- 1Division of Experimental Oncology, Department of Oncology, Cross Cancer Institute, University of Alberta, Edmonton, AB, Canada
- 2Dipartimento di Ingegneria Meccanica e Aerospaziale, Politecnico di Torino, Turin, Italy
- 3Autem Therapeutics, Hanover, NH, United States
- 4Oncology Department, Hospital Sírio-Libanês, São Paulo, Brazil
Exposure to Low-Energy Amplitude-Modulated Radiofrequency Electromagnetic Fields (LEAMRFEMF) represents a new treatment option for patients with advanced hepatocellular carcinoma (AHCC). We focus on two medical devices that modulate the amplitude of a 27.12 MHz carrier wave to generate envelope waves in the low Hz to kHz range. Each provides systemic exposure to LEAMRFEMF via an intrabuccal antenna. This technology differs from so-called Tumour Treating Fields because it uses different frequency ranges, uses electromagnetic rather than electric fields, and delivers energy systemically rather than locally. The AutemDev also deploys patient-specific frequencies. LEAMRFEMF devices use 100-fold less power than mobile phones and have no thermal effects on tissue. Tumour type-specific or patient-specific treatment frequencies can be derived by measuring haemodynamic changes induced by exposure to LEAMRFEMF. These specific frequencies inhibited growth of human cancer cell lines in vitro and in mouse xenograft models. In uncontrolled prospective clinical trials in patients with AHCC, minorities of patients experienced complete or partial tumour responses. Pooled comparisons showed enhanced overall survival in treated patients compared to historical controls. Mild transient somnolence was the only notable treatment-related adverse event. We hypothesize that intracellular oscillations of charged macromolecules and ion flows couple resonantly with LEAMRFEMF. This resonant coupling appears to disrupt cell division and subcellular trafficking of mitochondria. We provide an estimate of the contribution of the electromagnetic effects to the overall energy balance of an exposed cell by calculating the power delivered to the cell, and the energy dissipated through the cell due to EMF induction of ionic flows along microtubules. We then compare this with total cellular metabolic energy production and conclude that energy delivered by LEAMRFEMF may provide a beneficial shift in cancer cell metabolism away from aberrant glycolysis. Further clinical research may confirm that LEAMRFEMF has therapeutic value in AHCC.
Introduction
Liver cancer remains the fourth most common cause of cancer death and the second most lethal cancer in terms of 5-year survival, despite recent approvals of new systemic therapies (1). Patients with late-stage disease often lack adequate liver function and therefore may not be suitable candidates for systemic therapies. Exposure to low-power electromagnetic fields (EMFs) with specific frequencies is an emerging treatment for patients with advanced hepatocellular carcinoma (HCC) and other cancers (2–4).
In this review, we focus on medical devices that deliver low-power EMFs with a carrier wave frequency of 27.12 MHz, amplitude-modulated at multiple tumour type-specific or personalized envelope wave frequencies in the 10 Hz–150 kHz range (2, 3). The identification of specific amplitude-modulation frequencies is based on the physiological effects of exposure on excitable cells (5). This enables haemodynamic changes to be used as biomarkers of potentially therapeutic frequencies for particular cancers or for individual patients (6–8). We review evidence on the efficacy and safety of amplitude-modulated radiofrequency electromagnetic field (LEAM RF EMF) exposure in patients with HCC using these devices. The power delivered is far too low to cause detectable heating of cells or tissues (9). Rather, the effects of LEAM RF EMF exposure rely on the resonant interactions of electromagnetic waves with subcellular structures of normal cells and cancer cells in the human body (2–4, 10).
Radio waves interact with both moving and stationary electrical charges in cells, including atomic ions and molecular ions. When the frequency of the radio waves matches the timescales characteristic of cellular processes, exposure to the EMF may influence these processes by inducing movements of ions, because of resonant coupling of the oscillations. Cells partition charged molecules across membranes, which produces electrical and chemical potential differences, similar to those in a battery (11). These potential differences provide the ion-motive forces that underlie nearly all physiological processes in cells (12, 13). Cells also contain highly charged macromolecular structures, including microtubules, which are involved in cellular electrical signal propagation in addition to their well-known role in cell division (14, 15). We discuss potential mechanisms of action of LEAM RF EMF exposure in patients with cancer, at cellular and subcellular levels. These include potential overlapping effects of both the carrier wave and the envelope wave on ion flows, microtubules, mitochondrial localization and function, and cancer cell metabolism.
Medical devices
Two existing medical devices provide systemic exposure to low-power LEAM RF EMFs with a carrier wave frequency of 27.12 MHz: the P1 (TheraBionic GmbH, Ettlingen, Germany) and the AutEMdev, an investigational device in development (Autem Therapeutics, Hanover, NH, USA). These small battery-operated devices emit extremely low-power EMFs, each delivering less than 100 mW into a spoon-shaped stainless-steel antenna that is placed into the patient's mouth (8, 9, 16). The resulting whole body-specific absorption rate of 1.77 mW/kg lies far below international safety limits and is too low to cause detectable heating (9). The device power is about 1,000 times lower than that of a mobile phone and 100,000 times lower than that of thermal tumour ablation devices (16). Furthermore, the frequency ranges differ from those used in telecommunications, where exposure to EMFs has controversial and inconsistent potential effects on health (reportedly both positive and negative) (17–20).
The carrier wave frequency of 27.12 MHz is one of the frequency bands reserved internationally for medical devices. Both devices use sinusoidal amplitude modulation of the carrier wave to generate envelope waves, with frequencies ranging from 10 Hz to 20 kHz for the AutEMdev and 0.1 Hz to 150 kHz for the P1 (8, 9). These envelope waves are generated individually in sequence at very precise frequencies, specified to three decimal places (21). For the P1 device, the envelope wave frequencies were selected as tumour-specific frequencies based on biofeedback responses defined by the magnitude of increased amplitude and/or the number of beats with increased amplitude of the radial pulse (5, 16). For the AutEMdev, personalized frequencies are selected in real-time by simultaneous monitoring of multiple haemodynamic parameters that may indicate potentially clinically beneficial effects in each individual (8).
Both the fixed 27.12 MHz carrier wave and the variable tumour-specific and/or patient-specific amplitude-modulated envelope waves may be involved in the physiological and therapeutic effects of EMF exposure. We hypothesize that electromagnetic waves at these frequencies couple and resonate with oscillations of charged macromolecular structures and ion flows within cells, similar to the way that a bell rings in sympathy when exposed to sound waves of the right frequency. This means that, although the device antenna is placed in the patient's mouth, the entire human body also becomes an antenna. With the exception of the bones, the effects of exposure to the carrier wave and the envelope waves are therefore distributed throughout the entire body.
In addition to the two above-mentioned devices, a third company (Novocure, Saint Helier, Jersey) has developed a treatment which exposes patients to so-called Tumor Treating Fields (TTFields) using the “Optune” device which is placed in the tumour region via an arrangement of two orthogonal sets of transducer arrays (22–24). These transducer arrays generate electric fields directed at the tumour site/cells and are activated sequentially on a second timescale. This enables a directional change of the incident electric field.
The intensity of TTFields is on the order of 1 V/cm and its optimal oscillation frequency depends on the tumour type. For example, glioblastoma multiforme patients are typically exposed to a frequency of 200 kHz. Therefore, TTFields involve frequencies which are two orders of magnitude lower than the carrier wave, and between two and five orders of magnitude higher than envelope frequencies used by LEAM RF EMFs. Also of note is that TTFields are entirely electric fields while LEAM RF EMFs are electromagnetic, i.e., they have both an electric and a magnetic component. Moreover, LEAM RF EMFs are applied systemically while TTFields are locally directed at the tumour site. Finally, LEAM RF EMF technology allows for a patient-specific frequency selection for the envelope wave while TTFields are tumour type-specific only.
The carrier wave is a high-frequency oscillation with amplitude modulated by a slow-frequency variation (Figure 1). This is a different mode of electromagnetic energy-based cancer therapy than TTFields. It also differs from therapies based on static and low-frequency magnetic fields and magnetic nanoparticles (25, 26). In Table 1 we have compared and contrasted the various wave-based (electromagnetic and ultrasound) technologies used in cancer therapy.
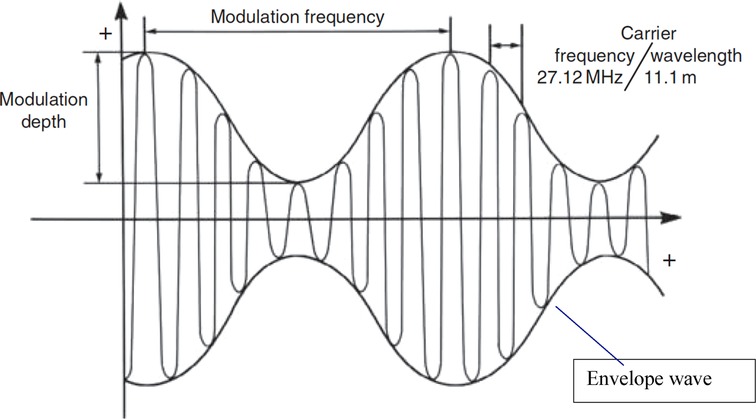
Figure 1. Schematic description of an amplitude-modulated radiofrequency electromagnetic wave. The carrier frequency (27.12 MHz) is sinusoidally modulated at specific frequencies (16).
Physiological effects
A sleep-restoring effect in patients with insomnia was the first reported potential clinical benefit of LEAM RF EMF exposure, at specific effective envelope wave frequencies (27–29). A similar phenomenon was observed as mild, transient somnolence in patients with cancer undergoing LEAM RF EMF exposure, as described below. An effect of radiofrequency EMFs on the α waves of the human brain, which have a frequency of 8–13 Hz, may underlie these observations, and this may ultimately derive from modification of the behaviour of central nervous system neurons (30).
Alterations in haemodynamic regulation are also observed in patients exposed to LEAM RF EMFs. In studies using the P1 device, haemodynamic changes were detected as variations in skin electrical resistance, pulse amplitude and blood pressure. Frequencies eliciting the best biofeedback responses, defined by the magnitude of increased amplitude and/or the number of beats with increased amplitude of the radial pulse, were selected as tumour-specific (5). A study using the AutEMdev involved real-time automated monitoring of multiple haemodynamic parameters employing the AutEMdev and a separate monitoring device (6–8). Specific envelope wave frequencies that induced subtle but reproducible alterations in haemodynamic regulation were then identified in each individual. Similar effects on haemodynamic regulation have been reported for other EMF exposure modalities (31–33). These effects presumably result from modification of the behaviour of excitable cells in the heart and central/peripheral nervous system.
Treatment of HCC
In vitro and animal studies
A transverse electromagnetic cell culture system was used to mimic the effects of LEAM RF EMF exposure with devices using a 27.12 MHz carrier wave on cancer cell lines in two in vitro studies (9, 21). Growth inhibition of the Huh-7 and HepG2 liver cancer cell lines was greater with previously identified liver cancer-specific envelope wave frequencies than with breast cancer-specific frequencies or randomly selected frequencies (21). Conversely, growth inhibition of the MCF-7 breast cancer cell line was greater with breast cancer-specific frequencies than with liver cancer-specific frequencies (although this was not the case for the MCF-10A epithelial breast cell line) (21). Evidence for involvement of Ca2+ influx through the CACNA1H voltage-gated calcium channel in the anti-proliferative effects was provided by experiments in Huh7 and Hep3B cells (9). Ca2+ influx through this channel was only observed with liver cancer-specific frequencies, not with breast cancer-specific frequencies or randomly selected frequencies (9).
In mouse xenograft models of HCC, exposure to LEAM RF EMFs led to tumour shrinkage or reduced tumour growth, depending on the human tumour cell type injected (9). These effects were observed with liver cancer-specific envelope wave frequencies in the range of 100 Hz to 21 kHz, but not with randomly selected frequencies or sham exposure. Histological analysis indicated that tumour shrinkage was associated with differentiation of the injected cancer cells into quiescent cells with a fibroblast-like morphology (9). Similar findings have also been reported in xenograft models of metastatic breast cancer (34).
Clinical studies
Five clinical study reports using the AutEMdev or P1 devices in patients with HCC have been published, to our knowledge. There is one study currently recruiting patients (Table 2). Barbault et al. described the selection of tumour type-specific envelope wave frequencies using the P1 device in patients with cancer, by monitoring variations in amplitude of the radial pulse (5). The range of these frequencies was higher than the frequencies previously identified in patients with insomnia (27–29), and also differed among patients with different cancers. Of the 1,524 frequencies identified across four cancer types, 170 were active in patients with HCC and 144 of these were specific to HCC. Anecdotal evidence of disease stabilization was provided in a small group of patients with cancer, of whom only one had HCC (5).
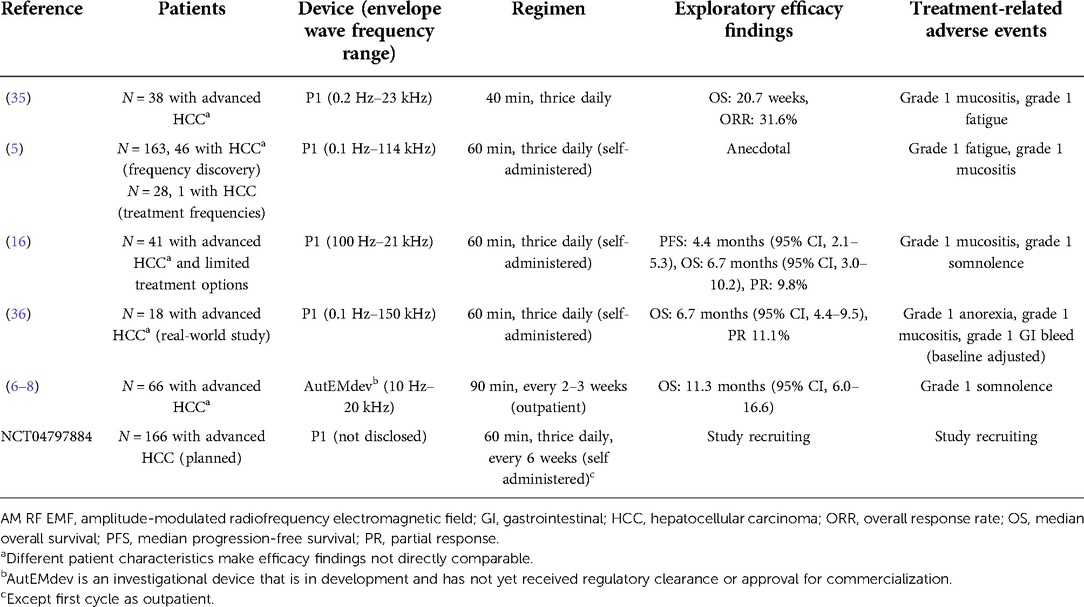
Table 2. Clinical studies of low-power AM RF EMF devices using a 27.12 MHz carrier wave in patients with HCC.
The HCC-specific frequencies identified by Barbault et al. were subsequently deployed in prospective phase 2 studies of the P1 device in patients with advanced HCC (Table 2) (5, 16, 35, 36). Although these studies were open-label and uncontrolled, evidence of antitumour effects was reported, with partial and complete radiological responses in small numbers of patients. In a pooled comparison, median overall survival for Child-Pugh B patients receiving TheraBionic P1 device as first line therapy was slightly higher than the 4.6 months median OS of historical controls receiving Sorafenib as first line therapy (36). These findings led to initiation of a multicentre, double-blind, randomized study comparing the P1 device with a placebo device in patients with advanced HCC following unsuccessful treatment with at least two lines of conventional systemic therapy (Table 2) (5, 16).
The AutEMdev deploys personalized frequencies in each patient (6–8). In an open-label clinical feasibility study in patients with advanced HCC (Table 2), personalized frequencies were identified in a 30-minute discovery phase using real-time multi-parameter haemodynamic monitoring. The selected frequencies were then applied in a subsequent 30-minute treatment phase. The subtle but reproductible haemodynamic alterations at personalized frequencies are hypothesized to represent a biological surrogate of antitumour activity (6–8). The studies using AutEMdev and P1 devices provided evidence for anti-tumour efficacy and/or maintained or improved health-related quality of life (HRQoL) in patients remaining on treatment (Table 2), and overall survival in the prospective study cohort was longer compared with a retrospective control cohort (5–8, 16).
After hundreds of weekly procedures over periods of several years, mild, self-limiting somnolence or fatigue was the only frequently reported adverse event related to LEAM RF EMF exposure among patients receiving treatment with this modality alone or in combination with conventional anticancer treatment (Table 2). This side effect profile is consistent with the sleep-promoting effect of LEAM RF EMF exposure in patients with insomnia (27–29). The subtle effects of exposure on haemodynamic regulation do not appear to be associated with adverse events. Some patients receiving LEAM RF EMF exposure in combination with chemotherapy also experienced mild mucositis during exposure (5).
Mechanisms of action
Carcinogenesis can be viewed as a phase transition in which normally synchronized, homogeneous, and differentiated cells in ordered tissues become asynchronized, heterogeneous, dedifferentiated, and proliferative cancer cells in disordered tumours (37). Resting membrane potentials are also altered (significantly lowered) and metabolism shifts from oxidative phosphorylation to glycolysis (11, 38–40). Because all subcellular processes occur at characteristic frequencies, these frequencies may also alter during carcinogenesis. This underlies the concept of favourable and unfavourable coherent frequency patterns in cancer, which may be manipulable using systemic LEAM RF EMFs at specific frequencies (4, 41). A similar phenomenon may also underlie the potential application of extremely low frequency EMFs to wound healing (42).
LEAM RF EMF exposure causes non-thermal effects in tissues (<1°C for nominal perfusion values). Experience with modelling of hyperthermal tumour damage indicates partial self-regeneration of normal tissue at tumour boundaries associated with perfusion by arterial blood should not be ignored (43, 44). Thermal damage can also change the interstitial structure and porosity of the tumour, according to recent mathematical models (45).
Although muscle cells and neurons were the first identified excitable cells (46, 47), nearly all cells have a resting membrane potential, and voltage-activated ion channels are expressed in non-excitable cells as well (48). One of these is the voltage-gated Ca2+ channel implicated in the anti-proliferative effects of LEAM RF EMF exposure in cancer cell lines in vitro (9, 21). Ion flows resulting from the motive forces provided by membrane potentials govern not only intracellular signalling and metabolism but also cell-to-cell communication (11).
Actin filaments, collagen, DNA, and microtubules are all highly charged linear macromolecular polymeric structures that can conduct electrical currents via the counterions that surround them (14, 49). This makes them potential candidates for resonant interactions with LEAM RF EMFs. Induction of DNA breaks has been proposed as a mechanism of action for potential anticancer effects of EMF exposure (42, 50–54). A direct effect of EMFs on mitochondrial electrochemistry has also been proposed (41). We consider, however, that microtubules are most likely the principal cellular bio-antenna for therapeutic LEAM RF EMFs due to their exceptionally high electric charge and dipole moment values directly coupling with EMFs. Below, we argue that the effect of exposure on microtubule ion flows may impair cell division and disrupt subcellular trafficking of mitochondria. We also hypothesize that LEAM RF EMF exposure alters cancer cell metabolism away from aberrant glycolysis, potentially inhibiting tumour growth.
Microtubules
Microtubules are dynamic αβ-tubulin polymers that form part of the cytoskeleton and are involved in intracellular organization, organelle trafficking, and chromosome segregation. The cylindrical structure of microtubules is formed of 13 protofilaments of stacked tubulin dimers surrounding a 15 nm lumen, with a 25 nm outer diameter. Guanosine triphosphate-driven assembly and disassembly of tubulin dimers enables microtubules to exert push–pull forces, for example during cell division, which requires substantial force generation to segregate chromosomes. The adenosine triphosphate (ATP)-driven motor protein kinesin-1 tracks along microtubules, driving intracellular transport of mitochondria and other organelles.
Microtubules also support three established modes of ionic wave propagation: longitudinally on the surface, longitudinally within the lumen, and axially in and out of the nanopores between tubulin monomers (14). These ionic waves are formed by oscillation of counterions that are attracted to the charged inner and outer surfaces of the microtubule, and can be induced or modified by EMF exposure at the right frequency (49). Because microtubules are more conductive under specific ionic conditions than the cytoplasm, they act as the principal conduits for propagation of cellular ionic waves. Resonant coupling of EMFs with microtubules may therefore form the principal basis of the medical applications of EMFs (15). The specific frequency of oscillations depends on the length of the microtubule and the ionic strength and pH of the surrounding solution, and may also be influenced by cellular architecture (55, 56).
Carrier wave effects on microtubules
Microtubules are extremely longitudinally conductive to alternating currents at frequencies close to the 27.12 MHz carrier wave of the LEAM RF EMF medical devices, in the range of approximately 12–50 MHz (57–61). Furthermore, the highly charged C-terminal tail domains of tubulin protrude from the microtubule surface and also oscillate at frequencies close to that of the carrier wave (14). Although induced oscillations at these frequencies may disrupt microtubule function and motor protein traffic, the carrier wave frequency is constant and cannot therefore be responsible for the personalized or tumour-specific effects of specific envelope wave frequencies. Furthermore, an entire microtubule with a length of only 20 nm would resonate at 27 MHz, but most microtubules are much longer, spanning the length of the cell and achieving lengths up to 50 µm (or even higher under laboratory conditions). We therefore speculate that the carrier wave has a potentiating effect on ion flows along microtubules that renders cells susceptible to the effects of the lower-frequency envelope wave.
Envelope wave effects on microtubules
Spontaneous electrical oscillation of microtubules at fundamental resonant frequencies of 29 and 39 Hz has been reported in patch-clamp electrophysiological experiments using purified microtubule sheets and bundles, and permeabilized neurites in vitro (62, 63). Under constant holding potentials of 1 mV, microtubule-associated charges spontaneously oscillated at these frequencies, with a more than sixfold concomitant increase in conductivity (62, 63). These observations are consistent with axial ion flows in and out of the nanopores between tubulin monomers in a microtubule, and the frequencies fall within the range of envelope wave frequencies produced by LEAM RF EMF devices. Resonant frequencies of microtubules in vivo may differ from those observed in the in vitro electrophysiological studies because of differences in factors such as ionic strength, pH, protein-protein interactions, and cellular architecture. We therefore speculate that the combination of carrier wave and envelope wave interactions with different microtubule ion flows may underlie the physiological and potential clinical effects of LEAM RF EMF exposure.
Mitochondria
The subcellular positioning of mitochondria is tightly associated with their bioenergetic and cell-signalling functions. Mitochondria dynamically alter their subcellular localization by trafficking along microtubules using kinesin-1, and may also form a network enabling exchange of energy and signals between cells via membrane nanotubes (64–66). Perturbation of microtubules by EMF and resulting disruption of mitochondrial trafficking could therefore interfere with multiple mitochondrial functions. Consistent with this possibility, blocking mitochondrial anterograde trafficking by inhibiting integrin recycling led to detrimental amplification of reactive oxygen species in an in vitro study in breast cancer cell lines (67).
In a hypothesis-generating and exploratory experiment, we used transmission electron microscopy to evaluate mitochondrial ultrastructure in liver tumour biopsies taken from patients with HCC who had undergone LEAM RF EMF exposure (Figures 2, 3). Mitochondria appeared to congregate in cellular locations distant from microtubules, and to show morphological changes characterized by disorganized crista and swelling of the matrix. To our knowledge, these abnormalities have not been previously reported in the context of HCC. This finding needs replication in future studies, but is consistent with effects of LEAM RF EMF exposure on mitochondria.
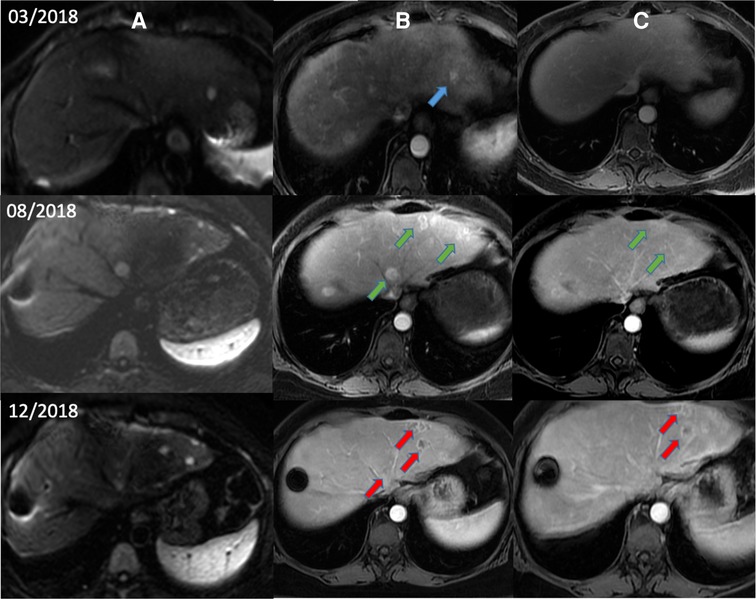
Figure 2. Magnetic resonance images from a patient with HCC after low-power amplitude-modulated radiofrequency electromagnetic field exposure. Diffusion-weighted imaging (A), arterial phase (B), and delayed phase (C) are shown. Patient with advanced HCC began treatment with sorafenib in March 2018 after diagnosis of recurrent HCC by diffusion and arterial phases (blue arrows). After documented radiological progression in August 2018 (green arrows), sorafenib was suspended and AutEMdev was started in 2-week intervals. In December 2018, lytic changes in the tumour lesions were observed by the arterial and delayed phases (red arrows). The patient was submitted to a surgical procedure to remove those lesions. Electronic micrographs of this patient are shown in Figure 3. HCC, hepatocellular carcinoma.
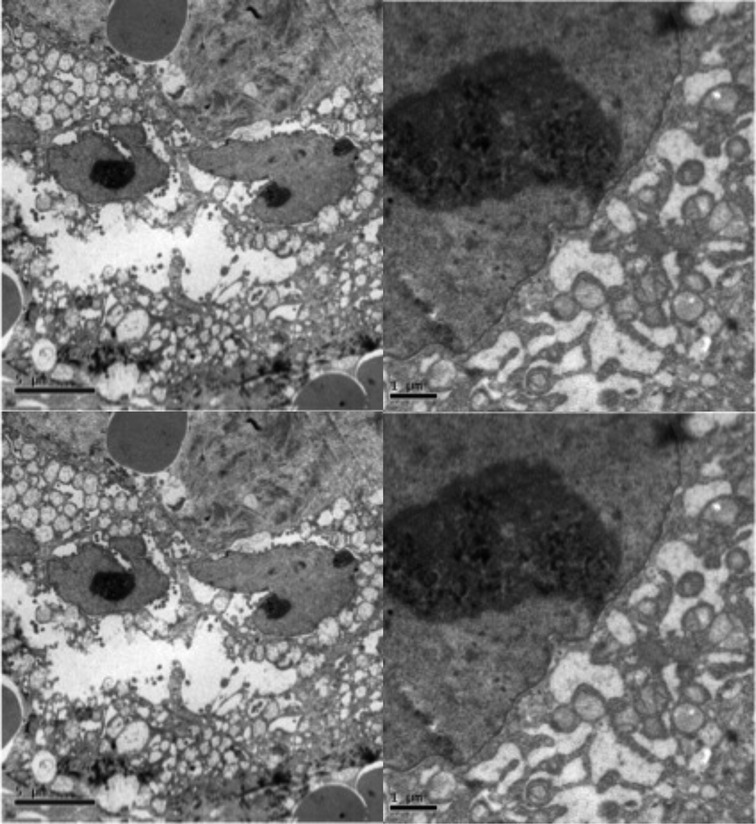
Figure 3. Abnormal mitochondrial morphology in a patient with HCC after low-power amplitude-modulated radiofrequency electromagnetic field exposure. Tissue sections at ultrastructural level of the patient given in Figure 2 are shown. Left: HCC cell with disorganized cytoplasmic organelles and diffuse electron-lucent dilation of reticulum, probably reflecting water accumulation, and mitochondria present in dilated regions. Right: HCC cell with hydropic dilated reticulum around isolated and atypical mitochondria showing morphological changes characterized by disorganized crista and swelling of the matrix. HCC, hepatocellular carcinoma.
Metabolism
Malignant cancer cells produce lactate by fermentation of glucose even in non-hypoxic conditions, in a phenomenon known as the Warburg effect (38, 39). This is far less energy-efficient than oxidative phosphorylation for ATP production, but provides the cell with glycolytic intermediates to support biosynthesis of nucleotides and amino acids for cell growth (11). Because the Warburg effect is a defining feature of malignancy, reversing it has been proposed as a route to new cancer therapies, for example using microRNAs (40, 68, 69).
We hypothesize that LEAM RF EMF exposure may shift cellular metabolism away from glycolysis in cancer cells and hence lead to the restoration of the normal phenotype.(70) Although the effects of low-power AM RF EMF exposure are non-thermal, the energy delivered is ultimately dissipated as molecular motion. In the following sections, we calculate the power delivered to the cell and the energy dissipation due to EMF induction of ionic flows in microtubules, and compare this with total cellular metabolic energy production. We conclude that this energy delivery may contribute significantly towards shifting the cellular energy balance.
Estimation of power delivered to liver cells by EMF device
The starting point is the outcome of calculations is in vitro experiments with modulated radiofrequency using a previously published exposure system designed to replicate in vivo (human) conditions (16, 21). The sXcTEM027 exposure system apparatus (IT'IS Foundation, Zürich Switzerland) operating in the 27 MHz carrier wave region was used for in vitro experiments. The dosimetric assessments of 35 mm Petri dishes were performed for 2D culture cells in monolayer and suspension. The numerical dosimetry was verified by dosimetric temperature measurements. The non-uniformity of the specific absorption rate (SAR) distribution in the Petri dish filled with a small volume of cell culture media was determined and adjusted to represent SAR values determined in humans, as previously published (71). The heterogeneity of SAR with LEAM RF EMFs has not been evaluated in realistic tumour anatomies, to our knowledge, as has been performed for magnetic nanoparticle hyperthermia using micro computed tomography scans (72). Nevertheless, published dosimetry models for LEAM RF EMF provide an adequate starting point for estimating the power delivered to individual liver cells during exposure, as follows (16, 21, 71).
Dosimetric experiments with an RF EMF generator device were performed to estimate the specific absorption rate and distribution inside the human body. Numerical analysis based on the standardized anatomically based model of the human male (“Duke”) from the “Virtual Population” was performed and the simulation results were verified using SAR measurements in a simple rectangular phantom (73). Dosimetric experiments with radiofrequency in the MHz range exposure system for use during live cell imaging provided excellent exposure control and homogeneity.
Published dosimetry values for the P1 device calculate the average power delivered to a single human liver cell during exposure (9). Specific absorption rates were based on the “Duke” adult male model (weight 73 kg), one of ten models in the “Virtual Family” of whole-body anatomical models (73). The average power delivered by the EMF exposure device was 8.4 mW/kg for the liver (9). The average human liver weighs 1.3 kg and comprises 2.4 × 1011 cells (74), so we can readily calculate that the average power delivered per cell is approximately 50 fW. Thermal modelling was performed with a 2 mm voxel size in tissues. The thermal results reached a steady state after approximately 20–25 min with a maximum increase of 0.24°C for nominal perfusion values (75).
Estimation of EMF power dissipated by microtubules
The electrical current flowing through a single microtubule nanopore during oscillation at 29 Hz was estimated at 0.02–0.14 fA under an applied potential difference of 5 mV, in the electrophysiological study described above (62). From this, we can calculate the resistance of a single nanopore as 62.5 TΩ using Ohm's law (R = V/I). Ions flow in from one side of the microtubule wall and out from the other, so each pair of nanopores is a series resistor with a total resistance twice that of a single nanopore, equalling 125 TΩ. If a typical microtubule is 10 µm long and has 13 protofilaments each comprising 1,250 tubulin dimers, it contains 16,250 nanopores. Viewing this as a parallel arrangement of 8,125 nanopore pairs each with a resistance of 125 TΩ, the overall resistance of the microtubule can be estimated to be approximately 15 GΩ.
In a mitotic liver cancer cell, a microtubule plus-end is attached to a kinetochore and a minus-end interacts with a centrosome. If the potential difference across the microtubule in this setting is on the order of 1 mV, then we can calculate the current flowing as 0.07 pA from its resistance of 15 GΩ, again using Ohm's law. (47, 54–55) This translates into an ionic flow rate of 6 × 105 unit charges per second per microtubule, which equates to 30 unit charges per second per nanopore for our typical microtubule with 16,250 nanopores. The frequency of charge transfer is therefore approximately 30 Hz. This is close to both the fundamental oscillation frequency in the electrophysiological study (62) and within the envelope wave frequency of the LEAM RF EMF devices. In this setting, one charge passes through each microtubule nanopore per cycle of the envelope wave, although the exact frequency would depend on cellular microtubule geometry and other factors. Nevertheless, the similarity in frequency between microtubule ion flows and the envelope wave provides a potential mechanism for transfer of electromagnetic energy into cancer cells.
We can also calculate the power dissipated by a single typical microtubule as 0.03 fW (using P = ½ V2/R since this represents the root mean square value of the power in an oscillating current situation described here, with the above values of 1 mV and 15 GΩ). Each kinetochore is attached to 30 microtubules and there are two copies of each of the 23 chromosomes, so we can estimate that there are 1,380 microtubules (excluding astral and polar microtubules) in a mitotic cell. The total power dissipated by microtubule ion flows in a single mitotic cell during oscillation at 29 Hz is therefore 46 fW, under the above assumptions. This value is very similar to the estimated power delivery of 50 fW per liver cell by the clinical EMF exposure device (see above).
Delivered EMF power relative to cellular metabolic power
We next consider what proportion of the normal cellular energy budget corresponds to the approximately 50 fW delivered by LEAM RF EMFs and dissipated by microtubule ion flows. We start by estimating the metabolic power of normal cells as 3 pW per cell using two separate methods. First, the human body uses about 100 W of metabolic power at rest and comprises 3.7 × 1013 cells on average, meaning that each cell uses 3 pW (76). Secondly, each cell contains about 2,000 mitochondria, which each produce about 30,000 ATP molecules per second by oxidative phosphorylation (74). ATP releases 30 kJ/mol of free energy upon hydrolysis or 5 × 10–20 J per molecule (76). Multiplying these values together also gives a metabolic power of 3 pW per cell. The estimates of approximately 50 fW for EMF power delivered and dissipated per cell therefore correspond to approximately 1.5% of metabolic power in normal cells.
In liver cancer cells, mitochondrial oxidative phosphorylation can be reduced by as much as 50%, depending on the disease stage, as cancer cells undergo the Warburg effect and shift to rely increasingly on glycolytic ATP production (77, 78). The human liver normally produces 30 mmol of ATP per minute by oxidative phosphorylation, or 3 × 1020 molecules of ATP per second, each releasing 5 × 10–20 J upon hydrolysis (35), which corresponds to a metabolic power of 15 W (or 15% of whole-body metabolic power).
Glycolysis is nine times less efficient than oxidative phosphorylation, so to maintain the same energy output, cells need to increase glucose consumption by an equivalent factor (77, 78). Consequently, 50 fW per cell may correspond to as much as 13.5% of glucose consumption in cells using glycolysis, compared with 1.5% in those using oxidative phosphorylation. Two-thirds of resting metabolic rate is devoted to heat production (76), so the energy delivered by EMFs may contribute meaningfully to the cellular energy budget. This may hence represent a significant shift in energy production away from glycolysis, which could counteract the Warburg effect and deprive cells of energy-rich metabolites produced from glycolysis (70) with a potential reduction in tumour growth and an overall decrease of the energetic burden the tumour imposes on the rest of the patient's body, consistently with the reported quality of life amelioration of the treated patients.
Summary of proposed metabolic hypothesis
We have shown that the frequency of charge transfer across isolated microtubules in vitro (approximately 30 Hz) falls within the envelope wave frequency range of therapeutic LEAM RF EMF devices. This frequency is likely to vary depending on cell morphology, physiology, and other factors. It may therefore fall within the same range as haemodynamically active and potentially therapeutic envelope wave frequencies (approximately 10–1,000 Hz). This provides a potential mechanism for transfer of EMF energy into cells, and dissipation of power via the ionic flows across microtubules. The power level is far too low to cause detectable heating of tissues, at about 8 mW for the whole liver and 50 fW per liver cell, but may nevertheless result in a significant shift of up to 13.5% in metabolic energy balance away from glycolysis. This could lead to suppression of the Warburg effect and promotion of a normal cellular metabolic phenotype. We hypothesize that this may be one of the mechanisms underlying the long-term disease control observed in patients with HCC in clinical trials. Direct effects of LEAM RF EMFs most likely involve subcellular electrically charged structures such as microtubules and possibly ionic flows around them, with predominant influence on mitosis. Hence, given a typically low mitotic fraction of cells within a tumour relative to the overall cell population and the much higher proportion of time cells reside within other phases of the cell cycle, a more complete explanation of long-lasting effects of EMFs on the tumour most likely requires metabolic effects which can endure long enough to affect cell fate/survival including a change of the phenotype mentioned above.
The structure of the cytoskeleton may underlie the potentially beneficial clinical effects of exposure to LEAM RM EMF using devices producing a 27.12 MHz carrier wave and envelope waves at specific frequencies in the range of approximately 10 Hz to 20 kHz. In particular, oscillating ionic flows may form in the condensed ions surrounding and within microtubules by resonant coupling with envelope waves of specific frequencies. These ionic flows may then lead to interference with ion channels, cell division, motor proteins, mitochondrial trafficking and morphology, and cellular energy balance. These putative mechanisms of action are potentially overlapping and not mutually exclusive, and all require further investigation by specifically designed assays. The efficacy of LEAM RF EMF in patients with HCC will be tested in randomized controlled studies. Nevertheless, we conclude that systemic exposure to LEAM RF EMFs of specific frequencies is a potential route to modifying the behaviour of cancer cells in patients with advanced disease.
Contribution to the field statement
We provide an overview of the existing and developing cancer therapies based on the interactions of electromagnetic fields with cancer cells. In addition to comparing and contrasting the various modalities, we propose a mechanism of action at a cellular and molecular level, which involves microtubules, ionic flows and changes in cellular metabolism. It is of utmost importance to understand how these fields interact with biological matter in order to maximize their efficacy and minimize any potential side effects.
Author contributions
JAT and FC contributed equally to writing the manuscript. All authors contributed to the article and approved the submitted version.
Funding
Hospital Sírio-Libanês sponsored the investigator-initiated trial that resulted in some data shown in this paper. Under the direction of the authors, medical writing support was provided by Oxford PharmaGenesis, Oxford, UK with funding from Autem Therapeutics. The funders were not involved in the study design, collection, analysis, interpretation of data, the writing of this article or the decision to submit it for publication.
Acknowledgments
We thank Luis F. L. Reis and Hospital Sírio-Libanês for their support and Bertram Wiedenmann from Autem Therapeutics for reviewing the manuscript for scientific accuracy.
Conflict of interest
JAT and FC were employed by Autem Therapeutics. JAT and FC have stock and honoraria from Autem Medical and patents relating to the AutEMdev device.
Publisher's note
All claims expressed in this article are solely those of the authors and do not necessarily represent those of their affiliated organizations, or those of the publisher, the editors and the reviewers. Any product that may be evaluated in this article, or claim that may be made by its manufacturer, is not guaranteed or endorsed by the publisher.
References
1. Villanueva A. Hepatocellular carcinoma. N Engl J Med. (2019) 380(15):1450–62. doi: 10.1056/NEJMra1713263
2. Jimenez H, Blackman C, Lesser G, Debinski W, Chan M, Sharma S, et al. Use of non-ionizing electromagnetic fields for the treatment of cancer. Front Biosci (Landmark Ed). (2018) 23(2):284–97. doi: 10.2741/4591
3. Zimmerman JW, Jimenez H, Pennison MJ, Brezovich I, Morgan D, Mudry A, et al. Targeted treatment of cancer with radiofrequency electromagnetic fields amplitude-modulated at tumor-specific frequencies. Chin J Cancer. (2013) 32(11):573–81. doi: 10.5732/cjc.013.10177
4. Meijer DKF, Geesink HJH. Favourable and unfavourable EMF frequency patterns in cancer: perspectives for improved therapy and prevention. J Cancer Ther. (2018) 09(03):188–230. doi: 10.4236/jct.2018.93019
5. Barbault A, Costa FP, Bottger B, Munden RF, Bomholt F, Kuster N, et al. Amplitude-modulated electromagnetic fields for the treatment of cancer: discovery of tumor-specific frequencies and assessment of a novel therapeutic approach. J Exp Clin Cancer Res. (2009) 28:51. doi: 10.1186/1756-9966-28-51
6. Sousa MC, Costa FP, Lima PD, Setogute Y, Gumz BP, Azevedo F, et al. Low energy amplitude modulated radiofrequency electromagnetic fields in combination with standard treatmetnt or as a monotherapy to show improvement in quality of life in patients with advanced hepatocellular carcinoma. J Clin Oncol. (2021) 39:290. doi: 10.1200/JCO.2021.39.3_suppl.290
7. Setogute Y, Costa FP, Sousa MC, Lima PD, Carvalho L, Santos E, et al. Low energy amplitude modulated radiofrequency electromagnetic fields to show antitumor effect in combination with standard treatmetnt or as a monotherapy in patients with advanced hepatocellular carcinoma. J Clin Oncol. (2021) 39(S3):332. doi: 10.1200/JCO.2021.39.3_suppl.332
8. Capareli F, Costa FP, Tuszynski JA, Sousa MC, Setogute Y, Lima PD, et al. Low-energy amplitude-modulated electromagnetic field exposure: feasibility, safety and haemodynamic alterations in patients with advanced hepatocellular carcinoma. Br J Cancer. (2022) [manuscript submitted].
9. Jimenez H, Wang M, Zimmerman JW, Pennison MJ, Sharma S, Surratt T, et al. Tumour-specific amplitude-modulated radiofrequency electromagnetic fields induce differentiation of hepatocellular carcinoma via targeting Cav3.2T-type voltage-gated calcium channels and Ca(2+) influx. EBioMedicine. (2019) 44:209–24. doi: 10.1016/j.ebiom.2019.05.034
10. Kavet R. EMF And current cancer concepts. Bioelectromagnetics. (1996) 17(5):339–57. doi: 10.1002/(SICI)1521-186X(1996)17:5%3C339::AID-BEM1%3E3.0.CO;2-4
11. Schofield Z, Meloni GN, Tran P, Zerfass C, Sena G, Hayashi Y, et al. Bioelectrical understanding and engineering of cell biology. J R Soc Interface. (2020) 17(166):20200013. doi: 10.1098/rsif.2020.0013
12. Mitchell P. Coupling of phosphorylation to electron and hydrogen transfer by a chemi-osmotic type of mechanism. Nature. (1961) 191:144–8. doi: 10.1038/191144a0
13. Zerfaβ C, Asally M, Soyer OS. Interrogating metabolism as an electron flow system. Curr Opin Syst Biol. (2019) 13:59–67. doi: 10.1016/j.coisb.2018.10.001
14. Kalra AP, Eakins BB, Patel SD, Ciniero G, Rezania V, Shankar K, et al. All wired up: an exploration of the electrical properties of microtubules and tubulin. ACS Nano. (2020) 14:16301–20. doi: 10.1021/acsnano.0c06945
15. Salari V, Barzanjeh S, Cifra M, Simon C, Scholkmann F, Alirezaei Z, et al. Electromagnetic fields and optomechanics in cancer diagnostics and treatment. Front Biosci (Landmark Ed). (2018) 23(8):1391–406. doi: 10.2741/4651
16. Costa FP, de Oliveira AC, Meirelles R, Machado MC, Zanesco T, Surjan R, et al. Treatment of advanced hepatocellular carcinoma with very low levels of amplitude-modulated electromagnetic fields. Br J Cancer. (2011) 105(5):640–8. doi: 10.1038/bjc.2011.292
17. Kim JH, Lee JK, Kim HG, Kim KB, Kim HR. Possible effects of radiofrequency electromagnetic field exposure on central nerve system. Biomol Ther (Seoul). (2019) 27(3):265–75. doi: 10.4062/biomolther.2018.152
18. Naarala J, Hoyto A, Markkanen A. Cellular effects of electromagnetic fields. Altern Lab Anim. (2004) 32(4):355–60. doi: 10.1177/026119290403200406
19. Klimek A, Rogalska J. Extremely low-frequency magnetic field as a stress factor-really detrimental?-insight into literature from the last decade. Brain Sci. (2021) 11(2):174. doi: 10.3390/brainsci11020174
20. Saliev T, Begimbetova D, Masoud AR, Matkarimov B. Biological effects of non-ionizing electromagnetic fields: two sides of a coin. Prog Biophys Mol Biol. (2019) 141:25–36. doi: 10.1016/j.pbiomolbio.2018.07.009
21. Zimmerman JW, Pennison MJ, Brezovich I, Yi N, Yang CT, Ramaker R, et al. Cancer cell proliferation is inhibited by specific modulation frequencies. Br J Cancer. (2012) 106(2):307–13. doi: 10.1038/bjc.2011.523
22. Kirson ED, Dbaly V, Tovarys F, Vymazal J, Soustiel JF, Itzhaki A, et al. Alternating electric fields arrest cell proliferation in animal tumor models and human brain tumors. Proc Natl Acad Sci USA. (2007) 104(24):10152–7. doi: 10.1073/pnas.0702916104
23. Kirson ED, Gurvich Z, Schneiderman R, Dekel E, Itzhaki A, Wasserman Y, et al. Disruption of cancer cell replication by alternating electric fields. Cancer Res. (2004) 64(9):3288–95. doi: 10.1158/0008-5472.CAN-04-0083
24. Naveh A, Bomzon Z, Farber O, Urman N, Yesharim O, Kirson E, et al. Abstract 3204: transducer array configuration optimization for treatment of pancreatic cancer using Tumor Treating Fields (TTFields). Cancer Res. (2018) 78(13_Suppl.):3204. doi: 10.1158/1538-7445.AM2018-3204
25. Singh M, Singh T, Soni S. Pre-operative assessment of ablation margins for Variable blood perfusion metrics in a magnetic resonance imaging based Complex breast tumour anatomy: simulation paradigms in thermal therapies. Comput Methods Programs Biomed. (2021) 198:105781. doi: 10.1016/j.cmpb.2020.105781
26. Xu A, Wang Q, Lv X, Lin T. Progressive study on the non-thermal effects of magnetic field therapy in oncology. Front Oncol. (2021) 11:638146. doi: 10.3389/fonc.2021.638146
27. Reite M, Higgs L, Lebet JP, Barbault A, Rossel C, Kuster N, et al. Sleep inducing effect of low energy emission therapy. Bioelectromagnetics. (1994) 15(1):67–75. doi: 10.1002/bem.2250150110
28. Pasche B, Erman M, Hayduk R, Mitler MM, Reite M, Higgs L, et al. Effects of low energy emission therapy in chronic psychophysiological insomnia. Sleep. (1996) 19(4):327–36. doi: 10.1093/sleep/19.4.327
29. Kelly TL, Kripke DF, Hayduk R, Ryman D, Pasche B, Barbault A. Bright light and LEET effects on circadian rhythms, sleep and cognitive performance. Stress Med. (1997) 13(4):251–8. doi: 10.1002/(SICI)1099-1700(199710)13:4%3C251::AID-SMI750%3E3.0.CO;2-0
30. Wallace J, Selmaoui B. Effect of mobile phone radiofrequency signal on the alpha rhythm of human waking EEG: a review. Environ Res. (2019) 175:274–86. doi: 10.1016/j.envres.2019.05.016
31. Misek J, Belyaev I, Jakusova V, Tonhajzerova I, Barabas J, Jakus J. Heart rate variability affected by radiofrequency electromagnetic field in adolescent students. Bioelectromagnetics. (2018) 39(4):277–88. doi: 10.1002/bem.22115
32. Misek J, Veternik M, Tonhajzerova I, Jakusova V, Janousek L, Jakus J. Radiofrequency electromagnetic field affects heart rate variability in rabbits. Physiol Res. (2020) 69(4):633–43. doi: 10.33549/physiolres.934425
33. Andrzejak R, Poreba R, Poreba M, Derkacz A, Skalik R, Gac P, et al. The influence of the call with a mobile phone on heart rate variability parameters in healthy volunteers. Ind Health. (2008) 46(4):409–17. doi: 10.2486/indhealth.46.409
34. Sharma S, Wu SY, Jimenez H, Xing F, Zhu D, Liu Y, et al. Ca(2+) and CACNA1H mediate targeted suppression of breast cancer brain metastasis by AM RF EMF. EBioMedicine. (2019) 44:194–208. doi: 10.1016/j.ebiom.2019.05.038
35. Costa FP, de Oliveira AC, Meirelles R, Zanesco T, Surjan R, Chammas MC, et al. A phase II study of amplitude-modulated electromagnetic fields in the treatment of advanced hepatocellular carcinoma (HCC). J Clin Oncol. (2007) 18(Suppl):15155. doi: 10.1200/jco.2007.25.18_suppl.15155
36. Blackstock AW, Benson AB, Kudo M, Jimenez H, Achari PF, McGrath C, et al. Safety and efficacy of amplitude-modulated radiofrequency electromagnetic fields in advanced hepatocellular carcinoma. 4open. (2021) 4:3. doi: 10.1051/fopen/2021003
37. Davies PC, Demetrius L, Tuszynski JA. Cancer as a dynamical phase transition. Theor Biol Med Model. (2011) 8:30. doi: 10.1186/1742-4682-8-30
38. Koppenol WH, Bounds PL, Dang CV. Otto Warburg’s contributions to current concepts of cancer metabolism. Nat Rev Cancer. (2011) 11(5):325–37. doi: 10.1038/nrc3038
39. Warburg O. Über den Stoffwechsel der Carcinomzelle. Klin Wochenschr. (1925) 4:534–6. doi: 10.1007/BF01726151
40. Demetrius LA, Coy JF, Tuszynski JA. Cancer proliferation and therapy: the Warburg effect and quantum metabolism. Theor Biol Med Model. (2010) 7:2. doi: 10.1186/1742-4682-7-2
41. Geesink H, Meijer D. Quantum wave information of life revealed: an algorithm for electromagnetic frequencies that create stability of biological order, with implications for brain function and consciousness. NeuroQuantology. (2016) 14:106–25. doi: 10.14704/nq.2016.14.1.911
42. Gualdi G, Costantini E, Reale M, Amerio P. Wound repair and extremely low frequency-electromagnetic field: insight from in vitro study and potential clinical application. Int J Mol Sci. (2021) 22(9):5037–49. doi: 10.3390/ijms22095037
43. Singh M. Incorporating vascular-stasis based blood perfusion to evaluate the thermal signatures of cell-death using modified Arrhenius equation with regeneration of living tissues during nanoparticle-assisted thermal therapy. Int Commun Heat Mass Transfer. (2022) 135:106046. doi: 10.1016/j.icheatmasstransfer.2022.106046
44. Dombrovsky LA. Laser-induced thermal treatment of superficial human tumors: an advanced heating strategy and non-arrhenius law for living tissues. Front Therm Eng. (2022) 1:807083. doi: 10.3389/fther.2021.807083
45. Singh M, Ma R, Zhu L. Quantitative evaluation of effects of coupled temperature elevation, thermal damage, and enlarged porosity on nanoparticle migration in tumors during magnetic nanoparticle hyperthermia. Int Commun Heat Mass Transfer. (2021) 126:105393. doi: 10.1016/j.icheatmasstransfer.2021.105393
46. Hodgkin AL, Huxley AF. A quantitative description of membrane current and its application to conduction and excitation in nerve. J Physiol. (1952) 117(4):500–44. doi: 10.1113/jphysiol.1952.sp004764
47. Linz KW, von Westphalen C, Streckert J, Hansen V, Meyer R. Membrane potential and currents of isolated heart muscle cells exposed to pulsed radio frequency fields. Bioelectromagnetics. (1999) 20(8):497–511. doi: 10.1002/(SICI)1521-186X(199912)20:8%3C497::AID-BEM4%3E3.0.CO;2-5
48. Kaestner L, Wang X, Hertz L, Bernhardt I. Voltage-activated ion channels in non-excitable cells-a viewpoint regarding their physiological justification. Front Physiol. (2018) 9:450. doi: 10.3389/fphys.2018.00450
49. Tuszynski JA, Wenger C, Friesen DE, Preto J. An overview of sub-cellular mechanisms involved in the action of TTFields. Int J Environ Res Public Health. (2016) 13(11):1128–50. doi: 10.3390/ijerph13111128
50. Vadala M, Morales-Medina JC, Vallelunga A, Palmieri B, Laurino C, Iannitti T. Mechanisms and therapeutic effectiveness of pulsed electromagnetic field therapy in oncology. Cancer Med. (2016) 5(11):3128–39. doi: 10.1002/cam4.861
51. Cosic I, Cosic D, Lazar K. Is it possible to predict electromagnetic resonances in proteins, DNA and RNA? EPJ Nonlinear Biomed Phys. (2015) 3:5–12. doi: 10.1140/epjnbp/s40366-015-0020-6
52. Cosic I. The resonant recognition model of macromolecular bioactivity : theory and applications. Basel, Boston: Birkhäuser Verlag (1997). xii, 143 p.
53. Kim J, Ha CS, Lee HJ, Song K. Repetitive exposure to a 60-Hz time-varying magnetic field induces DNA double-strand breaks and apoptosis in human cells. Biochem Biophys Res Commun. (2010) 400(4):739–44. doi: 10.1016/j.bbrc.2010.08.140
54. Elson EI. The little explored efficacy of magnetic fields in cancer treatment and postulation of the mechanism of action. Electromagn Biol Med. (2009) 28(3):275–82. doi: 10.3109/15368370903114271
55. Tuszynski JA, Friesen D, Freedman H, Sbitnev VI, Kim H, Santelices I, et al. Microtubules as sub-cellular memristors. Sci Rep. (2020) 10(1):2108. doi: 10.1038/s41598-020-58820-y
56. Gutierrez BC, Pita Almenar MR, Martinez LJ, Sineriz Louis M, Albarracin VH, Cantero MDR, et al. Honeybee brain oscillations are generated by microtubules. The concept of a brain central oscillator. Front Mol Neurosci. (2021) 14:727025. doi: 10.3389/fnmol.2021.727025
57. Santelices IB, Friesen DE, Bell C, Hough CM, Xiao J, Kalra A, et al. Response to alternating electric fields of tubulin dimers and microtubule ensembles in electrolytic solutions. Sci Rep. (2017) 7(1):9594. doi: 10.1038/s41598-017-09323-w
58. Pokorny J, Vedruccio C, Cifra M, Kucera O. Cancer physics: diagnostics based on damped cellular elastoelectrical vibrations in microtubules. Eur Biophys J. (2011) 40(6):747–59. doi: 10.1007/s00249-011-0688-1
59. Friesen DE, Craddock TJ, Kalra AP, Tuszynski JA. Biological wires, communication systems, and implications for disease. Biosystems. (2015) 127:14–27. doi: 10.1016/j.biosystems.2014.10.006
60. Sahu S, Ghosh S, Ghosh B, Aswani K, Hirata K, Fujita D, et al. Atomic water channel controlling remarkable properties of a single brain microtubule: correlating single protein to its supramolecular assembly. Biosens Bioelectron. (2013) 47:141–8. doi: 10.1016/j.bios.2013.02.050
61. Sahu S, Ghosh S, Hirata K, Fujita D, Bandyopadhyay A. Multi-level memory-switching properties of a single brain microtubule. Appl Phys Lett. (2013) 102:123701. doi: 10.1063/1.4793995
62. MdR C, Perez PL, Smoler M, Villa Etchegoyen C, Cantiello HF. Electrical oscillations in two-dimensional microtubular structures. Sci Rep. (2016) 6:27143. doi: 10.1038/srep27143
63. Cantero MDR, Villa Etchegoyen C, Perez PL, Scarinci N, Cantiello HF. Bundles of brain microtubules generate electrical oscillations. Sci Rep. (2018) 8(1):11899. doi: 10.1038/s41598-018-30453-2
64. Woods LC, Berbusse GW, Naylor K. Microtubules are essential for mitochondrial dynamics – fission, fusion, and motility – in dictyostelium discoideum. Front Cell Dev Biol. (2016) 4:1–9. doi: 10.3389/fcell.2016.00019
65. Scholkmann F. Long range physical cell-to-cell signalling via mitochondria inside membrane nanotubes: a hypothesis. Theor Biol Med Model. (2016) 13(1):16. doi: 10.1186/s12976-016-0042-5
66. Rustom A, Saffrich R, Markovic I, Walther P, Gerdes HH. Nanotubular highways for intercellular organelle transport. Science. (2004) 303(5660):1007–10. doi: 10.1126/science.1093133
67. Onodera Y, Nam J-M, Horikawa M, Shirato H, Sabe H. Arf6-driven cell invasion is intrinsically linked to TRAK1-mediated mitochondrial anterograde trafficking to avoid oxidative catastrophe. Nat Commun. (2018) 9(1):2682. doi: 10.1038/s41467-018-05087-7
68. Fu Y, Liu S, Yin S, Niu W, Xiong W, Tan M, et al. The reverse Warburg effect is likely to be an Achilles’ heel of cancer that can be exploited for cancer therapy. Oncotarget. (2017) 8(34):57813–25. doi: 10.18632/oncotarget.18175
69. Gaál Z. MicroRNAs and metabolism: revisiting the Warburg effect with emphasis on epigenetic background and clinical applications. Biomolecules. (2021) 11(10):1531–47. doi: 10.3390/biom11101531
70. Gatenby RA, Gillies RJ. Why do cancers have high aerobic glycolysis? Nat Rev Cancer. (2004) 4(11):891–9. doi: 10.1038/nrc1478
71. Nikoloski N, Frohlich J, Samaras T, Schuderer J, Kuster N. Reevaluation and improved design of the TEM cell in vitro exposure unit for replication studies. Bioelectromagnetics. (2005) 26(3):215–24. doi: 10.1002/bem.20067
72. Singh M, Gu Q, Ma R, Zhu L. Heating protocol design affected by nanoparticle redistribution and thermal damage model in magnetic nanoparticle hyperthermia for cancer treatment. J Heat Transfer. (2020) 142:07250. doi: 10.1115/1.4046967
73. Gosselin MC, Neufeld E, Moser H, Huber E, Farcito S, Gerber L, et al. Development of a new generation of high-resolution anatomical models for medical device evaluation: the Virtual Population 3.0. Phys Med Biol. (2014) 59(18):5287–303. doi: 10.1088/0031-9155/59/18/5287
74. Suzuki K, Epstein ML, Kohlbrenner R, Garg S, Hori M, Oto A, et al. Quantitative radiology: automated CT liver volumetry compared with interactive volumetry and manual volumetry. AJR Am J Roentgenol. (2011) 197(4):W706–12. doi: 10.2214/AJR.10.5958
75. Capstick M. IT'IS Foundation, Zürich, Switzerland. Personal communication and data on file at Autem Medical. (2022).
76. Lam YY, Ravussin E. Analysis of energy metabolism in humans: a review of methodologies. Mol Metab. (2016) 5(11):1057–71. doi: 10.1016/j.molmet.2016.09.005
77. Wang B, Hsu SH, Frankel W, Ghoshal K, Jacob ST. Stat3-mediated activation of microRNA-23a suppresses gluconeogenesis in hepatocellular carcinoma by down-regulating glucose-6-phosphatase and peroxisome proliferator-activated receptor gamma, coactivator 1 alpha. Hepatology. (2012) 56(1):186–97. doi: 10.1002/hep.25632
Keywords: electromagnetic fields, radiofrequency, cancer therapy, microtubules, metabolism, mitochondria, hepatocellular carcinoma, Warburg effect
Citation: Tuszynski JA and Costa F (2022) Low-energy amplitude-modulated radiofrequency electromagnetic fields as a systemic treatment for cancer: Review and proposed mechanisms of action. Front. Med. Technol. 4:869155. doi: 10.3389/fmedt.2022.869155
Received: 3 February 2022; Accepted: 19 August 2022;
Published: 8 September 2022.
Edited by:
Sundeep Singh, University of Calgary, CanadaReviewed by:
Ola Rominiyi, The University of Sheffield, United KingdomManpreet Singh, University of Maryland, Baltimore County, United States
© 2022 Tuszynski and Costa. This is an open-access article distributed under the terms of the Creative Commons Attribution License (CC BY). The use, distribution or reproduction in other forums is permitted, provided the original author(s) and the copyright owner(s) are credited and that the original publication in this journal is cited, in accordance with accepted academic practice. No use, distribution or reproduction is permitted which does not comply with these terms.
*Correspondence: Jack A. Tuszynski amFja3RAdWFsYmVydGEuY2E=
Specialty Section: This article was submitted to Diagnostic and Therapeutic Devices, a section of the journal Frontiers in Medical Technology
Abbreviations ATP, adenosine triphosphate; EMF, electromagnetic field; HCC, hepatocellular carcinoma; LEAM RF EMF, Low-energy amplitude-modulated radiofrequency electromagnetic fields; TTFields, Tumor Treating Fields