- 1 Ocean and Earth Science, National Oceanography Centre – Southampton, University of Southampton, Southampton, UK
- 2 Bermuda Institute of Ocean Sciences, St. George’s, Bermuda
Iron (Fe) is an essential micronutrient for marine organisms, and it is now well established that low Fe availability controls phytoplankton productivity, community structure, and ecosystem functioning in vast regions of the global ocean. The biogeochemical cycle of Fe involves complex interactions between lithogenic inputs (atmospheric, continental, or hydrothermal), dissolution, precipitation, scavenging, biological uptake, remineralization, and sedimentation processes. Each of these aspects of Fe biogeochemical cycling is likely influenced by organic Fe-binding ligands, which complex more than 99% of dissolved Fe. In this review we consider recent advances in our knowledge of Fe complexation in the marine environment and their implications for the biogeochemistry of Fe in the ocean. We also highlight the importance of constraining the dissolved Fe concentration value used in interpreting voltammetric titration data for the determination of Fe speciation. Within the published Fe speciation data, there appear to be important temporal and spatial variations in Fe-binding ligand concentrations and their conditional stability constants in the marine environment. Excess ligand concentrations, particularly in the truly soluble size fraction, seem to be consistently higher in the upper water column, and especially in Fe-limited, but productive, waters. Evidence is accumulating for an association of Fe with both small, well-defined ligands, such as siderophores, as well as with larger, macromolecular complexes like humic substances, exopolymeric substances, and transparent exopolymers. The diverse size spectrum and chemical nature of Fe ligand complexes corresponds to a change in kinetic inertness which will have a consequent impact on biological availability. However, much work is still to be done in coupling voltammetry, mass spectrometry techniques, and process studies to better characterize the nature and cycling of Fe-binding ligands in the marine environment.
Introduction – Iron Biogeochemistry in the Ocean and the Importance of Iron Speciation
Approximately 30% of surface waters in the open ocean are known as high nutrient low chlorophyll (HNLC) regions (Boyd et al., 2007). These areas are replete in the macronutrients nitrate and phosphate, but present lower phytoplankton biomass, in terms of chlorophyll concentrations, than expected from residual macronutrient concentrations (Figure 1). The restriction of phytoplankton growth in these regions is now acknowledged to be the result of iron (Fe) limitation (Martin and Fitzwater, 1988; Boyd et al., 2007). Fe is a micronutrient required for proteins involved in fundamental cellular processes, including both photosynthesis and respiration (Raven et al., 1999). Despite being the fourth most abundant element in the Earth’s crust (Liu and Millero, 2002), dissolved Fe (dFe: <0.2 or 0.45 μm) concentrations in open ocean surface waters typically fall below 0.2 nM, (De Baar and De Jong, 2001; Boyd and Ellwood, 2010) and dFe generally exhibits a nutrient-type depth profile in the ocean, depicting removal in surface waters by biological uptake, and increased concentrations at depth from remineralization processes occurring through the water column (Boyd and Ellwood, 2010). Although low, dFe concentrations in the ocean can be much higher than might be predicted given that the solubility of ferric hydroxide in seawater at pH 8.1 and 25°C has been determined to be as low as 0.01 nM (Liu and Millero, 2002). The presence of dFe at concentrations beyond the inorganic solubility of Fe is thought to be facilitated by organic complexation of Fe with stabilizing ligands, which buffer dFe in seawater against hydrolysis and ensuing precipitation (Hunter and Boyd, 2007; Boyd and Ellwood, 2010). However, the overall physico-chemical speciation of dFe, which encompasses all of its possible physical and chemical forms in seawater, is more complex than implied by the consideration of organic complexation alone. The different physico-chemical fractions of dFe include Fe(II), colloidal, truly soluble, and inorganic iron in addition to organically complexed iron. These different fractions have different environmental and biological mobility (Kuma et al., 1996; Maldonado et al., 2005; Hunter and Boyd, 2007; Kitayama et al., 2009; Boyd and Ellwood, 2010; Hassler et al., 2011b). The motivation for understanding the physico-chemical speciation of Fe, therefore, results from a desire to understand how these different fractions influence the overall biogeochemistry of Fe in the oceans.
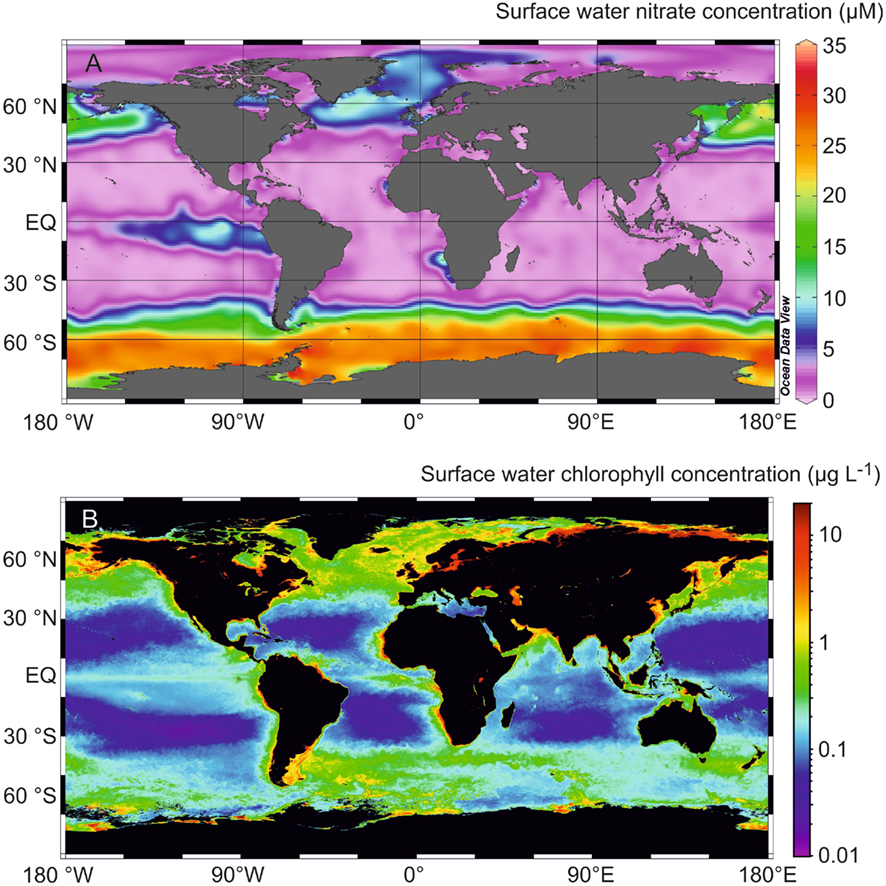
Figure 1. (A) Annualized average nitrate (μM) and (B) composite chlorophyll a (mg L−1) distributions observed in surface waters in the global ocean. The nitrate distribution was obtained using data from the World Ocean Atlas 2009 (http://www.nodc.noaa.gov/OC5/WOA09/pr_woa09.html), while the chlorophyll a distribution represents the 2009 Aqua MODIS chlorophyll composite (http://oceancolor.gsfc.nasa.gov/cgi/l3).
It is important to note that dissolved iron (dFe) is operationally defined by filtration, with early studies employing 0.45 μm or, more recently, 0.2 μm membrane filters (De Baar and De Jong, 2001; Cutter et al., 2010). However, it has been shown that a significant proportion of dFe is colloidal (Fecolloidal; Wu and Luther, 1994; Cullen et al., 2006; Bergquist et al., 2007; Kondo et al., 2008; Schlosser and Croot, 2008; Boye et al., 2010). Colloidal Fe is characterized as the difference between the Fe concentration determined in the <0.2 μm fraction (dFe) and the >1 kDa or >0.02 μm fraction, depending on whether cross flow filtration or membrane filtration techniques are used for the determination (Schlosser and Croot, 2008). The colloidal fraction is not measured directly, but inferred from the difference between dissolved (<0.2 μm) and soluble (<1 kDa or <0.02 μm) fractions.
The mass balances for Fe when considering its physical distribution can be described as

while the mass balance from a chemical perspective might be described as

where Fe′ represents labile inorganic Fe complexes, FeL represents Fe organic ligand complexes exchangeable within a time scale of <1 day, and Feinert represents the Fe fraction bound up in matrices that are essentially non-labile. As our analytical methods for the determination of the physico-chemical speciation of Fe tend to focus on either the physical (e.g., Schlosser and Croot, 2008; Baalousha et al., 2011) or the chemical (e.g., Gledhill and van den Berg, 1994; Rue and Bruland, 1995; van den Berg, 1995; Wu and Luther, 1995; Laglera et al., 2007; Mawji et al., 2008a; Velasquez et al., 2011) perspective, reconciling these two approaches remains a major challenge to Fe biogeochemists.
In recent years there has been a concerted effort to understand more about both the physical partitioning of Fe in the marine environment, and the chemical nature of the Fe ligand pool. The application of filtration with trace metal clean 0.02 μm pore size membrane filtration, ultrafiltration (10 kDa cut offs), and flow field flow fractionation (FFFF) coupled to ultra-violet (UV) and inductively coupled plasma-mass spectrometry (ICP-MS) detection techniques have considerably improved our knowledge of the physical partitioning of Fe in marine waters (Schlosser and Croot, 2008; Baalousha et al., 2011). Characterization of the FeL pool has been tackled through the utilization of high performance liquid chromatography–electrospray ionization-mass spectrometry (HPLC–ESI-MS) and development of novel electroanalytical techniques (McCormack et al., 2003; Laglera et al., 2007; Velasquez et al., 2011). In parallel to these advances a concerted effort is being made to improve our understanding of the robustness of competitive ligand exchange–adsorptive cathodic stripping voltammetry (CLE–ACSV), the technique most commonly used to determine Fe complexation in seawater (Buck et al., under review;Laglera et al., 2011). These advances have indicated that although the absolute physical partitioning determined varies from study to study as a result of the different techniques and filter cut offs, the colloidal Fe pool makes up between 30 and 91% of the dFe pool (Wu and Luther, 1994; Nishioka et al., 2001; Cullen et al., 2006; Bergquist et al., 2007; Hurst and Bruland, 2008; Kondo et al., 2008; Schlosser and Croot, 2008; Boye et al., 2010). The presence of Fe-binding ligands has been inferred in colloidal and measured in soluble fractions (Wu et al., 2001; Boye et al., 2010; Thuroczy et al., 2010), but both FFFF and ultrafiltration studies indicate that not all of the colloidal Fe (organic or inorganic) is exchangeable or under saturated with respect to Fe (Wu et al., 2001; Boye et al., 2010; Stolpe and Hassellov, 2010; Stolpe et al., 2010; Thuroczy et al., 2010). The existence of an inert colloidal fraction has broad implications for our understanding of Fe biogeochemistry and its significance in the ocean has yet to be properly assessed. Thus, while Fesoluble might reasonably be expected to include Fe′, FeL, or Feinert are unlikely to be discreet to Fesoluble, Fecolloidal, or Feparticulate.
Even though we have not fully characterized either colloidal or organic Fe associations, efforts to understand the overall effects of Fe speciation on Fe biogeochemistry have been made (Archer and Johnson, 2000; Moore et al., 2004; Parekh et al., 2005; Weber et al., 2005; Fan, 2008; Moore and Braucher, 2008; Tagliabue et al., 2009; Fan and Dunne, 2011; Tagliabue and Voelker, 2011). Modelers have made considerable progress toward capturing the complexity of iron speciation, incorporating inorganic iron scavenging and up to two ligand classes, in order to investigate the large scale implications of iron speciation on ocean productivity and the potential effects of a changing climate (Tagliabue et al., 2009; Ye et al., 2009; Tagliabue and Voelker, 2011). Fe biogeochemical models incorporating Fe speciation tend to highlight the importance of photoreduction processes in determining the dissolved concentrations of Fe in surface waters, while indicating that organic ligands are likely to strongly influence the thermodynamic solubility of Fe (Weber et al., 2005; Fan, 2008; Tagliabue et al., 2009; Tagliabue and Voelker, 2011). Furthermore, variations in organic ligand concentration and conditional stability constants have also been shown to influence the residence time and potential bioavailability of Fe in models (Tagliabue et al., 2009). Other studies have shown that different organic ligands have different chemical labilities and different susceptibilities to photoreduction (Hutchins et al., 1999; Barbeau et al., 2001, 2003; Maldonado et al., 2005; Hassler et al., 2011b). Overall, such studies highlight the need to characterize more fully the physico-chemical speciation of dFe.
In this paper, we will review current knowledge of the organic complexation of Fe, the characterization and distributions of specific FeL complexes, and the characterization of colloidal Fe fractions as a first attempt at reconciling the different approaches to understanding the physico-chemical speciation of dFe in seawater. We will not consider the reduced Fe pool, Fe(II), in this review as our understanding of the interactions between Fe(II), Fe(III), and organic matter (e.g., Toner et al., 2009; Bligh and Waite, 2010) require further investigation. However, it should be born in mind that Fe(II) is likely important to Fe biogeochemistry as it is thought to be highly biologically available and can make up 50–60% of the dFe pool in illuminated surface waters or near sediments, hydrothermal vent systems, or oxygen minimum zones (Hong and Kester, 1986; Kuma et al., 1992; Johnson et al., 1994; Gledhill and van den Berg, 1995; Croot et al., 2005; Ussher et al., 2007; Hansard et al., 2009; Sarthou et al., 2011).
Iron Complexation as Determined by Competitive Equilibrium Experiments
The organic complexation of dFe, including organic Fe-binding ligand concentrations ([Li]) and their associated conditional stability constants is primarily measured in seawater using the electrochemical technique CLE–ACSV. The ambient Fe-binding ligands determined by this technique are typically described as ligand “classes,” which are operationally defined by the associated conditional stability constant measured. Ligand classes are denoted as Li, where i = 1 for stronger ligand classes and i = 2, 3, etc., for progressively weaker ligand classes. Conditional stability constants for these ligand classes are expressed as either
where
and αFe′ is the inorganic side reaction coefficient for Fe, with αFe′ = [Fe′]/[Fe3+]. αFe′ varies with pH (Byrne et al., 1988), but a value of 1010 is commonly used for αFe′ in pH 8 seawater (Hudson et al., 1992; Sunda and Huntsman, 2003), although other values have been used (Gledhill and van den Berg, 1994; Rue and Bruland, 1997; Gledhill et al., 1998; Nolting et al., 1998). In CLE–ACSV, samples are buffered prior to analyses, typically to pH 8, and mostly equilibrated and measured at room temperature, so the use of an αFe′ = 1010 is reasonable for these cases, regardless of original sample pH or temperature. To better facilitate comparisons across studies, we encourage analysts to consider adjusting their
using the appropriate αFe′ for the analytical pH employed. Similarly, those who report
are encouraged to specify the αFe′ used.
Competitive Ligand Exchange–Adsorptive Cathodic Stripping Voltammetry
Aliquots of a filtered, but otherwise unaltered, seawater sample are first buffered to maintain pH near 8, and then titrated with increasing additions of dissolved Fe (dFe) from +0 to typically 10×–20× the ambient dFe concentration. It is recommended to have two +0 Fe additions in the titration, with at least eight Fe amendments, for a total of 10 or more titration points to provide the best data structure for interpretation (Garnier et al., 2004; Sander et al., 2011). DFe additions are left to equilibrate with ambient Fe-binding ligands, often for several hours, before each aliquot is amended with a given concentration of a well-characterized added ligand (AL) and allowed an additional equilibration time of minutes to hours. Once equilibrated with both added dFe and AL, each sample aliquot of the titration is analyzed sequentially by ACSV on a hanging mercury drop electrode (HMDE).
Over a deposition time predetermined to allow sufficient preconcentration of Fe(AL)x in the sample, the Fe(AL)x complex formed in the titrations is adsorbed at a set initial potential to the surface of the HMDE while stirring the solution. As the deposition time employed is applied to all aliquots in a given titration, it is essential that the length of time chosen is long enough to distinguish the small increases in the added dFe between vials early in the titration, but not so long that the larger amendments of dFe at the end of the titration saturates the HMDE surface. After the deposition time concludes, there is often a few seconds of “quiet time,” when stirring is stopped, before the voltage applied to the HMDE is ramped in the negative direction (cathodic stripping) following the optimal excitation signal wave form (differential pulse, linear sweep, Osteryoung square wave) for the Fe(AL)x chosen.
At the reduction potential of Fe, roughly −0.5 V (depending on AL chosen), Fe is reduced from the Fe(AL)x complex, or, in some cases, the Fe(AL)x itself may reduce, generating a peak in current measured at the HMDE. The height of this peak in current, typically expressed in absolute nA units, is recorded for each aliquot as a titration point and plotted against the added dFe to generate a titration curve. This raw titration data is then interpreted using either linear (Scatchard, 1949; Ružic, 1982; Van Den Berg, 1982) or non-linear (Gerringa et al., 1995) transformations to determine ambient Fe-binding ligand concentrations and their thermodynamic conditional stability constants.
The concentration and choice of AL employed, along with the thermodynamic characteristics of the Fe(AL)x complex formed, defines the analytical window of the analyses. This analytical window is described as where
The analytical window applied constrains the measured ligand concentrations and conditional stability constants such that the end result is best described as averages of the different ligand classes identified within an analytical window. These parameters produce an
where
In most cases, only one ligand class is identified in Fe speciation titrations, but in the case of more than one ligand class,
While the use of multiple analytical windows has not yet been addressed for Fe speciation, there is some indication from recent GEOTRACES inter calibration efforts that the analytical window applied to Fe speciation may impact calculated results for [Li] and (Buck et al., under review). This effect is much less pronounced, however, than has been established previously for dissolved copper (Cu) speciation (Bruland et al., 2000), likely reflecting the very different inorganic side reactions of Cu and Fe, as well as the relative breadth of the ligand pools for these elements.
With respect to ligand classes, it should be noted that analysts commonly apply the notation of “L1” to the strongest (or only) ligand class detected in their sample, regardless of the associated . Thus, ligand class definitions may vary widely between analysts and sample data sets, complicating comparisons across studies. For example, the range of
reported in the literature, for either the single ligand class identified or the designated L1 in two ligand systems, covers more than four orders of magnitude (from ∼9 to ∼13.5; Table 1). Even in field studies distinguishing two ligand classes, the
reported for the stronger ligand class range between 11.1 and 13.9, overlapping with the weaker ligand class
values of 9.7–11.95 reported (Table 1; Rue and Bruland, 1995, 1997; Cullen et al., 2006; Buck and Bruland, 2007; Buck et al., 2007; Ibisanmi et al., 2011). Correspondingly, it would be useful to agree on definitions for ligand classes based on the range of
reported in natural systems. Along these lines, we would propose using “L1” for
“L2” for log
and “L3” for
Regardless of whether such new definitions are employed, when comparing results between studies we implore analysts to consider making comparisons between
instead of designated ligand classes.
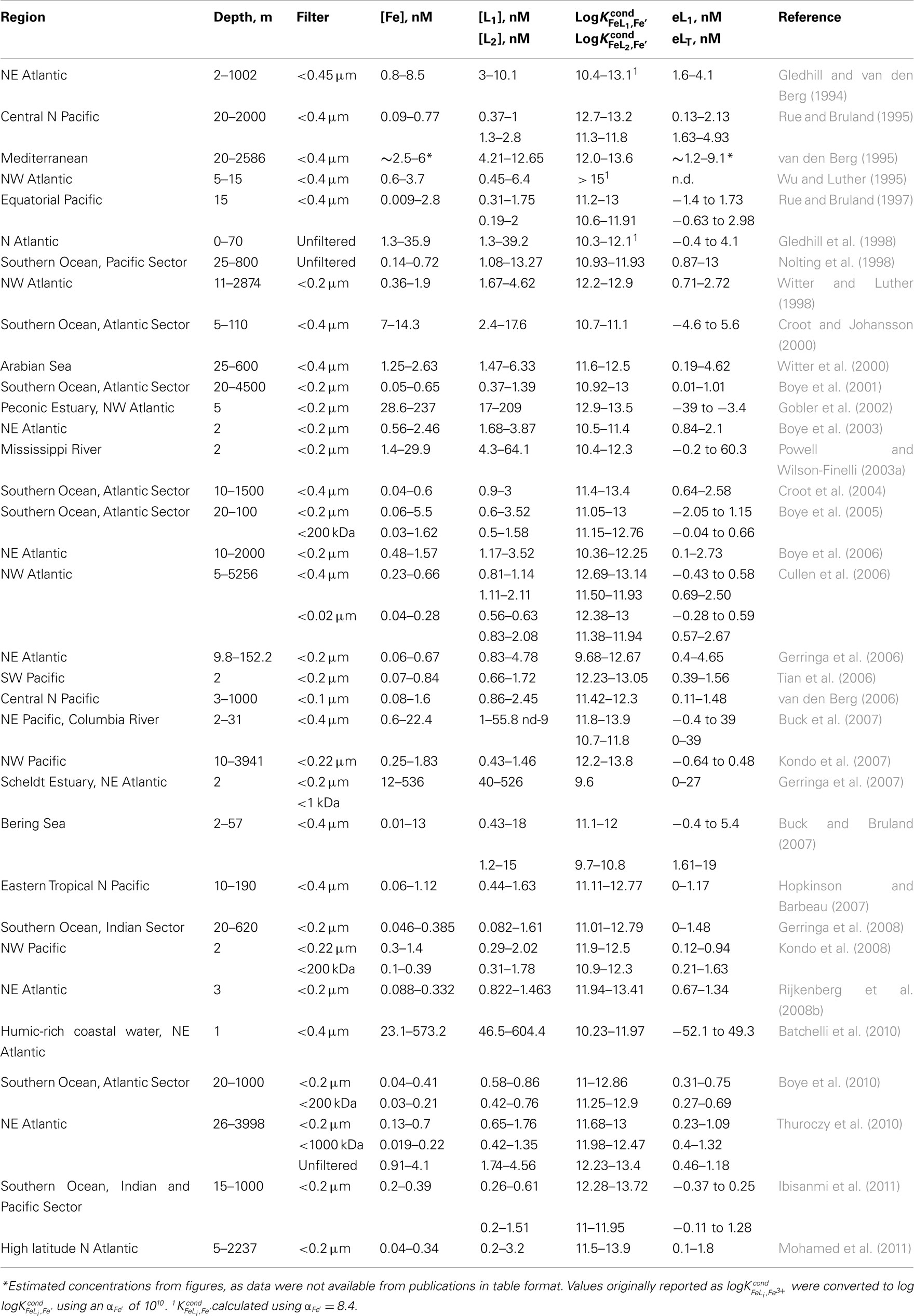
Table 1. Compilation of published field data on the organic complexation of dFe in marine environments, as measured by CLE–ACSV techniques.
Limitations of Interpretation
Competitive ligand exchange–ACSV is an indirect approach to determining the organic complexation of dFe. As such, there are some fundamental assumptions and limitations inherent to CLE–ACSV data interpretation, which are worth reviewing in brief before attempting to contextualize published field data. First, raw titration is interpreted assuming that ambient ligands are coordinated with dFe in a 1:1 ratio. In reality, the wide range of dissolved organic matter present in seawater likely includes ligands that may bind dFe in ratios greater than or less than 1:1. Thus, the reported ligand concentrations measured from CLE–ACSV represent the binding capacity for organic ligand classes present in the sample, not necessarily an absolute concentration of ligand molecules. As a consequence, some analysts report their measured ligand concentrations in terms of nanoequivalents (nEq; e.g., Nolting et al., 1998; Gerringa et al., 2008) or nanomolar Fe equivalents (e.g., Croot et al., 2004) instead of simply nanomolar concentrations to highlight this distinction.
A second, and more problematic, assumption in the interpretation of CLE–ACSV data is that all of the dFe in a given sample is in an exchangeable form with respect to the added competitive ligand (AL). Arising from this assumption, any dFe that does not bind to the AL to form Fe(AL)x is then assumed in the data interpretation to be complexed by an organic ligand class with a larger than the
applied. This is clearly problematic in natural samples, where much of the dFe, especially in high dFe environments, may exist as stable inorganic or organically associated colloids, or as other phases of dFe not exchangeable with AL (e.g., Feinert). It has been noted previously that inclusion of Feinert in calculations of ligand concentrations results in an overestimation of [Li] and a shift in the
toward higher stabilities (Bruland and Rue, 2001). This becomes particularly critical if ligand titrations are carried out in unfiltered samples (Gledhill et al., 1998; Thuroczy et al., 2010), but given the evidence for an inert colloidal fraction (see below), is also likely to affect determination of ligands in dissolved samples. Determinations of [Li] and
in the ocean are, thus, potentially subject to systematic errors when using the total dFe concentration in the interpretation of CLE–ACSV titration data.
To further explore the implications of overestimating exchangeable Fe, we consider the data in Thuroczy et al. (2010) and data on titrations in unfiltered samples from Gledhill et al. (1998), recalculated using the reactive Fe concentration (FeR) determined in the unfiltered samples (Gledhill et al., 1998). Although the datasets used were both obtained for unfiltered samples, the same systematic errors with respect to Feinert and exchangeable Fe will occur within the dFe pool, albeit to a lesser degree.
Plots of [Li] and determined using FeT (unfiltered), against [Li] and
determined using either dissolved or reactive Fe concentration (dFe or FeR), are presented in Figures 2A,B. In these studies, FeT was determined in unfiltered samples either by flow injection analysis (FIA) after acidification (pH 1.8, samples analyzed after 1 year; Thuroczy et al., 2010) or by ACSV after acidification (pH 2) and UV irradiation (Gledhill et al., 1998). Dissolved Fe was determined by FIA after filtration (<0.2 μm) and acidification (pH 1.8, samples analyzed after 12 h, Thuroczy et al., 2010) and FeR was determined in unfiltered samples by ACSV and defined as the amount of Fe freely complexed by 20 μM 1-nitroso-2-napthol at pH 6.9 (Gledhill et al., 1998).
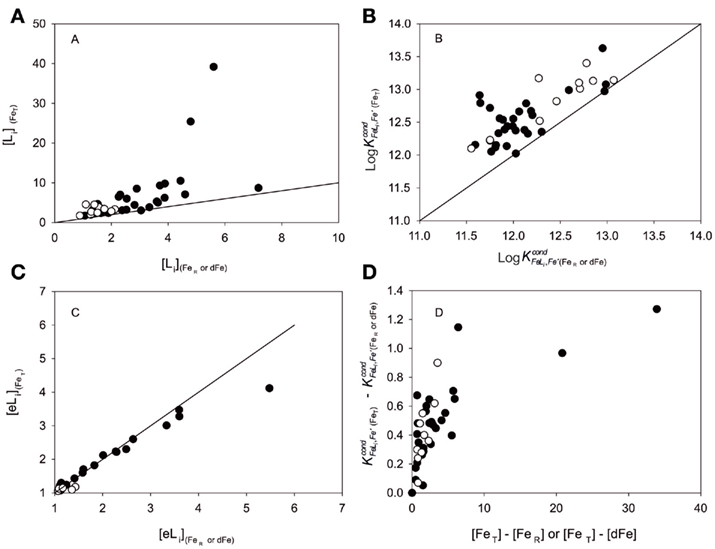
Figure 2. Comparison of data obtained for (A) the ligand concentration [Li], (B) conditional stability constant and (C) excess ligand concentration [eLi] determined using either total Fe concentrations or the lower dissolved or reactive Fe concentrations. Closed symbols represent data from Gledhill et al. (1998), where FeR was used in the comparison, and open symbols represent data from Thuroczy et al. (2010), where dFe was used in the comparison. (D) Plot of the dependence of the change in
on the difference between FeT and the lower Fe concentration. Solid lines represent a 1:1 relationship.
From these plots, it is clear that both [Li] and log are overestimated if the Fe concentration used in data transformation includes Feinert (Figures 2A,B). An overestimation of [Li] and
is not desirable as this will result in an overestimation of the degree to which L can stabilize Fe in solution, and can thus underestimate the potential for competing biogeochemical processes such as colloidal aggregation, scavenging, and uptake. Calculation of the excess ligand (eLi) for each case confirms results shown previously (Thuroczy et al., 2010), that this parameter is more or less independent of the Fe concentration used in the transformation, at least at low excess ligand concentrations (Figure 2C).
Figure 2D shows that overestimation of is likely to be important only in areas where the Feinert concentration is high. Such areas will include coastal regions as observed in Gledhill et al. (1998), hydrothermal vents, and the edge of the ice shelf (Bennett et al., 2008; van der Merwe et al., 2009; Batchelli et al., 2010; Stolpe and Hassellov, 2010; Stolpe et al., 2010). In these regions a good distinction between the dissolved Feinert and exchangeable Fe could be very informative, and may strongly influence our interpretation of the role of ligands in stabilizing Fe in solution (e.g., Sander and Koschinsky, 2011). Further work is therefore required in order to identify a practical method which can distinguish between Feinert and dFe. One approach may simply be the standard addition of dFe with over-competition with a higher concentration of AL, although this may be complicated by limits in the concentration of AL that may be used before saturation of the HMDE becomes problematic.
Clearly, the fractionation of Fe, particularly within the dissolved phase, between Feinert and exchangeable Fe deserves further attention. In the meantime, analysts may qualify ligand concentrations by reporting excess L ([eL] = [L] − [dFe]) as a proxy for ligand under saturation (Wu and Luther, 1995; Witter and Luther, 1998; Witter et al., 2000; Boye et al., 2001; Tian et al., 2006; Rijkenberg et al., 2008b; Thuroczy et al., 2010; Ibisanmi et al., 2011).
Distributions of Fe-Binding Ligands in the Marine Environment
The organic complexation of Fe, with typically >99.9% of dFe complexed by ligands in the marine environment, is a nearly ubiquitous feature of dFe speciation in seawater. While these Fe-binding ligands are present seemingly everywhere, from surface to deep waters of the coastal and open ocean, there are some distinguishable trends in their distributions and thermodynamic characteristics. Previous reviews have highlighted the predominant feature of higher ligand concentrations in surface waters of depth profiles, often with stronger stability constants, compared to deep waters (Bruland and Rue, 2001; Hunter and Boyd, 2007). Here we review trends of organic complexation of Fe in terms of excess ligand concentrations ([eLi] = [Li] − [dFe]), since total ligand concentrations ([Li]) determined by CLE–ACSV will include stable inorganic colloidal Fe or other Feinert components (see above).
In most waters, Fe-binding ligand concentrations exceed dFe (Table 1). Excess ligand concentrations are commonly highest and most variable in the upper water column (Rue and Bruland, 1995; van den Berg, 1995, 2006; Witter and Luther, 1998; Boye et al., 2001, 2006, 2010; Croot et al., 2004; Gerringa et al., 2006; Hopkinson and Barbeau, 2007; Kondo et al., 2007; Rijkenberg et al., 2008b; Thuroczy et al., 2010; Ibisanmi et al., 2011; Mohamed et al., 2011), and relatively static at depth within individual profiles (Rue and Bruland, 1995; van den Berg, 1995; Nolting et al., 1998; Boye et al., 2001, 2006, 2010; Ibisanmi et al., 2011). The highest excess ligand concentrations are often associated with the fluorescence or chlorophyll biomass maxima (Rue and Bruland, 1995; van den Berg, 1995, 2006; Boye et al., 2001, 2006; Croot et al., 2004; Gerringa et al., 2006, 2008; Tian et al., 2006; Buck and Bruland, 2007; Wagener et al., 2008; Ibisanmi et al., 2011). The uptake of dFe associated with fluorescence or biomass maxima results in elevated and variable excess ligand concentrations at low dFe (e.g., Buck and Bruland, 2007).
An atmospheric source has been hypothesized for surface waters of the NE Atlantic with low chlorophyll (Gerringa et al., 2006), though a reduction in excess ligand after dust deposition was observed by Rijkenberg et al. (2008b). Excess ligands in low chlorophyll surface waters may alternatively be the remnants of a previous bloom, as excess ligands have been shown in incubation experiments to increase in proportion to chlorophyll consumption by grazers (Sato et al., 2007). In some cases, a minimum in excess ligands within the upper water column is attributed to photochemical ligand destruction at the surface (Boye et al., 2001; Croot et al., 2004), although the photochemical lability of ambient Fe-binding ligands has also been shown to be rather unpredictable (Powell and Wilson-Finelli, 2003b; Rijkenberg et al., 2006b).
Anomalously high excess ligand concentrations have been reported in shelf and bottom boundary layers (Croot and Johansson, 2000; Gobler et al., 2002; Boye et al., 2003; Buck et al., 2007; Kondo et al., 2007; Gerringa et al., 2008; Batchelli et al., 2010), as well as in river plumes (Powell and Wilson-Finelli, 2003a; Buck et al., 2007; Kondo et al., 2007). The excess Fe-binding ligands measured in these coastal waters may be humic substances (HS), which have been suggested to be an important component of the ligand pool for dFe in margin environments (Laglera et al., 2007; Laglera and van den Berg, 2009). Regardless of chemical nature, river plumes and sediment resuspension on shelves appears to be a source of both dFe and Fe-binding ligands to the marine water column (Croot and Johansson, 2000; Powell and Wilson-Finelli, 2003a; Buck et al., 2007; Gerringa et al., 2008; Batchelli et al., 2010). As with dFe, total dissolved ligand concentrations also typically decrease from shore to sea (Boye et al., 2003; Buck and Bruland, 2007).
When two ligand classes are detected, a particularly strong ligand class is reported primarily in the upper water column, and the weaker ligand class
exists throughout the water column (Rue and Bruland, 1995, 1997; Cullen et al., 2006; Ibisanmi et al., 2011). When only one ligand class is measured, no trend in
values (∼11–12) is reported with depth in profiles, with similar log
values through the entire water column (van den Berg, 1995, 2006; Witter and Luther, 1998; Boye et al., 2001, 2006, 2010; Croot et al., 2004; Gerringa et al., 2006, 2008; Thuroczy et al., 2010).
values reported for coastal and open ocean samples are within the same range (∼9–14; Table 1), as are values from river plumes (10.4–13.9; Powell and Wilson-Finelli, 2003b; Buck et al., 2007; Table 1). Transects conducted between the shore and sea found no significant trends in
values with distance from shore (Boye et al., 2003; Buck and Bruland, 2007; Hopkinson and Barbeau, 2007).
Recent size fractionation studies of the dissolved ligand pool have shown that the excess ligand is observed predominantly in the soluble size fraction throughout the water column (Cullen et al., 2006; Boye et al., 2010; Thuroczy et al., 2010), but particularly in upper waters (Cullen et al., 2006; Kondo et al., 2008; Boye et al., 2010; Thuroczy et al., 2010). Colloidal ligands, on the other hand, approach Fe saturation (Gobler et al., 2002; Boye et al., 2005, 2010; Cullen et al., 2006; Gerringa et al., 2007; Kondo et al., 2008; Batchelli et al., 2010; Thuroczy et al., 2010), with the colloidal fraction of Fe, and presumably L, increasing with depth (Thuroczy et al., 2010).
The conditional stability constants of Fe-binding ligands in the soluble (<1–1000 kDa) size fraction are largely within 1 SD of the values reported in the total dissolved (<0.2–0.4 μm) size fraction (Boye et al., 2005, 2010; Cullen et al., 2006; Gerringa et al., 2007; Kondo et al., 2008; Thuroczy et al., 2010; Table 1). When two ligand classes were detected within the soluble and dissolved size fractions (Cullen et al., 2006), most of the ligands of both classes were present in the soluble (<0.02 μm) fraction, and excess ligand concentrations of both L1 and L2 were higher in the soluble fraction. The conditional stability constants of these ligand classes were similar in both size fractions (Cullen et al., 2006; Table 1). In mesoscale Fe fertilization experiments, Fe additions were observed primarily in the colloidal fraction (Boye et al., 2005; Kondo et al., 2008), and both Fe and ligand concentrations increased in response to Fe-enrichment (Rue and Bruland, 1997; Boye et al., 2005; Kondo et al., 2008). Excess ligand concentrations in these experiments, however, typically decreased as Fe additions saturated ligands, and some of the increase in [Li] may reflect an artifact in interpretation. At the conclusion of the SEEDS II experiment in the NW Pacific, excess ligand concentrations increased, predominantly in the soluble fraction, as the stimulated bloom declined (Kondo et al., 2008). Shipboard microzooplankton grazing experiments conducted during the SEEDS II experiment demonstrated an increase in ligand concentrations proportional to the chlorophyll consumed by added grazers (Sato et al., 2007).
In environments high in Fe, dFe concentrations may meet or exceed measured ligand concentrations. Examples of this, where [dFe] > [LT], have been reported in hydrothermal vent plumes (Bennett et al., 2008), artificial Fe-enrichment experiments (Rue and Bruland, 1997; Boye et al., 2005; Kondo et al., 2008), and Fe-rich coastal shelf environments (Croot and Johansson, 2000; Gobler et al., 2002; Powell and Wilson-Finelli, 2003a; Buck and Bruland, 2007; Buck et al., 2007; Kondo et al., 2007; Batchelli et al., 2010). In the Eastern Tropical North Pacific suboxic zone, dFe concentrations also approach dissolved ligand concentrations, resulting in diminished excess ligand concentrations compared to surrounding oxygenated waters (Hopkinson and Barbeau, 2007). The conditional stability constant of the ligand measured in the suboxic zone was also slightly stronger than the ligands measured in the oxic waters above (Hopkinson and Barbeau, 2007). In the suboxic zone of the Arabian sea, on the other hand, excess ligand concentrations were much greater than in the oxic waters, with a similar or slightly lower conditional stability constant of these suboxic zone ligands (Witter et al., 2000).
Links between Biological Activity and Ligand Concentrations
Previous reviews have emphasized the important interplay between biological activity and Fe-binding ligand cycling (Bruland and Rue, 2001; Hirose, 2006, 2007; Hunter and Boyd, 2007; Boyd and Ellwood, 2010). There are multiple lines of evidence in support of a biological source of Fe-binding ligands in the marine environment. As mentioned above, field studies have commonly found highest excess ligand concentrations within and around the biomass maximum in the water column (Rue and Bruland, 1995; van den Berg, 1995, 2006; Boye et al., 2001, 2006; Croot et al., 2004; Gerringa et al., 2006, 2008; Tian et al., 2006; Buck and Bruland, 2007; Wagener et al., 2008; Ibisanmi et al., 2011; Mohamed et al., 2011). Excess ligand concentrations have been observed to show an annual cycle (Wagener et al., 2008), with increases in excess ligand concentrations observed during the most productive periods. Surface transects also show elevated excess ligand concentrations with high productivity in Fe-depleted waters (Boye et al., 2003; Gerringa et al., 2006; Tian et al., 2006; Buck and Bruland, 2007). The particularly strong Fe-binding ligand class, detected only in the upper water column (Rue and Bruland, 1995, 1997; Cullen et al., 2006; Ibisanmi et al., 2011), presents the same class of log K values (log K > 12) as siderophore-type ligands measured by CLE–ACSV (Rue and Bruland, 1995; Witter et al., 2000; Buck et al., 2010; Poorvin et al., 2011).
Recent incubations of natural surface seawater have shown active production of Fe-binding ligands concomitant with diatom growth under Fe-limiting conditions (Buck et al., 2010; King et al., 2012). In these incubations, ligand production was only observed in the unamended bottles, Fe-amended bottles presented much higher growth but no ligand production, suggesting that the Fe-limitation status of the diatoms in the unamended bottles was related to the ligand production observed (Buck et al., 2010; King et al., 2012). In the field, Gerringa et al. (2006) found that 63% of the variability in ligand concentrations in profiles from the Canary Basin was explained by changes in phytoplankton biomass and silicic acid concentrations, indicating a correlation between diatom growth and Fe-binding ligand concentrations. Recent incubation studies have furthermore shown an increase in Fe bioavailability due to redox speciation changes specifically in diatom cultures (Rijkenberg et al., 2008a). In combination, these studies suggest a connection between Fe-stressed diatom communities and Fe-binding ligand concentrations that deserves further attention.
The passive production of Fe-binding ligands from grazing and bacterial remineralization of organic matter is another important biological source of ligands. HS, degradation products of terrestrial and marine organic matter, may be a substantial component of the Fe-binding ligand pool in some coastal and deep ocean waters (Laglera and van den Berg, 2009). A recent study of coastal estuarine waters found that Fe was strongly but reversibly complexed with HS, predominantly in the colloidal fraction (Batchelli et al., 2010).
Increased excess ligand concentrations, largely in the soluble size fraction, were reported during the bloom decline of the SEEDS II experiment (Kondo et al., 2008). Shipboard grazer experiments, also conducted during SEEDS II, showed that dFe and ligand concentrations increased as chlorophyll biomass was consumed (Sato et al., 2007). The highest increases in ligand concentrations, normalized to biomass consumption, were measured in the copepod grazing experiments (Sato et al., 2007). The ligands produced in these grazer experiments were similar to the L1 type ligands and were found to be more bioavailable to diatom species than to picoplankton in the incubations (Sato et al., 2007).
The ability of ligands to solubilize natural Fe sources may also be linked to biological productivity. An annual cycle in the dissolution of Fe from Saharan dust has been observed in water sampled at different times of the year from the Mediterranean (Wagener et al., 2008), with dust derived Fe being less soluble in water sampled during the winter period (Dec–Feb). This was linked to a change in excess ligand concentration, and a potential change in ligand characteristics (although stability constants were not reported in the study), as Fe dissolution could not be determined in the winter, despite the presence of excess ligands (Wagener et al., 2008).
Bacterial remineralization of sinking biogenic particles, on the other hand, produces weaker Fe-binding ligands ( or lower) concomitant with dFe release (Boyd et al., 2010). Viral lysis of cells in grazing experiments has also recently been shown to produce similarly weak Fe-binding ligands (Poorvin et al., 2011). The weakly complexed Fe released by these passive grazing processes (Boyd et al., 2010; Poorvin et al., 2011) may be more biologically available to the phytoplankton community than the strongly complexed siderophore-bound Fe (Poorvin et al., 2011).
The Chemical Characterization of the Dissolved Iron Ligand Pool
Since the first evidence for Fe complexation by natural organic ligands in seawater was presented (Gledhill and van den Berg, 1994; Rue and Bruland, 1995; van den Berg, 1995; Wu and Luther, 1995), the question of the identity, chemical structure, and source of Fe-binding organic ligands in the oceans has fueled research across disciplines. While we have learned a great deal about Fe-binding ligands, it is still not possible to give definitive answers to these questions some 17 years later. Initially, the strength and concentration of the ligands measured by competitive equilibrium techniques indicated that ambient ligands had a high affinity for Fe (Gledhill and van den Berg, 1994; Rue and Bruland, 1995; van den Berg, 1995; Wu and Luther, 1995), while, as had been highlighted above, many profiles of ligand distributions in the ocean point to an autochthonous biological source. Determination of the conditional stability constants of potential ligand types produced by phytoplankton and bacteria indicated that some of these ligand types had very similar binding strengths in seawater to the detected natural ligands (Rue and Bruland, 1995; Witter et al., 2000; Macrellis et al., 2001). Hence these biologically produced ligand types, which may include compounds like siderophores and porphyrins, were hypothesized to make up a major part of the ligand pool. However, characterization of Fe ligands in seawater is a considerable challenge because the ligands are of unknown composition, are likely complex in chemical nature and are present at very low concentrations in a matrix of high ionic strength. The recent progress made in this field has been greatly facilitated by improvements in the sensitivity, mass accuracy and robustness of both inorganic and organic mass spectrometers. Such improvements have resulted in the detection of specific iron organic complexes such as siderophores by HPLC–ESI-MS (McCormack et al., 2003; Gledhill et al., 2004; Mawji et al., 2008a, 2011; Velasquez et al., 2011) and the detection of Fe associated with more complex organic fractions by FFFF–ICP-MS (Stolpe and Hassellov, 2010; Stolpe et al., 2010; Baalousha et al., 2011).
Small, Defined Organic Ligands
Siderophores are compounds produced by bacteria in order to sequester Fe from their environment (Hider and Kong, 2010). Siderophores are known to be produced by a wide variety of bacteria, including marine bacteria (Amin et al., 2009; Cabaj and Kosakowska, 2009; Vraspir and Butler, 2009). Bacteria appear to be able to produce families of different but related siderophores (Martinez et al., 2000, 2003; Ito and Butler, 2005; Martinez and Butler, 2007; Homann et al., 2009a,b), but uptake of siderophores by bacteria is not necessarily specific (Stintzi et al., 2000), so that a bacterial species may be able to acquire siderophores produced by other species. There has been much progress in the characterization of specific siderophores produced by marine bacteria (Amin et al., 2009; Vraspir and Butler, 2009), in particular by the A. Butler research group at the University of California in Santa Barbara.
Relatively large quantities of isolated siderophore are currently required for a complete description of chemical structure so that it is only possible to fully characterize siderophores in bacteria that can be cultured in the laboratory. In addition, the time and effort required also imposes restrictions on the number of siderophores that can be fully characterized. Characterized siderophores are, thus, likely to represent only a fraction of the potential siderophore pool. Marine siderophores produced in laboratory cultures have been found to contain all the major siderophore chelating groups of hydroxamate, catecholate, and carboxylate functional groups (Vraspir and Butler, 2009). To date, the majority of marine siderophores characterized contain mixed ligand carboxylate groups, and many of these characterized marine siderophores have fatty acid tails attached to the chelating head (Martinez et al., 2000, 2003; Xu et al., 2002; Owen et al., 2005; Martin et al., 2006; Martinez and Butler, 2007; Homann et al., 2009a,b). Such fatty acid tails are likely to strongly influence siderophore biogeochemistry, affecting siderophore partitioning between dissolved and particulate phases and perhaps mitigating against diffusive losses of siderophores from the bacterial cell (Xu et al., 2002; Martinez and Butler, 2007).
Progress has also been made in the detection and characterization of siderophores in natural seawater itself (Gledhill et al., 2004; Mawji et al., 2008a, 2011; Velasquez et al., 2011; Table 2; Figure 3). The first reports of dissolved siderophores in seawater were made by Kosakowska et al. (Kosakowska et al., 1999; Mucha et al., 1999) using capillary electrophoresis to detect hydroxamate siderophores in the Baltic Sea. Macrellis et al. (2001) used siderophore assays to report the presence of siderophore like functional groups in coastal NE Pacific upwelling waters. More recently, chromatographic techniques have been coupled to ESI or ICP-MS in order to either characterize or quantify specific siderophores in seawater (Mawji et al., 2008a; Velasquez et al., 2011; Table 2). Separation techniques coupled to mass spectrometry show much promise for both the identification and quantification of specific metal complexes like with siderophores, largely as a result of the high sensitivity of these techniques, which are capable of detecting in the picomolar–nanomolar range. Mass spectrometry has been used to detect unknown and known siderophores in the Southern Ocean and in the Atlantic Ocean (Mawji et al., 2008a; Velasquez et al., 2011). In the Southern Ocean, unidentified siderophore-type complexes were detected with the molecular masses varying from station to station (Velasquez et al., 2011). In the Atlantic Ocean, ferrioxamine type siderophores were detected ubiquitously and were found to be present at concentrations of upto 20 pM, and to make up between 0.5 and 5% of the total dissolved Fe pool (Mawji et al., 2008a). The somewhat sparse data so far obtained indicates that siderophore distributions may be linked to bacterial abundance, but otherwise gives little clue as to how siderophore concentrations might vary with, for example, depth. It is clear, however, that the presence of marine siderophores is not limited to low dFe waters.
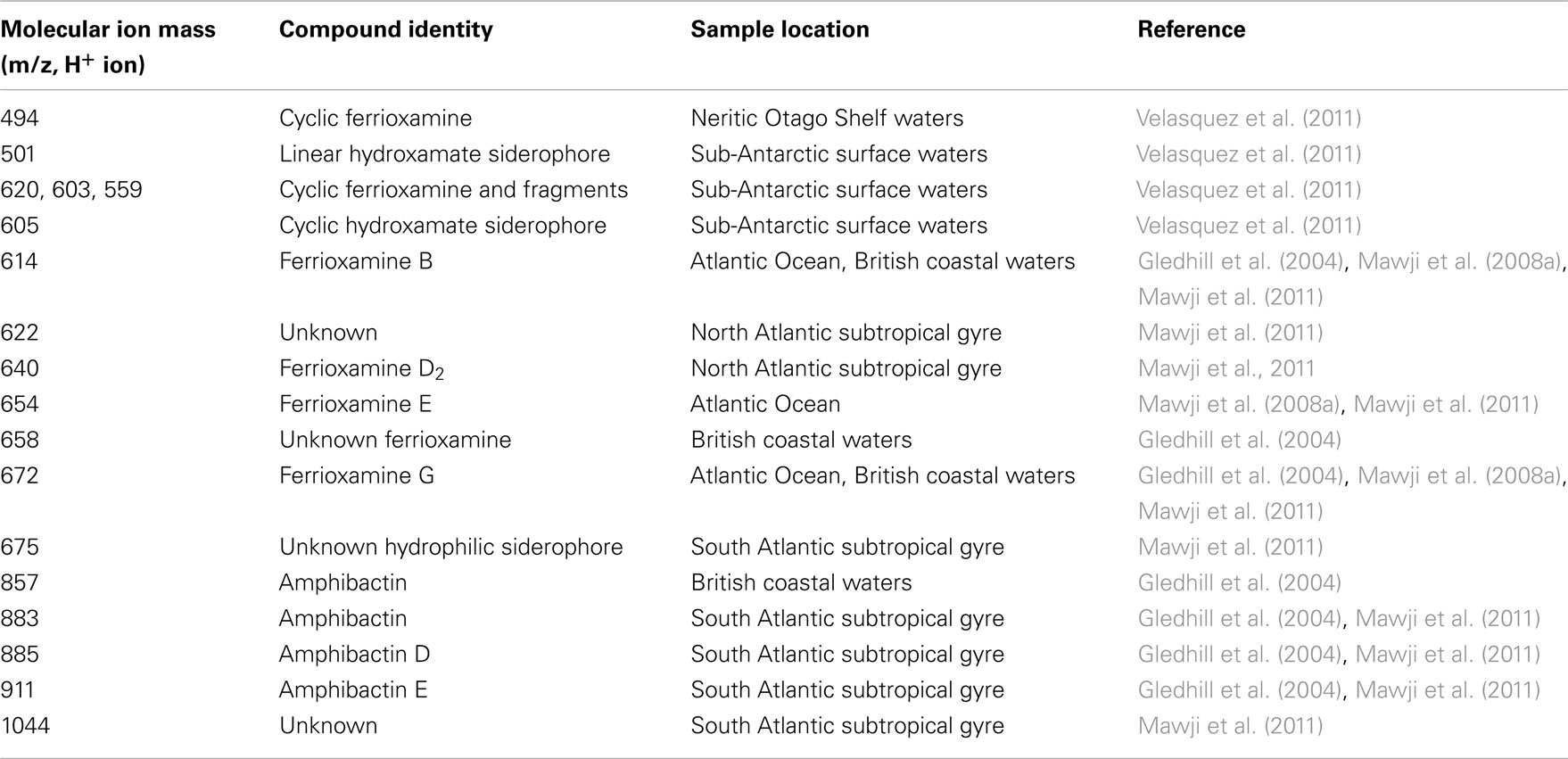
Table 2. Siderophores identified in seawater or natural seawater incubations by high performance liquid chromatography – electrospray ionization mass spectrometry.
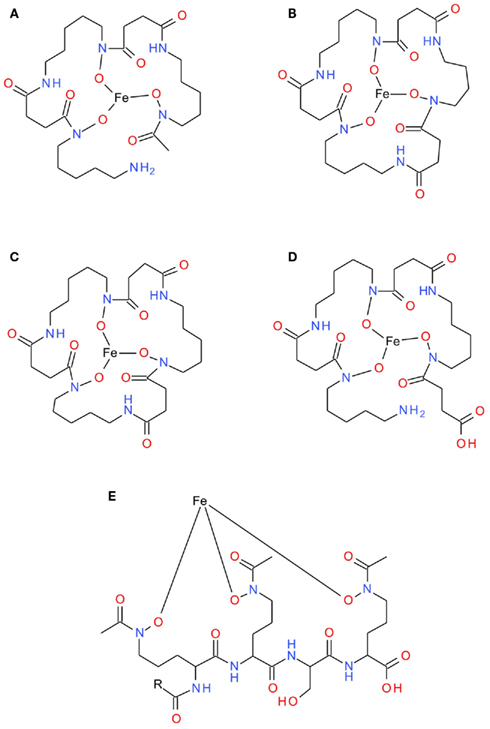
Figure 3. Structures of fully characterized siderophores that have been identified in seawater or natural seawater incubations. (A) Ferrioxamine B, (B) Ferrioxamine D2, (C) Ferrioxamine E, (D) Ferrioxamine G, and (E) Amphibactins D (R = C13H27) and E (R = C15H29).
It is notable that, while the available data is limited, the siderophores detected in seawater to date are all hydroxamates, with no reports of any identifiable siderophores containing carboxylate or catecholate functional groups being recovered from the dissolved phase in seawater. The lack of detected carboxylate or catecholate type siderophores indicates that either these siderophore types are not present in the dissolved phase, or more likely, that the analytical techniques used to detect these compounds are biased toward the detection of hydroxamate siderophores. Current methods to detect siderophores in seawater rely on a preconcentration step, necessary in order to both remove interfering matrices, and to increase siderophore concentrations to detectable ranges (Mucha et al., 1999; Mawji et al., 2008a; Velasquez et al., 2011). Such preconcentration techniques introduce bias and probably restrict the types of siderophores that can be detected. For example, at the natural pH of seawater (∼8), both carboxylate and catecholate type Fe–siderophore complexes will be deprotonated and negatively charged (Harris et al., 1979a; Loomis and Raymond, 1991). Thus, the preconcentration efficiency of catecholate and carboxylate siderophore types onto the commonly used hydrophobic resins (e.g., C18, polystyrene divinyl benzene, XAD) will be reduced. Acidification of the sample prior to preconcentration (Mucha et al., 1999; Velasquez et al., 2011), in order to neutralize negatively charged complexed siderophores and thus make them more hydrophobic may also result in siderophore hydrolysis or precipitation (Harris et al., 1979b; Loomis and Raymond, 1991). Changes in sample pH during analysis are also common, with chromatographic separations performed at low pH (McCormack et al., 2003; Velasquez et al., 2011). However, catecholate type siderophore–Fe complexes undergo hydrolysis at low pH and are not detectable when either the sample pH is low or the chromatographic conditions employ low pH eluants (Loomis and Raymond, 1991; Gledhill, unpublished data). Consequently, further work is necessary in order to develop robust preconcentration and analysis techniques applicable to a wider variety of siderophore types.
Identification of siderophore complexes in mass spectra takes place via the utilization of distinctive isotopic ratios endowed upon the complex by the metal ion (McCormack et al., 2003; Velasquez et al., 2011). Two strategies are currently employed; the first involves complexation of the siderophore with gallium, which has a very identifiable isotopic ratio for 69Ga:71Ga of 3:2 and allows for several mechanisms of checking against false positives, increasing the robustness of the analysis (McCormack et al., 2003; Mawji et al., 2011). Such checks include analysis of the original sample for the presence of the Fe-complexed or apo- (metal free) siderophore and analysis by ICP-MS in order to check that gallium is indeed present at the expected relative retention time (Mawji et al., 2011). The gallium exchange method suffers from disadvantages in that it involves reduction of the sample pH in order to keep gallium in solution and, thus, is likely to result in losses of chemically unstable siderophores (see above).
A second promising technique recently applied to the identification of siderophores in seawater is based on the distinctive isotopic ratio endowed on a molecule by Fe (Velasquez et al., 2011). The natural abundance of the 54Fe isotope is approximately 5.6% of the 56Fe isotope. When incorporated into an organic complex, the combination of the Fe and carbon isotopic abundances increases the abundance of the lighter molecular ion so that ferrioxamine B will have expected isotopic ratios of 6.4:100:32.4 for m/z (M + H+) 612, 614, and 617. A key component of this technique is the ability to detect the putative 54Fe containing molecular ion and then confirm it otherwise has the same structure as the 56Fe containing molecular ion. This has been shown to be possible with a nano-HPLC coupled to a high resolution mass spectrometer (Velasquez et al., 2011). Unfortunately, the background noise in lower resolution instruments working with higher flow rates may make it difficult to identify the 54Fe isotope, as it is present at quite a low relative abundance in MS spectra. However, even in lower resolution instruments fragment ions obtained on collision induced dissociation of pseudo molecular ions can be used to provide evidence for Fe complexes (Velasquez et al., 2011) as Fe is strongly retained in fragment ions (Mawji et al., 2008b; Velasquez et al., 2011). In theory, Fe could also be determined in parallel by ICP-MS in analogy to the gallium technique in order to confirm the presence of the metal at the expected retention time, although more care would have to be taken with respect to contamination. Furthermore the determination of Fe by ICP-MS can be more difficult due to isobaric interferences (Mawji et al., 2008a, 2011).
The limited number of reports on siderophore concentrations in the dissolved phase in the ocean indicates that the concentrations of individual siderophores are likely to be quite low, in the picomolar range (Mawji et al., 2008a). However, as has been pointed out above, while the pool of potential siderophores is apparently quite large (e.g., Amin et al., 2009; Cabaj and Kosakowska, 2009; Vraspir and Butler, 2009), it is likely that only a limited number of siderophores in seawater are detectable using the currently applied methods. Thus, although it is now clear that siderophores can be present in the open ocean, it is not possible at this stage to say what proportion of the dissolved ligand pool is made up of siderophores.
Porphyrins (molecular weight 600–1000 Da) are another biologically produced class of compounds that have been suggested as potential Fe ligands (Witter et al., 2000; Hunter and Boyd, 2007). Porphyrins function as prosthetic groups in proteins and are useful for their ability to absorb light, transfer electrons, and bind oxygen (Mochizuki et al., 2010). Porphyrins, which include chlorophylls, chlorophyll breakdown products like phaeophytin, hemes, and vitamin B12, are produced by almost all living organisms via a well conserved tetrapyrrole biosynthesis pathway (Mochizuki et al., 2010). The biological production of these compounds is tightly controlled, as they cause oxidative stress if present in excess of their proteins (Mochizuki et al., 2010). Hemes have been detected at picomolar concentrations in particulate material (Gledhill et al., under review) and heme like compounds have been detected in the dissolved fraction at nanomolar concentrations in river and estuarine waters (Vong et al., 2007). However, given that porphyrins have a very low solubility in water at pH 8 and more recent work has found little evidence to show that Fe(III) spontaneously complexes with porphyrins in solution (Rijkenberg et al., 2006a; Schlosser and Croot, 2008) it remains questionable as to whether porphyrins form a part of the dissolved ligand pool.
Domoic acid (DA), an algal toxin produced by Pseudo-nitzschia spp., has also been shown to complex Fe (Rue and Bruland, 2001), although with a weak stability constant that is unlikely to make it an effective competitor in the ocean. However, there is evidence that DA, through its ability to complex dissolved Cu, may facilitate the high affinity Fe uptake from strongly complexed Fe by diatoms in Fe-limited environments (Wells et al., 2005), regions where DA levels can be elevated (Silver et al., 2010). Thus, while possibly not a significant component of the Fe-binding ligand pool, DA may nonetheless play an important role in the biogeochemical cycling of Fe, particularly in Fe-limited environments.
Larger, Less Well-Defined Organic Ligands
Recent evidence has indicated that more diffuse, less well-defined organic compounds such as HS and exopolysaccharides (EPS) may contribute to the Fe ligand pool (Batchelli et al., 2009, 2010; Laglera and van den Berg, 2009; Stolpe and Hassellov, 2010; Stolpe et al., 2010; Hassler et al., 2011a,b). Laglera and van den Berg recently reported an electrochemical technique that allows for the direct detection of humic like substances in seawater by ACSV (Laglera et al., 2007; Laglera and van den Berg, 2009). The technique determines the catalytically enhanced reduction current produced by Fe bound to HS, and is standardized using the Suwannee River fulvic acid (SRFA) standard (Laglera et al., 2007). Determination of the conditional stability constants and binding capacities of these putative humic like substances by competition with ethylene-diaminetetraacetic acid (EDTA) has shown that these ligands have similar stability constants to the natural organic ligands measured by CLE–ACSV (Laglera and van den Berg, 2009). Apparent binding capacities for these humic like substances in seawater are also similar to those determined by CLE–ACSV (Laglera and van den Berg, 2009), and it has been suggested that HS can account for the majority of the total ligand pool in coastal and deep waters (Batchelli et al., 2009, 2010; Laglera and van den Berg, 2009). The coincidence of similar reduction potentials and binding capacities for the detected ambient Fe ligand, SRFA, and Suwannee River humic acid (SRHA) make it tempting to identify the ambient ligand detected using the technique as a humic like substance (Laglera et al., 2007). However, the technique is not specific to HS, as EPS will also become electrochemically active upon addition of Fe and are, thus, indistinguishable from HS by ACSV (Hassler et al., 2011b). Further support for identification of a ligand fraction as HS is, however, provided by FFFF. Studies in coastal waters using FFF–ICP-MS and FFFF coupled to UV and fluorescence detectors have indicated that dFe in coastal waters is associated with fluorescent, colored, dissolved humic like organic matter (Stolpe and Hassellov, 2010; Stolpe et al., 2010), and that, furthermore, freshly added Fe readily associates with this fraction (Stolpe et al., 2010).
By their very nature, both HS and EPS are challenging to chemically characterize. Both HS and EPS are oxygen rich – the SRFA used in Laglera and van den Berg (2009) consists of 44% oxygen (% w/w, http://www.ihss.gatech.edu/elements.html), while EPS are predominantly composed of neutral sugars and contain a significant fraction of acidic polysaccharides like uronic acids (Mancuso Nichols et al., 2004). Uronic acids are known to complex Fe (Gyurcsik and Nagy, 2000), with complexes being more stable at high pH (Gyurcsik and Nagy, 2000), as observed for Fe–HS complexes (Laglera et al., 2007). Classical pH-metric determinations of stability constants for simple acidic polysaccharide-Fe complexes show that they are low (log β 3–4; Gyurcsik and Nagy, 2000) and Fe complexation of model polysaccharides carrageenan, laminarin, and alginic acid in seawater has been found to be undetectable by CLE–ACSV (Strmecki et al., 2010), although this may have been an artifact of the selected AL (Laglera et al., 2011). In other studies, EPS from bacteria have been observed to be associated with Fe (Hassler et al., 2011a,b) and EPS has been shown to reduce the lability of Fe to other Fe ligands while enhancing Fe availability to phytoplankton when compared to Fe–siderophore complexes (Hassler et al., 2011b).
In addition to an Fe–HS fraction, Stolpe and Hassellov (2010) also observed an association of Fe with a higher molecular weight organic fraction described as nanofibrils, particularly at a time of enhanced productivity (Stolpe and Hassellov, 2010). The nanofibrils were consistent in size and appearance to transparent exopolymer (TEP) like compounds (Stolpe and Hassellov, 2010). However, although Fe present in the samples was found to co-elute with the nanofibril fraction, freshly added Fe did not associate with the nanofibrils. Stolpe and Hassellov (2010) suggested that this behavior could be explained as an inorganic Fe-nanoparticle association (Stolpe and Hassellov, 2010), although it could also be a reflection of under saturation of binding sites in the HS fraction, coupled to a weaker affinity between Fe and the larger nanofibrils.
Approaching an Overview of Iron Complexation and Physico-Chemical Speciation in Seawater
Several types of Fe organic complexes and associations have now been shown to exist in seawater, ranging from high affinity siderophores present at low concentrations, to weaker but more abundant HS and EPS associations, and likely also including associations between inorganic Fe nanoparticles and larger TEP like organic macromolecules (Figure 4). These organic complexes and associations are currently best distinguished by size fractionation, as they generally fall along an increasing size spectrum from siderophores to macromolecules. Often coupled to this increasing size spectrum is a decreasing thermodynamic stability of the complexes as measured by CLE–ACSV, but increasing kinetic inertness, leading to an overall potential decrease in biological availability. It is, therefore, becoming possible to unravel the different biogeochemical behaviors of the many components of the dFe pool.
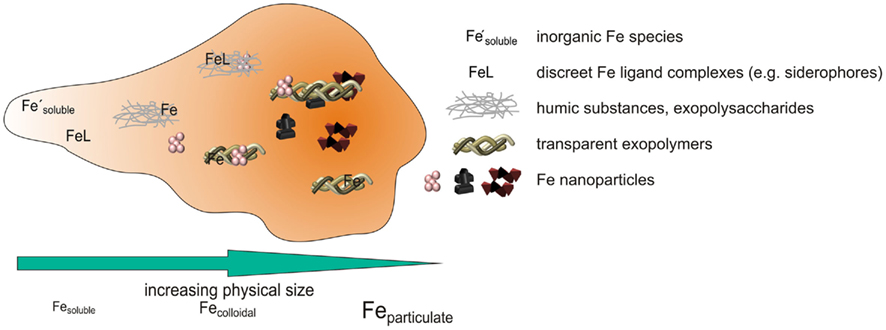
Figure 4. Schematic figure illustrating potential components of the dFe pool so far identified in seawater. Decreasing kinetic lability of Fe within the components is represented by deeper orange background shading.
Siderophores are associated with bacterial productivity and may only be produced in significant quantities when readily available carbon is abundant (Mawji et al., 2011). Siderophores maybe important in regions where organic carbon concentrations are high enough to support significant bacterial productivity, but where dFe is not readily available. The photochemical lability of siderophores suggests that many siderophore types will not persist in the water column, although their breakdown products may be more stable and form weaker, more bioavailable, Fe complexes (Barbeau et al., 2001, 2003). Siderophores may play an important role in increasing the availability of Fe for particular types of bacteria, influencing bacterial diversity and productivity in the ocean. Siderophores may also play a role in the solubilization of particulate and colloidal Fe. Given their origin, function and reactivity, siderophores are anticipated to contribute to Fe-binding ligand pools primarily in the upper water column, and especially in the truly soluble size fraction of ligands, although siderophores may also be associated with colloidal and particulate fractions as well.
Field studies of Fe-binding ligand distributions consistently report higher excess ligand concentrations in the upper water column, and these maxima in eLi are often, though not always, associated with elevated fluorescence and phytoplankton biomass. When size fractionation is assessed, these excess ligand concentrations also appear to be predominantly in the soluble size fraction. In combination, field studies support a siderophore-type origin of ambient Fe-binding ligands in the upper oceans. However, siderophores extracted from natural waters to date have been found in only the picomolar range, while ambient strong Fe-binding ligands are measured by CLE–ACSV in the nanomolar range. Recent incubation studies of natural surface seawater have shown strong Fe-binding ligand production, also measured by CLE–ACSV in the nanomolar range, under conditions of both Fe-limited phytoplankton growth and stimulated bloom decay (Sato et al., 2007; Buck et al., 2010). Furthermore, the thermodynamic stability constants (log K′) of some known Fe–siderophore complexes are too high to detect with usual CLE–ACSV approaches. Altogether, this would suggest that, in addition to siderophores, other strong Fe-binding ligand types are also likely to contribute to the Fe-binding ligand pool measured by CLE–ACSV in surface waters.
Humic substances and EPS may be either terrestrial or autochthonous in nature, and are typically found in the colloidal fraction. It appears that the HS fraction is quite refractory and persists into the deep ocean while EPS is likely to be produced in surface waters as it is, by definition, associated with phytoplankton productivity. Fe bound to model humic acids and Fe bound to EPS have been shown to be as available to phytoplankton as inorganic Fe (Chen and Wang, 2008; Hassler et al., 2011b). However, both EPS and HS are complex molecules that remain poorly defined; greater effort is required in order to characterize the association between Fe, HS, and EPS, and the contribution of HS and EPS to the ambient ligand pool in the open ocean. It is apparent that current techniques may not distinguish particularly well between HS and EPS, yet the biogeochemistry of these two organic fractions is potentially quite different.
The biological availability of colloidal Fe is rather hard to define as it is probably highly dependent on the chemical nature of the colloids (Kuma and Matsunaga, 1995; Yoshida et al., 2006), as well as the capabilities of organisms present to extract Fe from the colloids, whether by reduction (Maldonado and Price, 2001; Rijkenberg et al., 2008a) or by dissolution via a high affinity uptake mechanism (Vraspir and Butler, 2009). The biological availability of some colloids, like Fe nanoparticles, is poorly understood. Colloidal Fe biogeochemistry is also likely to be strongly influenced by temperature, another consideration that has yet to be investigated fully. However, it is becoming apparent that Fe biogeochemistry in the open ocean may be strongly influenced by the stability of the colloidal fraction. While it has been suggested that organic Fe colloids formed in surface waters are scavenged quite rapidly through the water column (Wu and Luther, 1994; Nishioka et al., 2001; Wu et al., 2001; Cullen et al., 2006; Bergquist et al., 2007; Hurst and Bruland, 2008; Kondo et al., 2008; Schlosser and Croot, 2008; Boye et al., 2010; Thuroczy et al., 2010), stable Fe colloids from continental shelves have also been implicated in the long range transport of Fe to the open ocean (Elrod et al., 2004; Lam and Bishop, 2008). More information is, thus, needed on the nature of Fe colloids in open ocean surface and continental shelf waters in order to understand more fully these potentially contrasting behaviors. Investigation of an organic Fe colloid-like fraction in future global ocean models incorporating Fe speciation would also allow the role of this fraction in the Fe biogeochemical cycle to be further examined.
Taken together, the evidence described above points toward the existence of a spectrum of Fe ligands in seawater, and we found that the different experimental approaches employed by Fe biogeochemists appear to be converging toward a consistent overview. Increases in the size and complexity of the Fe species appear to be accompanied by a weakening of the thermodynamic stability of the Fe complex. The weaker complexation between Fe′ and the larger sized organic macromolecules is, however, potentially mitigated by the existence of associations between inert Fe nanoparticles and nanofibrils like TEP, resulting in an apparently Fe saturated inert colloidal ligand pool. Substantial progress has been made in trace metal clean size fractionation, CLE–ACSV, and HPLC–ESI-MS techniques over the last decade. We anticipate that future studies will increasingly couple these techniques, along with incubations and other biological process studies, providing much needed insight on the nature and cycling of Fe-binding ligands in the oceans. Finally, we conclude that the biogeochemical role of each of the organic ligand fractions appears to be very different and deserves further investigation, as this spectrum of organic Fe-binding ligands overwhelmingly complexes dFe in the marine environment.
Conflict of Interest Statement
The authors declare that the research was conducted in the absence of any commercial or financial relationships that could be construed as a potential conflict of interest.
Acknowledgments
Gledhill was supported by UK – NERC Advanced Fellowship (NE/E013546/1) and contributed to the manuscript whilst a visiting scientist at the Royal Netherlands Institute of Sea Research, Texel, The Netherlands. Buck was supported by institutional funding from the G. Unger Vetlesen Foundation at the Bermuda Institute of Ocean Sciences (BIOS). This manuscript constitutes BIOS contribution number 2017 for co-author Buck.
References
Amin, S. A., Green, D. H., Hart, M. C., Kupper, F. C., Sunda, W. G., and Carrano, C. J. (2009). Photolysis of iron-siderophore chelates promotes bacterial-algal mutualism. Proc. Natl. Acad. Sci. U.S.A. 106, 17071–17076.
Archer, D. E., and Johnson, K. (2000). A model of the iron cycle in the ocean. Global Biogeochem. Cycles 14, 269–279.
Baalousha, M., Stolpe, B., and Lead, J. R. (2011). Flow field-flow fractionation for the analysis and characterization of natural colloids and manufactured nanoparticles in environmental systems: a critical review. J. Chromatogr. A 1218, 4078–4103.
Barbeau, K., Rue, E. L., Trick, C. G., Bruland, K. T., and Butler, A. (2003). Photochemical reactivity of siderophores produced by marine heterotrophic bacteria and cyanobacteria based on characteristic Fe(III) binding groups. Limnol. Oceanogr. 48, 1069–1078.
Barbeau, K. A., Rue, E. L., Bruland, K. W., and Butler, A. (2001). Photochemical cycling of iron in the surface ocean mediated by microbial iron(III)-binding ligands. Nature 413, 409–413.
Batchelli, S., Muller, F. L. L., Baalousha, M., and Lead, J. R. (2009). Size fractionation and optical properties of colloids in an organic-rich estuary (Thurso, UK). Mar. Chem. 113, 227–237.
Batchelli, S., Muller, F. L. L., Chang, K. C., and Lee, C. L. (2010). Evidence for strong but dynamic iron-humic colloidal associations in humic-rich coastal waters. Environ. Sci. Technol. 44, 8485–8490.
Bennett, S. A., Achterberg, E. P., Connelly, D. P., Statharn, P. J., Fones, G. R., and Gernian, C. R. (2008). The distribution and stabilisation of dissolved Fe in deep-sea hydrothermal plumes. Earth Planet. Sci. Lett. 270, 157–167.
Bergquist, B. A., Wu, J., and Boyle, E. A. (2007). Variability in oceanic dissolved iron is dominated by the colloidal fraction. Geochim. Cosmochim. Acta 71, 2960–2974.
Bligh, M. W., and Waite, T. D. (2010). Role of heterogeneous precipitation in determining the nature of products formed on oxidation of Fe(II) in seawater containing natural organic matter. Environ. Sci. Technol. 44, 6667–6673.
Boyd, P. W., and Ellwood, M. J. (2010). The biogeochemical cycle of iron in the ocean. Nat. Geosci. 3, 675–682.
Boyd, P. W., Ibisanmi, E., Sander, S. G., Hunter, K. A., and Jackson, G. A. (2010). Remineralization of upper ocean particles: implications for iron biogeochemistry. Limnol. Oceanogr. 55, 1271–1288.
Boyd, P. W., Jickells, T., Law, C. S., Blain, S., Boyle, E. A., Buesseler, K. O., Coale, K. H., Cullen, J. J., De Baar, H. J. W., Follows, M., Harvey, M., Lancelot, C., Levasseur, M., Owens, N. P. J., Pollard, R., Rivkin, R. B., Sarmiento, J., Schoemann, V., Smetacek, V., Takeda, S., Tsuda, A., Turner, S., and Watson, A. J. (2007). Mesoscale iron enrichment experiments 1993-2005: synthesis and future directions. Science 315, 612–617.
Boye, M., Aldrich, A., Van Den Berg, C. M. G., De Jong, J. T. M., Nirmaier, H., Veldhuis, M., Timmermans, K. R., and De Baar, H. J. W. (2006). The chemical speciation of iron in the north-east Atlantic Ocean. Deep Sea Res. Part I Oceanogr. Res. Pap. 53, 667–683.
Boye, M., Nishioka, J., Croot, P., Laan, P., Timmermans, K. R., Strass, V. H., Takeda, S., and De Baar, H. J. W. (2010). Significant portion of dissolved organic Fe complexes in fact is Fe colloids. Mar. Chem. 122, 20–27.
Boye, M., Nishioka, J., Croot, P. L., Laan, P., Timmermans, K. R., and De Baar, H. J. W. (2005). Major deviations of iron complexation during 22 days of a mesoscale iron enrichment in the open Southern Ocean. Mar. Chem. 96, 257–271.
Boye, M., Van Den Berg, C. M. G., De Jong, J. T. M., Leach, H., Croot, P., and De Baar, H. J. W. (2001). Organic complexation of iron in the Southern Ocean. Deep Sea Res. Part I Oceanogr. Res. Pap. 48, 1477–1497.
Boye, M. B., Aldrich, A. P., Van Den Berg, C. M. G., De Jong, J. T. M., Veldhuis, M. J. W., and De Baar, H. J. W. (2003). Horizontal gradient of the chemical speciation of iron in surface waters of N.E. Atlantic Ocean. Mar. Chem. 80, 129–143.
Bruland, K. W., Rue, E. L., Donat, J. R., Skrabal, S. A., and Moffett, J. W. (2000). Intercomparison of voltammetric techniques to determine the chemical speciation of dissolved copper in a coastal seawater sample. Anal. Chim. Acta 405, 99–113.
Bruland, K. W., and Rue, E. L. (2001). “Analytical methods for the determination of concentrations and speciation of iron,” in The Biogeochemistry of Iron in Seawater, eds D. R. Turner and K. A. Hunter (Chichester: Wiley), 255–290.
Buck, K. N., and Bruland, K. W. (2007). The physicochemical speciation of dissolved iron in the Bering Sea, Alaska. Limnol. Oceanogr. 52, 1800–1808.
Buck, K. N., Lohan, M. C., Berger, C. J. M., and Bruland, K. W. (2007). Dissolved iron speciation in two distinct river plumes and an estuary: implications for riverine iron supply. Limnol. Oceanogr. 52, 843–855.
Buck, K. N., Selph, K. E., and Barbeau, K. A. (2010). Iron-binding ligand production and copper speciation in an incubation experiment of Antarctic Peninsula shelf waters from the Bransfield Strait, Southern Ocean. Mar. Chem. 122, 148–159.
Byrne, R. H., Kump, L. R., and Cantrell, K. J. (1988). The influence of temperature and pH on trace metal speciation in seawater. Mar. Chem. 25, 163–181.
Cabaj, A., and Kosakowska, A. (2009). Iron-dependent growth of and siderophore production by two heterotrophic bacteria isolated from brackish water of the southern Baltic Sea. Microbiol. Res. 164, 570–577.
Chen, M., and Wang, W. X. (2008). Accelerated uptake by phytoplankton of iron bound to humic acids. Aquat. Biol. 3, 155–166.
Croot, P. L., Andersson, K., Öztürk, M., and Turner, D. R. (2004). The distribution and speciation of iron along 6°E in the Southern Ocean. Deep Sea Res. Part II Top. Stud. Oceanogr. 51, 2857–2879.
Croot, P. L., and Johansson, M. (2000). Determination of iron speciation by cathodic stripping voltammetry in seawater using the competing ligand 2-(2-thiazolylazo)-p-cresol (TAC). Electroanalysis 12, 565–576.
Croot, P. L., Laan, P., Nishioka, J., Strass, V., Cisewski, B., Boye, M., Timmermans, K. R., Bellerby, R. G., Goldson, L., Nightingale, P., and De Baar, H. J. W. (2005). Spatial and temporal distribution of Fe(II) and H2O2 during EisenEx, an open ocean mescoscale iron enrichment. Mar. Chem. 95, 65–88.
Cullen, J. T., Bergquist, B. A., and Moffett, J. W. (2006). Thermodynamic characterization of the partitioning of iron between soluble and colloidal species in the Atlantic Ocean. Mar. Chem. 98, 295–303.
Cutter, G., Andersson, P., Codispoti, L., Croot, P., Francois, R., Lohan, M., Obata, H., and Rutgers Van Der Loeff, M. (eds). (2010). Sampling and Sample-Handling Protocols for GEOTRACES Cruises. GEOTRACES. Available at: http://www.geotraces.org/library/geotraces-policies/170-sampling-and-sample-handling-protocols-for-geotraces-cruises
De Baar, H. J. W., and De Jong, J. T. M. (2001). “Distribution, sources and sinks of iron in seawater,” in The Biogeochemistry of Iron in Seawater, eds D. R. Turner and K. A. Hunter (Chichester: Wiley), 123–253.
Elrod, V. A., Berelson, W. M., Coale, K. H., and Johnson, K. S. (2004). The flux of iron from continental shelf sediments: a missing source for global budgets. Geophys. Res. Lett. 31, L12307.
Fan, S. M. (2008). Photochemical and biochemical controls on reactive oxygen and iron speciation in the pelagic surface ocean. Mar. Chem. 109, 152–164.
Fan, S. M., and Dunne, J. P. (2011). Models of iron speciation and concentration in the stratified epipelagic ocean. Geophys. Res. Lett. 38, L15611.
Garnier, C., Pizeta, I., Mounier, S., Benaim, J. Y., and Branica, M. (2004). Influence of the type of titration and of data treatment methods on metal complexing parameters determination of single and multi-ligand systems measured by stripping voltammetry. Anal. Chim. Acta 505, 263–275.
Gerringa, L. J. A., Herman, P. M. J., and Poortvliet, T. C. W. (1995). Comparison of the linear Van den Berg/RuziC transformation and the non-linear fit of the Langmuir isotherm applied to Cu speciation data in the estuarine environment. Mar. Chem. 48, 131–142.
Gerringa, L. J. A., Blain, S., Laan, P., Sarthou, G., Veldhuis, M. J. W., Brussaard, C. P. D., Viollier, E., and Timmermans, K. R. (2008). Fe-binding dissolved organic ligands near the Kerguelen Archipelago in the Southern Ocean (Indian Sector). Deep Sea Res. Part I Oceanogr. Res. Pap. 55, 606–621.
Gerringa, L. J. A., Rijkenberg, M. J. A., Wolterbeek, H. T., Verburg, T. G., Boye, M., and De Baar, H. J. W. (2007). Kinetic study reveals weak Fe-binding ligand, which affects the solubility of Fe in the Scheldt estuary. Mar. Chem. 103, 30–45.
Gerringa, L. J. A., Veldhuis, M. J. W., Timmermans, K. R., Sarthou, G., and De Baar, H. J. W. (2006). Co-variance of dissolved Fe-binding ligands with phytoplankton characteristics in the Canary Basin. Mar. Chem. 102, 276–290.
Gledhill, M., Mccormack, P., Ussher, S., Achterberg, E. P., Mantoura, R. F. C., and Worsfold, P. J. (2004). Production of siderophore type chelates by mixed bacterioplankton populations in nutrient enriched seawater incubations. Mar. Chem. 88, 75–83.
Gledhill, M., and van den Berg, C. M. G. (1994). Determination of complexation of iron (III) with natural organic complexing ligands in sea water using cathodic stripping voltammetry. Mar. Chem. 47, 41–54.
Gledhill, M., and van den Berg, C. M. G. (1995). Measurement of the redox speciation of iron in seawater by catalytic cathodic stripping voltammetry. Mar. Chem. 50, 51–61.
Gledhill, M., Van Den Berg, C. M. G., Nolting, R. F., and Timmermans, K. R. (1998). Variability in the speciation of iron in the northern North Sea. Mar. Chem. 59, 283–300.
Gobler, C. J., Donat, J. R., Consolvo, J. A. III, and Sanudo-Whilhelmy, S. A. (2002). Physicochemical speciation of iron during coastal algal blooms. Mar. Chem. 77, 71–89.
Gyurcsik, B., and Nagy, L. (2000). Carbohydrates as ligands: coordination equilibria and structure of the metal complexes. Coord. Chem. Rev. 203, 81–149.
Hansard, S. P., Landing, W. M., Measures, C. I., and Voelkar, B. M. (2009). Dissolved iron(II) in the Pacific Ocean: measurements from PO2 and P16N Clivar/CO2 repeat hydrography expeditions. Deep Sea Res. Part I Oceanogr. Res. Pap. 56, 1117–1129.
Harris, W. R., Carrano, C. J., and Raymond, K. N. (1979a). Coordination chemistry of microbial iron transport compounds.16. Isolation, characterization, and formation-constants of ferric aerobactin. J. Am. Chem. Soc. 101, 2722–2727.
Harris, W. R., Carrano, C. J., and Raymond, K. N. (1979b). Spectrophotometric determination of the proton-dependent stability constant of ferric enterobactin. J. Am. Chem. Soc. 101, 2213–2214.
Hassler, C. S., Alasonati, E., Nichols, C. A. M., and Slaveykova, V. I. (2011a). Exopolysaccharides produced by bacteria isolated from the pelagic Southern Ocean – role in Fe binding, chemical reactivity, and bioavailability. Mar. Chem. 123, 88–98.
Hassler, C. S., Schoemann, V., Nichols, C. M., Butler, E. C. V., and Boyd, P. W. (2011b). Saccharides enhance iron bioavailability to Southern Ocean phytoplankton. Proc. Natl. Acad. Sci. U.S.A. 108, 1076–1081.
Hider, R. C., and Kong, X. L. (2010). Chemistry and biology of siderophores. Nat. Prod. Rep. 27, 637–657.
Hirose, K. (2006). Chemical speciation of trace metals in seawater: a review. Anal. Sci. 22, 1055–1063.
Hirose, K. (2007). Metal-organic matter interaction: ecological roles of ligands in oceanic DOM. Appl. Geochem. 22, 1636–1645.
Homann, V. V., Edwards, K. J., Webb, E. A., and Butler, A. (2009a). Siderophores of Marinobacter aquaeolei: petrobactin and its sulfonated derivatives. Biometals 22, 565–571.
Homann, V. V., Sandy, M., Tincu, J. A., Templeton, A. S., Tebo, B. M., and Butler, A. (2009b). Loihichelins A-F, a suite of amphiphilic siderophores produced by the marine bacterium Halomonas LOB-5. J. Nat. Prod. 72, 884–888.
Hong, H., and Kester, D. R. (1986). Redox state of iron in the offshore waters of Peru. Limnol. Oceanogr. 31, 512–524.
Hopkinson, B. M., and Barbeau, K. A. (2007). Organic and redox speciation of iron in the eastern tropical North Pacific suboxic zone. Mar. Chem. 106, 2–17.
Hudson, R. J. M., Covault, D. T., and Morel, F. M. M. (1992). Investigations of iron coordination and redox reactions in seawater using 59Fe radiometry and ion-pair solvent extraction of amphiphilic iron complexes. Mar. Chem. 38, 209–235.
Hunter, K. A., and Boyd, P. W. (2007). Iron-binding ligands and their role in the ocean biogeochemistry of iron. Environ. Chem. 4, 221–232.
Hurst, M. P., and Bruland, K. W. (2008). The effects of the San Francisco Bay plume on trace metal and nutrient distributions in the Gulf of the Farallones. Geochim. Cosmochim. Acta 72, 395–411.
Hutchins, D. A., Witter, A. E., Butler, A., and Luther, G. W. III. (1999). Competition among marine phytoplankton for different chelated iron species. Nature 400, 858–861.
Ibisanmi, E., Sander, S. G., Boyd, P. W., Bowie, A. R., and Hunter, K. A. (2011). Vertical distributions of iron-(III) complexing ligands in the Southern Ocean. Deep Sea Res. Part II Top. Stud. Oceanogr. 58, 2113–2125.
Ito, Y., and Butler, A. (2005). Structure of synechobactins, new siderophores of the marine cyanobacterium Synechococcus sp PCC 7002. Limnol. Oceanogr. 50, 1918–1923.
Johnson, K. S., Coale, K. H., Elrod, V. A., and Tindale, N. W. (1994). Iron photochemistry in waters from the equatorial Pacific. Mar. Chem. 46, 319–334.
King, A. L., Buck, K. N., and Barbeau, K. A. (2012). Quasi-Lagrangian drifter studies of iron speciation and cycling off Point Conception, California. Mar. Chem. 128–129, 1–12.
Kitayama, S., Kuma, K., Manabe, E., Sugie, K., Takata, H., Isoda, Y., Toya, K., Saitoh, S. I., Takagi, S., Kamei, Y., and Sakaoka, K. (2009). Controls on iron distributions in the deep water column of the North Pacific Ocean: iron(III) hydroxide solubility and marine humic-type dissolved organic matter. J. Geophys. Res. 114, C08019.
Kondo, Y., Takeda, S., and Furuya, K. (2007). Distribution and speciation of dissolved iron in the Sulu Sea and its adjacent waters. Deep Sea Res. Part II Top. Stud. Oceanogr. 54, 60–80.
Kondo, Y., Takeda, S., Nishioka, J., Obata, H., Furuya, K., Johnson, W. K., and Wong, C. S. (2008). Organic iron(III) complexing ligands during an iron enrichment experiment in the western subarctic North Pacific. Geophys. Res. Lett. 35, L12601.
Kosakowska, A., Kupryszewski, G., Mucha, P., Rekowski, P., Lewandowska, J., and Pazdro, K. (1999). Identification of selected siderophores in the Baltic Sea environment by the use of capillary electrophoresis. Oceanologia 41, 573–587.
Kuma, K., and Matsunaga, K. (1995). Availability of colloidal ferric oxides to coastal marine-phytoplankton. Mar. Biol. 122, 1–11.
Kuma, K., Nakabayashi, S., Suzuki, Y., Kudo, I., and Matsunaga, K. (1992). Photo-reduction of Fe(III) by dissolved organic substances and existence of Fe(II) in seawater during spring blooms. Mar. Chem. 37, 15–27.
Kuma, K., Nishioka, J., and Matsunaga, K. (1996). Controls on iron(III) hydroxide solubility in seawater: the influence of pH and natural organic chelators. Limnol. Oceanogr. 41, 396–407.
Laglera, L. M., Battaglia, G., and Van Den Berg, C. M. G. (2007). Determination of humic substances in natural waters by cathodic stripping voltammetry of their complexes with iron. Anal. Chim. Acta 599, 58–66.
Laglera, L. M., Battaglia, G., and Van Den Berg, C. M. G. (2011). Effect of humic substances on the iron speciation in natural waters by CLE/CSV. Mar. Chem. 127, 134–143.
Laglera, L. M., and van den Berg, C. M. G. (2009). Evidence for geochemical control of iron by humic substances in seawater. Limnol. Oceanogr. 54, 610–619.
Lam, P. J., and Bishop, J. K. B. (2008). The continental margin is a key source of iron to the HNLC North Pacific Ocean. Geophys. Res. Lett. 35, L07608.
Loomis, L. D., and Raymond, K. N. (1991). Solution equilibria of enterobactin and metal enterobactin complexes. Inorg. Chem. 30, 906–911.
Macrellis, H. M., Trick, C. G., Rue, E. L., Smith, G., and Bruland, K. (2001). Collection and detection of natural iron-binding ligands from seawater. Mar. Chem. 76, 175–187.
Maldonado, M. T., and Price, N. M. (2001). Reduction and transport of organically bound iron by Thalassiosira oceanica (Bacillariophyceae). J. Phycol. 37, 298–309.
Maldonado, M. T., Strzepek, R. F., Sander, S., and Boyd, P. W. (2005). Acquisition of iron bound to strong organic complexes, with different Fe binding groups and photochemical reactivities, by plankton communities in Fe-limited subantarctic waters. Global Biogeochem. Cycles 19, GB4S23.
Mancuso Nichols, C. A., Garon, S., Bowman, J. P., Raguenes, G., and Guezennec, J. (2004). Production of exopolysaccharides by Antarctic marine bacterial isolates. J. Appl. Microbiol. 96, 1057–1066.
Martin, J. D., Ito, Y., Homann, V. V., Haygood, M. G., and Butler, A. (2006). Structure and membrane affinity of new amphiphilic siderophores produced by Ochrobactrum sp SP18. J. Biol. Inorg. Chem. 11, 633–641.
Martin, J. H., and Fitzwater, S. (1988). Iron deficiency limits phytoplankton growth in the north-east Pacific subarctic. Nature 331, 341–343.
Martinez, J. S., and Butler, A. (2007). Marine amphiphilic siderophores: marinobactin structure, uptake, and microbial partitioning. J. Inorg. Biochem. 101, 1692–1698.
Martinez, J. S., Carter-Franklin, J. N., Mann, E. L., Martin, J. D., Haygood, M. G., and Butler, A. (2003). Structure and membrane affinity of a suite of amphiphilic siderophores produced by a marine bacterium. Proc. Natl. Acad. Sci. U.S.A. 100, 3754–3759.
Martinez, J. S., Zhang, G. P., Holt, P. D., Jung, H.-T., Carrano, C. J., Haygood, M. G., and Butler, A. (2000). Self-assembling amphiphilic siderophores from marine bacteria. Science 287, 1245–1247.
Mawji, E., Gledhill, M., Milton, J. A., Tarran, G. A., Ussher, S., Thompson, A., Wolff, G. A., Worsfold, P. J., and Achterberg, E. P. (2008a). Hydroxamate siderophores: occurrence and importance in the Atlantic Ocean. Environ. Sci. Technol. 42, 8675–8680.
Mawji, E., Gledhill, M., Worsfold, P. J., and Achterberg, E. P. (2008b). Collision-induced dissociation of three groups of hydroxamate siderophores: ferrioxamines, ferrichromes and coprogens/fusigens. Rapid Commun. Mass Spectrom. 22, 2195–2202.
Mawji, E., Gledhill, M., Milton, J. A., Zubkov, M. V., Thompson, A., Wolff, G. A., and Achterberg, E. P. (2011). Production of siderophore type chelates in Atlantic Ocean waters enriched with different carbon and nitrogen sources. Mar. Chem. 124, 90–99.
McCormack, P., Worsfold, P. J., and Gledhill, M. (2003). Separation and detection of siderophores produced by marine bacterioplankton using high-performance liquid chromatography with electrospray ionization mass spectrometry. Anal. Chem. 75, 2647–2652.
Mochizuki, N., Tanaka, R., Grimm, B., Masuda, T., Moulin, M., Smith, A. G., Tanaka, A., and Terry, M. J. (2010). The cell biology of tetrapyrroles: a life and death struggle. Trends Plant Sci. 15, 488–498.
Mohamed, K. N., Steigenberger, S., Nielsdottir, M. C., Gledhill, M., and Achterberg, E. P. (2011). Dissolved iron(III) speciation in the high latitude North Atlantic Ocean. Deep Sea Res. Part I Oceanogr. Res. Pap. 58, 1049–1059.
Moore, J. K., and Braucher, O. (2008). Sedimentary and mineral dust sources of dissolved iron to the world ocean. Biogeosciences 5, 631–656.
Moore, J. K., Doney, S. C., and Lindsay, K. (2004). Upper ocean ecosystem dynamics and iron cycling in a global three-dimensional model. Global Biogeochem. Cycles 18, GB4028.
Mucha, P., Rekowski, P., Kosakowska, A., and Kupryszewski, G. (1999). Separation of siderophores by capillary electrophoresis. J. Chromatogr. A 830, 183–189.
Nishioka, J., Takeda, S., Wong, C. S., and Johnson, W. K. (2001). Size-fractionated iron concentrations in the northeast Pacific Ocean: distribution of soluble and small colloidal iron. Mar. Chem. 74, 157–179.
Nolting, R. F., Gerringa, L. J. A., Swagerman, M. J. W., Timmermans, K. R., and De Baar, H. J. W. (1998). Fe (III) speciation in the high nutrient, low chlorophyll Pacific region of the Southern Ocean. Mar. Chem. 62, 335–352.
Owen, T., Pynn, R., Martinez, J. S., and Butler, A. (2005). Micelle-to-vesicle transition of an iron-chelating microbial surfactant, marinobactin E. Langmuir 21, 12109–12114.
Parekh, P., Follows, M. J., and Boyle, E. A. (2005). Decoupling of iron and phosphate in the global ocean. Global Biogeochem. Cycles 19, GB2020.
Poorvin, L., Sander, S. G., Velasquez, I., Ibisanmi, E., Lecleir, G. R., and Wilhelm, S. W. (2011). A comparison of Fe bioavailability and binding of a catecholate siderophore with virus-mediated lysates from the marine bacterium Vibrio alginolyticus PWH3a. J. Exp. Mar. Biol. Ecol. 399, 43–47.
Powell, R. T., and Wilson-Finelli, A. (2003a). Importance of organic Fe complexing ligands in the Mississippi River plume. Estuar Coast Shelf Sci 58, 757–763.
Powell, R. T., and Wilson-Finelli, A. (2003b). Photochemical degradation of organic iron complexing ligands in seawater. Aquat Sci 65, 367–374.
Raven, J. A., Evans, M. C. W., and Korb, R. E. (1999). The role of trace metals in photosynthetic electron transport in O2-evolving organisms. Photosyn. Res. 60, 111–149.
Rijkenberg, M. J. A., Gerringa, L. J. A., Carolus, V. E., Velzeboer, I., and De Baar, H. J. W. (2006a). Enhancement and inhibition of iron photoreduction by individual ligands in open ocean seawater. Geochim. Cosmochim. Acta 70, 2790–2805.
Rijkenberg, M. J. A., Gerringa, L. J. A., Velzeboer, I., Timmermans, K. R., Buma, A. G. J., and De Baar, H. J. W. (2006b). Iron-binding ligands in Dutch estuaries are not affected by UV induced photochemical degradation. Mar. Chem. 100, 11–23.
Rijkenberg, M. J. A., Gerringa, L. J. A., Timmermans, K. R., Fischer, A. C., Kroon, K. J., Buma, A. G. J., Wolterbeek, B. T., and De Baar, H. J. W. (2008a). Enhancement of the reactive iron pool by marine diatoms. Mar. Chem. 109, 29–44.
Rijkenberg, M. J. A., Powell, C. F., Dall’osto, M., Nielsdottir, M. C., Patey, M. D., Hill, P. G., Baker, A. R., Jickells, T. D., Harrison, R. M., and Achterberg, E. P. (2008b). Changes in iron speciation following a Saharan dust event in the tropical North Atlantic Ocean. Mar. Chem. 110, 56–67.
Rue, E., and Bruland, K. (2001). Domoic acid binds iron and copper: a possible role for the toxin produced by the marine diatom Pseudo-nitzschia. Mar. Chem. 76, 127–134.
Rue, E. L., and Bruland, K. W. (1995). Complexation of iron(III) by natural organic ligands as determined by a new competitive equilibration/adsorptive cathodic stripping voltammetry method. Mar. Chem. 50, 117–139.
Rue, E. L., and Bruland, K. W. (1997). The role of organic complexation on ambient iron chemistry in the Equatorial Pacific Ocean and the response of a mesoscale iron addition experiment. Limnol. Oceanogr. 42, 901–910.
Ružic, I. (1982). Theoretical aspects of the direct titration of natural-waters and its information yield for trace-metal speciation. Anal. Chim. Acta 140, 99–113.
Sander, S. G., Hunter, K. A., Harms, H., and Wells, M. (2011). Numerical approach to speciation and estimation of parameters used in modeling trace metal bioavailability. Environ. Sci. Technol. 45, 6388–6395.
Sander, S. G., and Koschinsky, A. (2011). Metal flux from hydrothermal vents increased by organic complexation. Nat. Geosci. 4, 145–150.
Sarthou, G., Bucciarelli, E., Chever, F., Hansard, S. P., Gonzalez-Davila, M., Santana-Casiano, J. M., Planchon, F., and Speich, S. (2011). Labile Fe(II) concentrations in the Atlantic sector of the Southern Ocean along a transect from the subtropical domain to the Weddell Sea Gyre. Biogeosciences 8, 2461–2479.
Sato, M., Takeda, S., and Furuya, K. (2007). Iron regeneration and organic iron(III)-binding ligand production during in situ zooplankton grazing experiment. Mar. Chem. 106, 471–488.
Scatchard, G. (1949). The attractions of proteins for small molecules and ions. Ann. N.Y. Acad. Sci. 51, 606–672.
Schlosser, C., and Croot, P. L. (2008). Application of cross-flow filtration for determining the solubility of iron species in open ocean seawater. Limnol. Oceanogr. 6, 630–642.
Silver, M. W., Bargu, S., Coale, S. L., Benitez-Nelson, C. R., Garcia, A. C., Roberts, K. J., Sekula-Wood, E., Bruland, K. W., and Coale, K. H. (2010). Toxic diatoms and domoic acid in natural and iron enriched waters of the oceanic Pacific. Proc. Natl. Acad. Sci. U.S.A. 107, 20762–20767.
Stintzi, A., Barnes, C., Xu, L., and Raymond, K. N. (2000). Microbial iron transport via a siderophore shuttle: a membrane ion transport paradigm. Proc. Natl. Acad. Sci. U.S.A. 97, 10691–10696.
Stolpe, B., Guo, L., Shiller, A. M., and Hassellov, M. (2010). Size and composition of colloidal organic matter and trace elements in the Mississippi River, Pearl River and the northern Gulf of Mexico, as characterized by flow field-flow fractionation. Mar. Chem. 118, 119–128.
Stolpe, B., and Hassellov, M. (2010). Nanofibrils and other colloidal biopolymers binding trace elements in coastal seawater: significance for variations in element size distributions. Limnol. Oceanogr. 55, 187–202.
Strmecki, S., Plavsic, M., Steigenberger, S., and Passow, U. (2010). Characterization of phytoplankton exudates and carbohydrates in relation to their complexation of copper, cadmium and iron. Mar. Ecol. 408, 33–46.
Sunda, W., and Huntsman, S. (2003). Effect of pH, light, and temperature on Fe-EDTA chelation and Fe hydrolysis in seawater. Mar. Chem. 84, 35–47.
Tagliabue, A., Bopp, L., Aumont, O., and Arrigo, K. R. (2009). Influence of light and temperature on the marine iron cycle: from theoretical to global modeling. Global Biogeochem. Cycles 23, 2017.
Tagliabue, A., and Voelker, C. (2011). Towards accounting for dissolved iron speciation in global ocean models. Biogeosciences 8, 3025–3039.
Thuroczy, C. E., Gerringa, L. J. A., Klunder, M. B., Middag, R., Laan, P., Timmermans, K. R., and De Baar, H. J. W. (2010). Speciation of Fe in the Eastern North Atlantic Ocean. Deep Sea Res. Part I Oceanogr. Res. Pap. 57, 1444–1453.
Tian, F., Frew, R. D., Sander, S., Hunter, K. A., and Ellwood, M. J. (2006). Organic iron(III) speciation in surface transects across a frontal zone: the Chatham Rise, New Zealand. Mar. Freshw. Res. 57, 533–544.
Toner, B. M., Fakra, S. C., Manganini, S. J., Santelli, C. M., Marcus, M. A., Moffett, J., Rouxel, O., German, C. R., and Edwards, K. J. (2009). Preservation of iron(II) by carbon-rich matrices in a hydrothermal plume. Nat. Geosci. 2, 197–201.
Ussher, S. J., Worsfold, P. J., Achterberg, E. P., Laes, A., Blain, S., Laan, P., and De Baar, H. J. W. (2007). Distribution and redox speciation of dissolved iron on the European continental margin. Limnol. Oceanogr. 52, 2530–2539.
Van Den Berg, C. M. G. (1982). Determination of copper complexation with natural organic ligands in seawater by equilibration with MnO2. I. theory. Mar. Chem. 11, 307–322.
van den Berg, C. M. G. (1995). Evidence for organic complexation of iron in seawater. Mar. Chem. 50, 139–157.
van den Berg, C. M. G. (2006). Chemical speciation of iron in seawater by cathodic stripping voltammetry with dihydroxynaphthalene. Anal. Chem. 78, 156–163.
van der Merwe, P., Lannuzel, D., Nichols, C. A. M., Meiners, K., Heil, P., Norman, L., Thomas, D. N., and Bowie, A. R. (2009). Biogeochemical observations during the winter-spring transition in East Antarctic sea ice: evidence of iron and exopolysaccharide controls. Mar. Chem. 115, 163–175.
Velasquez, I., Nunn, B. L., Ibisanmi, E., Goodlet, D. R., Hunter, K. A., and Sander, S. G. (2011). Detection of hydroxamate siderophores in coastal and sub-Antarctic waters off the South Eastern coast of New Zealand. Mar. Chem. 126, 97–107.
Vong, L., Laes, A., and Blain, S. (2007). Determination of iron-porphyrin-like complexes at nanomolar levels in seawater. Anal. Chim. Acta 588, 237–244.
Vraspir, J., and Butler, A. (2009). Chemistry of marine ligands and siderophores. Ann. Rev. Mar. Sci. 1, 43–63.
Wagener, T., Pulido-Villena, E., and Guieu, C. (2008). Dust iron dissolution in seawater: results from a one-year time-series in the Mediterranean Sea. Geophys. Res. Lett. 35, L16601.
Weber, L., Volker, C., Schartau, M., and Wolf-Gladrow, D. A. (2005). Modeling the speciation and biogeochemistry of iron at the Bermuda Atlantic Time-series Study site. Global Biogeochem. Cycles 19, GB1019.
Wells, M. L., Trick, C. G., Cochlan, W. P., Hughes, M. P., and Trainer, V. L. (2005). Domoic acid: the synergy of iron, copper, and the toxicity of diatoms. Limnol. Oceanogr. 50, 1908–1917.
Witter, A. E., Hutchins, D. A., Butler, A., and Luther, G. W. III. (2000). Determination of conditional stability constants and kinetic constants for strong model Fe-binding ligands in seawater. Mar. Chem. 69, 1–17.
Witter, A. E., and Luther, G. W. III. (1998). Variation in Fe-organic complexation with depth in the Northwestern Atlantic Ocean as determined using a kinetic approach. Mar. Chem. 62, 241–258.
Wu, J., and Luther, G. W. III. (1994). Size fractionated iron concentrations in the water column of the Northwest Atlantic Ocean. Limnol. Oceanogr. 39, 1119–1129.
Wu, J., and Luther, G. W. III. (1995). Complexation of iron(III) by natural organic ligands in the Northwest Atlantic Ocean by a competitive ligand equilibration method and a kinetic approach. Mar. Chem. 50, 159–179.
Wu, J. F., Boyle, E., Sunda, W., and Wen, L. S. (2001). Soluble and colloidal iron in the oligotrophic North Atlantic and North Pacific. Science 293, 847–849.
Xu, G. F., Martinez, J. S., Groves, J. T., and Butler, A. (2002). Membrane affinity of the amphiphilic marinobactin siderophores. J. Am. Chem. Soc. 124, 13408–13415.
Ye, Y., Voelker, C., and Wolf-Gladrow, D. A. (2009). A model of Fe speciation and biogeochemistry at the Tropical Eastern North Atlantic Time-Series Observatory site. Biogeosciences 6, 2041–2061.
Keywords: seawater, speciation, colloids, siderophores, exopolymeric substances, humic substances, nanoparticles, ligands
Citation: Gledhill M and Buck KN (2012) The organic complexation of iron in the marine environment: a review. Front. Microbio. 3:69. doi: 10.3389/fmicb.2012.00069
Received: 14 November 2011;
Accepted: 09 February 2012;
Published online: 28 February 2012.
Edited by:
Benjamin Twining, Bigelow Laboratory for Ocean Sciences, USAReviewed by:
Sylvia G. Sander, University of Otago, New ZealandAlessandro Tagliabue, Council for Scientific and Industrial Research, South Africa
Copyright: © 2012 Gledhill and Buck. This is an open-access article distributed under the terms of the Creative Commons Attribution Non Commercial License, which permits non-commercial use, distribution, and reproduction in other forums, provided the original authors and source are credited.
*Correspondence: Martha Gledhill, Ocean and Earth Science, National Oceanography Centre – Southampton, University of Southampton, Southampton SO14 3ZH, UK. e-mail:bWFydGhhQHNvdG9uLmFjLnVr; Kristen N. Buck, Bermuda Institute of Ocean Sciences, Ferry Reach, St. George’s GE 01, Bermuda. e-mail:a3Jpc3Rlbi5idWNrQGJpb3MuZWR1
†Martha Gledhill and Kristen N. Buck have contributed equally to this work.