- Département de Sciences Biologiques, Institut de Recherche en Biologie Végétale, Université de Montréal, Montréal QC, Canada
The cosmopolitan presence of dinoflagellates in aquatic habitats is now believed to be a direct consequence of the different trophic modes they have developed through evolution. While heterotrophs ingest food and photoautotrophs photosynthesize, mixotrophic species are able to use both strategies to harvest energy and nutrients. These different trophic modes are of particular importance when nitrogen nutrition is considered. Nitrogen is required for the synthesis of amino acids, nucleic acids, chlorophylls, and toxins, and thus changes in the concentrations of various nitrogenous compounds can strongly affect both primary and secondary metabolism. For example, high nitrogen concentration is correlated with rampant cell division resulting in the formation of the algal blooms commonly called red tides. Conversely, nitrogen starvation results in cell cycle arrest and induces a series of physiological, behavioral and transcriptomic modifications to ensure survival. This review will combine physiological, biochemical, and transcriptomic data to assess the mechanism and impact of nitrogen metabolism in dinoflagellates and to compare the dinoflagellate responses with those of diatoms.
Introduction
Dinoflagellates are unicellular eukaryotes that appeared ~400 MYA and still thrive today in most marine and freshwater ecosystems (Fensome et al., 1999). They have evolved various life styles, which has enabled them to populate a great diversity of ecological niches. Many dinoflagellates are found within the phytoplankton, and are important contributors to oceanic primary production. Others, such as Pfiesteria or Protoperidinium, are predators that are known to feed on a wide array of prey. Still other dinoflagellates can be symbiotic, as exemplified by the endosymbiotic associations formed between Symbiodinium and some anthozoans. This mutualistic symbiosis is of immense ecological importance because many tropical reef corals live in nutrient-poor water and the photosynthetic products supplied by the zooxanthellae symbionts are essential for growth and survival of the host (Davy et al., 2012). The order Syndiniales is comprised exclusively of parasitic species that infect tintinnid ciliates, crustaceans, dinoflagellates and fish (Park et al., 2002; Skovgaard et al., 2005; Gestal et al., 2006; Harada et al., 2007; Guillou et al., 2008; Small et al., 2012). Curiously, some dinoflagellate genera, such as Gambierdiscus, Ostreopsis or Prorocentrum, can live fixed to a substrate. They can be found both in epiphytic associations with macroalgae and in benthic sediments (Vila et al., 2001). The benthic zone also contains dinoflagellate temporary or resting cysts. It is now believed that one explanation for the ecological versatility of dinoflagellates comes from the three trophic modes, autotrophy, mixotrophy, and heterotrophy they have evolved to harvest energy.
Traditionally, dinoflagellates have been categorized as either photoautotrophic or heterotrophic, based on the presence or absence of chloroplasts. Over the past 30 years, however, it became evident that these two trophic modes were actually the extremes of a continuum, with the middle region being composed of mixotrophic species. Mixotrophs combine photosynthesis and food ingestion to harvest both energy and nutrients, and are quite common in marine phytoplankton, with the diatoms being a noteworthy exception (Stoecker et al., 2009). Mixotrophy can be found in all dinoflagellate orders, even if evidence is stronger in some taxa (Stoecker, 1999). Most dinoflagellates have complex life cycles, and in some cases mixotrophic behavior is only apparent in some life stages. For example, P. piscicida lacks chloroplasts and is heterotrophic for most of its life, except in its flagellated zoospore stage where the cells contain functional kleptochloroplasts stolen from ingested cryptophytes (Steidinger et al., 1996; Stoecker, 1999).
Although dinoflagellate life styles are diverse, all species require carbon (C), phosphorus (P) and nitrogen (N). Of these, N nutrition is of particular interest, because high concentrations of various N sources are often correlated with the appearance of harmful algal blooms (HABs) dominated by dinoflagellates (Lee, 2006; Anderson et al., 2008, 2012b; San Diego-McGlone et al., 2008; Zhou et al., 2008). There is a general scientific consensus that HAB events have globally increased in frequency, magnitude and geographic extent over the last 40 years (Anderson et al., 2012b). Concurrently, the impacts of HABs on public health, tourism, fisheries and ecosystems have also increased. Some HABs are toxic, such as those caused by the widespread Alexandrium genera, as they can synthesize a suite of paralytic shellfish toxins (PST; Wang, 2008; Anderson et al., 2012a). These toxins accumulating within filter-feeding mollusks can cause paralytic shellfish poisoning. PSTs all contain N and their concentration within Alexandrium cells can increase up to 76% following N-enrichment (Hu et al., 2006; Wang, 2008). A better understanding of N metabolism in dinoflagellates could help to better predict the occurrence of HABs and limit their impact.
This review will cover N metabolism in dinoflagellates of various marine life styles. Unfortunately, even though some physiological and transcriptional studies are available, there is little known about the molecular components involved in N metabolism for these organisms. However, it is known that most dinoflagellates species with permanent chloroplasts can live in medium supplemented strictly with various inorganic nutrients, nitrate () being the predominant N form (Hansen, 2011). This implies that N assimilation genes are present in these species and, because this process is remarkably well conserved in plants and algae, it is likely that parallels can be made with dinoflagellates (Figure 1, see further sections for details). This review will first address these parallels by describing what is currently reported about the molecular components involved in N metabolism in plants and eukaryotic algae. We will then discuss the particular case of mixotrophy for the uptake of N in dinoflagellates. This nutrient being essential for synthesis of amino acids, nucleic acids, chlorophylls, and toxins, it is a major factor limiting growth. In conditions of N-stress dinoflagellate cells either die or modify their metabolism and trophic behavior to ensure their survival. We will finish by presenting the various adaptations used by dinoflagellates to cope with N stress. Throughout the text, we will compare the dinoflagellate N responses to those of diatoms to examine which environmental conditions could favor one group of organisms over the other.
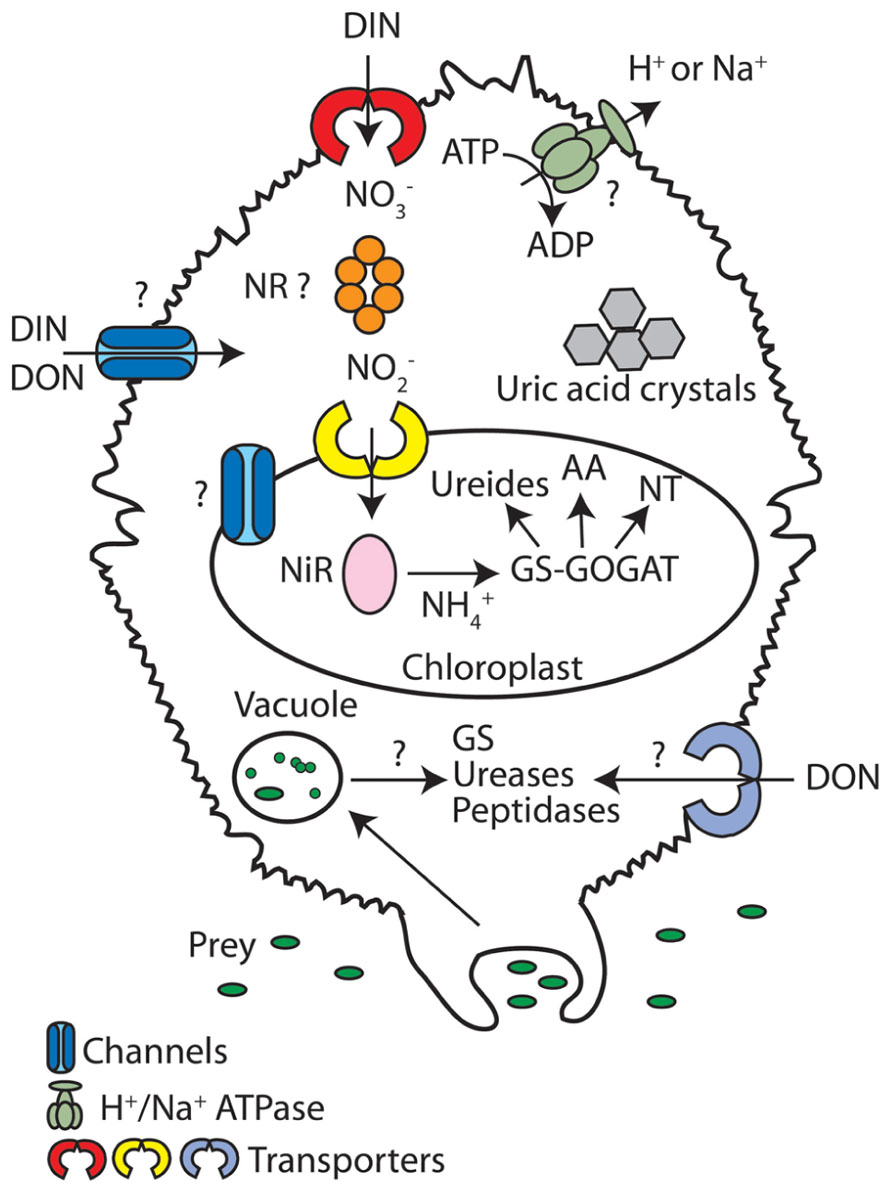
FIGURE 1. Nitrogen metabolism in dinoflagellates. In this schematic overview of N metabolism, transporters and channels are depicted as individual proteins that transport a range of N forms, even though transporters/channels are normally selective for particular N forms. A (?) signifies that the pathways or proteins have not been reported in dinoflagellates, but can be present in other photosynthetic eukaryotes. The (NR ?) indicates that while this enzyme is usually localized to the cytoplasm, a study in L. polyedrum indicated it was found to the chloroplast (Fritz et al., 1996). AA, amino acids; DIN, dissolved inorganic nitrogen; DON, dissolved organic nitrogen; GS, glutamine synthetases; GOGAT, glutamine 2-oxoglutarate amidotransferase; , ammonium; , nitrate; ; nitrite; NiR; nitrite reductase; NR, nitrate reductase; NT, nucleotides.
Overview of the Marine N Cycle
The marine N cycle is probably the most complex of the biogeochemical cycles, as it involves various chemical forms and multiple transformations that connect all marine organisms. In this section and the following one, we will begin by giving a brief overview of the N cycle to better understand now N flux in the oceans and what chemical forms are the most relevant for dinoflagellates. We will then describe the molecular mechanisms of N transport in plants and algae. We will finish with what is currently known about physiological N uptake in dinoflagellates and how our understanding has been helped by genomic and functional studies in diatoms.
About 94% of the oceanic N inventory exists as biologically unavailable dissolved nitrogen gas (N2; Gruber, 2008). This gas can be made bioavailable through N2-fixation, a process carried out by photoautotrophic prokaryotes, mainly cyanobacteria, using iron-dependent nitrogenases to catalyze reduction of N2 to . N2-fixation thus provides a counterbalance to the loss of bioavailable N through denitrification ( to N2) and anaerobic ammonium oxidation (Annamox, to N2), both of which are anaerobic reactions catalyzed by bacteria. The 6% of biologically available N exists primarily as (~88%) and dissolved organic nitrogen (DON, ~11.7%; Gruber, 2008). The remaining 0.3% is found in other chemical forms, such as , , nitrous oxide (N2O) and particulate organic nitrogen (PON; Gruber, 2008).
The distribution and composition of fixed N forms vary with depth as direct consequences of combined biological and physical processes. In the euphotic zone, C fixation by photosynthesis drives the assimilation of inorganic N in order to sustain growth. If the resulting organic N produced is released into the seawater in the euphotic zone, most can be directly reassimilated, remineralized into inorganic N or respired for energy production. However, some organic N will sink down to the aphotic zone where ammonification and nitrification will remineralize it back to inorganic N. Finally, ocean circulation and mixing can return this remineralized N to the euphotic zone where it can be used to sustain new growth. The direct consequences of this biogeochemical loop are that surface waters are generally depleted in inorganic N while the deep oceans are enriched (Gruber, 2008). Conversely, concentrations of PON and DON are much higher near the surface than deeper in the oceans (Gruber, 2008). While this scenario holds in the open ocean, anthropogenically derived N additions have significantly changed the nutrient states in the coastal regions of the world (Rabalais, 2002; Beman et al., 2005). Agricultural runoffs and aquaculture industries bring sizeable amounts of new inorganic and organic N which tend to accelerate the N cycle in coastal ecosystems (Galloway et al., 2004; Beman et al., 2005; Gruber, 2008; San Diego-McGlone et al., 2008). The main engine driving this accelerated N cycle is the ability of phytoplanktonic species to take up N directly from the environment using very efficient transport systems.
Uptake of Nitrogen Using Transporters
The work of Epstein and Hagen on ionic transport in plant rhizodermal cells was among the first to describe the dynamics of transporters (Epstein and Hagen, 1952). They found that the kinetics of ion uptake shared all the characteristics of classic Michaelis-Menten enzyme catalyzed reactions, although uptake of potassium (K) and rubidium was later shown to have a low Km or a high Km depending if the external ionic concentrations were low (μM) or high (mM), respectively (Epstein et al., 1963). In plants and eukaryotic algae, physiological import of inorganic and organic N is also generally dependent on environmental concentrations (Doddema and Telkamp, 1979; Franco et al., 1988; Wilson and Walker, 1988; Siddiqi et al., 1990; Galvan et al., 1996; Howitt and Udvardi, 2000; Rentsch et al., 2007; Wang et al., 2008). Expression of transport proteins in heterologous systems such as yeast and Xenopus oocytes has greatly helped to determine their biochemical properties (Galvan and Fernandez, 2001; Rentsch et al., 2007). At the molecular level, dual affinity can be explained by the presence of a group of transporters, which individually have either a high or a low affinity for their substrates. However, dual affinity can also result when an individual transporter is able to switch between the two affinities. The best example of a switching transporter is CHL1/AtNRT1.1 in Arabidopsis, where phosphorylation of threonine 101 (T101) changes its activity from a low-affinity to a high-affinity transporter (Liu and Tsay, 2003). Interestingly, T101 was also shown to be involved in sensing, as assessed by the ability of to induce expression of genes involved in metabolism. Mutants mimicking the phosphorylated form of the transporter were unable to elicit a low-affinity response, whereas mutants mimicking the dephosphorylated form had an increased response at all concentrations of N (Ho et al., 2009). CHL1/AtNRT1.1 was thus named a “transceptor.” Finally, a last group of transporters is made up of channel-like proteins such as the major intrinsic proteins (MIPs), a family which also contains aquaporins. MIPs provide facilitated diffusion of and urea into plant cells and tonoplasts and have only low affinity for their substrates (Gaspar et al., 2003; Liu et al., 2003b; Jahn et al., 2004; Loque et al., 2005).
Nitrogen transporters and channels are often multi-selective and differentially regulated. For example, the Chlamydomonas reinhardtii transporters CrNRT2.1 and CrNRT2.3 transport both and with identical or different affinities, respectively. CrNRT2.1 has a high affinity for both and , while CrNRT2.3 has a low affinity for and a high affinity for (Fernandez and Galvan, 2007). Generally, however, most transporters of the same family or subfamily share similar substrate selectivity and affinity. As for the regulation patterns, some transporters are constitutively expressed while others are influenced by different conditions such as pH, light and level of substrates in the environment.
Facilitated diffusion such as carried out by MIPs follows down the concentration gradient and thus has no requirement for energy. However, for the majority of inorganic and organic N compounds, transport goes against a concentration gradient and thus needs a source of energy. Plants and algae produce a proton motive force (PMF) using H+-ATPases at their plasma membranes and other cell compartments. The PMF is an electrochemical gradient exploited by transporters, such as those for , , the high-affinity system of urea, some amino acids and peptides. These transporters work either by the symport or antiport of H+, and their activities are thus dependent on pH (Galvan and Fernandez, 2001; Liu et al., 2003a; De Angeli et al., 2006; Fernandez and Galvan, 2007; Rentsch et al., 2007; Sugiura et al., 2007). Others, such as the high-affinity uniporter AMT1.1 from Arabidopsis, exploit the electrical gradient generated by the PMF, and thus do not require H+ transport (Ninnemann et al., 1994; Howitt and Udvardi, 2000).
One problem concerning the use of a PMF in marine organisms is that seawater is typically alkaline (pH ≥ 7.8). Fortunately, seawater also contains abundant sodium (Na+, 450–500 mM), and as in animal cells, many marine algae can exploit these Na+ gradients for uptake of nutrients such as , , glucose, amino acids and silica (Hellebust, 1978; Bhattacharyya and Volcani, 1980; Raven and Smith, 1980; Boyd and Gradmann, 1999). Moreover, existence of P-type Na+-ATPases in marine algae was confirmed in Tetraselmis viridis and Heterosigma akashiwo (Gimmler, 2000). The Na+ versus H+ powered-transport in, respectively, marine and freshwater/terrestrial organisms, is however not an absolute rule. For example, the freshwater chlorophyte Ankistrodesmus braunii requires Na+ for the transport of , while Charales living in brackish waters typically use H+ symporters for nutrient transport (Ullrich and Glaser, 1982; Bisson et al., 2006). The strong negative membrane potential of characean cells is thought to enable H+-symport even in alkaline environments (Bisson et al., 2006). Notably, Charales are also able to use Na+-coupled transport for , urea and Cl- (Sanders et al., 1985; Walker et al., 1993; Reid et al., 2000). To date, no comparisons of the energetics of transport have been made between dinoflagellates living in freshwater and those living in seawater.
Physiological uptake of , , , urea and other DON by chloroplast-containing dinoflagellates has been reported in both field and laboratory studies (Harrison, 1976; Kudela and Cochlan, 2000; Fan et al., 2003; Leong and Taguchi, 2004; Collos et al., 2007; Maguer et al., 2007; Lee, 2008; Loureiro et al., 2009; Leong et al., 2010; Pernice et al., 2012). Most describe the uptake kinetics in relation to cell growth, and variability in the kinetics has emerged as an important feature. Depending on the experimental sampling conditions, different intraspecific half-saturation constant (Km) values for , and urea were reported in Lingulodinium polyedrum and Alexandrium catenella (Smayda, 1997; Kudela and Cochlan, 2000; Collos et al., 2007). In the latter species, Km for and Km for urea varied from 0.2 to 20 μM and 0.1 to 44 μM over a 4-year period, respectively (Collos et al., 2007). In the same study, the authors’ measured Km values range from 0.5 to 6.2 μM within a 3-day interval, showing how fast transport kinetics can change within the same dinoflagellate population. Similar variations were also noted in L. polyedrum (Harrison, 1976). In a 2005 review, Collos et al. (2005) noted a linear relationship between Km for and ambient concentrations for various freshwater and marine unicellular algae in the field. They proposed that most phytoplankton possess an ability to physiologically acclimate to different concentrations. The variations of Km observed for and urea in dinoflagellates suggest that acclimation could be generalized to various N forms, not only . From a molecular perspective, these results suggest that different combinations of transporters each with particular kinetics and level of expression/activity will be found in dinoflagellates.
Generally, when growing in presence of various different N compounds, dinoflagellates (as well as plants and algae) prefer to take up . However, there is a concentration threshold above which becomes toxic to the cells, and this threshold seems to be species-specific. For example, in A. minutum, concentrations of 25 μM and higher lead to growth inhibition while for A. tamarense and Cochlodinium polykrikoides, this threshold was not observed until 50 μM (Chang and McClean, 1997; Leong and Taguchi, 2004; Lee, 2008). Another tendency in dinoflagellates is inhibition of uptake when in the presence of . In A. minutum, this inhibition was found to be greater when the cells were in N-sufficient compared to N-deprived conditions (Maguer et al., 2007). This suggests that when N is limiting, uptake of different forms will be favored over strict assimilation of which has a reduced energy cost. Curiously, different blooming populations of dinoflagellates were found to have high uptake rates for urea and/or amino acids, and these rates were always higher than the rates for uptake (Kudela and Cochlan, 2000; Fan et al., 2003; Collos et al., 2007). In L. polyedrum, the urea uptake rate was also about 2 times more than that of , even if environmental urea concentrations were less than (Kudela and Cochlan, 2000). Taken together, these observations suggest that dinoflagellates possess a full suite of transporters for inorganic N and organic N forms, that they have the biochemical means to assimilate these N forms, and that they show a great physiological plasticity in response to external N types and concentrations.
Dinoflagellates are able to store large amounts of inorganic and organic N forms. Comparison of N uptake and assimilation rates at various growth rates in A. minutum showed that most of the and taken up in 1 h was not assimilated, and it was hypothesized that the unassimilated N was stored within the cell (Maguer et al., 2007). This species was also found to have a great storage capacity for amino acids (Flynn et al., 1996), and a similar storage capability was also described in A, catenella, A. tamarense, and Amphidinium cartarae as well as other unicellular algae (Dortch et al., 1984; Collos et al., 2004; Fauchot et al., 2005). One recent study, using Nanoscale Secondary-Ion Mass Spectrometry (NanoSIMS) and transmission electron microscopy, showed that Symbiodinium spp. temporarily stored N in the form of uric acid crystals after sudden increases in environmental N (Kopp et al., 2013). Indeed, pulses of 15N-labeled , or aspartic acid promoted accumulation of cytosolic crystalline uric acid inclusions in the zooxanthellae, which were formed in only 45 min in the case of . After 24 h of chasing with unlabeled- seawater, the inclusions completely dissolved and were remobilized uniformly within the cell. These results suggest that dinoflagellates might store their N within the cytosol, in contrast to plants where up to 50 mM of can be stored in the vacuoles (Cookson et al., 2005). The chemical nature of the long-term storage of N in dinoflagellates is still unclear.
Another interesting feature of N transport in dinoflagellates is the ability of some species to take up substantial amounts of various N forms in the darkness (Table 1). Dinoflagellates often display a diurnal vertical migration (DVM) in the water column and, because concentrations increase with depth, dark uptake was first described as a means to sustain uninterrupted growth by meeting their N requirements under conditions where the cells cannot photosynthesize (Harrison, 1976). It was further suggested that the DVM of dinoflagellates gave them a competitive advantage for N uptake over the non-motile diatoms (Harrison, 1976; Smayda, 1997). Paasche et al. (1984) showed that uptake efficiency and the N form preferentially taken up in the dark were species-specific. At one end of the spectrum, P. minimum took up and at similar rates in the light or in the dark, while at the other end, Gyrodinium aureolum (Hulburt) in the dark had smaller rates of uptake for and did not take up in N-sufficient conditions (Paasche et al., 1984). Recently, dark uptake of , and urea was confirmed in A. tamarense (Leong et al., 2010).
In contrast to dinoflagellates where molecular characteristics of transporters are mainly based on predictions from physiological studies, the three presently available diatom genomes have helped considerably to better appreciate the full extent of N transport and assimilation in these organisms (Armbrust et al., 2004; Bowler et al., 2008; Lommer et al., 2012). Consistent with their fast growth rate and high productivity, diatom genomes were found to contain multiple transporters for , , urea and other organic N forms (Armbrust et al., 2004; Bender et al., 2012). Analysis of genomes and studies in Cylindrotheca fusiformis revealed that diatoms seemed to possess twice as many transporters for compared to (Hildebrand and Dahlin, 2000; Allen, 2005; Hildebrand, 2005). It was suggested that these numbers reflect the fact that marine phytoplankton generally face low concentrations of , while concentrations are higher (Allen, 2005). Amino acid sequence analysis of five transporters of C. fusiformis showed that they shared 40% similarity with the vascular plant transporters AMT1 and AMT2 (Hildebrand, 2005). Furthermore, rescue by functional complementation of a yeast strain missing all three of its native transporters not only confirmed the functionality of the identified transporters, but also showed that AMT1 in diatoms were much more efficient transporters than AMT2 (Hildebrand, 2005). Identification and characterization of transporter sequences were also made in C. fusiformis (Hildebrand and Dahlin, 2000). As a general rule, genomic data and functional characterization showed that marine N transporters share sequence homology with N transporters of terrestrial and freshwater organisms, and that these tools can be used to better understand the responses of an organism to different N forms and concentrations. In dinoflagellates, the immense sizes of their genomes and the high gene copy number have long hindered sequencing projects. However, efforts are now being made to sequence the smallest of dinoflagellate genome, Symbiodinium (Shoguchi et al., 2013). There is no doubt that the presently available transcriptomic data for Alexandrium, Karenia, Lingulodinium, and Symbiodinium, as well as the upcoming genome sequences will help in unraveling the complexity of N transport and assimilation in dinoflagellates (Morey et al., 2011; Bayer et al., 2012; Beauchemin et al., 2012; Hackett et al., 2013).
Uptake of Nitrogen by Feeding
Because “food” comes from whole organisms (or parts of them), it contains a large spectrum of inorganic and organic matter, in which each individual compound is not ingested differently. For this reason, the following section will begin by describing general feeding mechanisms measured in mixotrophic studies of dinoflagellates, taking for granted that N is not ingested differentially than from other nutrients. Following this, we will discuss some specific examples of the N contribution obtained from feeding and of the inorganic N influence on the mixotrophic behavior of different dinoflagellates. We will finish with a model proposed by Jeong et al. (2010) where mixotrophy explains the outbreak and persistence of HABs in aquatic ecosystems limited in inorganic nutrients.
Mixotrophic dinoflagellates (MTDs) and heterotrophic dinoflagellates (HTDs) have similar feeding strategies. These strategies include (1) raptorial feeding, where the predator actively searches for its prey, (2) filter/interception, where the predator generates feeding currents to drag the prey into proximity of its feeding parts, and (3) diffusion feeding, where the predator passively wait until the prey comes close (Fenchel, 1987; Jeong et al., 2010). Raptorial feeding uses 3 mechanisms for ingestion of preys, (1) direct engulfment (phagocytosis), (2) pallium feeding, where a feeding veil is deployed around the prey, and (3) peduncle feeding, in which a straw-like structure is used to siphon out the prey’s cytoplasm (Jacobson, 1987; Jeong et al., 2010). Pallium feeding has still not been observed in MTDs, and thus seems to be unique to HTDs. HTDs are also able to engulf bigger preys than MTDs. In fact, the upper size limit of prey is generally proportional to the size of MTDs, while this correlation is not observed for HTDs (Jeong et al., 2005b). It was suggested that morphological adaptations to the sulcus, the “mouth” of most HTDs, as well as a stronger pulling force for ingestion of prey, could enable them to ingest larger prey (Jeong et al., 2010). In general, the prey upper size limits are greater when using pallium and peduncle feeding than when using engulfment. Spectacularly, some pallium and peduncle feeders are able to ingest prey up to 10 times their size (Jacobson, 1987). As for prey types, MTDs and HTDs feed on a wide array of taxa. They were shown to ingest cryptophytes, haptophytes, chlorophytes, prasiophytes, raphidophytes, diatoms, heterotrophic nanoflagellates, ciliates, and other dinoflagellates (Jacobson and Anderson, 1986; Hansen, 1991; Bockstahler and Coats, 1993b; Strom and Buskey, 1993; Nakamura et al., 1995; Tillmann, 2004; Jeong et al., 2005a, 2008; Menden-Deuer et al., 2005; Adolf et al., 2007; Berge et al., 2008). However, while some HTDs can feed on blood, flesh, eggs and early naupliar stages and adults forms of metazoans, no MTDs have been shown to do so (Miller and Belas, 2003; Parrow and Burkholder, 2004; Jeong et al., 2007).
In 1998, Stocker has proposed three physiological types of mixotrophic protists (Stoecker, 1998). Type III contains “photosynthetic” protozoa that require prey for growth and survival. In the same manner as HTDs, type III MTDs take the majority of their nutrients from feeding. Type I is made of “ideal” mixotrophs, which grow equally well either using light and inorganic nutrients or when they consume food. Very few MTDs with permanent chloroplasts are of this type. In fact, only 3 out of 36 reported species grew in the dark when prey was provided (Hansen, 2011). Most mixotrophs belong to type II, which was defined as “phagotrophic” algae that primarily photosynthesize and can assimilate inorganic nutrients. In this group, different proportions of N can be taken up either by transporters or through feeding. Estimations of these proportions have been made using in situ grazing experiments in Ceratium furca and Akashiwo sanguinea (Bockstahler and Coats, 1993a; Smalley and Coats, 2002). In C. furca, hourly ingestion rates (I) of the fluorescently labeled prey Strobilidium spp., were measured by dividing the mean number of food vacuoles in the predator with the incubation time of prey and predator. The % body N was then estimated as daily ingestion relative to cellular N content of C. furca [100 × 24 h × I × (cellular N of prey/cellular N C. furca)]. On average, the % N obtained through feeding was 6.5 % with a maximal value of 51 % (Smalley and Coats, 2002). Similarly in A. sanguinea, a gut clearance/gut fullness approach estimated an average of 4 % N obtained through feeding with a maximal value of 18.5 % (Bockstahler and Coats, 1993a). Both of these species have low averages, which signifies that under low light and with inorganic nutrient concentrations normally found in the environment, uptake of N by transporters is dominant over feeding. Interestingly, C. furca and A. sanguinea show only a marginal increase in growth rate with increasing prey concentrations (Hansen, 2011). However, in another 6 out of 36 chloroplast-containing species, a large increase in growth rates with increasing prey concentrations was observed (Hansen, 2011). Although the % body N in these species was not estimated, it could be argued that a larger proportion of N was obtained through feeding compared to C. furca or A. sanguinea. An extreme case of mixotrophy is observed in some dinoflagellates such as the Dinophysis genera that may contain chloroplasts of cryptophyte, haptophyte or cyanobacterial origin (Qiu et al., 2011). All the species studied in this genus cannot grow on an inorganic medium alone, as all require both light and prey for growth and survival (Park et al., 2006; Kim et al., 2008; Nishitani et al., 2008a,b; Garcia-Cuetos et al., 2010; Hansen, 2011). Dinophysis spp. are thus considered obligate mixotrophs. It is still unclear what are the proportions of N obtained by feeding and by transporters in these organisms.
The grazing behavior of some MTDs was shown to be under the influence of light and inorganic nutrient concentrations. In laboratory cultures of Gyrodinium galatheanum and C. furca, the incidence of feeding was negatively correlated with the amount of inorganic N present in the medium (Li et al., 2000; Smalley et al., 2003). In C. furca, cells only started to ingest food after 11 to 16 days in -depleted medium (Smalley et al., 2003). Similarly in P. minimum, additions of inhibited feeding (Stoecker et al., 1997). Curiously, this organism followed a diel pattern of prey ingestion with peaks in the afternoon and evening, and a trough in the morning. Moreover, the number of food vacuoles observed within P. minimum cells did not change between surface populations and the ones deeper in the water column. Because of these spatio-temporal evidences, the authors suggested that feeding in this organism was primarily a means for obtaining limiting nutrients, and not a mechanism to supplement C nutrition during light limitation (Stoecker et al., 1997). In contrast to the above-mentioned species, prey ingestion rates in Fragilidium cf. mexicanum was shown to be independent of inorganic N concentrations (Jeong et al., 1999). Also, most of the chloroplast-containing MTDs were reported to ingest prey in N-replete conditions (Hansen, 2011). Thus, the effects of inorganic N on grazing behavior must be species-specific.
It was long believed that bacteria were too small to be ingested by dinoflagellates. In the last few years, however, fluorescence and transmission electron microscopy observations revealed that multiple HTDs and MTDs were able to feed on heterotrophic bacteria and cyanobacteria (Jeong et al., 2005a, 2008; Seong et al., 2006; Glibert et al., 2009). In particular, feeding on the N2-fixing Synechococcus spp. was seen in 18 species reported to form HABs (Jeong et al., 2005a; Seong et al., 2006; Glibert et al., 2009). Generally, when prey concentration was high (106 cells/ml), the ingestion rates increased with increasing size of the dinoflagellate predators (Jeong et al., 2005a). Moreover, ingestion rates of Synechococcus were comparable to those observed in heterotrophic nanoflagellates (Seong et al., 2006). A mixture of P. mininum and P. donghaiense was able to remove up to 98% of the Synechococcus population within 1 h, showing that grazing by these species on bacteria could be very substantial (Jeong et al., 2005a). Thus, bacterivory in dinoflagellates was suggested to be a cause of HABs outbreaks and persistence in nutrient-limited waters (Glibert et al., 2009; Jeong et al., 2010). A model was further proposed where MTDs would supply their N requirement by ingesting cyanobacteria, while meeting their P requirement by ingesting heterotrophic bacteria, which are reported to generally have a high P:N ratio (Jeong et al., 2010). This model as yet to be tested in the environment.
Nitrogen Assimilation and Metabolism
Once inorganic N has entered the cells, phototrophic and MTDs can either store it or assimilate it in the form of amino acids. Whether organic N is taken up by means of transport or by ingestion of food, all dinoflagellates must metabolize it for further use using enzymes such as ureases, hydrolases, peptidases, and aminotransferases (also known as transaminases). In this section, we will start by giving a brief description of the enzymes involved in inorganic N assimilation, based on the available knowledge from plants and algae. We will follow with different evidence on the presence of these enzymes in dinoflagellates, particularly the NR of L. polyedrum, which was found to be under the control of a circadian clock. We will finish with a description of N assimilation in diatoms with emphasis on the newly discovered ornithine-urea cycle (OUC).
There are two structurally different types of NR, dissimilatory and assimilatory. The first type is found mainly in anaerobic prokaryotes, but is also present in some eukaryotes, such as the benthic foraminiferans and some fungi (Shoun and Tanimoto, 1991; Usuda et al., 1995; Risgaard-Petersen et al., 2006). These organisms use their dissimilatory NR to respire instead of O2. Direct reduction of to is a process named dissimilatory reduction to ammonium (DNRA), and is well documented in prokaryotes such as large sulfur bacteria (Preisler et al., 2007). Surprisingly, DNRA was also identified in the benthic diatom Amphora coffeaeformis (Kamp et al., 2011). The authors suggested that this organism respired as a means to survive dark and anoxic conditions. Considering that some dinoflagellates can form resting cysts that will sink in the benthic zone, and that others are also living there, dinoflagellates might also rely on the energy produced by dissimilatory NRs for excystment and for survival. However, the second type, assimilatory NR (usually just called NR) is the most common form in both eukaryotes and prokaryotes and is the type that was implied until now in this review. It catalyzes the reduction of to for assimilation. NRs usually form homodimers, but homotetramers have been reported in organisms such as the green algae Chlorella (Howard and Solomonson, 1982). Each monomer contains an electron transport chain made of three prosthetic groups: a flavine adenine dinucleotide (FAD), a cytochrome b557 and a molybdenum cofactor (Guerrero et al., 2003; Heldt and Piechulla, 2011). These groups are ubiquitous in all reported NR and are necessary for the stepwise transfer of 2 electrons from NAD(P)H to in eukaryotes. In contrast, prokaryotes use ferredoxins (Fd) or NADH for reduction of and their NR is generally membrane-bound whereas the eukaryotic one is soluble (Moreno-Vivian et al., 1999; Guerrero et al., 2003). Eukaryotic NRs are generally localized to the cytoplasm.
In photosynthetic eukaryotes, once has been reduced, the resulting is imported into the chloroplasts where it will be further reduced. There are two types of assimilatory NiR. The first one uses reduced-Fd from the photosynthetic transport chain as an electron donor, while the second one, usually found in non-photosynthetic organisms, uses NAD(P)H (Guerrero et al., 2003). Both types require six electrons to catalyze the reduction of to . Similarly to NR, Fd-NiRs contain three prosthetic groups: an iron-sulfur center, a FAD and a siroheme (Guerrero et al., 2003; Heldt and Piechulla, 2011). Most of NiRs are soluble and work as individual enzymes. Generally, NiRs have a greater affinity for than NRs have for (Guerrero et al., 2003). As a consequence, is completely reduced to and does not accumulate within the cells. In plants and algae, there are GS localized to the chloroplast and the cytosol (Chen and Silflow, 1996; Fernandez and Galvan, 2007; Forde and Lea, 2007). GS adds to Glu using ATP to produce 1 molecule of Gln. Gln is a central metabolite, which acts as a precursor for the biosynthesis of purines, pyrimidines, proteins, and ureides (Forde and Lea, 2007). GOGATs are found exclusively in the chloroplasts/plastids in plants and algae (Fernandez and Galvan, 2007; Forde and Lea, 2007). They combine Gln to 2-oxoglutarate using 2 electrons from either reduced-Fd or NAD(P)H and produce 2 molecules of Glu. The α-amino group of Glu can then be transferred by amidotransferases to a wide variety of 2-oxo acid acceptors to form amino acids, and back to form Glu, when amino acids and 2-oxoglutatare are abundant. GDH present in the mitochondria can also use and 2-oxoglutarate to produce Glu. Thus, Glu and Gln are at the crossroads of amino acid metabolism where they act both as N acceptors and N donors.
The presence of all inorganic N assimilation enzymes was tested indirectly or directly in some MTDs. In Symbiodinium, analysis of transcriptomic data revealed sequences for NR and NiR (Leggat et al., 2007; Bayer et al., 2012). Moreover, NanoSIMS observations showed that after pulses of 15N-labeled , zooxanthellae assimilated this N form before translocation to the coral host (Kopp et al., 2013). Because the host does not encode any NR or NiR genes, it was concluded that the dinoflagellate symbionts were responsible for the assimilation. In dinoflagellates, it is believed that N is principally assimilated by the GS-GOGAT pathway. In fact, GS activities were directly measured in S. microadriaticum and the use of the GS inhibitor L-methionine sulfoximine (MSX) strongly reduced the uptake of (Anderson and Burris, 1987). Use of the GOGAT inhibitor azaserine also led to inhibition of uptake (Rahav et al., 1989). Moreover, 1 h pulses in -enriched seawater led to a relative ~2 fold increase in levels of Gln and Glu, followed by a decrease back to control levels after a 3 h chase with normal seawater (Pernice et al., 2012). Increases in levels of Gln and Glu were also reported when pulses of 15N-labeled , or urea were applied (Summons and Osmond, 1981). In Karenia brevis, a microarray-analysis of N-depleted cultures revealed that sequences for GS and transporters of and were upregulated compared to N-replete cultures (Morey et al., 2011). In L. polyedrum, a NR was purified by affinity chromatography and its activity was measured using as substrate (Ramalho et al., 1995). Antibodies were then raised against the protein in order to measure the daily level of expression. Interestingly, it was found that both NR activity and the amount of the protein oscillated under light-dark and constant light conditions, the latter being a hallmark of circadian rhythms (Ramalho et al., 1995). Furthermore, it was reported in this organism that deficiency shortened the period of the circadian rhythms of bioluminescence and photosynthesis. MSX added to cultures containing a saturating amount of mimicked the effects of deficiency on circadian period, arguing for the existence of GS. Altogether, these experiments strongly suggest MTDs possess the full suite of enzymes required for N assimilation and that these enzymes are homologous to those of other photosynthetic eukaryotes.
Circadian clocks confer a selective advantage to organisms by preparing their internal biochemistry for upcoming rhythmic environmental cues, the most typical of these being light and darkness produced the succession of days and nights. These rhythmic cues are called zeitgebers (time givers), because an organism’s circadian clock can entrain or synchronize to it. The fact that -deficiency or pulses act on the phase and the period of the endogenous clock of L. polyedrum means that acts as a non-photic zeitgeber (Roenneberg and Rehman, 1996). It also indicates that the internal clock can react adaptively to changes in concentration. Usually the greatest responses of a zeitgeber happen during the times when the organism is least likely to receive a cue, for example by light during the night. L. polyedrum swims at the surface during the day, but in the late afternoon sinks in the water column to where concentrations are higher. Thus, the effects of pulses are greatest early at dawn where the organisms must optimize between taking and assimilating more or photosynthesizing. However, these effects on the clock must be interpreted carefully since the greatest uptake rates of or in all dinoflagellate species tested, including L. polyedrum, were obtained during the middle of the light period rather than the night (Harrison, 1976; Dortch and Maske, 1982; Paasche et al., 1984). This suggests that the C skeletons and electrons provided by photosynthesis are more important factors affecting the efficiency of uptake than is the N abundance alone. Furthermore, because the circadian clock also controls the activity and expression of the NR in L. polyedrum, it means the N metabolism in this species is both involved in the inputs and the outputs of the clock. Circadian-control of the NiR activity in C. reinhardtii and on the expression of the NR gene, NIA2, in Arabidopsis thaliana has also been reported (Pilgrim et al., 1993; Iliev et al., 2006). Thus, circadian regulation of the N metabolism in MTDs might be more common than what is currently appreciated.
All the enzymes needed for N assimilation have been identified in diatoms by analysis of their genome sequence (Armbrust et al., 2004; Bowler et al., 2008; Lommer et al., 2012). Surprisingly, the genome also encoded all enzymes required for the OUC, present in metazoans but absent in plants and green algae (Armbrust et al., 2004; Allen et al., 2011). This cycle seems to be fully integrated within diatom metabolism, principally through ornithine and arginine intermediates. Ornithine is a precursor for the synthesis of polyamines, which, among other functions, are necessary for the precipitation of silica during frustule formation, in a reaction catalyzed by ornithine decarboxylase (Armbrust et al., 2004). Ornithine can also be directly converted to proline by ornithine cyclodeaminase, making this intermediate an entry point into amino acid metabolism. As for arginine, it is used by nitric oxide synthase to synthesize nitric oxide, an important signaling molecule in plants and animals (Gupta et al., 2011). A pathway leading to the formation of the energy-storage molecule creatine-phosphate, was also found to originate from arginine (Armbrust et al., 2004). Integration of the OUC with the TCA cycle and the GS-GOGAT pathway (see next section) were also shown in Phaeodactylum tricornutum (Allen et al., 2011). In order to check if dinoflagellates could also possess all the genes necessary for the OUC, BLAST searches against dinoflagellate nucleotide databases were performed using the protein sequences of T. pseudonana and P. tricornutum as queries. In fact, Alexandrium tamarense had candidates for all OUC enzymes (Figure 2, Table 2). This suggests dinoflagellates could also possess a complete OUC. In diatoms, OUC was recognized as an important N remobilization hub, and it has been suggested that it might give a general advantage when urea concentrations are high or any form of N are abundant in the environment. This was thought to perhaps explain the success and prevalence of diatoms over other phytoplankton species in eutrophic waters (Allen et al., 2011), but this idea may have to be revisited if dinoflagellates are also able to catalyze the same reactions.
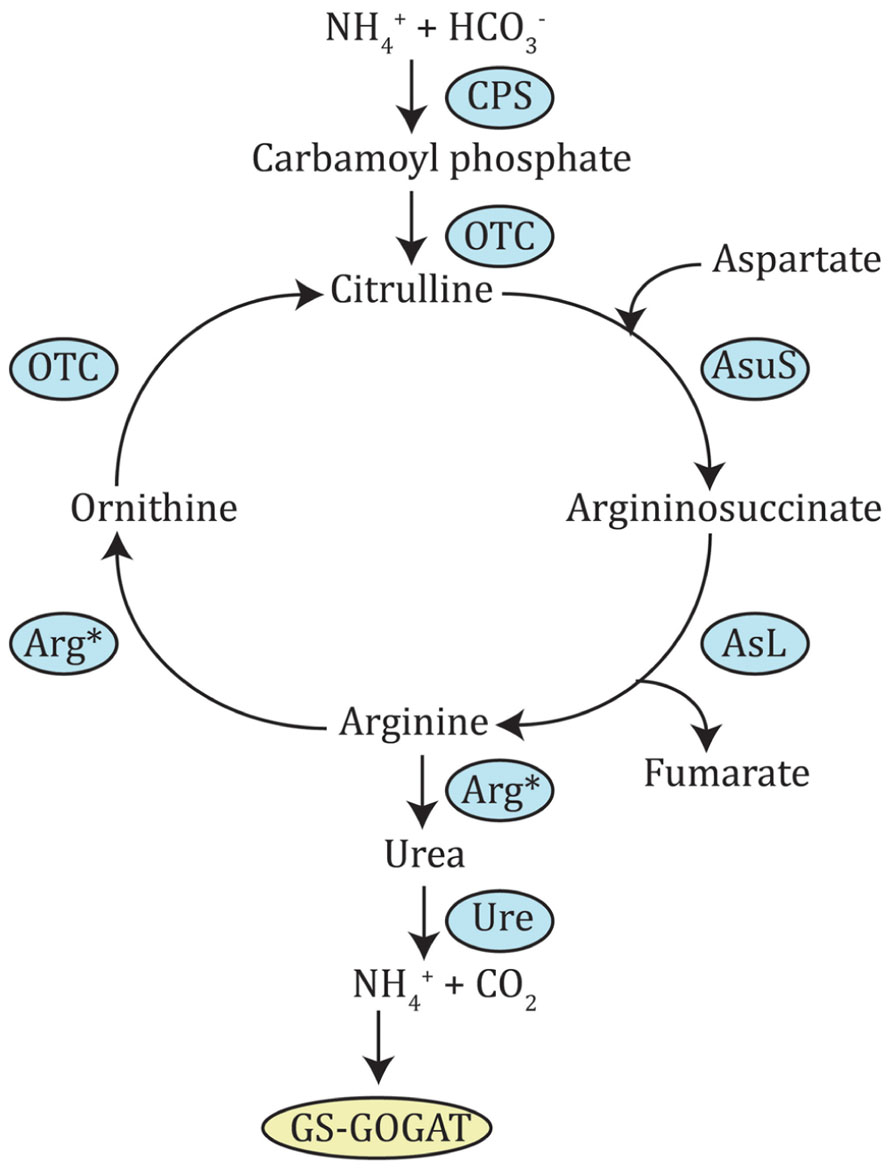
FIGURE 2. Ornithine-urea cycle. This pathway is derived from that identified in diatoms. CPS, carbamoyl phosphate synthase; OTC, ornithine carbamoyltransferase; AsuS, argininosuccinate synthase; AsL, argininosuccinate lyase; Arg*, arginase (depicted twice, because it produces both ornithine and urea); Ure, urease.
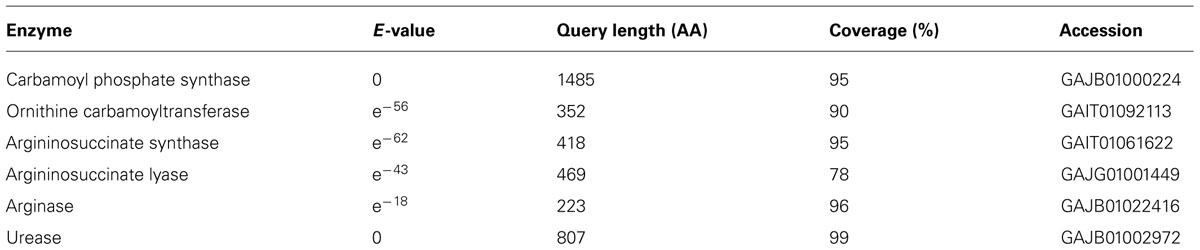
TABLE 2. Nitrogen metabolizing enzymes similar to those in diatoms in the transcriptome of Alexandrium tamarense.
Adaptations to Nitrogen Stress
Generally, dinoflagellates and diatoms need to cope with varying concentrations of N, particularly in the open ocean where it is often limiting. Thus, each group has developed various strategies, some of which are used by both, to respond and survive to oligotrophic environments (Table 1). In this section, we will present these responses based on environmental and laboratory experiments. We will discuss symbiosis and various physiological, behavioral and transcriptomic responses, with an emphasis on dinoflagellates.
Symbiosis with diazotrophs is an example of a strategy that is shared by some diatom and dinoflagellate species. The diatom genera Hemiaulus and Rhizosolenia both form endosymbiotic associations with the cyanobacteria Richelia intracellularis (Venrick, 1974; Carpenter et al., 1999). Both the hosts and the symbionts were observed to bloom together in the oligotrophic waters of the North Pacific Central Gyre and South West Atlantic Ocean. N2-fixation by Richelia introduced an amount of “new N” to the ecosystems that could even exceed the N2 fixed by non-blooming Trichodesmium. Carpenter et al. (1999) suggested that the silicate- and iron-enriched water of the Amazon River could have been factors in initiating and sustaining the blooms in the SW Atlantic Ocean. Silicate is required for the formation of the diatom frustule, while iron is necessary for the action of the diazotroph nitrogenases.
In N-limited conditions, endo- and ecto-symbiosis with cyanobacteria were exclusively identified within the dinoflagellate order Dinophysiales (Tarangkoon et al., 2010). In the genera Amphisolenia and Triposolenia, the symbionts were found inside the host, while the genera Ornithocercus, Histioneis, Parahistioneis, and Citharistes appeared to “farm” cultures of cyanobacteria outside the cells in an enlarged cingulum or in special cavities (Tarangkoon et al., 2010; Hansen, 2011). Further microscopic observations of Ornithocercus spp. suggested that they could also ingest their symbionts, depending on the size, shape, and color of the prey and on the presence of peduncle in the dinoflagellates. However, it is not clear if these species take up the majority of the external N fixed by the cyanobacteria using transporters and ingest the bacteria only occasionally, or if they “farm” the symbionts exclusively to feed on them. While it was not directly shown that Symbiodinium formed symbiotic association with cyanobacteria, the coral host was found to do so. In fact, whole communities of beneficial bacteria including N2-fixers and chitin decomposers were identified in all coral structures, including the surface mucous layer, tissue layers and the skeleton (Lesser et al., 2004; Rosenberg et al., 2007). Interestingly, amplification of the nitrogenase gene nifH in tissues of 3 different coral species revealed that 71% of the sequences came from a bacterial group closely related to rhizobia, the N2-fixers symbiotic with legumes (Lema et al., 2012). The products of N2-fixation were initially assimilated by the zooxanthellae, then translocated to the animal host, as determined by δ15N analysis (Lesser et al., 2007). Moreover, Symbiodinium population density was positively correlated with nifH sequence copy number, suggesting that growth and division of the zooxanthellae might be dependent upon the product of N2-fixation (Olson et al., 2009). Taken together, these examples suggest that the cyanobacteria barter their N2-fixing ability for protection and nutrients from their hosts, thus providing a selective advantage to the hosts in N-limited environments.
For the majority of diatom and dinoflagellate species, unable to form any symbiotic associations with diazotrophs, N-limitation sets in motion a series of physiological and behavioral modifications to avoid cell death. Generally, in both groups, N-limitation results in a decrease in the levels of proteins and chlorophylls (Lei and Lu, 2011; Hockin et al., 2012; Pleissner and Eriksen, 2012). Toxin content in Alexandrium sp. and Ostreopsis ovata was also shown to diminish when N-stressed (Wang and Hsieh, 2002; Leong et al., 2004; Hu et al., 2006; Vanucci et al., 2012). Furthermore, N-limitation or complete N-starvation induces a reduction or an arrest of the cell cycle in both groups (Lei and Lu, 2011; Morey et al., 2011; Hockin et al., 2012; Vanucci et al., 2012). The phase at which arrest occurs was shown to vary in different dinoflagellate species. For example, when completely N deprived, A. cartarae cells stop in G1, while P. piscicida cells stall at both G1 and G2/M phases (Olson and Chisholm, 1986; Lin et al., 2004). Of course, independent of the phase of cell cycle arrest, the resulting consequence is the same: cells cease dividing. At this point, organisms reach a state of stasis where the cells will either form cysts or store/remobilize their internal metabolites until N becomes available again. In photosynthetic eukaryotes, photosynthesis still occurs when N is limiting and C skeletons can accumulate. These were shown to be stored in the form of starch and/or as lipids in plants and green algae (Wingler et al., 2006; Wang et al., 2009). In dinoflagellates, accumulation of lipids in the form of triacylglycerol was found to be ~200% higher in N-limited conditions compared to control (Fuentes-Grunewald et al., 2012). In contrast, N-limitation in the HTD Crypthecodinium cohnii did not have any significant effects on the rates of lipid production (Pleissner and Eriksen, 2012). Thus, N stress may have different effects on the regulation of the enzymes involved in C storage between dinoflagellate groups.
Diatoms can also accumulate lipids, but only when cultured under very low N concentrations (Armbrust et al., 2004; Kroth et al., 2008; Hockin et al., 2012; Yang et al., 2013). At the early stages of N-stress, when the intracellular concentrations of , amino acids and proteins were about half the amount measured in N-replete cultures, no changes in C stores such as starch or lipids were detected in T. pseudonana (Hockin et al., 2012). It was proposed that diatoms had a greater tendency to remobilize rather than to store C compounds, because their OUC gives them the potential to increase the efficiency of N reassimilation from catabolic processes (Allen et al., 2006). An augmentation of reassimilation would lead to a greater use of C skeletons compared to organisms lacking a urea cycle (Hockin et al., 2012). This N remobilization hypothesis was suggested by the finding that recovery from N-stress in P. tricornutum was impaired in a RNA interference (RNAi) line having a reduced level of carbamoyl phosphate synthase, the enzyme catalyzing the first committed step of the OUC (Allen et al., 2011). Indeed, compared to wild type, this mutant accumulates less of most metabolites in the tricarboxylic acid (TCA) cycle and OUC. There are also reduced levels of Gln and other amino acids in the RNAi line, indicating that the OUC acts to link the TCA cycle and the GS-GOGAT pathway together. Thus, the OUC, being at the crossroad of catabolic and anabolic metabolism, was described as a distribution and repackaging hub for C and N compounds, which could have contributed to the success of diatoms in the modern oceans (Allen et al., 2011).
In some dinoflagellates, such as the MTD species G. galatheanum, C. furca, and P. minimum, N-limitation elicits a change in their N nutritional strategy, which switches from a primarily inorganic N uptake by transporters to uptake relying on both transporters and feeding mechanisms. A similar change in behavior was also recently shown to occur in free-living Symbiodinium spp. (Jeong et al., 2012), as while the cells ingested prey in both N-replete and N-limited conditions, the feeding rates were higher in the latter. In these experiments, ingestion of the raphidophyte H. akashiwo in -limited conditions contributed up to 229% of Symbiodinium spp. daily body N compared to 105% in -replete conditions. In other dinoflagellates, such as the type III MTD P. piscicida, when the amount of prey was reduced to ~1% of the predator population in the presence of high concentrations of DIN and DON (principally ), uptake rates by transporters increased to levels similar to those cited for phytoplankton (Lewitus et al., 1999). Indeed, the highest rates of N uptake by transporters were comparable to the rates of N uptake by ingestion in P. piscicida cultures containing high amounts of prey (Lewitus et al., 1999). Clearly, some MTDs adapt to the N status of the environment by modifying their feeding behavior and altering the expression or activity of N transporters.
Transcriptional regulation of N metabolism is widespread in plants and algae (Galvan et al., 1996; Fernandez and Galvan, 2008; Lillo, 2008). In diatoms, comparative analysis of expressed sequence tags (ESTs) libraries under N stress in T. pseudonana and P. tricornutum revealed altered expression of numerous genes involved in N transport and assimilation (Maheswari et al., 2010). In this study, an transporter was among the most up-regulated genes in N-depleted P. tricornutum cells. Similarly, in C. fusiformis, AMT1 and AMT2 transcript levels were highest in N-starved conditions (Hildebrand, 2005). Curiously, other transcripts, such as the one encoding NR in C. fusiformis, do not respond to variations in concentrations, although its expression is inhibited by (Poulsen and Kröger, 2005). These examples highlight the complex regulation of different N metabolism genes in diatoms.
Interestingly, in dinoflagellates, different high-throughput analysis of transcriptomes under N-stressed conditions revealed that transcriptional control also seems to occur in these organisms. Massively parallel signature sequencing (MPSS) in Alexandrium fundyense revealed that ~10% of the signatures were differentially expressed between N-stressed and P-stressed cells (Erdner and Anderson, 2006). This does not mean that 10% of the transcripts varied, however, as rapid amplification of cDNA ends (RACE) sequencing and mapping to known dinoflagellate genes showed that multiple signatures arose from sequence variants of individual genes. This redundancy was ascribed to the gene duplication events commonly described in dinoflagellate genomes (Erdner and Anderson, 2006). MPSS of Alexandrium tamarense cultures grown under various stresses was also performed, but in this study most of the differentially expressed genes were observed in comparisons between xenic and axenic cultures, rather than the N-limited ones (Moustafa et al., 2010). In Karenia brevis, a microarray comparison of N-limited and N-replete cells showed that ~11% of the probes differ between the two conditions (Morey et al., 2011). Interestingly, an transporter, / transporters and type III GSs were among the sequences up regulated under N-limitation. These experiments show that stress can induce transcriptional responses in dinoflagellates, which presumably act to fine tune dinoflagellate N metabolism in relation to environmental N status.
Conclusion
Dinoflagellates use various strategies to acquire and assimilate N, depending on their trophic preferences and life styles. Some make an extensive use of transporters, others preferentially ingest prey and still others exploit both strategies equally. Enzymes for uptake and assimilation of N seem to share homology and kinetic properties with those reported in other photosynthetic eukaryotes. A remarkable feature both found in diatoms and dinoflagellates is their adaptability to changes in environmental N concentrations, particularly under stress conditions. Some mechanisms are common, such as symbiosis and transcriptional control; others are particular to dinoflagellates, like mixotrophy and vertical migration. With the advent of next-generation sequencing technologies, transcriptomic and even genomic tools will soon help in identifying and characterizing the molecular components involved in dinoflagellate N metabolism. Understanding how N is put in dinoflagellates will certainly help to better predict their behavior into our future anthropogenically altered aquatic ecosystems.
Conflict of Interest Statement
The authors declare that the research was conducted in the absence of any commercial or financial relationships that could be construed as a potential conflict of interest.
Acknowledgments
Funding by the National Science and Engineering Research Council of Canada (NSERC) in the form of a student fellowship (Steve Dagenais-Bellefeuille) and a research grant (David Morse, Grant number 171382-03) is gratefully acknowledged.
Author Contributions
Steve Dagenais-Bellefeuille and David Morse wrote the text and figures were drafted by Steve Dagenais-Bellefeuille.
References
Adolf, J. E., Krupatkina, D., Bachvaroff, T., and Place, A. R. (2007). Karlotoxin mediates grazing by Oxyrrhis marina on strains of Karlodinium veneficum. Harmful Algae 6, 400–412. doi: 10.1016/j.hal.2006.12.003
Allen, A. E. (2005). Beyond sequence homology: redundant ammonium transporters in a marine diatom are not functionally equivalent. J. Phycol. 41, 4–6. doi: 10.1111/j.0022-3646.2005.41101_2.x
Allen, A. E., Dupont, C. L., Obornik, M., Horak, A., Nunes-Nesi, A., McCrow, J. P., et al. (2011). Evolution and metabolic significance of the urea cycle in photosynthetic diatoms. Nature 473, 203–207. doi: 10.1038/nature10074
Allen, A. E., Vardi, A., and Bowler, C. (2006). An ecological and evolutionary context for integrated nitrogen metabolism and related signaling pathways in marine diatoms. Curr. Opin. Plant Biol 9, 264–273. doi: 10.1016/j.pbi.2006.03.013
Anderson, D. M., Alpermann, T. J., Cembella, A. D., Collos, Y., Masseret, E., and Montresor, M. (2012a). The globally distributed genus Alexandrium: multifaceted roles in marine ecosystems and impacts on human health. Harmful Algae 14, 10–35. doi: 10.1016/j.hal.2011.10.012
Anderson, D. M., Cembella, A. D., and Hallegraeff, G. M. (2012b). Progress in understanding harmful algal blooms: paradigm shifts and new technologies for research, monitoring, and management. Annu. Rev. Mar. Sci. 4, 143–176. doi: 10.1146/annurev-marine-120308-081121
Anderson, D. M., Burkholder, J. M., Cochlan, W. P., Glibert, P. M., Gobler, C. J., Heil, C. A., et al. (2008). Harmful algal blooms and eutrophication: examining linkages from selected coastal regions of the United States. Harmful Algae 8, 39–53. doi: 10.1016/j.hal.2008.08.017
Anderson, S. L., and Burris, J. E. (1987). Role of glutamine synthetase in ammonia assimilation by symbiotic marine dinoflagellates (zooxanthellae). Mar. Biol. 94, 451–458. doi: 10.1007/BF00428252
Armbrust, E. V., Berges, J. A., Bowler, C., Green, B. R., Martinez, D., Putnam, N. H., et al. (2004). The genome of the diatom Thalassiosira pseudonana: ecology, evolution, and metabolism. Science 306, 79–86. doi: 10.1126/science.1101156
Bayer, T., Aranda, M., Sunagawa, S., Yum, L. K., DeSalvo, M. K., Lindquist, E., et al. (2012). Symbiodinium transcriptomes: genome insights into the dinoflagellate symbionts of reef-building corals. PLoS ONE 7:e35269. doi: 10.1371/journal.pone.0035269
Beauchemin, M., Roy, S., Daoust, P., Dagenais-Bellefeuille, S., Bertomeu, T., Letourneau, L., et al. (2012). Dinoflagellate tandem array gene transcripts are highly conserved and not polycistronic. Proc. Natl. Acad. Sci. U.S.A. 109, 15793–15798. doi: 10.1073/pnas.1206683109
Beman, M. J., Arrigo, K. R., and Matson, P. A. (2005). Agricultural runoff fuels large phytoplankton blooms in vulnerable areas of the ocean. Nature 434, 211–214. doi: 10.1038/nature03370
Bender, S. J., Parker, M. S., and Armbrust, E. V. (2012). Coupled effects of light and nitrogen source on the urea cycle and nitrogen metabolism over a diel cycle in the marine diatom Thalassiosira pseudonana. Protist 163, 232–251. doi: 10.1016/j.protis.2011.07.008
Berge, T., Hansen, P., and Moestrup, O. (2008). Feeding mechanism, prey specificity and growth in light and dark of the plastidic dinoflagellate Karlodinium armiger. Aquat. Microb. Ecol. 50, 279–288. doi: 10.3354/ame01165
Bhattacharyya, P., and Volcani, B. E. (1980). Sodium-dependent silicate transport in the apochlorotic marine diatom Nitzschia alba. Proc. Natl. Acad. Sci. U.S.A. 77, 6386–6390. doi: 10.1073/pnas.77.11.6386
Bisson, M. A., Beilby, M. J., and Shepherd, V. A. (2006). Electrophysiology of turgor regulation in marine siphonous green algae. J. Membr. Biol. 211, 1–14. doi: 10.1007/s00232-006-0860-1
Bockstahler, K. R., and Coats, D. W. (1993a). Grazing of the mixotrophic dinoflagellate Gymnodinium sanguineum on ciliate populations of Chesapeake Bay. Mar. Biol. 116, 477–487. doi: 10.1007/BF00350065
Bockstahler, K. R., and Coats, D. W. (1993b). Spatial and temporal aspects of mixotrophy in Chesapeake Bay dinoflagellates. J. Eukaryot. Microbiol. 40, 49–60. doi: 10.1111/j.1550-7408.1993.tb04881.x
Bowler, C., Allen, A. E., Badger, J. H., Grimwood, J., Jabbari, K., Kuo, A., et al. (2008). The Phaeodactylum genome reveals the evolutionary history of diatom genomes. Nature 456, 239–244. doi: 10.1038/nature07410
Boyd, C. M., and Gradmann, D. (1999). Electrophysiology of the marine diatom Coscinodiscus wailesii III. Uptake of nitrate and ammonium. J. Exp. Bot. 50, 461–467. doi: 10.1093/jxb/50.333.461
Carpenter, E. J., Montoya, J. P., Burns, J., Mulholland, M. R., Subramaniam, A., and Capone, D. G. (1999). Extensive bloom of a N2-fixing diatom/cyanobacterial association in the tropical Atlantic Ocean. Mar. Ecol. Prog. Ser. 185, 273–283. doi: 10.3354/meps185273
Chang, F. H., and McClean, M. (1997). Growth responses of Alexandrium minutum (Dinophyceae) as a function of three different nitrogen sources and irradiance. N. Z. J. Mar. Freshwater Res. 31, 1–7. doi: 10.1080/00288330.1997.9516740
Chen, Q., and Silflow, C. D. (1996). Isolation and characterization of glutamine synthetase genes in Chlamydomonas reinhardtii. Plant Physiol. 112, 987–996. doi: 10.1104/pp.112.3.987
Collos, Y., Gagne, C., Laabir, M., Vaquer, A., Cecchi, P., and Souchu, P. (2004). Nitrogenous nutrition of Alexandrium catenella (Dinophyceae) in cultures and in Thau Lagoon, Southern France. J. Phycol. 40, 96–103. doi: 10.1046/j.1529-8817.2004.03034.x
Collos, Y., Vaquer, A., Laabir, M., Abadie, E., Laugier, T., Pastoureaud, A., et al. (2007). Contribution of several nitrogen sources to growth of Alexandrium catenella during blooms in Thau lagoon, southern France. Harmful Algae 6, 781–789. doi: 10.1016/j.hal.2007.04.003
Collos, Y., Vaquer, A., and Souchu, P. (2005). Acclimation of nitrate uptake by phytoplankton to high substrate levels. J. Phycol. 41, 466–478. doi: 10.1111/j.1529-8817.2005.00067.x
Cookson, S. J., Williams, L. E., and Miller, A. J. (2005). Light-dark changes in cytosolic nitrate pools depend on nitrate reductase activity in Arabidopsis leaf cells. Plant Physiol. 138, 1097–1105. doi: 10.1104/pp.105.062349
Davy, S. K., Allemand, D., and Weis, V. M. (2012). Cell biology of cnidarian-dinoflagellate symbiosis. Microbiol. Mol. Biol. Rev. 76, 229–261. doi: 10.1128/MMBR.05014-11
De Angeli, A., Monachello, D., Ephritikhine, G., Frachisse, J. M., Thomine, S., Gambale, F., et al. (2006). The nitrate/proton antiporter AtCLCa mediates nitrate accumulation in plant vacuoles. Nature 442, 939–942. doi: 10.1038/nature05013
Doddema, H., and Telkamp, G. P. (1979). Uptake of nitrate by mutants of Arabidopsis thaliana, disturbed in uptake or reduction of nitrate. Physiol. Plant. 45, 332–338. doi: 10.1111/j.1399-3054.1979.tb02593.x
Dortch, Q., Clayton, J. R. Jr., Thoresen, S. S., and Ahmed, S. I. (1984). Species differences in accumulation of nitrogen pools in phytoplankton. Mar. Biol. 81, 237–250. doi: 10.1007/BF00393218
Dortch, Q., and Maske, H. (1982). Dark uptake of nitrate and nitrate reductase activity of a red-tide population off peru. Mar. Ecol. Prog. Ser. 9, 299–303. doi: 10.3354/meps009299
Epstein, E., and Hagen, C. E. (1952). A kinetic study of the absorption of alkali cations by barley roots. Plant Physiol. 27, 457–474. doi: 10.1104/pp.27.3.457
Epstein, E., Rains, D. W., and Elzam, O. E. (1963). Resolution of dual mechanisms of potassium absorption by barley roots. Proc. Natl. Acad. Sci. U.S.A. 49, 684–692. doi: 10.1073/pnas.49.5.684
Erdner, D. L., and Anderson, D. M. (2006). Global transcriptional profiling of the toxic dinoflagellate Alexandrium fundyense using massively parallel signature sequencing. BMC Genomics 7:88. doi: 10.1186/1471-2164-7-88
Fan, C., Glibert, P. M., and Burkholder, J. M. (2003). Characterization of the affinity for nitrogen, uptake kinetics, and environmental relationships for Prorocentrum minimum in natural blooms and laboratory cultures. Harmful Algae 2, 283–299. doi: 10.1016/S1568-9883(03)00047-7
Fauchot, J., Levasseur, M., Roy, S., Gagnon, R., and Weise, A. M. (2005). Environmental factors controlling Alexandrium tamarense (Dinophyceae) growth rate during a red tide in the St-Lawrence estuary (Canada). J. Phycol. 41, 263–272. doi: 10.1111/j.1529-8817.2005.03092.x
Fenchel, T. (1987). Ecology of protozoa: the biology of free-living phagotrophic protists. Berlin: Springer-Verlag.
Fensome, R. A., Saldarriaga, J. F., and Taylor, F. J. R. M. (1999). Dinoflagellate phylogeny revisited: reconciling morphological and molecular based phylogenies. Grana 38, 66–80. doi:10.1080/00173139908559216
Fernandez, E., and Galvan, A. (2007). Inorganic nitrogen assimilation in Chlamydomonas. J. Exp. Bot. 58, 2279. doi: 10.1093/jxb/erm106
Fernandez, E., and Galvan, A. (2008). Nitrate assimilation in Chlamydomonas. Eukaryot. Cell 7, 555–559. doi: 10.1128/EC.00431-07
Flynn, K., Jones, K. J., and Flynn, K. J. (1996). Comparisons among species of Alexandrium (Dinophyceae) grown in nitrogen- or phosphorus-limiting batch culture. Mar. Biol. 126, 9–18. doi: 10.1007/BF00571372
Forde, B. G., and Lea, P. J. (2007). Glutamate in plants: metabolism, regulation, and signalling. J. Exp. Bot. 58, 2339–2358. doi: 10.1093/jxb/erm121
Franco, A. R., Cardenas, J., and Fernandez, E. (1988). Two different carriers transport both ammonium and methylammonium in Chlamydomonas reinhardtii. J. Biol. Chem. 263, 14039–14043.
Fritz, L., Stringher, C. G., and Colepicolo, P. (1996). Immunolocalization of nitrate reductase in the marine dinoflagellate Gonyaulax polyedra (Pyrrophyta). J. Phycol. 32, 632–637 doi: 10.1111/j.0022-3646.1996.00632.x
Fuentes-Grunewald, C., Garces, E., Alacid, E., Sampedro, N., Rossi, S., and Camp, J. (2012). Improvement of lipid production in the marine strains Alexandrium minutum and Heterosigma akashiwo by utilizing abiotic parameters. J. Ind. Microbiol. Biotechnol. 39, 207–216. doi: 10.1007/s10295-011-1016-6
Galloway, J. N., Dentener, F. J., Capone, D. G., Boyer, E. W., Howarth, R. W., Seitzinger, S. P., et al. (2004). Nitrogen cycles: past, present, and future. Biogeochemistry 70, 153–226. doi: 10.1007/s10533-004-0370-0
Galvan, A., and Fernandez, E. (2001). Eukaryotic nitrate and nitrite transporters. Cell. Mol. Life Sci. 58, 225–233. doi: 10.1007/PL00000850
Galvan, A., Quesada, A., and Fernandez, E. (1996). Nitrate and nitrite are transported by different specific transport systems and by a bispecific transporter in Chlamydomonas reinhardtii. J. Biol. Chem. 271, 2088–2092. doi: 10.1074/jbc.271.4.2088
Garcia-Cuetos, L., Moestrup, Ò., Hansen, P. J., and Daugbjerg, N. (2010). The toxic dinoflagellate Dinophysis acuminata harbors permanent chloroplasts of cryptomonad origin, not kleptochloroplasts. Harmful Algae 9, 25–38. doi: 10.1016/j.hal.2009.07.002
Gaspar, M., Bousser, A., Sissoeff, I., Roche, O., Hoarau, J., and Mahe, A. (2003). Cloning and characterization of ZmPIP1-5b, an aquaporin transporting water and urea. Plant Sci. 165, 21–31. doi: 10.1016/S0168-9452(03)00117-1
Gestal, C., Novoa, B., Posada, D., Figueras, A., and Azevedo, C. (2006). Perkinsoide chabelardi n. gen., a protozoan parasite with an intermediate evolutionary position: possible cause of the decrease of sardine fisheries? Environ. Microbiol. 8, 1105–1114. doi: 10.1111/j.1462-2920.2006.01008.x
Gimmler, H. (2000). Primary sodium plasma membrane ATPases in salt-tolerant algae: facts and fictions. J. Exp. Bot. 51, 1171–1178. doi: 10.1093/jexbot/51.348.1171
Glibert, P. M., Burkholder, J. M., Kana, T. M., Alexander, J., Skelton, H., and Shilling, C. (2009). Grazing by Karenia brevis on Synechococcus enhances its growth rate and may help to sustain blooms. Aquat. Microb. Ecol. 55, 17–30. doi: 10.3354/ame01279
Gruber, N. (2008). “The marine nitrogen cycle: overview and challenges,” in Nitrogen in the Marine Environment, 2nd Edn, eds D. G. Capone, D. A. Bronk, M. R. Mulholland and E. J. Carpenter (San Diego: Academic Press), 1–50.
Guerrero, M. G., Vega, J. M., and Losada, M. (2003). The assimilatory nitrate-reducing system and its regulation. Annu. Rev. Plant Physiol. 32, 169–204. doi: 10.1146/annurev.pp.32.060181.001125
Guillou, L., Viprey, M., Chambouvet, A., Welsh, R. M., Kirkham, A. R., Massana, R., et al. (2008). Widespread occurrence and genetic diversity of marine parasitoids belonging to Syndiniales (Alveolata). Environ. Microbiol. 10, 3349–3365. doi: 10.1111/j.1462-2920.2008.01731.x
Gupta, K. J., Fernie, A. R., Kaiser, W. M., and van Dongen, J. T. (2011). On the origins of nitric oxide. Trends Plant Sci. 16, 160–168. doi: 10.1016/j.tplants.2010.11.007
Hackett, J. D., Wisecaver, J. H., Brosnahan, M. L., Kulis, D. M., Anderson, D. M., Bhattacharya, D., et al. (2013). Evolution of saxitoxin synthesis in Cyanobacteria and dinoflagellates. Mol. Biol. Evol. 30, 70–78. doi: 10.1093/molbev/mss142
Hansen, P. J. (1991). Quantitative importance and trophic role of heterotrophic dinoflagellates in coastal pelagial food web. Mar. Ecol. Prog. Ser. 73, 253–261. doi: 10.3354/meps073253
Hansen, P. J. (2011). The role of photosynthesis and food uptake for the growth of marine mixotrophic dinoflagellates. J. Eukaryot. Microbiol. 58, 203–214. doi: 10.1111/j.1550-7408.2011.00537.x
Harada, A., Ohtsuka, S., and Horiguchi, T. (2007). Species of the parasitic genus Duboscquella are members of the enigmatic Marine Alveolate Group I. Protist 158, 337–347. doi: 10.1016/j.protis.2007.03.005
Harrison, W. G. (1976). Nitrate metabolism of the red tide dinoflagellate Gonyaulax polyedra Stein. J. Exp. Mar. Biol. Ecol. 21, 199–209. doi: 10.1016/0022-0981(76)90115-5
Heldt, H.-W., and Piechulla, B. (2011). “Nitrate assimilation is essential for the synthesis of organic matter,” in Plant Biochemistry, 4th Edn, (San Diego: Academic Press), 273–305.
Hellebust, J. A. (1978). Uptake of organic substrates by Cyclotella cryptica (Bacillariophyceae): effects of ions, ionophores and metabolic and transport inhibitors. J. Phycol. 14, 79–83. doi: 10.1111/j.1529-8817.1978.tb00635.x
Hildebrand, M. (2005). Cloning and functional characterization of ammonium transporters from the marine diatom Cylindrotheca fusiformis (Bacillariophyceae). J. Phycol. 41, 105–113. doi: 10.1111/j.1529-8817.2005.04108.x
Hildebrand, M., and Dahlin, K. (2000). Nitrate transporter genes from the diatom Cylindrotheca fusiformis (Bacillariophyceae): mRNA levels controlled by nitrogen source and by the cell cycle. J. Phycol. 36, 702–713. doi: 10.1046/j.1529-8817.2000.99153.x
Ho, C.-H., Lin, S.-H., Hu, H.-C., and Tsay, Y.-F. (2009). CHL1 functions as a nitrate sensor in plants. Cell 138, 1184–1194. doi: 10.1016/j.cell.2009.07.004
Hockin, N. L., Mock, T., Mulholland, F., Kopriva, S., and Malin, G. (2012). The response of diatom central carbon metabolism to nitrogen starvation is different from that of green algae and higher plants. Plant Physiol. 158, 299–312. doi: 10.1104/pp.111.184333
Howard, W. D., and Solomonson, L. P. (1982). Quaternary structure of assimilatory NADH:nitrate reductase from Chlorella. J. Biol. Chem. 257, 10243–10250.
Howitt, S. M., and Udvardi, M. K. (2000). Structure, function and regulation of ammonium transporters in plants. Biochim. Biophys. Acta 1465, 152–170. doi: 10.1016/S0005-2736(00)00136-X
Hu, H. H., Chen, W. D., Shi, Y. J., and Cong, W. (2006). Nitrate and phosphate supplementation to increase toxin production by the marine dinoflagellate Alexandrium tamarense. Mar. Pollut. Bull. 52, 756–760. doi: 10.1016/j.marpolbul.2005.11.005
Iliev, D., Voytsekh, O., Schmidt, E. M., Fiedler, M., Nykytenko, A., and Mittag, M. (2006). A heteromeric RNA-binding protein is involved in maintaining acrophase and period of the circadian clock. Plant Physiol. 142, 797–806. doi: 10.1104/pp.106.085944
Jacobson, D. M. (1987). The ecology and feeding biology of thecate heterotrophic dinoflagellates. Ph.D thesis, Woods Hole Oceanographic Institution, Massachusetts Institute of Technology, USA, 210.
Jacobson, D. M., and Anderson, D. M. (1986). Thecate heterotrophic dinoflagellates – feeding-behavior and mechanisms. J. Phycol. 22, 249–258. doi: 10.1111/j.1529-8817.1986.tb00021.x
Jahn, T. P., Moller, A. L., Zeuthen, T., Holm, L. M., Klaerke, D. A., Mohsin, B., et al. (2004). Aquaporin homologues in plants and mammals transport ammonia. FEBS Lett. 574, 31–36. doi: 10.1016/j.febslet.2004.08.004
Jeong, H., Yoo, Y., Kim, J., Seong, K., Kang, N., and Kim, T. (2010). Growth, feeding and ecological roles of the mixotrophic and heterotrophic dinoflagellates in marine planktonic food webs. Ocean Sci. J. 45, 65–91. doi: 10.1007/s12601-010-0007-2
Jeong, H. J., Ha, J. H., Yoo, Y. D., Park, J. Y., Kim, J. H., Kang, N. S., et al. (2007). Feeding by the Pfiesteria-like heterotrophic dinoflagellate Luciella masanensis. J. Eukaryot. Microbiol. 54, 231–241. doi: 10.1111/j.1550-7408.2007.00259.x
Jeong, H. J., Park, J. Y., Nho, J. H., Park, M. O., Ha, J. H., Seong, K. A., et al. (2005a). Feeding by red-tide dinoflagellates on the cyanobacterium Synechococcus. Aquat. Microb. Ecol. 41, 131–143. doi: 10.3354/ame041131
Jeong, H. J., Yoo, Y. D., Park, J. Y., Song, J. Y., Kim, S. T., Lee, S. H., et al. (2005b). Feeding by phototrophic red-tide dinoflagellates: five species newly revealed and six species previously known to be mixotrophic. Aquat. Microb. Ecol. 40, 133–150. doi: 10.3354/ame040133
Jeong, H. J., Seong, K. A., Du Yoo, Y., Kim, T. H., Kang, N. S., Kim, S., et al. (2008). Feeding and grazing impact by small marine heterotrophic dinoflagellates on heterotrophic bacteria. J. Eukaryot. Microbiol. 55, 271–288. doi: 10.1111/j.1550-7408.2008.00336.x
Jeong, H. J., Shim, J. H., Kim, J. S., Park, J. Y., Lee, C. W., and Lee, Y. (1999). Feeding by the mixotrophic thecate dinoflagellate Fragilidium cf. mexicanum on red-tide and toxic dinoflagellates. Mar. Ecol. Prog. Ser. 176, 263–277. doi: 10.3354/meps176263
Jeong, H. J., Yoo, Y. D., Kang, N. S., Lim, A. S., Seong, K. A., Lee, S. Y., et al. (2012). Heterotrophic feeding as a newly identified survival strategy of the dinoflagellate Symbiodinium. Proc. Natl. Acad. Sci. U.S.A. 109, 12604–12609. doi: 10.1073/pnas.1204302109
Kamp, A., de Beer, D., Nitsch, J. L., Lavik, G., and Stief, P. (2011). Diatoms respire nitrate to survive dark and anoxic conditions. Proc. Natl. Acad. Sci. U.S.A. 108, 5649–5654. doi: 10.1073/pnas.1015744108
Kim, S., Kang, Y., Kim, H., Yih, W., Coats, D., and Park, M. (2008). Growth and grazing responses of the mixotrophic dinoflagellate Dinophysis acuminata as functions of light intensity and prey concentration. Aquat. Microb. Ecol. 51, 301–310. doi: 10.3354/ame01203
Kopp, C., Pernice, M., Domart-Coulon, I., Djediat, C., Spangenberg, J. E., Alexander, D. T. L., et al. (2013). Highly dynamic cellular-level response of symbiotic coral to a sudden increase in environmental nitrogen. MBio 4, e00052. doi: 10.1128/mBio.00052-13
Kroth, P. G., Chiovitti, A., Gruber, A., Martin-Jezequel, V., Mock, T., Parker, M. S., et al. (2008). A model for carbohydrate metabolism in the diatom Phaeodactylum tricornutum deduced from comparative whole genome analysis. PLoS ONE 3:e1426. doi: 10.1371/journal.pone.0001426
Kudela, R. M., and Cochlan, W. P. (2000). Nitrogen and carbon uptake kinetics and the influence of irradiance for a red tide bloom off southern California. Aquat. Microb. Ecol. 21, 31–47. doi: 10.3354/ame021031
Lee, Y. S. (2006). Factors affecting outbreaks of high-density Cochlodinium polykrikoides red tides in the coastal seawaters around Yeosu and Tongyeong, Korea. Mar. Pollut. Bull. 52, 1249–1259. doi: 10.1016/j.marpolbul.2006.02.024
Lee, Y. S. (2008). Utilization of various nitrogen, phosphorus, and selenium compounds by Cochlodinium polykrikoides. J. Environ. Biol. 29, 799–804.
Leggat, W., Hoegh-Guldberg, O., Dove, S., and Yellowlees, D. (2007). Analysis of an EST library from the dinoflagellate (Symbiodinium sp.) symbiont of reef-building corals1. J. Phycol. 43, 1010–1021. doi: 10.1111/j.1529-8817.2007.00387.x
Lei, Q. Y., and Lu, S. H. (2011). Molecular ecological responses of dinoflagellate, Karenia mikimotoi to environmental nitrate stress. Mar. Pollut. Bull. 62, 2692–2699. doi: 10.1016/j.marpolbul.2011.09.021
Lema, K. A., Willis, B. L., and Bourne, D. G. (2012). Corals form characteristic associations with symbiotic nitrogen-fixing bacteria. Appl. Environ. Microbiol. 78, 3136–3144. doi: 10.1128/AEM.07800-11
Leong, S. C., Murata, A., Nagashima, Y., and Taguchi, S. (2004). Variability in toxicity of the dinoflagellate Alexandrium tamarense in response to different nitrogen sources and concentrations. Toxicon 43, 407–415. doi: 10.1016/j.toxicon.2004.01.015
Leong, S. C., and Taguchi, S. (2004). Response of the dinoflagellate Alexandrium tamarense to a range of nitrogen sources and concentrations: growth rate, chemical carbon and nitrogen, and pigments. Hydrobiologia 515, 215–224. doi: 10.1023/B:HYDR.0000027331.49819.a4
Leong, S. C. Y., Maekawa, M., and Taguchi, S. (2010). Carbon and nitrogen acquisition by the toxic dinoflagellate Alexandrium tamarense in response to different nitrogen sources and supply modes. Harmful Algae 9, 48–58. doi: 10.1016/j.hal.2009.07.003
Lesser, M. P., Falcon, L. I., Rodriguez-Roman, A., Enriquez, S., Hoegh-Guldberg, O., and Iglesias-Prieto, R. (2007). Nitrogen fixation by symbiotic cyanobacteria provides a source of nitrogen for the scleractinian coral Montastraea cavernosa. Mar. Ecol. Prog. Ser. 346, 143–152. doi: 10.3354/meps07008
Lesser, M. P., Mazel, C. H., Gorbunov, M. Y., and Falkowski, P. G. (2004). Discovery of symbiotic nitrogen-fixing cyanobacteria in corals. Science 305, 997–1000. doi: 10.1126/science.1099128
Lewitus, A. J., Willis, B. M., Hayes, K. C., Burkholder, J. M., Glasgow, H. B. Jr., Glibert, P. M., et al. (1999). Mixotrophy and nitrogen uptake by Pfiesteria piscicida (Dinophyceae) J. Phycol. 35, 1430–1437. doi: 10.1046/j.1529-8817.1999.3561430.x
Li, A., Stoecker, D. K., and Coats, D. W. (2000). Mixotrophy in Gyrodinium galatheanum (Dinophyceae): grazing responses to light intensity and inorganic nutrients. J. Phycol. 36, 33–45. doi: 10.1046/j.1529-8817.2000.98076.x
Lillo, C. (2008). Signalling cascades integrating light-enhanced nitrate metabolism. Biochem. J. 415, 11–19. doi: 10.1042/BJ20081115
Lin, S., Mulholland, M. R., Zhang, H., Feinstein, T. N., Jochem, F. J., and Carpenter, E. J. (2004). Intense grazing and prey-dependent growth of Pfiesteria piscicida (Dinophyceae). J. Phycol. 40, 1062–1073. doi: 10.1111/j.1529-8817.2004.03217.x
Liu, K.-H., and Tsay, Y.-F. (2003). Switching between the two action modes of the dual-affinity nitrate transporter CHL1 by phosphorylation. EMBO J. 22, 1005–1013. doi: 10.1093/emboj/cdg118
Liu, L.-H., Ludewig, U., Frommer, W. B., and von Wiren, N. (2003a). AtDUR3 encodes a new type of high-affinity urea/H+ symporter in Arabidopsis. Plant Cell 15, 790–800. doi: 10.1105/tpc.007120
Liu, L. H., Ludewig, U., Gassert, B., Frommer, W. B., and von Wiren, N. (2003b). Urea transport by nitrogen-regulated tonoplast intrinsic proteins in Arabidopsis. Plant Physiol. 133, 1220–1228. doi: 10.1104/pp.103.027409
Lommer, M., Specht, M., Roy, A. S., Kraemer, L., Andreson, R., Gutowska, M. A., et al. (2012). Genome and low-iron response of an oceanic diatom adapted to chronic iron limitation. Genome Biol. 13, R66. doi: 10.1186/gb-2012-13-7-r66
Loque, D., Ludewig, U., Yuan, L., and von Wiren, N. (2005). Tonoplast intrinsic proteins AtTIP2;1 and AtTIP2;3 facilitate NH3 transport into the vacuole. Plant Physiol. 137, 671–680. doi: 10.1104/pp.104.051268
Loureiro, S., Garces, E., Collos, Y., Vaque, D., and Camp, J. (2009). Effect of marine autotrophic dissolved organic matter (DOM) on Alexandrium catenella in semi-continuous cultures. J. Plankton Res. 31, 1363–1372. doi: 10.1093/plankt/fbp080
Maguer, J.-F., L’Helguen, S., Madec, C., Labry, C., and Le Corre, P. (2007). Nitrogen uptake and assimilation kinetics in Alexandrium minutum (Dynophyceae): effect of N-limited growth rate on nitrate and ammonium interactions. J. Phycol. 43, 295–303. doi: 10.1111/j.1529-8817.2007.00334.x
Maheswari, U., Jabbari, K., Petit, J.-L., Porcel, B., Allen, A., Cadoret, J.-P., et al. (2010). Digital expression profiling of novel diatom transcripts provides insight into their biological functions. Genome Biol. 11, R85. doi: 10.1186/gb-2010-11-8-r85
Menden-Deuer, S., Lessard, E. J., Satterberg, J., and Grunbaum, D. (2005). Growth rates and starvation survival of three species of the pallium-feeding, thecate dinoflagellate genus Protoperidinium. Aquat. Microb. Ecol. 41, 145–152. doi: 10.3354/ame041145
Miller, T. R., and Belas, R. (2003). Pfiesteria piscicida, P. shumwayae, and other Pfiesteria-like dinoflagellates. Res. Microbiol. 154, 85–90. doi: 10.1016/S0923-2508(03)00027-5
Moreno-Vivian, C., Cabello, P., Martinez-Luque, M., Blasco, R., and Castillo, F. (1999). Prokaryotic nitrate reduction: molecular properties and functional distinction among bacterial nitrate reductases. J. Bacteriol. 181, 6573–6584.
Morey, J. S., Monroe, E. A., Kinney, A. L., Beal, M., Johnson, J. G., Hitchcock, G. L., et al. (2011). Transcriptomic response of the red tide dinoflagellate, Karenia brevis, to nitrogen and phosphorus depletion and addition. BMC Genomics 12:346. doi: 10.1186/1471-2164-12-346
Moustafa, A., Evans, A. N., Kulis, D. M., Hackett, J. D., Erdner, D. L., Anderson, D. M., et al. (2010). Transcriptome profiling of a toxic dinoflagellate reveals a gene-rich protist and a potential impact on gene expression due to bacterial presence. PLoS ONE 5:e9688. doi: 10.1371/journal.pone.0009688
Nakamura, Y., Suzuki, S., and Hiromi, J. (1995). Population dynamics of heterophytic dinoflagellates during a Gymnodinium mikimotoi red tide in the Seto Inland Sea. Mar. Ecol. Prog. Ser. 125, 269–277. doi: 10.3354/meps125269
Ninnemann, O., Jauniaux, J., and Frommer, W. (1994). Identification of a high affinity NH4+ transporter from plants. EMBO J. 13, 3464–3471
Nishitani, G., Nagai, S., Takano, Y., Sakiyama, S., Baba, K., and Kamiyama, T. (2008a). Growth characteristics and phylogenetic analysis of the marine dinoflagellate Dinophysis infundibulus (Dinophyceae). Aquat. Microb. Ecol. 52, 209–221. doi: 10.3354/ame01233
Nishitani, G. O. H., Nagai, S., Sakiyama, S., and Kamiyama, T. (2008b). Successful cultivation of the toxic dinoflagellate Dinophysis caudata (Dinophyceae). Plankton Benthos Res. 3, 78–85. doi: 10.3800/pbr.3.78
Olson, N. D., Ainsworth, T. D., Gates, R. D., and Takabayashi, M. (2009). Diazotrophic bacteria associated with Hawaiian Montipora corals: diversity and abundance in correlation with symbiotic dinoflagellates. J. Exp. Mar. Biol. Ecol. 371, 140–146. doi: 10.1016/j.jembe.2009.01.012
Olson, R. J., and Chisholm, S. W. (1986). Effect of light and nitrogen limitation on the cell cycle of the dinoflagellate Amphidinium carteri. J. Plankton Res. 8, 785–793 doi: 10.1093/plankt/8.4.785
Paasche, E., Bryceson, I., and Tangen, K. (1984). Interspecific variation in dark nitrogen uptake by dinoflagellates. J. Phycol. 20, 394–401. doi: 10.1111/j.0022-3646.1984.00394.x
Park, M., Kim, S., Kang, Y., and Yih, W. (2006). First successful culture of the marine dinoflagellate Dinophysis acuminata. Aquat. Microb. Ecol. 45, 101–106. doi: 10.3354/ame045101
Park, M. G., Cooney, S. K., Yih, W., and Coats, D. W. (2002). Effects of two strains of the parasitic dinoflagellate Amoebophrya on growth, photosynthesis, light absorption, and quantum yield of bloom-forming dinoflagellates. Mar. Ecol. Prog. Ser. 227, 281–292. doi: 10.3354/meps227281
Parrow, M. W., and Burkholder, J. M. (2004). The sexual life cycles of Pfiesteria piscicida and cryptoperidiniopsoids (Dinophyceae). J. Phycol. 40, 664–673. doi: 10.1111/j.1529-8817.2004.03202.x
Pernice, M., Meibom, A., Van Den Heuvel, A., Kopp, C., Domart-Coulon, I., Hoegh-Guldberg, O., et al. (2012). A single-cell view of ammonium assimilation in coral-dinoflagellate symbiosis. ISME J. 6, 1314–1324. doi: 10.1038/ismej.2011.196
Pilgrim, M. L., Caspar, T., Quail, P. H., and McClung, C. R. (1993). Circadian and light-regulated expression of nitrate reductase in Arabidopsis. Plant Mol. Biol. 23, 349–364. doi: 10.1007/BF00029010
Pleissner, D., and Eriksen, N. T. (2012). Effects of phosphorous, nitrogen, and carbon limitation on biomass composition in batch and continuous flow cultures of the heterotrophic dinoflagellate Crypthecodinium cohnii. Biotechnol. Bioeng. 109, 2005–2016. doi: 10.1002/bit.24470
Poulsen, N., and Kröger, N. (2005). A new molecular tool for transgenic diatoms. FEBS J. 272, 3413–3423. doi: 10.1111/j.1742-4658.2005.04760.x
Preisler, A., de Beer, D., Lichtschlag, A., Lavik, G., Boetius, A., and Jorgensen, B. B. (2007). Biological and chemical sulfide oxidation in a Beggiatoa inhabited marine sediment. ISME J. 1, 341–353. doi:10.1038/ismej.2007.50
Qiu, D., Huang, L., Liu, S., and Lin, S. (2011). Nuclear, mitochondrial and plastid gene phylogenies of Dinophysis miles (Dinophyceae): evidence of variable types of chloroplasts. PLoS ONE 6:e29398. doi: 10.1371/journal.pone.0029398
Rahav, O., Dubinsky, Z., Achituv, Y., and Falkowski, P. G. (1989). Ammonium metabolism in the Zooxanthellate Coral, Stylophora pistillata. Proc. R. Soc. Lond. 236, 325–337. doi: 10.1098/rspb.1989.0026
Ramalho, C. B., Hastings, J. W., and Colepicolo, P. (1995). Circadian oscillation of nitrate reductase activity in Gonyaulax polyedra is due to changes in cellular protein levels. Plant Physiol. 107, 225–231. doi: 10.1104/pp.107.1.225
Raven, J. A., and Smith, F. A. (1980). Intracellular pH regulation in the giant-celled marine alga Chaetomorpha darwinii. J. Exp. Bot. 31, 1357–1369. doi: 10.1093/jxb/31.5.1357
Reid, R. J., Mimura, T., Ohsumi, Y., Walker, N. A., and Smith, F. A. (2000). Phosphate uptake in Chara: membrane transport via Na/Pi cotransport. Plant Cell Environ. 23, 223–228. doi: 10.1046/j.1365-3040.2000.00524.x
Rentsch, D., Schmidt, S., and Tegeder, M. (2007). Transporters for uptake and allocation of organic nitrogen compounds in plants. FEBS Lett. 581, 2281–2289. doi: 10.1016/j.febslet.2007.04.013
Risgaard-Petersen, N., Langezaal, A. M., Ingvardsen, S., Schmid, M. C., Jetten, M. S., Op den Camp, H. J., et al. (2006). Evidence for complete denitrification in a benthic foraminifer. Nature 443, 93–96. doi: 10.1038/nature05070
Roenneberg, T., and Rehman, J. (1996). Nitrate, a nonphotic signal for the circadian system. FASEB J. 10, 1443–1447.
Rosenberg, E., Koren, O., Reshef, L., Efrony, R., and Zilber-Rosenberg, I. (2007). The role of microorganisms in coral health, disease and evolution. Nat. Rev. Microbiol. 5, 355–362. doi: 10.1038/nrmicro1635
Sanders, D., Smith, F. A., and Walker, N. A. (1985). Proton/chloride cotransport in Chara: mechanism of enhanced influx after rapid external acidification. Planta 163, 411–418. doi: 10.1007/BF00395151
San Diego-McGlone, M. L., Azanza, R. V., Villanoy, C. L., and Jacinto, G. S. (2008). Eutrophic waters, algal bloom and fish kill in fish farming areas in Bolinao, Pangasinan, Philippines. Mar. Pollut. Bull. 57, 295–301. doi: 10.1016/j.marpolbul.2008.03.028
Seong, K. A., Jeong, H. J., Kim, S., Kim, G. H., and Kang, J. H. (2006). Bacterivory by co-occurring red-tide algae, heterotrophic nanoflagellates, and ciliates. Mar. Ecol. Prog. Ser. 322, 85–97. doi: 10.3354/meps322085
Shoguchi, E., Shinzato, C., Kawashima, T., Gyoja, F., Mungpakdee, S., Koyanagi, R., et al. (2013). Draft assembly of the Symbiodinium minutum nuclear genome reveals dinoflagellate gene structure. Curr. Biol. 23, 1399–1408. doi: 10.1016/j.cub.2013.05.062
Shoun, H., and Tanimoto, T. (1991). Denitrification by the fungus Fusarium oxysporum and involvement of cytochrome P-450 in the respiratory nitrite reduction. J. Biol. Chem. 266, 11078–11082.
Siddiqi, M. Y., Glass, A. D., Ruth, T. J., and Rufty, T. W. (1990). Studies of the uptake of nitrate in barley: I. kinetics of NO(3) influx. Plant Physiol. 93, 1426–1432. doi: 10.1104/pp.93.4.1426
Skovgaard, A., Massana, R., Balague, V., and Saiz, E. (2005). Phylogenetic position of the copepod-infesting parasite Syndinium turbo (Dinoflagellata, Syndinea). Protist 156, 413–423. doi: 10.1016/j.protis.2005.08.002
Small, H. J., Shields, J. D., Reece, K. S., Bateman, K., and Stentiford, G. D. (2012). Morphological and molecular characterization of Hematodinium perezi (Dinophyceae: Syndiniales), a dinoflagellate parasite of the harbour crab, Liocarcinus depurator. J. Eukaryot. Microbiol. 59, 54–66. doi: 10.1111/j.1550-7408.2011.00592.x
Smalley, G. W., and Coats, D. W. (2002). Ecology of the red-tide dinoflagellate Ceratium furca: distribution, mixotrophy, and grazing impact on ciliate populations of Chesapeake Bay. J. Eukaryot. Microbiol. 49, 63–73. doi: 10.1111/j.1550-7408.2002.tb00343.x
Smalley, G. W., Coats, D. W., and Stoecker, D. K. (2003). Feeding in the mixotrophic dinoflagellate Ceratium furca is influenced by intracellular nutrient concentrations. Mar. Ecol. Prog. Ser. 262, 137–151. doi: 10.3354/meps262137
Smayda, T. (1997). Harmful algal blooms: their ecophysiology and general relevance to phytoplankton blooms in the sea. Limnol. Oceanogr. 42, 1137–1153. doi: 10.4319/lo.1997.42.5_part_2.1137
Steidinger, K. A., Burkholder, J. M., Glasgow, H. B., Hobbs, C. W., Garrett, J. K., Truby, E. W., et al. (1996). Pfiesteria piscicida gen. et sp. nov. (Pfiesteriaceae fam. nov.) a new toxic dinoflagellate with a complex life cycle and behavior. J. Phycol. 32, 157–164. doi: 10.1111/j.0022-3646.1996.00157.x
Stoecker, D., Johnson, M., deVargas, C., and Not, F. (2009). Acquired phototrophy in aquatic protists. Aquat. Microb. Ecol. 57, 279–310. doi: 10.3354/ame01340
Stoecker, D., Li, A., Coats, D., Gustafson, D., and Nannen, M. (1997). Mixotrophy in the dinoflagellate Prorocentrum minimum. Mar. Ecol. Prog. Ser. 152, 1–12. doi: 10.3354/meps152001
Stoecker, D. K. (1998). Conceptual models of mixotrophy in planktonic protists and some ecological and evolutionary implications. Eur. J. Protistol. 34, 281–290. doi: 10.1016/S0932-4739(98)80055-2
Stoecker, D. K. (1999). Mixotrophy among dinoflagellates. J. Eukaryot. Microbiol. 46, 397–401. doi: 10.1111/j.1550-7408.1999.tb04619.x
Strom, S. L., and Buskey, E. J. (1993). Feeding, growth, and behavior of the thecate heterotrophic dinoflagellate Oblea rotunda. Limnol. Oceanogr. 38, 965–977. doi: 10.4319/lo.1993.38.5.0965
Sugiura, M., Georgescu, M. N., and Takahashi, M. (2007). A nitrite transporter associated with nitrite uptake by higher plant chloroplasts. Plant Cell Physiol. 48, 1022–1035. doi: 10.1093/pcp/pcm073
Summons, R. E., and Osmond, C. B. (1981). Nitrogen assimilation in the symbiotic marine alga Gymnodinium microadriaticum: direct analysis of 15N incorporation by gc-ms methods. Phytochemistry 20, 575–578. doi: 10.1016/0031-9422(81)85136-9
Tarangkoon, W., Hansen, G., and Hansen, P. (2010). Spatial distribution of symbiont-bearing dinoflagellates in the Indian Ocean in relation to oceanographic regimes. Aquat. Microb. Ecol. 58, 197–213. doi: 10.3354/ame01356
Tillmann, U. (2004). Interactions between planktonic microalgae and protozoan grazers. J. Eukaryot. Microbiol. 51, 156–168. doi: 10.1111/j.1550-7408.2004.tb00540.x
Ullrich, W. R., and Glaser, E. (1982). Sodium-phosphate cotransport in the green alga Ankistrodesmus braunii. Plant Sci. Lett. 27, 155–161. doi: 10.1016/0304-4211(82)90144-4
Usuda, K., Toritsuka, N., Matsuo, Y., Kim, D. H., and Shoun, H. (1995). Denitrification by the fungus Cylindrocarpon tonkinense: anaerobic cell growth and two isozyme forms of cytochrome P-450nor. Appl. Environ. Microbiol. 61, 883–889.
Vanucci, S., Pezzolesi, L., Pistocchi, R., Ciminiello, P., Dell’Aversano, C., Dello Iacovo, E., et al. (2012). Nitrogen and phosphorus limitation effects on cell growth, biovolume, and toxin production in Ostreopsis cf. ovata. Harmful Algae 15, 78–90. doi: 10.1016/j.hal.2011.12.003
Venrick, E. L. (1974). The distribution and significance of Richelia intracellularis Schmidt in the North Pacific central gyre. Limnol. Oceanogr. 19, 437–444. doi: 10.4319/lo.1974.19.3.0437
Vila, M., Garces, E., and Maso, M. (2001). Potentially toxic epiphytic dinoflagellate assemblages on macroalgae in the NW Mediterranean. Aquat. Microb. Ecol. 26, 51–60. doi: 10.3354/ame026051
Walker, N. A., Reid, R. J., and Smith, F. A. (1993). The uptake and metabolism of urea by Chara australis: IV. symport with sodium – a slip model for the high and low affinity systems. J. Membr. Biol. 136, 263–271. doi: 10.1007/BF00233665
Wang, D. Z. (2008). Neurotoxins from marine dinoflagellates: a brief review. Mar. Drugs 6, 349–371. doi: 10.3390/md6020349
Wang, D. Z., and Hsieh, D. (2002). Effects of nitrate and phosphate on growth and C2 toxin productivity of Alexandrium tamarense CI01 in culture. Mar. Pollut. Bull. 45, 286–289. doi: 10.1016/S0025-326X(02)00183-2
Wang, W.-H., Kohler, B., Cao, F.-Q., and Liu, L.-H. (2008). Molecular and physiological aspects of urea transport in higher plants. Plant Sci. 175, 467–477. doi: 10.1016/j.plantsci.2008.05.018
Wang, Z. T., Ullrich, N., Joo, S., Waffenschmidt, S., and Goodenough, U. (2009). Algal lipid bodies: stress induction, purification, and biochemical characterization in wild-type and starchless Chlamydomonas reinhardtii. Eukaryot. Cell 8, 1856–1868. doi: 10.1128/EC.00272-09
Wilson, M. R., and Walker, N. A. (1988). The transport and metabolism of urea in chara australis: I. passive diffusion, specific transport and metabolism of urea, thiourea and methylurea. J. Exp. Bot. 39, 739–751. doi: 10.1093/jxb/39.6.739
Wingler, A., Purdy, S., MacLean, J. A., and Pourtau, N. (2006). The role of sugars in integrating environmental signals during the regulation of leaf senescence. J. Exp. Bot. 57, 391–399. doi: 10.1093/jxb/eri279
Yang, Z. K., Niu, Y. F., Ma, Y. H., Xue, J., Zhang, M. H., Yang, W. D., et al. (2013). Molecular and cellular mechanisms of neutral lipid accumulation in diatom following nitrogen deprivation. Biotechnol. Biofuels 6, 67. doi: 10.1186/1754-6834-6-67
Keywords: dinoflagellates, diatoms, nitrogen metabolism, nitrogen stress, autotrophy, mixotrophy, heterotrophy
Citation: Dagenais-Bellefeuille S and Morse D (2013) Putting the N in dinoflagellates. Front. Microbiol. 4:369. doi: 10.3389/fmicb.2013.00369
Received: 16 July 2013; Accepted: 19 November 2013;
Published online: 04 December 2013.
Edited by:
Senjie Lin, University of Connecticut, USAReviewed by:
Kathleen Scott, University of South Florida, USABethany Jenkins, University of Rhode Island, USA
Senjie Lin, University of Connecticut, USA
Copyright © 2013 Dagenais-Bellefeuille and Morse. This is an open-access article distributed under the terms of the Creative Commons Attribution License (CC BY). The use, distribution or reproduction in other forums is permitted, provided the original author(s) or licensor are credited and that the original publication in this journal is cited, in accordance with accepted academic practice. No use, distribution or reproduction is permitted which does not comply with these terms.
*Correspondence: David Morse, Département de Sciences Biologiques, Institut de Recherche en Biologie Végétale, Université de Montréal, 4101 Sherbrooke est, Montréal, QC H1X 2B2, Canada e-mail:ZGF2aWQubW9yc2VAdW1vbnRyZWFsLmNh