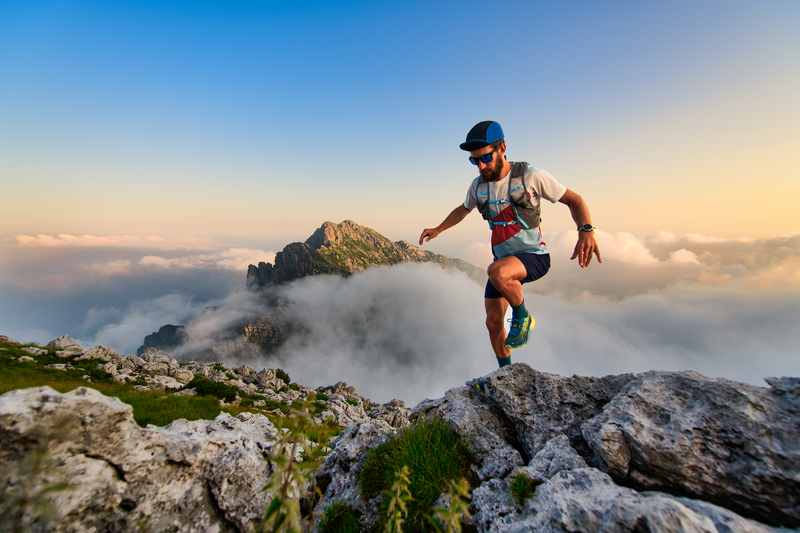
95% of researchers rate our articles as excellent or good
Learn more about the work of our research integrity team to safeguard the quality of each article we publish.
Find out more
REVIEW article
Front. Microbiol. , 05 May 2014
Sec. Extreme Microbiology
Volume 5 - 2014 | https://doi.org/10.3389/fmicb.2014.00199
This article is part of the Research Topic Proceedings of Halophiles 2013: The international congress on halophilic microorganisms View all 27 articles
Molecular studies of salt tolerance of eukaryotic microorganisms have until recently been limited to the baker's yeast Saccharomyces cerevisiae and a few other moderately halotolerant yeast. Discovery of the extremely halotolerant and adaptable fungus Hortaea werneckii and the obligate halophile Wallemia ichthyophaga introduced two new model organisms into studies on the mechanisms of salt tolerance in eukaryotes. H. werneckii is unique in its adaptability to fluctuations in salt concentrations, as it can grow without NaCl as well as in the presence of up to 5 M NaCl. On the other hand, W. ichthyophaga requires at least 1.5 M NaCl for growth, but also grows in up to 5 M NaCl. Our studies have revealed the novel and intricate molecular mechanisms used by these fungi to combat high salt concentrations, which differ in many aspects between the extremely halotolerant H. werneckii and the halophilic W. ichthyophaga. Specifically, the high osmolarity glycerol signaling pathway that is important for sensing and responding to increased salt concentrations is here compared between H. werneckii and W. ichthyophaga. In both of these fungi, the key signaling components are conserved, but there are structural and regulation differences between these pathways in H. werneckii and W. ichthyophaga. We also address differences that have been revealed from analysis of their newly sequenced genomes. The most striking characteristics associated with H. werneckii are the large genetic redundancy, the expansion of genes encoding metal cation transporters, and a relatively recent whole genome duplication. In contrast, the genome of W. ichthyophaga is very compact, as only 4884 protein-coding genes are predicted, which cover almost three quarters of the sequence. Importantly, there has been a significant increase in their hydrophobins, cell-wall proteins that have multiple cellular functions.
Studies of fungal populations in hypersaline environments have revealed the high diversity of fungal species (Gunde-Cimerman et al., 2000), most of which do not require salt for growth, and have their growth optimum in the absence of salt. The dominant fungal group in the hypersaline waters of salterns are the melanized polymorphic black yeast, the most abundant and adapted species of which is Hortaea werneckii. H. werneckii is naturally adapted to fluctuating salt concentrations in its environment, and it can grow without salt and in up to saturated NaCl. Its optimum for growth is between 0.8 M and 1.7 M NaCl. Another successful survivor in these extremely salty environments is the basidiomycetous fungus Wallemia ichthyophaga (Zalar et al., 2005b), which does not grow without salt, and is therefore obligately halophilic.
Due to their different ecology and halotolerances, these two fungi represent highly relevant organisms for the study of eukaryotic adaptation to life at high salt. Studies of haloadaptation mechanisms of H. werneckii started some 15 years ago (for reviews, see Petrovič et al., 2002; Gunde-Cimerman and Plemenitaš, 2006; Plemenitaš et al., 2008; Gostinčar et al., 2011), while with W. ichthyophaga, these studies began later, and are thus less advanced.
H. werneckii (Horta) Nishim and Miyaji (Capnodiales, Dothideomycetes) is a melanized yeast-like ascomycete that is known as the causative agent of tinea nigra, a superficial mycotic infection of the human palm (de Hoog and Gerrits van den Ende, 1992). H. werneckii has been isolated from diverse environments with low water activity (aw), including salty food (Mok et al., 1981), seawater (Iwatsu and Udagawa, 1988), beach soil (de Hoog and Guého, 1998), rocks (Stanley et al., 1982), wood immersed in hypersaline waters (Wollenzien et al., 1995; Zalar et al., 2005a) and microbial mats (Cantrell et al., 2006). However, it appears that its primary habitat is hypersaline water in the evaporate ponds of solar eutrophic salterns (Gunde-Cimerman et al., 2000).
While H. werneckii has been extensively described in our earlier review papers (Petrovič et al., 2002; Gunde-Cimerman and Plemenitaš, 2006; Plemenitaš et al., 2008; Gostinčar et al., 2011), W. ichthyophaga has not been reviewed to date, and thus it is presented below in more detail.
Wallemia Johan-Olsen (Wallemiales, Wallemiomycetes) is a genus of cosmopolitan xerophilic fungi that can be found in a wide variety of environments that are characterized by low aw (Samson et al., 2004; Zalar et al., 2005b). Its phylogenetic position was unclear until recently, and previously it has been placed in various positions in the Basidiomycota phylogenetic tree, from the root of basidiomycetes (Zalar et al., 2005b), to incertae sedis (Hibbett et al., 2007), to being a sister group of the Agaricomycotina and Ustilaginomycotina (Matheny et al., 2006). Genome sequencing has shown that it is indeed a sister group of the Agaricomycotina (Padamsee et al., 2012; Zajc et al., 2013). The Wallemiomycetes split from the Agaricomycotina ancestors an estimated 250 million years ago (Zajc et al., 2013). Initially the genus contained only one species, but it was later segregated into three species based on differences in conidial size, xerotolerance, and sequence data: W. ichthyophaga, Wallemia sebi and Wallemia muriae (Zalar et al., 2005b). To date, only around 20 strains of W. ichthyophaga have been isolated from hypersaline waters of solar salterns, bitterns (i.e., magnesium-rich residual solutions in salt production from sea water) and salted meat (Zalar et al., 2005b). In addition to phylogenetic differences, W. ichthyophaga is also distinguished from the other two representatives of this genus by its characteristic morphology and halophilic physiology (Zalar et al., 2005b; Kralj Kunčič et al., 2010).
Although xerotolerance is rare in the Basidiomycota, all three Wallemia spp. are among the most xerophilic fungal taxa known to date (Zalar et al., 2005b). However, while W. sebi and W. muriae strongly prefer high concentrations of non-ionic solutes over those of NaCl (Kralj Kunčič et al., 2013), the opposite is true for W. ichthyophaga (Zalar et al., 2005b). For growth, W. ichthyophaga requires at least 1.5 M NaCl, or some other osmolyte at an equivalent aw. Such a narrow ecological amplitude of salt concentrations is common for specialized archaeal halophiles, but it is exceptional in the fungal kingdom. Hence, W. ichthyophaga is a rare fungal example of an obligate extremophilic specialist (Gostinčar et al., 2010), and it is considered to be the most halophilic fungus known to date. Although it even thrives in saturated NaCl solution, its in vitro growth optimum is between 2.6 M and 3.5 M NaCl, which is the highest described among fungi (Zajc et al., 2014). It also tolerates high concentrations of salts other than NaCl; e.g., MgCl2 (our unpublished data).
Exposure to high salinity includes two different environmental stimuli for the cell: osmotic stress, and ionic stress. In general, hyperosmotic stress in non-adapted organisms causes immediate water efflux from the cell, which reduces the turgor pressure and triggers cytosol dehydration, thereby increasing the concentrations of the solutes in the cytoplasm (Petelenz-Kurdziel et al., 2011). In particular, under high ionic stress conditions, ions (e.g., Na+) enter the cell, which leads to increased intracellular ion concentrations, which subsequently damage the membranes as well as the cytosolic systems. The main survival strategies to counteract changes in turgor pressure for fungi that are adapted to life at low aw are an accumulation of compatible solutes that do not interfere with vital cellular protein functions, and maintenance of intracellular concentrations of Na+ below toxic levels (Blomberg and Adler, 1992). Both H. werneckii and W. ichthyophaga use the strategy of compatible organic solutes to maintain low intracellular Na+ concentrations, with glycerol being the main solute used.
The main signaling pathway in fungi that is responsible for cellular stress responses is the high osmolarity glycerol (HOG) pathway, which has been extensively studied in the context of osmotic stress in S. cerevisiae. The production and homeostasis of the compatible solute glycerol is one of the main targets under the control of this signaling pathway (Hohmann et al., 2007). The core of the pathway is represented by the mitogen-activated protein kinase (MAPK) signaling module, which is known for its high evolutionary conservation and its activation by sequential phosphorylations (Widmann et al., 1999). This upstream part of the HOG pathway consists of two branches, which are functionally redundant but structurally distinct. They are known as the SHO1 and SLN1 branches and they converge at the MAPK kinase (MAPKK) Pbs2. Upon hyperosmotic shock, when the cell loses some of its water, the MAPK Hog1 is phosphorylated and activated by the upstream MAPKK Pbs2. The main effect of this HOG pathway activation is glycerol production, which restores the cellular osmotic balance. When turgor is re-established, Hog1 is dephosphorylated by phosphatases (Saito and Posas, 2012).
In H. werneckii and W. ichthyophaga, several components of the HOG pathway have been identified and characterized (Lenassi and Plemenitas, 2007; Fettich et al., 2011; Konte and Plemenitaš, 2013). Since their sequenced genomes became available, the presence of some novel HOG components has been confirmed through homology searches. Altogether, there are many similarities between the HOG pathways in H. werneckii, W. ichthyophaga, and S. cerevisiae, although there are also some important differences that might explain the different halotolerant/halophilic characters of H. werneckii and W. ichthyophaga.
The presence of homologs of Sho1, Ste20, and Ste11 has been confirmed for the genomes of H. werneckii and W. ichthyophaga, with two copies of each component in H. werneckii (Figure 1; Lenassi et al., 2013; Zajc et al., 2013; our unpublished data). Two isoforms of the H. werneckii putative osmosensor protein HwSho1A and HwSho1B fully complement the function of the homologous S. cerevisiae Sho1 protein and they can activate the HOG pathway under osmotic stress in S. cerevisiae. Structurally, when compared to other fungal Sho1 homologs, they contain a conserved SH3 domain and a divergent Ste11-binding motif (Fettich et al., 2011). On the other hand, the SH3 domain of W. ichthyophaga (Wi)Sho1 is functional when it is attached to the N-terminal part of S. cerevisiae Sho1, although the whole sequence of WiSho1 does not appear to function correctly in S. cerevisiae (our unpublished data). Regardless of the complementation of the HwSho1 protein and the WiSho1 SH3 domain, data from recent preliminary investigations addressing the role of the SHO1 branch pathway in osmo-adaptation do not support the involvement of this branch in the signal transfer downstream to heterologously expressed HwPbs2 and WiPbs2 in S. cerevisiae. WiSte11 also failed to complement ScSte11 in S. cerevisiae ste11Δssk2ssk22Δ cells, further supporting this hypothesis (our unpublished data).
The other branch of the HOG pathway, which was named as SLN1 after the transmembrane Sln1 hybrid histidine kinase, transmits its signals via a Sln1–Ypd1–Ssk1 phosphorelay. Sln1 kinase is inactive under hyperosmolar conditions, where Ssk1 is dephosphorylated and therefore binds to the autoinhibitory region of Ssk2 and Ssk22, which triggers their autophosphorylation (Saito and Posas, 2012). The H. werneckii histidine kinases HwHhk7A and HwHhk7B have been identified and characterized in more detail (Figure 1; Lenassi and Plemenitas, 2007). HwHhk7A and HwHhk7B lack the transmembrane domain, but otherwise they have a typical eukaryotic hybrid histidine-kinase-domain composition. Their transcription in H. werneckii depends on the extracellular salt concentration, and when they are expressed in S. cerevisiae, they increase its osmotolerance (Lenassi and Plemenitas, 2007). Both the H. werneckii and W. ichthyophaga genomes also contain homologs of the group III histidine kinases. This group of cytosolic histidine kinases can act as osmosensors through their HAMP domain repeats (Meena et al., 2010). On the other hand, the membrane-spanning Sln1-like histidine kinase is present in the genome of H. werneckii, again in two copies, but there is no evidence for it in the genome of W. ichthyophaga (Figure 1), which suggests that the group III histidine kinases are probably involved in osmosensing in this halophilic fungus. Of the other proteins involved in the SLN1 branch, two forms of each of the S. cerevisiae homologs have been found in the genome of H. werneckii, HwYpd1A/B, HwSsk1A/B, and two homologs of the MAPKK kinase Ssk2, HwSsk2A/B (Table 2) (Lenassi et al., 2013; our unpublished data). Also W. ichthyophaga has the proteins WiYpd1, WiSsk1, and WiSsk2 (Figure 1, Table 2; Zajc et al., 2013; our unpublished data).
The signals from both branches of the HOG pathway in S. cerevisiae converge at the MAPKK Pbs2 scaffold, which transmits the signals further to Hog1 (Saito and Posas, 2012). Two gene copies of the MAPKK HwPbs2 have been identified in H. werneckii and one in W. ichthyophaga, WiPbs2 (Figure 1; Lenassi et al., 2013; Zajc et al., 2013; our unpublished data). However, preliminary data show that the kinases HwPbs2 and WiPbs2 do not interact with the S. cerevisiae Sho1 protein (our unpublished data). This suggests that the SHO1 branch is not involved in HOG pathway activation in H. werneckii and W. ichthyophaga.
We had previously identified and characterized only one isoform of the final MAPK HwHog1 (Turk and Plemenitaš, 2002; Lenassi et al., 2007); however, the H. werneckii whole genome sequence revealed another copy of the HwHOG1 gene, the characterization of which is currently in progress. We have also identified two Hog1-like kinase paralogs in W. ichthyophaga, although all of the other HOG pathway components are represented by only single gene copies (Figure 1; Konte and Plemenitaš, 2013).
HwHog1A, HwHog1B, WiHog1A, and WiHog1B are all considerably shorter than ScHog1, although they contain the conserved domains and motifs that are characteristic of the MAPKs, such as the ATP-binding region, Asp in the active site, a TGY phosphorylation motif, a common docking domain, and a Pbs2-binding domain. While HwHog1A, HwHog1B, and WiHog1B are fully functional kinases in the S. cerevisiae hog1Δ background (Lenassi et al., 2007; Konte and Plemenitaš, 2013; our unpublished data), WiHog1A can only partly restore the osmotolerance of the hog1Δ strain. Lower phosphorylation levels, lower GPD1 induction, and greater cross-talk with the mating pathway indicate that WiHog1A cannot interact optimally with the protein partners in S. cerevisiae. WiHog1B, on the other hand, is a fully functional kinase in the S. cerevisiae hog1Δ background. Moreover, WiHog1B even improves the salt tolerance of S. cerevisiae (Konte and Plemenitaš, 2013). We have also demonstrated that in contrast to S. cerevisiae, where the levels of HOG1 mRNA remain unchanged when the cells are exposed to osmotic shock (Brewster et al., 1993), the transcript levels of HOG1-like kinases in H. werneckii and W. ichthyophaga are salt-dependent (Lenassi et al., 2007; Konte and Plemenitaš, 2013).
Upon hyperosmotic shock in S. cerevisiae, Hog1 is rapidly phosphorylated and it translocates into the nucleus. After the cell adapts to the higher osmolarity, Hog1 is dephosphorylated by phosphatases in a negative-feedback manner (Hohmann et al., 2007). Phosphorylation patterns in the extremely halotolerant H. werneckii and the obligate halophile W. ichthyophaga appear to be more complex. In H. werneckii, we have observed a phosphorylation mechanism that is similar to that of S. cerevisiae, although the HwHog1 kinase is noticeably phosphorylated only when the H. werneckii cells were exposed to ≥3 M NaCl (Turk and Plemenitaš, 2002). While in S. cerevisiae constitutive Hog1 phosphorylation is lethal (Maeda et al., 1994), in W. ichthyophaga this is not the case. Even more interestingly, W. ichthyophaga has a completely “opposite” phosphorylation pattern to that of S. cerevisiae: WiHog1 kinase is dephosphorylated after hypo-osmotic or hyperosmotic shock in W. ichthyophaga, and it is constitutively phosphorylated under optimal osmotic conditions (3.4 M NaCl). These data indicate an important role for the phosphatases in the regulation of the HOG pathway in W. ichthyophaga (Konte and Plemenitaš, 2013). This model has already been reported for Cryptococcus neoformans, where some serotypes show inverted W. ichthyophaga-like phosphorylation patterns (Bahn et al., 2007).
When activated, HwHog1 is translocated into the nucleus, where it associates with the chromatin of osmoresponsive genes and induces or represses their expression (Vaupotič and Plemenitaš, 2007). A transcriptional response to hyperosmolar stress of 95 differentially expressed genes has been reported for the comparison of moderately (3 M NaCl) and extremely (4.5 M) osmolar environments. Data from the ChIP method show that 36 of these genes physically interact with HwHog1 in long-term adaptation to extreme environments (Vaupotič and Plemenitaš, 2007). In 17 out of these 36 genes, simultaneous co-localization of RNA polymerase II was seen. More than half of differentially expressed genes are related to general metabolism and energy production, and the other osmoresponsive genes are involved in the biogenesis of mitochondria, protein biosynthesis, protein quality control, transport facilitation, the cell cycle, and the cell wall (Vaupotič and Plemenitaš, 2007). Thirteen of these 95 genes could not be classified. Certain osmoresponsive genes controlled by MAPK HwHog1 have been studied in greater detail. Genes that code for the P-type ATPases HwEna1 and HwEna2 are the S. cerevisiae ENA1 homologs, and therefore they are believed to be involved in the maintenance of a low intracellular K+/Na+ ratio. Their transcription is salt regulated (Gorjan and Plemenitaš, 2006). Two homologs of the S. cerevisiae key enzyme in glycerol biosynthesis, the glycerol-3-phosphate dehydrogenase Gpd1, have been characterized in H. werneckii. These both show similar transcription profiles in response to different salt concentrations (Lenassi et al., 2011). We have also demonstrated that the MAPK WiHog1 can up-regulate the transcription of GPD1 in S. cerevisiae. The regulation of other osmoresponsive genes that are potential targets of WiHog1 remains to be defined.
The genome of H. werneckii was recently sequenced and it has been deposited at DDBJ/EMBL/GenBank under the accession number AIJO00000000 (Lenassi et al., 2013). The genome statistics are summarized in Table 1. The genome of H. werneckii has a size of 51.6 Mb, which is relatively large. In species belonging to the same order as H. werneckii (Capnodiales), the genome sizes are very variable, as they range from 21.88 to 74.12 Mb. The larger genome sizes are mostly due to a substantial amount of repetitive sequences. However, in H. werneckii, despite its large genome size, the proportion of repetitive sequences is only 1.02%. On the other hand, it contains 23,333 predicted genes, which is twice as many as the average number of predicted genes in other related fungi (approx. 11,955 genes) (Ohm et al., 2012). This large number of genes can be attributed to a relatively recent whole genome duplication, which resulted in two nearly identical copies of almost every protein of H. werneckii (Lenassi et al., 2013). This discovery is in line with our previous studies of several individual genes from H. werneckii that were present in two copies (Gorjan and Plemenitaš, 2006; Lenassi and Plemenitas, 2007; Fettich et al., 2011). In most cases, the expression of both of the gene copies is salt dependent, although their expression profiles differ (Lenassi and Plemenitas, 2007). It may well be that as a consequence of this whole genome duplication, H. werneckii can benefit from the potential advantages of large genetic redundancy, even though it is formally in a haploid stage (i.e., it is not a diploid that has resulted from the mating of two strains with opposite mating types, which would regain the haploid stage with meiosis before the next mating event; see below).
Table 1. Genome statistics for W. ichthyophaga and H. werneckii (after Lenassi et al., 2013; Zajc et al., 2013).
The H. werneckii genome sequence has offered the opportunity to gain insight into the genetic information on the mating type(s) and on the mating strategy. To date, no sexual cycle has been described for H. werneckii. Using M. graminicola proteins that contain the alpha1 domain (Mat1-1-1) and the HMG domain (Mat1-1-2), we identified the putative HwMAT1-1-1A and HwMAT1-1-1B genes (Figure 1, Table 2), both of which are translated into 358 amino-acid proteins that contain the alpha1 domain and have an overall amino-acid sequence identity of 87.5% (Lenassi et al., 2013). Importantly, no homologs of the HMG-domain-containing Mat1-1-2 protein were found in H. werneckii, which indicates that this species is heterothallic, and that if it can still undergo sexual reproduction, this requires a strain that codes for the opposite mating type (in the case of the sequenced strain, this would be a strain with a Mat1-1-2 homolog; Lenassi et al., 2013).
Table 2. Major proteins, identified in H. werneckii and W. ichthyophaga and presumably involved in adaptation mechanisms to increased salinity.
Eukaryotic microorganisms have developed numerous plasma-membrane transport systems to maintain their appropriate alkali cation levels, and in particular, to eliminate any surplus of toxic Na+ ions. The alkali-cation transport systems in S. cerevisiae and in non-conventional yeast have recently been reviewed (Arino et al., 2010; Ramos et al., 2011).
In S. cerevisiae, the plasma-membrane transporters Trk1 and Trk2 for K+ uptake, the Tok1 K+ channel, the Pho98 inorganic phosphate (Pi)-Na+ symporter, the Ena Na+-ATPases, and the Nha1 Na+/H+ antiporter have all been well characterized (Arino et al., 2010). Together with these, non-specific protein transporters (e.g., Pm3, Qdr2) have been described to be involved in K+/Na+ fluxes across the plasma membrane (Arino et al., 2010). Trk transporters for K+ uptake, Nha antiporters, Ena ATPases, and Tok1 channels have also been identified in non-conventional yeast, together with the Hak K+/H+ symporters and the rare K+/Na+-uptake ATPase Acu (Ramos et al., 2011).
Physiological studies have shown that H. werneckii maintains very low intracellular K+ and Na+ levels (Kogej et al., 2005), even when it grows in the presence of 4.5 M NaCl, which suggested that it can effectively extrude Na+ ions and also prevent their influx. Analysis of H. werneckii genome has revealed considerable expansion of families of genes that encode plasma-membrane metal cation transporters, as presented schematically in Figure 1 and summarized in Table 2 (Lenassi et al., 2013). We identified eight homologs of the Trk1 and Trk2 K+ channels, with each containing the conserved TrkH domain that is typical for cation transport proteins. In general, they show low homology to the Trk1 protein, but the amino-acid sequence identity increases in the TrkH domain. We also identified four homologs of the Tok1 K+ channels, each of which contains two conserved transmembrane helices that are typical of this ion-channel family. Again, the homology to the Tok1 protein is low, but the identity is high in the transmembrane helices. The presence of eight homologs of the Nha1 Na+/K+, H+ antiporters was demonstrated, each of which contains a transmembrane region at the N-terminal, which is conserved through the Na+/K+, H+ exchanger family, and only two of them additionally contain the C-terminal cytoplasmic region. Extensive expansion has also been observed for the Pho89 homologs in H. werneckii, as we identified six homologs of the Pho89 Na+, Pi symporter, with each homolog containing at least one PHO4 domain. In contrast with the abundant transporter families mentioned, only four homologs of three S. cerevisiae Ena Na+ P-type ATPases have been identified in the H. werneckii genome (Lenassi et al., 2013). Previously, we identified and characterized two Ena-like P-ATPases (Gorjan and Plemenitaš, 2006). Analysis of the Ena Na+ P-type ATPases identified in the genome of H. werneckii reveals that each homolog contains all four of the conserved domains found in the S. cerevisiae Ena proteins. Considering their multiplication, it appears that Nha transporters are more important than Ena. On the other hand, based on our previous data that demonstrated that HwENA genes are highly induced at alkaline pH (Gorjan and Plemenitaš, 2006), we speculate that they have complementary functions: Ena ATPases are more important at high pH, where the Nha antiporters cannot function correctly.
As well as the important role of plasma-membrane transport systems in ion homeostasis, in the cytosol, K+ homeostasis and Na+ detoxification are also connected to cation transport across the organelle membranes (Arino et al., 2010). In S. cerevisiae, endosomal Nhx1 (Nass and Rao, 1999) and Kha1 from the Golgi apparatus (Maresova and Sychrova, 2005) are Na+/H+ exchangers, similar to Nha1 at the plasma membrane (Prior et al., 1996). The vacuolar Vnx1 (Cagnac et al., 2007) and the mitochondrial Mdm38 and Mrs7 (Nowikovsky et al., 2004; Zotova et al., 2010) have similar Na+/K+, H+ exchanger functions, but different structures.
We found that homologs of Nhx1 and Kha1 are duplicated in the H. werneckii genome, all of which contain the domains that are typical for the Na+/H+ exchanger family. The same has been observed for the Kha1 homologs. We also identified two homologs of transporters with high homology to the Mrs7 and Mdm38 transporters from S. cerevisiae. Of the intracellular cation transporters, only the homologs of the vacuolar Vnx1 are enriched in H. werneckii in comparison to S. cerevisiae. We identified eight homologs of the Vnx1 Na+/K+, H+ antiporter, but the homology of the HwVnx proteins compared to Vnx1 is low (Lenassi et al., 2013).
The activities of many transporters are closely connected to the proton gradients across the membranes, which are generated by the Pma1 P-type ATPase at the plasma membrane (Serrano et al., 1986; Ambesi et al., 2000) and the V-type ATPase at the vacuolar membrane (Graham et al., 2000). Different P-type ATPases use ATP hydrolysis as a source of energy for the transport of ions through the membrane, and they are structurally similar (Kuhlbrandt, 2004).
The enrichment of the transporters responsible for supplying the energy for the cation transporters in H. werneckii supports the importance of the complex cation transporter system for combating high environmental Na+. We identified four homologs of Pma1 in H. werneckii, with each homolog containing three conserved domains that are also found in the S. cerevisiae Pma1 and Pma2 proteins. The importance of all four of the H. werneckii Pma homologs for cation homeostasis is also supported by expression analysis, as the expression profiles of the PMA1 and PMA2 homologs show different levels of response to saline conditions (Lenassi et al., 2013). Comparisons of the expression profiles of the PMA genes in H. werneckii with those described in S. cerevisiae have shown that in S. cerevisiae, PMA1 is not induced by salt stress (Yale and Bohnert, 2001), while in H. werneckii, both PMA1 and PMA2 have salt-regulated transcription.
The yeast vacuolar ATPase does not only have a crucial role in the acidification of the vacuolar lumen, but it is also important for the correct functioning of other organelles (Arino et al., 2010). In the H. werneckii genome, we found homologs of all of the subunits of the S. cerevisiae V-ATPase complex. The H. werneckii vacuolar subunits in general share a lot of similarity with the S. cerevisiae subunits, which is not surprising, as their structures and function have been highly conserved through evolution (Graham et al., 2000). S. cerevisiae vacuolar ATPases are localized at different cellular locations; however, it remains to be determined where they are specifically localized in H. werneckii. In contrast to the transcription of the HwPMAs, which is salt regulated, no such trends have been seen for the expression of the VMA homologs under different salinities.
The genome of W. ichthyophaga has been deposited as a Whole Genome Shotgun project at DDBJ/EMBL/GenBank under the accession number APLC00000000 (Zajc et al., 2013). The genome of W. ichthyophaga is 9.6 Mb in size, and the sequence currently consists of 101 contigs and 82 scaffolds (Table 1). Most basidiomycetous haploid genomes are more than twice this size (and in some cases, larger by 40-fold or more; Gregory et al., 2007). The closely related species W. sebi also has a slightly larger genome (9.8 Mb) (Padamsee et al., 2012). Of the species investigated thus far, only the dandruff- and seborrhoeic-dermatitis-causing Malassezia globosa has a smaller genome (9.0 Mb; Gregory et al., 2007). The compactness of the genome of W. ichthyophaga is reflected in its low level of repetitive sequences (1.67%), and high density of genes (514 genes/Mb scaffold) (Zajc et al., 2013). This is only slightly lower than W. sebi (538 genes/Mb), but more than in M. globosa (476 genes/Mb). This means that the coding DNA sequences in W. ichthyophaga cover almost three quarters of the genome. The GC content in W. ichthyophaga is 45.35%, while in W. sebi this is even lower, at 40.01% (Table 1). The absolute number of predicted proteins in W. ichthyophaga (4884; Zajc et al., 2013) is also unusually small for a basidiomycete (where more than 10,000 proteins are not uncommon), and is in the range observed for Escherichia coli (Lukjancenko et al., 2010). For comparison, the W. sebi genome codes for 5284 proteins, while M. globosa contains 4285 proteins. Interestingly, the reduction in genome size and gene number is not accompanied by a reduction in intron number, such as has been reported for some other fungi with small genomes (Kelkar and Ochman, 2012).
It has not been possible to assign functions for an unproportionally large number of the proteins that are found in W. ichthyophaga but not in W. sebi. With searches through the Pfam database, three quarters of these proteins could not be classified into any of the protein families (Zajc et al., 2013). Among those that could be identified, there were several proteins related to DNA processing and DNA damage.
The sequencing of the transcriptomes of W. ichthyophaga grown in 10% and 30% (w/v) NaCl has revealed that 13.1% of the genes are differentially expressed under these conditions (Zajc et al., 2013). Of these, two thirds are more expressed at lower salinity. Alternative splicing, which has been identified as intron retention, was detected for 15.0% of the genes, and in more than half of the cases (51.6%), alternative splicing was detected only at one of the two tested salinities (Zajc et al., 2013).
The strategy of osmo-adaptation of both the extremely halotolerant H. werneckii and the halophilic W. ichthyophaga is the accumulation of a mixture of polyols that act as compatible solutes (Figure 1). The main osmotically regulated polyol of both H. werneckii and W. ichthyophaga is glycerol, the levels of which are increased with increasing salinity and decreased after hypo-osmotic shock (Kogej et al., 2007; Zajc et al., 2014). In addition to glycerol, we have reported erythritol, arabitol, and mannitol in H. werneckii (Plemenitaš et al., 2008), and smaller amounts of arabitol, and traces of mannitol in W. ichthyophaga (Zajc et al., 2014). The genes for the enzymes known to be involved in compatible solute management are found in the genome of W. ichthyophaga (Table 2). These are present in several copies, with the exception of the glycerol-3-phosphatase Gpp. W. ichthyophaga contains a homolog of GPD1, WiGPD1, the expression of which is salt-induced (Lenassi et al., 2011). A second homolog was also found by searching the genome. When compared with the homologs of H. werneckii, the expression level of WiGPD1 is lower, and the response to hyperosmotic shock is slower (Lenassi et al., 2011). Expression of WiGPD1 in S. cerevisiae boosted the osmotolerance of the gpd1 and gpd1gpd2 mutants. As was reported for homologs from H. werneckii, WiGPD1 lacks the N-terminal peroxisomal targeting (PTS2) sequence (Lenassi et al., 2011), which is important for peroxisome localization of WiGpd1 (Jung et al., 2010). This might mean that WiGpd1 remains in the cytosol, which would be an advantage when living in extremely saline environments, as it is this fraction that is important for the synthesis of the compatible solutes (Lenassi et al., 2011). During hyperosmotic shock, S. cerevisiae counteracts glycerol leakage by its active re-import using the protein Stl1, a glycerol/H+ symporter in the plasma membrane (Ferreira et al., 2005). Similarly, the aquaglyceroporin channel Fps1 remains closed (while it opens during hypo-osmotic shock, to facilitate expulsion of excess glycerol) (Luyten et al., 1995). In W. ichthyophaga, four homologs of Stl1 have been found (Figure 1, Table 2), as well as three aquaglyceroporin-related proteins.
In the basidiomycete Agaricus bisporus, the solute D-mannitol is synthesized from fructose via a reduction step that is catalysed by two NADP-dependent mannitol dehydrogenases (Stoop and Mooibroek, 1998). W. ichthyophaga also contains two homologs of D-arabinitol-2-dehydrogenases, which are used in other fungi for the production of arabitol from an intermediate of the pentose phosphate pathway, D-ribulose-5-phosphate.
In line with the strategy of compatible solutes, the intracellular levels of K+ and Na+ in W. ichthyophaga remain low at constant salinities (not above 30 nmol/mg dry biomass) (Zajc et al., 2013), even when compared to H. werneckii (not above 180 nmol/mg dry biomass) (Kogej et al., 2007). However, when under hyperosmotic shock, the levels of both cations increase significantly in W. ichthyophaga, indicating its poor capability to adjust to changing environments. The ratio between these cations decreases with increasing salinity, due to the rising levels of Na+ and the lowering of K+. However, the intracellular K+/Na+ ratio is higher across the whole salinity range in W. ichthyophaga compared to H. werneckii (Figure 1) and some other halotolerant fungi (e.g., Aureobasidium pullulans, Debaryomyces hansenii). In addition, the K+/Na+ decrease over the salinity range is less steep in W. ichthyophaga compared to H. werneckii. The growth performance of W. ichthyophaga is greatest when the Na+ content exceeds that of K+ (Zajc et al., 2014). This indicates that these intracellular concentrations of Na+ ions are not toxic to the cells.
Data from the genome show that there are only a low number of cation transporters, except for the enriched protein family of P-type ATPases (Figure 1, Table 2). Also, expression of the cation transporters is low and independent of salt, with only three minor exceptions (described below). This is probably associated with the life of W. ichthyophaga at relatively constant (although extremely high) salinities. Nevertheless, in its genome we observed a significant enrichment of the cation-transporting ATPases family. The identified proteins of this family are three H+ and two Na+ P-type ATPases (all of which are assumed to be located at the plasma membrane), two Ca2+ P-type ATPases (vacuolar Pmc1 and Pmr1 from the Golgi apparatus), and a putative transporter of unknown specificity (Zajc et al., 2013). The W. ichthyophaga genome encodes three putative Pma proton pumps, while W. sebi contains only two (Zajc et al., 2013).
In environments with high concentrations of Na+ salts, the cell must prevent the intracellular accumulation of the highly toxic Na+, without lowering the levels of K+. This is achieved by a variety of other secondary active transporters. W. ichthyophaga contains homologs of most known transporters from S. cerevisiae (Arino et al., 2010) and unconventional yeast (Ramos et al., 2011), as either those located on intracellular membranes (Kha1, Mrs7/Mdm37, Nhx1, Pmc1, Pmr1, Vnx1, Vma1) or at the plasma membrane (Ena, Nha1, Pho89, Pma, Trk1).
Judging by the genes that encode alkali metal cation transporters, the extremely halotolerant ascomycete H. werneckii and W. ichthyophaga use different salt-combating strategies. As described above, in H. werneckii, the numbers of most of the plasma-membrane alkali cation transporters are substantially increased. In W. ichthyophaga this is not the case (Figure 1, Table 2). W. ichthyophaga contains only one Trk homolog (inward K+ transporter, with eight copies in H. werneckii) and no Tok homologs (outward K+ channel, with four copies in H. werneckii). Similarly, W. ichthyophaga has only two Nha homologs (Na+/K+ proton antiporters) and one Pho89 (Na+/Pi symporter), while H. werneckii contains eight and six, respectively (Lenassi et al., 2013; Zajc et al., 2013).
Active import of K+ might contribute to ion homeostasis in hypersaline environments, and this would complement the action of passive K+ channels. The known active transporters are K+-H+symporters (Hak symporters) and K+(Na+)-ATPase (Acu, alkali cation uptake transporters) (Benito et al., 2004; Ramos et al., 2011). While H. werneckii has no homologs of either of these transporter types (Lenassi et al., 2013), W. ichthyophaga contains two possible homologs of the otherwise rare Acu ATPases, of which only one contains a P-type ATPase domain (Zajc et al., 2013).
Several different membrane alkali metal transporters (mainly cation/H+ antiporters) are also located on the organelle membranes: the Golgi apparatus (Kha1), mitochondria (Mdm38 or Mrs7), endosomes (Nhx1), and vacuole (Vnx1) (Arino et al., 2010). In W. ichthyophaga, there are single-copy genes of all of these proteins (with the exception of the duplicated Kha1).
W. ichthyophaga can live at extremely high salinity; however, the above-described findings indicate that the salinity remains relatively constant and thus no rapid responses are needed. Under constant conditions, there is no need for a quick release of surplus K+, and thus the absence of the Tok outward K+ channel might not be detrimental. On the other hand, W. ichthyophaga contains three homologs of aquaglyceroporins (Zajc et al., 2013), which fulfil the need for rapid expulsion of the accumulated compatible solute glycerol (Luyten et al., 1995). Two explanations are possible: (1) W. ichthyophaga might deal with hypo-osmotic shock-related K+ expulsion in other ways than in other fungi; and (2) the aquaglyceroporin channels might serve some other functions than expulsion of glycerol during the shock.
In cells grown at 10 and 30% (w/v) NaCl, the large majority of the genes that encode metal-cation transporters are not differentially expressed. This is not as expected, since these transporters are believed to have crucial roles in adaptation to salt. The only exceptions are a homolog of the Pho89 Na+/Pi symporter, which shows elevated expression at high salinity, and a putative P-type Na+ ATPase and a possible Acu K+ importer, with the expression of both of these latter higher at low salinity. This is in stark contrast, for example, with other halotolerant fungi (e.g., D. hansenii, H. werneckii), where even at different constant salinities, differential expression of P-type H+ and Na+ ATPases has been observed (Almagro et al., 2001; Gorjan and Plemenitaš, 2006; Lenassi et al., 2013). Furthermore, in W. ichthyophaga the expression of transporter coding genes is relatively low: of the total of 4884 genes, neither the Na+-exporting P-type ATPases nor the two Na+/H+ antiporters are among the 2000 most-expressed genes at high salinity. The possible post-transcriptional control of all of these genes remains to be investigated.
Continuous removal and/or compartmentalization of Na+ at a constant high salinity is extremely demanding energetically. The low numbers of transporters in W. ichthyophaga might reflect the relatively low adaptive potential of this species to changes in salt concentrations and/or its specialization with other mechanisms that are energetically more efficient. The apparent transcriptional non-responsiveness of transporters to salt can lead to similar conclusions. This would mean that the halophilic strategy of W. ichthyophaga, which is a unique example of a narrowly specialized fungal halophile (Gostinčar et al., 2010), is substantially different from that of H. werneckii, which contains a collection of K+ channels and can adapt to a wide salinity range (Zajc et al., 2013).
The analysis of the W. ichthyophaga genome has revealed a significant expansion of seven protein families and contraction of 19. The most interesting of the expanded families are the hydrophobins, which are proteins that potentially have a role in the particular morphological adaptations of W. ichthyophaga (Figure 1; Zajc et al., 2013).
W. ichthyophaga has a characteristic morphology that can be seen in many stress-tolerant species: compact multicellular clumps that are similar to sarcinae (Zalar et al., 2005b). This morphology has been observed in, and it is believed to enhance survival in, high-stress environments (Wollenzien et al., 1995; Palkova and Vachova, 2006; Gostinčar et al., 2011). These cells have an abundant cover of extracellular polysaccharides (Kralj Kunčič et al., 2010) that can serve as protectants during desiccation (Selbmann et al., 2005), and possibly also when exposed to high concentrations of salt (Zajc et al., 2013). Additionally, at high salinity the morphology of W. ichthyophaga cells changes strikingly. The size of the meristematic cell clumps increases four-fold, and the cell walls become three-fold thicker, which also substantially decreases the intracellular volume (Kralj Kunčič et al., 2010).
The hydrophobins are proteins in the cell wall of filamentous fungi. These small (≤20 kDa) and amphipathic molecules (Linder et al., 2005) are involved in a range of processes of cellular growth and development (Wosten, 2001). Possibly as a reflection of the many different roles they have, the hydrophobin genes are often present in multiple different copies. From an estimated 15 hydrophobin genes in the last common ancestor of W. ichthyophaga and W. sebi, these genes are enriched to 26 in W. ichthyophaga, while the number has fallen to 12 in W. sebi. This is the most significant protein family expansion in the genome of W. ichthopyhaga (Zajc et al., 2013).
The hydrophobins that have been identified in W. ichthyophaga and W. sebi contain the characteristic hydrophobin pattern of the conserved spacing of eight cysteine residues (Zajc et al., 2013) that form four disulphide bridges (Hektor and Scholtmeijer, 2005; Linder et al., 2005). These hydrophobins in both W. ichthyophaga and W. sebi contain a high proportion of acidic amino acids compared to homologs from other fungi (Zajc et al., 2013). This is similar to the archaeal halophilic proteins (Madern et al., 2000) and it might represent an adaptation to salt exposure. If acidic amino acids are exposed on a protein surface, they can bind salt and water, and thus help to avoid salt-induced changes in conformation that would lead to loss of activity (Siglioccolo et al., 2011). This might also be the case for the hydrophobins of W. ichthyophaga, as these are among the few of its proteins that are actually exposed to high concentrations of NaCl and are not protected in the intracellular compatible-solute-rich environment. Interestingly, half of the genes encoding hydrophobins are differentially expressed during growth of W. ichthyophaga at different salinities, although their responses are not the same: at high salinity some of these hydrophobins have higher expression, and some have lower expression (Zajc et al., 2013).
The hydrophobins can self-assemble into amphipathic monolayers on hydrophobic–hydrophilic interfaces, which is crucial for their diverse functions. With their help, the cell can attach to hydrophobic surfaces, break through a water-air interface, and avoid water-logging without impeding the exchange of gasses. Hydrophobins can also strengthen the cell wall and make it more rigid, and they also impact on the movement of solutes (Wosten, 2001; Bayry et al., 2012). It is not difficult to imagine that these functions will be beneficial to cells exposed to high salinity (Zajc et al., 2013). Under these conditions, the leakage of compatible solutes and intrusion of toxic salt ions are among the greatest challenges to the cell. Increased strength and rigidity of the cell wall will help the cell to survive the structural stress that can be imposed by changes in the environmental osmolarity.
The hydrophobins might also have a role in the characteristic sarcina-like morphology of W. ichthyophaga, which is similar to Fusarium verticillioides, where the hydrophobins trigger microconidial chain formation (Fuchs et al., 2004). This might additionally be involved in the aggregation of W. ichthyophaga cells into compact cell clusters, as is characteristic of W. ichthyophaga, and which is probably a form of salt-stress response (Gostinčar et al., 2010). This characteristic sarcina-like morphology is considered to be among the main haloadaptations of W. ichthyophaga. Indeed, both the changes in the cell wall and the formation of multicellular structures have previously been suggested to be among the main adaptations of W. ichthyophaga to hypersaline environments (Kralj Kunčič et al., 2010).
Traditional mycological approaches have not lead to any descriptions of mating behaviors for either H. werenckii or the Wallemia spp.. In both cases, no descriptions of fruiting bodies or reports of teleomorphs can be found in the literature. Basidomycetous fungi have a tetrapolar MAT locus. Two additional unlinked loci encode the homeodomain-containing transcription factors and pheromone/pheromone receptors. In some cases, other mating architectures have evolved through the expansion and fusion of these MAT loci (Lee et al., 2010). The genome of W. sebi contains a single mating-type locus and lacks only a few meiosis-specific genes, which suggested that it can undergo sexual reproduction (Padamsee et al., 2012). This is not the case for W. ichthyophaga (Zajc et al., 2013). Searches of the W. ichthyophaga genome for proteins that are similar to the gene products involved in mating in other basidiomycetes has resulted in the identification of only a few proteins, and even these are very dissimilar to proteins from other fungi. No discernible mating locus has been identified, and only three of eight meiosis-specific genes (as listed in Malik et al., 2008) have been found (Zajc et al., 2013).
Asexuality in fungi is not unusual. Asexual reproduction saves energy, as it eliminates the need to produce gametes and attractants, and this might be useful in extreme environments where careful management of energy is of key importance. In habitats that do not undergo major changes over longer time scales, this would also prevent the drowning of specific adaptations of local populations in the larger gene pool of the species (Gostinčar et al., 2010; Sun and Heitman, 2011). Therefore, in the case of these extremophilic fungal species, an asexual lifestyle might have evolutionary advantages (Gostinčar et al., 2010). This appears to be the case for W. ichthyophaga, and this might also be true for H. werneckii. While a putative mating type locus has been found in the genome of H. werneckii, no sexual stage of this species has been described to date. As mating genes can have roles outside of mating (Srikantha et al., 2012), their presence in itself is not confirmation of sexual reproduction in a species. The potential role of sexual reproduction in H. werneckii therefore needs to be further investigated.
The extremely halotolerant and adaptable H. werneckii and the obligately halophilic W. ichthyophaga live in environments that are defined by low aw and high concentrations of toxic inorganic ions. To withstand these harsh environmental conditions they use some common molecular mechanisms and also some specific molecular mechanisms. A model of the key adaptations in H. werneckii and W. ichthyophaga that have been discussed here is illustrated in Figure 1.
When a cell is exposed to a high osmolarity (salinity) environment, it has to react rapidly to the consequent loss of water. In H. werneckii and W. ichthyophaga this is achieved by the synthesis of glycerol and a few other compatible solutes (Figure 1). The expression of the key enzyme in glycerol synthesis, Gpd1, is under the control of the HOG signaling pathway, which is also important for other aspects of adaptation to high osmolarity environments. The key proteins of the HOG pathway are conserved in H. werneckii and W. ichthyophaga, although the HOG pathway architecture and regulation is different. While all of the HOG pathway components are present in at least two isoforms in H. werneckii, there are only two isoforms of the Hog1 kinase in W. ichthyophaga (Figure 1). In H. werneckii, HwHog1 is only phosphorylated under extracellular conditions of ≥3 M NaCl (Turk and Plemenitaš, 2002), while in W. ichthyophaga, the WiHog1 kinase is constitutively phosphorylated under optimal osmotic conditions and is dephosphorylated upon hyperosmotic or hypo-osmotic shock (Konte and Plemenitaš, 2013). In H. werneckii, HwHog1 promotes differential induction or repression of osmoresponsive genes, depending on the osmolarity, and also by physically interacting with chromatin and RNA polymerase II (Vaupotič and Plemenitaš, 2007). While this specific architecture of the HOG pathway might represent the background for the extreme halotolerance of H. werneckii, the constitutive phosphorylation of the Hog1-like kinase in W. ichthyophaga might support its obligate halophilic nature.
In many natural hypersaline environments, the concentrations of toxic Na+ ions are far greater than those of K+ ions, and therefore the mechanisms that maintain the stable and high intracellular K+/Na+ ratio are crucial for the survival in such environments. Both, H. werneckii and W. ichthyophaga can maintain high K+/Na+ ratios over a wide range of environmental Na+ concentrations. In H. werneckii, this homeostasis is maintained by regulated transport of K+ and Na+ across the plasma membrane, as cation transporters are diverse and highly enriched in this fungus (Figure 1). W. ichthyophaga also regulates the entry and expulsion of cations; however, it mainly prevents their entry by dynamic cell-wall restructuring. An explanation for these observed differences in the way that these fungi combat the toxic environmental Na+ might be the need for H. werneckii to adapt rapidly to highly dynamic concentrations of NaCl (and other salts) that it typically encounters in its natural environment, while W. ichthyophaga thrives instead under continuously high salinity.
Large genetic redundancy is an important characteristic of H. werneckii, which has presumably resulted from the whole genome duplication. Although the whole genome duplication that is seen in H. werneckii is not uncommon for fungi, it is interesting that this duplication has not yet been followed by selective gene loss, as the large majority of genes in H. werneckii is still present in two copies. Such redundancy might be an excellent reservoir for cryptic genetic variability, which is of importance under stress environments that require good adaptability (Gostinčar et al., 2010). This might be especially the case, as the extent of sexual recombination as a means of generating genetic diversity in H. werneckii is still not known. Indeed, in H. werneckii there is a recognizable putative mating type locus, which indicates the possibility of (although so far not observed) a sexual reproduction cycle, while W. ichthyophaga lacks the cellular machinery for sexual reproduction altogether (Figure 1).
We believe that the recently published genome and transcriptome sequences of H. werneckii and W. ichthyophaga will accelerate research into the osmotic strategies of these fungi that can thrive in high salinity environments that allow the survival of only the most specialized minority of eukaryotes and prokaryotes.
The authors declare that the research was conducted in the absence of any commercial or financial relationships that could be construed as a potential conflict of interest.
The authors acknowledge financial support from the state budget of the Slovenian Research Agency (Infrastructural Centre Mycosmo, MRIC UL, Programme P1, Postdoctoral Project Z4-5531 to Cene Gostinčar, and Young Researcher Grants to Janja Zajc, Tilen Konte and Anja Kejžar). The study was also partly financed via the operation “Centre of excellence for integrated approaches in chemistry and biology of proteins,” number OP13.1.1.2.02.0005, financed by the European Regional Development Fund (85% share of financing) and the Slovenian Ministry of Higher Education, Science and Technology (15% share of financing).
Almagro, A., Prista, C., Benito, B., Loureiro-Dias, M. C., and Ramos, J. (2001). Cloning and expression of two genes coding for sodium pumps in the salt-tolerant yeast Debaryomyces hansenii. J. Bacteriol. 183, 3251–3255. doi: 10.1128/JB.183.10.3251-3255.2001
Ambesi, A., Miranda, M., Petrov, V. V., and Slayman, C. W. (2000). Biogenesis and function of the yeast plasma-membrane H+-ATPase. J. Exp. Biol. 203(pt 1), 155–160.
Arino, J., Ramos, J., and Sychrova, H. (2010). Alkali metal cation transport and homeostasis in yeasts. Microbiol. Mol. Biol. Rev. 74, 95–120. doi: 10.1128/MMBR.00042-09
Bahn, Y. S., Geunes-Boyer, S., and Heitman, J. (2007). Ssk2 mitogen-activated protein kinase kinase kinase governs divergent patterns of the stress-activated Hog1 signaling pathway in Cryptococcus neoformans. Eukaryot. Cell 6, 2278–2289. doi: 10.1128/EC.00349-07
Bayry, J., Aimanianda, V., Guijarro, J. I., Sunde, M., and Latge, J. P. (2012). Hydrophobins-unique fungal proteins. PLoS Pathog. 8:e1002700. doi: 10.1371/journal.ppat.1002700
Benito, B., Garciadeblas, B., Schreier, P., and Rodriguez-Navarro, A. (2004). Novel P-type ATPases mediate high-affinity potassium or sodium uptake in fungi. Eukaryot. Cell 3, 359–368. doi: 10.1128/EC.3.2.359-368.2004
Blomberg, A., and Adler, L. (1992). Physiology of osmotolerance in fungi. Adv. Microb. Physiol. 33, 145–212. doi: 10.1016/S0065-2911(08)60217-9
Brewster, J. L., de Valoir, T., Dwyer, N. D., Winter, E., and Gustin, M. C. (1993). An osmosensing signal transduction pathway in yeast. Science 259, 1760–1763. doi: 10.1126/science.7681220
Cagnac, O., Leterrier, M., Yeager, M., and Blumwald, E. (2007). Identification and characterization of Vnx1p, a novel type of vacuolar monovalent Cation/H+ antiporter of Saccharomyces cerevisiae. J. Biol. Chem. 282, 24284–24293. doi: 10.1074/jbc.M703116200
Cantrell, S. A., Casillas-Martinez, L., and Molina, M. (2006). Characterization of fungi from hypersaline environments of solar salterns using morphological and molecular techniques. Mycol. Res. 110, 962–970. doi: 10.1016/j.mycres.2006.06.005
de Hoog, G. S., and Gerrits van den Ende, A. H. (1992). Nutritional pattern and eco-physiology of Hortaea werneckii, agent of human tinea nigra. Anton. Leeuw. 62, 321–329. doi: 10.1007/BF00572601
de Hoog, G. S., and Guého, E. (1998). “Agents of white piedra, black piedra and tinea nigra,” in Topley and Wilsons Microbiology and Microbial Infections, Vol. 4, eds L. Ajello and R. J. Hay (London, UK: Arnold Publications), 191–197.
Ferreira, C., van Voorst, F., Martins, A., Neves, L., Oliveira, R., Kielland-Brandt, M. C., et al. (2005). A member of the sugar transporter family, Stl1p is the glycerol/H+ symporter in Saccharomyces cerevisiae. Mol. Biol. Cell 16, 2068–2076. doi: 10.1091/mbc.E04-10-0884
Fettich, M., Lenassi, M., Veranič, P., Gunde-Cimerman, N., and Plemenitaš, A. (2011). Identification and characterization of putative osmosensors, HwSho1A and HwSho1B, from the extremely halotolerant black yeast Hortaea werneckii. Fungal Genet. Biol. 48, 475–484. doi: 10.1016/j.fgb.2011.01.011
Fuchs, U., Czymmek, K. J., and Sweigard, J. A. (2004). Five hydrophobin genes in Fusarium verticillioides include two required for microconidial chain formation. Fungal Genet. Biol. 41, 852–864. doi: 10.1016/j.fgb.2004.04.004
Gorjan, A., and Plemenitaš, A. (2006). Identification and characterization of ENA ATPases HwENA1 and HwENA2 from the halophilic black yeast Hortaea werneckii. FEMS Microbiol. Lett. 265, 41–50. doi: 10.1111/j.1574-6968.2006.00473.x
Gostinčar, C., Grube, M., de Hoog, S., Zalar, P., and Gunde-Cimerman, N. (2010). Extremotolerance in fungi: evolution on the edge. FEMS Microbiol. Ecol. 71, 2–11. doi: 10.1111/j.1574-6941.2009.00794.x
Gostinčar, C., Lenassi, M., Gunde-Cimerman, N., and Plemenitaš, A. (2011). Fungal adaptation to extremely high salt concentrations. Adv. Appl. Microbiol. 77, 71–96. doi: 10.1016/B978-0-12-387044-5.00003-0
Graham, L. A., Powell, B., and Stevens, T. H. (2000). Composition and assembly of the yeast vacuolar H+-ATPase complex. J. Exp. Biol. 203, 61–70.
Gregory, T. R., Nicol, J. A., Tamm, H., Kullman, B., Kullman, K., Leitch, I. J., et al. (2007). Eukaryotic genome size databases. Nucleic Acids Res. 35, 332–338. doi: 10.1093/nar/gkl828
Gunde-Cimerman, N., and Plemenitaš, A. (2006). Ecology and molecular adaptations of the halophilic black yeast Hortaea werneckii. Rev. Environ. Sci. Biotechnol. 5, 323–331. doi: 10.1007/s11157-006-9105-0
Gunde-Cimerman, N., Zalar, P., de Hoog, S., and Plemenitaš, A. (2000). Hypersaline waters in salterns - natural ecological niches for halophilic black yeasts. FEMS Microbiol. Ecol. 32, 235–240. doi: 10.1016/S0168-6496(00)00032-5
Hektor, H. J., and Scholtmeijer, K. (2005). Hydrophobins: proteins with potential. Curr. Opin. Biotechnol. 16, 434–439. doi: 10.1016/j.copbio.2005.05.004
Hibbett, D. S., Binder, M., Bischoff, J. F., Blackwell, M., Cannon, P. F., Eriksson, O. E., et al. (2007). A higher-level phylogenetic classification of the Fungi. Mycol. Res. 111, 509–547. doi: 10.1016/j.mycres.2007.03.004
Hohmann, S., Krantz, M., and Nordlander, B. (2007). Yeast osmoregulation. Method Enzymol. 428, 29–45. doi: 10.1016/S0076-6879(07)28002-4
Iwatsu, T. U., and Udagawa, S. (1988). Hortaea werneckii isolated from sea-water. Jpn. J. Med. Mycol. 29, 142–145. doi: 10.3314/jjmm1960.29.142
Jung, S., Marelli, M., Rachubinski, R. A., Goodlett, D. R., and Aitchison, J. D. (2010). Dynamic changes in the subcellular distribution of Gpd1p in response to cell stress. J. Biol. Chem. 285, 6739–6749. doi: 10.1074/jbc.M109.058552
Kelkar, Y. D., and Ochman, H. (2012). Causes and consequences of genome expansion in fungi. Genome Biol. Evol. 4, 13–23. doi: 10.1093/gbe/evr124
Kogej, T., Ramos, J., Plemenitaš, A., and Gunde-Cimerman, N. (2005). The halophilic fungus Hortaea werneckii and the halotolerant fungus Aureobasidium pullulans maintain low intracellular cation concentrations in hypersaline environments. Appl. Environ. Microbiol. 71, 6600–6605. doi: 10.1128/AEM.71.11.6600-6605.2005
Kogej, T., Stein, M., Volkmann, M., Gorbushina, A. A., Galinski, E. A., and Gunde-Cimerman, N. (2007). Osmotic adaptation of the halophilic fungus Hortaea werneckii: role of osmolytes and melanization. Microbiology 153, 4261–4273. doi: 10.1099/mic.0.2007/010751-0
Konte, T., and Plemenitaš, A. (2013). The HOG signal transduction pathway in the halophilic fungus Wallemia ichthyophaga: identification and characterisation of MAP kinases WiHog1A and WiHog1B. Extremophiles 17, 623–636. doi: 10.1007/s00792-013-0546-4
Kralj Kunčič, M., Kogej, T., Drobne, D., and Gunde-Cimerman, N. (2010). Morphological response of the halophilic fungal genus Wallemia to high salinity. Appl. Environ. Microbiol. 76, 329–337. doi: 10.1128/AEM.02318-09
Kralj Kunčič, M., Zajc, J., Drobne, D., Pipan Tkalec, Z., and Gunde-Cimerman, N. (2013). Morphological responses to high sugar concentrations differ from adaptation to high salt concentrations in the xerophilic fungi Wallemia spp. Fungal Biol. 117, 466–478. doi: 10.1016/j.funbio.2013.04.003
Kuhlbrandt, W. (2004). Biology, structure and mechanism of P-type ATPases. Nat. Rev. Mol. Cell Biol. 5, 282–295. doi: 10.1038/nrm1354
Lee, S. C., Ni, M., Li, W. J., Shertz, C., and Heitman, J. (2010). The evolution of sex: a perspective from the fungal kingdom. Microbiol. Mol. Biol. R 74, 298–340. doi: 10.1128/MMBR.00005-10
Lenassi, M., Gostinčar, C., Jackman, S., Turk, M., Sadowski, I., Nislow, C., et al. (2013). Whole genome duplication and enrichment of cation transporters are associated with the halotolerant lifestyle of the black yeast Hortaea werneckii. PLoS ONE 30:e71328. doi: 10.1371/journal.pone.0071328
Lenassi, M., and Plemenitas, A. (2007). Novel group VII histidine kinase HwHhk7B from the halophilic fungi Hortaea werneckii has a putative role in osmosensing. Curr. Genet. 51, 393–405. doi: 10.1007/s00294-007-0131-4
Lenassi, M., Vaupotič, T., Gunde-Cimerman, N., and Plemenitaš, A. (2007). The MAP kinase HwHog1 from the halophilic black yeast Hortaea werneckii: coping with stresses in solar salterns. Saline Systems 3, 3. doi: 10.1186/1746-1448-3-3
Lenassi, M., Zajc, J., Gostinčar, C., Gorjan, A., Gunde-Cimerman, N., and Plemenitaš, A. (2011). Adaptation of the glycerol-3-phosphate dehydrogenase Gpd1 to high salinities in the extremely halotolerant Hortaea werneckii and halophilic Wallemia ichthyophaga. Fungal Biol. 115, 959–970. doi: 10.1016/j.funbio.2011.04.001
Linder, M. B., Szilvay, G. R., Nakari-Setala, T., and Penttila, M. E. (2005). Hydrophobins: the protein-amphiphiles of filamentous fungi. FEMS Microbiol. Rev. 29, 877–896. doi: 10.1016/j.femsre.2005.01.004
Lukjancenko, O., Wassenaar, T. M., and Ussery, D. W. (2010). Comparison of 61 sequenced Escherichia coli genomes. Microb. Ecol. 60, 708–720. doi: 10.1007/s00248-010-9717-3
Luyten, K., Albertyn, J., Skibbe, W. F., Prior, B. A., Ramos, J., Thevelein, J. M., et al. (1995). Fps1, a yeast member of the mip family of channel proteins, is a facilitator for glycerol uptake and efflux and is inactive under osmotic-stress. EMBO J. 14, 1360–1371.
Madern, D., Ebel, C., and Zaccai, G. (2000). Halophilic adaptation of enzymes. Extremophiles 4, 91–98. doi: 10.1007/s007920050142
Maeda, T., Wurglermurphy, S. M., and Saito, H. (1994). A 2-component system that regulates an osmosensing Map kinase cascade in yeast. Nature 369, 242–245. doi: 10.1038/369242a0
Malik, S. B., Pightling, A. W., Stefaniak, L. M., Schurko, A. M., and Logsdon, J. M. (2008). An expanded inventory of conserved meiotic genes provides evidence for sex in Trichomonas vaginalis. PLoS ONE 3:e2879. doi: 10.1371/journal.pone.0002879
Maresova, L., and Sychrova, H. (2005). Physiological characterization of Saccharomyces cerevisiae kha1 deletion mutants. Mol. Microbiol. 55, 588–600. doi: 10.1111/j.1365-2958.2004.04410.x
Matheny, P. B., Gossmann, J. A., Zalar, P., Kumar, T. K. A., and Hibbett, D. S. (2006). Resolving the phylogenetic position of the Wallemiomycetes: an enigmatic major lineage of Basidiomycota. Can. J. Bot. 84, 1794–1805. doi: 10.1139/b06-128
Meena, N., Kaur, H., and Mondal, A. K. (2010). Interactions among HAMP domain repeats act as an osmosensing molecular switch in group III hybrid histidine kinases from Fungi. J. Biol. Chem. 285, 12121–12132. doi: 10.1074/jbc.M109.075721
Mok, W. Y., Castelo, F. P., and Barreto Da Silva, M. S. (1981). Occurrence of Exophiala werneckii on salted freshwater fish Osteoglossum bicirrhosum. Int J Food Sci Technol 16, 505–512. doi: 10.1111/j.1365-2621.1981.tb01843.x
Nass, R., and Rao, R. (1999). The yeast endosomal Na+/H+ exchanger, Nhx1, confers osmotolerance following acute hypertonic shock. Microbiology 145, 3221–3228.
Nowikovsky, K., Froschauer, E. M., Zsurka, G., Samaj, J., Reipert, S., Kolisek, M., et al. (2004). The LETM1/YOL027 gene family encodes a factor of the mitochondrial K+ homeostasis with a potential role in the Wolf-Hirschhorn syndrome. J. Biol. Chem. 279, 30307–30315. doi: 10.1074/jbc.M403607200
Ohm, R. A., Feau, N., Henrissat, B., Schoch, C. L., Horwitz, B. A., Barry, K. W., et al. (2012). Diverse lifestyles and strategies of plant pathogenesis encoded in the genomes of eighteen Dothideomycetes fungi. PLoS Pathog. 8:e1003037. doi: 10.1371/journal.ppat.1003037
Padamsee, M., Kumar, T. K., Riley, R., Binder, M., Boyd, A., Calvo, A. M., et al. (2012). The genome of the xerotolerant mold Wallemia sebi reveals adaptations to osmotic stress and suggests cryptic sexual reproduction. Fungal Genet. Biol. 49, 217–226. doi: 10.1016/j.fgb.2012.01.007
Palkova, Z., and Vachova, L. (2006). Life within a community: benefit to yeast long-term survival. FEMS Microbiol. Rev. 30, 806–824. doi: 10.1111/j.1574-6976.2006.00034.x
Petelenz-Kurdziel, E., Eriksson, E., Smedh, M., Beck, C., Hohmann, S., and Goksor, M. (2011). Quantification of cell volume changes upon hyperosmotic stress in Saccharomyces cerevisiae. Integr. Biol. 3, 1120–1126. doi: 10.1039/c1ib00027f
Petrovič, U., Gunde-Cimerman, N., and Plemenitaš, A. (2002). Cellular responses to environmental salinity in the halophilic black yeast Hortaea werneckii. Mol. Microbiol. 45, 665–672. doi: 10.1046/j.1365-2958.2002.03021.x
Plemenitaš, A., Vaupotič, T., Lenassi, M., Kogej, T., and Gunde-Cimerman, N. (2008). Adaptation of extremely halotolerant black yeast Hortaea werneckii to increased osmolarity: a molecular perspective at a glance. Stud. Mycol. 61, 67–75. doi: 10.3114/sim.2008.61.06
Prior, C., Potier, S., Souciet, J. L., and Sychrova, H. (1996). Characterization of the NHA1 gene encoding a Na+/H+-antiporter of the yeast Saccharomyces cerevisiae. FEBS Lett. 387, 89–93. doi: 10.1016/0014-5793(96)00470-X
Ramos, J., Arino, J., and Sychrova, H. (2011). Alkali-metal-cation influx and efflux systems in nonconventional yeast species. FEMS Microbiol. Lett. 317, 1–8. doi: 10.1111/j.1574-6968.2011.02214.x
Saito, H., and Posas, F. (2012). Response to hyperosmotic stress. Genetics 192, 289–318. doi: 10.1534/genetics.112.140863
Samson, R. A., Hoekstra, E. S., and Frisvad, J. C. (2004). Introduction to Food- and Airborne Fungi. Utrecht: Centraalbureau voor Schimmelcultures.
Selbmann, L., de Hoog, G. S., Mazzaglia, A., Friedmann, E. I., and Onofri, S. (2005). Fungi at the edge of life: cryptoendolithic black fungi from Antarctic desert. Stud. Mycol. 1–32.
Serrano, R., Kiellandbrandt, M. C., and Fink, G. R. (1986). Yeast plasma-membrane ATPase is essential for growth and has homology with (Na+/K+), K+- and Ca2+-ATPases. Nature 319, 689–693. doi: 10.1038/319689a0
Siglioccolo, A., Paiardini, A., Piscitelli, M., and Pascarella, S. (2011). Structural adaptation of extreme halophilic proteins through decrease of conserved hydrophobic contact surface. BMC Struct. Biol. 11:50. doi: 10.1186/1472-6807-11-50
Srikantha, T., Daniels, K. J., Pujol, C., Sahni, N., Yi, S., and Soll, D. R. (2012). Nonsex genes in the mating type locus of Candida albicans play roles in a/alpha biofilm formation, including impermeability and fluconazole resistance. PLoS Pathog. 8:e1002476. doi: 10.1371/journal.ppat.1002476
Stanley, J. T., Palmer, F., and Adams, J. B. (1982). Microcolonial fungi: common inhabitants on desert rocks? Science 215, 1093–1095. doi: 10.1126/science.215.4536.1093
Stoop, J. M. H., and Mooibroek, H. (1998). Cloning and characterization of NADP mannitol dehydrogenase cDNA from the button mushroom, Agaricus bisporus, and its expression in response to NaCl stress. Appl. Environ. Microbiol. 64, 4689–4696.
Turk, M., and Plemenitaš, A. (2002). The HOG pathway in the halophilic black yeast Hortaea werneckii: isolation of the HOG1 homolog gene and activation of HwHog1p. FEMS Microbiol. Lett. 216, 193–199. doi: 10.1111/j.1574-6968.2002.tb11435.x
Vaupotič, T., and Plemenitaš, A. (2007). Differential gene expression and Hog1 interaction with osmoresponsive genes in the extremely halotolerant black yeast Hortaea werneckii. BMC Genomics 8:280. doi: 10.1186/1471-2164-8-280
Widmann, C., Gibson, S., Jarpe, M. B., and Johnson, G. L. (1999). Mitogen-activated protein kinase: conservation of a three-kinase module from yeast to human. Physiol. Rev. 79, 143–180.
Wollenzien, U., Dehoog, G. S., Krumbein, W. E., and Urzi, C. (1995). On the isolation of microcolonial fungi occurring on and in marble and other calcareous rocks. Sci. Total Environ. 167, 287–294. doi: 10.1016/0048-9697(95)04589-S
Wosten, H. A. (2001). Hydrophobins: multipurpose proteins. Annu. Rev. Microbiol. 55, 625–646. doi: 10.1146/annurev.micro.55.1.625
Yale, J., and Bohnert, H. J. (2001). Transcript expression in Saccharomyces cerevisiae at high salinity. J. Biol. Chem. 276, 15996–16007. doi: 10.1074/jbc.M008209200
Zajc, J., Kogej, T., Galinski, E. A., Ramos, J., and Gunde-Cimerman, N. (2014). Osmoadaptation strategy of the most halophilic fungus, Wallemia ichthyophaga, growing optimally at salinities above 15% NaCl. Appl. Environ. Microbiol. 80, 247–256. doi: 10.1128/AEM.02702-13
Zajc, J., Liu, Y. F., Dai, W. K., Yang, Z. Y., Hu, J. Z., Gostinčar, C., et al. (2013). Genome and transcriptome sequencing of the halophilic fungus Wallemia ichthyophaga: haloadaptations present and absent. BMC Genomics 14:617. doi: 10.1186/1471-2164-14-617
Zalar, P., Kocuvan, M. A., Plemenitaš, A., and Gunde-Cimerman, N. (2005a). Halophilic black yeasts colonize wood immersed in hypersaline water. Bot. Mar. 48, 323–326. doi: 10.1515/BOT.2005.042
Zalar, P., Sybren de Hoog, G., Schroers, H. J., Frank, J. M., and Gunde-Cimerman, N. (2005b). Taxonomy and phylogeny of the xerophilic genus Wallemia (Wallemiomycetes and Wallemiales, cl. et ord. nov.). Anton. Leeuw. 87, 311–328. doi: 10.1007/s10482-004-6783-x
Keywords: halophilic/halotolerant fungi, Hortaea werneckii genome, Wallemia ichthyophaga genome, HOG signaling pathway, ion homeostasis
Citation: Plemenitaš A, Lenassi M, Konte T, Kejžar A, Zajc J, Gostinčar C and Gunde-Cimerman N (2014) Adaptation to high salt concentrations in halotolerant/halophilic fungi: a molecular perspective. Front. Microbiol. 5:199. doi: 10.3389/fmicb.2014.00199
Received: 27 January 2014; Paper pending published: 11 February 2014;
Accepted: 14 April 2014; Published online: 05 May 2014.
Edited by:
Aharon Oren, The Hebrew University of Jerusalem, IsraelReviewed by:
Aharon Oren, The Hebrew University of Jerusalem, IsraelCopyright © 2014 Plemenitaš, Lenassi, Konte, Kejžar, Zajc, Gostinčar and Gunde-Cimerman. This is an open-access article distributed under the terms of the Creative Commons Attribution License (CC BY). The use, distribution or reproduction in other forums is permitted, provided the original author(s) or licensor are credited and that the original publication in this journal is cited, in accordance with accepted academic practice. No use, distribution or reproduction is permitted which does not comply with these terms.
*Correspondence: Ana Plemenitaš, Faculty of Medicine, Institute of Biochemistry, University of Ljubljana, Vrazov trg 2, Ljubljana 1000, Slovenia e-mail:YW5hLnBsZW1lbml0YXNAbWYudW5pLWxqLnNp
Disclaimer: All claims expressed in this article are solely those of the authors and do not necessarily represent those of their affiliated organizations, or those of the publisher, the editors and the reviewers. Any product that may be evaluated in this article or claim that may be made by its manufacturer is not guaranteed or endorsed by the publisher.
Research integrity at Frontiers
Learn more about the work of our research integrity team to safeguard the quality of each article we publish.