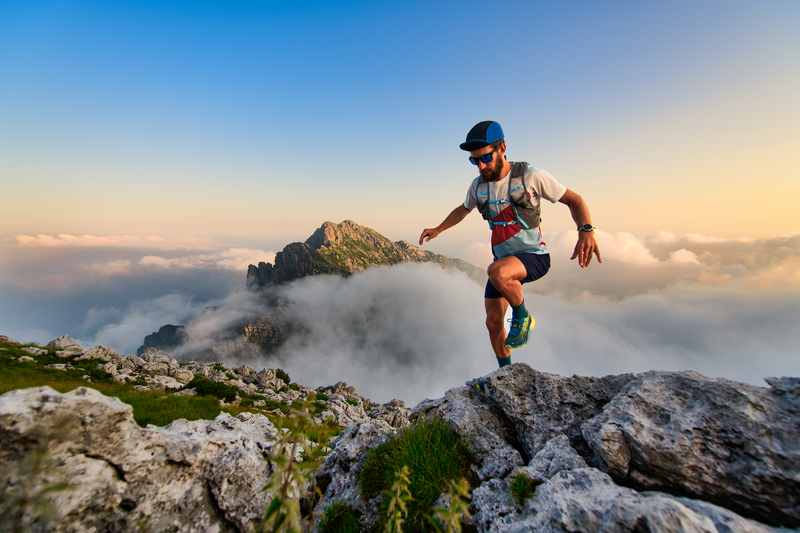
94% of researchers rate our articles as excellent or good
Learn more about the work of our research integrity team to safeguard the quality of each article we publish.
Find out more
REVIEW article
Front. Med. Technol. , 23 January 2024
Sec. Pharmaceutical Innovation
Volume 5 - 2023 | https://doi.org/10.3389/fmedt.2023.1330007
This article is part of the Research Topic Targeting Ion Homeostasis for Cancer Therapy: Mechanisms and Immunomodulatory Effects View all 7 articles
The emergence of nanotechnology as a field of study can be traced back to the 1980s, at which point the means to artificially produce, control, and observe matter on a nanometer level was made viable. Recent advancements in technology have enabled us to extend our reach to the nanoscale, which has presented an unparalleled opportunity to directly target biomolecular interactions. As a result of these developments, there is a drive to arise intelligent nanostructures capable of overcoming the obstacles that have impeded the progress of conventional pharmacological methodologies. After four decades, the gradual amalgamation of bio- and nanotechnologies is initiating a revolution in the realm of disease detection, treatment, and monitoring, as well as unsolved medical predicaments. Although a significant portion of research in the field is still confined to laboratories, the initial application of nanotechnology as treatments, vaccines, pharmaceuticals, and diagnostic equipment has now obtained endorsement for commercialization and clinical practice. The current issue presents an overview of the latest progress in nanomedical strategies towards alleviating antibiotic resistance, diagnosing and treating cancer, addressing neurodegenerative disorders, and an array of applications, encompassing dentistry and tuberculosis treatment. The current investigation also scrutinizes the deployment of sophisticated smart nanostructured materials in fields of application such as regenerative medicine, as well as the management of targeted and sustained release of pharmaceuticals and therapeutic interventions. The aforementioned concept exhibits the potential for revolutionary advancements within the field of immunotherapy, as it introduces the utilization of implanted vaccine technology to consistently regulate and augment immune functions. Concurrently with the endeavor to attain the advantages of nanomedical intervention, it is essential to enhance the unceasing emphasis on nanotoxicological research and the regulation of nanomedications' safety. This initiative is crucial in achieving the advancement in medicine that currently lies within our reach.
The fabrication of innovative engineered materials, especially nanomaterials, has experienced a significant surge within the past three to four decades. Emerging as versatile materials, they are utilized in diverse fields such as engineering, waste management, sports equipment, the electronic industry, optical devices, garments, food production, and cosmetic formulations, dominating virtually all sectors of daily living (1–6). The present issue focuses on an array of novel material applications in medicine facilitated through the amalgamation of nanotechnology and biotechnology. The distinctive characteristics of nanoscale materials, namely their inherent capacity for physiochemical customization and manipulation, enable the exploration of a vast array of possibilities within the medical realm. This includes the early identification of biomarkers, precise targeting of cellular and tissue components, development of sophisticated drug delivery mechanisms, accurate staging and evaluation of medical conditions, and treatment of degenerative ailments. Such capabilities have profound implications for medical advancements and innovations. Engineered nanomaterials have been precisely characterized as possessing one dimension measuring less than 100 nm (7, 8).
In the field of medicine, the definition of a drug is characterized by a degree of flexibility, wherein it may encompass a diverse range of formulations such as a nano drug comprising particles measuring 200 nm or greater in size. Moreover, the terminology “nanoparticle” possesses a comprehensive connotation, encompassing not only spherically-shaped organic and inorganic nanomaterials but also cuboidal, star-shaped, needle-like, spheroidal or intricately-structured forms possessing complex geometries, with an aerodynamic diameter of less than 100 nm. Certain articles expound upon the fundamental characteristics of particles within the respective scope of the subject matter, whilst varying articles do not prioritize such discourse. The objective of this disclosure is to ensure that the audience comprehends the broadest interpretation of this term, as it is utilized in the subsequent articles (9–12).
The issue of anti-microbial resistance poses a worldwide challenge that is currently impacting contemporary healthcare systems. The emergence of antimicrobial resistance across various classes of antibiotics can be attributed to the suboptimal prescribing patterns of antibiotics (13). Undoubtedly, this phenomenon will considerably influence the future effectiveness and utilization of antibiotics in the realms of community and hospital care on a worldwide scale (14, 15). The World Health Organization (WHO) unveiled its inaugural list of antibiotic-resistant pathogens in February of 2017, detailing a dire need for the prompt development of novel anti-microbial therapies (16, 17). Among the twelve pathogens exhibiting resistance, it was observed that seven strains evinced resistance to beta-lactam antibiotics. The three pathogens classified as “Critical” exhibit resistance towards carbapenems specifically imipenem, while four other pathogens are resistant to fluoroquinolones, such as ciprofloxacin, which are extensively employed in the clinical setting. The aforementioned fact is a source of concern as it portends challenges not only for prescribing practices but also regarding the acquisition of appropriate antibiotics for patient treatment in the future. Despite the current situation, the World Health Organization (WHO) has expressed that this presents a favorable circumstance for the research and development (R&D) industry to innovate novel antibiotics, thereby establishing a novel target for forthcoming research tactics. Bacteria, which are classified as prokaryotes due to their lack of a nuclear membrane, are categorized as either Gram-positive or Gram-negative based on the structure of their cell wall. A Gram stain is a widely used laboratory test for classifying bacteria based on the capacity of the bacterial cell wall to absorb and hold onto crystal violet dye. Gram positive and Gram negative bacteria differ in the thickness of their peptidoglycan layers in their cell membranes. As a result, while Gram negative bacteria lose their crystal violet stain during the decolorization process, Gram positive bacteria retain it (18). Gram-positive bacteria are characterized by the presence of a rigid cell wall comprised of a thick layer of peptidoglycan. This peptidoglycan is composed of carbohydrate polymers that are cross-linked by peptide residues (19). Teichoic acid is detected on the outer surface of Gram-positive bacteria, which endows them with the capacity to sequester metal ions and function as a safeguard system against the immune response mounted by the host organism (20). Lipoteichoic acids are detected in the cellular membrane, facilitating surface adherence. In contrast, Gram-negative bacteria possess a peptidoglycan layer that is thinner and more inflexible, featuring substantially reduced levels of cross-linking. This layer is enveloped by a lipid membrane that displays lipopolysaccharides (LPS) on its surface (21). Methicillin-resistant S. aureus (MRSA) is of particular clinical importance due to its resistance to multiple antibiotics. Staphylococcus aureus, a Gram-positive bacterium, exemplifies unique resistance to various antibiotics, with Methicillin-resistant MRSA serving as a notable instance of clinical significance. The layperson frequently associates MRSA with its antibiotic resistance. MRSA infections necessitate extended therapy regimens, frequently involving potent antibiotics, and consequently result in heightened occurrences of patient hospitalization and public expenditure. Multiple approaches seek to employ nanotechnology as a means of addressing this problem (22). Many strategies aim to use nanotechnology to tackle this issue.
The employment of conventional oral or intravenous pharmaceuticals to manage microbial infections is linked with a host of difficulties. The current treatment protocols, characterized by the administration of substantial doses as a strategy to guarantee the delivery of adequate quantities to the intended microbial targets, exhibit drawbacks such as inadequate efficacy and the potential for adverse reactions, culminating in the evolution of drug resistance amongst the targeted microorganisms. The insufficiency of unconventional therapeutic modalities and tactics to surmount the aforementioned issue has engendered considerable apprehension among governmental organizations, medical experts, and ultimately, the global populace owing to its conspicuous influence on public health. One viable strategy for combating this issue involves the utilization of nanomaterials to augment and potentiate the antimicrobial effectiveness of both established and innovative medicinal interventions (23–26). Through mechanisms such as disrupting the membrane potential and integrity of bacterial cells, preventing the formation of biofilms and ROS production, strengthening host immune responses, and blocking RNA and protein synthesis by inducing intracellular processes, these NPs primarily reduce the resistance properties of bacteria (Figure 1). Table 1 mentions several studies conducted in this field.
Figure 1. The primary mechanisms of nanoparticles’ antimicrobial activity are as follows: breaking down the pathogen cell wall, which increases permeability; producing reactive oxygen species (ROS), which upsets redox homeostasis and damages cellular structures; and attaching to intracellular structures and molecules, like DNA and protein, which causes their dysfunction.
Tuberculosis (TB) could be a zoonotic and anthropozoonotic infection with a complex pathogenesis, created by microbes from Mycobacterium tuberculosis complex (MtbC), primarily M. tuberculosis, and in a lesser sum by the contaminations with other mycobacteria such as M. bovis, M. canetti, M. caprae, M. africanum, and sometimes M. microti or Mycobacterium pinnipedii (35, 36). The present resurgence of TB at a regional and global level is impeded by factors such as the emergence of multidrug-resistant strains, intercurrent immunosuppressive conditions, the high costs and low production outputs of recently endorsed antitubercular antibiotics, and moderately effective vaccination measures. As a result, these factors have hindered progress toward eradicating TB. TB is a global infectious disease that affects over one-third of the human population. Despite recent progress in treatment and prevention, the latest report by the World Health Organization identifies it as the primary cause of infectious-bacterial deaths amongst adults, with a staggering 10 million new cases and 1.5 million deaths attributed to TB in 2018 alone. Furthermore, it has been observed that TB serves as the primary contributing factor to hospital fatalities in certain regions that are characterized by a high prevalence of the disease (37).
Nanotechnology and nanoparticle science providing innovative approaches and new-practical solutions for several critical-issues, including TB (37–39). Extended treatment durations and frequently changing drug dosages pose significant obstacles to the effectiveness of current TB medicines, since they frequently result in patients not adhering to prescribed regimens or receiving inadequate care. Regarding extensively drug-resistant (XDR) tuberculosis (DRT) and multidrug-resistant (MDR) TB, the primary contributing factor to the illness's recurrence is the patient's noncompliance. Medical researchers are faced with a difficulty since MDR-TB and XDR-TB are growing increasingly prevalent in developing countries and pose a significant danger to world health (40). Novel approaches to drug delivery that leveraged ligands and nanocarriers were investigated, and a summary of several nano delivery systems was provided. It is now quite helpful to employ pulmonary nanodrug delivery systems as a therapeutic agent to treat tuberculosis (Figure 2). The advantages of employing nanomaterials to deliver medications for the treatment of infectious lung disorders include targeted drug delivery, enhanced drug solubility, and decreased toxicity, fewer adverse effects compared to standard drug regimens that produce MDR and XDR, and synergistic therapeutic effects. Table 2 mentions a number of studies conducted in this field.
Figure 2. Nanotechnology is being used to develop medicine delivery systems that specifically target alveolar macrophages and granulomas for the treatment of TB.
Vaccination is a medical intervention that involves the administration of an antigenic substance into an individual's body to elicit an immune response and establish adaptive immunity against a targeted pathogen (61, 62). The efficacy and cost-efficiency of applying preventive measures to contain infectious diseases have been convincingly demonstrated. Numerous consequential maladies such as tetanus, mumps, measles, smallpox, polio, rubella, pertussis, yellow fever, and diphtheria have been effectively eliminated or effectively controlled by means of vaccinations (63, 64). Despite the notable achievements of vaccination therapies, numerous disease entities remain devoid of an effective prophylactic tactic. Examples of such ailments include acquired immunodeficiency syndrome (AIDS), tuberculosis, malaria, and dengue fever. Consequently, there is a continual pursuit of novel vaccination formulations and technologies (65). Vaccine formulations typically comprise attenuated subunit protein antigens and inactivated microorganisms that elicit a specific immunological response. Each system possesses distinct advantages and disadvantages, and there is often a trade-off between safety and efficacy (66, 67). Antigen might be encapsulated in the core of the nanoparticles or attached to their surface. Antibodies, Fab-fragments, peptides, and other targeting molecules can be used to decorate the surface of nanoparticles, which can enhance their distribution into antigen-presenting cells (APCs) and trigger both innate and adaptive immune responses (Figure 3) (68, 69).
Figure 3. Utilizing surface modified nanoparticles to deliver antigenic compounds specifically to APCs. Antigens that are produced inside the body are shown together with class I major histocompatibility complex (MHC I) on the surface of APCs to CD8+ T lymphocytes. Upon the interaction of MHC I and T-cell receptor (TCR) in the presence of co-stimulatory molecules and cytokines, the activated CD8+ cells initiate cytotoxicity to eliminate the infected cells. Furthermore, the antigens are displayed on the surface of the APCs through class II major histocompatibility complex (MHC II) molecules to activate the helper (CD4+) T cells. Afterward, CD4+ cells stimulate B-cells to generate antimicrobial antibodies.
The capacity of NPs to regulate immune responses toward attaining intended outcomes is imperative in the development of vaccines leveraging nanotechnology. NPs hold the potential as a dual-purpose agent in augmenting and intensifying protective immunity by serving as both a delivery vehicle and an immune-stimulatory adjuvant (70, 71). Nano vaccines, which utilize NPs as carriers or adjuvants, offer several distinct advantages over conventional vaccines. These advantages include the ability to decrease the rate of antigenic degradation, enhance the stability of antigens, immunogenicity and improve vaccine therapeutic efficacy, facilitate efficient phagocytosis and rapid processing by, enhance cellular membrane penetrability and APCs (72). The current research indicates that nanocarriers, including liposomes, dendrimers, and virosomes, exhibit properties that enhance cytokine induction and antibody response. As a result, recent efforts have been directed toward the development of vaccine delivery strategies employing these nanocarriers (73). The mentioned nanocarriers represent a diverse group of nanomaterials, renowned for their distinctive structural designs, suitable for serving as potential paradigms for drug delivery modalities. Furthermore, they enhance the bioavailability of compounds, offer stabilization and safeguarding of more delicate agents such as proteins, reduce the occurrence of adverse effects, and facilitate active targeting (74). Table 3 mentions several studies conducted in this field.
NPs are naturally occurring entities that are ubiquitous in the environment and hold significant utility in various daily applications. The advancement of nanotechnology has led to a sharp rise in the use of NPs in cosmetics. Better absorption of substances through the skin, longer-lasting effects, and increased stability are just a few of the benefits that come with using NPs. Currently, sunscreen products use NPs as UV filters most frequently in cosmetics. Zinc oxide (ZnO) or Titanium dioxide (TiO2) particles are frequently utilized as ultraviolet filters or as additives in toothpaste, with a focus on titanium dioxide or silicates in toothpaste formulations. NPs are ubiquitously found in numerous edibles, nutritional additions, and spritzers utilized for veneering, disinfecting, and saturating (82–84). They possess the ability to enhance, for instance, the preservation of the edibility and integrity of the comestible, its flavor profile, and its physical coherence. In certain jurisdictions, silicon dioxide, magnesium oxide, and titanium dioxide are subject to rigorous evaluation and authorization as permissible food additives (85). In dentistry, NPs have gained growing importance as deliberate inclusions in various products (Figure 4). This materials enhance the fundamental traits of resin-based composites, such as their capacity for high polish ability and retention of gloss stability (86). In addition to their conventional applications, these materials hold potential value as constituents of scaffolding frameworks used for tissue engineering purposes (87).
Figure 4. Utilizations of organic and inorganic NPs in dental care and treatment. NPs are utilized in dental inventions and diagnostic procedures. NPs are utilized in the development of oral disease preventative medications, prostheses, and dental implants. Nanomaterials have the ability to provide oral fluids or medications, effectively preventing and treating certain oral diseases such as oral cancer, while significantly promoting oral healthcare.
Dental materials that are designed to intentionally release NPs are relatively infrequent, such as those utilized in occlusion indicator foils and scanning sprays for computer-aided design or computer-aided manufacturing (CAD/CAM). Conversely, nanoparticles may be generated as by-products during milling procedures employed for filler production (88). Numerous dental materials, including but not limited to resin-based composites, cement, and impression materials, are known to comprise fillers. Consequently, it has been projected that nanoparticles are extant in roughly 3,500 dental materials. Nanotechnology exhibits considerable potential for numerous applications in everyday life. Diverse scientific entities at the global level, including both research collectives and national/international agencies, have invested significant efforts in the development of this innovative and auspicious technology (89, 90). Table 4 mentions several studies conducted in this field.
Cancer denotes a comprehensive class of ailments that are typified by the unregulated proliferation of cells and their invasiveness into surrounding tissues (108, 109). Considerable endeavors spanning multiple years have been devoted to identifying diverse risk elements associated with cancer. The etiology of certain cancers has been notably linked to particular acquired factors within the environment, including radiation and pollution. Adopting an unhealthy lifestyle, marked by bad dietary habits, consumption of tobacco products, smoking, stress, and absence of physical activity, profoundly influences the determination of cancer risk (110, 111). Although external factors have been widely acknowledged as significant contributors to carcinogenesis, the precise impact of somatic mutations in proto-oncogenes, alterations in tumor suppressor gene expression, and variations in the genes involved in DNA repair mechanisms remain a challenging proposition to assess accurately. A mere 5%-10% of cancer instances correlate with genetic inheritance (112). The progression of chronological age is deemed a pivotal determinant for the onset of cancer and its diverse manifestations. The traditional therapeutic modalities employed in the management of cancer consist of several methods which include surgical intervention, radiation therapy, chemotherapy, targeted therapy, hormone therapy and immunotherapy (113, 114). Radiation therapy and Chemotherapy exhibit cytostatic and cytotoxic capabilities (115). Frequently associated with severe adverse reactions and a significantly elevated chance of relapse, these methodologies constitute a notable concern within the medical community. The administration of this agent is commonly associated with the occurrence of suppression of bone marrow, neuropathies, skin disorders, and gastrointestinal, alopecia, and fatigue as the prevailing manifestations of treatment-related adverse effects. Moreover, the administration of certain drugs may entail unique adverse effects, such as anthracyclines and bleomycin-induced cardiotoxicity and pulmonary toxicity. The progress in precision medicine has been bolstered by the emergence of targeted therapy. Despite the advances in therapeutic interventions, numerous inescapable detrimental repercussions, such as the emergence of multi-drug resistance, continue to impede the effectiveness of the treatment regimens (116, 117). Immunotherapeutic agents have exhibited auspicious outcomes not only in the treatment of primary cancer but also in their ability to prevent distant metastasis and diminish the frequency of recurrence (118). Notwithstanding, immunotherapy constitutes a significant factor precipitating autoimmune diseases. Moreover, scholarly investigations and empirical findings posit that immunotherapy exhibits a comparatively lower efficacy in addressing solid tumors in contrast to lymphoma (119). The cancers under consideration manifest an anomalous extracellular matrix (ECM) that presents a formidable hurdle for the infiltration of immune cells (120). This statement suggests that recent developments in targeted therapies and immunotherapies have introduced interventions that disrupt vital signaling pathways implicated in both malignant and homeostatic properties within the epidermis and dermis. As a consequence, the implementation of these therapies may lead to adverse dermatologic events (dAEs) (121). About the aforementioned particulars, there has been an upsurge in the need for the development of innovative approaches toward attaining accurate cancer therapy in recent times. Contemporary undertakings have been initiated to tackle the constraints of prevailing therapeutic methodologies by employing nanoparticles (Figure 5) (122–129). Nanoparticle-mediated drug delivery systems have been observed to offer advantages in the treatment and control of cancer by exhibiting favorable pharmacokinetics, accurate targeting, diminished adverse effects, and mitigated drug resistance (130, 131).
Figure 5. The application of nanoparticles in the field of cancer. At present, several platforms are under investigation for their potential use in both cancer therapy and diagnosis.
Following the recent strides in the field of nanotechnology, numerous nanotherapeutic medications have been successfully commercialized and extensively promoted. Furthermore, an abundance of these drugs has advanced to clinical trials since 2010. Advances in the field of drug delivery systems and combatting multi-drug resistance (MDR) in the context of tumorigenesis have been achieved through the development of nanotherapeutic drugs. Such drugs have facilitated the pursuit of combination therapy and effectively inhibited resistance mechanisms associated with drug treatments (132). In the 1960s, an initial endeavor was taken to implement the use of nanotechnology within the field of medicine, specifically at the esteemed academic institution of ETH Zurich, it is a Swiss public research university located in Zürich (133). This amalgamation has demonstrated enhanced efficacy in the development of diverse diagnostic equipment and superior therapies. NPs have been documented to possess a significant ability to penetrate deep tissues, thereby promoting the enhancement of the permeability and retention (EPR) phenomenon. Moreover, it should be noted that the surface characteristics of a substance exert a significant influence on its bioavailability and half-life, primarily by facilitating its passage across epithelial fenestrations (134). An instance of this phenomenon is observed in the use of NPs that have undergone coating with polyethylene glycol (PEG), a hydrophilic polymer, which generates a decreased tendency for opsonization and also manages to evade clearance by the immune system (135). Furthermore, the modularity of the release kinetics of therapeutic substances or active components can be achieved through the manipulation of particle polymer attributes. Collectively, the unique characteristics exhibited by nanoparticles play a pivotal role in modulating their therapeutic efficacy in the management and treatment of cancer. Table 5 mentions several studies conducted in this field.
The brain assumes the mantle of being the most intricate organ in the human anatomy. The entity in question is intricately involved in the regulation of various cognitive, behavioral, and emotional activities. The organ in question is susceptible to various disorders and afflictions, ranging from physical trauma to malignancies and cognitive degenerative conditions. Neurological disorders are the primary contributors to impairment and a significant factor in mortality. Neurodegenerative disorders pose a significant risk to human welfare. The prevalence of age-dependent disorders has escalated, attributable to the rise in elderly populations witnessed in recent times. Prominent cases of neurodegenerative illnesses include Alzheimer's disease, Parkinson's disease, Huntington's disease, amyotrophic lateral sclerosis, frontotemporal dementia, and spinocerebellar ataxias. Many illnesses exhibit varying pathophysiological mechanisms; some result in cognitive dysfunction and memory impairments, while others disrupt an individual's motor, communication, and respiratory functions (160, 161). Numerous medications, which have demonstrated promise in enhancing cerebral architecture and operation in animal models, encounter a plethora of difficulties such as distribution, selectivity, and toxicity. For a considerable duration, researchers have encountered the formidable obstacles of formulating pharmaceutical substances capable of penetrating the physiological impediment of the blood-brain barrier, as well as navigating the electrical and chemical defenses of the brain, while simultaneously achieving targeted localization with minimal deleterious consequences. In recent times, nanotechnology has surfaced as a crucial methodology for the alteration and manipulation of diverse entities at the atomic scale to achieve targeted properties. The utilization of this particular methodology has demonstrated its efficacy in both diagnosis and treatment of cerebral diseases and disorders by improving drug delivery and enhancing their effectiveness. Given the present significance and ongoing advancements in research, technology may greatly improve healthcare systems by providing user-friendly and highly effective diagnosis and treatment approaches (162, 163). Table 6 mentions several studies conducted in this field.
Table 6. The utilization of nanotechnology in the context of central nervous system (CNS) conditions.
The practice of tissue and organ transplantation has been hindered by numerous challenges, including limited access to donors, the requirement for immunosuppression, as well as low success rates due to the rejection of the transplanted material. Consequently, the field of tissue engineering and regenerative medicine (TERM) has recently been experiencing a significant rise in interest as an alternative solution. This multidisciplinary field continues to rapidly expand. The interdisciplinary fields of biology, materials science, and engineering have been synthesized to facilitate the production and design of synthetic structures that mimic natural tissues and organs. These structures are not limited to implantable devices, but can also include miniature, modeled versions of the aforementioned organs (194). Achieving a biomimetic extracellular matrix (ECM) composition in a tissue's three-dimensional (3D) scaffold for cells that is endowed with suitable mechanical strength, facile monitoring of cellular activities, and provision of bioactive agents, necessitates a nanoscale methodology over a macroscopic one, for optimal performance. NPs have the potential to offer an efficacious means of regulating scaffolds' properties, including precise manipulation of their mechanical strength and the provision of controlled bioactive agent delivery (195, 196). Furthermore, several disadvantages and constraints, namely low solubility, unstable bioactivity, and truncated circulation half-life of bioactive molecules (e.g., growth factors, cytokines, inhibitors, genes, drugs, etc.), as well as contrast agents, have positioned NPs as among the most appropriate alternatives for the delivery and monitoring of bioactive agents in various applications (124, 197).
The ramifications of nanotechnology have resulted in a fundamental transformation of conventional and rudimentary modalities in TERM towards more intricate and productive mechanisms. In the realm of tissue engineering and regenerative medicine, nanoscale products, including nanofibers and nanopatterned surfaces, have been employed to influence cellular behavior alongside NPs. The employment of concurrent therapeutic and imaging mechanisms, incorporation of unconventional biomaterials possessing enhanced spatiotemporal management within scaffolds, manipulation of the discharge of diverse bioactive agents—notably growth factors—to govern the trajectory of stem cells and morphogenesis, regulation of the mechanical potency of scaffolds for hard tissue utilization, and reduction of toxicity and improvement of biocompatibility via tissue-targeted administration constitute a range of potential uses for NPs in TERM (198, 199). NPs can be developed utilizing a diverse range of materials, including ceramics, metals, and both natural and synthetic polymers. Nanostructured materials have emerged as highly favored candidates in tissue engineering and TERM due to their advantageous attributes, such as elevated penetration capability, amplified surface area with customizable surface properties, and compositional variability. These properties render them highly effective for a range of applications in TERM, including imaging, strength reinforcement, bioink, antimicrobial activity, and bioactive agent carrier functions (200). Table 7 mentions several studies conducted in this field.
The successful implementation of nanomedicine and attainment of its medical efficacy hinges upon the comprehension of the toxicity about nanomaterials. Nanostructures demonstrate significant prospects in the field of medicine due to their capacity to exhibit chemical and biological activity, as well as their capability to access areas that traditional techniques are unable to reach. Specifically, nanostructures can be administered via inhalation, ingestion, or translocation through the skin, and once within the body, can permeate tissues, cells, and physical barriers. This allows for the potential to traverse across biological barriers, such as the blood-brain barrier, and to reach vital organs. Nanostructures still carry the risk of unintentional bodily injury, regardless of any potential benefits. Over the course of twenty years, the field of nanotoxicology has demonstrated that the intricate interactions between nanomaterials and cellular, animal, human, and environmental systems are exceedingly intricate (218). These entities have been associated with a variety of detrimental health effects, including cellular apoptosis, inflammation, exacerbation of asthmatic symptoms, fibrosis, chronic lung diseases marked by persistent inflammation, and carcinogenic processes. Significantly, the aforementioned toxicological investigations have underscored the imperative need to shun certain physical and functional characteristics of artificially designed nanomaterials. The conscientious application of responsible research and innovation in the realm of nanomedicine is legitimately anchored in the paramountcy accorded to nanotoxicity and nanotoxicology as its focal points of inquiry. The field of nanotoxicology is one that experts, regulatory agencies, and researchers can work together to investigate. Investigating this interdisciplinary area provides a means for different stakeholders to work together, which will aid in the ongoing discussions about the proper regulation and safe use of NPs (219, 220). Table 8 mentions several studies conducted in this field.
There are a lot of chances to modify and control the activity of cells and tissues at the nanoscale in the rapidly developing field of nanotechnology. The combination of bio- and nanotechnologies is transforming the approaches used to identify, treat, and track illnesses, thereby addressing both present-day and future medical issues. This issue showcases noteworthy advancements in the field of nanomedicine, which covers a wide range of medical issues such as tissue regeneration, dental health, cancer, tuberculosis, antibiotic resistance, and vaccination efficacy. NPs can, however, inadvertently cause harm to individuals even if they have advantageous qualities that make them very helpful in medical applications. Finding the precise physicochemical characteristics of NPs linked to toxicity is the main goal of the study of nanotoxicology, which ultimately aims to direct the creation of safe nanomaterials. Optimizing the safety profile of nanomaterials meant for use in medical contexts is the main goal of this field of study.
AS-N: Writing – original draft. HB: Writing – review & editing. AA: Investigation, Writing – original draft. MK: Methodology, Writing – original draft. MA: Validation, Writing – review & editing. AD: Writing – review & editing. YM: Investigation, Writing – review & editing. AH-A: Conceptualization, Writing – review & editing.
The author(s) declare that no financial support was received for the research, authorship, and/or publication of this article.
The authors declare that the research was conducted in the absence of any commercial or financial relationships that could be construed as a potential conflict of interest.
All claims expressed in this article are solely those of the authors and do not necessarily represent those of their affiliated organizations, or those of the publisher, the editors and the reviewers. Any product that may be evaluated in this article, or claim that may be made by its manufacturer, is not guaranteed or endorsed by the publisher.
1. Contera S. Nano Comes to Life: How Nanotechnology is Transforming Medicine and the Future of Biology. Princeton, NJ: Princeton University Press (2019).
2. Moradi Hasan-Abad A, Esmaili MA, Ghotaslou A, Atapour A, Khoshroo A, Naghian E. A review: electrochemical biosensors for testosterone detection. Anal Bioanal Electrochem. (2022) 14(11):1060–77.
3. Akbari M, Nejati M, Davoodabadi A, Kashi EA, Alam AN, Nasab AS. The antibacterial effects of terbium vanadate-silver peroxide nanostructures against surgical wounds infected by Staphylococcus aureus and Pseudomonas aeruginosa infections in a rat model. Inorg Chem Commun. (2023) 150:110390. doi: 10.1016/j.inoche.2023.110390
4. Rostami M, Rahimi-Nasrabadi M, Ghaderi A, Hajiabdollah A, Banafshe HR, Nasab AS. Znfe2o4@ L-cysteine-N/RGO as efficient nano-sonosensitizers, pH-responsive drug carriers and surface charge switchable drug delivery system for targeted chemo-sonodynamic therapy of cancer. Diamond Relat Mater. (2023) 133:109701. doi: 10.1016/j.diamond.2023.109701
5. Akbari M, Mohammadnia MS, Ghalkhani M, Aghaei M, Sohouli E, Rahimi-Nasrabadi M, et al. Development of an electrochemical fentanyl nanosensor based on MWCNT-HA/cu-H3BTC nanocomposite. J Ind Eng Chem. (2022) 114:418–26. doi: 10.1016/j.jiec.2022.07.032
6. Ghalkhani M, Khosrowshahi EM, Sohouli E, Eskandari K, Aghaei M, Rahimi-Nasrabadi M, et al. Electrochemical monitoring of carbamazepine in biological fluids by a glassy carbon electrode modified with CuO/ZnFe2O4/rGO nanocomposite. Surf Interfaces. (2022) 30:101943. doi: 10.1016/j.surfin.2022.101943
7. Bayda S, Adeel M, Tuccinardi T, Cordani M, Rizzolio F. The history of nanoscience and nanotechnology: from chemical–physical applications to nanomedicine. Molecules. (2019) 25(1):112. doi: 10.3390/molecules25010112
8. Ratner BD. Biomaterials: been there, done that, and evolving into the future. Annu Rev Biomed Eng. (2019) 21:171–91. doi: 10.1146/annurev-bioeng-062117-120940
9. Hofmann-Amtenbrink M, Grainger DW, Hofmann H. Nanoparticles in medicine: current challenges facing inorganic nanoparticle toxicity assessments and standardizations. Nanomed Nanotechnol Biol Med. (2015) 11(7):1689–94. doi: 10.1016/j.nano.2015.05.005
10. Muhamad N, Plengsuriyakarn T, Na-Bangchang K. Application of active targeting nanoparticle delivery system for chemotherapeutic drugs and traditional/herbal medicines in cancer therapy: a systematic review. Int J Nanomed. (2018) 13:3921. doi: 10.2147/IJN.S165210
11. Marks L, Peng L. Nanoparticle shape, thermodynamics and kinetics. J Phys Condens Matter. (2016) 28(5):053001. doi: 10.1088/0953-8984/28/5/053001
12. Kinnear C, Moore TL, Rodriguez-Lorenzo L, Rothen-Rutishauser B, Petri-Fink A. Form follows function: nanoparticle shape and its implications for nanomedicine. Chem Rev. (2017) 117(17):11476–521. doi: 10.1021/acs.chemrev.7b00194
13. Ventola CL. The antibiotic resistance crisis: part 1: causes and threats. Pharm Ther. (2015) 40(4):277.
14. O'Neill J. Review on antimicrobial resistance: tackling drug-resistant infections globally: final report and recommendations. Review on Antimicrobial Resistance: Tackling Drug-Resistant Infections Globally: Final Report and Recommendations. (2016).
15. O'neill J. Antimicrobial Resistance: Tackling a Crisis for The Health and Wealth of Nations. London: HM Government and Wellcome Trust (2014) p. 1–20.
16. Tacconelli E. Global Priority List of Antibiotic-Resistant Bacteria to Guide Research. Geneva: World Health Organization (2017) p. 1–7.
17. Mohammadi M, Moradi Hasan-Abad A, Dehghani P, Nabipour I, Roozbehani M, Hemphill A, et al. Dicentracin-like from Asian sea bass fish and moronecidine-like from hippocampus comes: two candidate antimicrobial peptides against leishmanina major infection. Int J Pept Res Ther. (2021) 27:769–78. doi: 10.1007/s10989-020-10125-4
18. Thomson RB Jr. Commentary: one small step for the gram stain, one giant leap for clinical microbiology. J Clin Microbiol. (2016) 54(6):1416–7. doi: 10.1128/JCM.00303-16
19. Scott JR, Barnett TC. Surface proteins of gram-positive bacteria and how they get there. Annu Rev Microbiol. (2006) 60:397–423. doi: 10.1146/annurev.micro.60.080805.142256
20. Suzuki T, Campbell J, Kim Y, Swoboda JG, Mylonakis E, Walker S, et al. Wall teichoic acid protects Staphylococcus aureus from inhibition by Congo red and other dyes. J Antimicrob Chemother. (2012) 67(9):2143–51. doi: 10.1093/jac/dks184
21. Huang KC, Mukhopadhyay R, Wen B, Gitai Z, Wingreen NS. Cell shape and cell-wall organization in gram-negative bacteria. Proc Natl Acad Sci U S A. (2008) 105(49):19282–7. doi: 10.1073/pnas.0805309105
22. Lee AS, De Lencastre H, Garau J, Kluytmans J, Malhotra-Kumar S, Peschel A, et al. Methicillin-resistant Staphylococcus aureus. Nat Rev Dis Primers. (2018) 4:18033. doi: 10.1038/nrdp.2018.33
23. Gatadi S, Madhavi Y, Nanduri S. Nanoparticle drug conjugates treating microbial and viral infections: a review. J Mol Struct. (2021) 1228:129750. doi: 10.1016/j.molstruc.2020.129750
24. Akbari M, Rahimi-Nasrabadi M, Eghbali-Arani M, Banafshe HR, Ahmadi F, Ganjali MR. Cdte quantum dots prepared using herbal species and microorganisms and their anti-cancer, drug delivery and antibacterial applications; a review. Ceram Int. (2020) 46(8):9979–89. doi: 10.1016/j.ceramint.2020.01.051
25. Marsooli MA, Nasrabadi MR, Fasihi-Ramandi M, Adib K, Eghbali M, Pourmasoud S, et al. Preparation of Fe3O4/SiO2/TiO2/PrVO4 nanocomposite in various molar ratios: investigation on photocatalytic performance on organic contaminate and bacterial environments, and anti-cancer properties. Polyhedron. (2020) 176:114239. doi: 10.1016/j.poly.2019.114239
26. Marsooli MA, Rahimi-Nasrabadi M, Fasihi-Ramandi M, Adib K, Eghbali-Arani M, Ahmadi F, et al. Preparation of Fe3O4/SiO2/TiO2/CeVO4 nanocomposites: investigation of photocatalytic effects on organic pollutants, bacterial environments, and new potential therapeutic candidate against cancer cells. Front Pharmacol. (2020) 11:192. doi: 10.3389/fphar.2020.00192
27. Chen C-W, Hsu C-Y, Lai S-M, Syu W-J, Wang T-Y, Lai P-S. Metal nanobullets for multidrug resistant bacteria and biofilms. Adv Drug Delivery Rev. (2014) 78:88–104. doi: 10.1016/j.addr.2014.08.004
28. Dizaj SM, Lotfipour F, Barzegar-Jalali M, Zarrintan MH, Adibkia K. Antimicrobial activity of the metals and metal oxide nanoparticles. Mat Sci Eng: C. (2014) 44:278–84. doi: 10.1016/j.msec.2014.08.031
29. Rudramurthy GR, Swamy MK, Sinniah UR, Ghasemzadeh A. Nanoparticles: alternatives against drug-resistant pathogenic microbes. Molecules. (2016) 21(7):836. doi: 10.3390/molecules21070836
30. Hemeg HA. Nanomaterials for alternative antibacterial therapy. Int J Nanomed. (2017) 12:8211. doi: 10.2147/IJN.S132163
31. Zaidi S, Misba L, Khan AU. Nano-therapeutics: a revolution in infection control in post antibiotic era. Nanomed Nanotechnol Biol Med. (2017) 13(7):2281–301. doi: 10.1016/j.nano.2017.06.015
32. Cavassin ED, de Figueiredo LFP, Otoch JP, Seckler MM, de Oliveira RA, Franco FF, et al. Comparison of methods to detect the in vitro activity of silver nanoparticles (AgNP) against multidrug resistant bacteria. J Nanobiotechnol. (2015) 13(1):1–16. doi: 10.1186/s12951-015-0120-6
33. Chatterjee AK, Chakraborty R, Basu T. Mechanism of antibacterial activity of copper nanoparticles. Nanotechnology. (2014) 25(13):135101. doi: 10.1088/0957-4484/25/13/135101
34. Vandebriel RJ, De Jong WH. A review of mammalian toxicity of ZnO nanoparticles. Nanotechnol Sci Appl. (2012) 5:61–71. doi: 10.2147/NSA.S23932
35. Richter E, Weizenegger M, Rüsch-Gerdes S, Niemann S. Evaluation of genotype MTBC assay for differentiation of clinical Mycobacterium tuberculosis complex isolates. J Clin Microbiol. (2003) 41(6):2672–5. doi: 10.1128/JCM.41.6.2672-2675.2003
36. Thoen CO, LoBue PA, Enarson DA. Tuberculosis in humans and animals: an overview. Int J Tuberc Lung Dis. (2010) 14(9):1075–8. 20819249
37. Tăbăran A-F, Matea CT, Mocan T, Tăbăran A, Mihaiu M, Iancu C, et al. Silver nanoparticles for the therapy of tuberculosis. Int J Nanomed. (2020) 15:2231–58. doi: 10.2147/IJN.S241183
38. Sathiyavimal S, Vasantharaj S, Bharathi D, Saravanan M, Manikandan E, Kumar SS, et al. Biogenesis of copper oxide nanoparticles (CuONPs) using Sida acuta and their incorporation over cotton fabrics to prevent the pathogenicity of gram negative and gram positive bacteria. J Photochem Photobiol, B. (2018) 188:126–34. doi: 10.1016/j.jphotobiol.2018.09.014
39. Shankar PD, Shobana S, Karuppusamy I, Pugazhendhi A, Ramkumar VS, Arvindnarayan S, et al. A review on the biosynthesis of metallic nanoparticles (gold and silver) using bio-components of microalgae: formation mechanism and applications. Enzyme Microb Technol. (2016) 95:28–44. doi: 10.1016/j.enzmictec.2016.10.015
40. Günther G. Multidrug-resistant and extensively drug-resistant tuberculosis: a review of current concepts and future challenges. Clin Med. (2014) 14(3):279. doi: 10.7861/clinmedicine.14-3-279
41. Gajendiran M, Yousuf SMJ, Elangovan V, Balasubramanian S. Gold nanoparticle conjugated PLGA–PEG–SA–PEG–PLGA multiblock copolymer nanoparticles: synthesis, characterization, in vivo release of rifampicin. J Mater Chem B. (2014) 2(4):418–27. doi: 10.1039/C3TB21113D
42. Mdluli P, Tetyana P, Sosibo N, van der Walt H, Mlambo M, Skepu A, et al. Gold nanoparticle based tuberculosis immunochromatographic assay: the quantitative ESE quanti analysis of the intensity of test and control lines. Biosens Bioelectron. (2014) 54:1–6. doi: 10.1016/j.bios.2013.10.019
43. Tsai T-T, Huang C-Y, Chen C-A, Shen S-W, Wang M-C, Cheng C-M, et al. Diagnosis of tuberculosis using colorimetric gold nanoparticles on a paper-based analytical device. ACS Sensors. (2017) 2(9):1345–54. doi: 10.1021/acssensors.7b00450
44. Gifford JC, Bresee J, Carter CJ, Wang G, Melander RJ, Melander C, et al. Thiol-modified gold nanoparticles for the inhibition of Mycobacterium smegmatis. Chem Commun. (2014) 50(100):15860–3. doi: 10.1039/C4CC06236A
45. Barroso TG, Martins RC, Fernandes E, Cardoso S, Rivas J, Freitas PP. Detection of BCG bacteria using a magnetoresistive biosensor: a step towards a fully electronic platform for tuberculosis point-of-care detection. Biosens Bioelectron. (2018) 100:259–65. doi: 10.1016/j.bios.2017.09.004
46. Kim J, Lee K-S, Kim EB, Paik S, Chang CL, Park TJ, et al. Early detection of the growth of Mycobacterium tuberculosis using magnetophoretic immunoassay in liquid culture. Biosens Bioelectron. (2017) 96:68–76. doi: 10.1016/j.bios.2017.04.025
47. Kim J, Jang M, Lee KG, Lee K-S, Lee SJ, Ro K-W, et al. Plastic-chip-based magnetophoretic immunoassay for point-of-care diagnosis of tuberculosis. ACS Appl Mater Interfaces. (2016) 8(36):23489–97. doi: 10.1021/acsami.6b06924
48. Zhou B, Zhu M, Hao Y, Yang P. Potential-resolved electrochemiluminescence for simultaneous determination of triple latent tuberculosis infection markers. ACS Appl Mater Interfaces. (2017) 9(36):30536–42. doi: 10.1021/acsami.7b10343
49. Lee C-N, Wang Y-M, Lai W-F, Chen T-J, Yu M-C, Fang C-L, et al. Super-paramagnetic iron oxide nanoparticles for use in extrapulmonary tuberculosis diagnosis. Clin Microbiol Infect. (2012) 18(6):E149–E57. doi: 10.1111/j.1469-0691.2012.03809.x
50. Saikia C, Hussain A, Ramteke A, Sharma HK, Maji TK. Crosslinked thiolated starch coated Fe3O4 magnetic nanoparticles: effect of montmorillonite and crosslinking density on drug delivery properties. Starch-Stärke. (2014) 66(7–8):760–71. doi: 10.1002/star.201300277
51. Hwang J, Son J, Seo Y, Jo Y, Lee K, Lee D, et al. Functional silica nanoparticles conjugated with beta-glucan to deliver anti-tuberculosis drug molecules. J Ind Eng Chem. (2018) 58:376–85. doi: 10.1016/j.jiec.2017.09.051
52. Clemens DL, Lee B-Y, Xue M, Thomas CR, Meng H, Ferris D, et al. Targeted intracellular delivery of antituberculosis drugs to Mycobacterium tuberculosis-infected macrophages via functionalized mesoporous silica nanoparticles. Antimicrob Agents Chemother. (2012) 56(5):2535–45. doi: 10.1128/AAC.06049-11
53. Pandey R, Khuller G. Oral nanoparticle-based antituberculosis drug delivery to the brain in an experimental model. J Antimicrob Chemother. (2006) 57(6):1146–52. doi: 10.1093/jac/dkl128
54. Pandey R, Khuller GK. Nanoparticle-based oral drug delivery system for an injectable antibiotic–streptomycin. Chemotherapy. (2007) 53(6):437–41. doi: 10.1159/000110009
55. Pandey R, Zahoor A, Sharma S, Khuller G. Nanoparticle encapsulated antitubercular drugs as a potential oral drug delivery system against murine tuberculosis. Tuberculosis. (2003) 83(6):373–8. doi: 10.1016/j.tube.2003.07.001
56. Sharma A, Sharma S, Khuller G. Lectin-functionalized poly (lactide-co-glycolide) nanoparticles as oral/aerosolized antitubercular drug carriers for treatment of tuberculosis. J Antimicrob Chemother. (2004) 54(4):761–6. doi: 10.1093/jac/dkh411
57. Kumar PV, Asthana A, Dutta T, Jain NK. Intracellular macrophage uptake of rifampicin loaded mannosylated dendrimers. J Drug Targeting. (2006) 14(8):546–56. doi: 10.1080/10611860600825159
58. Bellini RG, Guimarães AP, Pacheco MA, Dias DM, Furtado VR, de Alencastro RB, et al. Association of the anti-tuberculosis drug rifampicin with a PAMAM dendrimer. J Mol Graph Model. (2015) 60:34–42. doi: 10.1016/j.jmgm.2015.05.012
59. Rawal T, Parmar R, Tyagi RK, Butani S. Rifampicin loaded chitosan nanoparticle dry powder presents an improved therapeutic approach for alveolar tuberculosis. Colloids Surf B Biointerfaces. (2017) 154:321–30. doi: 10.1016/j.colsurfb.2017.03.044
60. Ansari N, Ghazvini K, Ramezani M, Shahdordizadeh M, Yazdian-Robati R, Abnous K, et al. Selection of DNA aptamers against Mycobacterium tuberculosis Ag85A, and its application in a graphene oxide-based fluorometric assay. Microchim Acta. (2018) 185:1–8. doi: 10.1007/s00604-017-2550-3
61. Chavda VP, Chen Y, Dave J, Chen Z-S, Chauhan SC, Yallapu MM, et al. COVID-19 and vaccination: myths vs science. Expert Rev Vaccines. (2022) 21(11):1603–20. doi: 10.1080/14760584.2022.2114900
62. Sisakht M, Mahmoodzadeh A, Zahedi M, Rostamzadeh D, Hasan-Abad AM, Atapour A. In silico approach for designing a novel recombinant fusion protein as a candidate vaccine against hpv. Curr Proteomics. (2021) 18(4):549–62. doi: 10.2174/1570164617999201014162235
63. Fenner F, Henderson DA, Arita I, Jezek Z, Ladnyi ID. Smallpox and its Eradication. Geneva: World Health Organization (1988).
64. Hajj Hussein I, Chams N, Chams S, El Sayegh S, Badran R, Raad M, et al. Vaccines through centuries: major cornerstones of global health. Front Public Health. (2015) 3:269. doi: 10.3389/fpubh.2015.00269
65. Pulendran B, Ahmed R. Translating innate immunity into immunological memory: implications for vaccine development. Cell. (2006) 124(4):849–63. doi: 10.1016/j.cell.2006.02.019
66. D’Amico C, Fontana F, Cheng R, Santos HA. Development of vaccine formulations: past, present, and future. Drug Deliv Transl Res. (2021) 11:353–72. doi: 10.1007/s13346-021-00924-7
67. Hasan-Abad AM, Adabi E, Sadroddiny E, Khorramizadeh MR, Mazlomi M, Mehravar S, et al. Functional deimmunization of interferon beta-1b by identifying and silencing human T cells epitopes. Iran J Allergy Asthma Immunol. (2019) 18(4):427–40. doi: 10.18502/ijaai.v18i4.1421
68. Reljic R, González-Fernández Á. Editorial: nanoparticle vaccines against infectious diseases. Front Immunol. (2019) 10:2615. doi: 10.3389/fimmu.2019.02615
69. Pati R, Shevtsov M, Sonawane A. Nanoparticle vaccines against infectious diseases. Front Immunol. (2018) 9:2224. doi: 10.3389/fimmu.2018.02224
70. Kheirollahpour M, Mehrabi M, Dounighi NM, Mohammadi M, Masoudi A. Nanoparticles and vaccine development. Pharm Nanotechnol. (2020) 8(1):6–21. doi: 10.2174/2211738507666191024162042
71. Chavda VP, Yao Q, Vora LK, Apostolopoulos V, Patel CA, Bezbaruah R, et al. Fast-track development of vaccines for SARS-CoV-2: the shots that saved the world. Front Immunol. (2022) 13:961198. doi: 10.3389/fimmu.2022.961198
72. Cai J, Wang H, Wang D, Li Y. Improving cancer vaccine efficiency by nanomedicine. Adv Biosyst. (2019) 3(3):1800287. doi: 10.1002/adbi.201800287
73. Mottram PL, Leong D, Crimeen-Irwin B, Gloster S, Xiang SD, Meanger J, et al. Type 1 and 2 immunity following vaccination is influenced by nanoparticle size: formulation of a model vaccine for respiratory syncytial virus. Mol Pharm. (2007) 4(1):73–84. doi: 10.1021/mp060096p
74. Karewicz A. Polymeric and liposomal nanocarriers for controlled drug delivery. In Biomaterials for Bone Regeneration. Woodhead Publishing (2014). p. 351–73. doi: 10.1533/9780857098104.3.351
75. Tian Y, Wang H, Liu Y, Mao L, Chen W, Zhu Z, et al. A peptide-based nanofibrous hydrogel as a promising DNA nanovector for optimizing the efficacy of HIV vaccine. Nano Lett. (2014) 14(3):1439–45. doi: 10.1021/nl404560v
76. Touzé A, Gaitan J, Arnold F, Cazal R, Fleury MJ, Combelas N, et al. Generation of merkel cell polyomavirus (MCV)-like particles and their application to detection of MCV antibodies. J Clin Microbiol. (2010) 48(5):1767–70. doi: 10.1128/JCM.01691-09
77. Kole S, Qadiri SSN, Shin S-M, Kim W-S, Lee J, Jung S-J. PLGA Encapsulated inactivated-viral vaccine: formulation and evaluation of its protective efficacy against viral haemorrhagic septicaemia virus (VHSV) infection in olive flounder (paralichthys olivaceus) vaccinated by mucosal delivery routes. Vaccine. (2019) 37(7):973–83. doi: 10.1016/j.vaccine.2018.12.063
78. Tao W, Ziemer KS, Gill HS. Gold nanoparticle–M2e conjugate coformulated with CpG induces protective immunity against influenza a virus. Nanomedicine. (2014) 9(2):237–51. doi: 10.2217/nnm.13.58
79. Thomas C, Rawat A, Hope-Weeks L, Ahsan F. Aerosolized PLA and PLGA nanoparticles enhance humoral, mucosal and cytokine responses to hepatitis B vaccine. Mol Pharm. (2011) 8(2):405–15. doi: 10.1021/mp100255c
80. Li P, Luo Z, Liu P, Gao N, Zhang Y, Pan H, et al. Bioreducible alginate-poly (ethylenimine) nanogels as an antigen-delivery system robustly enhance vaccine-elicited humoral and cellular immune responses. J Controlled Release. (2013) 168(3):271–9. doi: 10.1016/j.jconrel.2013.03.025
81. Olczak P, Roden RB. Progress in L2-based prophylactic vaccine development for protection against diverse human papillomavirus genotypes and associated diseases. Vaccines (Basel). (2020) 8(4):568. doi: 10.3390/vaccines8040568
82. Lomer MC, Hutchinson C, Volkert S, Greenfield SM, Catterall A, Thompson RP, et al. Dietary sources of inorganic microparticles and their intake in healthy subjects and patients with Crohn’s disease. Br J Nutr. (2004) 92(6):947–55. doi: 10.1079/BJN20041276
83. Bouwmeester H, Dekkers S, Noordam M, Hagens W, Bulder A, De Heer C, et al. Health impact of nanotechnologies in food production. RIKILT Report 2007014, RIVM rapport 000200702. (2007).
84. Chaudhry Q, Scotter M, Blackburn J, Ross B, Boxall A, Castle L, et al. Applications and implications of nanotechnologies for the food sector. Food Addit Contam. (2008) 25(3):241–58. doi: 10.1080/02652030701744538
85. Kohlhuber M, Winterhalter R, Dietrich S, Schenten J, Franz R, Kemmer D, et al. Nanomaterialien in Lebensmitteln und Verbraucherprodukten, Anwendungsbereiche, Analytik, rechtliche Rahmenbedingungen. Band 24 der Schriftenreihe Gesundheit und Umwelt. (2012).
86. Besinis A, De Peralta T, Tredwin CJ, Handy RD. Review of nanomaterials in dentistry: interactions with the oral microenvironment, clinical applications, hazards, and benefits. ACS nano. (2015) 9(3):2255–89. doi: 10.1021/nn505015e
87. Sreenivasalu PKP, Dora CP, Swami R, Jasthi VC, Shiroorkar PN, Nagaraja S, et al. Nanomaterials in dentistry: current applications and future scope. Nanomaterials. (2022) 12(10):1676. doi: 10.3390/nano12101676
88. Rupf S, Berger H, Buchter A, Harth V, Ong MF, Hannig M. Exposure of patient and dental staff to fine and ultrafine particles from scanning spray. Clin Oral Investig. (2015) 19:823–30. doi: 10.1007/s00784-014-1300-8
89. Murashov V. WHO guidelines on nanomaterials and workers’ health. BARENTS Б AP Е Н Ц. (2012) 15:43–4.
91. Akasaka T, Nakata K, Uo M, Watari F. Modification of the dentin surface by using carbon nanotubes. Bio-Med Mater Eng. (2009) 19(2–3):179–85. doi: 10.3233/BME-2009-0578
92. Sharifi S, Behzadi S, Laurent S, Forrest ML, Stroeve P, Mahmoudi M. Toxicity of nanomaterials. Chem Soc Rev. (2012) 41(6):2323–43. doi: 10.1039/C1CS15188F
93. Yin PT, Shah S, Chhowalla M, Lee K-B. Design, synthesis, and characterization of graphene–nanoparticle hybrid materials for bioapplications. Chem Rev. (2015) 115(7):2483–531. doi: 10.1021/cr500537t
94. Sarosi C, Biris AR, Antoniac A, Boboia S, Alb C, Antoniac I, et al. The nanofiller effect on properties of experimental graphene dental nanocomposites. J Adhes Sci Technol. (2016) 30(16):1779–94. doi: 10.1080/01694243.2016.1161969
95. Pepla E, Besharat LK, Palaia G, Tenore G, Migliau G. Nano-hydroxyapatite and its applications in preventive, restorative and regenerative dentistry: a review of literature. Ann Stomatol (Roma). (2014) 5(3):108–14. 25506416.25506416
96. Wang J, Wang L, Fan Y. Adverse biological effect of TiO2 and hydroxyapatite nanoparticles used in bone repair and replacement. Int J Mol Sci. (2016) 17(6):798. doi: 10.3390/ijms17060798
97. Asadpour E, Sadeghnia HR, Ghorbani A, Boroushaki MT. Effect of zirconium dioxide nanoparticles on glutathione peroxidase enzyme in PC12 and N2a cell lines. Iran J Pharm Res. (2014) 13(4):1141–8. 25587301.25587301
98. Wang ML, Tuli R, Manner PA, Sharkey PF, Hall DJ, Tuan RS. Direct and indirect induction of apoptosis in human mesenchymal stem cells in response to titanium particles. J Orthop Res. (2003) 21(4):697–707. doi: 10.1016/S0736-0266(02)00241-3
99. Arefian Z, Pishbin F, Negahdary MA, Ajdary M. Potential toxic effects of zirconia oxide nanoparticles on liver and kidney factors. Biomed Res. (2015) 26(1):89–97.
100. Murugadoss S, Lison D, Godderis L, Van Den Brule S, Mast J, Brassinne F, et al. Toxicology of silica nanoparticles: an update. Arch Toxicol. (2017) 91(9):2967–3010. doi: 10.1007/s00204-017-1993-y
101. Ahamed M. Silica nanoparticles-induced cytotoxicity, oxidative stress and apoptosis in cultured A431 and A549 cells. Hum Exp Toxicol. (2013) 32(2):186–95. doi: 10.1177/0960327112459206
102. Chu Z, Huang Y, Li L, Tao Q, Li Q. Physiological pathway of human cell damage induced by genotoxic crystalline silica nanoparticles. Biomaterials. (2012) 33(30):7540–6. doi: 10.1016/j.biomaterials.2012.06.073
103. Shi H, Magaye R, Castranova V, Zhao J. Titanium dioxide nanoparticles: a review of current toxicological data. Part Fibre Toxicol. (2013) 10:1–33. doi: 10.1186/1743-8977-10-1
104. Hext PM, Tomenson JA, Thompson P. Titanium dioxide: inhalation toxicology and epidemiology. Ann Occup Hyg. (2005) 49(6):461–72. doi: 10.1093/annhyg/mei012
105. Baan R, Straif K, Grosse Y, Secretan B, El Ghissassi F, Cogliano V. Carcinogenicity of carbon black, titanium dioxide, and talc. Lancet Oncol. (2006) 7(4):295–6. doi: 10.1016/S1470-2045(06)70651-9
106. Katsumiti A, Gilliland D, Arostegui I, Cajaraville MP. Mechanisms of toxicity of ag nanoparticles in comparison to bulk and ionic ag on mussel hemocytes and gill cells. PloS One. (2015) 10(6):e0129039. doi: 10.1371/journal.pone.0129039
107. Beer C, Foldbjerg R, Hayashi Y, Sutherland DS, Autrup H. Toxicity of silver nanoparticles—nanoparticle or silver ion? Toxicol Lett. (2012) 208(3):286–92. doi: 10.1016/j.toxlet.2011.11.002
108. Keyvani V, Riahi E, Yousefi M, Esmaeili S-A, Shafabakhsh R, Moradi Hasan-Abad A, et al. Gynecologic cancer, cancer stem cells, and possible targeted therapies. Front Pharmacol. (2022) 13:823572. doi: 10.3389/fphar.2022.823572
109. Jafari S, Ravan M, Karimi-Sani I, Aria H, Hasan-Abad AM, Banasaz B, et al. Screening and identification of potential biomarkers for pancreatic cancer: an integrated bioinformatics analysis. Pathol Res Pract. (2023) 249:154726. doi: 10.1016/j.prp.2023.154726
110. Wu S, Zhu W, Thompson P, Hannun YA. Evaluating intrinsic and non-intrinsic cancer risk factors. Nat Commun. (2018) 9(1):3490. doi: 10.1038/s41467-018-05467-z
111. Quazi S. Telomerase gene therapy: a remission toward cancer. Med Oncol. (2022) 39(6):105. doi: 10.1007/s12032-022-01702-2
112. Anand P, Kunnumakara AB, Sundaram C, Harikumar KB, Tharakan ST, Lai OS, et al. Cancer is a preventable disease that requires major lifestyle changes. Pharm Res. (2008) 25:2097–116. doi: 10.1007/s11095-008-9661-9
113. Park W, Heo Y-J, Han DK. New opportunities for nanoparticles in cancer immunotherapy. Biomater Res. (2018) 22:1–10. doi: 10.1186/s40824-017-0112-8
114. Jovčevska I, Muyldermans S. The therapeutic potential of nanobodies. BioDrugs Clin Immunotherap Biopharm Gene Therapy. (2020) 34(1):11–26. doi: 10.1007/s40259-019-00392-z
115. Zitvogel L, Apetoh L, Ghiringhelli F, Kroemer G. Immunological aspects of cancer chemotherapy. Nat Rev Immunol. (2008) 8(1):59–73. doi: 10.1038/nri2216
116. Chan H-K, Ismail S. Side effects of chemotherapy among cancer patients in a Malaysian general hospital: experiences, perceptions and informational needs from clinical pharmacists. Asian Pac J Cancer Prev. (2014) 15(13):5305–9. doi: 10.7314/APJCP.2014.15.13.5305
117. Quazi S. Artificial intelligence and machine learning in precision and genomic medicine. Med Oncol. (2022) 39(8):120. doi: 10.1007/s12032-022-01711-1
118. Mahapatro A, Singh DK. Biodegradable nanoparticles are excellent vehicle for site directed in-vivo delivery of drugs and vaccines. J Nanobiotechnol. (2011) 9:1–11. doi: 10.1186/1477-3155-9-55
119. Kroemer G, Zitvogel L. The breakthrough of the microbiota. Nat Rev Immunol. (2018) 18(2):87–8. doi: 10.1038/nri.2018.4
120. Rosenberg SA, Restifo NP, Yang JC, Morgan RA, Dudley ME. Adoptive cell transfer: a clinical path to effective cancer immunotherapy. Nat Rev Cancer. (2008) 8(4):299–308. doi: 10.1038/nrc2355
121. Melero I, Rouzaut A, Motz GT, Coukos G. T-cell and NK-cell infiltration into solid tumors: a key limiting factor for efficacious cancer immunotherapy. Cancer Discov. (2014) 4(5):522–6. doi: 10.1158/2159-8290.CD-13-0985
122. Mohammadi AH, Sobhani-Nasab A, Nejati M, Hadi S, Behjati M, Mirzaii-Dizgah I, et al. Preparation and characterization of CuO, Ag2O and ZnO nanoparticles and investigation of their antibacterial and anticancer properties on HCT-116 and C26 cells. Inorg Chem Commun. (2023) 149:110404. doi: 10.1016/j.inoche.2023.110404
123. Malehmir S, Abedini A, Sobhani-Nasab A, Eshraghi R, Akbari M, Atapour A, et al. A review of biogenic routes for the manufacture of manganese oxide nanostructures and its anti-cancer, drug delivery, anti-bacterial, and bioimaging potentialsmanrathanami. Inorg Chem Commun. (2023) 156:111306. doi: 10.1016/j.inoche.2023.111306
124. Malehmir S, Esmaili MA, Mahabady MK, Sobhani-Nasab A, Atapour A, Ganjali MR, et al. A review: hemocompatibility of magnetic nanoparticles and their regenerative medicine, cancer therapy, drug delivery, and bioimaging applications. Front Chem. (2023) 11:1249134. doi: 10.3389/fchem.2023.1249134
125. Nejati M, Rostami M, Mirzaei H, Rahimi-Nasrabadi M, Vosoughifar M, Nasab AS, et al. Green methods for the preparation of MgO nanomaterials and their drug delivery, anti-cancer and anti-bacterial potentials: a review. Inorg Chem Commun. (2022) 136:109107. doi: 10.1016/j.inoche.2021.109107
126. Behvandi S, Sobhani-Nasab A, Karimi MA, Sohouli E, Karimi M, Ganjali MR, et al. Synthesis and characterization of Sm2 (MoO4) 3, Sm2 (MoO4) 3/GO and Sm2 (MoO4) 3/C3N4 nanostructures for improved photocatalytic performance and their anti-cancer the MCF-7 cells. Polyhedron. (2020) 180:114424. doi: 10.1016/j.poly.2020.114424
127. Peymani-Motlagh SM, Moeinian N, Rostami M, Fasihi-Ramandi M, Sobhani-Nasab A, Rahimi-Nasrabadi M, et al. Effect of Gd3+-, Pr3+-or Sm3+-substituted cobalt–zinc ferrite on photodegradation of methyl orange and cytotoxicity tests. J Rare Earths. (2019) 37(12):1288–95. doi: 10.1016/j.jre.2019.04.010
128. Gandomi F, Peymani-Motlagh SM, Rostami M, Sobhani-Nasab A, Fasihi-Ramandi M, Eghbali-Arani M, et al. Simple synthesis and characterization of li 0.5 fe 2.5 O 4, LiMg 0.5 fe 2 O 4 and LiNi 0.5 fe 2 O 4, and investigation of their photocatalytic and anticancer properties on hela cells line. J Mater Sci: Mater Electron. (2019) 30:19691–702. doi: 10.1007/s10854-019-02320-x
129. Peymani-Motlagh SM, Sobhani-Nasab A, Rostami M, Sobati H, Eghbali-Arani M, Fasihi-Ramandi M, et al. Assessing the magnetic, cytotoxic and photocatalytic influence of incorporating yb 3+ or pr 3+ ions in cobalt–nickel ferrite. J Mater Sci: Mater Electron. (2019) 30:6902–9. doi: 10.1007/s10854-019-01005-9
130. Lacouture M, Sibaud V. Toxic side effects of targeted therapies and immunotherapies affecting the skin, oral mucosa, hair, and nails. Am J Clin Dermatol. (2018) 19(Suppl 1):31–9. doi: 10.1007/s40257-018-0384-3
131. Dadwal A, Baldi A, Kumar Narang R. Nanoparticles as carriers for drug delivery in cancer. Artif Cells Nanomed Biotechnol. (2018) 46(sup2):295–305. doi: 10.1080/21691401.2018.1457039
132. Palazzolo S, Bayda S, Hadla M, Caligiuri I, Corona G, Toffoli G, et al. The clinical translation of organic nanomaterials for cancer therapy: a focus on polymeric nanoparticles, micelles, liposomes and exosomes. Curr Med Chem. (2018) 25(34):4224–68. doi: 10.2174/0929867324666170830113755
133. Li W, Zhang H, Assaraf YG, Zhao K, Xu X, Xie J, et al. Overcoming ABC transporter-mediated multidrug resistance: molecular mechanisms and novel therapeutic drug strategies. Drug Resist Updates. (2016) 27:14–29. doi: 10.1016/j.drup.2016.05.001
134. Tiwari JN, Tiwari RN, Kim KS. Zero-dimensional, one-dimensional, two-dimensional and three-dimensional nanostructured materials for advanced electrochemical energy devices. Prog Mater Sci. (2012) 57(4):724–803. doi: 10.1016/j.pmatsci.2011.08.003
135. Prokop A, Davidson JM. Nanovehicular intracellular delivery systems. J Pharm Sci. (2008) 97(9):3518–90. doi: 10.1002/jps.21270
136. Bakshi S, Zakharchenko A, Minko S, Kolpashchikov DM, Katz E. Towards nanomaterials for cancer theranostics: a system of DNA-modified magnetic nanoparticles for detection and suppression of RNA marker in cancer cells. Magnetochemistry. (2019) 5(2):24. doi: 10.3390/magnetochemistry5020024
137. González-Ballesteros N, Diego-González L, Lastra-Valdor M, Rodríguez-Argüelles M, Grimaldi M, Cavazza A, et al. Immunostimulant and biocompatible gold and silver nanoparticles synthesized using the ulva intestinalis L. Aqueous extract. J Mater Chem B. (2019) 7(30):4677–91. doi: 10.1039/C9TB00215D
138. Bhowmik T, Gomes A. Down–regulation of cyclin–dependent kinase-4 and MAPK through estrogen receptor mediated cell cycle arrest in human breast cancer induced by gold nanoparticle tagged toxin protein NKCT1. Chem-Biol Interact. (2017) 268:119–28. doi: 10.1016/j.cbi.2017.03.009
139. Ma Y-C, Zhu Y-H, Tang X-F, Hang L-F, Jiang W, Li M, et al. Au nanoparticles with enzyme-mimicking activity-ornamented ZIF-8 for highly efficient photodynamic therapy. Biomater Sci. (2019) 7(7):2740–8. doi: 10.1039/C9BM00333A
140. Nebu J, Devi JA, Aparna R, Abha K, Sony G. Erlotinib conjugated gold nanocluster enveloped magnetic iron oxide nanoparticles–A targeted probe for imaging pancreatic cancer cells. Sens Actuators, B. (2018) 257:1035–43. doi: 10.1016/j.snb.2017.11.017
141. Zhang T, Li Y, Hong W, Chen Z, Peng P, Yuan S, et al. Glucose oxidase and polydopamine functionalized iron oxide nanoparticles: combination of the photothermal effect and reactive oxygen species generation for dual-modality selective cancer therapy. J Mater Chem B. (2019) 7(13):2190–200. doi: 10.1039/C8TB03320J
142. Das S, Roy A, Barui AK, Alabbasi MMA, Kuncha M, Sistla R, et al. Anti-angiogenic vanadium pentoxide nanoparticles for the treatment of melanoma and their in vivo toxicity study. Nanoscale. (2020) 12(14):7604–21. doi: 10.1039/D0NR00631A
143. Wang P, Liu S, Hu M, Zhang H, Duan D, He J, et al. Peroxidase-like nanozymes induce a novel form of cell death and inhibit tumor growth in vivo. Adv Funct Mater. (2020) 30(21):2000647. doi: 10.1002/adfm.202000647
144. Chen M, Deng G, He Y, Li X, Liu W, Wang W, et al. Ultrasound-enhanced generation of reactive oxygen species for MRI-guided tumor therapy by the fe@ Fe3O4-based peroxidase-mimicking nanozyme. ACS Appl Bio Mater. (2019) 3(1):639–47. doi: 10.1021/acsabm.9b01006
145. Miao Z, Jiang S, Ding M, Sun S, Ma Y, Younis MR, et al. Ultrasmall rhodium nanozyme with RONS scavenging and photothermal activities for anti-inflammation and antitumor theranostics of colon diseases. Nano Lett. (2020) 20(5):3079–89. doi: 10.1021/acs.nanolett.9b05035
146. Wu F, Zhu J, Li G, Wang J, Veeraraghavan VP, Krishna Mohan S, et al. Biologically synthesized green gold nanoparticles from siberian ginseng induce growth-inhibitory effect on melanoma cells (B16). Artif Cells Nanomed Biotechnol. (2019) 47(1):3297–305. doi: 10.1080/21691401.2019.1647224
147. Khiavi MA, Safary A, Barar J, Farzi-Khajeh H, Barzegari A, Mousavi R, et al. PEGylated gold nanoparticles-ribonuclease induced oxidative stress and apoptosis in colorectal cancer cells. BioImpacts: BI. (2020) 10(1):27. doi: 10.15171/bi.2020.04
148. Chen C-C, Hsieh D-S, Huang K-J, Chan Y-L, Hong P-D, Yeh M-K, et al. Improving anticancer efficacy of (–)-epigallocatechin-3-gallate gold nanoparticles in murine B16F10 melanoma cells. Drug Des Devel Ther. (2014) 8:459–74. doi: 10.2147/DDDT.S58414
149. Al-Sheddi ES, Farshori NN, Al-Oqail MM, Al-Massarani SM, Saquib Q, Wahab R, et al. Anticancer potential of green synthesized silver nanoparticles using extract of Nepeta deflersiana against human cervical cancer cells (HeLA). Bioinorg Chem Appl. (2018) 8:459–74. doi: 10.2147/DDDT.S58414
150. Du Y, Zhang J, Yan S, Tao Z, Wang C, Huang M, et al. PEGylated zinc oxide nanoparticles induce apoptosis in pancreatic cancer cells through reactive oxygen species. IET Nanobiotechnol. (2019) 13(5):536–40. doi: 10.1049/iet-nbt.2018.5327
151. Shen C, James SA, de Jonge MD, Turney TW, Wright PF, Feltis BN. Relating cytotoxicity, zinc ions, and reactive oxygen in ZnO nanoparticle–exposed human immune cells. Toxicol Sci. (2013) 136(1):120–30. doi: 10.1093/toxsci/kft187
152. Dobrucka R, Romaniuk-Drapała A, Kaczmarek M. Evaluation of biological synthesized platinum nanoparticles using Ononidis radix extract on the cell lung carcinoma A549. Biomed Microdevices. (2019) 21:1–10. doi: 10.1007/s10544-019-0424-7
153. Fujiwara R, Luo Y, Sasaki T, Fujii K, Ohmori H, Kuniyasu H. Cancer therapeutic effects of titanium dioxide nanoparticles are associated with oxidative stress and cytokine induction. Pathobiology. (2015) 82(6):243–51. doi: 10.1159/000439404
154. Murugan C, Murugan N, Sundramoorthy AK, Sundaramurthy A. Nanoceria decorated flower-like molybdenum sulphide nanoflakes: an efficient nanozyme for tumour selective ROS generation and photo thermal therapy. Chem Commun. (2019) 55(55):8017–20. doi: 10.1039/C9CC03763B
155. Nejdl L, Kudr J, Moulick A, Hegerova D, Ruttkay-Nedecky B, Gumulec J, et al. Platinum nanoparticles induce damage to DNA and inhibit DNA replication. PLoS One. (2017) 12(7):e0180798. doi: 10.1371/journal.pone.0180798
156. Nourmohammadi E, Khoshdel-Sarkarizi H, Nedaeinia R, Sadeghnia HR, Hasanzadeh L, Darroudi M, et al. Evaluation of anticancer effects of cerium oxide nanoparticles on mouse fibrosarcoma cell line. J Cell Physiol. (2019) 234(4):4987–96. doi: 10.1002/jcp.27303
157. Parvathya S, Venkatramanb B. In vitro antibacterial and anticancer potential of CeO2 nanoparticles prepared by co-precipitation and green synthesis method. J Nanosci Curr Res. (2017) 2(02):1–9. doi: 10.4172/2572-0813.1000111
158. Ahamed M, Khan MM, Akhtar MJ, Alhadlaq HA, Alshamsan A. Ag-doping regulates the cytotoxicity of TiO2 nanoparticles via oxidative stress in human cancer cells. Sci Rep. (2017) 7(1):17662. doi: 10.1038/s41598-017-17559-9
159. Ferrari R, Sponchioni M, Morbidelli M, Moscatelli D. Polymer nanoparticles for the intravenous delivery of anticancer drugs: the checkpoints on the road from the synthesis to clinical translation. Nanoscale. (2018) 10(48):22701–19. doi: 10.1039/C8NR05933K
161. Voet S, Srinivasan S, Lamkanfi M, van Loo G. Inflammasomes in neuroinflammatory and neurodegenerative diseases. EMBO Mol Med. (2019) 11(6):e10248. doi: 10.15252/emmm.201810248
162. Asefy Z, Hoseinnejhad S, Ceferov Z. Nanoparticles approaches in neurodegenerative diseases diagnosis and treatment. Neurol Sci. (2021) 42:2653–60. doi: 10.1007/s10072-021-05234-x
163. Cano A, Sánchez-López E, Ettcheto M, López-Machado A, Espina M, Souto EB, et al. Current advances in the development of novel polymeric nanoparticles for the treatment of neurodegenerative diseases. Nanomedicine. (2020) 15(12):1239–61. doi: 10.2217/nnm-2019-0443
164. Titze-de-Almeida R, Titze-de-Almeida SS, Ferreira NR, Fontanari C, Faccioli LH, Del Bel E. Suppressing nNOS enzyme by small-interfering RNAs protects SH-SY5Y cells and nigral dopaminergic neurons from 6-OHDA injury. Neurotox Res. (2019) 36:117–31. doi: 10.1007/s12640-019-00043-9
165. Tacconelli E, Carrara E, Savoldi A, Harbarth S, Mendelson M, Monnet DL, et al. Discovery, research, and development of new antibiotics: the WHO priority list of antibiotic-resistant bacteria and tuberculosis. Lancet Infect Dis. (2018) 18(3):318–27. doi: 10.1016/S1473-3099(17)30753-3
166. Yue P, Miao W, Gao L, Zhao X, Teng J. Ultrasound-triggered effects of the microbubbles coupled to GDNF plasmid-loaded PEGylated liposomes in a rat model of Parkinson’s disease. Front Neurosci. (2018) 12:222. doi: 10.3389/fnins.2018.00222
167. Kuo Y-C, Tsao C-W. Neuroprotection against apoptosis of SK-N-MC cells using RMP-7-and lactoferrin-grafted liposomes carrying quercetin. Int J Nanomed. (2017) 12:2857. doi: 10.2147/IJN.S132472
168. Kuo Y-C, Lee Y-J. Rescuing cholinergic neurons from apoptotic degeneration by targeting of serotonin modulator-and apolipoprotein E-conjugated liposomes to the hippocampus. Int J Nanomed. (2016) 11:6809. doi: 10.2147/IJN.S123442
169. Sintov AC, Levy HV, Greenberg I. Continuous transdermal delivery of L-DOPA based on a self-assembling nanomicellar system. Pharm Res. (2017) 34:1459–68. doi: 10.1007/s11095-017-2162-y
170. Chen T, Li Y, Li C, Yi X, Wang R, Lee SM-Y, et al. Pluronic P85/F68 micelles of baicalein could interfere with mitochondria to overcome MRP2-mediated efflux and offer improved anti-parkinsonian activity. Mol Pharm. (2017) 14(10):3331–42. doi: 10.1021/acs.molpharmaceut.7b00374
171. Yang H, Li X, Zhu L, Wu X, Zhang S, Huang F, et al. Heat shock protein inspired nanochaperones restore amyloid-β homeostasis for preventative therapy of Alzheimer’s disease. Adv Sci. (2019) 6(22):1901844. doi: 10.1002/advs.201901844
172. Yang P, Sheng D, Guo Q, Wang P, Xu S, Qian K, et al. Neuronal mitochondria-targeted micelles relieving oxidative stress for delayed progression of Alzheimer’s disease. Biomaterials. (2020) 238:119844. doi: 10.1016/j.biomaterials.2020.119844
173. Mirzaie Z, Ansari M, Kordestani SS, Rezaei MH, Mozafari M. Preparation and characterization of curcumin-loaded polymeric nanomicelles to interference with amyloidogenesis through glycation method. Biotechnol Appl Biochem. (2019) 66(4):537–44. doi: 10.1002/bab.1751
174. Igartúa DE, Martinez CS, Temprana CF, Alonso S, Prieto MJ. PAMAM Dendrimers as a carbamazepine delivery system for neurodegenerative diseases: a biophysical and nanotoxicological characterization. Int J Pharm. (2018) 544(1):191–202. doi: 10.1016/j.ijpharm.2018.04.032
175. Sobhani-Nasab A, Behpour M, Rahimi-Nasrabadi M, Ahmadi F, Pourmasoud S. New method for synthesis of BaFe12O19/Sm2Ti2O7 and BaFe12O19/Sm2Ti2O7/ag nano-hybrid and investigation of optical and photocatalytic properties. J Mater Sci: Mater Electron. (2019) 30(6):5854–65. doi: 10.1007/s10854-019-00883-3
176. Gothwal A, Kumar H, Nakhate KT, Ajazuddin Dutta A, Borah A, et al. Lactoferrin coupled lower generation PAMAM dendrimers for brain targeted delivery of memantine in aluminum-chloride-induced Alzheimer’s disease in mice. Bioconjugate Chem. (2019) 30(10):2573–83. doi: 10.1021/acs.bioconjchem.9b00505
177. Milowska K, Grochowina J, Katir N, El Kadib A, Majoral J-P, Bryszewska M, et al. Viologen-phosphorus dendrimers inhibit α-synuclein fibrillation. Mol Pharm. (2013) 10(3):1131–7. doi: 10.1021/mp300636h
178. Mominur Rahman M, Islam F, Saidur Rahaman M, Sultana NA, Fahim NF, Ahmed M. Studies on the prevalence of HIV/AIDS in Bangladesh including other developing countries. Adv Tradit Med. (2023) 23:647–58. doi: 10.1007/s13596-021-00610-6
179. Milowska K, Szwed A, Mutrynowska M, Gomez-Ramirez R, de la Mata FJ, Gabryelak T, et al. Carbosilane dendrimers inhibit α-synuclein fibrillation and prevent cells from rotenone-induced damage. Int J Pharm. (2015) 484(1–2):268–75. doi: 10.1016/j.ijpharm.2015.02.066
180. Pahuja R, Seth K, Shukla A, Shukla RK, Bhatnagar P, Chauhan LKS, et al. Trans-blood brain barrier delivery of dopamine-loaded nanoparticles reverses functional deficits in parkinsonian rats. ACS Nano. (2015) 9(5):4850–71. doi: 10.1021/nn506408v
181. Moradian H, Keshvari H, Fasehee H, Dinarvand R, Faghihi S. Combining NT3-overexpressing MSCs and PLGA microcarriers for brain tissue engineering: a potential tool for treatment of Parkinson’s disease. Mater Sci Eng C. (2017) 76:934–43. doi: 10.1016/j.msec.2017.02.178
182. Rodríguez-Nogales C, Garbayo E, Martínez-Valbuena I, Sebastián V, Luquin M, Blanco-Prieto M. Development and characterization of polo-like kinase 2 loaded nanoparticles-A novel strategy for (serine-129) phosphorylation of alpha-synuclein. Int J Pharm. (2016) 514(1):142–9. doi: 10.1016/j.ijpharm.2016.06.044
183. Cui N, Lu H, Li M. Magnetic nanoparticles associated PEG/PLGA block copolymer targeted with anti-transferrin receptor antibodies for Alzheimer’s disease. J Biomed Nanotechnol. (2018) 14(5):1017–24. doi: 10.1166/jbn.2018.2512
184. Meng D-L, Shang L, Feng X-H, Huang X-F, Che X. Xanthoceraside hollow gold nanoparticles, green pharmaceutics preparation for poorly water-soluble natural anti-AD medicine. Int J Pharm. (2016) 506(1–2):184–90. doi: 10.1016/j.ijpharm.2016.04.042
185. Garcia-Leis A, Torreggiani A, Garcia-Ramos JV, Sanchez-Cortes S. Hollow au/ag nanostars displaying broad plasmonic resonance and high surface-enhanced Raman sensitivity. Nanoscale. (2015) 7(32):13629–37. doi: 10.1039/C5NR02819A
186. Liu Y, Zhou H, Yin T, Gong Y, Yuan G, Chen L, et al. Quercetin-modified gold-palladium nanoparticles as a potential autophagy inducer for the treatment of Alzheimer’s disease. J Colloid Interface Sci. (2019) 552:388–400. doi: 10.1016/j.jcis.2019.05.066
187. Liu D, Li W, Jiang X, Bai S, Liu J, Liu X, et al. Using near-infrared enhanced thermozyme and scFv dual-conjugated au nanorods for detection and targeted photothermal treatment of Alzheimer’s disease. Theranostics. (2019) 9(8):2268. doi: 10.7150/thno.30649
188. Ji D, Xu N, Liu Z, Shi Z, Low SS, Liu J, et al. Smartphone-based differential pulse amperometry system for real-time monitoring of levodopa with carbon nanotubes and gold nanoparticles modified screen-printing electrodes. Biosens Bioelectron. (2019) 129:216–23. doi: 10.1016/j.bios.2018.09.082
189. Tisch U, Aluf Y, Ionescu R, Nakhleh M, Bassal R, Axelrod N, et al. Detection of asymptomatic nigrostriatal dopaminergic lesion in rats by exhaled air analysis using carbon nanotube sensors. ACS Chem Neurosci. (2012) 3(3):161–6. doi: 10.1021/cn200093r
190. Marei HE, Elnegiry AA, Zaghloul A, Althani A, Afifi N, Abd-Elmaksoud A, et al. Nanotubes impregnated human olfactory bulb neural stem cells promote neuronal differentiation in trimethyltin-induced neurodegeneration rat model. J Cell Physiol. (2017) 232(12):3586–97. doi: 10.1002/jcp.25826
191. Guo Q, You H, Yang X, Lin B, Zhu Z, Lu Z, et al. Functional single-walled carbon nanotubes ‘CAR’for targeting dopamine delivery into the brain of parkinsonian mice. Nanoscale. (2017) 9(30):10832–45. doi: 10.1039/C7NR02682J
192. Derakhshankhah H, Hajipour MJ, Barzegari E, Lotfabadi A, Ferdousi M, Saboury AA, et al. Zeolite nanoparticles inhibit aβ–fibrinogen interaction and formation of a consequent abnormal structural clot. ACS Appl Mater Interfaces. (2016) 8(45):30768–79. doi: 10.1021/acsami.6b10941
193. Swar S, Máková V, Stibor I. Effectiveness of diverse mesoporous silica nanoparticles as potent vehicles for the drug L-DOPA. Materials (Basel). (2019) 12(19):3202. doi: 10.3390/ma12193202
194. Dvir T, Timko BP, Kohane DS, Langer R. Nanotechnological strategies for engineering complex tissues. Nat Nanotechnol. (2011) 6(1):13–22. doi: 10.1038/nnano.2010.246
195. Mi P, Kokuryo D, Cabral H, Wu H, Terada Y, Saga T, et al. A pH-activatable nanoparticle with signal-amplification capabilities for non-invasive imaging of tumour malignancy. Nat Nanotechnol. (2016) 11(8):724–30. doi: 10.1038/nnano.2016.72
196. Bahal R, Ali McNeer N, Quijano E, Liu Y, Sulkowski P, Turchick A, et al. In vivo correction of anaemia in β-thalassemic mice by γPNA-mediated gene editing with nanoparticle delivery. Nat Commun. (2016) 7(1):13304. doi: 10.1038/ncomms13304
197. Cheng CJ, Tietjen GT, Saucier-Sawyer JK, Saltzman WM. A holistic approach to targeting disease with polymeric nanoparticles. Nat Rev Drug Discovery. (2015) 14(4):239–47. doi: 10.1038/nrd4503
198. Wang Q, Xia B, Xu J, Niu X, Cai J, Shen Q, et al. Biocompatible small organic molecule phototheranostics for NIR-II fluorescence/photoacoustic imaging and simultaneous photodynamic/photothermal combination therapy. Mater Chem Front. (2019) 3(4):650–5. doi: 10.1039/C9QM00036D
199. Ryu JH, Koo H, Sun I-C, Yuk SH, Choi K, Kim K, et al. Tumor-targeting multi-functional nanoparticles for theragnosis: new paradigm for cancer therapy. Adv Drug Delivery Rev. (2012) 64(13):1447–58. doi: 10.1016/j.addr.2012.06.012
200. Fathi-Achachelouei M, Knopf-Marques H, Ribeiro da Silva CE, Barthès J, Bat E, Tezcaner A, et al. Use of nanoparticles in tissue engineering and regenerative medicine. Front Bioeng Biotechnol. (2019) 7:113. doi: 10.3389/fbioe.2019.00113
201. Li K, Li D, Li C-H, Zhuang P, Dai C, Hu X, et al. Retraction: efficient in vivo wound healing using noble metal nanoclusters. Nanoscale. (2022) 14(10):3972. doi: 10.1039/D2NR90041F
202. Alexander JW. History of the medical use of silver. Surg Infect (Larchmt). (2009) 10(3):289–92. doi: 10.1089/sur.2008.9941
203. Paiva-Santos AC, Herdade AM, Guerra C, Peixoto D, Pereira-Silva M, Zeinali M, et al. Plant-mediated green synthesis of metal-based nanoparticles for dermopharmaceutical and cosmetic applications. Int J Pharm. (2021) 597:120311. doi: 10.1016/j.ijpharm.2021.120311
204. Arafa MG, El-Kased RF, Elmazar MM. Thermoresponsive gels containing gold nanoparticles as smart antibacterial and wound healing agents. Sci Rep. (2018) 8(1):13674. doi: 10.1038/s41598-018-31895-4
205. Taufikurohmah T, Sanjaya IGM, Syahrani A. Nanogold synthesis using matrix mono glyceryl stearate as antiaging compounds in modern cosmetics. J Mater Sci Eng A. (2011) 1(6A):857.
206. Ovais M, Ahmad I, Khalil AT, Mukherjee S, Javed R, Ayaz M, et al. Wound healing applications of biogenic colloidal silver and gold nanoparticles: recent trends and future prospects. Appl Microbiol Biotechnol. (2018) 102:4305–18. doi: 10.1007/s00253-018-8939-z
207. Stefan LM, Iosageanu A, Ilie D, Stanciuc A-M, Matei C, Berger D, et al. Extracellular matrix biomimetic polymeric membranes enriched with silver nanoparticles for wound healing. Biomed Mater. (2021) 16(3):035010. doi: 10.1088/1748-605X/abe55d
208. Bundjaja V, Santoso SP, Angkawijaya AE, Yuliana M, Soetaredjo FE, Ismadji S, et al. Fabrication of cellulose carbamate hydrogel-dressing with rarasaponin surfactant for enhancing adsorption of silver nanoparticles and antibacterial activity. Mater Sci Eng C. (2021) 118:111542. doi: 10.1016/j.msec.2020.111542
209. Ribeiro FM, De Oliveira MM, Singh S, Sakthivel TS, Neal CJ, Seal S, et al. Ceria nanoparticles decrease UVA-induced fibroblast death through cell redox regulation leading to cell survival, migration and proliferation. Front Bioeng Biotechnol. (2020) 8:577557. doi: 10.3389/fbioe.2020.577557
210. Sadidi H, Hooshmand S, Ahmadabadi A, Javad Hoseini S, Baino F, Vatanpour M, et al. Cerium oxide nanoparticles (nanoceria): hopes in soft tissue engineering. Molecules. (2020) 25(19):4559. doi: 10.3390/molecules25194559
211. Alizadeh S, Seyedalipour B, Shafieyan S, Kheime A, Mohammadi P, Aghdami N. Copper nanoparticles promote rapid wound healing in acute full thickness defect via acceleration of skin cell migration, proliferation, and neovascularization. Biochem Biophys Res Commun. (2019) 517(4):684–90. doi: 10.1016/j.bbrc.2019.07.110
212. Ahmed KBA, Anbazhagan V. Synthesis of copper sulfide nanoparticles and evaluation of in vitro antibacterial activity and in vivo therapeutic effect in bacteria-infected zebrafish. RSC Adv. (2017) 7(58):36644–52. doi: 10.1039/C7RA05636B
213. Kong Y, Hou Z, Zhou L, Zhang P, Ouyang Y, Wang P, et al. Injectable self-healing hydrogels containing CuS nanoparticles with abilities of hemostasis, antibacterial activity, and promoting wound healing. ACS Biomater Sci Eng. (2020) 7(1):335–49. doi: 10.1021/acsbiomaterials.0c01473
214. Haghniaz R, Rabbani A, Vajhadin F, Khan T, Kousar R, Khan AR, et al. Anti-bacterial and wound healing-promoting effects of zinc ferrite nanoparticles. J Nanobiotechnol. (2021) 19:1–15. doi: 10.1186/s12951-021-00776-w
215. Kaur G, Narayanan G, Garg D, Sachdev A, Matai I. Biomaterials-based regenerative strategies for skin tissue wound healing. ACS Appl Bio Mater. (2022) 5(5):2069–106. doi: 10.1021/acsabm.2c00035
216. Patel KK, Surekha DB, Tripathi M, Anjum MM, Muthu M, Tilak R, et al. Antibiofilm potential of silver sulfadiazine-loaded nanoparticle formulations: a study on the effect of DNase-I on microbial biofilm and wound healing activity. Mol Pharm. (2019) 16(9):3916–25. doi: 10.1021/acs.molpharmaceut.9b00527
217. Banerjee J, Seetharaman S, Wrice NL, Christy RJ, Natesan S. Delivery of silver sulfadiazine and adipose derived stem cells using fibrin hydrogel improves infected burn wound regeneration. PLoS One. (2019) 14(6):e0217965. doi: 10.1371/journal.pone.0217965
218. Yang W, Wang L, Mettenbrink EM, DeAngelis PL, Wilhelm S. Nanoparticle toxicology. Annu Rev Pharmacol Toxicol. (2021) 61:269–89. doi: 10.1146/annurev-pharmtox-032320-110338
219. Hu W, Wang C, Gao D, Liang Q. Toxicity of transition metal nanoparticles: a review of different experimental models in the gastrointestinal tract. J Appl Toxicol. (2023) 43(1):32–46. doi: 10.1002/jat.4320
220. Cornu R, Béduneau A, Martin H. Influence of nanoparticles on liver tissue and hepatic functions: a review. Toxicology. (2020) 430:152344. doi: 10.1016/j.tox.2019.152344
221. Shi D, Mi G, Webster T. The synthesis, application, and related neurotoxicity of carbon nanotubes. Neurotoxicity of nanomaterials and nanomedicine. Amsterdam: Elsevier. (2017). p. 259–84. doi: 10.1016/B978-0-12-804598-5.00011-8
222. Gholamine B, Karimi I, Salimi A, Mazdarani P, Becker LA. Neurobehavioral toxicity of carbon nanotubes in mice: focus on brain-derived neurotrophic factor messenger RNA and protein. Toxicol Ind Health. (2017) 33(4):340–50. doi: 10.1177/0748233716644381
223. Kisin ER, Murray AR, Keane MJ, Shi X-C, Schwegler-Berry D, Gorelik O, et al. Single-walled carbon nanotubes: geno-and cytotoxic effects in lung fibroblast V79 cells. J Toxicol Environ Health, Part A. (2007) 70(24):2071–9. doi: 10.1080/15287390701601251
224. Shvedova AA, Kisin E, Murray AR, Johnson VJ, Gorelik O, Arepalli S, et al. Inhalation vs. Aspiration of single-walled carbon nanotubes in C57BL/6 mice: inflammation, fibrosis, oxidative stress, and mutagenesis. Am J Physiol Lung Cell Mol Physiol. (2008) 295(4):L552–L65. doi: 10.1152/ajplung.90287.2008
225. Zhu L, Chang DW, Dai L, Hong Y. DNA Damage induced by multiwalled carbon nanotubes in mouse embryonic stem cells. Nano Lett. (2007) 7(12):3592–7. doi: 10.1021/nl071303v
226. Landsiedel R, Kapp MD, Schulz M, Wiench K, Oesch F. Genotoxicity investigations on nanomaterials: methods, preparation and characterization of test material, potential artifacts and limitations—many questions, some answers. Mutat Res Rev Mutat Res. (2009) 681(2–3):241–58. doi: 10.1016/j.mrrev.2008.10.002
227. Muller J, Decordier I, Hoet PH, Lombaert N, Thomassen L, Huaux F, et al. Clastogenic and aneugenic effects of multi-wall carbon nanotubes in epithelial cells. Carcinogenesis. (2008) 29(2):427–33. doi: 10.1093/carcin/bgm243
228. Ema M, Masumori S, Kobayashi N, Naya M, Endoh S, Maru J, et al. In vivo comet assay of multi-walled carbon nanotubes using lung cells of rats intratracheally instilled. J Appl Toxicol. (2013) 33(10):1053–60. doi: 10.1002/jat.2810
229. Pothmann D, Simar S, Schuler D, Dony E, Gaering S, Le Net J-L, et al. Lung inflammation and lack of genotoxicity in the comet and micronucleus assays of industrial multiwalled carbon nanotubes graphistrength© C100 after a 90-day nose-only inhalation exposure of rats. Part Fibre Toxicol. (2015) 12(1):1–28. doi: 10.1186/s12989-015-0096-2
230. Patlolla AK, Hussain SM, Schlager JJ, Patlolla S, Tchounwou PB. Comparative study of the clastogenicity of functionalized and nonfunctionalized multiwalled carbon nanotubes in bone marrow cells of Swiss-Webster mice. Environ Toxicol. (2010) 25(6):608–21. doi: 10.1002/tox.20621
231. Patlolla AK, Patra PK, Flountan M, Tchounwou PB. Cytogenetic evaluation of functionalized single-walled carbon nanotube in mice bone marrow cells. Environ Toxicol. (2016) 31(9):1091–102. doi: 10.1002/tox.22118
232. Rahman M, Wang J, Patterson T, Saini U, Robinson B, Newport G, et al. Expression of genes related to oxidative stress in the mouse brain after exposure to silver-25 nanoparticles. Toxicol Lett. (2009) 187(1):15–21. doi: 10.1016/j.toxlet.2009.01.020
233. Kim S, Ryu DY. Silver nanoparticle-induced oxidative stress, genotoxicity and apoptosis in cultured cells and animal tissues. J Appl Toxicol. (2013) 33(2):78–89. doi: 10.1002/jat.2792
234. Souza TA, Franchi LP, Rosa LR, da Veiga MA, Takahashi CS. Cytotoxicity and genotoxicity of silver nanoparticles of different sizes in CHO-K1 and CHO-XRS5 cell lines. Mutat Res Genet Toxicol Environ Mutagen. (2016) 795:70–83. doi: 10.1016/j.mrgentox.2015.11.002
235. Jiang X, Foldbjerg R, Miclaus T, Wang L, Singh R, Hayashi Y, et al. Multi-platform genotoxicity analysis of silver nanoparticles in the model cell line CHO-K1. Toxicol Lett. (2013) 222(1):55–63. doi: 10.1016/j.toxlet.2013.07.011
236. AshaRani P, Low Kah Mun G, Hande MP, Valiyaveettil S. Cytotoxicity and genotoxicity of silver nanoparticles in human cells. ACS Nano. (2009) 3(2):279–90. doi: 10.1021/nn800596w
237. Joksić G, Stašić J, Filipović J, Šobot AV, Trtica M. Size of silver nanoparticles determines proliferation ability of human circulating lymphocytes in vitro. Toxicol Lett. (2016) 247:29–34. doi: 10.1016/j.toxlet.2016.02.007
238. Huk A, Izak-Nau E, Reidy B, Boyles M, Duschl A, Lynch I, et al. Is the toxic potential of nanosilver dependent on its size? Part Fibre Toxicol. (2014) 11(1):1–16. doi: 10.1186/1743-8977-11-1
239. Di Bucchianico S, Fabbrizi MR, Cirillo S, Uboldi C, Gilliland D, Valsami-Jones E, et al. Aneuploidogenic effects and DNA oxidation induced in vitro by differently sized gold nanoparticles. Int J Nanomed. (2014) 9:2191. doi: 10.2147/IJN.S58397
240. Fraga S, Faria H, Soares ME, Duarte JA, Soares L, Pereira E, et al. Influence of the surface coating on the cytotoxicity, genotoxicity and uptake of gold nanoparticles in human HepG2 cells. J Appl Toxicol. (2013) 33(10):1111–9. doi: 10.1002/jat.2865
241. Li JJ, Lo S-L, Ng C-T, Gurung RL, Hartono D, Hande MP, et al. Genomic instability of gold nanoparticle treated human lung fibroblast cells. Biomaterials. (2011) 32(23):5515–23. doi: 10.1016/j.biomaterials.2011.04.023
242. Di Bucchianico S, Migliore L, Marsili P, Vergari C, Giammanco F, Giorgetti E. Cyto-and genotoxicity assessment of gold nanoparticles obtained by laser ablation in A549 lung adenocarcinoma cells. J Nanopart Res. (2015) 17:1–14. doi: 10.1007/s11051-015-3023-4
243. Cardoso E, Rezin GT, Zanoni ET, de Souza Notoya F, Leffa DD, Damiani AP, et al. Acute and chronic administration of gold nanoparticles cause DNA damage in the cerebral cortex of adult rats. Mutat Res Fundam Mol Mech Mutagen. (2014) 766:25–30. doi: 10.1016/j.mrfmmm.2014.05.009
244. Song B, Zhang Y, Liu J, Feng X, Zhou T, Shao L. Unraveling the neurotoxicity of titanium dioxide nanoparticles: focusing on molecular mechanisms. Beilstein J Nanotechnol. (2016) 7(1):645–54. doi: 10.3762/bjnano.7.57
245. Long TC, Tajuba J, Sama P, Saleh N, Swartz C, Parker J, et al. Nanosize titanium dioxide stimulates reactive oxygen species in brain microglia and damages neurons in vitro. Environ Health Perspect. (2007) 115(11):1631–7. doi: 10.1289/ehp.10216
246. Rahman Q, Lohani M, Dopp E, Pemsel H, Jonas L, Weiss DG, et al. Evidence that ultrafine titanium dioxide induces micronuclei and apoptosis in Syrian hamster embryo fibroblasts. Environ Health Perspect. (2002) 110(8):797–800. doi: 10.1289/ehp.02110797
247. Kang SJ, Kim BM, Lee YJ, Chung HW. Titanium dioxide nanoparticles trigger p53-mediated damage response in peripheral blood lymphocytes. Environ Mol Mutagen. (2008) 49(5):399–405. doi: 10.1002/em.20399
248. Valdiglesias V, Fernández-Bertólez N, Kiliç G, Costa C, Costa S, Fraga S, et al. Are iron oxide nanoparticles safe? Current knowledge and future perspectives. J Trace Elem Med Biol. (2016) 38:53–63. doi: 10.1016/j.jtemb.2016.03.017
249. Yarjanli Z, Ghaedi K, Esmaeili A, Rahgozar S, Zarrabi A. Iron oxide nanoparticles may damage to the neural tissue through iron accumulation, oxidative stress, and protein aggregation. BMC Neurosci. (2017) 18:1–12. doi: 10.1186/s12868-017-0369-9
250. Pisanic TR II, Blackwell JD, Shubayev VI, Fiñones RR, Jin S. Nanotoxicity of iron oxide nanoparticle internalization in growing neurons. Biomaterials. (2007) 28(16):2572–81. doi: 10.1016/j.biomaterials.2007.01.043
251. Grigg J, Tellabati A, Rhead S, Almeida GM, Higgins JA, Bowman KJ, et al. DNA Damage of macrophages at an air-tissue interface induced by metal nanoparticles. Nanotoxicology. (2009) 3(4):348–54. doi: 10.3109/17435390903276917
252. Kückelhaus S, De Cuyper MM, Lacava Z. Magnetoliposome evaluation using cytometry and micronucleus test. Eur Cells Mater. (2002) 3(2):154–5. doi: 10.1016/j.biomaterials.2007.01.043
253. Sadeghiani N, Barbosa L, Silva L, Azevedo R, Morais P, Lacava Z. Genotoxicity and inflammatory investigation in mice treated with magnetite nanoparticles surface coated with polyaspartic acid. J Magn Magn Mater. (2005) 289:466–8. doi: 10.1016/j.jmmm.2004.11.131
254. You R, Ho Y-S, Hung CH-L, Liu Y, Huang C-X, Chan H-N, et al. Silica nanoparticles induce neurodegeneration-like changes in behavior, neuropathology, and affect synapse through MAPK activation. Part Fibre Toxicol. (2018) 15:1–18. doi: 10.1186/s12989-017-0237-x
255. Gerloff K, Albrecht C, Boots AW, Förster I, Schins RP. Cytotoxicity and oxidative DNA damage by nanoparticles in human intestinal caco-2 cells. Nanotoxicology. (2009) 3(4):355–64. doi: 10.3109/17435390903276933
256. Wang JJ, Sanderson BJ, Wang H. Cytotoxicity and genotoxicity of ultrafine crystalline SiO2 particulate in cultured human lymphoblastoid cells. Environ Mol Mutagen. (2007) 48(2):151–7. doi: 10.1002/em.20287
257. Sayes CM, Reed KL, Glover KP, Swain KA, Ostraat ML, Donner EM, et al. Changing the dose metric for inhalation toxicity studies: short-term study in rats with engineered aerosolized amorphous silica nanoparticles. Inhalation Toxicol. (2010) 22(4):348–54. doi: 10.3109/08958370903359992
258. Teleanu DM, Chircov C, Grumezescu AM, Teleanu RI. Neurotoxicity of nanomaterials: an up-to-date overview. Nanomaterials. (2019) 9(1):96. doi: 10.3390/nano9010096
259. Zeng Y, Kurokawa Y, Win-Shwe T-T, Zeng Q, Hirano S, Zhang Z, et al. Effects of PAMAM dendrimers with various surface functional groups and multiple generations on cytotoxicity and neuronal differentiation using human neural progenitor cells. J Toxicol Sci. (2016) 41(3):351–70. doi: 10.2131/jts.41.351
Keywords: nanoparticle, antibacterial, anti-cancer, tissue regeneration, dentistry
Citation: Sobhani-Nasab A, Banafshe HR, Atapour A, Khaksary Mahabady M, Akbari M, Daraei A, Mansoori Y and Moradi Hasan-Abad A (2024) The use of nanoparticles in the treatment of infectious diseases and cancer, dental applications and tissue regeneration: a review. Front. Med. Technol. 5:1330007. doi: 10.3389/fmedt.2023.1330007
Received: 6 November 2023; Accepted: 12 December 2023;
Published: 23 January 2024.
Edited by:
Khashayar Khoshmanesh, RMIT University, AustraliaReviewed by:
Aparna Datta, NSHM Knowledge Campus, India© 2024 Sobhani-Nasab, Banafshe, Atapour, Khaksary Mahabady, Akbari, Daraei, Mansoori and Moradi Hasan-Abad. This is an open-access article distributed under the terms of the Creative Commons Attribution License (CC BY). The use, distribution or reproduction in other forums is permitted, provided the original author(s) and the copyright owner(s) are credited and that the original publication in this journal is cited, in accordance with accepted academic practice. No use, distribution or reproduction is permitted which does not comply with these terms.
*Correspondence: Amin Moradi Hasan-Abad YW1pbi5tb3JhZGkxNDAwQHlhaG9vLmNvbQ==
†ORCID Amin Moradi Hasan-Abad orcid.org/0000-0003-4769-3847
Disclaimer: All claims expressed in this article are solely those of the authors and do not necessarily represent those of their affiliated organizations, or those of the publisher, the editors and the reviewers. Any product that may be evaluated in this article or claim that may be made by its manufacturer is not guaranteed or endorsed by the publisher.
Research integrity at Frontiers
Learn more about the work of our research integrity team to safeguard the quality of each article we publish.