- Belowground Ecosystem Group, Department of Forest and Conservation Sciences, University of British Columbia, Vancouver, BC, Canada
Mycorrhizal associations are ubiquitous and form a substantial component of the microbial biomass in forest ecosystems and fluxes of C to these belowground organisms account for a substantial portion of carbon assimilated by forest vegetation. Climate change has been predicted to alter belowground plant-allocated C which may cause compositional shifts in soil microbial communities, and it has been hypothesized that this community change will influence C mitigation in forest ecosystems. Some 10,000 species of ectomycorrhizal fungi are currently recognized, some of which are host specific and will only associate with a single tree species, for example, Suillus grevillei with larch. Mycorrhizae are a strong sink for plant C, differences in mycorrhizal anatomy, particularly the presence and extent of emanating hyphae, can affect the amount of plant C allocated to these assemblages. Mycorrhizal morphology affects not only spatial distribution of C in forests, but also differences in the longevity of these diverse structures may have important consequences for C sequestration in soil. Mycorrhizal growth form has been used to group fungi into distinctive functional groups that vary qualitatively and spatially in their foraging and nutrient acquiring potential. Through new genomic techniques we are beginning to understand the mechanisms involved in the specificity and selection of ectomycorrhizal associations though much less is known about arbuscular mycorrhizal associations. In this review we examine evidence for tree species- mycorrhizal specificity, and the mechanisms involved (e.g., signal compounds). We also explore what is known about the effects of these associations and interactions with other soil organisms on the quality and quantity of C flow into the mycorrhizosphere (the area under the influence of mycorrhizal root tips), including spatial and seasonal variations. The enormity of the mycorrhizosphere biome in forests and its potential to sequester substantial C belowground highlights the vital importance of increasing our knowledge of the dynamics of the different mycorrhizal functional groups in diverse forests.
Introduction
Soil organic matter (SOM) is the largest carbon (C) pool in terrestrial ecosystems (Falkowski et al., 2000; Fontaine et al., 2003), greater than terrestrial biomass C and atmospheric C combined (Jobbágy and Jackson, 2000). Carbon enters the SOM pool via litter (leaves, coarse and fine roots), brash (branches and coarse woody debris) and root exudates. The proportion of recently photosynthesized C allocated to leaves, storage, metabolism and root exudates has important consequences for soil C storage and varies depending on the environment, plant type, age of the plant, microbial symbionts and nutrient availability (Litton et al., 2007; Epron et al., 2012). Belowground C allocation is notoriously difficult to measure and varies depending on the spatial heterogeneity of belowground structures, the assemblage of microorganisms in the rhizosphere and environmental conditions (Subke et al., 2009; Kuzyakov and Gavrichkova, 2010; Mencuccini and Holtta, 2010; Warren et al., 2012). Recent studies have challenged our understanding of the mechanisms of C sequestration in soil. Clemmensen et al. (2013) showed that 50–70% of C stored in soil is derived from roots or root-associated microorganisms and that humus accumulation in boreal forests is regulated mainly by C allocation to roots and associated mycelium rather than decomposition of litter by saprophytes. Consequently, studies are beginning to focus on quantifying not only C allocation belowground, but also the spatial and temporal distribution of this C and how it is influenced by root-associated mycorrhizae (Litton and Giardina, 2008; Chapin et al., 2009; Warren et al., 2012).
Ninety percent of vascular plants form symbiotic relationships with mycorrhizal fungi (Wang and Qui, 2006; Smith and Read, 2008). Mycorrhizae can be generalized into two groups, endomycorrhizae, where hyphae penetrate root cells, and ectomycorrhizae, which do not penetrate. There are several types of endomycorrhizae including ericoid, arbutoid, monotropoid, orchid and, by far the most prevalent, arbuscular (occurring in approximately 85% of plant species) (Smith and Read, 2008). Arbuscular mycorrhizae (AM) are generally Glomeromycota, and form vesicles or arbuscules after invaginating the cell membranes of root cells. Ectomycorrhizae (ECM) are typically Basidiomycetes, Ascomycetes and Zygomycetes, occuring in 10% of plant species (mostly trees and woody plants). Ectomycorrhizae create a hyphal mantle covering the root tip and form a Hartig net within the root cortex, surrounding the root cells. Although saprotrophic fungi and bacteria are the primary decomposers in the soil, plant acquisition of released nutrients, such as N and P, is achieved through their symbiotic relationships with mycorrhizae (Read and Perez-Moreno, 2003; Lindahl et al., 2007; Talbot et al., 2008).
Mycorrhizae are involved in a number of important soil processes including: weathering of mineral nutrients (Landeweert et al., 2001; Finlay and Rosling, 2006; Wallander, 2006), C cycling, mediating plant responses to stress (Finlay, 2008), and interacting with soil bacteria (both negatively e.g., pathogens and positively e.g., mycorrhization helper bacteria) (Johansson et al., 2004; Frey-Klett et al., 2007). Ectomycorrhizae have broad enzymatic capabilities; they can decompose labile and recalcitrant SOM, and some can mineralize organic N (Chalot and Brun, 1998). This allows the mycorrhizae to transfer large amounts of N directly to their host plants (Hobbie and Hobbie, 2006). Arbuscular mycorrhizal fungal enzymatic capabilities are not thought to be as extensive as ECM; AM can only transfer small amounts of N to their hosts when soil-N levels are high (Tobar et al., 1994; Hodge et al., 2000; Govindarajulu et al., 2005; Reynolds et al., 2005). Arbuscular mycorrhizae mainly access inorganic N sources (Fellbaum et al., 2012), though organic N uptake by AM has been demonstrated in boreal forests (Whiteside et al., 2012). However, AM can transfer large amounts of P to their plant hosts (Smith and Read, 2008), either by hydrolysation of organic P from hyphal tips and subsequent transfer to the tree via arbuscules, or by uptake, conversion and transport of inorganic phosphorus along hyphae. Although some plant species can form symbiotic relationships with both AM and ECM, the dominance or presence of one over the other will alter tree-nutrient availability.
There are 10,000 ECM fungal species that are known to be associated with as many as 8,000 different plant species (Taylor and Alexander, 2005). Tree species select mycorrhizae and free-living microorganisms through exudation of distinct chemical signals into the rhizosphere (the area surrounding the root that is directly influenced by root exudates, Figure 1) (Pires et al., 2012; Shi et al., 2012). Specific exudates will trigger the expression of mycorrhization genes, which are associated with the initiation of hyphal growth toward the plant root rhizosphere (Martin et al., 2007; Podila et al., 2009). In addition, there is increasing evidence that tree-species-rhizosphere community differences are the result of the trees “selecting” for specific microbes through root exudates (Prescott and Grayston, 2013). Plants release several types of root exudates including: mucilage that maintains a constant moisture environment, metal chelators that mobilize iron and zinc, and various forms of C comprising of carbohydrates, amino acids, low-molecular-weight aliphatic- and aromatic-acids, fatty acids, enzymes and hormones (Grayston et al., 1997; Table 1). The composition and quantity of root exudates will vary depending on tree species (Tuason and Arocena, 2009), and will also be modified within a given tree species depending on which mycorrhizal species colonize the tree roots (van Hees et al., 2005). Different ECM can increase root exudation of organic acid (van Hees et al., 2003, 2005; Johansson et al., 2009) and can change organic acid composition compared to non-mycorrhizal trees (Klugh and Cumming, 2003; van Hees et al., 2005). The variation in C allocated to ECM- and AM-roots, and subsequently ECM and AM root exudates is due, in part, to hyphal exudation from the mycorrhizae and mycorrhizal morphology. These hyphal exudates create an area of greater microbial biomass and activity, termed the mycorrhizosphere (area surrounding the mycorrhizal root tip) or hyphosphere (Figure 1) (Jones et al., 2004; Frey-Klett et al., 2007; Finlay, 2008; Nazir et al., 2010). Although bacteria and archaea are omnipresent in the rhizosphere and mycorrhizosphere, their role in ecosystem processes is only beginning to be understood.
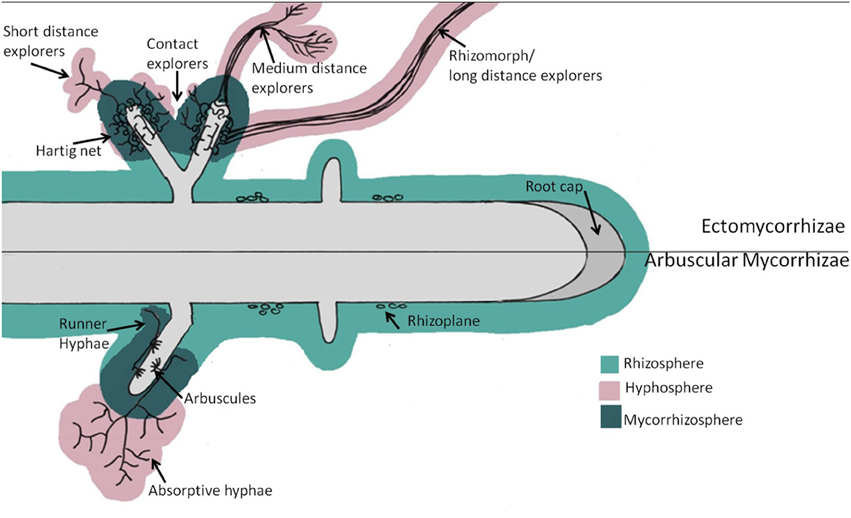
Figure 1. Schematic view of root-mycorrhizal zones of influence and the various mycorrhizal growth forms. Rhizoplane describes the area adjacent to the root where the soil particles adhere. The Rhizosphere is the area of soil around the root that is influenced by root-exuded labile C. The hyphosphere is the area of soil around mycorrhizal hyphae that is influenced by hyphal-exuded labile carbon and enzyme production. The mycorrhizosphere is the area of soil influenced by root and mycorrhizal communities combined.
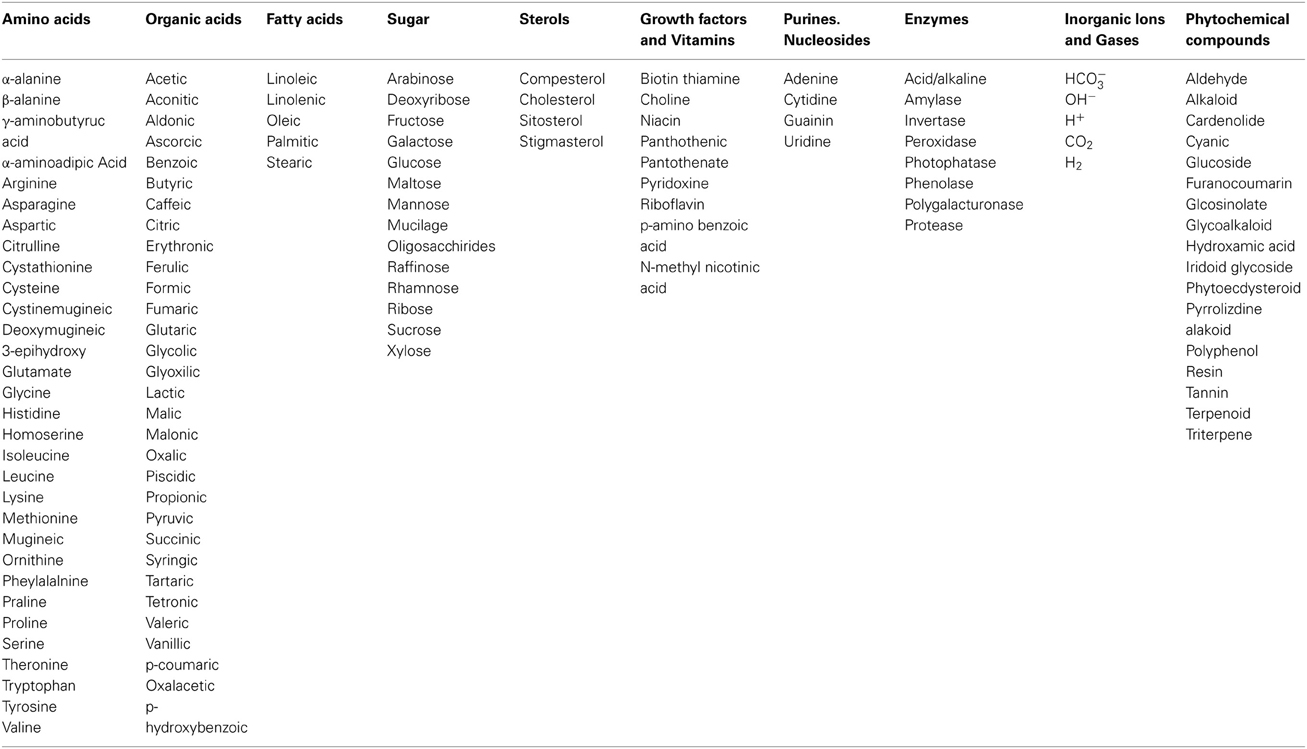
Table 1. Organic compounds and enzymes found in root exudates (Dakora and Phillips, 2002; Rasmann and Agrawal, 2008).
This review focuses on describing host-specificity of soil microorganisms and fauna in the mycorrhizosphere of trees, the signals involved in establishing these interactions, and their impact on soil C flow and sequestration. We concentrate on interactions within the mycorrhizosphere, as this is the active site of root exudation, nutrient cycling, and plant nutrient uptake. The spatial enormity of the mycorrhizosphere biome in forests hints at its potential to sequester substantial amounts of C belowground. An understanding of the controls on C allocation belowground, and the movement of that C throughout the soil environment is a vital knowledge gap.
The Mycorrhizosphere Biome
Tree-Mycorrhizal Specificity
Many temperate forest tree species have ECM associations (including: pine, spruce, larch, hemlock, true firs, Douglas-fir, aspen, birch); some species have AM associations (e.g., cedar, maple, ash) and some have both (e.g., alder, poplar). Some tree species, such as Douglas fir (which associated with more than 2000 known ECM, Molina and Trappe, 1982) have high fungal receptivity, whereas other tree species such as alder (which only associate with 50 known ECM, Pritsch et al., 1997) have narrow fungal receptivity. It has been estimated that ECM mycelia can account for up to 80% of the fungal community and 30% of the total microbial biomass in forest soils (Högberg and Högberg, 2002; Wallander, 2006).
The presence and abundance of specific plant species can influence soil microbial community composition and function (Kourtev et al., 2002; Edwards and Zak, 2010; Eisenhauer et al., 2010), which can, in turn, impact soil C cycling and sequestration as mycorrhizal species differ in growth strategies and C demand. There is evidence of specificity in many plant-microbe interactions, suggesting both strong selective pressure and competition within the rhizosphere microbiome (Podila et al., 2009). There are many species of ECM fungi (Smith and Read, 2008) and though many ECM (e.g., Lactarius) have a broad host range some (e.g., Suillus) have only narrow host range (Bruns et al., 2002; Kennedy et al., 2003). The signaling specificity by host tree species to engage ECM fungi has been well studied (Molina and Trappe, 1982; Ishida et al., 2007; Tedersoo et al., 2008). For example, distinct chemical signals (e.g., small-secreted proteins and hydrophobins) may enable trees such as Populus to recruit advantageous ectomycorrhizal fungi from the broad soil microbial community (Podila et al., 2009). Whole-genome sequencing is now enabling us to have a much greater understanding of the suite of important genes and signals involved in ECM symbiotic associations (Martin et al., 2008, 2010). However, we are only just beginning to understand the factors involved in specificity and selection in AM associations (Brachmann and Parniske, 2006; Bonfante and Genre, 2010).
Mycorrhizal Morphology
Variations in extrametrical mycelium (EMM) hyphal pattern production and in mycorrhizae type may have consequences for C flow and carbon sequestration. ECM fungal taxa vary in the growth patterns of their EMM as a result of their multifarious foraging strategies (Agerer, 2001); the dominance of one morphological type over the other may have consequences for the spatial distribution of recent photosynthates belowground. Agerer (2001) describes the following ECM anatomies: contact explorers, convoy explorers, long-distance explorers, medium distance explorers and short-distance explorers (Figure 1). Contact explorers are EMM with a smooth mantle and few emanating hyphae (diffuse hyphal cords), the tips of which are often in close contact with dead leaves. Examples of contact explorers are Lactarius and Russula species that produce exudates throughout their hyphae. Convoy explorers are EMM that grow within rhizomorphs (aggregated parallel hyphal cords that can conduct nutrients over long distances) or mantles and produce haustoria in cortical cells of roots. Long-distance exploring EMM are smooth with highly differentiated rhizomorphs. For example, Boletales species are long distance hydrophilic hyphal explorers, and only exude compounds from their tips. Medium distance explorers have some rhizomorph formation and form 3 subtypes: fringe, mat, and smooth. Fringe subtype hyphae fan out from hairy rhizomorphs, which ramify and interconnect (e.g., Dermocybe cinnamomeolutea). Mat subtype hyphae have a limited range of exploration and rhizomorphs do not differentiate (e.g., Hysterangium stoloniferum). Smooth subtype hyphae have internally undifferentiated rhizomorphs with a central core of thick hyphae, with smooth mantles, and a few emanating hyphae (e.g., Thelephora terrestris) (Agerer, 2001). Short-distance explorers have a voluminous envelope of emanating hyphae without rhizomorph formation (e.g., Quercirhiza squamosal) (Agerer, 2001).
Hyphae have the ability to move carbon both horizontally, over long distances, extending well beyond the roots of trees and vertically, down the soil profile. Most ECM are found in the F and H soil layer (area of highly decomposed leaves beneath surface of forest floor, Figure 2), but also can be found in the mineral soil, whereas other ECM prefer decaying wood (Amaranthus and Perry, 1989; Tedersoo et al., 2003). Some ECM are able to mobilize minerals from rocks in soil (Landeweert et al., 2001), whereas others access nutrients from coarse woody debris (Amaranthus et al., 1994). Some ECM fungi also have saprophytic growth capabilities e.g., Tomentella sp. (Kõljalg et al., 2000). It is hypothesized that these ECM may switch to a saprophytic lifestyle when photosynthate C becomes scarce e.g., during winter (Courty et al., 2008).
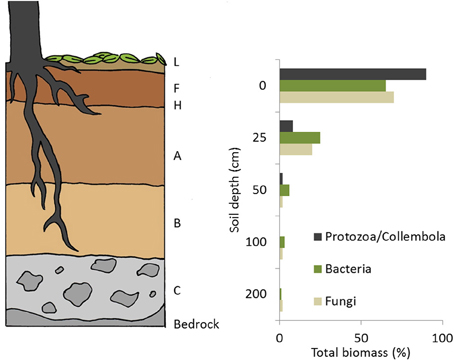
Figure 2. Diagram of soil profiles with depth and the relative proportion of collembola/protozoa, bacteria and fungi at each of these depths. L, characterized by the accumulation of organic matter; F: characterized by the accumulation of partially decomposed organic matter; H: characterized by the accumulation of decomposed organic matter where the original structure is indescernable. A: mineral horizon characterized by eluviation of materials in solution, or accumulation of organic matter, or both. B: mineral horizon characterized by enrichment of clay, organic matter, and iron and aluminium oxides or by in situ weathering. C: mineral horizon characterized by little or no alteration through the soil-forming processes, usually represents the parent material.
Arbuscular mycorrhizae do not form rhizomorphs and are considered to have five distinct hyphal architecture types (Figure 1). These include: infection networks, produced by spores and root fragments; germ tubes (only 20–30 mm long); hyphal bridges that connect runner-type hyphae and form patches of dense hyphal networks close to the root zone (Friese and Allen, 1991; Dodd et al., 2000); runner-types that expand rapidly through the soil or along roots (Mosse, 1962), seeking out new segments of roots to infect (Friese and Allen, 1991); and absorptive hyphal networks that explore the soil matrix for nutrients (Friese and Allen, 1991). Absorptive hyphal networks can extend 4–7 centimeters into the soil. Each network can have up to 8 branching orders, with each branch extending approximately 5 millimeters (Allen, 2007). Bago et al. (1998) described a 6th architectural form, where absorptive hyphae can form from runner hyphae, extending the potential range of nutrient absorption well beyond 4–7 centimeters. However, ECM EMM can extend even further from the roots as a result of rhizomorph formation. ECM rhizomorphs live, on average, 11 months, but have been observed to live for up to 7 years (Treseder et al., 2005). In contrast, AM hyphae only live on average 5–6 days (Staddon et al., 2003), suggesting the ECM dominated forests have greater C storage potential. The following section describes tree-rhizosphere C flow in greater detail.
Quantification and Characteristics of Mycorrhizosphere C Flow
Tree-Rhizosphere C Flow
Differences in root-associated fungi (both the presence/absence and type of fungal association) may be responsible for the large variation (10 X) in root exudation rates (Phillips et al., 2008, 2011). Exudation rates from root tips and hyphal tips tend to be greatest in the fine roots and in mycorrhizae that are allocated more C (Phillips et al., 2008, 2011). Carbon allocation to ECM hyphae will vary depending on ECM taxa (Bidartondo et al., 2001) and stage of colonization. For example, more C is allocated belowground during early stages of colonization (Cairney et al., 1989; Cairney and Alexander, 1992). Movement of recent photosynthates within EMM is not uniform, and will vary depending on the fungal species and their life stage (Cairney, 2012). Sun et al. (1999) demonstrated that ECM hyphal tips were active sites of exudation and re-adsorption of compounds, with little exudation along rhizomorphs. In addition, Leake et al. (2001) showed that more C was allocated to frontal tips of hyphae that occupied a hotspot of organic matter in soil. Infected ECM root tips may receive 42 times more carbon than uninfected root tips on the same plant (Cairney et al., 1989; Wu et al., 2002). Therefore there will be much patchiness in root exudate distribution in the forest floor, depending on root distribution and hyphal distribution.
Several techniques- including tree-girdling and stable-isotope labeling- have the potential to accurately measure the amount of C allocated belowground as well as the impact of root-exuded C on the microbial community. Tree girdling has demonstrated that labile C drives soil respiration (Högberg et al., 2001). Tree girdling stops the flow of photosynthates to tree roots, altering the availability and quality of C sources available to soil microbes in the rhizosphere (Subke et al., 2004; Högberg et al., 2007). However, how girdling affects the soil microbial community, particularly the bacterial community, is not consistent. Tree girdling caused significant decreases in the activity and biomass of the soil microbial community in boreal and temperate forests (Scott-Denton et al., 2006; Weintraub et al., 2007); this was mainly due to loss of ECM (45% decrease in ECM biomass relative to non-girdled plots) (Högberg and Högberg, 2002; Yarwood et al., 2009; Pena et al., 2010). The response of bacterial abundance and biomass to girdling has been marginal in boreal forests (Högberg et al., 2007; Yarwood et al., 2009) and in sub-tropical evergreen broadleaf forests (Li et al., 2009). Koranada et al. (2011) observed (using PLFA) a significant reduction in fungal biomass and Gram-positive bacterial biomass in girdled beech forests. As Gram-positive bacteria were less affected by exudates, Koranada et al. (2011) hypothesized that other effects of girdling treatments on rhizospheric conditions, such as alterations in oxygen supply, pH and redox potential (a result of the reduced root respiration or uptake of nutrients by plants) may have decreased Gram-positive bacterial populations. Other studies have shown no effect of girdling on microbial biomass or soil respiration; however in some of these studies trees re-sprouted (e.g., Eucalyptus), (Wu et al., 2011; Chen et al., 2012), and in other studies carbohydrates were still available in roots after girdling (Binkley et al., 2006). The increased availability of root carbohydrates may lead to a positive priming effects on SOM decomposition, increasing microbial community biomass and activity in the short-term (Subke et al., 2004; Scott-Denton et al., 2006). The variability of tree-girdling results may be the result of variation in tree-mycorrhizal species associations, or may be due to priming effects. Consequently developing non-destructive techniques may provide more insight into C-flow in forest ecosystems.
Natural-abundance stable-isotope ratios have recently been used to non-destructively investigate the flux of C from trees to the soil microbial community. In a second-growth coastal western hemlock forests in B.C. eighty-year-old Douglas-fir and western hemlock trees supplied C to the mycorrhizal symbionts for a distance up to ten meters (Churchland et al., 2013). Similarly, labeling of young trees with 13C-enriched CO2 has also been used to assess spatial and temporal C flux belowground. Epron et al. (2011) showed that there was rapid transfer of recent photosynthates to the mycorrhizosphere of beech (0.5–1 day), oak (0.5–1 day) and pine (1–2 days), and that the patterns of carbon allocation belowground varied seasonally in pine and beech, according to the phenology of the species. Similarly, Esperschütz et al. (2009) demonstrated using 13CO2 pulse-labeling and PLFA analysis, that the C in beech root exudates is first utilized by Gram-negative bacteria and mycorrhizal fungi. Stem-injection-labelling of mature trees has shown that C exudation from 22-year-old Sitka spruce in the field is rapid (24 h) and that these exudates are utilized first by fungi. The extent of influence of these trees exudates can be up to 20 m away from the base, and may, in part, be due to transport through EMM (Churchland et al., 2012). Although these techniques are too coarse to measure carbon movement in a single hypha, they show that C can move great distances away from the tree base and are utilized by the fungi and bacteria in the rhizosphere.
Root Exudates
Characterizing root exudation is challenging, but new techniques hold potential for breakthroughs. Most studies characterizing exudates released by different tree species have been microcosm studies conducted on seedlings in the laboratory under controlled conditions, either in hydroponic or sand systems, which do not scale up to mature trees and forests (Grayston et al., 1997). Hydroponic systems lack the physical substrates important for root growth; this affects exudation and can lead to re-uptake of exudates by plant roots. Studies in sand or soil systems are limited because of adsorption of exudates or degradation by the microbial community (Grayston et al., 1997, and references therein). There have been a few studies of tree root exudation in the field, mainly on young seedlings using either excavated root tips (which are surface-sterilized and placed in sterile tubes in the field) or soil extraction techniques. This latter approach has similar problems to the microcosms mentioned above (Phillips et al., 2008). In addition, it is difficult to extrapolate exudation rates from seedlings to mature trees, as a smaller portion (though, in total, a much greater amount) of recently-photosynthesized C is being allocated to the roots. Recently Shi et al. (2012) demonstrated an anion exchange membrane system that improved root exudate collection in situ from two-year-old radiata pine trees growing in large-scale biotrons. Because these anion exchange membranes rapidly adsorb root exudates there is little chance for consumption by microbes present in the biotron soil. This technique may result in a better understanding of root exudation from mature trees and forest stands.
The amount of C allocated to roots, root exudates, mycorrhizae and other rhizosphere microorganisms can change under different nutrient regimes and increase in the presence of specific microorganisms (Grayston et al., 1997). Ectomycorrhizal fungi influence both the quantity of C allocated to their roots, and the chemical composition of those exudates (van Schöll et al., 2006; Rineau and Garbaye, 2010). For example, ECM trees will allocate a third more C to their roots than non ECM trees (Durall et al., 1994; Rygiewicz and Anderson, 1994; Qu et al., 2004), likely because EMM have a large C demand (Rygiewicz and Anderson, 1994; Cairney and Burke, 1996; Cairney, 2012). Laboratory studies have shown that up to 29% of plant-assimilated C can be allocated to EMM (Rygiewicz and Anderson, 1994; Ek, 1997; Bidartondo et al., 2001). Environmental conditions also influence the degree to which tree roots are colonized, and likely mediate fluxes of labile C in forest soils (Meier et al., 2013). For instance, loblolly pine mass-specific exudation rates can vary by over three orders of magnitude under varying CO2 concentrations (Phillips et al., 2008, 2011). Plants have been observed to allocate more C to their roots and mycorrhizal symbionts under nutrient poor conditions (Zak et al., 1993; Franklin et al., 2012). In systems that are not N-limited, or in systems where N has been added, fungal biomass can decrease up to 45%, mainly due to decreased C allocation from trees to the mycorrhizal fungi (Högberg et al., 2007).
Root exudates represent semi-continuous input of labile C into soil, though exudation rates vary in time and space (Hinsinger et al., 2005), between deciduous and conifer species, over seasons (Collignon et al., 2011) and in different climates (Lin et al., 1999; Jones et al., 2004). Reviews on rhizodeposition from plants acknowledge the scant information on the character of exudates from trees (Grayston et al., 1997; Kuzyakov and Domanski, 2000; Neumann and Romheld, 2001; Jones et al., 2004). Plants are able to influence not only the quantity but also the composition of C exuded by their roots. This is thought to play a role in tree-microbe signaling and specificity in the rhizosphere. Production of enzymes, low-molecular-weight organic-acids (LMWOA), and other compounds support rhizosphere microbial communities (Bais et al., 2006). Root exudates also enhance nutrient availability by mobilizing poorly-soluble mineral-nutrients (Jones and Darrah, 1994; Marschner et al., 2011) and supplying labile-C substrates that increase rhizosphere microorganism activity and turnover (Phillips et al., 2012), ultimately influencing the decomposition of SOM (Rosling et al., 2004a,b). Most of the knowledge about the character of root exudates and how they may vary between tree species is on LMWOA and with ECM fungi (Cairney, 2012). In one of the few studies on carbohydrate characterization, Liebeke et al. (2009) used a gas-chromatograph-mass-spectrometer to reveal differences in the sugar content of soil extracts from different forest soils, demonstrating that oak soil contained mannitol and trehalose that was not present in beech soil. They hypothesized that the variation in sugar concentrations was responsible for differences in the bacterial communities under these tree species. There is increasing evidence that trees can actively restrict carbohydrate flow to their fungal partners. This is done through control of sucrose export and hydrolysis if the fungal partner does not deliver sufficient mineral nutrients (see review by Nehls et al., 2010).
Plant-Mycorrhizae Signaling Molecules
Several root exudates and hyphal exudates have the potential to induce mycorrhizal infection and change the microbial community structure of the rhizosphere. Secreted proteins, specifically a class of secreted proteins called effectors, have recently been established as plant-mycorrhizal signaling molecules (Lowe and Howlett, 2012). Effector proteins facilitate infection by suppressing immunity and/or inducing defense responses in plants (DeWit et al., 2009). For example, Laccaria bicolor was found to secrete the effector Mycorrhizal-Induced Small Secreted Protein 7 (MISSP7) during root colonization, in response to diffusible signals exuded from plant roots (Plett et al., 2011). Secretion and uptake of MISSP7 by the plant (via PI-3-P mediated endocytosis) affected cell wall chemistry, ultimately allowing hyphal penetration of the root apoplast. MISSP7 is the most upregulated protein during mycorrhization, and without it symbiosis does not occur (Plett et al., 2011). Following this discovery another effector protein, SP7, was uncovered (Maffei et al., 2012). Secreted by the AM fungi Gigaspora intraradices, SP7 interacts with a plant pathogenesis related transcription factor. SP7 was found to play a role in managing the formation of symbiosis with plant roots through the suppression of the plant immune system (Kloppholz et al., 2011). Plants have also been found to increase production of strigolactones under nutrient poor conditions (Maffei et al., 2012). Strigolactones have been found to induce fungal spore germination (Maffei et al., 2012) and hyphal branching (Bonfante and Requena, 2011), suggesting that plants might be signaling nearby mycorrhizae to promote infection. Much less is known about AM signaling, although recently it has been shown that AM fungi also produce active diffusible signals, similar to Nod factors released by rhizobia. These signals are needed for mycorrhizal formation (Bonfante and Requena, 2011). Similarly plant secreted effectors have also been found, which influence interactions between plant roots and free-living microorganisms (Hogenhout et al., 2009).
Modifications by ECM/AM on Exudates and Signals
Mycorrhizae modify the amount and composition of root exudates (van Schöll et al., 2006; Johansson et al., 2008, 2009), affecting exudation into the mycorrhizosphere and hyphosphere (Sun et al., 1999; Ahonen-Jonnarth et al., 2000; Jones et al., 2004; Johansson et al., 2008, 2009). The tips of growing ECM hyphae have been found to exude sugars, polyols, amino acids, peptides, proteins, hydroxamate siderophores, various LMWOA and pigments (growing front; Table 1) (Sun et al., 1999; Ahonen-Jonnarth et al., 2000; Jones et al., 2004; Johansson et al., 2008, 2009). Different ECM taxa vary the amount and composition of compounds exuded (Lapeyrie et al., 1987; Griffiths et al., 1994; van Schöll et al., 2006; Johansson et al., 2009; Tuason and Arocena, 2009). In general, the presence of ECM increases organic acid exudation (Johansson et al., 2008, 2009) and/or changes the type of organic acid exuded (van Schöll et al., 2006; Table 2). For example, van Hees et al. (2006a) found that Hebeloma crustuliniforme (ECM), when in symbiosis with Pinus sylvestris, exuded oxalate and ferricrocin and, to a lesser extent, malonate and acetate which were absent from non-mycorrhizal Scots pine soil.
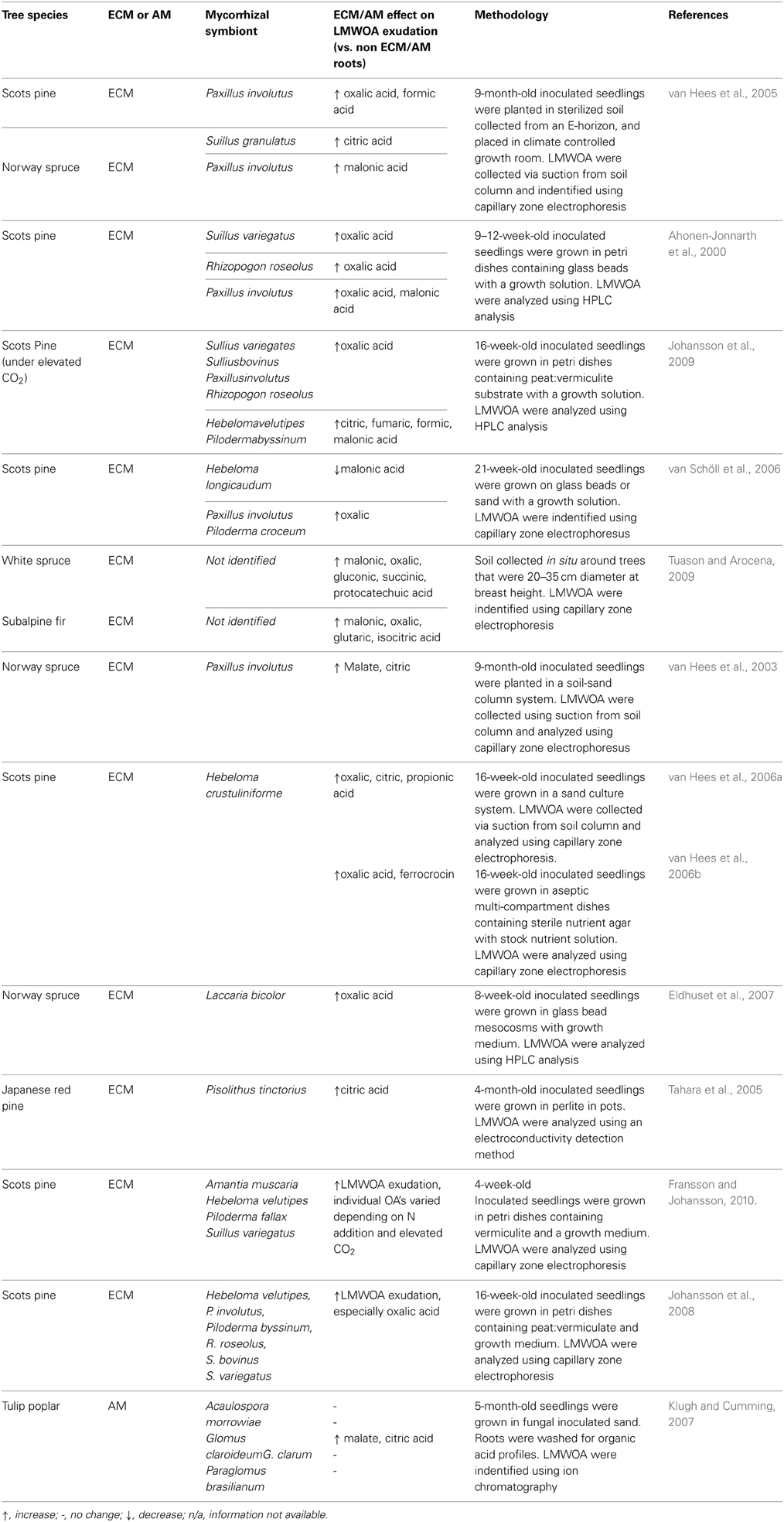
Table 2. Modification of low molecular weight organic acid (LMWOA) exudates from trees by different ectomycorrhizal (ECM) and arbuscular mycorrhizal (AM) fungi.
There is some evidence that hyphal exudates result in specific hyphosphere bacteria communities (Table 3; See Nazir et al., 2010 for list of bacterial-AM fungal relationships). It has been suggested that organic acids contribute to microbial selection in the mycorrhizosphere (de Boer et al., 2005). Differences in LMWOA ECM hyphal exudation are thought to be partially responsible for selecting specific microbial communities (Martin et al., 2008; Tuason and Arocena, 2009). Similarly, Toljander et al. (2007) found increased γ-proteobacteria abundance when extracted AM mycelial exudates were present, including formate, acetate, α and β glucose, and oligosaccharides. Trehalose has been reported to select specific bacterial communities in the mycorrhizosphere of several tree species including, Douglas-fir, Corsican pine and oak (Frey et al., 1997; Rangel-Castro et al., 2002; Izumi et al., 2006a,b; Uroz et al., 2007). Frey et al. (1997) suggested that the release of trehalose by the ECM fungus Laccaria bicolor exerts a nutrient-mediated selection on the surrounding bacteria. Specifically, trehalose has been found to have growth-promoting effects on the mycorrhization-helper bacteria (MHB), Pseudomonas monteilii, when inoculated with the ECM fungus Pisolithus albus in a plate-assay (Duponnois and Kisa, 2006). Trehalose released by the mycelium of Laccaria bicolor was shown to be a chemoattractant for Pseudomonas fluorescens BBc6R8 (Frey-Klett et al., 2007). At present it is not clear how hyphosphere microbial communities will impact a mycorrhizal's ability to acquire nutrients, but it is clear that exudation specificity has the potential to select for species-specific microbial communities.
Spatial and Seasonal Variation in Rhizosphere C Flow
Rhizosphere C flow varies spatially down the soil profile and horizontally with changes in root and hyphal distribution. Carbon flow also fluctuates seasonally and differs between coniferous and deciduous trees. In forest soil there is soil microbial community-composition stratification with depth due to a decrease in root biomass, root exudates, available C and a shift in SOM composition (Grayston et al., 1997; Berg et al., 1998; Fritze et al., 2000; Leckie et al., 2004; Lejon et al., 2005). Fungi are typically found in upper soil layers (Litter>Formulating >Humified, Figure 2) (Gardes and Bruns, 1993; Hirose et al., 2004). In contrast, actinomycete abundance has been shown to increase with depth (Fritze et al., 2000) while Gram–negative bacterial distribution is linked to root distribution (Soderberg et al., 2004). However, soil respiration rates and microbial activity are related to proximity to trees and tree roots (Churchland et al., 2013). In a free-air carbon-dioxide-enrichment (FACE) study, Phillips et al. (2008) showed that exudation rates could be predicted by the number of roots and mycorrhizal fine root tips (Pritchard et al., 2008b). This suggests that recent tree-carbon can be transported over large distances via roots and hyphae, supporting microbial communities meters away from the tree base.
There are different seasonal and physiological effects on rhizosphere C flow for deciduous and evergreen tree species. In a meta-analysis of C-allocation dynamics in trees Epron et al. (2012) showed that broadleaf trees exhibit, on average, 10 times higher rates of C transfer than coniferous species, although this varies depending on season. In spring (before bud break) and fall (during leaf senescence), broadleaves allocate a greater proportion of C to their roots (Epron et al., 2012). A number of studies have documented seasonal trends in soil microbial communities and activities in a variety of ecosystems (Allison and Treseder, 2008; Björk et al., 2008; Cruz-Martinez et al., 2009), including the coniferous forests of the Pacific Northwest (Brant et al., 2006; Moore-Kucera and Dick, 2008) and deciduous forests of Europe (Hibbard et al., 2005; Rasche et al., 2011). Studies specifically examining ECM fungi have found that their community structure, as well as enzymatic and metabolic capabilities, exhibit considerable temporal variation over a single year (Buée et al., 2005; Courty et al., 2007, 2008). This is likely related to differences in belowground C flow (Collignon et al., 2011). Burke et al. (2011) showed ECM, but not AM varied over a growing season in a mixed deciduous forest in Pennsylvania and that ECM and AM were associated with different enzyme activities involved in nutrient cycling. Specifically, AM fungi were associated with leucine aminopeptidase and urease, both enzymes involved in N acquisition. Arbuscular mycorrhizae were not traditionally considered able to supply their host with significant amounts of N (Smith and Read, 2008), though there is recent evidence that AM fungi can access both inorganic N (Fellbaum et al., 2012) and organic N (Whiteside et al., 2012) sources in forests. ECM were associated with most measured enzymes involved in C and N acquisition, but only during the late summer (Burke et al., 2011). This indicates that mycorrhizal ability to breakdown recalcitrant C and provide their host with N may vary seasonally.
Effects of Mycorrhizosphere C Flow on Other Organisms
Free-Living Fungi, Bacteria and Archaea
It is clear that variation in the quality and quantity of C released in root and hyphal exudates produced by different tree species can result in different rhizosphere and hyphosphere microbial communities and this varies between tree species associated with ECM and AM (Garbaye, 1991; Broeckling et al., 2008; Prescott and Grayston, 2013). Phillips and Fahey (2006) collected rhizosphere soil, bulk soil, and fine roots from the upper four centimeters of 12 monospecific tree species plots (six AM and six ECM tree species) planted on a common soil. The rhizosphere of AM trees and ECM trees were 10–12 and 25–30% more active (as measured by respired CO2) than bulk soil, demonstrating that ECM trees have a greater rhizosphere effect than AM trees. The magnitude of rhizosphere effects was negatively correlated with the degree of mycorrhizal colonization in AM tree species and with fine root biomass in ECM tree species. This suggests that different factors influence rhizosphere effects in tree species forming AM vs. ECM associations (Phillips and Fahey, 2006). Hyphal exudates from ECM tips support a diverse population of bacteria, archaea and fungi (Frey-Klett et al., 2007; Tedersoo et al., 2009; Bomberg et al., 2011). High throughput sequencing methods developed over recent years are enabling us to obtain much greater phylogenetic resolution to our studies of mycorrhizosphere microbial communities. For example, Kluber et al. (2010) used DNA sequencing to identify the rhizomorphic ECM mat-forming taxa (Hysterangium, Piloderma, Suillus and Russula species) in the forest floor and the hydrophobic mat-forming taxa (Gomphus and Ramaria species) in the mineral soil in a Douglas-fir forest. The two ECM mat forms had enhanced enzyme activities, specifically chitinase, phosphatase and phenol oxidase compared to non-mat forms in adjacent locations (Kluber et al., 2010). It was not established if the enhanced enzyme activity in the mats was the result of the ECM themselves or the distinctive bacteria and fungi in their mycorrhizosphere (Kluber et al., 2011). Bomberg and Timonen (2007, 2009) demonstrated (using PCR-DGGE of archaeal 16S rRNA genes) that there were specific archaeal communities in the ectomycorrhizosphere of several common boreal forest trees and that the type of ECM had the most influence on archaeal diversity. Bomberg et al. (2011) found no evidence of archaea in bulk humus samples lacking tree roots or ECM, indicating archaea are dependent on plant-derived C for growth. Similarly, Pires et al. (2012) used pyrosequencing and PCR-DGGE to reveal differences in archaeal richness between two mangrove species. Uroz et al. (2012) revealed (pyrosequencing 16S rRNA) that Alpha-, Beta-, and Gammaproteobacteria were significantly higher in the ectomycorrhizosphere of oak than in bulk soil and the bacterial communities found in the ectomycorrhizosphere of Xerocomus pruinatus and Scleroderma citrinum on oak were similar at the genus level, but different at the OTU level, demonstrating the specificity of the ectomycorrhizosphere. In the future, further refinements to molecular techniques, enhanced bioinformatic analysis and development of novel methods to culture and study these newly revealed organisms should enable links between these organisms and their functions to be elucidated. To date most of our knowledge on the role of associated microorganisms in the ectomycorrhizosphere has been based on studies of culturable organisms. The spectrum of plant-microbe relationships in the rhizosphere can range from mutualistic to pathogenic (Bais et al., 2006). Plant-growth-promoting rhizobacteria (PGPR)—which are found in the rhizosphere and mycorrhizosphere—benefit plants by creating biofilms that protect the root against pathogens (Akhtar and Siddiqui, 2009). These rhizosphere bacteria induce systemic acquired resistance (preparing the plant for attack; Pieterse et al., 2003) and enhance plant growth (Adesemoye et al., 2008; Yang et al., 2009). Several very good reviews have been written on PGPR (Vessey, 2003; Lugtenberg and Kamilova, 2009). There is some evidence of synergistic interactions between PGPR and mycorrhizal fungi, which may benefit the plants as a result of greater nutrient acquisition, inhibition of plant pathogens and greater mycorrhization (Artursson et al., 2006). Uroz et al. (2007) demonstrated positive interactions between ECM and bacteria that result in increased weathering of mineral nutrients, ultimately increasing nutrient uptake by the plant. AM have also been found to alter the structure of mycorrhizosphere microbial communities (Rillig and Mummey, 2006; Toljander et al., 2007; Welc et al., 2012). Isolation and identification of rhizobacteria found in the mycorrhizosphere around AM hyphae have shown bacteria with antagonistic properties toward soil-borne pathogens (Lioussanne et al., 2010), and antifungal properties (although they do not affect the AM symbiosis; Dwivedi et al., 2009). The N2 fixing ability of some AM plants improves when mycorrhizae are present vs. when they are absent (Kucey and Paul, 1982; Fitter and Garbaye, 1994).
Greater mycorrhization effects have been attributed to one specific group of PGPR, the so-called mycorrhization-helper bacteria (MHB) (Garbaye, 1994). Three life-stages in mycorrhizal fungi have been recognized, the free-living saprotrophic, the pre-infection stage and the symbiotic, mycorrhization stage (Deveau et al., 2007; Courty et al., 2008). During the pre-infection “free-living stage,” mycorrhizal fungi can interact with specific bacteria (e.g., Pseudomonas species) that are thought to enhance mycorrhizal establishment (Garbaye, 1994; Pivato et al., 2009). These mycorrhization-helper bacteria (MHB) can increase mycorrhization of a plant 1.2–17.5 times (Frey-Klett et al., 2007). Mycorrhization-helper bacteria are not plant-specific, but may be fungal-specific (Garbaye, 1994; Pivato et al., 2009). For example, Pseudomonas fluorescens BBc6R8 promotes survival of ECM Laccaria bicolour S238N when in its free-living stage, increasing radial fungal growth, hyphal density and branching angle. Mycorrhization-helper bacteria also change mycelial physiology from the free-living saprotrophic state to a “pre-symbiotic” stage (Deveau et al., 2007). During mycorrhization, a proliferation of bacteria can improve the receptivity of roots (Aspray et al., 2006), accelerate germination of fungal propagules in soil (Garbaye, 1994), and increase production of compounds such as auxofurans (Tylka et al., 1991) which have been shown to affect fungal metabolism and gene expression (Riedlinger et al., 2006). Mycorrhization-helper bacterial strains identified thus far include: Gram-negative Proteobacteria, Gram-positive Firmicutes and Gram-positive Actinomycetes (Frey-Klett et al., 2007) (Table 3). How MHB encourage mycorrhization is only beginning to be unraveled. Most MHB increase fungal colonization of the roots via: stimulating mycelia extension and branching (Garbaye, 1994; Poole et al., 2001; Schrey et al., 2005), increasing root-fungus contacts/colonization, and influencing soil environmental conditions (Frey-Klett et al., 2007). Mycorrhization-helper bacteria have been observed to stimulate spore germination of Glomus mosseae and Glomus clarum (AM) (Mosse, 1962; Xavier and Germida, 2003, respectively). In the case of Glomus clarum there may have been a complex bacterial consortium producing antagonistic volatiles (Tylka et al., 1991). The release of a number of different compounds, including gasses (Duponnois and Kisa, 2006) and secondary metabolites (e.g., auxofuran)(Keller et al., 2006; Riedlinger et al., 2006) by MHB have been shown to increase mycelial growth. Mycorrhization-helper bacteria are thought to reduce plant and mycorrhizal stress by detoxifying soil (e.g., Polyphenolic substances produced by Paxillus involutus are toxic to the fungus, but can be broken down by MHB; Duponnois and Garbaye, 1990). The potential for MHB to increase and support mycorrhizal infection has been demonstrated only under laboratory conditions. However, as in the case of PGPR, little is known about the effect these bacteria have on mycorrhization in situ.
Tree-Mycorrhizal-Microbial and Faunal Interactions
The term rhizosphere fauna has typically been used to refer to agricultural pests, specifically root herbivores (Bonkowski et al., 2009). However, rhizosphere fauna encompass a broad range of feeding types, including those that feed on bacteria, mycelium, and other fauna. Soil fauna influence the composition and activity of microbial populations by: directly grazing on bacteria and fungal hyphae, transporting fungal and microbial cells in their gut (thus facilitating microbial dispersion) and changing physical and chemical conditions of the soil (i.e., worm casts) (Oades, 2003). Several fungivorous collembola species have the capacity to influence development of Basidiomycete mycelia (Tordoff et al., 2008; Crowther et al., 2011b), and the extent of that influence is directly dependent on collembola density (Hanlon and Anderson, 1979; Kaneko et al., 1998). Setälä (1995) compared control soil (no fauna) and faunal-inoculated soil in Scots pine and silver birch microcosms. In all cases the presence of soil fauna reduced ECM abundance, reduced microbial biomass and increased shoot production. Faunal community impacts on decomposer fungi have also been shown to be density dependent, although there is evidence that the faunal community composition may have a greater impact on the microbial community. Crowther and A'Bear (2012) found that grazing pressures exerted by low-density woodlouse populations on saprotrophic fungi surpassed grazing pressures exerted by high density millipedes or high density collembola populations, ultimately limiting mycelial development. Grazing of mycelium not only influences microbial populations, but also has direct impacts on nutrient cycling because it increases enzyme release into the mycorrhizosphere (Crowther et al., 2011a), particularly in the presence of macrofauna (Crowther et al., 2011b). This increase will, in turn, affect soil nutrient availability (both N and P) and SOM turnover.
Mycophagous soil fauna grazing on AM and ECM in forests will affect C flow into the mycorrhizosphere by disrupting the movement of C along rhizomorphs and runner-type hyphae (Setälä, 1995; Coleman et al., 2004). Once removed from its C source, the growing hyphal front will stop releasing exudates, stop growing and potentially die off or convert to a saprotrophic life stage. The amount of hyphal grazing varies with mycorrhizal species, as soil fauna have been shown to be selective in their feeding preferences (Klironomos and Kendrick, 1996; Crowther and A'Bear, 2012). Klironomos and Kendrick (1996) showed that mites and collembola preferentially graze fungi growing on litter. However, when offered only AM growing on maple, they consume the fine hyphae most distant from the root. Cesarz et al. (2013) demonstrated that ECM vs. AM mycorrhizal-tree identity had a major influence on belowground nematode communities. Ash, which forms AM symbiosis, had greater populations of bacterial-feeding nematodes and lesser populations of fungal-feeding nematodes. In contrast beech, which forms EM symbiosis, had enhanced fungal-feeding nematode populations. Grazing on hyphal mycorrhizal networks can also significantly influence plant-C allocation belowground, and may influence C sequestration (Johnson et al., 2005).
Consequences of the Mycorrhizosphere on Soil C
Differences in tree-mycorrhizal symbiosis types may impact C-cycling and C sequestration because of differences in C allocation and longevity of these structures in soil. ECM trees with extensive mycelia have two to three times more C flux to the soil than AM trees (Finlay and Söderström, 1992; Phillips and Fahey, 2005; Pumpanen et al., 2009). This may be due to ECM roots being “more leaky,” possibly due to higher exudation rates (Phillips and Fahey, 2006). Pumpanen et al. (2009) demonstrated that roots and ECM growth account for 13–21% of recently assimilated C, whereas 9–26% of recently assimilated C is respired from the roots and rhizosphere. The turnover times of ECM and AM are also dramatically different; the turnover times of EMM and mycorrhizal fine roots are in the order of months to years (Cairney, 2012) and AM days to weeks (Langley and Hungate, 2003). The slower turnover of ECM is thought to be due to the chitin content of this fungal tissue, although in AM fungi the production of the glycoprotein glomalin can decrease AM hyphal turn over times significantly. Glomalin binds the soil matrix forming a soil aggregate within which AM hyphae are trapped and are slow to decompose, having an estimated residence time of 6–42 years (Rillig, 2004). These soil aggregates represent more the 5% of total soil C, significantly contributing to long term soil C sequestration (Wright and Upadhyaya, 1998; Rillig et al., 2001). However, the grazing of AM fungi is also higher than ECM because of the thin walls of AM fungi, which reduces residence times of this C in soil (Klironomos and Kendrick, 1996). Cheng et al. (2012) recently suggested that AM fungi diminish rather than enhance soil C pools in the short-term, as a result of accelerated decomposition of litter, when sites are exposed to elevated CO2. Although ECM production of proteolytic and lignolytic enzymes enables increased degradation of SOM (releasing more C) relative to AM (Read, 1992; Chalot and Brun, 1998), the recent study by Clemmensen et al. (2013) has suggested that accumulation and preservation of root and root-associated fungal residues is responsible for up to two-thirds of the C sequestered in boreal forests. This suggests that ECM dominated soils are more likely to sequester soil C, at least in the short term. However, long-term effects (decadal) may be qualitatively different from short-term effects; specifically there may be a long-term gain in recalcitrant compounds (Verbruggen et al., 2012).
ECM differ in nutrient uptake and transfer rates, altering the net primary production (NPP) of trees and may ultimately influence ecosystem C-cycling and C sequestration (Burgess et al., 1993). The ability of ECM to promote tree NPP varies depending on the extent of root colonization, the type of hyphae (Colpaert et al., 1992; Thomson et al., 1994) and the ability of the hyphae to acquire and transfer nutrients to the tree (Agerer, 2001). ECM fungi also have broad enzymatic capabilities (Chalot and Brun, 1998) that allow them to decompose labile and recalcitrant components of SOM, access organic sources of N, and transfer large amounts of N to host plants (Hobbie and Hobbie, 2006). AM fungi can also acquire substantial N from SOM (Hodge et al., 2010; Whiteside et al., 2012), although they do not have as broad an N-based enzymatic capability and appear to transfer only a small fraction of their host plants demand for N (Hodge and Fitter, 2010). This is particularly evident in dry soil conditions when N transport by roots is restricted, but soil N levels are still high (Tobar et al., 1994; Govindarajulu et al., 2005). There is some evidence that AM hyphae hydrolyze organic C at their root tips (Koide and Kabir, 2000), but there is limited evidence of AM derived phosphatases in the mycorrhizosphere along the hyphae (Joner et al., 2000). Belowground C allocation in AM-fungal-dominated ecosystems may not return sufficient N (or P) to offset the C investment by the tree, limiting the increase in NPP associated with greater atmospheric CO2 concentrations (Drake et al., 2011). Turnover of SOM has been shown to be faster in forest stands with AM mycorrhizal associations compared to ECM (Vesterdal et al., 2012). Phillips et al. (2013) proposed that forests dominated by AM and ECM associated trees vary in their C cycling and nutrient acquisition and may respond to global changes in predictable ways. They have proposed a new framework for predicting these variations in biogeochemical processes between forests [the Mycorrhizal-Associated Nutrient Economy model (MANE)] using forest inventory analysis maintained by the US Forest Service and previously described mycorrhizal designations (Brundrett et al., 1990; Wang and Qui, 2006). AM-dominated forest stands will have an inorganic nutrient economy resulting from elevated rates of C, N, and P mineralization and high quality litter. In contrast, ECM-dominated forest stands will have an organic nutrient economy as a result of slow rates of C, N, and P turnover and a lower quality litter. Thus further supports the hypothesis that ECM dominant forests will sequester more C.
ECM have the potential to act as a strong C sink, acquiring large amounts of C from their plant hosts (Smith and Read, 2008). The ECM then move the plant C to their hyphal tips, generating new biomass and exuding various compounds for nutrient acquisition. This movement of C can be a significant transport of plant C beyond the rhizosphere (Norton et al., 1990; Erland et al., 1991; Finlay and Söderström, 1992), and the recalcitrant chitinous cell wall of the mycelium will remain in the soil for months (Setälä et al., 1999; Treseder and Allen, 2000). The life-span of ECM root tips may be anywhere from 3 to 22 months (Orlov, 1960; Majdi et al., 2001), and may increase with soil depth (Pritchard et al., 2008a; McCormack et al., 2010). The consequences of C movement throughout the soil via hyphae is only beginning to be understood. Carbon will be transported out of the rhizosphere, moving as little as a few centimeters to as much as tens of meters (Gryta et al., 1997; Dunham et al., 2003; Murata et al., 2005; Churchland et al., 2012). However, long-distance, continuous, transport of C in hyphae is likely small as EMM are often fragmented, due to foraging by soil fauna (Dahlberg and Stenlid, 1995) and there are impermeable cell walls that form physiologically separated regions along hyphae (Olsson, 1999). However, C movement along hyphae would be very difficult to measure, and the potential impacts of the movement on C sequestration is large. Depending on forest type, climate and measurement methods, estimates of fungal biomass in ECM root tips can range from 20–10,000 kg/ha (Fogel and Hunt, 1979; Vogt et al., 1982; Dahlberg et al., 1997; Satomura et al., 2003; Sims et al., 2007; Helmisaari et al., 2009; Okada et al., 2011). The majority of this biomass is found in the forest floor and organic soil layers (Bååth et al., 2004; Wallander et al., 2004; Göransson et al., 2006), and constitute up to 1/3 of the total microbial biomass in forests (Swedish conifer forest; Högberg and Högberg, 2002). A recent study by Clemmensen et al. (2013) determined that 50–70% of stored C belowground was derived from root and root-associated microorganisms. Using 14C bomb-carbon modeling Clemmensen et al. (2013) found preservation of fungal residues in late-successional forests and in particular root-associated fungi, not saprotrophs, are the important regulators of ecosystem C dynamics. The sheer volume of tree C allocated belowground, and the ability of this C to move throughout the soil profile and soil ecosystem, shows how important it is to determine accurate C models of forests and other mycorrhizal-dominated soil ecosystems.
Conclusions
Carbon allocation to mycorrhizal hyphae enhances the degree to which tree C can impact soil microbial communities and soil C cycling. Different mycorrhizal morphotypes will vary the spatial distribution of this C considerably, although the vast, delicate nature of mycorrhizal hyphae makes this a difficult area of study. The greater C allocation to mycorrhizal roots, coupled with slower turnover times of mycorrhizal roots compared to non-mycorrhizal roots, hints at the potential of mycorrhizal associations to increase C sequestration in soil. However, differences between ECM and AM may impact soil C sequestration. ECM roots have longer turnover times than AM, and, due to their chitinous cell walls, are less likely to be grazed by fauna. Recent improvements in stable-isotope labeling and probing methods have resulted in a better understanding of the quantity and quality of C exuded belowground and spatial and temporal dynamics of C flow in forest soil. In addition whole-genome sequencing is showing us the large suite of important genes and signals involved in symbiotic associations. These new techniques should enable great strides to be made on our understanding of the role of different mycorrhizal functional groups in forest C cycling. We suggest the next step in developing this understanding would be tracing the flow C throughout the different hyphal morphotypes and measuring turnover times of this C to establish how ECM and AM distribute C in forest soil. This could be done through a combination of stable-isotope labeling and probing techniques conducted in large-scale controlled conditions, such as a biotron, coupled with nanosims technology to increase sensitivity and isotopic detection at high spatial resolution. We may then be able to determine the consequences of these variations for C-cycling and C sequestration.
Conflict of Interest Statement
The authors declare that the research was conducted in the absence of any commercial or financial relationships that could be construed as a potential conflict of interest.
References
Adesemoye, A. O., Torbert, H. A., and Kloepper, J. W. (2008). Enhanced plant nutrient use efficiency with PGPR and AMF in an integrated nutrient management system. Can. J. Microb. 54, 876–886. doi: 10.1139/W08-081
Agerer, R. (2001). Exploration types of ectomycorrhizae. a proposal to classify ectomycorrhizal mycelial systems according to their patterns of differentiation and putative ecological importance. Mycorrhiza 11, 107–114. doi: 10.1007/s005720100108
Ahonen-Jonnarth, U., van Hees, P. A. W., Lundström, U. S., and Finlay, R. D. (2000). Organic acids produced by mycorrhizal Pinus sylvestris exposed to elevated aluminium and heavy metal concentrations. New Phyt. 146, 557–567. doi: 10.1046/j.1469-8137.2000.00653.x
Akhtar, M. S., and Siddiqui, Z. A. (2009). Use of plant growth-promoting rhizobacteria for the biocontrol of root-rot disease complex of chickpea. Australas. Plant Path. 38, 44–50. doi: 10.1071/AP08075
Allen, M. F. (2007). Mycorrhixal fungi: highways for water and nutrients in arid soils. Vadose Zone J. 6, 291–297. doi: 10.2136/vzj2006.0068
Allison, S. D., and Treseder, K. K. (2008). Warming and drying suppress microbial activity and carbon cycling in boreal forest soils. Glob. Change Biol. 14, 2898–2909. doi: 10.1111/j.1365-2486.2008.01716.x
Amaranthus, M. P., and Perry, D. A. (1989). Rapid root tip and mycorrhiza formation and increased survival of Douglas-fir seedlings after soil transfer. New For. 3, 259–264. doi: 10.1007/BF00028933
Amaranthus, M., Trappe, J. M., Bednar, L., and Arthur, D. (1994). Hypogeous fungal production in mature Douglas-fir forest fragments and surrounding plantations and its relation to coarse woody debris and animal mycophagy. Can. J. For. Res. 24, 2157–2165. doi: 10.1139/x94-278
Artursson, V., Finlay, R. D., and Jansson, J. K. (2006). Interactions between arbuscular mycorrhizal fungi and bacteria and their potential for stimulating plant growth. Environ. Microbiol. 8, 1–10. doi: 10.1111/j.1462-2920.2005.00942.x
Aspray, T. J., Frey-Klett, P., Jones, J. E., Whipps, J. M., Garbaye, J., and Bending, G. D. (2006). Mycorrhization helper bacteria: a case of specificity for altering ectomycorrhiza architecture but not ectomycorrhiza formation. Mycorrhiza 16, 533–541. doi: 10.1007/s00572-006-0068-3
Bååth, E., Nilsson, L. O., Göransson, H., and Wallander, H. (2004). Can the extent of degradation of soil fungal mycelium during soil incubation be used to estimate ectomycorrhizal biomass in soil? Soil Biol. Biochem. 26, 2105–2109. doi: 10.1016/j.soilbio.2004.06.004
Bago, B., Azcon-Aguilar, C., and Piche, Y. (1998). Architecture and developmental dynamics of the external mecelium of the arbuscular mycorrhizal fungus Glomus intraradices grown under monoxenic conditions. Mycologia 90, 52–62. doi: 10.2307/3761011
Bais, H. P., Weir, T. L., Perry, L. G., Gilroy, S., and Vivanco, J. M. (2006). The role of root exudates in rhizosphere interactions with plants and other organisms. Annu. Rev. Plant Biol. 57, 233–266. doi: 10.1146/annurev.arplant.57.032905.105159
Bending, G. D., Poole, E. J., Whipps, J. M., and Read, D. J. (2002). Characterisation of bacteria from Pinus sylvestris-Suillus luteus mycorrhizas and their effects on root-fungus interactions and plant growth. FEMS Microbiol. Ecol. 39, 219–227. doi: 10.1016/S0168-6496(01)00215-X
Berg, B., Johansson, M., Meentemeyer, V., and Kratz, W. (1998). Decomposition of tree root litter in a climatic transect of coniferous forests in northern Europe: a synthesis. Scand. J. For. Res. 13, 402–412. doi: 10.1080/02827589809383000
Bidartondo, M. I., Ek, H., Wallander, H., and Söderström, B. (2001). Do nutrient additions alter carbon sink strength of ectomycorrhizal fungi? New Phyt. 151, 543–550. doi: 10.1046/j.1469-8137.2001.00180.x
Binkley, D., Stape, J. L., Takahashi, E. N., and Ryan, M. G. (2006). Tree-girdling to separate root and heterotrophic respiration in two eucalyptus stands in Brazil. Oecologia 148, 447–454. doi: 10.1007/s00442-006-0383-6
Björk, R. G., Björkman, M. P., Andersson, M. X., and Klemedtsson, L. (2008). Temporal variation in soil microbial communities in Alpine tundra. Soil Biol. Biochem. 40, 266–268. doi: 10.1016/j.soilbio.2007.07.017
Bomberg, M., Münster, U., Pumpanen, J., Ilvesniemi, H., and Heinonsalo, J. (2011). Archaeal communities in boreal forest tree rhizospheres respond to changing soil temperatures. Microb. Ecol. 62, 205–217. doi: 10.1007/s00248-011-9837-4
Bomberg, M., and Timonen, S. (2007). Distribution of cren- and Euryarchaeota in scots pine mycorrhizospheres and boreal forest humus. Microb. Ecol. 54, 406–416. doi: 10.1007/s00248-007-9232-3
Bomberg, M., and Timonen, S. (2009). Effect of tree species and mycorrhizal colonization on the archaeal population of boreal forest rhizospheres. Appl. Environ. Microbiol. 75, 308–315. doi: 10.1128/AEM.01739-08
Bonfante, P., and Genre, A. (2010). Mechanisms underlying beneficial plant-fungus interations in mycorrhizal symbiosis. Nat. Commun. 1, 48. doi: 10.1038/ncomms1046
Bonfante, P., and Requena, N. (2011). Dating in the dark: how roots respond to fungal signals to establish arbuscular mycorrhizal symbiosis. Curr. Opin. Plant Biol. 14, 451–457. doi: 10.1016/j.pbi.2011.03.014
Bonkowski, M., Villenave, C., and Giffiths, B. (2009). Rhizosphere faunal: the functional and structural diversity of intimate interactions of soil fauna with plant roots. Plant Soil 321, 213–233. doi: 10.1007/s11104-009-0013-2
Brachmann, A., and Parniske, M. (2006). The most widespread symbiosis on earth. PLoS Biol. 4:e239. doi: 10.1371/journal.pbio.0040239
Brant, J. B., Sulzman, E. W., and Myrold, D. D. (2006). Microbial community utilization of added carbon substrates in response to long-term carbon input manipulation. Soil Biol. Biochem. 38, 2219–2232. doi: 10.1016/j.soilbio.2006.01.022
Broeckling, C. D., Broz, A. K., Bergelson, J., Manter, D. K., and Vivanco, J. M. (2008). Root exudates regulate soil fungal community composition and diversity. Appl. Environ. Microbiol. 74, 738–744. doi: 10.1128/AEM.02188-07
Brundrett., M., Murase, G., and Kendrick, B. (1990). Comparative anatomy of roots and mycorrhizae of common ontario trees. Can. J. Bot. 68, 551–578. doi: 10.1139/b90-076
Bruns, T. D., Bidartondo, M. I., and Taylor, D. L. (2002). Host specificity in ectomycorrhizal communities: what do the exceptions tell us? Integr. Comp. Biol. 42, 352–359. doi: 10.1093/icb/42.2.352
Buée, M., Vairelles, D., and Garbaye, J. (2005). Year-round monitoring of diversity and potential metabolic activity of the ectomycorrhizal community in a beech (Fagus silvatica) forest subjected to two thinning regimes. Mycorrhiza 15, 235–245. doi: 10.1007/s00572-004-0313-6
Burgess, T. I., Malajczuk, N., and Groves, T. S. (1993). The ability of 16 ectomycorrhizal fungi to increase growth and phosphorus update of Eucalyptus globulus Labill. and E. diversicolor F. Muell. Plant Soil 153, 155–164. doi: 10.1007/BF00012988
Burke, D. J., Weintraub, M. N., Hewins, C. R., and Jalisz, S. (2011). Relationship between soil enzyme activities, nutrient cycling and soil fungal communities in a northern hardwood forest. Soil Biol. Biochem. 43, 795–803. doi: 10.1016/j.soilbio.2010.12.014
Cairney, J. W. G. (2012). Extramatrical mycelia of ectomycorrhizal fungi as moderators of carbon dynamics in forest soil. Soil Biol. Biochem. 47, 198–208. doi: 10.1016/j.soilbio.2011.12.029
Cairney, J. W. G., and Alexander, I. J. (1992). A study of ageing of spruce [Picea sitchensis(Bong.) Carr.] ectomycorrhizas. II. carbohydrate allocation in ageing Picea sitchensis/Tylospora fibrillosa (Burt.) Donk ectomycorrhizas. New Phyt. 122, 153–158. doi: 10.1111/j.1469-8137.1992.tb00061.x
Cairney, J. W. G., Ashford, A. E., and Allway, W. G. (1989). Distribution of photosynthetically fixed carbon within root systems of Eucalyptus pilularis ectomycorrhizal with Pisolithus tictorius. New Phyt. 112, 495–500. doi: 10.1111/j.1469-8137.1989.tb00343.x
Cairney, J. W. G., and Burke, R. M. (1996). Physiological heterogeneity within fungal mycelia: an important concept for a functional understanding of the ectomycorrhizal symbiosis. New Phyt. 134, 685–695. doi: 10.1111/j.1469-8137.1996.tb04934.x
Cesarz, S., Ruess, L., Jacob, M., Schaefer, M., and Scheu, S. (2013). Tree species diversity versus tree species identity: driving forces in structuring forest food webs as indicated by soil namatodes. Soil Biol. Biochem. 62, 36–45. doi: 10.1016/j.soilbio.2013.02.020
Chalot, M., and Brun, A. (1998). Physiology of organic nitrogen acquisition by ectomycorrhizal fungi and ectomycorrhizas. FEMS Microb. Rev. 22, 21–44. doi: 10.1111/j.1574-6976.1998.tb00359.x
Chapin, I., Stuart, F., MacFarland, J., McGuire, A. D., Euskirchen, E. S., Ruess, R. W., et al. (2009). The changing global carbon cycle: linking plant-soil carbon dynamics to global consequences. J. Ecol. 97, 840–850. doi: 10.1111/j.1365-2745.2009.01529.x
Chen, D. M., Zhou, L. X., Wu, J. P., Hsu, J. N., Lin, Y. B., and Fu, S. L. (2012). Tree girdling affects the soil microbial community by modifying resource availability in two subtropical plantations. Appl. Soil Ecol. 53, 108–115. doi: 10.1016/j.apsoil.2011.10.014
Cheng, L., Booker, F. L., Tu, C., Burkey, K. O., Zhou, L., Shew, H. D., et al. (2012). Arbuscular mycorrhizal fungi increase organic carbon decomposition under elevated CO2. Science 337, 1084–1087. doi: 10.1126/science.1224304
Churchland, C., Grayston, S. J., and Bengtson, P. (2013). Spatial variability of soil fungal and bacterial abundance: consequences for carbon turnover along a transition from a forested to clear-cut site. Soil Biol. Biochem. 63, 5–13. doi: 10.1016/j.soilbio.2013.03.015
Churchland, C., Weatherall, A., Briones, M. J. I., and Grayston, S. J. (2012). Stable-isotope labeling and probing of recent photosynthates into respired CO2, soil microbes and soil mesofauna using a xylem and phloem stem-injection technique on Sitka spruce (Picea sitchensis). Rapid Comm. Mass Spec. 26, 2493–2501. doi: 10.1002/rcm.6368
Clemmensen, K. E., Bahr, A., Ovaskainen, O., Dahlberg, A., Ekblad, A., Wallander, H., et al. (2013). Roots and associated fungi drive long-term carbon sequestration in boreal forests. Science 339, 1615–1618. doi: 10.1126/science.1231923
Coleman, D. C., Crossley, D. A. Jr., and Hendrix, P. F. (2004). Fundamentals of Soil Ecology, 2nd Edn. London: Elsevier inc.
Collignon, C., Uroz, S., Turpault, M. P., and Frey-Klett, P. (2011). Seasons differently impact the structure of mineral weathering bacterial communities in beech and spruce stands. Soil Biol. Biochem. 43, 2012–2022. doi: 10.1016/j.soilbio.2011.05.008
Colpaert, J. V., Vanassche, J. A., and Luijtens, K. (1992). The growth of the extramatrical mycelium of ectomycorrhizal fungi and the growth-response of Pinus sylvestris L. New Phyt. 120, 127–135. doi: 10.1111/j.1469-8137.1992.tb01065.x
Courty, P. E., Breda, N., and Garbaye, J. (2007). Relationship between oak tree phenology and the secretion of organic matter degrading enzymes by Lactarius quietus ectomycorrhizas before and during bud break. Soil Biol. Biochem. 39, 1655–1663. doi: 10.1016/j.soilbio.2007.01.017
Courty, P. E., Franc, A., Pierrat, J. C., and Garbaye, J. (2008). Temporal changes in the ectomycorrhizal community in two soil horizons of a temperate oak forest. App. Enviro. Microb. 74, 5792–5801. doi: 10.1128/AEM.01592-08
Crowther, T. W., and A'Bear, A. D. (2012). Impacts of grazing soil fauna on decomposer fungi are species-specific and density-dependent. Fungal Ecol. 5, 277–281. doi: 10.1016/j.funeco.2011.07.006
Crowther, T. W., Boddy, L., and Jones, T. H. (2011b). Species-specific effects of soil fauna on fungal foraging and decomposition. Oecologia 167, 535–545. doi: 10.1007/s00442-011-2005-1
Crowther, T. W., Jones, T. H., Boddy, L., and Baldrian, P. (2011a). Invertebrate grazing determines enzyme production by basidiomycete fungi. Soil Biol. Biochem. 43, 2060–2068. doi: 10.1016/j.soilbio.2011.06.003
Cruz-Martinez, K., Suttle, K. B., Brodie, E. L., Power, M. E., Andersen, G. L., and Banfield, J. F. (2009). Despite strong seasonal responses, soil microbial consortia are more resilient to long-term changes in rainfall than overlying grassland. ISME J. 3, 738–744. doi: 10.1038/ismej.2009.16
Dahlberg, A., Jonsson, L., and Nylund, J. E. (1997). Species diversity and distribution of biomass above and belowground among ectomycorrhizal fungi in an old-growth Norway spruce forest in south Sweden. Can. J. Bot. 75, 1323–1335. doi: 10.1139/b97-844
Dahlberg, A., and Stenlid, J. (1995). Spatiotemporal patterns in ectomycorrhizal populations. Can. J. Bot. 73, S1222–S1230. doi: 10.1139/b95-382
Dakora, F. D., and Phillips, D. A. (2002). Root exudates as mediators of mineral acquisition in low-nutrient environments. Plant Soil 245, 35–47. doi: 10.1023/A:1020809400075
de Boer, W., Folman, L. B., Summerbell, R. C., and Boddy, L. (2005). Living in a fungal world: impact of fungi on soil bacteria niche development. FEMS Microb. Rev. 29, 795–811. doi: 10.1016/j.femsre.2004.11.005
Deveau, A., Palin, B., Delarulle, C., Peter, M., Kohler, A., Pierrat, J. C., et al. (2007). The mycorrhiza helper Psuedomonas fluoresces BBc6R8 has a specific priming effect on the growth, morphology and gene expression of the ectomycorrhizal fungus Laccaria bicolor S238N. New Phytol. 1175, 743–755. doi: 10.1111/j.1469-8137.2007.02148.x
DeWit, P. J. G. M., Mehrabi, R., Van Den Burg, H. A., and Stergiopoulos, I. (2009). Fungal effector proteins: past present and future. Mol. Plant Patho. 10, 735–747. doi: 10.1111/j.1364-3703.2009.00591.x
Dodd, J. C., Boddington, C. L., Rodriguez, A., Gonzalez-Chavez, V., and Mansur, I. (2000). Mycelium of arbuscular mycorrhizal fungi (AMF) from different genera: form, function and detection. Plant Soil 226, 131–151. doi: 10.1023/A:1026574828169
Drake, J. E., Gallet-Budynek, A., Hofmockel, K. S., Bernhardt, E. S., Billings, S. A., Jackson, R. B., et al. (2011). Increases in the flux of carbon belowground stimulate nitrogen uptake and sustain the long-term enhancement of forest productivity under elevated CO2. Ecol. Lett. 14, 349–357. doi: 10.1111/j.1461-0248.2011.01593.x
Dunham, S. M., Kretzer, A., and Pfrender, M. E. (2003). Characterization of pacific golden chanterelle (Cantjarellus formosus) genet size using co-dominant microsatellite markers. Mol. Ecol. 12, 1607–1618. doi: 10.1046/j.1365-294X.2003.01837.x
Dunstan, W. A., Malajczuk, N., and Dell, B. (1998). Effects of bacteria on mycorrhizal development and growth of container grown Eucalyptus diversicolor F. Muell. seedlings. Plant Soil 201, 241–249. doi: 10.1023/A:1004329626763
Duponnois, R., and Garbaye, J. (1990). Some mechanisms involved in growth simulation of ectomycorrhizal fungi by bacteria. Can. J. Bot. 68, 2148–2152. doi: 10.1139/b90-280
Duponnois, R., and Garbaye, J. (1991). Mycorrhization helper bacteria associated with the Douglas-fir laccaria-laccata symbiosis: effects in aseptic and in glasshouse conditions. Annal. Sci. For. 48, 239–251. doi: 10.1051/forest:19910301
Duponnois, R., and Kisa, M. (2006). The possible roles of trehalose in the mycorrhiza helper effect. Can. J. Bot. 84, 1005–1008. doi: 10.1139/b06-053
Duponnois, R., and Plenchette, C. (2003). A mycorrhiza helper bacterium enhances ectomycorrhizal and endomycorrhizal symbiosis of Australian Acacia species. Mycorrhiza 13, 85–91. doi: 10.1007/s00572-002-0204-7
Durall, D. M., Jones, M. D., and Tinker, P. B. (1994). Allocation of 14C-carbon in ectomycorrhizal willow. New Phyt. 128, 109–114. doi: 10.1111/j.1469-8137.1994.tb03993.x
Dwivedi, D., Johri, B. N., Ineichen, K., Wray, V., and Wiemken, A. (2009). Impact of antifungals producing rhizobacteria on the performance of Vigna radiata in the presence of arbuscular mycorrhizal fungi. Mycorrhiza 19, 559–570. doi: 10.1007/s00572-009-0253-2
Edwards, I. P., and Zak, D. R. (2010). Chronic simulated atmospheric N deposition alters actinobacterial community composition in forest floor and surface soil. Soil Sci. Soc. Am. J. 74, 1157–1166. doi: 10.2136/sssaj2009.0240
Eisenhauer, S. D., Beßler, H., Engels, C., Gleixner, G., Habekost, H., Milcu, A., et al. (2010). Plant diversity effects on soil microorganisms support the singular hypothesis. Ecology 91, 485–496. doi: 10.1890/08-2338.1
Ek, H. (1997). The influence of nitrogen fertilization on the carbon economy of Paxillus involutus in ectomycorrhizal association with Betula pendula. New Phyt. 135, 133–142. doi: 10.1046/j.1469-8137.1997.00621.x
Eldhuset, T. D., Swensen, B., Wickstrom, T., and Wollebaek, G. (2007). Organic acids in root exudates from Picea abies seedlings influenced by mycorrhiza and aluminum. J. Plant Nut. Soil Sci. 170, 645–648. doi: 10.1002/jpln.200700005
Epron, D., Bahn, M., Derrien, D., Lattanzi, F. A., Pumpanen, J., Gessler, A., et al. (2012). Pulse-labelling trees to study carbon allocation dynamics: a review of methods, current knowledge and future prospects. Tree Physiol. 32, 776–798. doi: 10.1093/treephys/tps057
Epron, D., Ngao, J., Dannoura, M., Bakker, M. R., Zeller, B., Bazot, S., et al. (2011). Seasonal variation of belowground carbon transfer assessed by in situ (CO2)-C-13 pulse labelling of trees. Biogeosciences 8, 1153–1168. doi: 10.5194/bg-8-1153-2011
Erland, S., Finlay, R., and Söderström, B. (1991). The influence of substrate pH on carbon translation in ectomycorrhizal and non-mycorrhizal pine seedlings. New Phyt. 119, 235–242. doi: 10.1111/j.1469-8137.1991.tb01026.x
Esperschütz, J. A., Gattinger, A., Buegger, F., Lang, H., Munch, J., Schloter, M., et al. (2009). A continuous labelling approach to recover photosynthetically fixed carbon in plant tissue and rhizosphere organisms of young beech trees (Fagus sylvatica L.) using 13C depleted CO2. Plant Soil 323, 21–29. doi: 10.1007/s11104-009-9998-9
Falkowski, P., Scholes, R. J., Boyle, E., Canadell, J., Canfield, D., Elser, J., et al. (2000). The global carbon cycle: a test of our knowledge of earth as a system. Science 290, 291–296. doi: 10.1126/science.290.5490.291
Fellbaum, C. R., Gachomo, E. W., Beesetty, Y., Choudhari, S., Strahan, G. D., Pfeffer, P. E., et al. (2012). Carbon availability triggers fungal nitrogen uptake and transport in arbuscular mycorrhizal symbiosis. Proc. Nat. Acad. Sci. U.S.A. 109, 2666–2671. doi: 10.1073/pnas.1118650109
Finlay, R. D. (2008). Ecological aspects of mycorrhizal symbiosis: with special emphasis on the functional diversity of interactions involving the extraradical mycelium. J. Exp. Biol. 59, 1115–1126. doi: 10.1093/jxb/ern059
Finlay, R. D., and Rosling, A. (2006). “Integrated nutrient cycles in forest ecosystems, the role of ectomycorrhizal fungi,” in Fungi in Biogeochemical Cycles, ed G. M. Gadd (Cambridge: Cambridge University Press), 28–50. doi: 10.1017/CBO9780511550522.003
Finlay, R., and Söderström, B. (1992). “Mycorrhiza and carbon flow to the soil,” in Mycorrhizal Functioning: and Integrative Plant-Fungal Process, ed M. F. Allen (New York, NY: Chapman and Hall), 134–160.
Fitter, A. H., and Garbaye, J. (1994). Interactions between mycorrhizal fungi and other soil organisms. Plant Soil 159, 123–132.
Fogel, R., and Hunt, G. (1979). Fungal and arboreal biomass in western oregon douglas-fir ecosystems: distribution patterns and turnover. Can. J. For. Res. 9, 245–256. doi: 10.1139/x79-041
Fontaine, S., Mariotti, A., and Abbadie, L. (2003). The priming effect of organic matter: a question of microbial competition? Soil Biol. Biochem. 35, 837–843. doi: 10.1016/S0038-0717(03)00123-8
Founoune, H., Duponnois, R., Ba, A. M., Sall, S., Branget, I., Lorquin, J., et al. (2002a). Mycorrhiza helper bacteria stimulated ectomycorrhizal symbiosis of Acacia holosericea with Pisolithus alba. New Phytol. 153, 81–89. doi: 10.1046/j.0028-646X.2001.00284.x
Founoune, H., Duponnois, R., Meyer, J. M., Thioulouse, J., Masse, D., Chotte, J. L., et al. (2002b). Interactions between ectomycorrhizal symbiosis and fluorescent pseudomonads on Acacia holosericea: isolation of mycorrhiza helper bacteria (MHB) from a Soudano-Sahelian soil. FEMS Microbiol. Ecol. 41, 37–46. doi: 10.1111/j.1574-6941.2002.tb00964.x
Franklin, O., Johansson, J., Dewar, R. C., Dieckmann, U., McMurtrie, R. E., Brännström, Å., et al. (2012). Modeling carbon allocation in trees: a search for principles. Tree Physiol. 32, 648–666. doi: 10.1093/treephys/tpr138
Fransson, P. M. A., and Johansson, E. M. (2010). Elevated CO2 and nitrogen influence exudation of soluble organic compounds by ectomycorrhizal root systems. FEMS Microbiol. Ecol. 71, 186–196. doi: 10.1111/j.1574-6941.2009.00795.x
Frey, P., Frey-Klett, P., Garbaye, J., Berge, O., and Heulin, T. (1997). Metabolic and genotypic fingerprinting of fluorescent pseudomonas associated with the Douglas-fir-Laccaria bicolor mycorrhizosphere. Appl. Environ. Microb. 63, 1852–1860.
Frey-Klett, P., Garbaye, J., and Tarkka, M. (2007). The mycorrhiza helper bacteria revisited. New Phyt. 176, 22–36. doi: 10.1111/j.1469-8137.2007.02191.x
Friese, C. F., and Allen, M. F. (1991). The spread of VA mycorrhizal fungal hyphae in the soil—Inoculum types and external hyphal architecture. Mycologia 83, 409–418. doi: 10.2307/3760351
Fritze, H., Pietikainen, J., and Pennanen, T. (2000). Distribution of microbial biomass and phospholipid fatty acids in Podzol profiles under coniferous forest. Eur. J. Soil Sci. 51, 565–573. doi: 10.1046/j.1365-2389.2000.00346.x
Garbaye, J. (1991). Biological interactions in the mycorrhizosphere. Experentia 47, 370–375. doi: 10.1007/BF01972079
Garbaye, J. (1994). Helper bacteria- a new dimension to the mycorrhizal symbiosis. New Phyt. 128, 197–210. doi: 10.1111/j.1469-8137.1994.tb04003.x
Garbaye, J., and Bowen, G. D. (1989). Stimulation of ectomycorrhizal infection of Pinus-radiata by some microorganisms associated with the mantle of ectomycorrhizas. New Phytol. 112, 383–388. doi: 10.1111/j.1469-8137.1989.tb00327.x
Gardes, M., and Bruns, T. D. (1993). ITS primers with enhanced specificity for basidiomycetes—application to the identification of mycorrhizae and rusts. Mol. Ecol. 2, 113–118. doi: 10.1111/j.1365-294X.1993.tb00005.x
Göransson, H., Wallander, H., Ingerslev, M., and Rosengren, U. (2006). Estimating the relative nutrient uptake from different soil depths in Quercus robur, Fagus sylvatica and Picea abies. Plant Soil 286, 87–97. doi: 10.1007/s11104-006-9028-0
Govindarajulu, M., Pfeffer, P. E., Jin, H. R., Abubaker, J., Douds, D. D., Allen, J. W., et al. (2005). Nitrogen transfer in the arbuscular mycorrhizal symbiosis. Nature 435, 819–823. doi: 10.1038/nature03610
Grayston, S. J., Vaughan, D., and Jones, D. (1997). Rhizosphere carbon flow in trees, in comparison with annual plants: the importance of root exudation and its impact on microbial activity and nutrient availability. App. Soil Ecol. 5, 29–56. doi: 10.1016/S0929-1393(96)00126-6
Griffiths, R. P., Baham, J. E., and Caldwell, B. A. (1994). Soil solution chemistry of ectomycorrhizal mats in forest soil. Soil Biol. Biochem. 26, 331–337. doi: 10.1016/0038-0717(94)90282-8
Gryta, H., Debaud, J.-C., Effose, A., Gay, G., and Marmeisse, R. (1997). Fine-Scale structure of populations of the ecotomycorrhizal fungus Hebeloma cylindrosporum in coastal sand dune forest ecosystems. Mol. Ecol. 6, 353–364. doi: 10.1046/j.1365-294X.1997.00200.x
Hanlon, R. D. G., and Anderson, J. M. (1979). Effects of collembola grazing on microbial activity in decomposing leaf litter. Oecologia 38, 93–99. doi: 10.1007/BF00347827
Helmisaari, H. S., Ostonen, I., Lõhmus, K., Derome, J., Lindroos, A. J., Merilä, P., et al. (2009). Ectomycorrhizal root tips in relation to site and stand characteristics in Norway spruce and Scots pine stands in boreal forests. Tree Physiol. 29, 445–456. doi: 10.1093/treephys/tpn042
Hibbard, K. A., Law, B. E., Reichstein, M., and Sulzman, J. (2005). An analysis of soil respiration across northern hemisphere temperate ecosystems. Biogeochem 73, 29–70. doi: 10.1007/s10533-004-2946-0
Hinsinger, P., Gobran, G. R., Gergory, P. J., and Wenzel, W. W. (2005). Rhizosphere geometry and heterogeneity arising from root-mediated physical and chemical processes. New Phyt. 168, 293–303. doi: 10.1111/j.1469-8137.2005.01512.x
Hirose, D., Kikuchi, J., Kanzaki, N., and Futai, K. (2004). Genet distribution of sporocarps and ectomycorrhizas of Sullis pictus in a Japanese white pine plantation. New Phyt. 164, 527–554. doi: 10.1111/j.1469-8137.2004.01188.x
Hobbie, J. E., and Hobbie, E. A. (2006). N-15 in symbiotic fungi and plants estimates nitrogen and carbon flux rates in Arctic tundra. Ecology 87, 816–822. doi: 10.1890/0012-9658(2006)87[816:NISFAP]2.0.CO;2
Hodge, A., and Fitter, A. H. (2010). Substantial nitrogen acquisition by arbuscular mycorrhizal fungus accelerates decomposition and acquires nitrogen direction from organic material. Nature 413, 297–299. doi: 10.1038/35095041
Hodge, A., Helgason, T., and Fitter, A. H. (2010). Nutritional ecology of arbuscular mycorrhizal fungi. Fungal Ecol. 3, 267–273. doi: 10.1016/j.funeco.2010.02.002
Hodge, A., Robinson, D., and Fitter, A. H. (2000). An arbuscular mycorrhizal inoculum enhances root proliferation in, but not nitrogen capture from, nutrient-rich patches in soil. New Phyt. 145, 575–584. doi: 10.1046/j.1469-8137.2000.00602.x
Högberg, M. N., and Högberg, P. (2002). Extramatrical ectomycorrhizal mycelium contributes one-third of microbial biomass and produces, together with associated roots, half the dissolved organic carbon in a forest soil. New Phyt. 154, 791–795. doi: 10.1046/j.1469-8137.2002.00417.x
Högberg, M. N., Högberg, P., and Myrold, D. D. (2007). Is microbial community composition in Boreal forest soils determined by pH, C-to-N ratio, the trees, or all three? Oecologia 150, 590–601. doi: 10.1007/s00442-006-0562-5
Högberg, P., Nordgren, A., Buchmann, N., Taylor, A. F. S., Ekblad, A., Högberg, M. N., et al. (2001). Large-scale forest girdling shows that current photosynthesis drives soil respiration. Nature 411, 789–792. doi: 10.1038/35081058
Hogenhout, S. A., Van der Hoorn, R. A. L., Terauchi, F., and Kamoun, S. (2009). Emerging concepts in effector biology of plant-associated organisms. Mol. Plant Microbe Interact. 22, 115–122. doi: 10.1094/MPMI-22-2-0115
Hrynkiewicz, K., Baum, C., Niedojadlo, J., and Dahm, H. (2009). Promotion of mycorrhiza formation and growth of willows by the bacterial strain Sphingomonas sp. 23L on fly ash. Biol. Fertil. Soils 45, 385–394. doi: 10.1007/s00374-008-0346-7
Ishida, T. A., Nara, K., and Hogetsu, T. (2007). Host effects on ectomycorrhizal fungal communities: insight from eight host species in mixed conifer-broadleaf forests. New Phyt. 174, 430–440. doi: 10.1111/j.1469-8137.2007.02016.x
Izumi, H., Anderson, I. C., Alexander, I. J., Killam, K., and Moore, E. R. B. (2006a). Diversity and expression of nitrogenase genes (nifH) from ectomycorrhizas of Corsican pine (Pinus nigra). Environ. Microbiol. 8, 2224–2230. doi: 10.1111/j.1462-2920.2006.01104.x
Izumi, H., Anderson, I. C., Alexander, I. J., Killam, K., and Moore, E. R. B. (2006b). Endobacteria in some ectomycorrhiza of Scots pine (Pinus sylvestris). FEMS Microb. Ecol. 56, 34–43. doi: 10.1111/j.1574-6941.2005.00048.x
Jobbágy, E. G., and Jackson, R. B. (2000). The vertical distribution of soil organic carbon and its relation to climate and vegetation. Ecol. App. 10, 423–436. doi: 10.1890/1051-0761(2000)010[0423:TVDOSO]2.0.CO;2
Johansson, E. M., Fransson, P. M. A., Finlay, R. D., and van Hees, P. A. W. (2009). Quantitative analysis of soluble exudates produced by ectomycorrhizal roots as a response to ambient and elevated CO2. Soil Biol. Biochem. 41, 1111–1116. doi: 10.1016/j.soilbio.2009.02.016
Johansson, J. F., Fransson, P. M. A., Finlay, R. D., and van Hees, P. A. W. (2008). Quantitative analysis of root and ectomycorrhizal exudates as a response to Pb, Cd and As stress. Plant Soil 313, 39–54. doi: 10.1007/s11104-008-9678-1
Johansson, J. F., Paul, L. R., and Finlay, R. D. (2004). Microbial interactions in the mycorrhizosphere and their significance for sustainable agriculture. FEMS Microb. Ecol. 48, 1–13. doi: 10.1016/j.femsec.2003.11.012
Johnson, D., Krsek, M., Wellington, M. H., Stott, A. W., Cole, L., Bardgett, R. D., et al. (2005). Soil invertebrates disrupt carbon flow through fungal networks. Science 309, 1047. doi: 10.1126/science.1114769
Joner, E. J., van Aarle, I. M., and Vosatka, M. (2000). Phosphatase activity of extra-radical arbuscular mycorrhizal hyphae: a review. Plant Soil 226, 199–210. doi: 10.1023/A:1026582207192
Jones, D. L., and Darrah, P. R. (1994). Role of root derived organic-acids in the mobilization of nutrients from the rhizosphere. Plant Soil 313, 39–54.
Jones, D. L., Hodge, A., and Kuzyakov, Y. (2004). Plant and mycorrhizal regulation of rhizodeposition. New Phyt. 163, 459–480. doi: 10.1111/j.1469-8137.2004.01130.x
Kaneko, N., McLean, M. A., and Parkinson, D. (1998). Do mites and Collembola affect pine litter fungal biomass and microbial respiration? Appl. Soil Ecol. 9, 209–213. doi: 10.1016/S0929-1393(98)00077-8
Kataoka, R., Siddiqui, Z. A., Taniguchi, T., and Futai, K. (2009). Quantification of Wautersia [Ralstonia] basilensis in the mycorrhizosphere of Pinus thunbergii Parl. and its effect on mycorrhizal formation. Soil Biol. Biochem. 41, 2147–2152. doi: 10.1016/j.soilbio.2009.07.027
Keller, S., Schneider, K., and Suessmuth, R. D. (2006). Structure elucidation of auxofuran, a metabolite involved in stimulating grwoth of fly agaric, produced by the mycorrhiza helper bacterial streptomyces AcH 505. J. Antibiot. 59, 801–803. doi: 10.1038/ja.2006.106
Kennedy, P. G., Izzo, A. D., and Bruns, T. D. (2003). There is high potential for the formation of common mycorrhizal networks between understory and canopy trees in a mixed evergreen forest. J. Ecol. 91, 1071–1080. doi: 10.1046/j.1365-2745.2003.00829.x
Klironomos, J. N., and Kendrick, W. B. (1996). Palatability of microfungi to soil arthropods in relation to the functioning of arbuscular mycorrhizae. Biol. Fert. Soils 21, 43–52. doi: 10.1007/BF00335992
Kloppholz, S., Kuhn, H., and Requena, N. (2011). A secreted fungal effector of Glomus intraradices promotes symbiotic biotrophy. Curr. Biol. 21, 1204–1209. doi: 10.1016/j.cub.2011.06.044
Kluber, L. A., Smith, J. E., and Myrold, D. D. (2011). Distinctive fungal and bacterial communities are assocaited with mats formed by ectomycorrhizal fungi. Soil Biol. Biochem. 43, 1042–1050. doi: 10.1016/j.soilbio.2011.01.022
Kluber, L. A., Tinnesand, K. M., Caldwell, B. A., Dunham, S. M., Yarwood, R. R., Bottomley, P. J., et al. (2010). Ectomycorrhizal mats alter forest soil biogeochemistry. Soil Biol. Biochem. 42, 1607–1613. doi: 10.1016/j.soilbio.2010.06.001
Klugh, K. R., and Cumming, J. R. (2003). Variation in organic acid exudates among mycorrhizal species colonizing Liriodendron tulipifera L. (yellow-poplar) in the presence of aluminum. 88th Annual Meeting of the Ecological Society of America held Jointly with the International Society for Ecological Modeling-North American Chapter. Ecol. Soc. Am. Ann. Meet. Abstr. 88, 186.
Klugh, K. R., and Cumming, J. R. (2007). Variations in organic acid exudation and aluminum resistance among arbuscular mycorrhizal species colonizing Liriodendron tulipifera. Tree Physiol. 27, 1103–1112.
Koide, R. T., and Kabir, Z. (2000). Extraradical hyphae of the mycorrhizal fungus Glomus intraradices can hydrolyse organic phosphate. New Phyt. 148, 511–517. doi: 10.1046/j.1469-8137.2000.00776.x
Kõljalg, U., Dahlberg, A., Taylor, A. F. S., Larsson, E., Hallenberg, N., Stenlid, J., et al. (2000). Diversity and abundance of Resupinate thelephoroid fungi as ectomycorrhizal symbionts in Swedish boreal forests. Mol. Ecol. 9, 1985–1996. doi: 10.1046/j.1365-294X.2000.01105.x
Koranada, M., Schnecker, J., Kaiser, C., Fuchslueger, L., Kitzler, B., Stange, C. F., et al. (2011). Microbial processes and community composition in the rhizosphere of European beech—The influence of plant C exudates. Soil Biol. Biochem. 43, 551–558. doi: 10.1016/j.soilbio.2010.11.022
Kourtev, P. S., Ehrenfeld, J. G., and Haggblom, M. (2002). Exotic plant species alter microbial structure and function in the soil. Ecology 83, 3152–3166. doi: 10.1890/0012-9658(2002)083[3152:EPSATM]2.0.CO;2
Kucey, R. M. N., and Paul, E. A. (1982). Carbon flow, photosynthesis and N2 fixation in mycorrhizal and nodulated faba beans (Vicia faba L). Soil Biol. Biochem. 14, 407–412. doi: 10.1016/0038-0717(82)90013-X
Kuzyakov, Y., and Domanski, G. (2000). Carbon input by plants into the soil. Review. J. Plant Nutr. Soil Sci. 4, 421–431. doi: 10.1002/1522-2624(200008)163:4<421::AID-JPLN421>3.0.CO;2-R
Kuzyakov, Y., and Gavrichkova, O. (2010). Time lag between photosynthesis and CO2 efflux from soil: a review of mechanisms and controls. Glob. Change Biol. 16, 3386–3406. doi: 10.1111/j.1365-2486.2010.02179.x
Landeweert, R., Hofflund, E., Finlay, R. D., and van Breeman, N. (2001). Linking plants to rocks: ectomycorrhizal fungi mobilize nutrients from minerals. Trends Ecol. Evol. 16, 248–254. doi: 10.1016/S0169-5347(01)02122-X
Langley, J. A., and Hungate, B. A. (2003). Mycorrhizal controls on belowground litter quality. Ecol. 84, 2301–2312. doi: 10.1890/02-0282
Lapeyrie, F., Chilvers, G. A., and Bhem, C. A. (1987). Oxalic acid synthesis by the ectomycorrhizal fungus Paxillus involutus. New Phyt. 106, 139–146. doi: 10.1111/j.1469-8137.1987.tb04797.x
Leake, J. R., Donnelly, D. P., Saunders, E. M., Boddy, L., and Read, D. J. (2001). Rates and quantities of carbon flux to ectomycorrhizal mycelium following 14C pulse labeling of Pinus sylvestris seedlings: effects of litter patches and interaction with a wood-decomposer fungus. Tree Physiol. 21, 71–82. doi: 10.1093/treephys/21.2-3.71
Leckie, S. E., Prescott, C. E., Grayston, S. J., Neufeld, J. D., and Mohn, W. W. (2004). Characterization of humus microbial communities in adjacent forest types that differ in nitrogen availability. Microb. Ecol. 48, 29–40. doi: 10.1007/s00248-003-1020-0
Lejon, D. P. H., Chausson, R., Ranger, J., and Ramjard, L. (2005). Microbial community structure and density under different tree species in an acid forest soil. Microb. Ecol. 50, 614–625. doi: 10.1007/s00248-005-5130-8
Leyval, C., and Berthelin, J. (1993). Rhizodeposition and net release of soluble organic-compounds by pine and beech seedlings inoculated with rhizobacteria and ectomycorrhizal fungi. Biol. Fertil. Soils 15, 259–267. doi: 10.1007/BF00337210
Li, Y. J., Yang, X. D., Zou, X. M., and Wu, J. H. (2009). Responses of soil nematode communities to tree girdling in a subtropical evergreen broad-leaved forest of southwest China. Soil Biol. Biochem. 41, 877–882. doi: 10.1016/j.soilbio.2008.07.031
Liebeke, M., Brozel, V. S., Hecker, M., and Lalk, M. (2009). Chemical characterization of soil extract as growth media for the ecophysiological study of bacteria. Appl. Microb. Biotech. 83, 161–173. doi: 10.1007/s00253-009-1965-0
Lin, G., Ehleringer, J. R., Rygiewicz, P. T., Johson, M. G., and Tingey, D. T. (1999). Elevated CO2 and temperature impacts on different components of soil CO2 efflux in Douglas-fir terracosms. Glob. Change Biol. 5, 157–168. doi: 10.1046/j.1365-2486.1999.00211.x
Lindahl, B. D., Ihrmark, K., Boberg, J., Trumbore, S., Högberg, P., Stenlid, J., et al. (2007). Spatial separation of litter decomposition and mycorrhizal nitrogen uptake in boreal forests. New Phyt. 173, 611–620. doi: 10.1111/j.1469-8137.2006.01936.x
Lioussanne, L., Perrault, F., Jolicoeur, M., and St-Arnaud, M. (2010). The bacterial community of tomato rhizosphere is modified by inoculation with arbuscular mycorrhizal fungi but unaffected by soil enrichment with mycorrhizal root exudates or inoculation with Phytophthora nicotianae. Soil Biol. Biochem. 42, 473–483. doi: 10.1016/j.soilbio.2009.11.034
Litton, C. M., and Giardina, C. P. (2008). Below-ground carbon flux and partitioning: global patterns and response to temperature. Func. Ecol. 22, 941–954. doi: 10.1111/j.1365-2435.2008.01479.x
Litton, C. M., Raich, J. W., and Ryan, M. G. (2007). Carbon allocation in forest ecosystems. Glob. Change. Biol. 13, 2089–2109. doi: 10.1111/j.1365-2486.2007.01420.x
Lowe, R. G. T., and Howlett, B. J. (2012). Indifferent, affectionate or deceitful: lifestyles and secretomes of fungi. PLoS Pathog. 8:e1002515. doi: 10.1371/journal.ppat.1002515
Lugtenberg, B., and Kamilova, F. (2009). Plant-growth-promoting-rhizobacteria. Annl. Rev. Microb. 63, 541–556. doi: 10.1146/annurev.micro.62.081307.162918
Maffei, M. E., Arimura, G. I., and Mithofer, A. (2012). Natural elicitors, effectors and modulators of plant responses. Nat. Prod. Rep. 29, 1288–1303. doi: 10.1039/c2np20053h
Majdi, H., Damm, E., and Nylund, J. E. (2001). Longevity of mycorrhizal roots depends on branching order and nutrient availability. New Phyt. 150, 195–202. doi: 10.1046/j.1469-8137.2001.00065.x
Marschner, P., Crowley, D., and Rengel, Z. (2011). Rhizosphere interactions between microorganisms and plants govern iron and phosphorus acquisition along the root axis—model and research methods. Soil Biol. Biochem. 43, 883–894. doi: 10.1016/j.soilbio.2011.01.005
Martin, F., Aerts, A., Ahren, D., Brun, A., Duchaussoy, F., Kohler, A., et al. (2008). The genome sequence of the basidiomycete fungus Laccaria bicolor provides insights into the mycorrhizal symbiosis. Nature 452, 88–92. doi: 10.1038/nature06556
Martin, F., Kohler, A., and Duplessis, S. (2007). Living in harmony in the wood underground: ectomycorrhizal genomics. Curr. Opin. Plant Biol. 10, 204–210. doi: 10.1016/j.pbi.2007.01.006
Martin, F., Kohler, A., Murat, C., Balestrini, R., Coutinho, P. M., Jaillon, O., et al. (2010). Périgord black truffle genome uncovers evolutionary origins and mechanisms of symbiosis. Nature 464, 1033–1038. doi: 10.1038/nature08867
McCormack, M. L., Pritchard, S. G., Breland, S., Davis, M. A., Prior, S. A., Runion, B., et al. (2010). Soil fungi respond more strongly than fine roots to elevated CO2 in a model regenerating longleaf pine-wiregrass ecosystem. Ecosystems 13, 901–916. doi: 10.1007/s10021-010-9360-3
Meier, I. C., Avids, P. G., and Phillips, R. P. (2013). Fungal communities influence root exudation rates in pine seedlings. FEMS Microb. Ecol. 83, 585–595. doi: 10.1111/1574-6941.12016
Mencuccini, M., and Holtta, T. (2010). The significance of phloem transport for the speed with which canopy photosynthesis and belowground respiration are linked. New Phyt. 185, 189–203. doi: 10.1111/j.1469-8137.2009.03050.x
Molina, R., and Trappe, J. M. (1982). Patterns of Ectomycorrhizal host specificity and potential among Pacific Northwest conifers and fungi. For. Sci. 28, 423–458.
Moore-Kucera, J., and Dick, R. P. (2008). PLFA profiling of microbial community structure and seasonal shifts in soils of a Douglas-fir chronosequence. Microb. Ecol. 55, 500–511. doi: 10.1007/s00248-007-9295-1
Mosse, B. (1962). The establishment of vesicular-arbuscular mycorrhiza under aseptic conditions. J. Gen. Microb. 27, 509–520. doi: 10.1099/00221287-27-3-509
Murata, H., Ohta, A., Yamada, A., Narimatsu, M., and Futamura, N. (2005). Genetic mosaics in the massive persisting rhizosphere colony “shiro” of the ectomycorrhizal basidiomycete Tricholoma matsutake. Mycorrhiza 15, 505–512. doi: 10.1007/s00572-005-0358-1
Nazir, R., Warmink, J. A., Boersma, H., and van Elsas, J. D. (2010). Mechanisms that promote bacterial fitness in fungal-affected soil microhabitats. FEMS Microb. Ecol. 71, 169–185. doi: 10.1111/j.1574-6941.2009.00807.x
Nehls, U., Gohringer, F., Wittulsky, S., and Dietz, S. (2010). Fungal carbohydrate support in the ectomycorrhizal symbiosis: a review. Plant Biol. 12, 292–301. doi: 10.1111/j.1438-8677.2009.00312.x
Neumann, G., and Romheld, V. (2001). “The release of root exudates as affect by the plant's physiological status,” in The Rhizosphere: Biochemistry and Organic Substances at the Soil-Plant Interface, eds R. Pinto, Z. Varanini, and P. Nannipieri (New York, NY: Dekker), 41–93.
Norton, J. M., Smith, J. L., and Firestone, M. K. (1990). Carbon flow in the rhizosphere of ponderosa pine seedlings. Soil Biol. Biochem. 22, 449–455. doi: 10.1016/0038-0717(90)90177-2
Oades, J. M. (2003). The Role of Biology in the Formation, Stabilization and Degradation of Soil Structure. Wageningen: International workshop on methods of research in soil structure, soil biota interrelationships in Wageningen.
Okada, K., Okada, S., Yasue, K., Fukuda, M., and Yamada, A. (2011). Six-year monitoring of pine ectomycorrhizal biomass under a temperate monsoon climate indicates significant annual fluctuations in relation to climatic factors. Ecol. Res. 26, 411–419. doi: 10.1007/s11284-011-0800-0
Olsson, S. (1999). “Nutrient translocation and electrical signalling in mycelia,” in The Fungal Colony, eds N. A. R. Gow, G. D. Robson, and G. M. Gadd (Cambridge: Cambridge University Press), 25–48. doi: 10.1017/CBO9780511549694.003
Orlov, A. (1960). Growth and changes with age of feeder roots of Picea excelsa Link. Bot. Z. 45, 888–896.
Pena, R., Offermann, C., Simon, J., Naumann, P. S., Gessler, A., Hlost, J., et al. (2010). Girdling affects ectomycorrhizal fungi (EMF) diversity and reveals functional differences in EMF community composition in a beech forest. App. Environ. Microb. 76, 1831–1841. doi: 10.1128/AEM.01703-09
Phillips, R. P., Brzostej, E., and Midgleym, M. G. (2013). The mycorrhizal associated nutrient economy: a new framework for predicting carbon-nutrient couplings in temperate forests. New Phytol. 199, 41–51. doi: 10.1111/nph.12221
Phillips, R. P., Erlitz, Y., Bier, R., and Bernhardt, E. S. (2008). New approach for capturing soluble root exudate in forest soils. Func. Ecol. 22, 990–999. doi: 10.1111/j.1365-2435.2008.01495.x
Phillips, R. P., and Fahey, T. J. (2005). Patterns of rhizosphere carbon flux in sugar maple (Acer saccharum) and yellow birch (Betula allegheniensis) saplings. Glob. Change. Biol. 11, 983–995. doi: 10.1111/j.1365-2486.2005.00959.x
Phillips, R. P., and Fahey, T. J. (2006). Tree species and mycorrhizal associations influence the magnitude of rhizosphere effects. Ecology 87, 1302–1313. doi: 10.1890/0012-9658(2006)87[1302:TSAMAI]2.0.CO;2
Phillips, R. P., Finzi, A. C., and Bernhardt, E. S. (2011). Enhanced root exudation induces microbial feedbacks to N cycling in a pine forest under long-term CO2 fumigation. Ecol. Lett.14, 1887–1894. doi: 10.1111/j.1461-0248.2010.01570.x
Phillips, R. P., Meier, I. C., Bernhardt, E. S., Grandy, A. S., Wickings, K., and Finzi, A. C. (2012). Roots and fungi accelerate carbon and nitrogen cycling in forest exposed to elevated CO2. Ecol. Lett. 15, 1042–1049. doi: 10.1111/j.1461-0248.2012.01827.x
Pieterse, C. M. J., Van Pelt, J. A., Verhagen, B. W. M., Ton, J., van Wees, S. C. M., Leon-Kloosterziel, K. M., et al. (2003). Induced systemic resistance by plant growth-promoting rhizobacteria. Symbiosis 35, 39–54.
Pires, A. C. C., Cleary, D. F. R., Almeida, A., Cunha, A., Dealtry, S., Mendonca-Hagler, L. C. S., et al. (2012). Denaturing gradient gel electrophoresis and barcoded pyrosequencing reveal unprecedented archaeal diversity in mangrove sediment and rhizosphere samples. App. Enviro. Microb. 78, 5520–5528. doi: 10.1128/AEM.00386-12
Pivato, B., Offre, P., Marchelli, S., Barbonaglia, B., Mougel, C., Lemanceau, P., et al. (2009). Bacterial effects on arbuscular mycorrhizal fungi and mycorrhiza development as influenced by the bacteria, fungi, and host plant. Mycorrhiza 19, 81–90. doi: 10.1007/s00572-008-0205-2
Plett, J. M., Kemppainen, M., Kale, S. D., Kohler, A., Legue, V., Brun, A., et al. (2011). A secreted effector protein of Laccaria Bicolor is required for symbiosis development. Curr. Biol. 21, 1197–1203. doi: 10.1016/j.cub.2011.05.033
Podila, G. K., Sreedasyam, A., and Muratet, M. A. (2009). Populus rhizosphere and the ectomycorrhizal interactome. Crit. Rev. Plant Sci. 28, 359–367. doi: 10.1080/07352680903241220
Poole, E. J., Bending, G. D., Whipps, J. M., and Read, D. J. (2001). Bacteria associated with Pinus sylvestri-Lactarius rufus ectomycorrhizas and their effects on mycorrhiza formation in vitro. New Phyt. 151, 743–751. doi: 10.1046/j.0028-646x.2001.00219.x
Prescott, C. E., and Grayston, S. J. (2013). Tree species influence on microbial communities in litter and soil: current knowledge and research needs. For. Ecol. Manag. 309, 19–27. doi: 10.1016/j.foreco.2013.02.034
Pritchard, S. G., Strand, A. E., McCormack, M. L., Davis, M. A., Finzi, A. C., Jackson, R. B., et al. (2008b). Fine root dynamics in a loblolly pine forest are influenced by Free-Air-CO2-Enrichment (FACE): a six year minirhizotron study. Glob Change Biol. 7, 829–837. doi: 10.1111/j.1365-2486.2007.01523.x.
Pritchard, S. G., Strand, A. E., McCormack, M. L., Davis, M. A., and Oren, R. (2008a). Mycorrhizal and rhizomorph dynamics in a loblolly pine forest during 5 years of free-air-CO2-enrichment. Glob. Change Biol. 14, 1–13. doi: 10.1111/j.1365-2486.2008.01567.x
Pritsch, K., Munch, J. C., and Buscot, F. (1997). Morphological and anatomical characterization of black alder Alnus glutinosa (L.) Gaertn. ectomycorrhizas. Mycorrhiza 7, 201–216. doi: 10.1007/s005720050182
Pumpanen, J. S., Heinonsalo, J., Rasilo, T., Hurme, K., and Ilvesniemi, H. (2009). Carbon balance and allocation of assimilated CO2 in Scots pine, Norway spruce, and Silver birch seedlings determined with gas exchange measurements an C-14 pulse labelling. Tree Struct. Func. 23, 611–621. doi: 10.1007/s00468-008-0306-8
Qu, L. Y., Shinano, T., Quoresji, A. M., Tamai, Y., Osaki, M., and Koike, T. (2004). Allocation of 14C carbon in two species of larch seedlings infected with ectomycorrhizal fungi. Tree Physiol. 24, 1369–1376. doi: 10.1093/treephys/24.12.1369
Rangel-Castro, J. I., Dannell, E., and Pfeffer, P. E. (2002). A C-13-NMR study of exudation and storage of carbohydrates and amino acids in the ectomycorrhizal edible mushroom Chantharellus cibarius. Mycologia 94, 190–199. doi: 10.2307/3761795
Rasche, F., Knapp, D., Kaiser, C., Koranada, M., Kitzler, B., Zechmeister-Boltenstern, S., et al. (2011). Seasonality and resource availability control bacterial and archeal communities in soils of a temperate beech forest. ISME J. 5, 389–402. doi: 10.1038/ismej.2010.138
Rasmann, S., and Agrawal, A. A. (2008). In defense of roots: a research agenda for studying plant resistance to belowground herbivory. Plant Physiol. 146, 875–880. doi: 10.1104/pp.107.112045
Read, D. J. (1992). “The mycorrhizal mycelium,” in Mycorrhizal Functioning: an Integrative Plant-Fungus Process, ed M. J. Allen (New York, NY: Chapmas and Hall), 102–133.
Read, D. J., and Perez-Moreno, J. (2003). Mycorrhizas and nutrient cycling in ecosystems—a journey towards relevance? New Phyt. 157, 475–492. doi: 10.1046/j.1469-8137.2003.00704.x
Reynolds, H. L., Hartley, A. E., Vogelsang, K. M., Bever, J. D., and Schultz, P. A. (2005). Arbuscular mycorrhizal fungi do not enhance nitrogen acquisition and growth of old-field perennials under low nitrogen supply in glasshouse culture. New Phyt. 167, 869–880. doi: 10.1111/j.1469-8137.2005.01455.x
Riedlinger, J., Schrey, S. D., Tarkka, M. T., Hampp, R., Kapur, M., and Fiedler, H. P. (2006). Auxofuran, a novel substance stimulating growth of fly agaric produced by the mycorrhiza helper bacteria Streptomycetes AcH 505. App. Enviro. Micro. 72, 3550–3557. doi: 10.1128/AEM.72.5.3550-3557.2006
Rillig, M. C. (2004). Arbuscular Mycorrhizae and terrestrial ecosystem processes. Ecol. Lett. 7, 740–754. doi: 10.1111/j.1461-0248.2004.00620.x
Rillig, M. C., and Mummey, D. L. (2006). Mycorrhizas and soil structure. New Phyt. 171, 41–53. doi: 10.1111/j.1469-8137.2006.01750.x
Rillig, M. C., Wright, S. F., Nichils, K. A., Schmidt, W. F., and Torn, M. S. (2001). Large contribution of arbuscular mycorrhizal fungi to soil carbon pools in tropical forest soils. Plant Soil. 233, 167–177. doi: 10.1023/A:1010364221169
Rineau, F., and Garbaye, J. (2010). Effects of liming on potential oxalate secretion and iron chelation of beech ectomycorrhizal root tips. Microb. Ecol. 60, 331–339. doi: 10.1007/s00248-010-9697-3
Rosling, A., Lindahl, B. D., and Finlay, R. D. (2004a). Carbon allocation to ectomycorrhizal roots and mycelium colonizing different mineral substrates. New Phyt. 162, 795–802. doi: 10.1111/j.1469-8137.2004.01080.x
Rosling, A., Lindahl, B. D., Taylor, A. F. S., and Finlay, R. D. (2004b). Mycelia growth and substrate acidification of ectomycorrhizal fungi in response to different minerals. FEMS Microb. Ecol. 47, 31–37. doi: 10.1016/S0168-6496(03)00222-8
Rygiewicz, P. T., and Anderson, C. P. (1994). Mycorrhizae alter quality and quantity of carbon allocated below ground. Nature 369, 58–60. doi: 10.1038/369058a0
Satomura, T., Nakatsubo, T., and Horikoshi, T. (2003). Estimation of the biomass of fine roots and mycorrhizal fungi: a cases study in a Japanese red pine (Pinus densiflora) stand. J. For. Res. 8, 221–225. doi: 10.1007/s10310-002-0023-x
Schrey, S. D., Schellhammer, M., Ecke, M., Hampp, R., and Tarkka, M. T. (2005). Mycorrhiza helper bacterium Streptomycetes sp. induces differential gene expression in the ectomycorrhizal fungus Amanita muscaria. New Phyt. 168, 205–216. doi: 10.1111/j.1469-8137.2005.01518.x
Scott-Denton, L. E., Rosensteil, T. N., and Monson, R. K. (2006). Differential controls by climate and substrate over the heterotrophic and rhizosphereic components of soil respiration. Glob. Change Biol. 12, 205–216. doi: 10.1111/j.1365-2486.2005.01064.x
Setälä, H. (1995). Growth of birch and pine seedlings in relation to grazing by soil fauna on ectomycorrhizal fungi. Ecology 76, 1844–1851. doi: 10.2307/1940716
Setälä, H., Kulmala, P., Mikola, J., and Markkola, A. M. (1999). Influence of ectomycorrhiza on the structure of detrital food webs in pine rhizosphere. Oikos 87, 113–122. doi: 10.2307/3547002
Shi, S. J., O'Callaghan, M., Jones, E. E., Richardson, A. E., Walter, A., Stewart, A., et al. (2012). Investigation of organic anions in tree root exudates and rhizosphere microbial communities using in situ and destructive sampling techniques. Plant Soil 359, 149–163. doi: 10.1007/s11104-012-1198-3
Sims, S. F., Hendricks, J. J., Mitchell, R. J., Kuehn, K. A., and Pecot, S. D. (2007). Nitrogen decreases and precipitation increases ectomycorrhizal extrametrical mycelia production in a longleaf pine forest. Mycorrhiza 17, 299–309. doi: 10.1007/s00572-007-0105-x
Soderberg, K. H., Probanza, A., Jumpponen, A., and Bååth, E. (2004). The microbial community in the rhizosphere determined by community-level physiological profiles (CLPP) and direct soil- and cfu-PLFA techniques. App. Soil Ecol. 25, 135–145. doi: 10.1016/j.apsoil.2003.08.005
Staddon, P. L., Ramsey, C. B., Ostle, N., Ineson, P., and Fitter, A. H. (2003). Rapid turnover of hyphae of mycorrhizal fungi determined by AMS microanalysis of C-14. Science 300, 1138–1140. doi: 10.1126/science.1084269
Subke, J. A., Hahn, V., Battipaglia, G., Linder, S., Buchmann, N., and Cotrufo, M. F. (2004). Feedback interactions between needle litter decomposition and rhizosphere activity. Oecologia 139, 551–559. doi: 10.1007/s00442-004-1540-4
Subke, J. A., Vallack, H. W., Magnusson, T., Keel, S. G., Metcalfe, D. B., Högberg, P., et al. (2009). Short-term dynamics of abiotic and biotic soil 13CO2 effluxes after in situ 13CO2 pulse labelling of a boreal pine forest. New Phyt. 183, 349–357. doi: 10.1111/j.1469-8137.2009.02883.x
Sun, Y.-P., Unestam, T., Lucas, S. D., Johanson, K. J., Kenne, L., and Finlay, R. D. (1999). Exudation-reabsorption in mycorrhizal fungi, the dynamic interface for interaction with soil and other microorganisms. Mycorrhiza 9, 137–144. doi: 10.1007/s005720050298
Tahara, K., Norisada, M., Tange, T., Yagi, H., and Kojima, K. (2005). Ecotomycorrhizal association enhances Al tolerance by inducing citrate secretion in Pinus densiflora. Soil Sci. Plant Nut. 51, 397–403. doi: 10.1111/j.1747-0765.2005.tb00045.x
Talbot, J. M., Allison, S. D., and Treseder, K. K. (2008). Decomposers in disguise: mycorrhizal fungi as regulators of soil C dynamics in ecosystems under global change. Func. Ecol. 22, 955–963. doi: 10.1111/j.1365-2435.2008.01402.x
Taylor, A. F. S., and Alexander, I. (2005). The ecotomycorrhizal symbiosis: life in the real world. Mycologist 19, 102–112. doi: 10.1017/S0269-915X(05)00303-4
Tedersoo, L., Jairus, T., Horton, B. M., Abarenkov, K., Suvi, T., Saar, I., et al. (2008). Strong host preference of ectomycorrhizal fungi in a Tasmanian wet sclerophyll forest as revealed by DNA barcoding and taxon-specific primers. New Phyt. 180, 479–490. doi: 10.1111/j.1469-8137.2008.02561.x
Tedersoo, L., Kõljalg, U., Hallenberg, N., and Larsson, K. H. (2003). Fine scale distribution of ectomycorrhizal fungi and roots across substrate layers including coarse woody debris in a mixed forest. New Phyt. 159, 153–165. doi: 10.1046/j.1469-8137.2003.00792.x
Tedersoo, L., Partel, K., Jairus, T., Gates, G., Poldmaa, K., and Tamm, H. (2009). Ascomycetes associated with ectomycorrhizas: molecular diversity and ecology with particular reference to the helotiales. Environ. Microb. 11, 3166–3178. doi: 10.1111/j.1462-2920.2009.02020.x
Thomson, B. D., Grove, T. S., Malajcuzuk, N., and Hardy, G. E. S. (1994). The effectiveness of ectomycorrhizal fungi increasing the growth og Eucalytus globulus Labill. In relation to root colonizing and hyphal development in soil. New Phyt. 126, 517–524. doi: 10.1111/j.1469-8137.1994.tb04250.x
Tobar, R. M., Azcon, R., and Barea, J. M. (1994). The improvement of plant N acquisition from an ammonium-treated, drought-stressed soil by the fungal symbiont in arbuscular mycorrhizae. Mycorrhiza 4, 105–108. doi: 10.1007/BF00203769
Toljander, J. F., Lindhal, B. D., Paul, L. R., Elfstrand, M., and Finlay, R. D. (2007). Influence of arbuscular mycorrhizal mycelial exudates on soil bacterial growth and community structure. FEMS Micriobiol. Ecol. 61, 295–304. doi: 10.1111/j.1574-6941.2007.00337.x
Tordoff, G. M., Boddy, L., and Jones, T. H. (2008). Species-specific impacts of collembola grazing on fungal foraging ecology. Soil Biol. Biochem. 40, 434–442. doi: 10.1016/j.soilbio.2007.09.006
Treseder, K. K., and Allen, M. F. (2000). Mycorrhizal fungi have a potential role in soil carbon storage under elevated CO2 and nitrogen deposition. New Phyt. 147, 189–200. doi: 10.1046/j.1469-8137.2000.00690.x
Treseder, K. K., Allen, M. F., Ruess, R. W., Pregitzer, K. S., and Hendrick, R. L. (2005). Lifespans of fungal rhizomorphs under nitrogen fertilization in a pinyon-juniper woodland. Plant Soil 270, 249–255. doi: 10.1007/s11104-004-1559-7
Tuason, M. M. S., and Arocena, J. M. (2009). Calcium oxalate biomineralization by Piloderma fallax in response to various levels of calcium and phophoruus. App. Environ. Microb. 75, 7079–7085. doi: 10.1128/AEM.00325-09
Tylka, G. L., Hussey, R. S., and Roncadori, R. W. (1991). Axenic germination of vesicular-arbuscular mycorrhizal fungi: effects of selected Steptomycetes speces. Phytopathol. 81, 754–759. doi: 10.1094/Phyto-81-754
Uroz, S., Calvaruso, C., Turpault, M. P., Pierrat, J. C., Mustin, C., and Frey-Klett, P. (2007). Effects of the mycorrhizosphere on the genotypuc and metabolic diversity of the soil bacterial communities involved in mineral weathering in a forest soil. Appl. Environ. Microbiol. 73, 3019–3027. doi: 10.1128/AEM.00121-07
Uroz, S., Oger, P., Morin, E., and Frey-Klett, P. (2012). Distinct ectomycorrhizospheres share similar bacterial communities as revealed by pyrosequencing-based analysis of 16S rRNA genes. Appl. Environ. Microbiol. 78, 3019–3027. doi: 10.1128/AEM.06742-11
van Hees, P. A. W., Godbold, D. L., Jentschke, G., and Jones, D. L. (2003). Impact of ectomycorrhizas on the concentration and biodegradation of simple organic acids in a forest soil. Eur. J. Soil Sci. 54, 697–706. doi: 10.1046/j.1351-0754.2003.0561.x
van Hees, P. A. W., Jones, D. L., Finlay, R. D., Godbold, D. L., and Lundstrom, U. S. (2005). The carbon we do not see—the impact of low molecular weight compounds on carbon dynamics and respiration in forest soils: a review. Soil Biol. Biochem. 37, 1–13. doi: 10.1016/j.soilbio.2004.06.010
van Hees, P. A. W., Rosling, A., Essén, S., Godbold, D. L., Jones, D. L., and Finlay, R. D. (2006a). Oxalate and ferricrocin exudation by the extramatrical mycelium of an ectomycorrhizal fungus in symbiosis with Pinus sylvestris. New Phyt. 169, 367–377. doi: 10.1111/j.1469-8137.2005.01600.x
van Hees, P. A. W., Rosling, A., Lundstrom, U. S., and Finlay, R. D. (2006b). The biogeochemical impact of ectomycorrhizal conifers on major soil elements (Al, Fe, K and Si). Geoderma 136, 364–377. doi: 10.1016/j.geoderma.2006.04.001
van Schöll, L., Hoffland, E., and van Breenan, N. (2006). Organic acid exudation by ectomycorrhizal fungi and Pinus sylvestris in response to nutrient deficiencies. New Phyt. 170, 153–163. doi: 10.1111/j.1469-8137.2006.01649.x
Varese, G. C., Portinaro, S., Trotta, A., Scannerini, S., LuppiMosca, A. M., and Martinotti, M. G. (1996). Bacteria associated with Suillus grevillei sporocarps and ectomycorrhizae and their effects on in vitro growth of the mycobiont. Symbiosis 21, 129–147.
Verbruggen, E., Kiers, E. T., Bakelaar, P. N. C., Roling, W. F. M., and van der Heijden, M. G. A. (2012). Provision of contrasting ecosystem services by soil communities from different agricultural fields. Plant Soil 350, 43–55. doi: 10.1007/s11104-011-0828-5
Vessey, J. K. (2003). Plant growth promoting rhizobacteria as biofertilizers. Plant Soil 255, 571–586. doi: 10.1023/A:1026037216893
Vesterdal, L., Elberling, B., Christiansen, J. R., Callesen, I., and Schmidt, I. K. (2012). Soil respiration and rates of soil carbon turnover differ among six common European tree species. For. Ecol. Mang. 264, 185–196. doi: 10.1016/j.foreco.2011.10.009
Vogt, K. A., Grier, C. C., Edmonds, R. L., and Meier, C. E. (1982). Mycorrhizal role in net primary production and nutrient cycling in Abies amabilis (Dougl) Forbes ecosystems in western Washington. Ecol. 63, 370–380. doi: 10.2307/1938955
Wallander, H. (2006). “Mineral dissolution by ectomycorrhizal fungi,” in Fungi in Biogeochemical Cycles, ed G. M. Gadd ed (Cambridge: Cambridge University Press), 28–50. doi: 10.1017/CBO9780511550522.015
Wallander, H., Göransson, H., and Rosengren, U. (2004). Production, standing biomass and natural abundance of 15N and 13C in ectomycorrhizal mycelia collected at different soil depths in two forest types. Oecologia 139, 89–97. doi: 10.1007/s00442-003-1477-z
Wang, B., and Qui, Y. L. (2006). Phylogenetic distribution and evolution of mycorrhizas in land plants. Mycorrhiza 16, 299–363. doi: 10.1007/s00572-005-0033-6
Warren, J. M., Iverson, C. M., Garten, C. T. Jr. Norby, R. J., Childs, J., Brice, D., et al. (2012). Timing and magnitude of C partitioning through a young loblolly pine (Pinus taeda L.) stand using C-13 labeling and shade treatments. Tree Physiol. 32, 799–813. doi: 10.1093/treephys/tpr129
Weintraub, M., Scott-Denton, L., Schmidt, S., and Monson, R. (2007). The effect of tree rhizodeposition on soil exoenzyme activity, dissolved organic carbon, and nutrient availability in subalpine forest ecosystem. Oecologia 154, 327. doi: 10.1007/s00442-007-0804-1
Welc, M., Bunemann, E. K., Fliessbach, A., Frossard, E., and Jansa, J. (2012). Soil bacterial and fungal communities along a soil chronosequence assessed by fatty acid profiling. Soil Biol. Biochem. 49, 184–192. doi: 10.1016/j.soilbio.2012.01.032
Whiteside, M. D., Digman, M. A., Gratton, E., and Treseder, K. K. (2012). Organic nitrogen uptake by arbuscular mycorrhizal fungi in a boreal forest. Soil Biol. Biochem. 55, 7–13. doi: 10.1016/j.soilbio.2012.06.001
Wright, S. F., and Upadhyaya, A. (1998). A survey of soils for aggregate stability and glomalin, a glycoprotein produced by hyphae of arbuscular mycorrhizal fungi. Plant Soil 198, 97–107. doi: 10.1023/A:1004347701584
Wu, B., Nara, K., and Hogetsu, T. (2002). Spatiotemporal transfer of carbon-14-labelled photosynthate from ectomycorrhizal Pinus densiflora seedlings to extraradical mycelia. Mycorrhiza 12, 83–88. doi: 10.1007/s00572-001-0157-2
Wu, Q. S., LI, G. H., and Zou, Y. N. (2011). Improvement of root system architecture in peach (Prunus persicalucose) seedlings by arbuscular mycorrhizal fungi, related to allocation of glucose/sucrose to root. Not. Bot. Hort. Agrobot. CLUJ. 39, 232–236.
Xavier, J. J. C., and Germida, J. J. (2003). Bacteria associated with Glomus clarum spores influence mycorrhizal activity. Soil Biol. Biochem. 35, 471–478. doi: 10.1016/S0038-0717(03)00003-8
Yang, J., Kloepper, J. W., and Ryu, C. (2009). Rhizosphere bacteria help plants tolerate abiotic stress. Trends Plant Sci. 14, 1–4. doi: 10.1016/j.tplants.2008.10.004
Yarwood, S., Myrold, D. D., and Högberg, M. N. (2009). Termination of belowground C allocation by trees alters soil fungal and bacterial communities in a boreal forest. FEMS Microb. Ecol. 70, 151–162. doi: 10.1111/j.1574-6941.2009.00733.x
Keywords: mycorrhizosphere, root exudates, plant-microbe interactions, LMWOA, signaling, carbon cycling, ectomycorrhizae, arbuscular mycorrhizae
Citation: Churchland C and Grayston SJ (2014) Specificity of plant-microbe interactions in the tree mycorrhizosphere biome and consequences for soil C cycling. Front. Microbiol. 5:261. doi: 10.3389/fmicb.2014.00261
Received: 22 May 2013; Accepted: 13 May 2014;
Published online: 03 June 2014.
Edited by:
Per Bengtson, Lund University, SwedenReviewed by:
Maarja Öpik, University of Tartu, EstoniaErin E. Nuccio, Lawrence Livermore National Laboratory, USA
Copyright © 2014 Churchland and Grayston. This is an open-access article distributed under the terms of the Creative Commons Attribution License (CC BY). The use, distribution or reproduction in other forums is permitted, provided the original author(s) or licensor are credited and that the original publication in this journal is cited, in accordance with accepted academic practice. No use, distribution or reproduction is permitted which does not comply with these terms.
*Correspondence: Sue J. Grayston, Belowground Ecosystem Group, Department of Forest and Conservation Sciences, Forest Sciences Centre, University of British Columbia, 2424 Main Mall, Vancouver, BC V6T 1Z4, Canada e-mail:c3VlLmdyYXlzdG9uQHViYy5jYQ==