 42 plasmids in Alphaproteobacteria were classified as pUT1- or pUT2 type plasmids, and 36 of these were the pUT1 type (Figure 3C). The average size of the pUT-type plasmids was 68.4 kb, which was larger than Rep20 type plasmids and smaller than RepABC-type plasmids (Figure 3C). Most of the plasmids classified into these three Rep types were in Alphaproteobacteria (Table S1), whereas two pUT1-type plasmids and six RepABC-type plasmids were found in other classes or phyla (Table S1). Thus, they are important vehicles among Alphaproteobacteria, although the plasmids with smaller sizes and lower GC contents were still unable to be classified (Figure 3C).
Plasmids in Firmicutes
Plasmids found in Firmicutes were smaller (1.3–627 kb, 39 kb average) and had lower GC contents (23–63%, 34.4% average) than those in Proteobacteria (0.74–2580 kb, 103 kb average; 22–76%, 50.2% average; Figures 1B,C). Among the 1129 plasmids in Firmicutes, 1021 plasmids, or 90%, were found in the class Bacilli (Figure 4A). Based on comparisons with the amino acids sequences of (putative) Rep genes of the Inc groups of staphylococci, 475 plasmids in Firmicutes were shown to have putative Rep genes of Inc1 to Inc18 plasmids (Figure 4B), and 311 plasmids were from Bacilliales, including Bacillus (57 plasmids) and Staphylococcus (220 plasmids; Table S1). A subset of 158 plasmids classified into Inc1 to Inc18 groups was found in Lactobacillales, including Enterococcus (36 plasmids), Lactobacilllus (56 plasmids), Lactococcus (15 plasmids), and Streptococcus (34 plasmids; Table S1). The Rep genes of the Inc4, Inc8, Inc9, Inc10, Inc11, Inc13 and Inc14 group plasmids are known as rolling-circle replication plasmids (Smith and Thomas, 2004; Khan, 2005; Guglielmetti et al., 2007), while those of Inc1, Inc7, and Inc18 are theta-type replication plasmids (Bruand et al., 1991; Jensen et al., 2010b; Liu et al., 2013). Another classification system based on the nucleotide sequences of plasmids was proposed for enterococci and staphylococci, which included 26 Rep families and 10 unique families (Jensen et al., 2010a; Lozano et al., 2012). Of the 4602 plasmids included in our analysis, 388 plasmids could be classified using the 26 Rep families, and 383 of them were found in Firmicutes (Table S1). Several plasmids were classified both a Rep family and an Inc group, but 91 plasmids were only classified into the Rep family (Figure 4B). The distribution of plasmid size was bimodal, and the 269 putative rolling-circle replication plasmids had a smaller average size (5713 bp), while the average size of the 200 theta-type replication plasmids was larger (45,498 bp; Figure 4B, left). The GC contents of both groups were similar, 32.3% and 34.1%, respectively (Figure 4B, right).
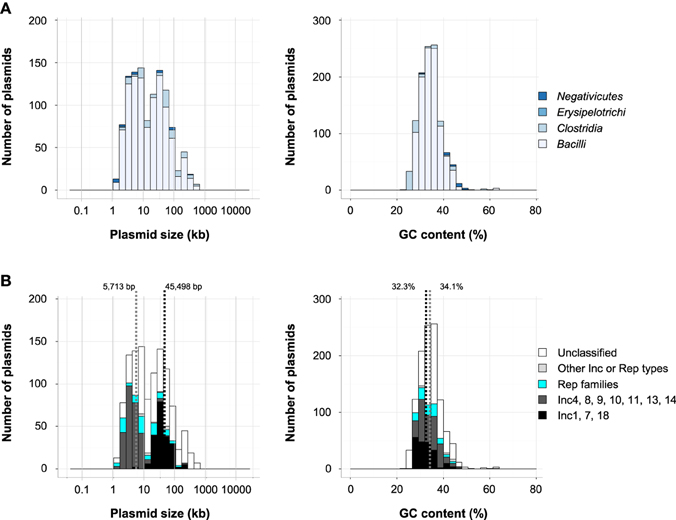
Figure 4. (A) Histograms of plasmid size (left) and GC content (right) of Firmicutes in each class. (B) Histograms of plasmid size (left) and GC content (right) in Bacilli. The distribution of classified plasmids is shown in black (Inc1, Inc7, and Inc18), dark gray (Inc4, Inc8, Inc9, Inc10, Inc11, Inc13, and Inc14), light blue (Rep families number 1–24 containing 7b, 10b), light gray (other Inc or Rep types) and white (unclassified). The average sizes and GC contents of groups Inc4, 8, 9, 10, 11, 13, 14 and those of Inc1, 7, 18 are represented by dotted lines.
Plasmids conferring resistance and virulence to Bacilli, including Enterococcus (Bacilliales) and Staphylococcus (Lactobacilliales), are causative agents of hospital infection outbreaks. The transmission of antibiotic resistance to Enterococcus is mediated by the pheromone-responsiveness and broad host range of Inc18 group plasmids (Palmer et al., 2010). The pheromone-responsive plasmids have mainly been described in Enterococcus faecalis. The representative examples of these plasmids are pCF10 and pAD1 (Clewell, 2007; Dunny, 2007). Their transfers are induced by pheromones generated from lipoprotein signal peptides encoded on the host chromosome (Clewell, 2007; Dunny, 2007). Pheromone receptors are encoded on the plasmid, and binding of the pheromone to its receptor causes an effective pair formation between donor and recipient cells. Rosvoll et al. have reported an in-depth investigation of Inc18 plasmids, which are found mostly in E. faecium (Rosvoll et al., 2010). Of these, only pAMβ1 and pIP501 have been well-characterized and were originally identified in E. faecalis and Streptococcus agalactiae, respectively. Within Staphylococcus, the pSK1 family (Inc1, Rep 20 family), which includes a multidrug-resistance plasmid like pSK4 and pSK7, and the pSK41 family (Inc7, Rep 15 family), including a conjugative multidrug-resistance plasmid like pGO1, have been investigated along with the previously discussed rolling-circle replicating plasmid (Jensen et al., 2010b; Liu et al., 2013).
The majority of the lactic acid bacteria (LAB) that belong to Bacilli, including Lactococcus, Lactobacillus, Leuconostoc, Pediococcus, and Streptococcus, as well as Bifidobacterium (phylum Actinobacteria), are important in food fermentation. Recently, the plasmids from Lactococcus lactis were reviewed in detail regarding plasmid-encoded traits of biotechnological significance (Ainsworth et al., 2014). Genetic engineering technology, including the development of cloning vectors for the LAB, is critical for the effective application of these bacteria in the food industry (Shareck et al., 2004). Versatile vectors have been developed for high-level, inducible gene expression in Lactobacillus sakei and Lactobacillus plantarum (Sorvig et al., 2005). In the GenBank database, 166 plasmids were found in Lactobacillus, and notably, multiple (five to ten) plasmids were found in individual strains, such as Lactobacillus fermentum MTCC 8711 (Jayashree et al., 2013), Lactobacillus plantarum 16 (Crowley et al., 2013), and Lactobacillus reuteri I5007 (Hou et al., 2014). Fukao et al. reported that one of the LAB, L. brevis KB290, has nine plasmids with RepABC systems (Fukao et al., 2013). Understanding how these plasmids could be maintained simultaneously in a single host cell will be important to the development of genetic tools for LAB.
Plasmids in Spirochaetes
Although 423 plasmids were found in Spirochaetes (Figure 1A), the majority of them were identified in genus Borrelia (416 out of 423), which is known to be an important pathogenic bacterium. The average GC contents of these plasmids were comparatively low (27.7%; Figure 1C). A unique feature of Borrelia is its segmented genome consisting of multiple circular and linear plasmids in addition to its linear chromosome within a single cell (Casjens et al., 2000). The features of Borrelia have been studied in detail (Chaconas and Kobryn, 2010; Chaconas and Norris, 2013). B. burgdorferi type strain B31, the causative agent of Lyme disease, possesses twelve linear plasmids and nine circular plasmids (Fraser et al., 1997; Casjens et al., 2000). Tilly et al. recently characterized the indispensable elements involved in the maintenance of cp26, a 26-kb circular plasmid found in B. burgdorferi (Tilly et al., 2012). Most plasmids can be lost without affecting the host growth; however, cp26 remains in all isolates of B. burgdorferi (Tilly et al., 2012). This plasmid carries genes required for survival of its host, including the resT gene, which encodes a telomere resolvase involved in the resolution of the replicated telomeres of the linear chromosome and plasmids in B. burgdorferi (Tilly et al., 2012).
Notably, several circular plasmids in B31 are homologous throughout almost their entire lengths (cp32s) and carry many genes encoding lipoproteins located on cell surfaces, although very few of these are metabolic or housekeeping genes (Casjens et al., 2000). Bunikis et al. proposed an efficient method to identify each plasmid based on multiplex PCR (Bunikis et al., 2011). The mechanisms used by the host strain to replicate and maintain similar plasmids have been studied in one of the cp32 plasmids (Eggers et al., 2002). There are still many interesting characteristics that require further investigation of plasmids in Spirochetes, and comparative genomic analyses of Borrelia in particular will reveal important information. Casjens et al. compared the genomic structures of plasmids identified in four different Borrelia strains (Casjens et al., 2012). They suggested that there are at least 28 plasmid compatibility types among the four strains and that several inter-plasmid genomic rearrangements may have occurred. More genomic information will be necessary for understanding the genetic evolution of these plasmids and their host chromosome.
Plasmids in Actinobacteria
All of the plasmids in the phylum Actinobacteria were found in class Actinobacteria, and, as shown in Figures 5A,B, they were found in nine suborders and one order (Bifidobacteriales). The plasmids in Actinobacteria were large and had high GC contents (Figures 1B,C). The phylum Actinobacteria includes a wide variety of bacteria with different morphologies, physiologies, and metabolic properties, and they have various kinds of plasmids (Ventura et al., 2007). In a review of the genomics of Actinobacteria, Ventura et al. showed the phylogenetic relationships between Rep proteins from actinobacterial plasmids (Ventura et al., 2007). In this review, sequences from 42 different types of Rep proteins from actinobacterial plasmids were used as queries, and of these proteins, 27 were found in more than two plasmids (Table S2-1). Of the 269 plasmids in Actinobacteria, 110 were found in Corynebacterineae, 69 in Streptomycineae, 32 in Micrococcineae, and 29 in Bifidobacteriales. The average sizes and GC contents of these plasmids varied widely (4631–200,330 bp; 56.1–70.3%; Figures 5A,B).
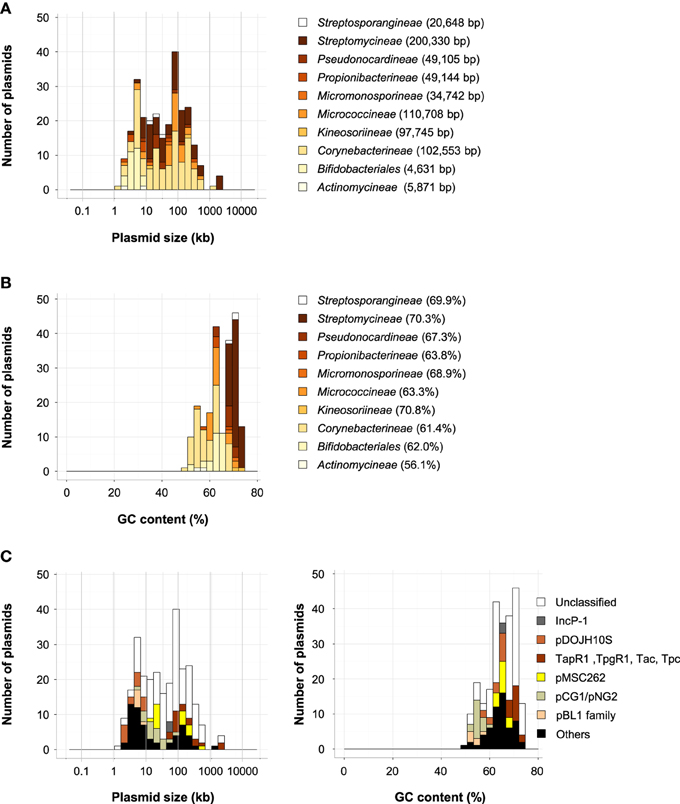
Figure 5. Histograms of plasmid size (A) and GC content (B) of Actinobacteria in each suborder and order (Bifidobacteriales). The average size of plasmids (A) and the average GC content (B) are shown in parentheses. (C) Histograms of plasmid size (left) and GC content (right) in phylum Actinobacteria. The distribution of classified plasmids is shown in beige (pBL1 family), spring green (pCG1/pNG2 family), yellow (pMSC262 family), brown (plasmids with TapR1, TpgR1 Tac, and/or Tpc), light brown (pDOJH10S-type), and gray (IncP-1 plasmids), black (other Rep types), and white (unclassified plasmids).
The majority of the plasmids from Corynebacterineae were found in Corynebacterium (38 plasmids), Mycobacterium (29 plasmids), and Rhodococcus (31 plasmids). Because C. glutamicum is widely used as an industrial producer of amino acids, plasmids of the amino acid-producing isolates were classified to develop efficient recombinant DNA techniques (Tauch et al., 2003a). Several rolling-circle replication plasmids were found in C. glutamicum, and the pBL1 (including pAG3 and pCG2) and pCG1 (including pCG4 and pGA2) families were proposed (Tauch et al., 2003a). A plasmid found in the human pathogen C. diphtheria S601, pNG2, has a Rep protein similar to those of the pCG1 family (Tauch et al., 2003a,b).
There were six pBL1-family plasmids and 22 pCG1/pNG2 plasmids in the GenBank database (Table S1). While plasmids in the pBL1 family had small sizes (4.4–6.8 kb), those in the pCG1/pNG2 family had a wide range of sizes (4.1–85 kb, Figure 5C). Tauch et al. also reported a theta-type replication plasmid family in Corynebacterium, the pCRY4 family, but among the 4602 plasmids analyzed, only one plasmid was classified into this family (Table S1). Of the plasmids found in Mycobacterium, only the low copy number plasmid pAL5000 has been widely used (Labidi et al., 1985). Plasmids in the pMSC262 family are compatible with pAL5000, including pVT2, pMUM001, and linear plasmid pCLP (Le Dantec et al., 2001), which had a wide range of sizes (24–615 kb, Figure 5C). Among the 29 plasmids of Mycobacterium, 16 plasmids were classified into the pMSC262 family (Table S1). Plasmids in Rhodococcus were found in the biphenyl degrader, R. jostii RHA1 (Seto et al., 1995). This strain has three plasmids, pRHL1, pRHL2, and pRHL3 (Masai et al., 1997; Shimizu et al., 2001; McLeod et al., 2006). The R. erythropolis PR4 strain is known to utilize n-alkanes and alkylbenzene, and it has three plasmids, pREL1, pREC1, and pREC2 (Sekine et al., 2006). Only a small number of plasmids in Rhodococcus were predicted to have Rep proteins similar to those of pRHL1, pRHL3, pREC1, or pREC2 (Table S1).
Plasmids in Streptomycieae are found in Streptomyces species, and several of them are large linear plasmids. These linear plasmids have conserved “telomeres” containing inverted repeat sequences (Chen et al., 1993; Pandza et al., 1998). The 5′ telomeric ends are blocked by covalently attached telomere terminal proteins (Bao and Cohen, 2001). They have sets of conserved telomere replication genes known as tpgR1 and tapR1 or tpc and tac (Bao and Cohen, 2001, 2003). In Streptomyces, 13 plasmids, all linear, carry these telomere replication gene sets (Table S1). Their sizes were relatively large (54–1797 kb, Figure 5C) and their GC contents were high (68.3–71.9%, Figure 5C). A rolling circle replication plasmid, pIJ101, from S. lividans has been used as a cloning vector in Streptomyces (Kendall and Cohen, 1988; Ventura et al., 2007), but only two other plasmids have been found to carry a similar Rep gene (Table S1).
Plasmids in Bifidobacteriales were small, with an average size of 4.6 kb (Figure 5A). Among the 29 plasmids in Bifidobacteriales, 28 of them were classified into the known Rep types pKJ50 (Park et al., 1999), pNAC2, pNAC3 (Corneau et al., 2004), and pDOJH10S (Lee and O'Sullivan, 2006). Rep proteins of pKJ50, pNAC2, and pNAC3 were found only in Bifidobacterium; however, those of pDOJH10S were found in plasmids of other suborders of Actinobacteria, including Corynebacterineae, Micrococcinaea, and Propionibacterineae (Table S1). It is likely that plasmids with Rep types of pDOJH10S have a broad host range in the phylum Actinobacteria.
Plasmids in Cyanobacteria
Cyanobacteria are known for the ability to undergo oxygenic photosynthesis and are a promising platform for the production of renewable chemicals and fuels. Thus, a plasmid vector to introduce exogenous genes into cyanobacterial cells has been developed. Taton et al. have developed a vector system for plasmids with a broad host range using the previously known plasmid origin of Cyanobacteria (http://golden.ucsd.edu/CyanoVECTOR/) (Taton et al., 2014). The origins of plasmids were from pDU1 in Nostoc sp. PCC 7524 (Anabaena sp. PCC 7120; Walton et al., 1992), pANS (pUH24) in Synechococus elongatus PCC 7942 (Golden and Sherman, 1983), pDC1 in Nostoc sp. PCC 8009 (Nostoc sp. ATCC 29133; Lambert and Carr, 1983), and pFDA in Fremyella diplosiphon PCC 7601 (Cobley et al., 1993). Although vectors containing these origins could be a useful module to construct new vectors for Cyanobacteria, the homologous rep genes were not found in other plasmids in our database. This finding indicates that Cyanobacteria may contain a wider variety of plasmids than other phyla. Smillie et al. (2010) proposed that cyanobacteria may use an as-yet-uncharacterized system to conjugate because, within the phylum, the plasmids encoding relaxase and/or T4CP did not possess known T4SS or VirB4 (Table S1). Indeed, plasmid transfer from Proteobacteria to Cyanobacteria has been previously reported (Encinas et al., 2014). Further in-depth analyses and classification of Cyanobacteria plasmids are necessary to understand the spread of plasmids in this phylum.
Plasmids in Archaea
Among the 4602 plasmids in the database, 137 plasmids were found in Archaea: 112 plasmids were in Euryarcheoata (Figure 1A) and 23 plasmids were in Crenarchaeota. There were large differences in the average sizes and GC contents between the two phyla. The plasmids in Euryarcheoata had an average size of 119 kb and GC content of 52.1%, while those in Crenarchaeota were 20 kb and 39.2%, respectively. Euryarcheoata includes all methanogens, haloarchaea, and some hyperthermophilic genera such as Pyrococcus and Thermococcus. Most known archaeal plasmids have cryptic phenotypes. The best characterized plasmids among methanogens are pME2001, pURB500, and pC2A (Greve et al., 2004). The putative Rep genes have been reported for pME2001 (Luo et al., 2012) and pURB500 (Tumbula et al., 1997), although multiple regions (or genes) were required for replication of pURB500. The putative gene involved in plasmid replication identified in pC2A showed limited homology with Rep proteins encoded by rolling-circle plasmids (del Solar et al., 1993; Metcalf et al., 1997). In Thermococcales, there are two well-characterized plasmids, pGT5 from Pyrococcus abyssi and pTN1 from Thermococcus nautilus, and their respective replication initiation proteins have been identified. Both pGT5 and pTN1 have been shown to use the rolling circle replication system (Arnold et al., 1999; Soler et al., 2007). The majority of plasmids in Crenarchaeota were found in Sulfolobales plasmids (21/23 plasmids), and they were classified into pRN-type and pNOB-type groups (Greve et al., 2004). Joshua et al. have reported the functional characterization of pRN1 (Joshua et al., 2013). A pRN-type plasmid, pSSVx, is known to be a virus-plasmid hybrid that coexists intracellularly with the fusellovirus SSV1 and can be packaged into viral particles (Arnold et al., 1999). The pNOB8-type plasmids are larger in size (around 30 kb) than pRN-type plasmids (less than 10 kb) and are known to be conjugative (She et al., 1998). The putative Rep gene for the pNOB8-type group has not been identified (Greve et al., 2004). Based on the TBLASTN analysis, these Rep genes of pRUB500, pME2001, pC2A, pGT5, pTN1, and pRN1 were found in only 15 plasmids of Archaea (Tables S1 and S2), suggesting that a wider variety of plasmids may exist in this kingdom than in others.
Genetic tools used in the analysis of archaea are currently being developed. Many archaeal species are resistant to conventional antibiotics, a factor that limits the use of genetic manipulation in archaea (Atomi et al., 2012). Several shuttle vectors are available for certain genera within Euryarcheoata, such as halophiles (Haloarcula), methanogens (Methaonococcus and Methonosarcina), and Thermococcales (Pyrococcus). However, those specific to Crenarcheota are only useful for the genus Sulfolobales. Methanogens are known to generate methane and were the first microorganisms identified as Archaea. Halophiles are the Archaea living in the most saline environments on the earth, while Thermococcales are found in high temperature environments, mostly above 80°C (Heuer and Smalla, 2012). Sulfolobales are the only members of Crenarcheota whose genetic manipulation methods have been established thus far (Leigh et al., 2011; Atomi et al., 2012). The plasmids of Archaea are typically introduced by transformation; conjugative transfer has not been reported with the exception of a small number of plasmids in Sulfolobus (Greve et al., 2004; Smillie et al., 2010).
Recent Work to Identify other Environmental Plasmids
A large number of plasmids have been identified by chance during the analysis of the host bacteria based on specific phenotypes. Indeed, in our recent report of the whole genome sequence of a biphenyl-degrading bacterium, Geobacillus sp. JF8, we showed that it carried a plasmid, pBt40, with biphenyl-degradative genes (Shintani et al., 2014b). The putative Rep gene of pBt40 was identified, and its homologs were recently found in plasmids isolated from other strains within the genus Geobacillus, although detailed characterizations of these plasmids have not been performed (Table S1). Considering that most environmental bacteria cannot be cultivated, culture-dependent methods to detect transconjugants of plasmids are known to be highly biased. Smalla and Sobecky (2002) proposed multiphasic approaches for the characterization of plasmids and other mobile genetic elements (Smalla and Sobecky, 2002). Culture-independent methods have recently been developed to isolate and identify novel types of plasmids and to detect plasmid transfers (Heuer and Smalla, 2012).
Methods for Plasmid Isolation and Identification
Notably, 28 plasmids in the database have been identified by culture-independent methods from unidentified host strains or uncultivated microbes (Table S1). PCR using plasmid-specific sequences is one of the most common methods used to detect plasmids in environmental samples. Various primers and PCR methods to identify plasmids have been designed, such as those for Enterobacteriaceae (Carattoli et al., 2005), enterococci (Rosvoll et al., 2010), and other Gram-positive bacteria, mostly staphylococci (Clewell, 2007; Jensen et al., 2010a; Lozano et al., 2012). Methods of direct detection by PCR using degenerate primers have been reported for IncP-1, IncP-7, and IncP-9 plasmids from environmental samples (Dealtry et al., 2014) and for plasmids of different MOB families (Alvarado et al., 2012). Many plasmids have been identified from environmental samples, including the human gut, by metagenomic analyses (Elsaied et al., 2011; Kristiansson et al., 2011; Zhang et al., 2011; Brolund et al., 2013; Song et al., 2013). Brown Kav et al. characterized the overall plasmid population in the bovine rumen (termed rumen plasmidomes) by sequencing the extracted circular plasmids (Brown Kav et al., 2012, 2013). They developed a bioinformatics pipeline that could successfully detect low abundance plasmids and remove contamination of chromosomal DNA (Brown Kav et al., 2012). Sentchilo et al. compared the plasmid metagenomes from two separate activated sludge systems and found that the plasmids from the two environments were strongly different (Sentchilo et al., 2013). Jorgensen et al. reported an in silico procedure for identifying small plasmids in metagenomics datasets of the rat cecum (Jorgensen et al., 2014). They successfully identified 160 circular sequences carrying a gene with a plasmid replication domain, and the majority of these sequences were novel (Jorgensen et al., 2014).
Exogenous plasmid isolation is a culture-independent method used to capture plasmids directly from microbial communities. This method is based on the ability of the plasmid mobility and replication in a recipient. Transconjugants are selected by their phenotypes, such as antibiotic resistances, conferred by plasmid carriage. This method enables researchers to search for plasmids in a wider microcosm containing the uncultivated microbial fraction (Heuer et al., 2012). Therefore, the potential to obtain novel types of plasmids is higher. Indeed, this method has been used to isolate a number of novel plasmids (Miyazaki et al., 2006; Sobecky and Hazen, 2009; Sen et al., 2011; Eikmeyer et al., 2012; Brown et al., 2013; Oliveira et al., 2013; Norberg et al., 2014). A transposon-aided capture (TRACA) method has been developed to study and isolate plasmids independent of plasmid-encoded traits in various bacterial habitats (Jones and Marchesi, 2007). This method has also facilitated the successful identification of several novel types of plasmids (Jones and Marchesi, 2007; Zhang et al., 2011; Burmolle et al., 2012).
Methods to Detect Plasmid Transfer
There are multiple reviews of the conventional methods to detect plasmid transfer in various environments based on culture-dependent approaches (Smalla and Sobecky, 2002; Heuer and Smalla, 2007, 2012; Shintani et al., 2010). Systems able to directly detect plasmid transfers by culture-independent methods have been developed using fluorescent protein markers, such as GFP, DsRed, mCherry, GusA,LuxAB, with antibiotic resistance genes (Amann and Fuchs, 2008). Fluorescence in situ hybridization (FISH) using specific probes targeting 16S rRNA sequences and in situ PCR in transconjugant cells are able to detect transconjugants at the single-cell level (Amann and Fuchs, 2008; Cenciarini-Borde et al., 2009; Wagner and Haider, 2012). Fluorescent cells are also detectable and separable with the use of flow cytometry or a micromanipulator at the single cell level; both methods have a strong potential to identify actual host ranges of plasmids (Musovic et al., 2010; Shintani et al., 2014a).
In silico analyses have been developed to identify horizontal gene transfer phenomena including plasmid transfer. Yamashita et al. carried out a plasmidome network analysis of all available complete bacterial plasmids to identify and characterize the most recent horizontal gene transfer or plasmid transfer (Yamashita et al., 2014). de Been et al. reported a novel approach for the reconstruction of mobile genetic elements from whole-genome sequence data, with which they discovered specific plasmid lineages shared between farm animals and humans (de Been et al., 2014).
Over the next decade, the number of fully-sequenced plasmids will greatly increase through the use of deep sequencing methods and in silico analyses of datasets. The classification methods using Rep genes and mobility types based on their nucleotide sequences are useful to identify newly isolated plasmids. In this review, it is discussed genes for replication and conjugative transfer, but not the other gene(s) on plasmids including genes for resistance to antibiotics, metabolism of natural and synthetic compounds, pathogenicity, and host symbiosis. The classification lists in this review will be helpful to understand how these “other” genes on plasmids could be spread among microbes. The classification will be also of help to predict host candidates of plasmids found by a metagenomics approach, rather than mostly a collection of results obtained.
Considering that less than half of the plasmids in the database were able to be classified, there may still be unknown or novel types of plasmid replication or transfer systems in microbes. Therefore, characterizations of plasmid features involved in replication, maintenance, transfer, and host range based on molecular biological and biochemical methods are still necessary. Culture-independent methods to detect plasmids and their transfers are currently available for analyzing non-culturable or uncultivated microbes. These studies will not only enable us to understand the evolution of microbes mediated by plasmid transfers but will also provide us with useful tools for genetic analyses of both culturable and non-culturable microbes.
Conflict of Interest Statement
The authors declare that the research was conducted in the absence of any commercial or financial relationships that could be construed as a potential conflict of interest.
Acknowledgments
This work was partially supported by JSPS KAKENHI grant number 24780087 to MS.
Supplementary Material
The Supplementary Material for this article can be found online at: http://www.frontiersin.org/journal/10.3389/fmicb.2015.00242/abstract
References
Adamczyk, M., and Jagura-Burdzy, G. (2003). Spread and survival of promiscuous IncP-1 plasmids. Acta Biochim. Pol. 50, 425–453.
Ainsworth, S., Stockdale, S., Bottacini, F., Mahony, J., and van Sinderen, D. (2014). The Lactococcus lactis plasmidome: much learnt, yet still lots to discover. FEMS Microbiol. Rev. 38, 1066–1088. doi: 10.1111/1574-6976.12074
PubMed Abstract | Full Text | CrossRef Full Text | Google Scholar
Alvarado, A., Garcillan-Barcia, M. P., and de la Cruz, F. (2012). A degenerate primer MOB typing (DPMT) method to classify gamma-proteobacterial plasmids in clinical and environmental settings. PLoS ONE 7:e40438. doi: 10.1371/journal.pone.0040438
PubMed Abstract | Full Text | CrossRef Full Text | Google Scholar
Amann, R., and Fuchs, B. M. (2008). Single-cell identification in microbial communities by improved fluorescence in situ hybridization techniques. Nat. Rev. Microbiol. 6, 339–348. doi: 10.1038/nrmicro1888
PubMed Abstract | Full Text | CrossRef Full Text | Google Scholar
Aminov, R. I. (2011). Horizontal gene exchange in environmental microbiota. Front. Microbiol. 2:158. doi: 10.3389/fmicb.2011.00158
PubMed Abstract | Full Text | CrossRef Full Text | Google Scholar
Arnold, H. P., She, Q., Phan, H., Stedman, K., Prangishvili, D., Holz, I., et al. (1999). The genetic element pSSVx of the extremely thermophilic crenarchaeon Sulfolobus is a hybrid between a plasmid and a virus. Mol. Microbiol. 34, 217–226. doi: 10.1046/j.1365-2958.1999.01573.x
PubMed Abstract | Full Text | CrossRef Full Text | Google Scholar
Atomi, H., Imanaka, T., and Fukui, T. (2012). Overview of the genetic tools in the Archaea. Front. Microbiol. 3:337. doi: 10.3389/fmicb.2012.00337
PubMed Abstract | Full Text | CrossRef Full Text | Google Scholar
Babic, M., Reskova, Z., Bugala, J., Cimova, V., Grones, P., and Grones, J. (2014). The Rep20 replication initiator from the pAG20 plasmid of Acetobacter aceti. Mol. Biotechnol. 56, 1–11. doi: 10.1007/s12033-013-9680-6
PubMed Abstract | Full Text | CrossRef Full Text | Google Scholar
Bao, K., and Cohen, S. N. (2001). Terminal proteins essential for the replication of linear plasmids and chromosomes in Streptomyces. Genes Dev. 15, 1518–1527. doi: 10.1101/gad.896201
PubMed Abstract | Full Text | CrossRef Full Text | Google Scholar
Bao, K., and Cohen, S. N. (2003). Recruitment of terminal protein to the ends of Streptomyces linear plasmids and chromosomes by a novel telomere-binding protein essential for linear DNA replication. Genes Dev. 17, 774–785. doi: 10.1101/gad.1060303
PubMed Abstract | Full Text | CrossRef Full Text | Google Scholar
Bertini, A., Poirel, L., Mugnier, P. D., Villa, L., Nordmann, P., and Carattoli, A. (2010). Characterization and PCR-based replicon typing of resistance plasmids in Acinetobacter baumannii. Antimicrob. Agents Chemother. 54, 4168–4177. doi: 10.1128/AAC.00542-10
PubMed Abstract | Full Text | CrossRef Full Text | Google Scholar
Brantl, S. (2004). “Plasmid replication control by antisense RNA,” in Plasmid Biology, eds G. Phillips and B. Funnell (Washington, DC: ASM Press), 47–62.
Brolund, A., Franzen, O., Melefors, O., Tegmark-Wisell, K., and Sandegren, L. (2013). Plasmidome-analysis of ESBL-producing Escherichia coli using conventional typing and high-throughput sequencing. PLoS ONE 8:e65793. doi: 10.1371/journal.pone.0065793
PubMed Abstract | Full Text | CrossRef Full Text | Google Scholar
Brown, C. J., Sen, D., Yano, H., Bauer, M. L., Rogers, L. M., Van der Auwera, G. A., et al. (2013). Diverse broad-host-range plasmids from freshwater carry few accessory genes. Appl. Environ. Microbiol. 79, 7684–7695. doi: 10.1128/AEM.02252-13
PubMed Abstract | Full Text | CrossRef Full Text | Google Scholar
Brown Kav, A., Benhar, I., and Mizrahi, I. (2013). A method for purifying high quality and high yield plasmid DNA for metagenomic and deep sequencing approaches. J. Microbiol. Methods 95, 272–279. doi: 10.1016/j.mimet.2013.09.008
PubMed Abstract | Full Text | CrossRef Full Text | Google Scholar
Brown Kav, A., Sasson, G., Jami, E., Doron-Faigenboim, A., Benhar, I., and Mizrahi, I. (2012). Insights into the bovine rumen plasmidome. Proc. Natl. Acad. Sci. U.S.A. 109, 5452–5457. doi: 10.1073/pnas.1116410109
PubMed Abstract | Full Text | CrossRef Full Text | Google Scholar
Bruand, C., Ehrlich, S. D., and Janniere, L. (1991). Unidirectional theta replication of the structurally stable Enterococcus faecalis plasmid pAM beta 1. EMBO J. 10, 2171–2177.
Bunikis, I., Kutschan-Bunikis, S., Bonde, M., and Bergström, S. (2011). Multiplex PCR as a tool for validating plasmid content of Borrelia burgdorferi. J. Microbiol. Methods 86, 243–247. doi: 10.1016/j.mimet.2011.05.004
PubMed Abstract | Full Text | CrossRef Full Text | Google Scholar
Burmolle, M., Norman, A., Sorensen, S. J., and Hansen, L. H. (2012). Sequencing of IncX-plasmids suggests ubiquity of mobile forms of a biofilm-promoting gene cassette recruited from Klebsiella pneumoniae. PLoS ONE 7:e41259. doi: 10.1371/journal.pone.0041259
PubMed Abstract | Full Text | CrossRef Full Text | Google Scholar
Carattoli, A. (2009). Resistance plasmid families in Enterobacteriaceae. Antimicrob. Agents Chemother. 53, 2227–2238. doi: 10.1128/AAC.01707-08
PubMed Abstract | Full Text | CrossRef Full Text | Google Scholar
Carattoli, A., Bertini, A., Villa, L., Falbo, V., Hopkins, K. L., and Threlfall, E. J. (2005). Identification of plasmids by PCR-based replicon typing. J. Microbiol. Methods 63, 219–228. doi: 10.1016/j.mimet.2005.03.018
PubMed Abstract | Full Text | CrossRef Full Text | Google Scholar
Casjens, S., Palmer, N., Van Vugt, R., Huang, W. M., Stevenson, B., Rosa, P., et al. (2000). A bacterial genome in flux: the twelve linear and nine circular extrachromosomal DNAs in an infectious isolate of the Lyme disease spirochete Borrelia burgdorferi. Mol. Microbiol. 35, 490–516. doi: 10.1046/j.1365-2958.2000.01698.x
PubMed Abstract | Full Text | CrossRef Full Text | Google Scholar
Casjens, S. R., Mongodin, E. F., Qiu, W. G., Luft, B. J., Schutzer, S. E., Gilcrease, E. B., et al. (2012). Genome stability of Lyme disease spirochetes: comparative genomics of Borrelia burgdorferi plasmids. PLoS ONE 7:e33280. doi: 10.1371/journal.pone.0033280
PubMed Abstract | Full Text | CrossRef Full Text | Google Scholar
Cenciarini-Borde, C., Courtois, S., and La Scola, B. (2009). Nucleic acids as viability markers for bacteria detection using molecular tools. Future Microbiol. 4, 45–64. doi: 10.2217/17460913.4.1.45
PubMed Abstract | Full Text | CrossRef Full Text | Google Scholar
Cesareni, G., Helmer-Citterich, M., and Castagnoli, L. (1991). Control of ColE1 plasmid replication by antisense RNA. Trends Genet. 7, 230–235. doi: 10.1016/0168-9525(91)90143-E
PubMed Abstract | Full Text | CrossRef Full Text | Google Scholar
Cevallos, M. A., Cervantes-Rivera, R., and Gutierrez-Rios, R. M. (2008). The repABC plasmid family. Plasmid 60, 19–37. doi: 10.1016/j.plasmid.2008.03.001
PubMed Abstract | Full Text | CrossRef Full Text | Google Scholar
Chaconas, G., and Kobryn, K. (2010). Structure, function, and evolution of linear replicons in Borrelia. Annu. Rev. Microbiol. 64, 185–202. doi: 10.1146/annurev.micro.112408.134037
PubMed Abstract | Full Text | CrossRef Full Text | Google Scholar
Chaconas, G., and Norris, S. J. (2013). Peaceful coexistence amongst Borrelia plasmids: getting by with a little help from their friends? Plasmid 70, 161–167. doi: 10.1016/j.plasmid.2013.05.002
PubMed Abstract | Full Text | CrossRef Full Text | Google Scholar
Chen, C. W., Yu, T. W., Lin, Y. S., Kieser, H. M., and Hopwood, D. A. (1993). The conjugative plasmid SLP2 of Streptomyces lividans is a 50 kb linear molecule. Mol. Microbiol. 7, 925–932. doi: 10.1111/j.1365-2958.1993.tb01183.x
PubMed Abstract | Full Text | CrossRef Full Text | Google Scholar
Clewell, D. B. (2007). Properties of Enterococcus faecalis plasmid pAD1, a member of a widely disseminated family of pheromone-responding, conjugative, virulence elements encoding cytolysin. Plasmid 58, 205–227. doi: 10.1016/j.plasmid.2007.05.001
PubMed Abstract | Full Text | CrossRef Full Text | Google Scholar
Cobley, J. G., Zerweck, E., Reyes, R., Mody, A., Seludo-Unson, J. R., Jaeger, H., et al. (1993). Construction of shuttle plasmids which can be efficiently mobilized from Escherichia coli into the chromatically adapting cyanobacterium, Fremyella diplosiphon. Plasmid 30, 90–105. doi: 10.1006/plas.1993.1037
PubMed Abstract | Full Text | CrossRef Full Text | Google Scholar
Corneau, N., Emond, E., and Lapointe, G. (2004). Molecular characterization of three plasmids from Bifidobacterium longum. Plasmid 51, 87–100. doi: 10.1016/j.plasmid.2003.12.003
PubMed Abstract | Full Text | CrossRef Full Text | Google Scholar
Crowley, S., Bottacini, F., Mahony, J., and van Sinderen, D. (2013). Complete genome sequence of Lactobacillus plantarum strain 16, a broad-spectrum antifungal-producing lactic acid bacterium. Genome Announc. 1, e00533–e00513. doi: 10.1128/genomeA.00533-13
PubMed Abstract | Full Text | CrossRef Full Text | Google Scholar
Dealtry, S., Holmsgaard, P. N., Dunon, V., Jechalke, S., Ding, G. C., Krogerrecklenfort, E., et al. (2014). Shifts in abundance and diversity of mobile genetic elements after the introduction of diverse pesticides into an on-farm biopurification system over the course of a year. Appl. Environ. Microbiol. 80, 4012–4020. doi: 10.1128/AEM.04016-13
PubMed Abstract | Full Text | CrossRef Full Text | Google Scholar
de Been, M., Lanza, V. F., de Toro, M., Scharringa, J., Dohmen, W., Du, Y., et al. (2014). Dissemination of cephalosporin resistance genes between Escherichia coli strains from farm animals and humans by specific plasmid lineages. PLoS Genet. 10:e1004776. doi: 10.1371/journal.pgen.1004776
PubMed Abstract | Full Text | CrossRef Full Text | Google Scholar
del Solar, G., Giraldo, R., Ruiz-Echevarria, M. J., Espinosa, M., and Diaz-Orejas, R. (1998). Replication and control of circular bacterial plasmids. Microbiol. Mol. Biol. Rev. 62, 434–464.
del Solar, G., Moscoso, M., and Espinosa, M. (1993). Rolling circle-replicating plasmids from gram-positive and gram-negative bacteria: a wall falls. Mol. Microbiol. 8, 789–796. doi: 10.1111/j.1365-2958.1993.tb01625.x
PubMed Abstract | Full Text | CrossRef Full Text | Google Scholar
Dijkshoorn, L., Nemec, A., and Seifert, H. (2007). An increasing threat in hospitals: multidrug-resistant Acinetobacter baumannii. Nat. Rev. Microbiol. 5, 939–951. doi: 10.1038/nrmicro1789
PubMed Abstract | Full Text | CrossRef Full Text | Google Scholar
Dunny, G. M. (2007). The peptide pheromone-inducible conjugation system of Enterococcus faecalis plasmid pCF10: cell-cell signalling, gene transfer, complexity and evolution. Philos. Trans. R. Soc. Lond. B Biol. Sci. 362, 1185–1193. doi: 10.1098/rstb.2007.2043
PubMed Abstract | Full Text | CrossRef Full Text | Google Scholar
Eggers, C. H., Caimano, M. J., Clawson, M. L., Miller, W. G., Samuels, D. S., and Radolf, J. D. (2002). Identification of loci critical for replication and compatibility of a Borrelia burgdorferi cp32 plasmid and use of a cp32-based shuttle vector for the expression of fluorescent reporters in the lyme disease spirochaete. Mol. Microbiol. 43, 281–295. doi: 10.1046/j.1365-2958.2002.02758.x
PubMed Abstract | Full Text | CrossRef Full Text | Google Scholar
Eikmeyer, F., Hadiati, A., Szczepanowski, R., Wibberg, D., Schneiker-Bekel, S., Rogers, L. M., et al. (2012). The complete genome sequences of four new IncN plasmids from wastewater treatment plant effluent provide new insights into IncN plasmid diversity and evolution. Plasmid 68, 13–24. doi: 10.1016/j.plasmid.2012.01.011
PubMed Abstract | Full Text | CrossRef Full Text | Google Scholar
Elsaied, H., Stokes, H. W., Kitamura, K., Kurusu, Y., Kamagata, Y., and Maruyama, A. (2011). Marine integrons containing novel integrase genes, attachment sites, attI, and associated gene cassettes in polluted sediments from Suez and Tokyo Bays. ISME J. 5, 1162–1177. doi: 10.1038/ismej.2010.208
PubMed Abstract | Full Text | CrossRef Full Text | Google Scholar
Encinas, D., Garcillan-Barcia, M. P., Santos-Merino, M., Delaye, L., Moya, A., and de la Cruz, F. (2014). Plasmid conjugation from proteobacteria as evidence for the origin of xenologous genes in cyanobacteria. J. Bacteriol. 196, 1551–1559. doi: 10.1128/JB.01464-13
PubMed Abstract | Full Text | CrossRef Full Text | Google Scholar
Espinosa, M., Cohen, S., Couturier, M., Del Solar, G., Diaz-Orejas, R., Giraldo, R., et al. (2000). “Plasmid replication and copy number control,” in The Horizontal Gene Pool: Bacterial Plasmids and Gene Spread, ed C. M. Thomas (Amsterdam: Harwood Academic Publishers), 1–47.
Evans, B. A., and Amyes, S. G. (2014). OXA beta-lactamases. Clin. Microbiol. Rev. 27, 241–263. doi: 10.1128/CMR.00117-13
PubMed Abstract | Full Text | CrossRef Full Text | Google Scholar
Fraser, C. M., Casjens, S., Huang, W. M., Sutton, G. G., Clayton, R., Lathigra, R., et al. (1997). Genomic sequence of a Lyme disease spirochaete, Borrelia burgdorferi. Nature 390, 580–586. doi: 10.1038/37551
PubMed Abstract | Full Text | CrossRef Full Text | Google Scholar
Frost, L., Leplae, R., Summers, A., and Toussaint, A. (2005). Mobile genetic elements: the agents of open source evolution. Nat. Rev. Microbiol. 3, 722–732. doi: 10.1038/nrmicro1235
PubMed Abstract | Full Text | CrossRef Full Text | Google Scholar
Frost, L. S., and Koraimann, G. (2010). Regulation of bacterial conjugation: balancing opportunity with adversity. Future Microbiol. 5, 1057–1071. doi: 10.2217/fmb.10.70
PubMed Abstract | Full Text | CrossRef Full Text | Google Scholar
Fukao, M., Oshima, K., Morita, H., Toh, H., Suda, W., Kim, S. W., et al. (2013). Genomic analysis by deep sequencing of the probiotic Lactobacillus brevis KB290 harboring nine plasmids reveals genomic stability. PLoS ONE 8:e60521. doi: 10.1371/journal.pone.0060521
PubMed Abstract | Full Text | CrossRef Full Text | Google Scholar
Fukaya, M., Okumura, H., Masai, H., Uozumi, T., and Beppu, T. (1985). Construction of new shuttle vectors for Acetobacter. Agric. Biol. Chem. 49, 2083–2090. doi: 10.1271/bbb1961.49.2083
Funnell, B., and Phillips, G. (2004). “Preface,” in Plasmid Biology, eds B. Funnell and G. Phillips (Washington, DC: ASM Press), xi.
Garcillán-Barcia, M. P., Alvarado, A., and de la Cruz, F. (2011). Identification of bacterial plasmids based on mobility and plasmid population biology. FEMS Microbiol. Rev. 35, 936–956. doi: 10.1111/j.1574-6976.2011.00291.x
PubMed Abstract | Full Text | CrossRef Full Text | Google Scholar
Garcillán-Barcia, M. P., Francia, M. V., and de la Cruz, F. (2009). The diversity of conjugative relaxases and its application in plasmid classification. FEMS Microbiol. Rev. 33, 657–687. doi: 10.1111/j.1574-6976.2009.00168.x
PubMed Abstract | Full Text | CrossRef Full Text | Google Scholar
Giraldo, R., and Fernandez-Tresguerres, M. E. (2004). Twenty years of the pPS10 replicon: insights on the molecular mechanism for the activation of DNA replication in iteron-containing bacterial plasmids. Plasmid 52, 69–83. doi: 10.1016/j.plasmid.2004.06.002
PubMed Abstract | Full Text | CrossRef Full Text | Google Scholar
Goessweiner-Mohr, N., Arends, K., Keller, W., and Grohmann, E. (2013). Conjugative type IV secretion systems in Gram-positive bacteria. Plasmid 70, 289–302. doi: 10.1016/j.plasmid.2013.09.005
PubMed Abstract | Full Text | CrossRef Full Text | Google Scholar
Golden, S. S., and Sherman, L. A. (1983). A hybrid plasmid is a stable cloning vector for the cyanobacterium Anacystis nidulans R2. J. Bacteriol. 155, 966–972.
Greve, B., Jensen, S., Brügger, K., Zillig, W., and Garrett, R. A. (2004). Genomic comparison of archaeal conjugative plasmids from Sulfolobus. Archaea 1, 231–239. doi: 10.1155/2004/151926
PubMed Abstract | Full Text | CrossRef Full Text | Google Scholar
Grones, P., and Grones, J. (2012). Characterization of the theta replication plasmid pGR7 from Acetobacter aceti CCM 3610. Res. Microbiol. 163, 419–426. doi: 10.1016/j.resmic.2012.07.002
PubMed Abstract | Full Text | CrossRef Full Text | Google Scholar
Guglielmetti, S., Mora, D., and Parini, C. (2007). Small rolling circle plasmids in Bacillus subtilis and related species: organization, distribution, and their possible role in host physiology. Plasmid 57, 245–264. doi: 10.1016/j.plasmid.2006.09.002
PubMed Abstract | Full Text | CrossRef Full Text | Google Scholar
Guglielmini, J., Quintais, L., Garcillán-Barcia, M. P., de la Cruz, F., and Rocha, E. P. (2011). The repertoire of ICE in prokaryotes underscores the unity, diversity, and ubiquity of conjugation. PLoS Genet. 7:e1002222. doi: 10.1371/journal.pgen.1002222
PubMed Abstract | Full Text | CrossRef Full Text | Google Scholar
Heuer, H., Binh, C. T., Jechalke, S., Kopmann, C., Zimmerling, U., Krogerrecklenfort, E., et al. (2012). IncP-1epsilon plasmids are important vectors of antibiotic resistance genes in agricultural systems: diversification driven by class 1 integron gene cassettes. Front. Microbiol. 3:2. doi: 10.3389/fmicb.2012.00002
PubMed Abstract | Full Text | CrossRef Full Text | Google Scholar
Heuer, H., and Smalla, K. (2007). Horizontal gene transfer between bacteria. Environ. Biosafety Res. 6, 3–13. doi: 10.1051/ebr:2007034
PubMed Abstract | Full Text | CrossRef Full Text | Google Scholar
Heuer, H., and Smalla, K. (2012). Plasmids foster diversification and adaptation of bacterial populations in soil. FEMS Microbiol. Rev. 36, 1083–1104. doi: 10.1111/j.1574-6976.2012.00337.x
PubMed Abstract | Full Text | CrossRef Full Text | Google Scholar
Hou, C., Wang, Q., Zeng, X., Yang, F., Zhang, J., Liu, H., et al. (2014). Complete genome sequence of Lactobacillus reuteri I5007, a probiotic strain isolated from healthy piglet. J. Biotechnol. 179, 63–64. doi: 10.1016/j.jbiotec.2014.03.019
PubMed Abstract | Full Text | CrossRef Full Text | Google Scholar
Huang, C. H., Tsai, H. H., Tsay, Y. G., Chien, Y. N., Wang, S. L., Cheng, M. Y., et al. (2007). The telomere system of the Streptomyces linear plasmid SCP1 represents a novel class. Mol. Microbiol. 63, 1710–1718. doi: 10.1111/j.1365-2958.2007.05616.x
PubMed Abstract | Full Text | CrossRef Full Text | Google Scholar
Jayashree, S., Pooja, S., Pushpanathan, M., Vishnu, U., Sankarasubramanian, J., Rajendhran, J., et al. (2013). Genome sequence of Lactobacillus fermentum strain MTCC 8711, a probiotic bacterium isolated from yogurt. Genome Announc. 1, e00770–e00713. doi: 10.1128/genomeA.00770-13
PubMed Abstract | Full Text | CrossRef Full Text | Google Scholar
Jensen, L. B., Garcia-Migura, L., Valenzuela, A. J., Løhr, M., Hasman, H., and Aarestrup, F. M. (2010a). A classification system for plasmids from enterococci and other Gram-positive bacteria. J. Microbiol. Methods 80, 25–43. doi: 10.1016/j.mimet.2009.10.012
PubMed Abstract | Full Text | CrossRef Full Text | Google Scholar
Jensen, S. O., Apisiridej, S., Kwong, S. M., Yang, Y. H., Skurray, R. A., and Firth, N. (2010b). Analysis of the prototypical Staphylococcus aureus multiresistance plasmid pSK1. Plasmid 64, 135–142. doi: 10.1016/j.plasmid.2010.06.001
PubMed Abstract | Full Text | CrossRef Full Text | Google Scholar
Jeon, C. O., Park, M., Ro, H. S., Park, W., and Madsen, E. L. (2006). The naphthalene catabolic (nag) genes of Polaromonas naphthalenivorans CJ2: evolutionary implications for two gene clusters and novel regulatory control. Appl. Environ. Microbiol. 72, 1086–1095. doi: 10.1128/AEM.72.2.1086-1095.2006
PubMed Abstract | Full Text | CrossRef Full Text | Google Scholar
Jeon, C. O., Park, W., Padmanabhan, P., Derito, C., Snape, J. R., and Madsen, E. L. (2003). Discovery of a bacterium, with distinctive dioxygenase, that is responsible for in situ biodegradation in contaminated sediment. Proc. Natl. Acad. Sci. U.S.A. 100, 13591–13596. doi: 10.1073/pnas.1735529100
PubMed Abstract | Full Text | CrossRef Full Text | Google Scholar
Johnson, T. J., and Nolan, L. K. (2009). Pathogenomics of the virulence plasmids of Escherichia coli. Microbiol. Mol. Biol. Rev. 73, 750–774. doi: 10.1128/MMBR.00015-09
PubMed Abstract | Full Text | CrossRef Full Text | Google Scholar
Jones, B. V., and Marchesi, J. R. (2007). Transposon-aided capture (TRACA) of plasmids resident in the human gut mobile metagenome. Nat. Methods 4, 55–61. doi: 10.1038/nmeth964
PubMed Abstract | Full Text | CrossRef Full Text | Google Scholar
Jorgensen, T. S., Xu, Z., Hansen, M. A., Sorensen, S. J., and Hansen, L. H. (2014). Hundreds of circular novel plasmids and DNA elements identified in a rat cecum metamobilome. PLoS ONE 9:e87924. doi: 10.1371/journal.pone.0087924
PubMed Abstract | Full Text | CrossRef Full Text | Google Scholar
Joshua, C. J., Perez, L. D., and Keasling, J. D. (2013). Functional characterization of the origin of replication of pRN1 from Sulfolobus islandicus REN1H1. PLoS ONE 8:e84664. doi: 10.1371/journal.pone.0084664
PubMed Abstract | Full Text | CrossRef Full Text | Google Scholar
Kendall, K. J., and Cohen, S. N. (1988). Complete nucleotide sequence of the Streptomyces lividans plasmid pIJ101 and correlation of the sequence with genetic properties. J. Bacteriol. 170, 4634–4651.
Khan, S. A. (2005). Plasmid rolling-circle replication: highlights of two decades of research. Plasmid 53, 126–136. doi: 10.1016/j.plasmid.2004.12.008
PubMed Abstract | Full Text | CrossRef Full Text | Google Scholar
Kristiansson, E., Fick, J., Janzon, A., Grabic, R., Rutgersson, C., Weijdegård, B., et al. (2011). Pyrosequencing of antibiotic-contaminated river sediments reveals high levels of resistance and gene transfer elements. PLoS ONE 6:e17038. doi: 10.1371/journal.pone.0017038
PubMed Abstract | Full Text | CrossRef Full Text | Google Scholar
Krüger, R., Rakowski, S. A., and Filutowicz, M. (2004). “Participating elements in the replication of iteron-containing plasmids,” in Plasmid Biology, eds G. Phillips and B. Funnell (Washington, DC: ASM Press), 25–45.
Labidi, A., David, H. L., and Roulland-Dussoix, D. (1985). Restriction endonuclease mapping and cloning of Mycobacterium fortuitum var. fortuitum plasmid pAL5000. Ann. Inst. Pasteur Microbiol. 136B, 209–215. doi: 10.1016/S0769-2609(85)80045-4
PubMed Abstract | Full Text | CrossRef Full Text | Google Scholar
Lambert, G., and Carr, N. (1983). A restriction map of plasmid pDC1 from the filamentous cyanobacterium Nostoc sp. MAC PCC 8009. Plasmid 10, 196–198. doi: 10.1016/0147-619X(83)90072-0
PubMed Abstract | Full Text | CrossRef Full Text | Google Scholar
Lawley, T., Wilkins, B. M., and Frost, L. S. (2004). “Bacterial conjugation in Gram-negative bacteria,” in Plasmid Biology, eds G. Phillips and B. Funnell (Washington, DC: ASM Press), 203–226.
Lawrence, J. G., and Ochman, H. (1998). Molecular archaeology of the Escherichia coli genome. Proc. Natl. Acad. Sci. U.S.A. 95, 9413–9417. doi: 10.1073/pnas.95.16.9413
PubMed Abstract | Full Text | CrossRef Full Text | Google Scholar
Le Dantec, C., Winter, N., Gicquel, B., Vincent, V., and Picardeau, M. (2001). Genomic sequence and transcriptional analysis of a 23-kilobase mycobacterial linear plasmid: evidence for horizontal transfer and identification of plasmid maintenance systems. J. Bacteriol. 183, 2157–2164. doi: 10.1128/JB.183.7.2157-2164.2001
PubMed Abstract | Full Text | CrossRef Full Text | Google Scholar
Lee, J. H., and O'Sullivan, D. J. (2006). Sequence analysis of two cryptic plasmids from Bifidobacterium longum DJO10A and construction of a shuttle cloning vector. Appl. Environ. Microbiol. 72, 527–535. doi: 10.1128/AEM.72.1.527-535.2006
PubMed Abstract | Full Text | CrossRef Full Text | Google Scholar
Leigh, J. A., Albers, S. V., Atomi, H., and Allers, T. (2011). Model organisms for genetics in the domain Archaea: methanogens, halophiles, Thermococcales and Sulfolobales. FEMS Microbiol. Rev. 35, 577–608. doi: 10.1111/j.1574-6976.2011.00265.x
PubMed Abstract | Full Text | CrossRef Full Text | Google Scholar
Liu, M. A., Kwong, S. M., Jensen, S. O., Brzoska, A. J., and Firth, N. (2013). Biology of the staphylococcal conjugative multiresistance plasmid pSK41. Plasmid 70, 42–51. doi: 10.1016/j.plasmid.2013.02.001
PubMed Abstract | Full Text | CrossRef Full Text | Google Scholar
Lozano, C., García-Migura, L., Aspiroz, C., Zarazaga, M., Torres, C., and Aarestrup, F. M. (2012). Expansion of a plasmid classification system for Gram-positive bacteria and determination of the diversity of plasmids in Staphylococcus aureus strains of human, animal, and food origins. Appl. Environ. Microbiol. 78, 5948–5955. doi: 10.1128/AEM.00870-12
PubMed Abstract | Full Text | CrossRef Full Text | Google Scholar
Luo, Y. R., Kang, S. G., Kim, S. J., Kim, M. R., Li, N., Lee, J. H., et al. (2012). Genome sequence of benzo(a)pyrene-degrading bacterium Novosphingobium pentaromativorans US6-1. J. Bacteriol. 194, 907. doi: 10.1128/JB.06476-11
PubMed Abstract | Full Text | CrossRef Full Text | Google Scholar
Masai, E., Sugiyama, K., Iwashita, N., Shimizu, S., Hauschild, J. E., Hatta, T., et al. (1997). The bphDEF meta-cleavage pathway genes involved in biphenyl/polychlorinated biphenyl degradation are located on a linear plasmid and separated from the initial bphACB genes in Rhodococcus sp. strain RHA1. Gene 187, 141–149. doi: 10.1016/S0378-1119(96)00748-2
PubMed Abstract | Full Text | CrossRef Full Text | Google Scholar
McLeod, M. P., Warren, R. L., Hsiao, W. W., Araki, N., Myhre, M., Fernandes, C., et al. (2006). The complete genome of Rhodococcus sp. RHA1 provides insights into a catabolic powerhouse. Proc. Natl. Acad. Sci. U.S.A. 103, 15582–15587. doi: 10.1073/pnas.0607048103
PubMed Abstract | Full Text | CrossRef Full Text | Google Scholar
Metcalf, W. W., Zhang, J. K., Apolinario, E., Sowers, K. R., and Wolfe, R. S. (1997). A genetic system for Archaea of the genus Methanosarcina: liposome-mediated transformation and construction of shuttle vectors. Proc. Natl. Acad. Sci. U.S.A. 94, 2626–2631. doi: 10.1073/pnas.94.6.2626
PubMed Abstract | Full Text | CrossRef Full Text | Google Scholar
Miller, T. R., Delcher, A. L., Salzberg, S. L., Saunders, E., Detter, J. C., and Halden, R. U. (2010). Genome sequence of the dioxin-mineralizing bacterium Sphingomonas wittichii RW1. J. Bacteriol. 192, 6101–6102. doi: 10.1128/JB.01030-10
PubMed Abstract | Full Text | CrossRef Full Text | Google Scholar
Miyazaki, R., Sato, Y., Ito, M., Ohtsubo, Y., Nagata, Y., and Tsuda, M. (2006). Complete nucleotide sequence of an exogenously isolated plasmid, pLB1, involved in gamma-hexachlorocyclohexane degradation. Appl. Environ. Microbiol. 72, 6923–6933. doi: 10.1128/AEM.01531-06
PubMed Abstract | Full Text | CrossRef Full Text | Google Scholar
Musovic, S., Dechesne, A., Sørensen, J., and Smets, B. F. (2010). Novel assay to assess permissiveness of a soil microbial community toward receipt of mobile genetic elements. Appl. Environ. Microbiol. 76, 4813–4818. doi: 10.1128/AEM.02713-09
PubMed Abstract | Full Text | CrossRef Full Text | Google Scholar
Nagata, Y., Kamakura, M., Endo, R., Miyazaki, R., Ohtsubo, Y., and Tsuda, M. (2006). Distribution of gamma-hexachlorocyclohexane-degrading genes on three replicons in Sphingobium japonicum UT26. FEMS Microbiol. Lett. 256, 112–118. doi: 10.1111/j.1574-6968.2005.00096.x
PubMed Abstract | Full Text | CrossRef Full Text | Google Scholar
Nagata, Y., Natsui, S., Endo, R., Ohtsubo, Y., Ichikawa, N., Ankai, A., et al. (2011). Genomic organization and genomic structural rearrangements of Sphingobium japonicum UT26, an archetypal γ-hexachlorocyclohexane-degrading bacterium. Enzyme Microb. Technol. 49, 499–508. doi: 10.1016/j.enzmictec.2011.10.005
PubMed Abstract | Full Text | CrossRef Full Text | Google Scholar
Nagata, Y., Ohtsubo, Y., Endo, R., Ichikawa, N., Ankai, A., Oguchi, A., et al. (2010). Complete genome sequence of the representative γ-hexachlorocyclohexane-degrading bacterium Sphingobium japonicum UT26. J. Bacteriol. 192, 5852–5853. doi: 10.1128/JB.00961-10
PubMed Abstract | Full Text | CrossRef Full Text | Google Scholar
Nishida, H. (2012). Comparative analyses of base compositions, DNA sizes, and dinucleotide frequency profiles in archaeal and bacterial chromosomes and plasmids. Int. J. Evol. Biol. 2012:342482. doi: 10.1155/2012/342482
PubMed Abstract | Full Text | CrossRef Full Text | Google Scholar
Norberg, P., Bergstrom, M., and Hermansson, M. (2014). Complete nucleotide sequence and analysis of two conjugative broad host range plasmids from a marine microbial biofilm. PLoS ONE 9:e92321. doi: 10.1371/journal.pone.0092321
PubMed Abstract | Full Text | CrossRef Full Text | Google Scholar
Okumura, H., Uozumi, T., and Beppu, T. (1985). Construction of plasmid vectors and a genetic transformation system for Acetobacter aceti. Agric. Biol. Chem. 49, 1011–1017. doi: 10.1271/bbb1961.49.1011
Oliveira, C. S., Moura, A., Henriques, I., Brown, C. J., Rogers, L. M., Top, E. M., et al. (2013). Comparative genomics of IncP-1epsilon plasmids from water environments reveals diverse and unique accessory genetic elements. Plasmid 70, 412–419. doi: 10.1016/j.plasmid.2013.06.002
PubMed Abstract | Full Text | CrossRef Full Text | Google Scholar
Palmer, K. L., Kos, V. N., and Gilmore, M. S. (2010). Horizontal gene transfer and the genomics of enterococcal antibiotic resistance. Curr. Opin. Microbiol. 13, 632–639. doi: 10.1016/j.mib.2010.08.004
PubMed Abstract | Full Text | CrossRef Full Text | Google Scholar
Pandza, S., Biukovic, G., Paravic, A., Dadbin, A., Cullum, J., and Hranueli, D. (1998). Recombination between the linear plasmid pPZG101 and the linear chromosome of Streptomyces rimosus can lead to exchange of ends. Mol. Microbiol. 28, 1165–1176. doi: 10.1046/j.1365-2958.1998.00877.x
PubMed Abstract | Full Text | CrossRef Full Text | Google Scholar
Park, M. S., Shin, D. W., Lee, K. H., and Ji, G. E. (1999). Sequence analysis of plasmid pKJ50 from Bifidobacterium longum. Microbiology 145(Pt 3), 585–592. doi: 10.1099/13500872-145-3-585
PubMed Abstract | Full Text | CrossRef Full Text | Google Scholar
Partridge, S. R. (2011). Analysis of antibiotic resistance regions in Gram-negative bacteria. FEMS Microbiol. Rev. 35, 820–855. doi: 10.1111/j.1574-6976.2011.00277.x
PubMed Abstract | Full Text | CrossRef Full Text | Google Scholar
Paterson, D. L. (2006). Resistance in gram-negative bacteria: Enterobacteriaceae. Am. J. Infect. Control 34, S20–S28. discussion: S64–S73. doi: 10.1016/j.ajic.2006.05.238
PubMed Abstract | Full Text | CrossRef Full Text | Google Scholar
Petersen, J. M., Zielinski, F. U., Pape, T., Seifert, R., Moraru, C., Amann, R., et al. (2011). Hydrogen is an energy source for hydrothermal vent symbioses. Nature 476, 176–180. doi: 10.1038/nature10325
PubMed Abstract | Full Text | CrossRef Full Text | Google Scholar
Pinto, U. M., Pappas, K. M., and Winans, S. C. (2012). The ABCs of plasmid replication and segregation. Nat. Rev. Microbiol. 10, 755–765. doi: 10.1038/nrmicro2882
PubMed Abstract | Full Text | CrossRef Full Text | Google Scholar
Poehlein, A., Kusian, B., Friedrich, B., Daniel, R., and Bowien, B. (2011). Complete genome sequence of the type strain Cupriavidus necator N-1. J. Bacteriol. 193, 5017. doi: 10.1128/JB.05660-11
PubMed Abstract | Full Text | CrossRef Full Text | Google Scholar
Polisky, B. (1988). ColE1 replication control circuitry: sense from antisense. Cell 55, 929–932. doi: 10.1016/0092-8674(88)90235-8
PubMed Abstract | Full Text | CrossRef Full Text | Google Scholar
Rakowski, S. A., and Filutowicz, M. (2013). Plasmid R6K replication control. Plasmid 69, 231–242. doi: 10.1016/j.plasmid.2013.02.003
PubMed Abstract | Full Text | CrossRef Full Text | Google Scholar
Ravin, N. V. (2011). N15: the linear phage-plasmid. Plasmid 65, 102–109. doi: 10.1016/j.plasmid.2010.12.004
PubMed Abstract | Full Text | CrossRef Full Text | Google Scholar
Rocha, E. P., and Danchin, A. (2002). Base composition bias might result from competition for metabolic resources. Trends Genet. 18, 291–294. doi: 10.1016/S0168-9525(02)02690-2
PubMed Abstract | Full Text | CrossRef Full Text | Google Scholar
Romine, M. F., Stillwell, L. C., Wong, K. K., Thurston, S. J., Sisk, E. C., Sensen, C., et al. (1999). Complete sequence of a 184-kilobase catabolic plasmid from Sphingomonas aromaticivorans F199. J. Bacteriol. 181, 1585–1602.
Rosvoll, T. C., Pedersen, T., Sletvold, H., Johnsen, P. J., Sollid, J. E., Simonsen, G. S., et al. (2010). PCR-based plasmid typing in Enterococcus faecium strains reveals widely distributed pRE25-, pRUM-, pIP501- and pHTbeta-related replicons associated with glycopeptide resistance and stabilizing toxin-antitoxin systems. FEMS Immunol. Med. Microbiol. 58, 254–268. doi: 10.1111/j.1574-695X.2009.00633.x
PubMed Abstract | Full Text | CrossRef Full Text | Google Scholar
Sekine, M., Tanikawa, S., Omata, S., Saito, M., Fujisawa, T., Tsukatani, N., et al. (2006). Sequence analysis of three plasmids harboured in Rhodococcus erythropolis strain PR4. Environ. Microbiol. 8, 334–346. doi: 10.1111/j.1462-2920.2005.00899.x
PubMed Abstract | Full Text | CrossRef Full Text | Google Scholar
Sen, D., Van der Auwera, G. A., Rogers, L. M., Thomas, C. M., Brown, C. J., and Top, E. M. (2011). Broad-host-range plasmids from agricultural soils have IncP-1 backbones with diverse accessory genes. Appl. Environ. Microbiol. 77, 7975–7983. doi: 10.1128/AEM.05439-11
PubMed Abstract | Full Text | CrossRef Full Text | Google Scholar
Sentchilo, V., Mayer, A. P., Guy, L., Miyazaki, R., Green Tringe, S., Barry, K., et al. (2013). Community-wide plasmid gene mobilization and selection. ISME J. 7, 1173–1186. doi: 10.1038/ismej.2013.13
PubMed Abstract | Full Text | CrossRef Full Text | Google Scholar
Seto, M., Kimbara, K., Shimura, M., Hatta, T., Fukuda, M., and Yano, K. (1995). A novel transformation of polychlorinated biphenyls by Rhodococcus sp. strain RHA1. Appl. Environ. Microbiol. 61, 3353–3358.
Shareck, J., Choi, Y., Lee, B., and Miguez, C. B. (2004). Cloning vectors based on cryptic plasmids isolated from lactic acid bacteria: their characteristics and potential applications in biotechnology. Crit. Rev. Biotechnol. 24, 155–208. doi: 10.1080/07388550490904288
PubMed Abstract | Full Text | CrossRef Full Text | Google Scholar
She, Q., Phan, H., Garrett, R. A., Albers, S. V., Stedman, K. M., and Zillig, W. (1998). Genetic profile of pNOB8 from Sulfolobus: the first conjugative plasmid from an archaeon. Extremophiles 2, 417–425. doi: 10.1007/s007920050087
PubMed Abstract | Full Text | CrossRef Full Text | Google Scholar
Shimizu, S., Kobayashi, H., Masai, E., and Fukuda, M. (2001). Characterization of the 450-kb linear plasmid in a polychlorinated biphenyl degrader, Rhodococcus sp. strain RHA1. Appl. Environ. Microbiol. 67, 2021–2028. doi: 10.1128/AEM.67.5.2021-2028.2001
PubMed Abstract | Full Text | CrossRef Full Text | Google Scholar
Shintani, M., Matsui, K., Inoue, J., Hosoyama, A., Ohji, S., Yamazoe, A., et al. (2014a). Single-cell analyses revealed transfer ranges of IncP-1, IncP-7, and IncP-9 plasmids in a soil bacterial community. Appl. Environ. Microbiol. 80, 138–145. doi: 10.1128/AEM.02571-13
PubMed Abstract | Full Text | CrossRef Full Text | Google Scholar
Shintani, M., and Nojiri, H. (2013). “Mobile genetic elements (MGEs) carrying catabolic genes,” in Management of Microbial Resources in the Environment, eds A. Malik, E. Grohmann, and M. Alves (Dordrecht: Springer), 167–214. doi: 10.1007/978-94-007-5931-2_8
Shintani, M., Ohtsubo, Y., Fukuda, K., Hosoyama, A., Ohji, S., Yamazoe, A., et al. (2014b). Complete genome sequence of the thermophilic polychlorinated biphenyl degrader Geobacillus sp. strain JF8 (NBRC 109937). Genome Announc 2, e01213–13. doi: 10.1128/genomeA.01213-13
PubMed Abstract | Full Text | CrossRef Full Text | Google Scholar
Shintani, M., Takahashi, Y., Yamane, H., and Nojiri, H. (2010). The behavior and significance of degradative plasmids belonging to Inc groups in Pseudomonas within natural environments and microcosms. Microbes Environ. 25, 253–265. doi: 10.1264/jsme2.ME10155
PubMed Abstract | Full Text | CrossRef Full Text | Google Scholar
Smalla, K., and Sobecky, P. A. (2002). The prevalence and diversity of mobile genetic elements in bacterial communities of different environmental habitats: insights gained from different methodological approaches. FEMS Microbiol. Ecol. 42, 165–175. doi: 10.1111/j.1574-6941.2002.tb01006.x
PubMed Abstract | Full Text | CrossRef Full Text | Google Scholar
Smillie, C., Garcillán-Barcia, M. P., Francia, M. V., Rocha, E. P., and de la Cruz, F. (2010). Mobility of plasmids. Microbiol. Mol. Biol. Rev. 74, 434–452. doi: 10.1128/MMBR.00020-10
PubMed Abstract | Full Text | CrossRef Full Text | Google Scholar
Smith, M. C., and Thomas, C. D. (2004). An accessory protein is required for relaxosome formation by small staphylococcal plasmids. J. Bacteriol. 186, 3363–3373. doi: 10.1128/JB.186.11.3363-3373.2004
PubMed Abstract | Full Text | CrossRef Full Text | Google Scholar
Sobecky, P. A., and Hazen, T. H. (2009). Horizontal gene transfer and mobile genetic elements in marine systems. Methods Mol. Biol. 532, 435–453. doi: 10.1007/978-1-60327-853-9_25
PubMed Abstract | Full Text | CrossRef Full Text | Google Scholar
Soler, N., Justome, A., Quevillon-Cheruel, S., Lorieux, F., Le Cam, E., Marguet, E., et al. (2007). The rolling-circle plasmid pTN1 from the hyperthermophilic archaeon Thermococcus nautilus. Mol. Microbiol. 66, 357–370. doi: 10.1111/j.1365-2958.2007.05912.x
PubMed Abstract | Full Text | CrossRef Full Text | Google Scholar
Song, X., Sun, J., Mikalsen, T., Roberts, A. P., and Sundsfjord, A. (2013). Characterisation of the plasmidome within Enterococcus faecalis isolated from marginal periodontitis patients in Norway. PLoS ONE 8:e62248. doi: 10.1371/journal.pone.0062248
PubMed Abstract | Full Text | CrossRef Full Text | Google Scholar
Sorvig, E., Mathiesen, G., Naterstad, K., Eijsink, V. G., and Axelsson, L. (2005). High-level, inducible gene expression in Lactobacillus sakei and Lactobacillus plantarum using versatile expression vectors. Microbiology 151, 2439–2449. doi: 10.1099/mic.0.28084-0
PubMed Abstract | Full Text | CrossRef Full Text | Google Scholar
Sota, M., and Top, E. (2008). “Horizontal gene transfer mediated by plasmids,” in Plasmids: Current Research and Future Trends, ed G. Lipps (Norfolk, VA: Caister Academic Press; Horizon Scientific Press), 111–181.
Stillwell, L. C., Thurston, S. J., Schneider, R. P., Romine, M. F., Fredrickson, J. K., and Saffer, J. D. (1995). Physical mapping and characterization of a catabolic plasmid from the deep-subsurface bacterium Sphingomonas sp. strain F199. J. Bacteriol. 177, 4537–4539.
Straley, S. C., Plano, G. V., Skrzypek, E., Haddix, P. L., and Fields, K. A. (1993). Regulation by Ca2+ in the Yersinia low-Ca2+ response. Mol. Microbiol. 8, 1005–1010. doi: 10.1111/j.1365-2958.1993.tb01644.x
PubMed Abstract | Full Text | CrossRef Full Text | Google Scholar
Taton, A., Unglaub, F., Wright, N. E., Zeng, W. Y., Paz-Yepes, J., Brahamsha, B., et al. (2014). Broad-host-range vector system for synthetic biology and biotechnology in cyanobacteria. Nucleic Acids Res. 42:e136. doi: 10.1093/nar/gku673
PubMed Abstract | Full Text | CrossRef Full Text | Google Scholar
Tauch, A., Bischoff, N., Brune, I., and Kalinowski, J. (2003a). Insights into the genetic organization of the Corynebacterium diphtheriae erythromycin resistance plasmid pNG2 deduced from its complete nucleotide sequence. Plasmid 49, 63–74. doi: 10.1016/S0147-619X(02)00115-4
PubMed Abstract | Full Text | CrossRef Full Text | Google Scholar
Tauch, A., Pühler, A., Kalinowski, J., and Thierbach, G. (2003b). Plasmids in Corynebacterium glutamicum and their molecular classification by comparative genomics. J. Biotechnol. 104, 27–40. doi: 10.1016/S0168-1656(03)00157-3
PubMed Abstract | Full Text | CrossRef Full Text | Google Scholar
Taylor, D. E., Gibreel, A., Lawley, T. D., and Tracz, D. M. (2004). “Anitibiotic resistance plasmids,” in Plasmid Biology, eds G. Phillips and B. Funnell (Washington, DC: ASM Press), 473–491.
Thomas, C. M., and Haines, A. S. (2004). “Plasmids of the genus Pseudomonas,” in Pseudomonas, ed J. L. Ramos (New York, NY: Plenum Publishing Corporation), 197–231.
Tilly, K., Checroun, C., and Rosa, P. A. (2012). Requirements for Borrelia burgdorferi plasmid maintenance. Plasmid 68, 1–12. doi: 10.1016/j.plasmid.2012.01.009
PubMed Abstract | Full Text | CrossRef Full Text | Google Scholar
Torres, M. J., Rubia, M. I., Bedmar, E. J., and Delgado, M. J. (2011). Denitrification in Sinorhizobium meliloti. Biochem. Soc. Trans. 39, 1886–1889. doi: 10.1042/BST20110733
PubMed Abstract | Full Text | CrossRef Full Text | Google Scholar
Tumbula, D. L., Bowen, T. L., and Whitman, W. B. (1997). Characterization of pURB500 from the archaeon Methanococcus maripaludis and construction of a shuttle vector. J. Bacteriol. 179, 2976–2986.
Udo, E. E., and Grubb, W. B. (1991). A new incompatibility group plasmid in Staphylococcus aureus. FEMS Microbiol. Lett. 62, 33–36. doi: 10.1111/j.1574-6968.1991.tb04412.x
PubMed Abstract | Full Text | CrossRef Full Text | Google Scholar
Ventura, M., Canchaya, C., Tauch, A., Chandra, G., Fitzgerald, G. F., Chater, K. F., et al. (2007). Genomics of Actinobacteria: tracing the evolutionary history of an ancient phylum. Microbiol. Mol. Biol. Rev. 71, 495–548. doi: 10.1128/MMBR.00005-07
PubMed Abstract | Full Text | CrossRef Full Text | Google Scholar
Wagner, M., and Haider, S. (2012). New trends in fluorescence in situ hybridization for identification and functional analyses of microbes. Curr. Opin. Biotechnol. 23, 96–102. doi: 10.1016/j.copbio.2011.10.010
PubMed Abstract | Full Text | CrossRef Full Text | Google Scholar
Walton, D. K., Gendel, S. M., and Atherly, A. G. (1992). Nucleotide sequence of the replication region of the Nostoc PCC 7524 plasmid pDU1. Nucleic Acids Res. 20:4660. doi: 10.1093/nar/20.17.4660
PubMed Abstract | Full Text | CrossRef Full Text | Google Scholar
Wang, J., Stephan, R., Karczmarczyk, M., Yan, Q., Hachler, H., and Fanning, S. (2013). Molecular characterization of bla ESBL-harboring conjugative plasmids identified in multi-drug resistant Escherichia coli isolated from food-producing animals and healthy humans. Front. Microbiol. 4:188. doi: 10.3389/fmicb.2013.00188
PubMed Abstract | Full Text | CrossRef Full Text | Google Scholar
Xiong, J., Alexander, D. C., Ma, J. H., Deraspe, M., Low, D. E., Jamieson, F. B., et al. (2013). Complete sequence of pOZ176, a 500-kilobase IncP-2 plasmid encoding IMP-9-mediated carbapenem resistance, from outbreak isolate Pseudomonas aeruginosa 96. Antimicrob. Agents Chemother. 57, 3775–3782. doi: 10.1128/AAC.00423-13
PubMed Abstract | Full Text | CrossRef Full Text | Google Scholar
Yagi, J. M., Sims, D., Brettin, T., Bruce, D., and Madsen, E. L. (2009). The genome of Polaromonas naphthalenivorans strain CJ2, isolated from coal tar-contaminated sediment, reveals physiological and metabolic versatility and evolution through extensive horizontal gene transfer. Environ. Microbiol. 11, 2253–2270. doi: 10.1111/j.1462-2920.2009.01947.x
PubMed Abstract | Full Text | CrossRef Full Text | Google Scholar
Yamashita, A., Sekizuka, T., and Kuroda, M. (2014). Characterization of antimicrobial resistance dissemination across plasmid communities classified by network analysis. Pathogens 3, 356–376. doi: 10.3390/pathogens3020356
PubMed Abstract | Full Text | CrossRef Full Text | Google Scholar
Zhang, T., Zhang, X. X., and Ye, L. (2011). Plasmid metagenome reveals high levels of antibiotic resistance genes and mobile genetic elements in activated sludge. PLoS ONE 6:e26041. doi: 10.1371/journal.pone.0026041
PubMed Abstract | Full Text | CrossRef Full Text | Google Scholar
Keywords: plasmid, host, replication, conjugative transfer, Inc group
Citation: Shintani M, Sanchez ZK and Kimbara K (2015) Genomics of microbial plasmids: classification and identification based on replication and transfer systems and host taxonomy. Front. Microbiol. 6:242. doi: 10.3389/fmicb.2015.00242
Received: 30 September 2014; Accepted: 12 March 2015;
Published: 31 March 2015.
Edited by:
Eva M. Top, University of Idaho, USAReviewed by:
Itzhak Mizrahi, Agricultural Research Organization, Volcani Research Center, IsraelClaudia Oliveira, University of Aveiro, Portugal
Timothy J. Johnson, University of Minnesota, USA
Copyright © 2015 Shintani, Sanchez and Kimbara. This is an open-access article distributed under the terms of the Creative Commons Attribution License (CC BY). The use, distribution or reproduction in other forums is permitted, provided the original author(s) or licensor are credited and that the original publication in this journal is cited, in accordance with accepted academic practice. No use, distribution or reproduction is permitted which does not comply with these terms.
*Correspondence: Masaki Shintani, Department of Applied Chemistry and Biochemical Engineering, Department of Bioscience, Graduate School of Engineering, Graduate School of Science and Technology, Shizuoka University, 3-5-1 Johoku, Naka-ku, Hamamatsu, 432-8561 Shizuoka, Japan, shintani.masaki@shizuoka.ac.jp