- 1Neural Tissue Engineering Keele, School of Life Sciences, Keele University, Keele, United Kingdom
- 2Department of Chemistry, Lancaster University, Lancaster, United Kingdom
- 3Materials Science Institute, Lancaster University, Lancaster, United Kingdom
Spinal cord injury (SCI) is a serious condition caused by damage to the spinal cord through trauma or disease, often with permanent debilitating effects. Globally, the prevalence of SCI is estimated between 40 to 80 cases per million people per year. Patients with SCI can experience devastating health and socioeconomic consequences from paralysis, which is a loss of motor, sensory and autonomic nerve function below the level of the injury that often accompanies SCI. SCI carries a high mortality and increased risk of premature death due to secondary complications. The health, social and economic consequences of SCI are significant, and therefore elucidation of the complex molecular processes that occur in SCI and development of novel effective treatments is critical. Despite advances in medicine for the SCI patient such as surgery and anaesthesiology, imaging, rehabilitation and drug discovery, there have been no definitive findings toward complete functional neurologic recovery. However, the advent of neural stem cell therapy and the engineering of functionalized biomaterials to facilitate cell transplantation and promote regeneration of damaged spinal cord tissue presents a potential avenue to advance SCI research. This review will explore this emerging field and identify new lines of research.
Introduction
Spinal cord injury (SCI) often results in significant neurological dysfunction and long-term disability. Globally, the prevalence of SCI is estimated between 40 to 80 cases per million population per year (1), with approximately 1000 new cases per year in the United Kingdom (UK) (2). SCI is often associated with negative health and socioeconomic effects for patients, affecting young males at comparatively higher proportions than females in age-matched controls (3). Secondary complications that are widely encountered clinically include respiratory dysfunction, loss of genitourinary and gastrointestinal function, thromboembolic disease, pressure sores, neuropathic pain, spasticity and obesity (4). Furthermore, the lack of physical activity from paralysis contributes to the development of coronary artery disease, hyperlipidaemia, insulin resistance and psychosocial issues of disability in chronic SCI patients (5). The management of SCI carries substantial economic impacts with high lifetime costs averaging £1.12 million per SCI case in the UK (6). It is quite evident that the health, social and economic consequences of SCI are significant, and therefore elucidation of the complex molecular processes that occur in SCI and development of novel effective treatments is critical. To date, there has been a wealth of research into treatments for SCI albeit without successful clinical translation (Figure 1). Herein we explore the combination of stem cell therapy and electroactive biomaterials as a potential solution to this problem.
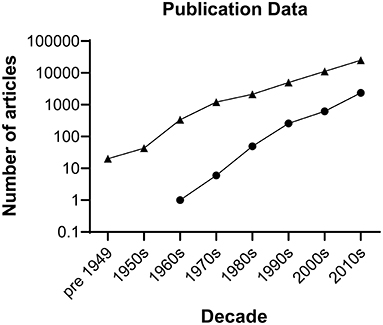
Figure 1. Number of publications by decade on spinal cord injury. Triangles represent hits generated searching the Web of Science repository performed on 16 December 2021 for literature describing spinal cord injury using the following keywords: (spinal cord injury) AND (spinal cord injury and imaging) OR (spinal cord injury and rehabilitation) OR (spinal cord injury and intervention) OR (spinal cord injury and surgery) OR (spinal cord injury and drug delivery) AND (spinal cord repair) AND (spinal cord injury and bioengineering) NOT (tumour) NOT (cancer). The search retrieved 42,923 articles. Circles represent hits generated searching the Cochrane Library for literature describing clinical trial articles on interventions for spinal cord injury by decade. The search retrieved 3,281 articles.
Current Clinical Management Strategies Are Not Truly Regenerative
As no cure exists, the routine clinical management of SCI aims to prevent further injury and disability through following strategies widely recommended by clinical guidelines across multiple healthcare systems (7–9). On admission to the emergency room, evidence-based guidance recommends a clinical assessment and, often, stabilization of the spine through traction realignment surgery to prevent further injury to the spinal cord (1, 8). Radiographic evaluation and other imaging techniques such as magnetic resonance imaging, are routinely performed to map the lesion's location to define the level and severity of injury on the spinal cord and column, thereby setting surgical outcomes (10). Further surgical recommendations for SCI post realignment aim to remove fractured bone and disk fragments, foreign objects or the repair of slipped disks. Often, decompression of pressure from cerebrospinal fluid that presses on the spinal cord (decompression laminoplasty) is performed within at least 24 h post-trauma to preserve surviving neurons from blood brain barrier breach processes and reduce the risk of secondary injury (11). Several studies suggest that early surgical management in the acute phase is intrinsically connected to an earlier initiation of rehabilitation protocols and an improvement in neurological outcomes (12, 13). In the scope of recommended guidelines for SCI management, timely surgical intervention essentially serves as a neuroprotective strategy and not a predictor of full functional recovery. An alternative school of thought points to recumbence as a management option for SCI as opposed to surgical decompression. There is evidence that Active Physiologic Conservative Management (ACPM) yields neurological recovery by reducing neurological deterioration, purported to be at risk of being exacerbated by surgical management (14–16). ACPM is reported to bear advantages over costly surgery in that neuropathic pain may be minimized in patients while allowing for maximal range of motion outcomes, dependent on the injury (17). However, a Cochrane review concluded that there was insufficient evidence to determine whether surgical management bears advantages over conservative management of spinal burst fractures (18). Clearly these management options would likely require well designed clinical trials to determine the equality or superiority of each management option in relation to neurological outcomes in SCI. Crucially though, no current therapeutic route is truly regenerative and cannot restore circuitries that have been lost after SCI.
The Complexity of SCI is a Major Challenge to Successful Repair
As outlined above there is a market need to develop new therapies which can restore neural pathways after SCI. However, this constitutes a major challenge given the complexity of the tissue and the processes that occur with associated injury. The spinal cord is part of the central nervous system (CNS) and is the major conduit and reflex center between the peripheral nervous system and the brain. Anatomically, a transverse section of the spinal cord can be divided into two sections: the white and gray matter. The white matter surrounds the gray matter and contains axons that form nerve tracts ascending to and descending from the brain. The gray matter in the center contains nerve cell bodies of both projection neurons and interneurons which form a complex circuitry to regulate neural information at the level of the spinal cord. Crucial to the function of the nervous system are the surrounding glial cells which consist of the astrocytes, oligodendrocytes, ependymal cells and microglia derived from blood. In the white matter, axons are ensheathed by myelin, produced by the oligodendrocytes. Astrocytes have many hypothesized functions including clearance and recycling of neurotransmitters at synapses; maintaining homeostasis; providing metabolic support to neural cells and responding and regulating the nervous system response to injury. Microglia are sometimes considered the immune cells of the brain and also mediate response to injury, whilst phagocytosing debris and releasing cues to surrounding cell types. Finally, the spinal cord parenchyma is separated from the blood-stream via a mixture of astrocytes, endothelial cells and pericytes which form the blood brain barrier (BBB). The first challenge to repairing the spinal cord then is to restore this complex cytoarchitecture essential for function.
The pathophysiology of SCI is also complex, characterized by the loss or degradation of motor, sensory and autonomic functions. A series of biological events have been identified that begin soon after the initial trauma (described as the primary injury process) and last for several hours, days and even up to years (contributing to what is described as the secondary injury process). From the onset of the initial trauma, blood-brain-barrier breach and tissue damage, an interdependent series of cellular and systemic events occur in the nervous, vascular and immune systems as they respond to injury, the exact details of which is still a major line of active research (19, 20). The timeline of events from the onset of injury is illustrated in Table 1. It is understood that an inflammatory cascade occurs as reactive astrocytes, macrophages, activated microglia and lymphocytes infiltrate the injury. These immune cells have been postulated to have the main function of clearing necrotic nerve fibers and myelin debris within the extracellular space of the spinal cord lesion (21). However these immune cells release pro-inflammatory cytokines, chemokines, free radicals and nitric oxide that further exacerbate secondary neuronal and glial death and subsequent necrosis during the inflammatory cascade (22). Whilst inflammation undoubtedly has an important role in stabilizing injury, there may be therapeutic opportunities for the modulation of inflammatory procedures to favor the environment for regeneration. Of the cumulative biochemical changes that occur in the secondary injury processes, the glial scar, a dynamic structure, forms over the time course from injury and largely plays a role in the inhibition of regeneration. It is evident that the glial scar contains multiple cellular components and a complex extracellular matrix (ECM) that induces a response to SCI which paradoxically inhibits regeneration whilst remodeling the spinal cord tissue to contain the injury (Figure 2). Activated astrocytes and microglia have been shown to secrete several types of proteoglycans such as chondroitin sulfate proteoglycans (CSPG), NG2 proteoglycan and phosphacan that are associated with scar tissue (24). Proteoglycans are ECM molecules which play roles in axonal plasticity, regeneration and remyelination. However, these ECM molecules form a chemical and physical barrier with reactive astrocytes around the lesion core, thereby impeding the formation of neural circuitry across the lesion (23, 25, 26). Furthermore, the distal endings of severed axons form dystrophic growth cones as they are exposed to myelin-associated inhibitors (MAI) released by necrotic oligodendrocytes, thus contributing to the scar and cavity formation (27). Some axonal sprouting, synapse formation and remyelination has been shown to occur in spared tissue through to the chronic phase of SCI, mediated by neural plasticity (28). However, the glial scar persists, hindering any endogenous regeneration of neural tissue at the injury site (29, 30).
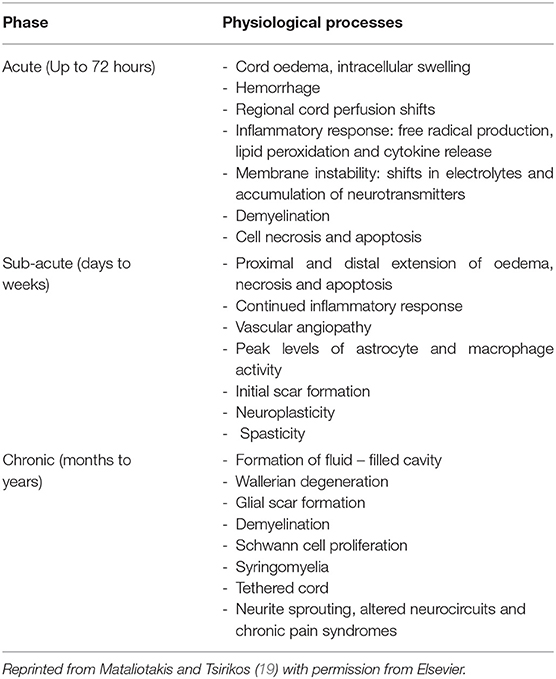
Table 1. A timeline of the sequence of pathophysiological processes that occur from the onset of primary injury to secondary injury.
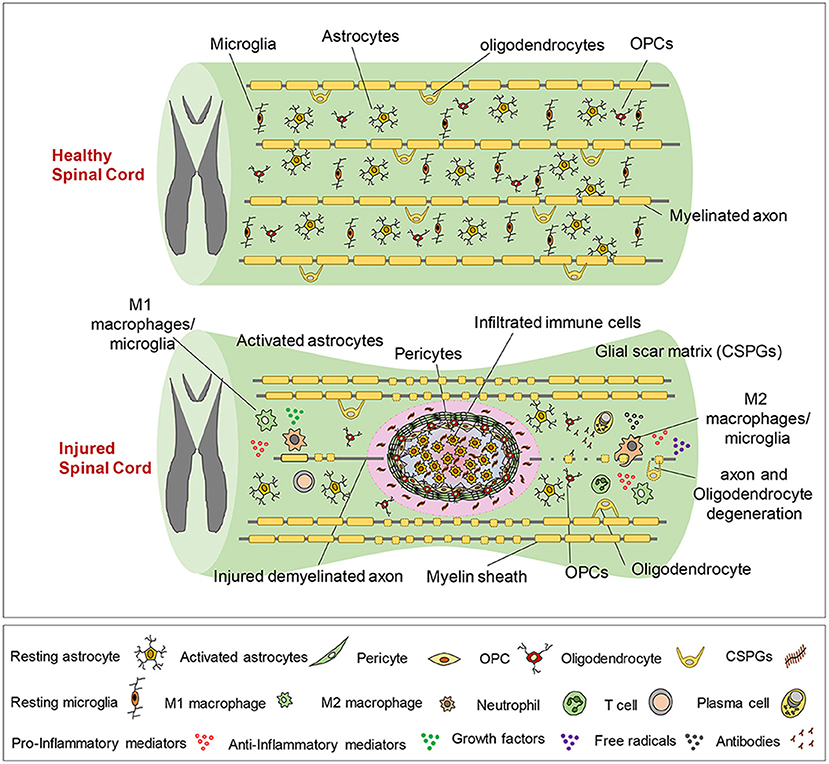
Figure 2. The pathophysiological processes of SCI. The healthy spinal cord is composed of quiescent astrocytes and microglia. Upon injury a series of complex events occur over time, including the infiltration of lymphocytes that initiate inflammatory processes at the lesion site. The release of proteoglycans by activated astrocytes and microglia results in the formation of a chemical and physical barrier with reactive astrocytes around the lesion core. Dystrophic growth cones form and limited axonal sprouting and remyelination occurs through to the chronic phase of injury. Reproduced from Alizadeh et al. (23) with permission from Frontiers.
Given the multiple inhibitory mechanisms present and limited intrinsic regeneration, an efficacious therapy for the successful repair of SCI would need to address all, if not most of the barriers to endogenous regeneration. The majority of experimental therapies aim to address one or two mechanisms of secondary injury processes with little success. It is therefore imperative to follow a combinatorial approach over the time course of SCI to facilitate neural tissue repair of the injured spinal cord.
Stem Cell Therapy as the Basis for Combinatorial Therapy
As previously discussed, SCI is complex, and this is reflected to date where almost all therapies that have shown promise at the preclinical stage have failed to translate into clinically effective treatments. Clearly, a combination of therapies would ideally be required to overcome the inhibitory environment in SCI through modulating the immune response, replacing lost cells and promoting nerve fiber growth to restore neuronal connectivity. In light of the above, stem cells are of particular interest as an avenue to treat SCI. Stem cells are defined as immature cells that have the capacity to self-renew and to develop into specialized cells, that is, they can become any cell type present within an organism. A wealth of research has gone into the identification and characterization of stem cells, leading to a profound interest in their potential for the treatment of SCI, traumatic brain injury and neurodegenerative diseases (31, 32). Preclinical studies indicate that the transplantation of stem cells may contribute to spinal cord repair by: replacing lost nerve cells; generating new nerve and glial cells that can act as a bridge across the injury site to stimulate the regeneration of damaged axons; protecting the cells at the injury site from further cell death by releasing protective growth factors (33) and preventing the spread of the injury by modulating the inflammatory response mediated after SCI (34). This section will outline some stem cell candidates for SCI therapy and argue neural stem cells (NSCs) may be particularly attractive as a transplant population.
Stem Cell Candidates for SCI Cell Therapy
A small number of ependymal cells, the endogenous stem cells of the CNS, have been characterized in the spinal canal. On review, it is believed that these endogenous multipotent cells are unlikely to contribute to any regeneration after SCI (35). The general consensus suggests that an upregulation of neurotrophic factors such as ciliary neurotrophic factor (CNTF) after injury largely promotes astrocytic differentiation of these multipotent cells. Johe et al. (36) report up to 98% of these cells differentiate into astrocytes in vitro through glial fibrillary acid protein (GFAP) expression analysis. Further reports have since corroborated this finding (37), which was initially discovered through pioneering research by Hughes et al. (38). In contrast, factors that promote neurogenesis and oligodendrogenesis such as brain derived neurotrophic factor (BDNF) which is largely secreted by neurons, are observed at low levels due to neuronal cell death (39, 40). The main idea from this research indicates that injury promotes the significant differentiation of ependymal cells to astrocytic cell fates which appear not to contribute to regeneration in the injured spinal cord, and, conversely contribute to the development of allodynia in SCI. The regeneration of neurons and oligodendrocytes, the other two major cell types within the CNS, is certainly crucial to reconstitute damaged tissue. Therefore, exogenous cell therapy bears the potential to replace lost neuronal and glial cells and also provide neurotrophic factors which are crucial to neural tissue repair. Recent advances in stem cell technology have translated into unlimited sources of neural progenitors and glial cells for cell based therapy. Olfactory ensheathing cells (OECs), NSCs, mesenchymal stem cells (MSCs), embryonic stem cells (ESCs) and induced pluripotent stem cells (iPSCs) have been widely assessed as cell sources for SCI cell therapy. OECs, albeit not stem cells, have been studied in neural tissue repair applications and shown to promote the growth of olfactory receptor neurons, and regenerate axons in pre-clinical SCI models (41). Nakhjavan-Shahrak et al. (42) conducted a meta-analysis to determine the efficacy of OEC transplantation across several preclinical studies and clinical trials, yielding some limitations. It appears that a significant limitation of this cell therapy appears to be the lack of effect on allodynia and possibility of aggravating hyperalgesia, despite significant motor function improvements reported consistently across preclinical studies (43–47). Whilst trial data largely concludes the feasibility and clinical safety of OEC therapy in SCI patients, reproducible and robust functional recovery has not been reported (48–50). Alternatively, MSCs indicate moderate therapeutic efficacy for the treatment of various neurological diseases and can be acquired from multiple autologous and allogeneic sources (51). MSCs can self-renew, differentiate into several lineages and have been shown to participate in immunomodulation that may reduce secondary injury and enhance remyelination and axonal regeneration in SCI (52–55). However, it is evident that further developments are required to determine the optimal source of MSCs for SCI and to determine the extent in which MSCs can replace neurons. “Neuron-like” cells have been generated from MSCs and characterized with variable purity and heterogeneity post-differentiation across several reports in the literature (55). Interestingly, a comparative study of bone-marrow derived MSCs (BM-MSCs), neural progenitors derived from a spinal fetal cell line (SPC-01) and iPSCs suggested the latter as the efficacious cell candidate for SCI cell therapy in rat SCI models (56). Such findings correlate with previously reported limitations of MSCs, attributed to improved graft survival, reduction of glial scarring, tissue sparring and increased axonal sprouting in iPSCs as reported by the authors. The current clinical trial data consistently indicates the safety of MSCs, however trials have failed to progress to Phase III due to findings of modest clinical efficacy, despite promising clinical developments underway (57–61). Indeed, there are reports of a combinatorial approach where the co-transplantation of multiple cell candidates, including MSCs, OECs and NSCs, has been tested in a bid to develop an efficacious cell therapy with synergistic cell properties for SCI repair (62–64). The outcomes are variable, although on review appear promising as a growing number of studies are following this approach in cell therapy applications for neurological diseases (65). However, a cell candidate that can enhance the key aspects of regeneration including cell survival, engraftment and migration into the lesion and provide neuroprotection may be the most viable strategy.
NSCs Are a Key Transplant Population for Repairing SCI
NSCs are multipotent cells that can self-renew and generate all the specialized neural cells within the spinal cord, that is, neurons, astrocytes and oligodendrocytes. NSCs are capable of surviving, migrating and differentiating into the aforementioned major cell types of the CNS to promote regeneration (66–68). The trilineage differentiation potential of NSCs is a remarkable feature following demyelination and cell death induced by SCI. For example, facilitation of remyelination by transplantation of exogenous NSCs, the myelinating cells of the CNS, has been reported across many studies (27, 69, 70). Furthermore, NSCs have been shown to secrete a large number of soluble factors including neurotrophins such as BDNF, CNTF, glial cell derived neurotrophic factor (GDNF) and nerve growth factor (NGF) across several studies (71). Schubert et al. (39) have since characterized the proteins secreted by NSCs. BDNF in particular, is associated with the survival and proliferation of damaged neurons to promote an environment permissive to growth. Astrocytes have also been shown to secrete some neurotrophic factors for axonal remodeling and plasticity, as well as homeostatic support functions relevant for spinal cord repair (72, 73). Such neural precursor cells may be derived from pluripotent stem cells, primary CNS tissue and potentially from human somatic cells once a robust and ethical protocol for transdifferentiation is developed as illustrated in Figure 3 (75–80). Pluripotent stem cells carry some ethical concerns and have been shown to carry karyotypic abnormalities and a risk of tumorigenicity (81, 82). Induced Pluripotent Stem Cells (iPSCs) in particular have lower ethical implications and immunogenic concerns as they can be derived from patient-matched sources and collected using non-invasive methods. It must be noted that the potential for genetic defects, tumorigenicity and immunogenicity of transplanted cells have largely hindered the successful clinical translation of iPSC-derived cell therapies for SCI (83). An accumulation of evidence for the safety of iPSC cell therapy is actively progressing for applications in the CNS. For example, efficient techniques to differentiate and modify cells prior to transplantation are being investigated in a bid to reduce the risk of teratoma formation in preclinical models (84, 85). Such developments have continued to emerge where Khazaei et al. (86) demonstrated the possibility of enhancing neuronal differentiation and even improving motor function in rodents through counteracting Notch signaling by expressing GDNF in transplanted NPCs, thus indicating potential to enhance the safety and efficacy of iPSC derived NSC therapy for SCI. Further hurdles exist, such as lineage control in neuronal differentiation, which is out of scope of this review.
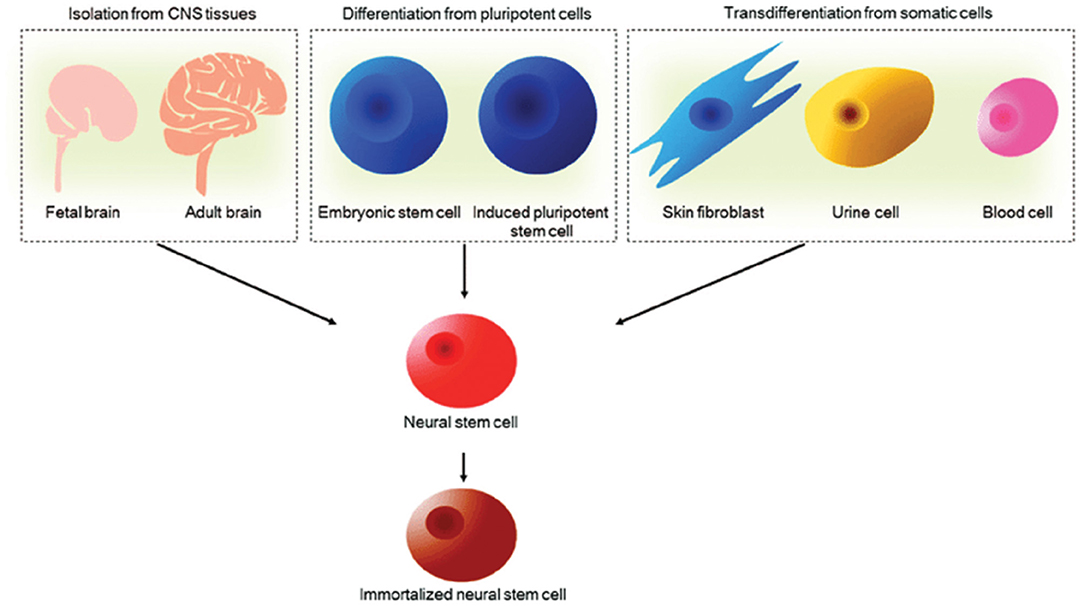
Figure 3. Sources of NSCs. NSCs can be derived from primary CNS tissues such as fetal and adult brain, pluripotent embryonic stem cells and induced pluripotent stem cells and transdifferentiated from human somatic cells. NSCs can be further immortalized to facilitate long-term culture for cell therapy applications. Reproduced from Tang et al. (74) with permission from Springer Nature.
Preclinical research largely reports significant functional improvements when NSCs are transplanted into SCI models. Based on their potential advantages, NSCs have been tested in phase I/IIa clinical trials for SCI cell therapy, which have been identified in the literature and listed below:
• Shin et al. (87), n = 19
• Ghobrial et al. (88), n = 5
• Curtis et al. (89), n = 4
• Levi et al. (90), n = 31
The low number of studies and small sample sizes across the studies invariably limits the quality of evidence for safety and efficacy outcomes for NSC therapy on review. Whilst a limited number of phase I/IIa clinical trials currently exist, Tiwari et al. (91) conclude the safety of NSC transplantation for SCI in humans in a systematic review. The conclusions from the systematic review largely indicate the safety and tolerability of NSC transplantation, albeit the lack of a quantitative assessment of adverse events and serious adverse events across the studies. Furthermore, clinical efficacy reports appear to show modest improvements in functional recovery. Such promising findings are highlighted by Shin's group whom reported positive motor and sensory outcomes from their phase I/IIa clinical trial when fetal cerebral NSCs were transplanted into 19 traumatic cervical SCI patients (87). There was no evidence of tumor formation, exacerbation of neurological deterioration or neuropathic pain and spasticity, thus warranting further investigation of NSCs as SCI cell therapy candidates. Another promising insight indicates that neural stem/progenitor (NSPC) grafts can successfully integrate into spinal cord lesions and generate extensive neuronal relays across the lesion and host axon regeneration into the lesion (92–94). Ceto et al. (95) have since expanded these findings by assessing the synaptic architecture of grafted NSCS. An important finding is the formation of functional synaptic connections to corroborate the functional improvements observed in NSC grafts through animal SCI studies.
Alongside their regenerative benefits, NSCs can also be isolated and rapidly expanded as neurospheres in culture through well-established protocols (96), to generate the numbers required for transplantation. They can also be manipulated in vitro to potentially improve cell transplantation, for example, through genetic engineering (97, 98). However, challenges still exist, some of which are generally consistent with other cell therapy candidates for SCI repair.
Challenges for NSC Transplantation in SCI
While exogenous NSC transplantation offers promise in reconstituting the architecture of the damaged spinal cord and promoting regeneration as discussed in the previous section, challenges for clinical translation have been encountered. Two key challenges are (i) the low survival and lack of retention of viable cell transplant populations at the injury site and (ii) the inability to control differentiation fates post transplantation as NSCs largely differentiate into astrocytes under pathological conditions. For example, Webber et al. (99) transplanted rat fetal NPCs into the dorsal column lesion site of adult rats and examined the survival of transplanted cells at 24h, 1 week, 2 weeks and 6 weeks after injury. Only minor sensory function improvement was observed and no motor function recovery was observed. The group suggested this to be the result of a high differentiation rate (40%) of grafted stem cells into glial cells as only 8% displayed neuronal morphology post-transplantation. Other reports indicate that recruited NSCs largely differentiate into astrocytes and, to a lesser degree, oligodendrocytes, but without evidence of neurogenesis after injury (100). However, Piltti et al. (101) observed predominantly oligodendrocytic differentiation when NSCs were transplanted into host parenchyma, and higher astrocytic differentiation in lesion-site transplantation in contusion injury rats suggesting the gross influence of the injury microenvironment on cell fates in vivo. Earlier studies concluded that NSC transplantation is associated with increased allodynia in animal behavioral studies, purported to be linked to the high astrocytic differentiation and maladaptive plasticity (102, 103). Thus a rationale to affect some control on cell fates in cell transplant populations could be important to improve functional outcome. Further challenges observed are the poor migration of the grafted cells and directionally guided axonal growth through the inhibitory microenvironment of the lesion and scar tissue (104). In light of the challenges of cell transplantation and the outcomes of NSC therapy in recent trials, tissue engineering presents as a promising avenue to address the limitations of cell differentiation fates, survival and integration of transplant populations to effect functional recovery.
Biomaterial Encapsulation of NSCs Could Improve Regeneration
Implantable biomaterials present as a viable solution to the limitations of cell therapy in SCI as they hold potential for combinatorial therapy. Biomaterials can function as carrier vehicles for encapsulated stem cells in order to enhance survival and engraftment at the site of transplantation as reported in injury models in the CNS. Previously, biomaterials have been functionalized as drug delivery vehicles and bioactive molecule carriers, for example, to facilitate NSC survival and promote significant aligned axonal growth through BDNF and growth factor cocktail incorporation in SCI models (105–107). Furthermore, biomaterials have been stiffness-matched to CNS tissue which appears to favor cell attachment and reduce inflammatory and immune responses, conducive to cell survival and regeneration in the inhibitory microenvironment of the injured spinal cord (108–111). There is a large body of research concerning the use of implantable materials to aid transplantation of cells into sites of neurological injury. This has been well-covered elsewhere (112–114). Significant functional recovery outcomes have been observed consistently at the preclinical stage when NSCs have been delivered via a supporting scaffold matrix over the last decade (115–117), and yet to be clinically translated. Meanwhile, clinical developments are underway regarding the safety and feasibility of implantable biomaterials for CNS repair. Xiao and colleagues report the feasibility and safety of transplanting NeuroRegen, an implantable collagen scaffold in complete chronic SCI patients, albeit the small sample size in the study (118). However further developments are required before progress can be made toward an efficacious implantable cell therapy for SCI. Design strategies for biomaterials have certainly advanced in the last decade: biomaterials can be tailored to enhance neural regeneration by modifying physical properties in the biofabrication process through 3D bioprinting and electrospinning technologies. Developments in electrospinning technology bear potential to enhance the anisotropic extension of neurites and neuronal differentiation when cultured as a substrate for NSCs, critical properties for CNS tissue regeneration (119). Alongside myriad advantages of biomaterial mediated cell transplantation, an emergent area of biomaterial design for neural tissue repair integrates electric conductivity and electric stimulation (ES) which have been shown to influence neurogenesis, proliferation, migration, cell-cell interactions and the modulation of synapse formation (120–124). We believe there may be specific advantages to improving the regenerative potential of NSCs by encapsulation in electroactive biomaterials for implantation, which is where we will focus the next parts of the review.
Rationale for Incorporating Electrical Stimulation Into Cell Transplantation Strategies and Challenges
Over the years, researchers have identified how endogenous electric fields play a critical role in the developing CNS and pathophysiological states since Luigi Galvani's landmark bioelectricity experiment (125). The presence of endogenous electric fields in the developing CNS has been well characterized. Ionic currents have been detected in the developing nervous systems of vertebrates (126). Furthermore, the disruption of the electric fields induced by ionic currents has been linked to developmental abnormalities in early pioneering studies (127). Endogenous electrical fields have been recorded during development and following injury, affecting the orientation of astrocytes and neurons, proliferation and neurite outgrowth of neurons and glia which has been demonstrated in vitro and in a rat sciatic nerve injury model (123, 128, 129). Similarly, early peripheral nerve studies report the benefits of delivering ES to transection injury models in adult rats. For example, ES significantly enhanced dorsal root ganglion (DRG) sensory neurons to regenerate axons after femoral trunk transection and surgical repair. This correlated with an increase in expression of growth-associated protein 43 (GAP-43) mRNA in the regenerating neurons (130). Further evidence in the literature suggests that ES upregulates neurotrophic factor release, which is essential to regeneration (Figure 4). Wenjin et al. (131) reported an increase in BDNF expression in spinal cord neurons after brief electrical stimulation for 1 h at 20 Hz after sciatic nerve transection compared to control and untreated sciatic nerve transection groups. These findings corroborated earlier work by Al-Majed et al. (132) in rat femoral neurons. In order to fully realize the potential of ES for SCI, a better understanding of the mechanisms through which ES enhances neural plasticity is warranted. Whilst peripheral nerve injury studies have largely informed the literature on SCI induced neuropathic pain, Vivó et al. (133) report enhanced axonal regeneration and functional sensory outcomes when ES was administered immediately after nerve injury in a sciatic nerve injury model. Such observations are promising and indicate the potential role that ES may play in facilitating the regeneration of nervous tissue in SCI, however there are limitations of the concept in this developing area of cell therapy, pertinent to CNS repair.
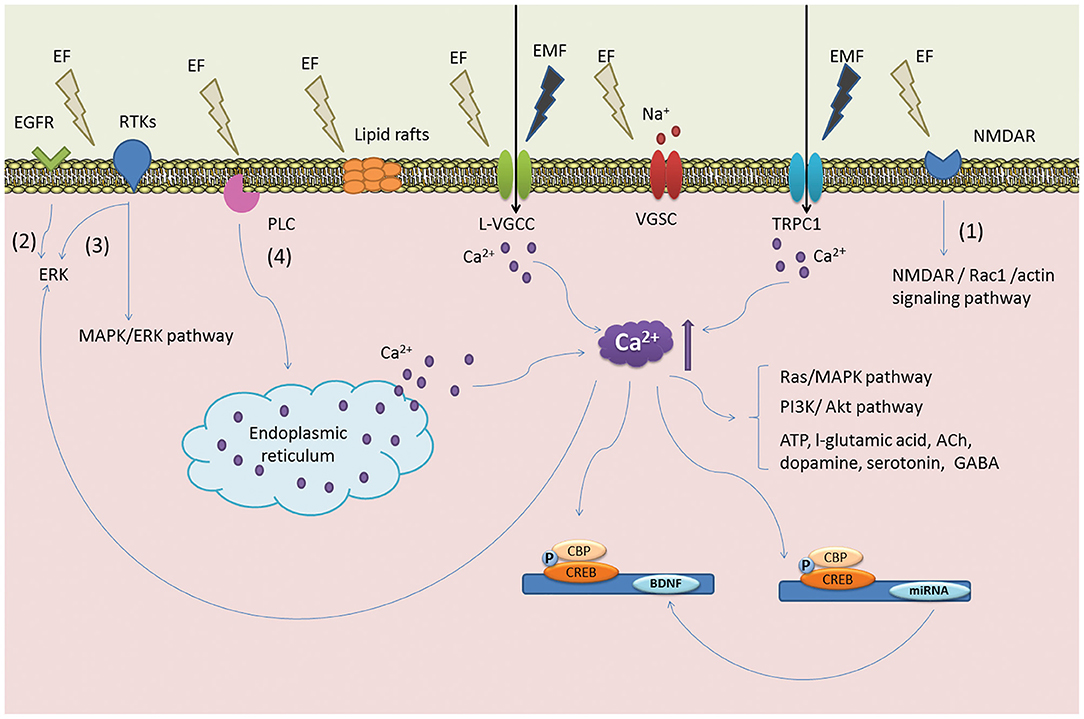
Figure 4. Postulated mechanism of ES on NSCs. The illustration denotes key signaling events that are thought to occur via ES. ES is believed to induce the reorganization of cytoskeletal filaments and lipid raft structures become polarized to initiate ERK signaling pathways and the upregulation of BDNF. These events are linked to the proliferation of NSCs and early neuronal differentiation. Reprinted from Zhu et al. (123) with permission from Elsevier.
The first challenge would be in the design of a strategy that is minimally invasive and clinically safe to provide ES. The delivery of localized ES in the injured spinal cord via implanted electrodes has been reported preclinically with promising findings for functional recovery outcomes that have led to the growing interest in ES for SCI (130, 131, 134–137). However, there are reports that implanted stiff materials can cause local scarring from the mechanical trauma and immune reactions in the CNS (138). Previously, Potter et al. (139) demonstrated a localized inflammatory response after microelectrode implantation in the CNS using immunohistochemical markers. Furthermore, the group demonstrated an accumulation of reactive oxygen species surrounding implanted microelectrodes which were thought to impact the viability of neural tissue adjacent to the implanted electrodes (138). The build-up of scar tissue at the neural tissue interface has been shown to reduce the efficiency of the recording and stimulating capacity of implanted electrodes, introducing a challenge that has led to further progression of neural interface research and growing interest in nanomaterial science (140). Alternatively, external stimulation is a minimally invasive method of delivering ES to transplanted cells albeit with low spatial resolution and some off-target effects such as tissue scarring around electrodes in in vivo models (58, 141). A systematic review of the efficacy of external or transcutaneous stimulation indicates the need for testing in large clinical trials so far (142). In summary, ES has been shown to improve cell regenerative responses (of both transplanted cells and cells at the injury site) but faces challenges in efficiently detecting and supplying charge to and from neural tissue with minimal signal attenuation, immune responses and undesirable chemical reactions.
Hybrid Electroactive Biomaterials: Combining Conductive Biomaterials With Stem Cell Therapy to Maximize Cell Transplantation Outcomes
To address the challenges outlined above, the functionalization of electroactive biomaterials presents as a promising strategy to deliver cells and modulate their behavior post-transplantation. The concept of an electroactive implantable cell transplant device has been well studied with promising reports (129, 143, 144). A vast library of electroactive biomaterials have been classified through rapidly developing fabrication technologies in regenerative medicine research, summarized in Table 2. Some well-studied electroactive biomaterials have been engineered from materials such as conductive polymers, carbon nanotubes (CNTs) and graphene for CNS repair, highlighted in this review (Table 3). Polymeric biomaterials have so far proven to provide mechanical support and potential to interact electrically with neurons in neural tissue engineering applications. Naturally occurring polymers, such as the ECM matrix protein collagen, can be fabricated with synthetic conductive polymers to form three-dimensional (3D) composite polymeric scaffolds with controlled chemical and physical properties (165). Several non-cytotoxic synthetic conductive polymers, including polypyrrole (PPY), Poly(ethylene glycol) diacrylate (PEGDA) and poly(lactic-co-glycolic acid) (PLGA), are currently being studied for the development of electroactive scaffolds for nerve injury applications (Table 3). An important aspect to inscribe is that electrical conductivity and electrical stimulation are defined as separate parameters. Coupled with ES, cells grown on electroactive scaffolds are consistently observed to possess significantly enhanced regenerative features which may improve cell therapy outcomes in SCI.
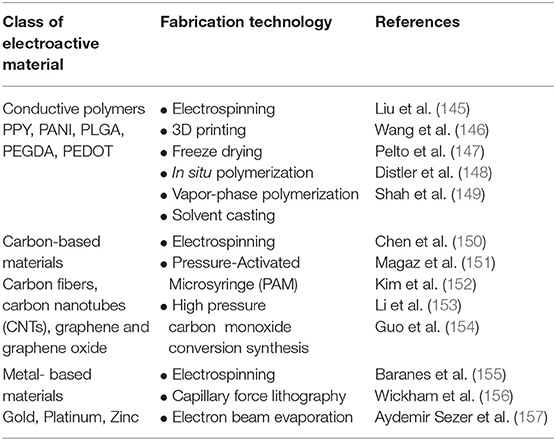
Table 2. A general classification of commonly studied electroactive biomaterials and fabrication methods applied in regenerative medicine applications.
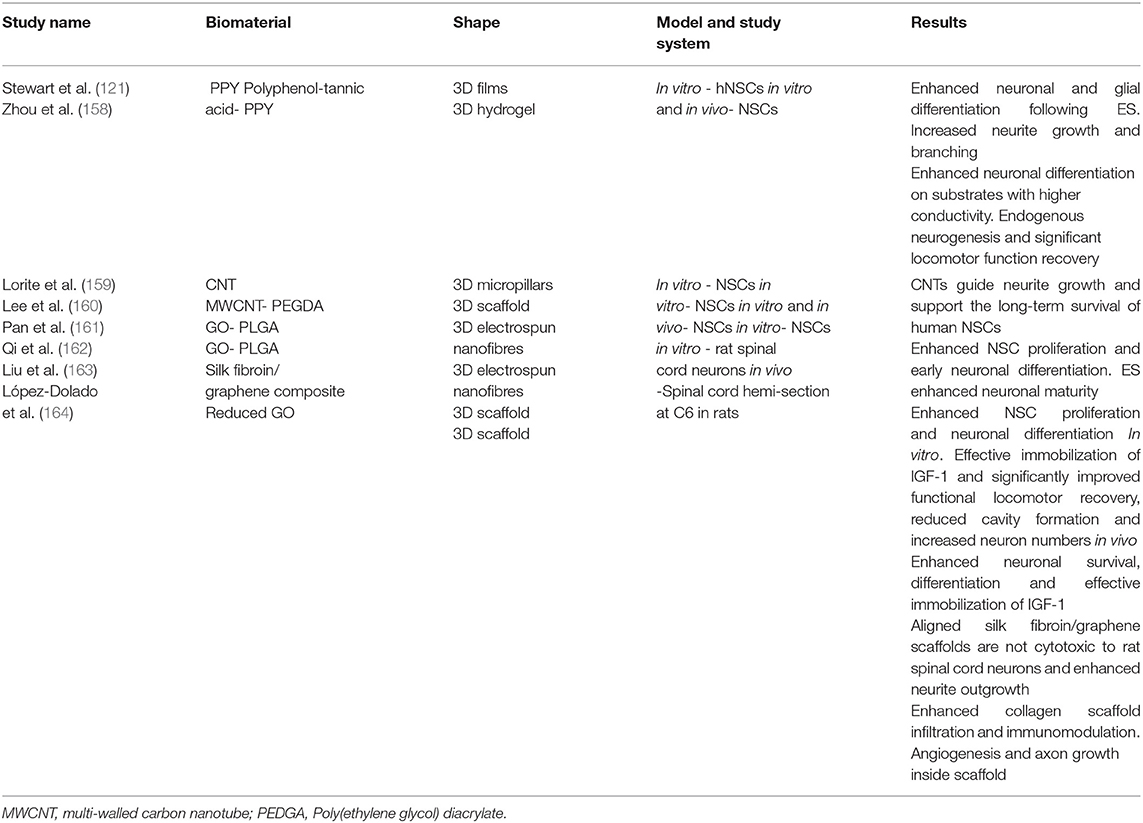
Table 3. A summary of some major findings in the investigation of electroactive scaffolds for neural tissue engineering.
Molecular Mechanisms for Improved Regenerative Responses of Cells on Electroactive Surfaces
It is well understood that neuronal cells are electroactive, and given the discovery of endogenous EFs in the developing CNS, the promotion of bioelectrical signal transmission would seem crucial for the functional restoration of damaged tissue after SCI. Electroactive scaffolds bear the advantage of enhancing the complex electrical transmission function of neuronal networks during cell-to-substrate and cell-to-cell interactions. Material scientists have made insights into how cells probe their surroundings within biomaterials by investigating conductivity as a biophysical cue, which seems to be a promising strategy to promote a well maintained homeostatic microenvironment that is tailored to enhance regeneration. In a bid to understand the mechanisms of electroactivity on nerve healing, electroactive materials are understood to regulate cellular responses to enhance regeneration. The cellular responses reported include cell adhesion, neuronal differentiation, migration and proliferation. Furthermore, electroactive materials have been shown to affect the microenvironment by modulating immune responses and oxidative stress, seemingly relevant for altering the inhibitory microenvironment of the injured CNS (Figure 5). Indeed, such anti-inflammatory responses and the regulation of oxidative stress have been reported across several studies (166–168). In consideration of the latter, the molecular mechanisms that have largely been proposed to affect nerve healing appear to do so primarily through enhanced survival, proliferation and neuronal differentiation, described in several reports in the literature.
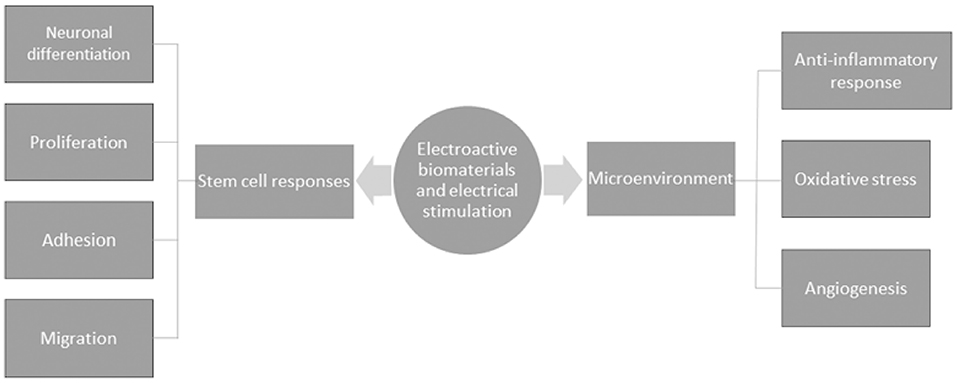
Figure 5. A schematic of the general mechanisms by which electroactive biomaterials and electrical stimulation enhance nerve healing.
Recently, Eftekhari et al. (169) demonstrated the capability of a novel conductive chitosan/polyaniline hydrogel in modulating neuronal differentiation through the upregulation of the MAP2 gene in rat adipose-derived stem cells at the material-tissue interface. Notably, conductivity and cell-imprinted topography were investigated simultaneously as biophysical cues to enhance the potential for neural regeneration. Many groups have corroborated the latter findings on investigating electroactive materials for NSC therapy. Stewart et al. (121) successfully induced human NSC differentiation on PPY with an electrode device. The group reported that ES of PPY induced human NSCs to predominantly β-III Tubulin (Tuj1) expressing neurons, with lower induction of glial fibrillary acidic protein (GFAP) expressing glial cells compared to controls. A morphological analysis revealed longer neurites and significant branches on stimulated cells compared to controls. Furthermore, the cultures were observed to possess clusters of neurons with longer neurites compared to unstimulated cultures. The study highlights the developments made toward directing the differentiation fates of NSCs to neuronal lineage via an implantable electroactive scaffold when considering a pioneer study by Schmidt et al. (170). The group reported the culture of PC-12 neuron-like cells on oxidized PPY films which were subjected to an electrical stimulus. The group reported a significant increase in neurite lengths compared with controls that were not subjected to electrical stimulation. Similarly, Moroder et al. (171) analyzed morphological changes in PC-12 cells and reported significant increases in the percentage of neurite bearing cells, neurites per cell and neurite lengths of PC-12 cells in the presence of ES compared with no ES on conductive polymer scaffolds. Notably, these studies employed cell lines, and recent preclinical developments appear to heavily report on cell lines for SCI repair applications (169, 172, 173). Whilst cell lines have their advantages in mitigating ethical implications arising from ESCs or fetal-derived NSCs, the move to physiologically relevant NSCs in CNS injury preclinical models would seem to be concomitant with clinical translation for SCI.
In addition to the impact on differentiation, ES appears to be cue for enhancing proliferation. Zhu et al. (174) reported a 35% increase in proliferation of NSCs cultured on electrospun carbon nanofibrous scaffolds, proposed to occur via proliferating cell nuclear antigen and extracellular signal-regulated kinases 1 and 2 mechanisms (175). Furthermore, the group concluded that ES increased the neuronal differentiation of hNSCs, with complex morphologies reported (Figure 6). More recently, Song et al. (176) demonstrated changes in protein expression including heparin binding EGF-like growth factor (hb-EGF), GDNF, BDNF and neurotrophin 3 (NTF3) and enolase 2 (ENO2) on human NPCs as a result of the change of dimensionality from 2D to 3D PPY scaffolds. The group linked these gene expression changes to enhanced cell survival. These studies have provided a basis to incorporate conductivity and ES into tissue engineering applications to enhance neural regeneration from the functional observations of improved cellular proliferation and neuronal differentiation.
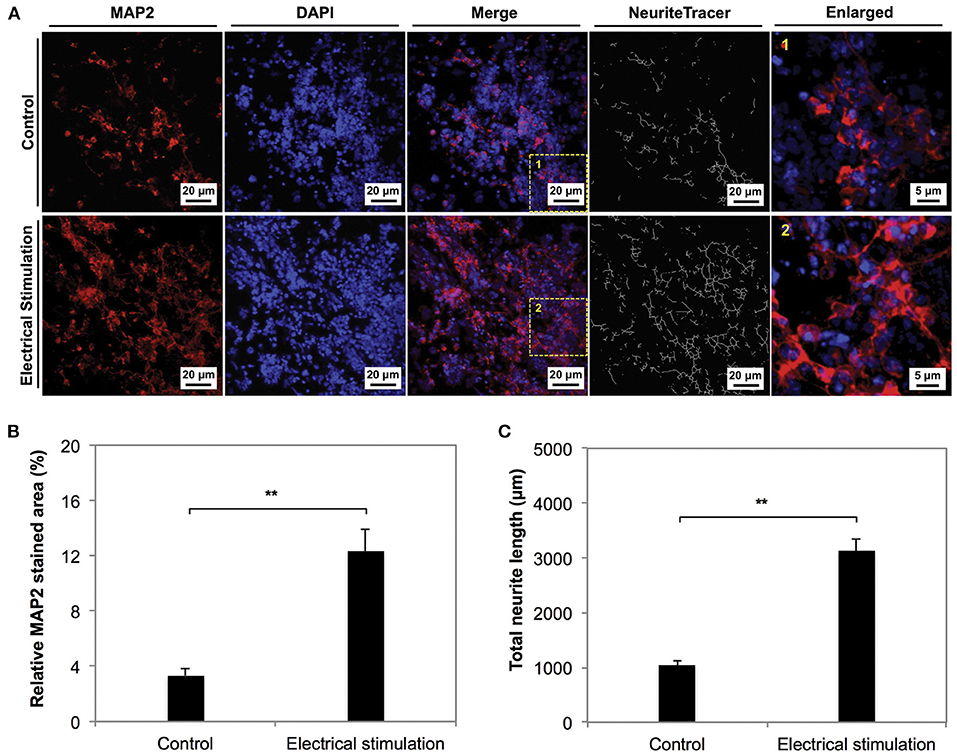
Figure 6. The phenotype of NSCs cultured on electrospun carbon nanofibers under electrical stimulation was compared to unstimulated controls for 7 days in culture. (A,B) The neuronal marker MAP2 was significantly expressed in the stimulated condition compared to the control. (C) Neurite length anaylsis indicated longer neurite outgrowth in stimulated conditions. Data was reported as mean ± standard deviation, n = 3, **P < 0.01. Reprinted from Zhu et al. (174) with permission from Elsevier.
CNTs, graphene and fullerenes, are nanomaterials with interesting optoelectronic and mechanical properties which have been incorporated in electroactive biomaterials. Reports in the literature indicate the potential of electroactive biomaterials may enhance survival, facilitate cell-cell adhesion, proliferation, differentiation and immune homeostasis, that are pertinent to neural regeneration (168, 177) (Table 3). The novelty of combining carbon-based electroactive material surface topography with a polymer and ES has been demonstrated with promising results for enhancing neural regeneration in vitro. Key findings by Shin et al. (178) indicate significant neuronal differentiation of human fetal neural stem cells (hfNSCs) and human induced pluripotent stem cell-derived neural progenitor cells (hiPSC-NPCs). Furthermore, improved electrophysiological functionality within catechol-functionalized hyaluronic acid (HA–CA) hydrogels containing CNTs and/or PPy was reported in this study. A recent in vitro study reported the feasibility of electrically stimulating NSCs on graphene oxide (GO)-incorporated poly(lactic-co-glycolic acid) (PLGA) electrospun nanofibers (179). The authors reported the enhanced proliferation, neuronal differentiation and neurite outgrowth of NSCs, similar to findings by Zhu et al. (174) thus indicating the potential of applying graphene oxide composites, albeit in a concentration-dependent manner due to reports of cytotoxicity, as electroactive scaffold implants to promote neurogenesis (180). The authors further demonstrated the feasibility of immobilizing insulin-like growth factor 1 (IGF-1) on GO with significant improvement in NSC survival outcomes, indicating further potential for incorporating nanoparticle drug delivery within these composite electroactive scaffolds (162). Combining electroactivity, ES and nanomaterial science to neural tissue engineering adds a level of complexity to scaffold biomaterial design for neural regeneration applications. The combined effects of these concepts show promise for an implantable electroactive scaffold as an effective combinatorial approach in SCI cell therapy research.
There are considerable challenges to overcome before clinical application of an electroactive cell implant can become a reality, such as control over mechanical properties to affect cell-cell interactions, responses and integration with the host tissue. In order to optimize their application in the CNS, the porosity and microarchitecture of hydrogels requires significant control. This has partly been achieved through traditional polymer processing techniques as hydrogel scaffolds of uniform porosity have successfully been developed, and even progressed to clinical trials for neural tissue repair as reported by Theodore et al. (181). This group demonstrated the safety and feasibility of the first human implantation of a porous bioresorbable polymer scaffold into the acutely contused spinal cord. Clinical efficacy has not yet been achieved, and despite promising results in preclinical models, hydrogel scaffolds require further development as the mechanical properties have proven difficult to control. Researchers like Prager et al. (182) have brought innovation to this area by reporting a protocol to determine a “target stiffness” for hydrogels, matched to the stiffness of the CNS, indicating development of the strategy for neural tissue repair. The application of electroactive hydrogel scaffolds and ES for SCI cell therapy, too, requires further development from the preclinical evidence gathered so far.
Future Directions
This review highlights the effects of electroactivity and ES on NSCs in 3D matrices, largely composed of in vitro preclinical reports. There is clear potential for these scaffolds to be used to modulate NSC behavior post-transplantation and enhance their regenerative capability. However, there are gaps in our current understanding. Much of the current data is focused on neurogenesis and neuronal maturation. Whilst important, the glial components of the CNS are also essential to its function and pathological response to injury. Therefore, systematic studies into the effect of electroactive scaffolds on glial cell production from NSCs are needed. Further, understanding the effect of electroactive materials and ES on microglia will be key to engineering the immune response to the implant, a major factor in successful implant integration and repair capacity.
The control of mechanical properties of electroactive scaffolds, such as stiffness, appears to be a challenge. The development of novel electroactive hydrogel scaffold composites incorporated with microfabrication techniques and nanomaterials may partly address this limitation (168). Whilst there is a growing body of in vitro evidence for the cytocompatibility of implants such as graphene-based composite scaffolds, standardized studies in animals with the objective of assessing their nanotoxicology seem necessary to determine the clinical translation of such developments. Some recent preclinical advances demonstrate the long term performance of electroactive scaffolds for neural tissue regeneration applications in vivo (183, 184). Thus the main hurdles to overcome appear to be in the domain of the biocompatibility and safety of electroactive scaffolds in vivo, which requires further assessment across different tissues. This would form an essential milestone for progression to clinical translation for a SCI cell therapy. Together with the latter analysis of the insights into electroactive scaffolds, the approvals process and regulatory requirements for medicines and medical devices must be considered to realistically endeavor the clinical translation of electroactive biomaterials for SCI cell therapy. The process is lengthy and often difficult, taking up to 15 years to market a new medicine/medical device. There are many points at which an implantable biomaterial/medical device may be rejected on the grounds of safety, effectiveness and cost over the course of preclinical studies, clinical trial phases, and nation-specific regulatory systems. Therefore, it is essential to carefully probe insights into the long term interaction of implantable electroactive scaffolds with neural tissue at the current preclinical stage.
Degradable electroactive hydrogelsalso have promise for eventual clinical translation. Xu et al. (185) in particular present this rationale in an in vivo study testing an injectable electroactive hydrogel composed of biodegradable germanium phosphide (GeP) nanosheets and hyaluronic acid (HA). New technologies could therefore mean translation of implantable electroactive biomaterials for NSC therapy is more realistic. Overall, the combination of electroactive implants and NSC transplantation is a complex therapeutic option. However, given the complexity of SCI injury and the social need for a new therapy we believe this strategy is worth investigating further.
Author Contributions
AM: methodology and writing—original draft preparation. CA: supervision. JH and CA: project administration and funding acquisition. All authors read, conceptualization, writing—review and editing, and agreed to the published version of the manuscript.
Funding
AM is supported by a Ph.D. studentship from the Faculty of Natural Sciences at Keele University. JH thank the UK Engineering and Physical Sciences Research Council (EPSRC) for financial support (via grants EP/R003823/1, EP/R511560/1, and EP/K03099X/1), the UK Biotechnology and Biological Sciences Research Council (BBSRC) for financial support (via grant BB/L0137971/1), and the UK Royal Society for financial support (via grant RG160449). CA thanks the UK EPSRC for financial support (via an ETERM Fellowship, grant EP/I017801/1) and the Royal Society for financial support (via grant RGS/R2/180328).
Conflict of Interest
The authors declare that the research was conducted in the absence of any commercial or financial relationships that could be construed as a potential conflict of interest.
Publisher's Note
All claims expressed in this article are solely those of the authors and do not necessarily represent those of their affiliated organizations, or those of the publisher, the editors and the reviewers. Any product that may be evaluated in this article, or claim that may be made by its manufacturer, is not guaranteed or endorsed by the publisher.
References
1. World Health Organisation. (2013). Spinal cord injury. Available online at: https://www.who.int/news-room/fact-sheets/detail/spinal-cord-injury (accessed July 8, 2021).
2. National Institute For Health And Care Excellence (2021). Spinal Injury: Assessment and initial management. london: national institute for health and care excellence. Available online at: https://www.nice.org.uk/guidance/ng41 (accessed July 8, 2021).
3. Chamberlain JD, Meier S, Mader L, Von Groote PM, Brinkhof MWG. Mortality and longevity after a spinal cord injury: systematic review and meta-analysis. Neuroepidemiology. (2015) 44:182–98. doi: 10.1159/000382079
4. Sweis R, Biller J. Systemic complications of spinal cord injury. Curr Neurol Neurosci Rep. (2017) 17:8. doi: 10.1007/s11910-017-0715-4
5. Anson CA, Shepherd C. Incidence of secondary complications in spinal cord injury. Int J Rehabil. (1996) 19:55–66. doi: 10.1097/00004356-199603000-00006
6. McDaid D, Park AL, Gall A, Purcell M, Bacon M. Understanding and modelling the economic impact of spinal cord injuries in the United Kingdom. Spinal Cord. (2019) 57:778–88. doi: 10.1038/s41393-019-0285-1
7. Fehlings MG, Tetreault LA, Aarabi B, Anderson P, Arnold PM, Brodke DS, et al. A clinical practice guideline for the management of patients with acute spinal cord injury: recommendations on the type and timing of rehabilitation. Global Spine J. (2017) 7:231s−8s. doi: 10.1177/2192568217701910
8. Walters BC, Hadley MN, Hurlbert RJ, Aarabi B, Dhall SS, Gelb DE, et al. Guidelines for the management of acute cervical spine and spinal cord injuries: 2013 update. Neurosurgery. (2013) 60:82–91. doi: 10.1227/01.neu.0000430319.32247.7f
9. Queensland Ambulance Service (2021). Clinical Quality And Patient Assessment Unit. Clinical Practice Guidelines: Trauma/Spinal Injury. Queensland, Australia. Available online at: https://www.ambulance.qld.gov.au/docs/clinical/cpg/cpg_spinal%20cord%20injury.pdf (accessed July 8, 2021).
10. Hadley MN, Walters BC, Aarabi B, Dhall SS, Gelb DE, Hurlbert RJ, et al. Clinical assessment following acute cervical spinal cord injury. Neurosurgery. (2013) 72:40–53. doi: 10.1227/NEU.0b013e318276edda
11. Bagnall AM, Jones L, Duffy S, Riemsma RP. Spinal fixation surgery for acute traumatic spinal cord injury. Cochrane Database System Rev. (2008) 1. doi: 10.1002/14651858.CD004725.pub2
12. Wilson JR, Tetreault LA, Kwon BK, Arnold PM, Mroz TE, Shaffrey C, et al. Timing of decompression in patients with acute spinal cord injury: a systematic review. Global Spine J. (2017) 7:95s−115s. doi: 10.1177/2192568217701716
13. Godzik J, Dalton J, Hemphill C, Walker C, Chapple K, Cook A, et al. Early surgical intervention among patients with acute central cord syndrome is not associated with higher mortality and morbidity. J Spine Surg. (2019) 5:466–74. doi: 10.21037/jss.2019.09.26
14. Katoh SEL, Masri WS. Neurological recovery after conservative treatment of cervical cord injuries. J Bone Joint Surg (Br). (1994) 76:225–8. doi: 10.1302/0301-620X.76B2.8113281
15. Katoh S, El Masri WS, Jaffray D, Mccall IW, Eisenstein SM, Pringle RG, et al. Neurologic outcome in conservatively treated patients with incomplete closed traumatic cervical spinal cord injuries. Spine. (1996) 21:2345–51. doi: 10.1097/00007632-199610150-00008
16. Masri WE, Kumar N. Active physiological conservative management in traumatic spinal cord injuries - an evidence-based approach. Trauma. (2017) 19:10–22. doi: 10.1177/1460408617698508
17. El Masri YWS. Traumatic spinal injury and spinal cord injury: point for active physiological conservative management as compared to surgical management. Spinal Cord Series Cases. (2018) 4:1–4. doi: 10.1038/s41394-018-0045-z
18. Abudou M, Chen X, Kong X, Wu T. Surgical versus non-surgical treatment for thoracolumbar burst fractures without neurological deficit. Cochrane Database Syst Rev. (2013) 6:CD005079. doi: 10.1002/14651858.CD005079.pub3
19. Mataliotakis GI, Tsirikos AI. Spinal cord trauma: pathophysiology, classification of spinal cord injury syndromes, treatment principles and controversies. Orthop Trauma. (2016) 30:440–9. doi: 10.1016/j.mporth.2016.07.006
20. Ahmed AI, Lucas JD. Spinal cord injury: pathophysiology and strategies for regeneration. Orthopaedics Trauma. (2020) 34:266–71. doi: 10.1016/j.mporth.2020.06.003
21. Albayar AA, Roche A, Swiatkowski P, Antar S, Ouda N, Emara E, et al. Biomarkers in spinal cord injury: prognostic insights and future potentials. Front Neurol. (2019) 10:27. doi: 10.3389/fneur.2019.00027
22. Anwar MA, Al Shehabi TS, Eid AH. Inflammogenesis of secondary spinal cord injury. Front Cell Neurosci. (2016) 10:98. doi: 10.3389/fncel.2016.00098
23. Alizadeh A, Dyck SM, Karimi-Abdolrezaee S. Traumatic spinal cord injury: an overview of pathophysiology, models and acute injury mechanisms. Front Neurol. (2019) 10:282. doi: 10.3389/fneur.2019.00282
24. Dyck SM, Karimi-Abdolrezaee S. Chondroitin sulfate proteoglycans: key modulators in the developing and pathologic central nervous system. Exp Neurol. (2015) 269:169–87. doi: 10.1016/j.expneurol.2015.04.006
25. Yiu G, He Z. Glial inhibition of cns axon regeneration. Nat Rev Neurosci. (2006) 7:617–27. doi: 10.1038/nrn1956
26. Bradbury EJ, Burnside ER. Moving beyond the glial scar for spinal cord repair. Nat Commun. (2019) 10:3879. doi: 10.1038/s41467-019-11707-7
27. Myers SA, Bankston AN, Burke DA, Ohri SS, Whittemore SR. Does the preclinical evidence for functional remyelination following myelinating cell engraftment into the injured spinal cord support progression to clinical trials? Experi Neurol. (2016) 283:560–72. doi: 10.1016/j.expneurol.2016.04.009
28. Liu J, Yang X, Jiang L, Wang C, Yang M. Neural plasticity after spinal cord injury. Neural Regener Res. (2012) 7:386–91. doi: 10.3969/j.issn.1673-5374.2012.05.010
29. Hill CE, Beattie MS, Bresnahan JC. Degeneration and sprouting of identified descending supraspinal axons after contusive spinal cord injury in the rat. Exp Neurol. (2001) 171:153–69. doi: 10.1006/exnr.2001.7734
30. Adams KL, Gallo V. The diversity and disparity of the glial scar. Nat Neurosci. (2018) 21:9–15. doi: 10.1038/s41593-017-0033-9
31. Tewarie RSN, Hurtado A, Bartels RH, Grotenhuis A, Oudega M. Stem cell-based therapies for spinal cord injury. J Spinal Cord Med. (2009) 32:105–14. doi: 10.1080/10790268.2009.11760761
32. Dalamagkas K, Tsintou M, Seifalian AM. Stem cells for spinal cord injuries bearing translational potential. Neural Regener Res. (2018) 13:35–42. doi: 10.4103/1673-5374.224360
33. Cízková D, Rosocha J, Vanický I, Jergová S, Cízek M. Transplants of human mesenchymal stem cells improve functional recovery after spinal cord injury in the rat. Cell Mol Neurobiol. (2006) 26:1167–80. doi: 10.1007/s10571-006-9093-1
34. Bottai D, Cigognini D, Madaschi L, Adami R, Nicora E, Menarini M, et al. Embryonic stem cells promote motor recovery and affect inflammatory cell infiltration in spinal cord injured mice. Exp Neurol. (2010) 223:452–63. doi: 10.1016/j.expneurol.2010.01.010
35. Stenudd M, Sabelström H, Frisén J. Role of endogenous neural stem cells in spinal cord injury and repair. JAMA Neurol. (2015) 72:235–7. doi: 10.1001/jamaneurol.2014.2927
36. Johe KK, Hazel TG, Muller T, Dugich-Djordjevic MM, McKay RD. Single factors direct the differentiation of stem cells from the fetal and adult central nervous system. Genes Dev. (1996) 10:3129–40. doi: 10.1101/gad.10.24.3129
37. Rajan P, McKay RD. Multiple routes to astrocytic differentiation in the CNS. J Neurosci. (1998) 18:3620–9. doi: 10.1523/JNEUROSCI.18-10-03620.1998
38. Hughes S, Lillien L, Raff M, Rohrer H, Sendtner M. Ciliary neurotrophic factor induces type-2 astrocyte differentiation in culture. Nature. (1988) 335:70–3. doi: 10.1038/335070a0
39. Schubert D, Herrera F, Cumming R, Read J, Low W, Maher P, et al. Neural cells secrete a unique repertoire of proteins. J Neurochem. (2009) 109:427–35. doi: 10.1111/j.1471-4159.2009.05968.x
40. Song XY Li F, Zhang FH, Zhong JH, Zhou XF. Peripherally-derived bdnf promotes regeneration of ascending sensory neurons after spinal cord injury. PLoS One. (2008) 3:1707. doi: 10.1371/journal.pone.0001707
41. Gilmour AD, Reshamwala R, Wright AA, Ekberg JA, St John JA. Optimizing olfactory ensheathing cell transplantation for spinal cord injury repair. J Neurotrauma. (2020) 37:817–29. doi: 10.1089/neu.2019.6939
42. Nakhjavan-Shahraki B, Yousefifard M, Rahimi-Movaghar V, Baikpour M, Nasirinezhad F, Safari S, et al. Transplantation of olfactory ensheathing cells on functional recovery and neuropathic pain after spinal cord injury; systematic review and meta-analysis. Sci Rep. (2018) 8:325. doi: 10.1038/s41598-017-18754-4
43. García-Alías G, López-Vales R, Forés J, Navarro X, Verdú E. Acute transplantation of olfactory ensheathing cells or schwann cells promotes recovery after spinal cord injury in the rat. J Neurosci Res. (2004) 75:632–41. doi: 10.1002/jnr.20029
44. Gorrie CA, Hayward I, Cameron N, Kailainathan G, Nandapalan N, Sutharsan R, et al. Effects of human oec-derived cell transplants in rodent spinal cord contusion injury. Brain Res. (2010) 1337:8–20. doi: 10.1016/j.brainres.2010.04.019
45. Lang BC, Zhang Z, Lv LY, Liu J, Wang TY, Yang LH, et al. Oecs transplantation results in neuropathic pain associated with bdnf regulating erk activity in rats following cord hemisection. BMC Neurosci. (2013) 2:80. doi: 10.1186/1471-2202-14-80
46. Deumens R, Van Gorp SF, Bozkurt A, Beckmann C, Führmann T, Montzka K, et al. Motor outcome and allodynia are largely unaffected by novel olfactory ensheathing cell grafts to repair low-thoracic lesion gaps in the adult rat spinal cord. Behav Brain Res. (2013) 15:185–9. doi: 10.1016/j.bbr.2012.09.036
47. Wang C, Sun C, Hu Z, Huo X, Yang Y, Liu X, et al. Improved neural regeneration with olfactory ensheathing cell inoculated plga scaffolds in spinal cord injury adult rats. Neurosignals. (2017) 25:1–14. doi: 10.1159/000471828
48. Huang H, Xi H, Chen L, Zhang F, Liu Y. Long-term outcome of olfactory ensheathing cell therapy for patients with complete chronic spinal cord injury. Cell Transplant. (2012) 21:23. doi: 10.3727/096368912X633734
49. Mackay-Sim A, Féron F, Cochrane J, Bassingthwaighte L, Bayliss C, Davies W, et al. Autologous olfactory ensheathing cell transplantation in human paraplegia: a 3-year clinical trial. Brain: J Neurol. (2008) 131:2376–86. doi: 10.1093/brain/awn173
50. Zheng Z, Liu G, Chen Y, Wei S. Olfactory ensheathing cell transplantation improves sympathetic skin responses in chronic spinal cord injury. Neural Regen Res. (2013) 8:2849–55.
51. Thompson M, Mei SHJ, Wolfe D, Champagne J, Fergusson D, Stewart DJ, et al. Cell therapy with intravascular administration of mesenchymal stromal cells continues to appear safe: an updated systematic review and meta-analysis. Clin Med. (2020) 19:100249. doi: 10.1016/j.eclinm.2019.100249
52. Akiyama Y, Radtke C, Kocsis JD. Remyelination of the rat spinal cord by transplantation of identified bone marrow stromal cells. J Neurosci. (2002) 22:6623–30. doi: 10.1523/JNEUROSCI.22-15-06623.2002
53. Abrams MB, Dominguez C, Pernold K, Reger R, Wiesenfeld-Hallin Z, Olson L, et al. Multipotent mesenchymal stromal cells attenuate chronic inflammation and injury-induced sensitivity to mechanical stimuli in experimental spinal cord injury. Restor Neurol Neurosci. (2009) 27:307–21. doi: 10.3233/RNN-2009-0480
54. Assinck P, Duncan GJ, Hilton J, Plemel JR, Tetzlaff W. Cell transplantation therapy for spinal cord injury. Nat Neurosci. (2017) 20:637–64. doi: 10.1038/nn.4541
55. Liau LL, Al-Masawa ME, Koh B, Looi QH, Foo JB, Lee SH, et al. The potential of mesenchymal stromal cell as therapy in neonatal diseases. Front Pediatr. (2020) 8:591693. doi: 10.3389/fped.2020.591693
56. Ruzicka J, Machova-Urdzikova L, Gillick J, Amemori T, Romanyuk N, Karova K, et al. A comparative study of three different types of stem cells for treatment of rat spinal cord injury. Cell Transplant. (2017) 26:585–603. doi: 10.3727/096368916X693671
57. Oh SK, Choi KH, Yoo JY, Kim DY, Kim SJ, Jeon SR. A phase III clinical trial showing limited efficacy of autologous mesenchymal stem cell therapy for spinal cord injury. Neurosurgery. (2015) 78:436–47. doi: 10.1227/NEU.0000000000001056
58. Chen C, Bai X, Ding Y, Lee I. Electrical stimulation as a novel tool for regulating cell behavior in tissue engineering. Biomatar Res. (2019) 23:25. doi: 10.1186/s40824-019-0176-8
59. Deng WS, Ma K, Liang B, Liu XY, Xu HY, Zhang J, et al. Collagen scaffold combined with human umbilical cord-mesenchymal stem cells transplantation for acute complete spinal cord injury. Neural Regen Res. (2020) 15:1686–700. doi: 10.4103/1673-5374.276340
60. Yang Y, Pang M, Chen YY, Zhang LM, Liu H, Tan J, et al. Human umbilical cord mesenchymal stem cells to treat spinal cord injury in the early chronic phase: study protocol for a prospective, multicenter, randomized, placebo-controlled, single-blinded clinical trial. Neural Regen Res. (2020) 15:1532–8. doi: 10.4103/1673-5374.274347
61. Albu S, Kumru H, Coll R, Vives J, Vallés M, Benito-Penalva J, et al. Clinical effects of intrathecal administration of expanded wharton jelly mesenchymal stromal cells in patients with chronic complete spinal cord injury: a randomized controlled study. Cytotherapy. (2021) 23:146–56. doi: 10.1016/j.jcyt.2020.08.008
62. Amemori T, Jendelová P, RuŽičková K, Arboleda D, Syková E. Co-transplantation of olfactory ensheathing glia and mesenchymal stromal cells does not have synergistic effects after spinal cord injury in the rat. Cytotherapy. (2010) 12:212–25. doi: 10.3109/14653240903440103
63. Niapour A, Karamali F, Nemati S, Taghipour Z, Mardani M, Nasr-Esfahani MH, et al. Cotransplantation of human embryonic stem cell-derived neural progenitors and schwann cells in a rat spinal cord contusion injury model elicits a distinct neurogenesis and functional recovery. Cell Transplant. (2012) 21:827–43. doi: 10.3727/096368911X593163
64. Salehi M, Pasbakhsh P, Soleimani M, Abbasi M, Hasanzadeh G, Modaresi MH, et al. Repair of spinal cord injury by co-transplantation of embryonic stem cell-derived motor neuron and olfactory ensheathing cell. Iran Biomed J. (2009) 13:125–135. Available online at: http://ibj.pasteur.ac.ir/article-1-71-en.html
65. Namestnikova DD, Cherkashova EA, Sukhinich KK, Gubskiy IL, Leonov GE, Gubsky LV, et al. Combined cell therapy in the treatment of neurological disorders. Biomedicines. (2020) 8:613. doi: 10.3390/biomedicines8120613
66. Takeuchi H, Natsume A, Wakabayashi T, Aoshima C, Shimato S, Ito M, et al. Intravenously transplanted human neural stem cells migrate to the injured spinal cord in adult mice in an SDF-1- and HGF-dependent manner. Neurosci Lett. (2007) 426:69–74. doi: 10.1016/j.neulet.2007.08.048
67. Cummings BJ, Uchida N, Tamaki SJ, Salazar DL, Hooshmand M, Summers R, et al. Human Neural Stem Cells Differentiate And Promote Locomotor Recovery In Spinal Cord-Injured Mice. Proc Natl Acad Sci U S A. (2005) 102:14069–74. doi: 10.1073/pnas.0507063102
68. Parr AM, Kulbatski I, Zahir T, Wang X, Yue C, Keating A, et al. Transplanted Adult Spinal Cord-Derived Neural Stem/Progenitor Cells Promote Early Functional Recovery After Rat Spinal Cord Injury. Neuroscience. (2008) 155:760–70. doi: 10.1016/j.neuroscience.2008.05.042
69. Uchida N, Chen K, Dohse M, Hansen KD, Dean J, Buser JR, et al. Human neural stem cells induce functional myelination in mice with severe dysmyelination. Sci Transl Med. (2012) 4:155ra136. doi: 10.1126/scitranslmed.3004371
70. Mothe AJ, Tator CH. Transplanted neural stem/progenitor cells generate myelinating oligodendrocytes and schwann cells in spinal cord demyelination and dysmyelination. Exp Neurol. (2008) 213:176–90. doi: 10.1016/j.expneurol.2008.05.024
71. Marsh SE, Blurton-Jones M. Neural stem cell therapy for neurodegenerative disorders: the role of neurotrophic support. Neurochem Int. (2017) 106:94–100. doi: 10.1016/j.neuint.2017.02.006
72. Lukovic D, Stojkovic M, Moreno-Manzano V, Jendelova P, Sykova E, Bhattacharya SS, et al. Concise review: reactive astrocytes and stem cells in spinal cord injury: good guys or bad guys? Stem Cells. (2015) 33:1036–41. doi: 10.1002/stem.1959
73. Kimelberg HK, Nedergaard M. Functions of astrocytes and their potential as therapeutic targets. Neurotherapeutics. (2010) 7:338–53. doi: 10.1016/j.nurt.2010.07.006
74. Tang Y, Yu P, Cheng L. Current progress in the derivation and therapeutic application of neural stem cells. Cell Death Dis. (2017) 8:E3108. doi: 10.1038/cddis.2017.504
75. Graf T, Enver T. Forcing cells to change lineages. Nature. (2009) 462:587–94. doi: 10.1038/nature08533
76. Their M, Wörsdörfer P, Lakes YB, Gorris R, Herms S, Opitz T, et al. Direct conversion of fibroblasts into stably expandable neural stem cells. Cell Stem Cell. (2012) 10:473–9. doi: 10.1016/j.stem.2012.03.003
77. Zhu S, Ambasudhan R, Sun W, Kim HJ, Talantova M, Wang X, et al. Small molecules enable oct4-mediated direct reprogramming into expandable human neural stem cells. Cell Res. (2014) 24:126–9. doi: 10.1038/cr.2013.156
78. Ge W, Ren C, Duan X, Geng D, Zhang C, Liu X. Differentiation of mesenchymal stem cells into neural stem cells using cerebrospinal fluid. Cell Biochem Biophys. (2015) 71:449–55. doi: 10.1007/s12013-014-0222-z
79. Feng N, Han Q, Li J, Wang S, Li H, Yao X, et al. Generation of highly purified neural stem cells from human adipose-derived mesenchymal stem cells by sox1 activation. Stem Cells Dev. (2014) 23:515–29. doi: 10.1089/scd.2013.0263
80. Zholudeva LV, Jin Y, Qiang L, Lane MA, Fischer I. Preparation of neural stem cells and progenitors: neuronal production and grafting applications. Methods Mol Biol. (2021) 2311:73–108. doi: 10.1007/978-1-0716-1437-2_7
81. Gutierrez-Aranda I, Ramos-Mejia V, Bueno C, Munoz-Lopez M, Real PJ, Mácia A, et al. Human induced pluripotent stem cells develop teratoma more efficiently and faster than human embryonic stem cells regardless the site of injection. Stem Cells. (2010) 28:1568–70. doi: 10.1002/stem.471
82. Yasuda S, Kusakawa S, Kuroda T, Miura T, Tano K, Takada N, et al. Tumorigenicity-associated characteristics of human ips cell lines. PLoS One. (2018) 13:E0205022. doi: 10.1371/journal.pone.0205022
83. Okano H, Nakamura M, Yoshida K, Okada Y, Tsuji O, Nori S, et al. Steps toward safe cell therapy using induced pluripotent stem cells. Circ Res. (2013) 112:523–33. doi: 10.1161/CIRCRESAHA.111.256149
84. Chen F, Cai B, Gao Y, Yuan X, Cheng F, Wang T, et al. Suicide gene-mediated ablation of tumor-initiating mouse pluripotent stem cells. Biomaterials. (2013) 34:1701–11. doi: 10.1016/j.biomaterials.2012.11.018
85. Chang EA, Jin SW, Nam MH, Kim SD. Human induced pluripotent stem cells: clinical significance and applications in neurologic diseases. J Korean Neurosurg Soc. (2019) 62:493–501. doi: 10.3340/jkns.2018.0222
86. Khazaei M, Ahuja CS, Nakashima H, Nagoshi N, Li L, Wang J., et al. Gdnf rescues the fate of neural progenitor grafts by attenuating notch signals in the injured spinal cord in rodents. Sci Transl Med. (2020) 12:3538. doi: 10.1126/scitranslmed.aau3538
87. Shin JC, Kim KN, Yoo J, Kim IS, Yun S, Lee H, et al. Clinical trial of human fetal brain-derived neural stem/progenitor cell transplantation in patients with traumatic cervical spinal cord injury. Neural Plast. (2015) 2015:630932. doi: 10.1155/2015/630932
88. Ghobrial GM, Anderson KD, Dididze M, Martinez-Barrizonte J, Sunn GH, Gant KL, et al. Human Neural Stem Cell Transplantation In Chronic Cervical Spinal Cord Injury: Functional Outcomes At 12 Months In A Phase Ii Clinical Trial. Neurosurgery. (2017) 64:87–91. doi: 10.1093/neuros/nyx242
89. Curtis E, Martin JR, Gabel B, Sidhu N, Rzesiewicz TK, Mandeville R, et al. A first-in-human, phase i study of neural stem cell transplantation for chronic spinal cord injury. Cell Stem Cell. (2018) 22:941–950.E6. doi: 10.1016/j.stem.2018.05.014
90. Levi AD, Anderson KD, Okonkwo DO, Park P, Bryce TN, Kurpad SN, et al. Clinical outcomes from a multi-center study of human neural stem cell transplantation in chronic cervical spinal cord injury. J Neurotrauma. (2019) 36:891–902. doi: 10.1089/neu.2018.5843
91. Tiwari S, Khan S, Kumar SV, Rajak R, Sultana A, Pasha SA, et al. Efficacy and safety of neural stem cell therapy for spinal cord injury: a systematic literature review. Therapie. (2021) 76:201–10. doi: 10.1016/j.therap.2020.06.011
92. Kumamaru H, Lu P, Rosenzweig ES, Kadoya K, Tuszynski MH. Regenerating corticospinal axons innervate phenotypically appropriate neurons within neural stem cell grafts. Cell Rep. (2019) 26:2329–39. doi: 10.1016/j.celrep.2019.01.099
93. Rosenzweig ES, Brock JH, Lu P, Kumamaru H, Salegio EA, Kadoya K, et al. Restorative effects of human neural stem cell grafts on the primate spinal cord. Nat Med. (2018) 24:484–90. doi: 10.1038/nm.4502
94. Lu P, Wang Y, Graham L, Mchale K, Gao M, Wu D, et al. Long-distance growth and connectivity of neural stem cells after severe spinal cord injury. Cell. (2012) 150:1264–73. doi: 10.1016/j.cell.2012.08.020
95. Ceto S, Sekiguchi KJ, Takashima Y, Nimmerjahn A, Tuszynski MH. Neural stem cell grafts form extensive synaptic networks that integrate with host circuits after spinal cord injury. Cell Stem Cell. (2020) 27:430–40. doi: 10.1016/j.stem.2020.07.007
96. Centeno EGZ, Cimarosti H, Bithell A. 2d Versus 3d human induced pluripotent stem cell-derived cultures for neurodegenerative disease modelling. Mol Neurodegener. (2018) 13:27. doi: 10.1186/s13024-018-0258-4
97. Du BL, Zeng X, Ma YH, Lai BQ, Wang JM, Ling EA, et al. Graft of the gelatin sponge scaffold containing genetically-modified neural stem cells promotes cell differentiation, axon regeneration, and functional recovery in rat with spinal cord transection. J Biomed Mater Res A. (2015) 103:1533–45. doi: 10.1002/jbm.a.35290
98. Muller AJ, Scherle PA. Targeting the mechanisms of tumoral immune tolerance with small-molecule inhibitors. Nat Rev Cancer. (2006) 6:613–25. doi: 10.1038/nrc1929
99. Webber DJ, Bradbury EJ, Mcmahon SB, Minger SL. Transplanted neural progenitor cells survive and differentiate but achieve limited functional recovery in the lesioned adult rat spinal cord. Regen Med. (2007) 2:929–45. doi: 10.2217/17460751.2.6.929
100. Meletis K, Barnabé-Heider F, Carlén M, Evergren E, Tomilin N, et al. Spinal cord injury reveals multilineage differentiation of ependymal cells. PLoS Biol. (2008) 6:E182. doi: 10.1371/journal.pbio.0060182
101. Piltti K. M., Salazar D. L., Uchida N., Cummings B. J., erson A. J. (2013). Safety of epicenter versus intact parenchyma as a transplantation site for human neural stem cells for spinal cord injury therapy. Stem Cells Translational Medicine, 2(3):204-216. doi: 10.5966/sctm.2012-0110
102. Hofstetter CP, Holmström NA, Lilja JA, Schweinhardt P, Hao J, Spenger C, et al. Allodynia limits the usefulness of intraspinal neural stem cell grafts; directed differentiation improves outcome. Nat Neurosci. (2005) 8:346–53. doi: 10.1038/nn1405
103. Macias MY, Syring MB, Pizzi MA, Crowe MJ, Alexanian AR, Kurpad SN. Pain with no gain: allodynia following neural stem cell transplantation in spinal cord injury. Exp Neurol. (2006) 201:335–48. doi: 10.1016/j.expneurol.2006.04.035
104. Takahashi Y, Tsuji O, Kumagai G, Hara CM, Okano HJ, Miyawaki A, et al. Comparative study of methods for administering neural stem/progenitor cells to treat spinal cord injury in mice. Cell Transplant. (2011) 20:727–39. doi: 10.3727/096368910X536554
105. Wang Y, Wei YT, Zu ZH. Combination of hyaluronic acid hydrogel scaffold and plga microspheres for supporting survival of neural stem cells. Pharm Res. (2011) 28:1406. doi: 10.1007/s11095-011-0452-3
106. Gao M, Lu P, Bednark B, Lynam D, Conner JM, Sakamoto J, et al. Templated agarose scaffolds for the support of motor axon regeneration into sites of complete spinal cord transection. Biomaterials. (2013) 34:1529–36. doi: 10.1016/j.biomaterials.2012.10.070
107. Robinson J, Lu P. Optimization of trophic support for neural stem cell grafts in sites of spinal cord injury. Exp Neurol. (2017) 291:87–97. doi: 10.1016/j.expneurol.2017.02.007
108. Leipzig ND, Shoichet MS. The effect of substrate stiffness on adult neural stem cell behavior. Biomaterials. (2009) 30:6867–78. doi: 10.1016/j.biomaterials.2009.09.002
109. Khaing ZZ, Milman BD, Vanscoy JE, Seidlits SK, Grill RJ, Schmidt CE. High molecular weight hyaluronic acid limits astrocyte activation and scar formation after spinal cord injury. J Neural Eng. (2011) 8:046033. doi: 10.1088/1741-2560/8/4/046033
110. Aurand ER, Lampe KJ, Bjugstad KB. Defining and designing polymers and hydrogels for neural tissue engineering. Neurosci Res. (2012) 72:199–213. doi: 10.1016/j.neures.2011.12.005
111. Moshayedi P, Ng G, Kwok JC, Yeo GS, Bryant CE, Fawcett JW, et al. The relationship between glial cell mechanosensitivity and foreign body reactions in the central nervous system. Biomaterials. (2014) 35:3919–25. doi: 10.1016/j.biomaterials.2014.01.038
112. Liu S, Schackel T, Weidner N, Puttagunta R. Biomaterial-supported cell transplantation treatments for spinal cord injury: challenges and perspectives. Front Cell Neurosci. (2018) 11:430. doi: 10.3389/fncel.2017.00430
113. Higuchi A, Suresh Kumar S, Benelli G, Ling Q, Li H, Alarfaj AA, et al. Biomaterials used in stem cell therapy for spinal cord injury. Prog Mater Sci. (2019) 103:374–424. doi: 10.1016/j.pmatsci.2019.02.002
114. Huang L, Fu C, Xiong F, He C, Wei Q. Stem cell therapy for spinal cord injury. Cell Transplant. (2021) 30:963689721989266. doi: 10.1177/0963689721989266
115. Hatami M, Mehrjardi NZ, Kiani S, Hemmesi K, Azizi H, Shahverdi A, et al. Human embryonic stem cell-derived neural precursor transplants in collagen scaffolds promote recovery in injured rat spinal cord. Null. (2009) 11:618–30. doi: 10.1080/14653240903005802
116. Kourgiantaki A, Tzeranis DS, Karali K, Georgelou K, Bampoula E, Psilodimitrakopoulos S, et al. Neural stem cell delivery via porous collagen scaffolds promotes neuronal differentiation and locomotion recovery in spinal cord injury. Npj Regenerative Medicine. (2020) 5:12. doi: 10.1038/s41536-020-0097-0
117. Cooke MJ, Vulic K, Shoichet MS. Design of biomaterials to enhance stem cell survival when transplanted into the damaged central nervous system. Soft Matter. (2010) 6:4988–98. doi: 10.1039/c0sm00448k
118. Xiao Z, Tang F, Tang J, Yang H, Zhao Y, Chen B, et al. One-year clinical study of neuroregen scaffold implantation following scar resection in complete chronic spinal cord injury patients. Sci China Life Sci. (2016) 59:647–55. doi: 10.1007/s11427-016-5080-z
119. Schaub NJ, Johnson CD, Cooper B, Gilbert RJ. Electrospun fibers for spinal cord injury research and regeneration. J Neurotrauma. (2016) 33:1405–15. doi: 10.1089/neu.2015.4165
120. Saberi A, Jabbari F, Zarrintaj P, Saeb MR, Mozafari M. Electrically conductive materials: opportunities and challenges in tissue engineering. Biomolecules. (2019) 9:448. doi: 10.3390/biom9090448
121. Stewart E, Kobayashi NR, Higgins MJ, Quigley AF, Jamali S, Moulton SE, et al. Electrical stimulation using conductive polymer polypyrrole promotes differentiation of human neural stem cells: a biocompatible platform for translational neural tissue engineering. Tissue Eng Part C, Methods. (2015) 21:385–393. doi: 10.1089/ten.tec.2014.0338
122. Calaresu I, Hernandez J, Rauti R, Rodilla BL, Arché-Núñez A, Perez L, et al. Polystyrene nanopillars with inbuilt carbon nanotubes enable synaptic modulation and stimulation in interfaced neuronal networks. Adv Mater Interfaces. (2021) 8:2002121. doi: 10.1002/admi.202002121
123. Zhu R, Sun Z, Li C, Ramakrishna S, Chiu K, He L. Electrical stimulation affects neural stem cell fate and function in vitro. Exp Neurol. (2019) 319:112963. doi: 10.1016/j.expneurol.2019.112963
124. He L, Sun Z, Li J, Zhu R, Niu B, Tam KL, et al. Electrical stimulation at nanoscale topography boosts neural stem cell neurogenesis through the enhancement of autophagy signaling. Biomaterials. (2021) 268:120585. doi: 10.1016/j.biomaterials.2020.120585
125. Cajavilca C, Varon J, Sternbach G. Luigi galvani and the foundations of electrophysiology. Resuscitation. (2009) 80:159–62. doi: 10.1016/j.resuscitation.2008.09.020
126. Mccaig CD, Zhao M. Physiological electrical fields modify cell behaviour. Bioessays. (1997) 19:819–826. doi: 10.1002/bies.950190912
127. Jenkins LS, Duerstock BS, Borgens RB. Reduction of the current of injury leaving the amputation inhibits limb regeneration in the red spotted newt. Dev Biol. (1996) 178:251–62. doi: 10.1006/dbio.1996.0216
128. Alexander J. K., Fuss B., Colello R. J. (2006). Electric field-induced astrocyte alignment directs neurite outgrowth. Neuron Glia Biology. 2(2):93-103 doi: 10.1017/S1740925X0600010X
129. Balint R, Cassidy NJ, Cartmell SH. Electrical stimulation: a novel tool for tissue engineering. Tissue Eng B: Rev. (2013) 19:48–57. doi: 10.1089/ten.teb.2012.0183
130. Geremia NM, Gordon T, Brushart TM, Al-Majed AA, Verge VMK. Electrical stimulation promotes sensory neuron regeneration and growth-associated gene expression. Exp Neurol. (2007) 205:347–59. doi: 10.1016/j.expneurol.2007.01.040
131. Wenjin W, Wenchao L, Hao Z, Feng L, Yan W, Wodong S, et al. Electrical stimulation promotes bdnf expression in spinal cord neurons through ca2+- and erk-dependent signaling pathways. Cell Mol Neurobiol. (2011) 31:459–67. doi: 10.1007/s10571-010-9639-0
132. Al-Majed AA, Brushart TM, Gordon T. Electrical stimulation accelerates and increases expression of bdnf and trkb mrna in regenerating rat femoral motor neurons. Eur J Neurosci. (2000) 12:4381–90. doi: 10.1046/j.1460-9568.2000.01341.x
133. Vivó M, Puigdemasa A, Casals L, Asensio E, Udina E, Navarro X. Immediate electrical stimulation enhances regeneration and reinnervation and modulates spinal plastic changes after sciatic nerve injury and repair. Exp Neurol. (2008) 211:180–93. doi: 10.1016/j.expneurol.2008.01.020
134. Wenger N, Moraud EM, Raspopovic S, Bonizzato M, DiGiovanna J, Musienko P, et al. Closed-loop neuromodulation of spinal sensorimotor circuits controls refined locomotion after complete spinal cord injury. Sci Transl Med. (2014) 6:255ra133. doi: 10.1126/scitranslmed.3008325
135. Capogrosso M, Milekovic T, Borton D, Wagner F, Moraud EM, Mignardot JB, et al. A brain-spine interface alleviating gait deficits after spinal cord injury in primates. Nature. (2016) 539:284–8. doi: 10.1038/nature20118
136. Wang S, Zhang LC, Fu HT, Deng JH, Xu GX Li T, et al. Epidural electrical stimulation effectively restores locomotion function in rats with complete spinal cord injury. Neural Regen Res. (2021) 16:573–9. doi: 10.4103/1673-5374.290905
137. Koh GP, Fouad C, Lanzinger W, Willits RK. Effect of intraoperative electrical stimulation on recovery after rat sciatic nerve isograft repair. Neurotrauma Reports. (2020) 1:181–91. doi: 10.1089/neur.2020.0049
138. Potter KA, Buck AC, Self WK, Callanan ME, Sunil S, Capadona JR. The effect of resveratrol on neurodegeneration and blood brain barrier stability surrounding intracortical microelectrodes. Biomaterials. (2013) 34:7001–15. doi: 10.1016/j.biomaterials.2013.05.035
139. Potter KA, Simon JS, Velagapudi B, Capadona JR. Reduction of autofluorescence at the microelectrode–cortical tissue interface improves antibody detection. J Neurosci Methods. (2012) 203:96–105. doi: 10.1016/j.jneumeth.2011.09.024
140. Rivnay J, Wang H, Fenno L, Deisseroth K, Malliaras GG. Next-generation probes, particles, and proteins for neural interfacing. Science Advances. (2017) 3:E1601649. doi: 10.1126/sciadv.1601649
141. Prasad A, Sanchez JC. Quantifying long-term microelectrode array functionality using chronic in vivo impedance testing. J Neural Eng. (2012) 9. doi: 10.1088/1741-2560/9/2/026028
142. Megía García A, Serrano-Muñoz D, Taylor J, Avendaño-Coy J, Gómez-Soriano J. Transcutaneous spinal cord stimulation and motor rehabilitation in spinal cord injury: a systematic review. Neurorehabil Neural Repair. (2020) 34:3–12. doi: 10.1177/1545968319893298
143. Distler T, Boccaccini AR. 3d printing of electrically conductive hydrogels for tissue engineering and biosensors – a review. Biomater. (2020) 1:1–13. doi: 10.1016/j.actbio.2019.08.044
144. Abidian MR, Daneshvar ED, Egeland BM, Kipke DR, Cederna PS, Urbanchek MG. Hybrid conducting polymer-hydrogel conduits for axonal growth and neural tissue engineering. Adv Healthc Mater. (2012) 1:762–7. doi: 10.1002/adhm.201200182
145. Liu L, Li P, Zhou G, Wang M, Jia X, Liu M. Increased proliferation and differentiation of pre-osteoblasts mc3t3-e1 cells on nanostructured polypyrrole membrane under combined electrical and mechanical stimulation. J Biomed Nanotechnol. (2013) 9:1532–9. doi: 10.1166/jbn.2013.1650
146. Wang L, Wu Y, Hu T, Guo B, Ma PX. Electrospun conductive nanofibrous scaffolds for engineering cardiac tissue and 3d bioactuators. Acta Biomater. (2017) 1:68–81. doi: 10.1016/j.actbio.2017.06.036
147. Pelto J, Björninen M, Pälli A, Talvitie E, Hyttinen J, Mannerström B, et al. Novel polypyrrole-coated polylactide scaffolds enhance adipose stem cell proliferation and early osteogenic differentiation. Tissue Eng Part A. (2013) 78:882–92. doi: 10.1089/ten.TEA.2012.0111
148. Distler T, Polley C, Shi F, Schneidereit D, Ashton MD, Friedrich O. Electrically Conductive And 3d-Printable Oxidized Alginate-Gelatin Polypyrrole:Pss Hydrogels For Tissue Engineering. Adv Healthc Mater. (2021) 10:E2001876. doi: 10.1002/adhm.202001876
149. Shah A, Firlak M, Berrow SR, Halcovitch NR, Baldock SJ, Yousafzai BM. Electrochemically enhanced drug delivery using polypyrrole films. Materials. (2018) 11:1123. doi: 10.3390/ma11071123
150. Chen X, Wu Y, Ranjan VD, Zhang Y. Three-dimensional electrical conductive scaffold from biomaterial-based carbon micro fiber sponge with bioinspired coating for cell proliferation and differentiation. Carbon N Y. (2018) 134:174–82. doi: 10.1016/j.carbon.2018.03.064
151. Magaz A, Li X, Gough JE, Blaker JJ. Graphene oxide and electroactive reduced graphene oxide-based composite fibrous scaffolds for engineering excitable nerve tissue. Mater Sci Eng C Mater Biol Appl. (2020) 119:111632. doi: 10.1016/j.msec.2020.111632
152. Kim JH, Kataoka M, Jung YC, Ko YI, Fujisawa K, Hayashi T, et al. Mechanically tough, electrically conductive polyethylene oxide nanofiber web incorporating dna-wrapped double-walled carbon nanotubes. Acs Appl Mater Interfaces. (2013) 5:4150–4. doi: 10.1021/am400715u
153. Li N, Zhang Q, Gao S, Song Q, Huang R, Wang L, et al. Three-dimensional graphene foam as a biocompatible and conductive scaffold for neural stem cells. Sci Rep. (2013) 3:1604. doi: 10.1038/srep01604
154. Guo W, Wang S, Yu X, Qiu J, Li J, Tang W, et al. Construction of a 3d rgo-collagen hybrid scaffold for enhancement of the neural differentiation of mesenchymal stem cells. Nanoscale. (2016) 8:1897–904. doi: 10.1039/C5NR06602F
155. Baranes K, Shevach M, Shefi O, Dvir T. Gold nanoparticle-decorated scaffolds promote neuronal differentiation and maturation. Nano Lett. (2016) 16:2916–20. doi: 10.1021/acs.nanolett.5b04033
156. Wickham A, Vagin M, Khalaf H, Bertazzo S, Hodder P, Dånmark S. Electroactive biomimetic collagen-silver nanowire composite scaffolds. Nanoscale. (2016) 8:14146–55. doi: 10.1039/C6NR02027E
157. Sezer UA, Ozturk K, Aru B, Demirel GY, Sezer S, Bozkurt MR. Zero valent zinc nanoparticles promote neuroglial cell proliferation: a biodegradable and conductive filler candidate for nerve regeneration. J Mater Sci: Mater Med. (2017) 28:19. doi: 10.1007/s10856-016-5831-1
158. Zhou L, Fan L, Yi X, Zhou Z, Liu C, Fu R, et al. Soft conducting polymer hydrogels cross-linked and doped by tannic acid for spinal cord injury repair. ACS Nano. (2018) 12:10957–67. doi: 10.1021/acsnano.8b04609
159. Lorite GS, Ylä-Outinen L, Janssen L, Pitkänen O, Joki T, Koivisto JT, et al. Carbon nanotube micropillars trigger guided growth of complex human neural stem cells networks. Nano Res. (2019) 12:2894–9. doi: 10.1007/s12274-019-2533-2
160. Lee SJ, Zhu W, Nowicki M, Lee G, Heo DN, Kim J, et al. 3D printing nano-conductive multi-walled carbon nanotube scaffolds for nerve regeneration. J Neural Eng. (2018) 15:016018. doi: 10.1088/1741-2552/aa95a5
161. Pan S, Qi Z, Li Q, Ma Y, Fu C, Zheng S, et al. Graphene oxide-plga hybrid nanofibres for the local delivery of igf-1 and bdnf in spinal cord repair. Artif Cells Nanomed Biotechnol. (2019) 47:651–64. doi: 10.1080/21691401.2019.1575843
162. Qi Z, Guo W, Zheng S, Fu C, Ma Y, Pan S, et al. Enhancement of neural stem cell survival, proliferation and differentiation by igf-1 delivery. In Rsc Adv. (2019) 8:8315–8325 doi: 10.1039/C8RA10103E
163. Liu H, Wang Y, Yang Y, Wang A, Huang C, Zhao Z, et al. Aligned graphene/silk fibroin conductive fibrous scaffolds for guiding neurite outgrowth in rat spinal cord neurons. J Biomed Mater Res A. (2021) 109:488–99. doi: 10.1002/jbm.a.37031
164. López-Dolado E, González-Mayorga A, Gutiérrez MC, Serrano MC. Immunomodulatory and angiogenic responses induced by graphene oxide scaffolds in chronic spinal hemisected rats. Biomaterials. (2016) 9:72–81. doi: 10.1016/j.biomaterials.2016.05.012
165. Lutolf M, Hubbell J. Synthetic biomaterials as instructive extracellular microenvironments for morphogenesis in tissue engineering. Nat Biotechnol. (2005) 23:47–55. doi: 10.1038/nbt1055
166. Lee HJ, Park J, Yoon OJ, Kim HW, Lee DY, Kim DH. Amine-modified single-walled carbon nanotubes protect neurons from injury in a rat stroke model. Nat Nanotechnol. (2011) 6:121–5. doi: 10.1038/nnano.2010.281
167. Zhou K, Motamed S, Thouas GA, Bernard CC, Li D, Parkington HC, et al. Graphene functionalized scaffolds reduce the inflammatory response and supports endogenous neuroblast migration when implanted in the adult brain. PLoS ONE. (2016) 11:E0151589. doi: 10.1371/journal.pone.0151589
168. Agarwal G, Kumar N, Srivastava A. Highly elastic, electroconductive, immunomodulatory graphene crosslinked collagen cryogel for spinal cord regeneration. Mater Sci Eng C Mater Biol Appl. (2021) 118:111518. doi: 10.1016/j.msec.2020.111518
169. Eftekhari BS, Eskandari M, Janmey PA, Samadikuchaksaraeic A, Gholipourmalekabadi M. Conductive chitosan/polyaniline hydrogel with cell-imprinted topography as a potential substrate for neural priming of adipose derived stem cells. RSC Adv. (2021) 11:15795. doi: 10.1039/D1RA00413A
170. Schmidt CE, Shastri VR, Vacanti JP, Langer R. Stimulation of neurite outgrowth using an electrically conducting polymer. Proc Natl Acad Sci U S A. (1997) 94:8948–53. doi: 10.1073/pnas.94.17.8948
171. Moroder P, Runge MB, Wang H, Ruesink T, Lu L, Spinner RJ, et al. Material properties and electrical stimulation regimens of polycaprolactone fumarate–polypyrrole scaffolds as potential conductive nerve conduits. Acta Biomater. (2011) 7:944–53. doi: 10.1016/j.actbio.2010.10.013
172. Farkhondehnia H, Mohammed TA, Zamani F. Fabrication of biocompatible plga/pcl/pani nanofibrous scaffolds with electrical excitability. Fibers Polymers. (2018) 19:1813–9. doi: 10.1007/s12221-018-8265-1
173. Qing H, Jin G, Zhao G, Huang G, Ma Y, Zhang X, et al. Heterostructured silk-nanofiber-reduced graphene oxide composite scaffold for sh-sy5y cell alignment and differentiation. Acs Appl Mater Interfaces. (2018) 10:39228–37. doi: 10.1021/acsami.8b12562
174. Zhu W, Ye T, Lee S, Cui H, Miao S, Zhou X, et al. Enhanced neural stem cell functions in conductive annealed carbon nanofibrous scaffolds with electrical stimulation. Nanomedicine. (2018) 14:2485–94. doi: 10.1016/j.nano.2017.03.018
175. Hernández-Bule ML, Paíno CL, Trillo MÁ, Úbeda A. Electric stimulation at 448 khz promotes proliferation of human mesenchymal stem cells. Cellular Physiol Biochem. (2014) 34:741–55. doi: 10.1159/000366375
176. Song S, Amores D, Chen C, McConnell K, Oh B, Poon A, et al. Controlling properties of human neural progenitor cells using 2d and 3d conductive polymer scaffolds. Sci Reports. (2019) 9:19565. doi: 10.1038/s41598-019-56021-w
177. Girão AF, Sousa J, Domínguez-Bajo A, González-Mayorga A, Bdikin I, Pujades-Otero E, et al. 3d reduced graphene oxide scaffolds with a combinatorial fibrous-porous architecture for neural tissue engineering. Acs Appl Mater Interfaces. (2020) 12:38962–75. doi: 10.1021/acsami.0c10599
178. Shin J, Choi EJ, Cho JH, Cho AN, Jin Y, Yang K, et al. Three-dimensional electroconductive hyaluronic acid hydrogels incorporated with carbon nanotubes and polypyrrole by catechol-mediated dispersion enhance neurogenesis of human neural stem cells. Biomacromolecules. (2017) 18:3060–72. doi: 10.1021/acs.biomac.7b00568
179. Fu C, Pan S, Ma Y, Kong W, Qi Z, Yang X. Effect of electrical stimulation combined with graphene-oxide-based membranes on neural stem cell proliferation and differentiation. Artificial Cells, Nanomedicine, Biotechnol. (2019) 47:1867–76. doi: 10.1080/21691401.2019.1613422
180. Pelin M, Fusco L, León V, Martín C, Criado A, Sosa S, et al. Differential cytotoxic effects of graphene and graphene oxide on skin keratinocytes. Sci Rep. (2017) 7:40572. doi: 10.1038/srep40572
181. Theodore N, Hlubek R, Danielson J, Neff K, Vaickus L, Ulich TR, et al. First human implantation of a bioresorbable polymer scaffold for acute traumatic spinal cord injury: a clinical pilot study for safety and feasibility. Neurosurgery. (2016) 79:E305–12. doi: 10.1227/NEU.0000000000001283
182. Prager J, Adams CF, Delaney AM, Chanoit G, Tarlton JF, Wong LF, et al. Stiffness-matched biomaterial implants for cell delivery: clinical, intraoperative ultrasound elastography provides a 'target' stiffness for hydrogel synthesis in spinal cord injury. J Tissue Eng. (2020) 11:2041731420934806. doi: 10.1177/2041731420934806
183. Qian Y, Yuan WE, Cheng Y, Yang Y, Qu X, Fan C. Concentrically integrative bioassembly of a three-dimensional black phosphorus nanoscaffold for restoring neurogenesis, angiogenesis, and immune homeostasis. Nano Lett. (2019) 19:8990–9001. doi: 10.1021/acs.nanolett.9b03980
184. Qian Y, Wang X, Song J, Chen W, Chen S, Jin Y, et al. Preclinical assessment on neuronal regeneration in the injury-related microenvironment of graphene-based scaffolds. Npj Regen Med. (2021) 6:31. doi: 10.1038/s41536-021-00142-2
Keywords: spinal cord injury, cell therapy, tissue engineering & regenerative medicine, electroactive, electrical stimulation
Citation: Mutepfa AR, Hardy JG and Adams CF (2022) Electroactive Scaffolds to Improve Neural Stem Cell Therapy for Spinal Cord Injury. Front. Med. Technol. 4:693438. doi: 10.3389/fmedt.2022.693438
Received: 10 April 2021; Accepted: 10 January 2022;
Published: 22 February 2022.
Edited by:
Kunal Mitra, Florida Institute of Technology, United StatesReviewed by:
Gwendolen Clair Reilly, The University of Sheffield, United KingdomPallab Datta, Indian Institute of Engineering Science and Technology, India
Copyright © 2022 Mutepfa, Hardy and Adams. This is an open-access article distributed under the terms of the Creative Commons Attribution License (CC BY). The use, distribution or reproduction in other forums is permitted, provided the original author(s) and the copyright owner(s) are credited and that the original publication in this journal is cited, in accordance with accepted academic practice. No use, distribution or reproduction is permitted which does not comply with these terms.
*Correspondence: John G. Hardy, ai5nLmhhcmR5JiN4MDAwNDA7bGFuY2FzdGVyLmFjLnVr; Christopher F. Adams, Yy5hZGFtcyYjeDAwMDQwO2tlZWxlLmFjLnVr