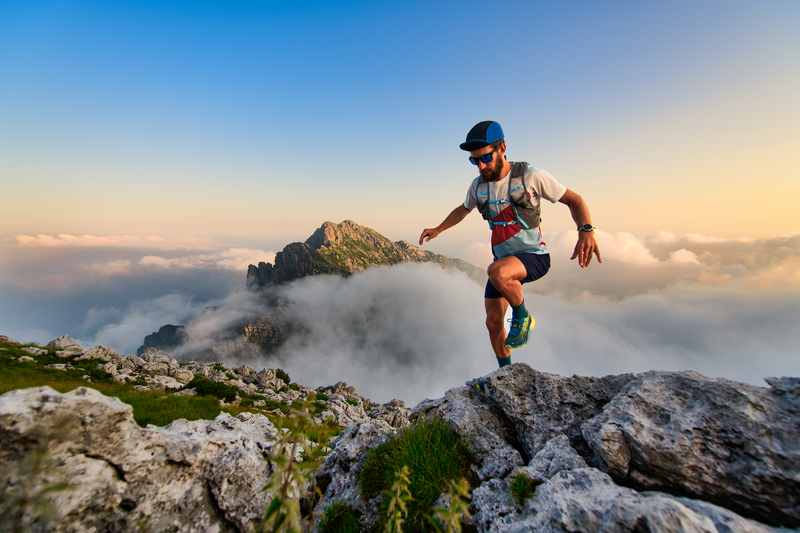
95% of researchers rate our articles as excellent or good
Learn more about the work of our research integrity team to safeguard the quality of each article we publish.
Find out more
ORIGINAL RESEARCH article
Front. Microbiol. , 01 May 2012
Sec. Evolutionary and Genomic Microbiology
Volume 3 - 2012 | https://doi.org/10.3389/fmicb.2012.00163
This article is part of the Research Topic Recent advances in genomic and genetic studies in the Archaea View all 12 articles
Pyrococcus furiosus grows optimally near 100°C by fermenting carbohydrates to produce hydrogen (H2) or, if elemental sulfur (S0) is present, hydrogen sulfide instead. It contains two cytoplasmic hydrogenases, SHI and SHII, that use NADP(H) as an electron carrier and a membrane-bound hydrogenase (MBH) that utilizes the redox protein ferredoxin. We previously constructed deletion strains lacking SHI and/or SHII and showed that they exhibited no obvious phenotype. This study has now been extended to include biochemical analyses and growth studies using the ΔSHI and ΔSHII deletion strains together with strains lacking a functional MBH (ΔmbhL). Hydrogenase activity in cytoplasmic extracts of various strains demonstrate that SHI is responsible for most of the cytoplasmic hydrogenase activity. The ΔmbhL strain showed no growth in the absence of S0, confirming the hypothesis that, in the absence of S0, MBH is the only enzyme that can dispose of reductant (in the form of H2) generated during sugar oxidation. Under conditions of limiting sulfur, a small but significant amount of H2 was produced by the ΔmbhL strain, showing that SHI can produce H2 from NADPH in vivo, although this does not enable growth of ΔmbhL in the absence of S0. We propose that the physiological function of SHI is to recycle H2 and provide a link between external H2 and the intracellular pool of NADPH needed for biosynthesis. This likely has a distinct energetic advantage in the environment, but it is clearly not required for growth of the organism under the usual laboratory conditions. The function of SHII, however, remains unknown.
Hydrogen gas (H2) plays an important role in anaerobic metabolism as the majority of anaerobes contain the enzyme hydrogenase responsible for the reversible interconversion of molecular hydrogen, protons, and electrons. Hydrogenases can be grouped into three classes based on the metal composition of their active site: [NiFe]-hydrogenases, [FeFe]-hydrogenases, and the more recently defined [Fe]-hydrogenases, so far restricted to certain methanogenic organisms (Vignais et al., 2001; Shima and Thauer, 2007). Organisms in the bacterial domain contain both [NiFe]- and [FeFe]-hydrogenases, while archaeal organisms are known to utilize [NiFe]- and [Fe]-hydrogenases (Vignais and Billoud, 2007). Almost all of the anaerobic archaea contain one or more [NiFe]-hydrogenases, implying that H2 metabolism plays an important role in the extreme environments in which many of these organisms are found (Vignais and Billoud, 2007).
Pyrococcus furiosus is a well-studied hyperthermophile belonging to the order Thermococcales. It grows optimally at 100°C by peptide and carbohydrate fermentation with H2, organic acids, and CO2 being the main fermentation products (Fiala and Stetter, 1986; Kengen and Stams, 1994; Driskill et al., 1999; Adams et al., 2001). P. furiosus degrades glucose via a modified Embden–Meyerhof glycolytic pathway that utilizes the low potential electron carrier protein ferredoxin (Fd) in place of NAD for all oxidative steps, resulting in no overall substrate level ATP yield (Kengen et al., 1994; de Vos et al., 1998; Verhees et al., 2004). Because of the low redox potential of Fd (−480 mV), H2 production (−420 mV) with Fd is thermodynamically favorable (Park et al., 1991; Smith et al., 1995; Hagedoorn et al., 1998). Therefore, all reducing equivalents generated in sugar metabolism can be disposed of as H2, with production of up to 4 mol of H2 per mol glucose oxidized (Verhees et al., 2004). The enzyme responsible for H2 formation is a unique membrane-bound hydrogenase (MBH) complex, which uses the energy from this exergonic reaction to create an ion gradient across the membrane that drives ATP synthesis, resulting in the generation of an estimated 0.3 mol of ATP per mol H2 (Sapra et al., 2003). This system is one of the simplest forms of respiration. Besides MBH, P. furiosus also contains two cytoplasmic hydrogenases (SHI and SHII), and these homologous enzymes each consist of four subunits. Based on kinetic studies, SHI and SHII are proposed to be involved in H2 recycling to provide NADPH for biosynthesis (Ma and Adams, 2001b; van Haaster et al., 2008).
Pyrococcus furiosus can utilize carbohydrates for growth in either the presence or absence of elemental sulfur (S0; Fiala and Stetter, 1986; Adams et al., 2001). In the presence of S0, P. furiosus undergoes a major metabolic shift to utilize S0 as an electron acceptor, thereby switching production from H2 to H2S. When S0 becomes available, there is a dramatic decrease in the expression of all three hydrogenase operons correlated with a decrease in hydrogenase activity, and concomitantly, there is a large increase in expression of genes related to S0 reduction, such as those encoding the membrane-bound oxidoreductase (MBX) as well as the NAD(P)H-linked sulfur reductase (NSR; Adams et al., 2001; Schut et al., 2007). The response to S0 is mediated at least in part by the redox switch containing transcriptional regulator SurR (Lipscomb et al., 2009; Yang et al., 2010). MBX is highly homologous to MBH and is thought to function analogously to MBH by using Fd as an electron acceptor and conserving energy via formation of an ion gradient (Silva et al., 2000; Schut et al., 2007; Bridger et al., 2011). NSR has been proposed to reduce S0 synergistically with MBX in which MBX can provide NADPH using Fd as electron donor (Schut et al., 2007; Bridger et al., 2011).
In a previous study it was shown that strains containing deletions of either SHI or SHII or both have no growth phenotype under the conditions tested, suggesting that other enzyme(s), independent of H2, can guide electrons from carbohydrate oxidation to feed the NADPH pool (Lipscomb et al., 2011). This study has now been extended to include strains containing a deletion of the active subunit of MBH (mbhL) either alone or in combination with deletions of the genes encoding both soluble hydrogenases. Herein, we address the physiological function of all three hydrogenases in P. furiosus both in the absence and presence of sufficient and limiting concentrations of S0.
The P. furiosus strains used or constructed in the study are listed in Table 1. Growth medium was prepared as previously described (Adams et al., 2001), and contained maltose (5 g/L) as the primary carbon source, supplemented with 0.5 g/L yeast extract, with or without addition of S0 at 0.5 or 2 g/L. Growth experiments were carried out in biological duplicates in 150-mL serum bottles with 75 mL medium, with incubation at 95°C and shaking at 150 rpm. Cultures for cell-free extracts were grown in a 20-L custom fermenter, and cytoplasmic fractions were prepared by ultra-centrifugation as described previously (Adams et al., 2001).
Gene splicing by overlap extension and PCR (SOE–PCR; Horton et al., 1989) was used to construct a PCR product containing 0.5 kb regions upstream and downstream of mbhL on either side of a genetic marker. The destination strains (COM1 and ΔSHIΔSHII) contain a deletion of the pyrF gene; therefore, a cassette containing pyrF expressed by the PEP synthase promoter (123 bp) was used as the marker. The 500-bp flanking regions were amplified from wild-type gDNA using the two primer sets WN011 (GTCATAAAACTAAATGATGAGCATTTGACTTCATTTCTTCTCCCTC), WN013 (TTGGAGAAGAGAATTGCCCAAC), and WN012 (AGAATGGAGCTCAAGATAAATATGAAAATTGTATATGGAGTTATTGG), WN014 (AGACATCAACACACTGCTTACAC). The deletion construct for mbhL was transformed into P. furiosus COM1 and ΔSHIΔSHII selecting uracil prototrophy on solid defined medium as previously described (Lipscomb et al., 2011). To obtain mbhL deletions, solid medium was supplemented with 8 mM polysulfide (equivalent to approximately 1 g/L S0) or 2 mM polysulfide with solid S0 powder sprinkled on the surface of the plate. The pyrF gene deleted in the COM1, ΔSHI, ΔSHII, and ΔSHIΔSHII strains was restored to the wild-type by transformation with a PCR product of the wild-type pyrF locus containing ∼0.5 kb on either side of the gene. This was done to ensure all strains were uracil prototrophic so growth could be achieved in identical media. DNA was extracted from transformants as previously described (Lipscomb et al., 2011) and screened for deletion by PCR amplification of the locus using primers outside the homologous flanking regions used to construct the deletions. Transformants were further colony purified by serial passage on solid medium. Mutants were confirmed by sequence analysis of the mbhL region and qPCR analyses (see below).
Total RNA from various P. furiosus strains was obtained, and cDNA was synthesized as previously described (Lipscomb et al., 2011). Quantitative PCR was carried out using an Mx3000P instrument (Agilent) and the Brilliant SYBR green qPCR master mix (Agilent) with primers specific to the genes encoding SHI and SHII beta subunits (PF0891 and PF1329), mbhK (PF1433), mbhL (PF1434), mbhM (PF1435), and pyrF (PF1114), along with the constitutively expressed genes encoding the pyruvate ferredoxin oxidoreductase (POR) gamma subunit (PF0971) and DNA Polymerase (PF0983) as internal controls. Successful gene deletions were verified by the absence of a specific qPCR signal.
To monitor cell growth, the Bradford method (Bradford, 1976) was used to estimate total cell protein concentration from 1 mL culture samples, with bovine serum albumin as the standard. For H2S and H2 analyses, headspace, and medium samples (500 μL each) were taken at 6 and 9 h during growth and transferred anaerobically into the double-vial system as previously reported (Schut et al., 2007). H2S production was assayed by the methylene blue method (Chen and Mortenson, 1977), and abiotic sulfide production was subtracted from the experimental samples using control bottles containing uninoculated medium. H2 production was measured using a gas chromatograph (GC-8A, Shimadzu, Columbia, MD, USA). Hydrogenase activity in cell-free extracts was determined by H2 production using sodium dithionite (5 mM) as the electron donor with methyl viologen (1 mM) as the electron carrier as described previously (Ma and Adams, 2001b).
We showed previously that strains containing deletions of either of the two cytosolic NADP-linked hydrogenases (SHI and SHII) alone or together did not produce any growth phenotype under the conditions tested (Lipscomb et al., 2011). In order to verify the effect of disruption of SHI and SHII on hydrogenase activity in the cytoplasm, we prepared cell-free extracts from ΔSHI, ΔSHII and ΔSHIΔSHII. The amount of hydrogenase activity (using the artificial electron carrier methyl viologen) in cytoplasmic fractions was not significantly affected in the ΔSHII strain but was much lower in the ΔSHI strain (<10% of that produced in the parental strains), while in the ΔSHIΔSHII strain, no hydrogenase activity could be detected (Figure 1). These data indicate that SHI is responsible for the majority of hydrogenase activity in the cytoplasm and confirms that the activity of the MBH is strictly associated with the membrane (Sapra et al., 2000; Silva et al., 2000).
Figure 1. Hydrogenase activity (using methyl viologen as electron carrier) in cytoplasmic fractions obtained from P. furiosus cultures of hydrogenase disruption mutants and parental strains. See Table 1 for strain definitions.
We have constructed strains containing a deletion of the catalytic subunit (mbhL) of the membrane-bound ferredoxin-linked H2-producing hydrogenase (MBH), either alone or in combination with deletions of SHI and SHII. Growth of these strains was compared on maltose-based medium containing minimal yeast extract with either no S0, limiting S0 (0.5 g/L), or sufficient S0 (2 g/L). Both mutants containing a deletion of mbhL displayed no detectable growth in the absence of S0, but had no growth defect in the presence of sufficient S0 (Figure 2). In the presence of limiting S0 (0.5 g/L), the MBH disruption strains exhibited ∼40% less final protein at the end of log phase, although growth rate was similar to the parental strains initially. The strain devoid of all three hydrogenases (ΔSHIΔSHIIΔmbhL) did not produce any detectable H2 under any of the growth conditions (Figure 3). With sufficient S0 (2 g/L) only a very small amount of H2 was produced in the parental strains and in ΔmbhL (<5% of that produced in the absence of S0, Figure 3; Schut et al., 2007). With limiting S0, both S0 is reduced and H2 is produced (c.a. 50% compared to no S0) in the parental strains (Figures 2 and 3). Interestingly, when ΔmbhL was grown with limiting S0, a small but significant amount of H2 was produced (c.a. 20% compared to the parental strains). Therefore, the H2 produced in the ΔmbhL strain must be catalyzed by SHI, showing that this cytosolic “uptake” hydrogenase can also produce H2 from NADPH in vivo. A concentration of 0.5 g/L S0 (equivalent to ca. 15 mM) appears to be limiting for the cell’s metabolism even though the cultures are continuously shaken during growth. We observed significant amounts of S0 left in suspension in the culture medium after growth. Since only up to 3 mM rather than 15 mM sulfide is produced in these cultures, it appears that not all S0 in the medium is accessible to the cells.
Figure 2. Growth characteristics of P. furiosus strains grown in maltose-based medium (A), supplemented with 0.5 g/L S0 (B), and supplemented with 2 g/L S0 (C). The symbols represent: closed circles, COM1c; open squares, ΔSHIΔSHII; open triangles, ΔmbhL; closed diamonds, ΔSHIΔSHIIΔmbhL.
Figure 3. Comparison of H2 (A) and H2S (B) production by P. furiosus strains. White bars, cells grown without S0 (no H2S is produced); light gray bars, cells grown with 0.5 g/L S0; dark gray bars, cells grown with 2 g/L S0. The amounts of H2 and H2S produced are given with respect to the total cell protein in the culture.
The glycolytic pathway of P. furiosus only uses a low potential ferredoxin that is linked to MBH, for the disposal of all reducing equivalents as H2, with simultaneous production of an ion gradient for energy generation (Sapra et al., 2003; Verhees et al., 2004). From this study involving all hydrogenases in P. furiosus, it is clear that MBH is the only enzyme that produces H2 in wild-type cells and that no alternative electron pathway is available to P. furiosus that can allow for growth in the absence of S0. In addition, no other enzyme (for example MBX) is capable of producing H2 in vivo, as shown by the lack of H2 formation in the strain lacking all three hydrogenases. Similar observations were made for the related archaeon Thermococcus kodakaraensis, in which the disruption of its MBH also did not allow growth under H2 evolving conditions (Kanai et al., 2005, 2011; Santangelo et al., 2011). We propose that the in vivo function of SHI is to recycle H2 for the formation of NADPH needed for biosynthesis. Although in the double deletion mutant lacking both SHI and SHII no hydrogenase activity was observed in the cytoplasmic fraction of cellular extracts, no growth phenotype was observed when either or both of these hydrogenases are absent (Lipscomb et al., 2011). In this case other enzymes must provide the pool of NADPH, and a potential candidate is the ferredoxin:NADPH oxidoreductase (FNOR) described previously (Ma and Adams, 2001a). In a previous study, an SHI overexpression strain was constructed, and this strain also did not display any obvious phenotype, although it contained almost an order of magnitude more SHI activity (Chandrayan et al., 2011). The relative amount of H2 produced in the strain lacking both SHI and SHII was not significantly different than that in the parental strains (Figure 3). However, conflicting results have been reported with a T. kodakaraensis deletion strain lacking its SHI. One study found only a small increase in H2 production (c.a. 10%; Kanai et al., 2011), which is more or less in agreement with our results, while another reported over a fivefold increase in relative H2 production (Santangelo et al., 2011). Both studies used similar growth media and H2 measurement methods, and it is not clear why these studies give such different results. In general, T. kodakaraensis displays growth yields and H2 production rates similar to what has been observed in P. furiosus and other Thermococcales (Kanai et al., 2005; Verhaart et al., 2010).
In the natural environment, the use of H2 recycling could have a distinct energetic advantage because the cytosolic hydrogenases could provide reductant in the form of NADPH without interfering with the energy balance through electron transport phosphorylation. However, we predict that there is a low level of H2 recycling in P. furiosus when grown with maltose as the carbon source, especially considering the low growth yields on this substrate (25 g cdw/mol glucose utilized; Kengen and Stams, 1994). Assuming all major cellular components (protein, nucleic acids, and lipids) are synthesized de novo, we estimate about 6% H2 recycling (Kanehisa et al., 2004, 2008). In the laboratory setting when these organisms are grown in nutrient rich conditions, H2 recycling would not be important to the overall growth of the organism, and this may be the reason why there is a lack of phenotype for the SHI and SHII deletion strains. When SHI was first described, it was proposed to be responsible for the production of H2, but the subsequent discovery of MBH called this into question (Ma et al., 1993; Sapra et al., 2000; Silva et al., 2000). The generation of H2 from NADPH is thermodynamically unfavorable; however, in vitro this reaction can be easily demonstrated (Ma and Adams, 2001b; Verhaart et al., 2010). In this study, we have now shown that this reaction can actually take place in vivo, although at a low level since SHI cannot compensate for the absence of MBH to allow growth of the ΔmbhL mutant in the absence of S0.
Members of the order Thermococcales are characterized by the ability to use S0 as an electron acceptor (Kelly and Adams, 1994). We have previously shown that peptides can only be utilized by P. furiosus (and likely most Thermococcales) in the presence of S0, and we have concluded that S0 is the preferred electron acceptor (Adams et al., 2001; Schut et al., 2007). When S0 is made available to the cell, a rapid switch from H2 production to S0 metabolism occurs, and this is orchestrated at least in part by the redox sensitive SurR regulator (Schut et al., 2007; Lipscomb et al., 2009; Yang et al., 2010). However, P. furiosus does not appear to possess a high affinity S0 binding system such as that described for Wolinella succinogenes (Sud; Klimmek et al., 1998). From the results presented herein it appears that the addition of sufficient S0 to a maltose-based medium seems to consistently reduce the overall cell yield (by c.a. 10–20%). At 0.5 g/L, S0 appears to be limiting to the cells, but the overall concentration (15 mM “S” atoms) should be sufficient to provide the sole electron sink. In this case, the cells are able to utilize both H2 and S0 metabolism simultaneously and produce both H2 and H2S. This type of mixed H2 and S0 metabolism has also been observed for Staphylothermus marinus which also contains orthologous MBH and MBX gene clusters (Hao and Ma, 2003; Anderson et al., 2009). Altogether, this suggests that P. furiosus has a relatively low affinity for S0, and that, when growing on carbohydrates, it might actually prefer to generate H2 rather than utilizing the poorly soluble S0 as an electron acceptor.
The authors declare that the research was conducted in the absence of any commercial or financial relationships that could be construed as a potential conflict of interest.
We acknowledge the Division of Chemical Sciences, Geosciences and Biosciences, Office of Basic Energy Sciences of the U.S. Department of Energy for funding in part the strain construction through grant DE-FG05-95ER20175 (to Michael W. W. Adams) and the Office of Biological and Environmental Research of the Office of Basic Energy Sciences, Office of Science, U.S. Department of Energy for funding strain analyses through grant FG02-08ER64690 (to Robert A. Scott).
Adams, M. W. W., Holden, J. F., Menon, A. L., Schut, G. J., Grunden, A. M., Hou, C., Hutchins, A. M., Jenney, F. E., Kim, C., Ma, K. S., Pan, G. L., Roy, R., Sapra, R., Story, S. V., and Verhagen, M. F. J. M. (2001). Key role for sulfur in peptide metabolism and in regulation of three hydrogenases in the hyperthermophilic archaeon Pyrococcus furiosus. J. Bacteriol. 183, 716–724.
Anderson, I. J., Dharmarajan, L., Rodriguez, J., Hooper, S., Porat, I., Ulrich, L. E., Elkins, J. G., Mavromatis, K., Sun, H., Land, M., Lapidus, A., Lucas, S., Barry, K., Huber, H., Zhulin, I. B., Whitman, W. B., Mukhopadhyay, B., Woese, C., Bristow, J., and Kyrpides, N. (2009). The complete genome sequence of Staphylothermus marinus reveals differences in sulfur metabolism among heterotrophic Crenarchaeota. BMC Genomics 10, 145. doi:10.1186/1471-2164-10-145
Bradford, M. M. (1976). A rapid and sensitive method for the quantitation of microgram quantities of protein utilizing the principle of protein-dye binding. Anal. Biochem. 72, 248–254.
Bridger, S. L., Clarkson, S. M., Stirrett, K., Debarry, M. B., Lipscomb, G. L., Schut, G. J., Westpheling, J., Scott, R. A., and Adams, M. W. (2011). Deletion strains reveal metabolic roles for key elemental sulfur-responsive proteins in Pyrococcus furiosus. J. Bacteriol. 193, 6498–6504.
Chandrayan, S. K., Mcternan, P. M., Hopkins, R. C., Sun, J., Jenney, F. E. Jr., and Adams, M. W. (2011). Engineering hyperthermophilic archaeon Pyrococcus furiosus to overproduce its cytoplasmic [NiFe]-hydrogenase. J. Biol. Chem. 287, 3257–3264.
Chen, J. S., and Mortenson, L. E. (1977). Inhibition of methylene blue formation during determination of the acid-labile sulfide of iron-sulfur protein samples containing dithionite. Anal. Biochem. 79, 157–165.
de Vos, W. M., Kengen, S. W. M., Voorhorst, W. G. B., and Van Der Oost, J. (1998). Sugar utilization and its control in hyperthermophiles. Extremophiles 2, 201–205.
Driskill, L. E., Kusy, K., Bauer, M. W., and Kelly, R. M. (1999). Relationship between glycosyl hydrolase inventory and growth physiology of the hyperthermophile Pyrococcus furiosus on carbohydrate-based media. Appl. Environ. Microbiol. 65, 893–897.
Fiala, G., and Stetter, K. O. (1986). Pyrococcus furiosus sp. nov., represents a novel genus of marine heterotrophic archaebacteria growing optimally at 100°C. Arch. Microbiol. 145, 56–61.
Hagedoorn, P. L., Driessen, M. C., Van Den Bosch, M., Landa, I., and Hagen, W. R. (1998). Hyperthermophilic redox chemistry: a re-evaluation. FEBS Lett. 440, 311–314.
Hao, X., and Ma, K. (2003). Minimal sulfur requirement for growth and sulfur-dependent metabolism of the hyperthermophilic archaeon Staphylothermus marinus. Archaea 1, 191–197.
Horton, R. M., Hunt, H. D., Ho, S. N., Pullen, J. K., and Pease, L. R. (1989). Engineering hybrid genes without the use of restriction enzymes: gene splicing by overlap extension. Gene 77, 61–68.
Kanai, T., Imanaka, H., Nakajima, A., Uwamori, K., Omori, Y., Fukui, T., Atomi, H., and Imanaka, T. (2005). Continuous hydrogen production by the hyperthermophilic archaeon, Thermococcus kodakaraensis KOD1. J. Biotechnol. 116, 271–282.
Kanai, T., Matsuoka, R., Beppu, H., Nakajima, A., Okada, Y., Atomi, H., and Imanaka, T. (2011). Distinct physiological roles of the three [NiFe]-hydrogenase orthologs in the hyperthermophilic archaeon Thermococcus kodakaraensis. J. Bacteriol. 193, 3109–3116.
Kanehisa, M., Araki, M., Goto, S., Hattori, M., Hirakawa, M., Itoh, M., Katayama, T., Kawashima, S., Okuda, S., Tokimatsu, T., and Yamanishi, Y. (2008). KEGG for linking genomes to life and the environment. Nucleic Acids Res. 36, D480–D484.
Kanehisa, M., Goto, S., Kawashima, S., Okuno, Y., and Hattori, M. (2004). The KEGG resource for deciphering the genome. Nucleic Acids Res. 32, D277–D280.
Kelly, R. M., and Adams, M. W. (1994). Metabolism in hyperthermophilic microorganisms. Antonie Van Leeuwenhoek 66, 247–270.
Kengen, S. W., De Bok, F. A., Van Loo, N. D., Dijkema, C., Stams, A. J., and De Vos, W. M. (1994). Evidence for the operation of a novel Embden-Meyerhof pathway that involves ADP-dependent kinases during sugar fermentation by Pyrococcus furiosus. J. Biol. Chem. 269, 17537–17541.
Kengen, S. W. M., and Stams, A. J. M. (1994). Growth and energy-conservation in batch cultures of Pyrococcus furiosus. FEMS Microbiol. Lett. 117, 305–309.
Klimmek, O., Kreis, V., Klein, C., Simon, J., Wittershagen, A., and Kroger, A. (1998). The function of the periplasmic Sud protein in polysulfide respiration of Wolinella succinogenes. Eur. J. Biochem. 253, 263–269.
Lipscomb, G. L., Keese, A. M., Cowart, D. M., Schut, G. J., Thomm, M., Adams, M. W., and Scott, R. A. (2009). SurR: a transcriptional activator and repressor controlling hydrogen and elemental sulphur metabolism in Pyrococcus furiosus. Mol. Microbiol. 71, 332–349.
Lipscomb, G. L., Stirrett, K., Schut, G. J., Yang, F., Jenney, F. E. Jr., Scott, R. A., Adams, M. W., and Westpheling, J. (2011). Natural competence in the hyperthermophilic archaeon Pyrococcus furiosus facilitates genetic manipulation: construction of markerless deletions of genes encoding the two cytoplasmic hydrogenases. Appl. Environ. Microbiol. 77, 2232–2238.
Ma, K., and Adams, M. W. (2001a). Ferredoxin:NADP oxidoreductase from Pyrococcus furiosus. Meth. Enzymol. 334, 40–45.
Ma, K., and Adams, M. W. (2001b). Hydrogenases I and II from Pyrococcus furiosus. Meth. Enzymol. 331, 208–216.
Ma, K., Schicho, R. N., Kelly, R. M., and Adams, M. W. (1993). Hydrogenase of the hyperthermophile Pyrococcus furiosus is an elemental sulfur reductase or sulfhydrogenase: evidence for a sulfur-reducing hydrogenase ancestor. Proc. Natl. Acad. Sci. U.S.A. 90, 5341–5344.
Park, J. B., Fan, C. L., Hoffman, B. M., and Adams, M. W. (1991). Potentiometric and electron nuclear double resonance properties of the two spin forms of the [4Fe-4S]+ cluster in the novel ferredoxin from the hyperthermophilic archaebacterium Pyrococcus furiosus. J. Biol. Chem. 266, 19351–19356.
Santangelo, T. J., Cubonova, L., and Reeve, J. N. (2011). Deletion of alternative pathways for reductant recycling in Thermococcus kodakarensis increases hydrogen production. Mol. Microbiol. 81, 897–911.
Sapra, R., Bagramyan, K., and Adams, M. W. (2003). A simple energy-conserving system: proton reduction coupled to proton translocation. Proc. Natl. Acad. Sci. U.S.A. 100, 7545–7550.
Sapra, R., Verhagen, M. F. J. M., and Adams, M. W. W. (2000). Purification and characterization of a membrane-bound hydrogenase from the hyperthermophilic archaeon Pyrococcus furiosus. J. Bacteriol. 182, 3423–3428.
Schut, G. J., Bridger, S. L., and Adams, M. W. (2007). Insights into the metabolism of elemental sulfur by the hyperthermophilic archaeon Pyrococcus furiosus: characterization of a coenzyme A- dependent NAD(P)H sulfur oxidoreductase. J. Bacteriol. 189, 4431–4441.
Shima, S., and Thauer, R. K. (2007). A third type of hydrogenase catalyzing H2 activation. Chem. Rec. 7, 37–46.
Silva, P. J., Van Den Ban, E. C., Wassink, H., Haaker, H., De Castro, B., Robb, F. T., and Hagen, W. R. (2000). Enzymes of hydrogen metabolism in Pyrococcus furiosus. Eur. J. Biochem. 267, 6541–6551.
Smith, E. T., Blamey, J. M., Zhou, Z. H., and Adams, M. W. (1995). A variable-temperature direct electrochemical study of metalloproteins from hyperthermophilic microorganisms involved in hydrogen production from pyruvate. Biochemistry 34, 7161–7169.
van Haaster, D. J., Silva, P. J., Hagedoorn, P. L., Jongejan, J. A., and Hagen, W. R. (2008). Reinvestigation of the steady-state kinetics and physiological function of the soluble NiFe-hydrogenase I of Pyrococcus furiosus. J. Bacteriol. 190, 1584–1587.
Verhaart, M. R., Bielen, A. A., Van Der Oost, J., Stams, A. J., and Kengen, S. W. (2010). Hydrogen production by hyperthermophilic and extremely thermophilic bacteria and archaea: mechanisms for reductant disposal. Environ. Technol. 31, 993–1003.
Verhees, C. H., Kengen, S. W. M., Tuininga, J. E., Schut, G. J., Adams, M. W. W., De Vos, W. M., and Van Der Oost, J. (2004). The unique features of glycolytic pathways in Archaea. Biochem. J. 377, 819–822.
Vignais, P. M., and Billoud, B. (2007). Occurrence, classification, and biological function of hydrogenases: an overview. Chem. Rev. 107, 4206–4272.
Vignais, P. M., Billoud, B., and Meyer, J. (2001). Classification and phylogeny of hydrogenases. FEMS Microbiol. Rev. 25, 455–501.
Keywords: hydrogenase, energy metabolism, sulfur, ferredoxin, Pyrococcus furiosus, thermophile, anaerobe
Citation: Schut GJ, Nixon WJ, Lipscomb GL, Scott RA and Adams MWW (2012) Mutational analyses of the enzymes involved in the metabolism of hydrogen by the hyperthermophilic archaeon Pyrococcus furiosus. Front. Microbio. 3:163. doi: 10.3389/fmicb.2012.00163
Received: 20 March 2012; Accepted: 12 April 2012;
Published online: 01 May 2012.
Edited by:
Frank T. Robb, University of California, USAReviewed by:
Rudolf Kurt Thauer, Max Planck Society – Institute for Terrestrial Microbiology, GermanyCopyright: © 2012 Schut, Nixon, Lipscomb, Scott and Adams. This is an open-access article distributed under the terms of the Creative Commons Attribution Non Commercial License, which permits non-commercial use, distribution, and reproduction in other forums, provided the original authors and source are credited.
*Correspondence: Michael W. W. Adams, Department of Biochemistry and Molecular Biology, University of Georgia, Athens, GA 30602, USA. e-mail:YWRhbXNAYm1iLnVnYS5lZHU=
†Gerrit J. Schut and William J. Nixon have contributed equally to this work.
Disclaimer: All claims expressed in this article are solely those of the authors and do not necessarily represent those of their affiliated organizations, or those of the publisher, the editors and the reviewers. Any product that may be evaluated in this article or claim that may be made by its manufacturer is not guaranteed or endorsed by the publisher.
Research integrity at Frontiers
Learn more about the work of our research integrity team to safeguard the quality of each article we publish.