- 1Department of Biomedical Engineering, Technion–Israel Institute of Technology, Haifa, Israel
- 2Graduate Program in Nanoscience and Nanotechnology, RBNI, Technion–Israel Institute of Technology, Haifa, Israel
In this short perspective, we explore the potential of leveraging electrostatic forces in the lungs to enhance pulmonary drug delivery methods and optimize drug delivery efficiency and therapeutic outcomes. Alongside conventional mechanisms such as diffusion, gravitational sedimentation, and impaction, we delve into electrostatic mechanisms, utilizing a non-dimensional analysis approach for insights into aerosol drug delivery. While often overlooked in inhalation therapy, our considerations emphasize the significance of electrostatic interactions on drug deposition, particularly in the deep lung, where, in the future, tailored electrostatic charges can strategically offer new possibilities for localized therapeutic effects for respiratory diseases.
Introduction
Inhaled aerosol therapy represents a cornerstone in treating respiratory conditions and diseases including asthma, chronic obstructive pulmonary disease (COPD), and cystic fibrosis (CF) to name a few (Laube, 2005; Stein and Thiel, 2017). Concurrently, beyond the delivery of inhaled medication for topical airway treatment, the lungs present a potent gateway for systemic delivery. Due to the high permeability of the thin alveolar-capillary barrier (∼1 µm thick) combined with the vast pulmonary surface area (on the order of ∼100 m2 in an adult), deposited molecules can be rapidly absorbed into the systemic circulation (Ji et al., 1995; Patton, 1996). Despite the clinical prevalence of inhalation therapy, pulmonary deposition efficiencies remain typically low (i.e., often <50% of a nominal dose inhaled), a reality that leads, for example, to over-compensation by administrating larger doses (de Boer et al., 2017; Choi et al., 2019). This situation is even more dire in young pediatric populations where deposition efficiencies can lie well below 10% using conventional inhalers (Amirav and Newhouse, 2012; Das et al., 2018). While the respiratory community has long recognized the pitfalls in the efficiency of inhalation therapy, understanding the transport dynamics of inhaled aerosols can help shed light on novel approaches to enhance deposition in targeted areas of the lungs while reducing superfluous deposition in undesired airway sites.
From an evolutionary perspective, the respiratory tract is well adapted to protect the delicate airway milieu from the deposition of undesired inhaled particulate matter (PM), e.g., airborne pathogens, smoke, debris, etc., via mucociliary clearance (King, 2006) and immune responses (Diamond et al., 2000), in conjunction with filtering occurring from the anatomic intricacies of the lungs (e.g., mouth-throat region). Most notably, the nasal cavities are highly efficient in screening and trapping coarse PM of ∼10 µm in diameter or larger (Schwab and Zenkel, 1998). In turn, pharmaceutical aerosols follow broad design recommendations within the size range of 1–5 µm for improved deposition in the peripheral airways (Darquenne, 2012). An often less recognized aspect lies in the tendency of off-the-shelf inhaler devices to accumulate high amounts of electrostatic charges on the generated airborne particles (Kwok and Chan, 2010), a phenomenon characterized by the electric charge
Although the effect of electrical charge has been recognized for nearly a century (Wilson, 1947), the discussion of electrostatic forces on inhaled aerosols is often overshadowed by the role of traditional deposition mechanisms (i.e., inertial impaction, gravitational sedimentation, Brownian diffusion). When electrical charges are indeed addressed, they are mostly alluded to as a source for additional losses in the extra-thoracic (e.g., mouth, throat) and upper respiratory airways (Cohen et al., 1995; Azhdarzadeh et al., 2014a; Azhdarzadeh et al., 2014b; Xi et al., 2014; Azhdarzadeh et al., 2015), thereby reducing the net dose available for effective treatment in the distal lungs. Since the electrostatic force
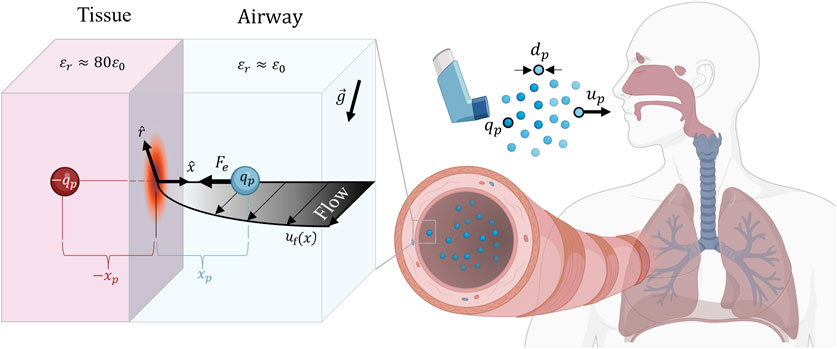
FIGURE 1. Schematic of the journey of an inhaled aerosol with mass
To date, the role of electrostatic forces on aerosol deposition in the distal lungs has received relatively little attention in either experiments or simulations (Bailey et al., 1998; Majid et al., 2012), with many questions remaining. In this short perspective, we first briefly revisit the physical underpinnings of electrostatic forces on inhaled aerosols and extract, via non-dimensional analysis, the relevant parameter spaces in which electrostatics appear to dominate aerosol deposition quickly. Furthermore, we discuss the potential of leveraging such electrostatic properties for improved pulmonary drug delivery and the outstanding engineering challenges to overcome in ultimately leveraging such effects. In a final step, we identify a number of clinical scenarios in which electrostatics effects deserve further consideration in inhalation therapy.
Electrostatics in off-the-shelf inhalers
In the realm of inhaler devices, a wide range of electric charges is observed, varying from single electrons, as seen in the case of nebulizers (Kwok and Chan, 2010), to several hundred electrons, such as in metered-dose inhalers (MDI) and Dry Powder Inhalers (DPI). For instance, a particle generated from DPI Pulmicort was estimated to carry approximately
Moreover, spacer devices, which play a pivotal role in enhancing MDI aerosol drug delivery, are found to be susceptible to the charged aerosols produced by MDIs. Spacers are predominantly constructed from non-conductive materials, leading to the accumulation of charges when handled by patients (Newman, 2004). These charges can interact with the highly charged aerosol produced by the MDI, potentially affecting drug output. Fortunately, effective solutions exist to mitigate this issue, including the use of conductive metal spacers (e.g., the Nebuchamber) (Janssens et al., 1999; Janssens et al., 2000), and the application of detergent or surfactant coatings (Waterer et al., 1998; Piérart et al., 1999; Kwok et al., 2006). These materials prevent the retention of charge concentrations, ultimately enhancing the efficiency of MDIs’ drug delivery.
It is important to note that there are various ways to adjust the mentioned inhalers to achieve desired aerosol charge levels. For MDIs, factors like propellant type (Kwok et al., 2005), water content (Chi Lip Kwok et al., 2008), drug formulation (Kwok and Chan, 2010), and inhaler components (Carter et al., 2011), influence charge levels. DPIs can be manipulated through component selection and particle surface properties (Amorphous vs Crystalline) (Bailey, 1993; Kwok and Chan, 2010), as well as Relative Humidity (RH) during storage and dispersion (Young et al., 2007; Kwok and Chan, 2008). Nebulizers exhibit charge modulation based on parameters such as temperature, pH, ionic content, and conductivity (Kwok and Chan, 2010; Yatsuzuka et al., 1994; Yatsuzuka et al., 1996; Vaaraslahti et al., 2002).
Particle dynamics and the electrostatic force
Various mechanistic forces act on an aerosol of diameter
Inhaled charged aerosol particles can interact with surrounding tissue and with one another. Notably, particles carrying similar charges exhibit more rapid dispersion of the droplet cloud (Balachandran et al., 1997). This behavior can be attributed to the fundamental principle of electrostatic repulsion between the aerosols and is referred to as “space charge” (Spc). This becomes particularly significant in environments with dense aerosol clouds characterized by high concentrations (>1012 particles/m³) and charge levels on the order of hundreds of electrons, as often used in industrial applications (Osman et al., 2015). However, it is important to note that this phenomenon is generally absent in the context of inhalation therapy due to notably lower aerosol concentrations. While it may occur near the device’s exit and within the mouth region, such phenomenon weakens considerably within the main respiratory tract. (Kwok et al., 2005). Hence, in the realm of aerosol deposition within the lungs, the primary electrostatic effect steams from the interaction between charged airborne particles and the lung tissue (Balachandran et al., 1997; Finlay, 2021).
While the airway surface epithelium typically maintains a negative charge, with ion transport regulating lung cell homeostasis (Zhao et al., 2022; Yeung et al., 2008), the electrical charge within human airways, from the perspective of an airborne particle, remains neutral (Kwok and Chan, 2010). Human mucus, composed of water, glycoproteins, and various molecules (Bansil and Turner, 2018) functions as a dielectric material, capable of storing electrical charge and responding to external electric fields, similar to liquid water. Although the electrical properties of both inflated and deflated lungs have been collectively measured (Sasaki et al., 2022), direct electrical measurements of tissue from the perspective of aerosol deposition remain unestablished. Techniques such as conductive atomic force microscopy (cAFM) could potentially verify tissue conductivity, but have never been reported, possibly due to the existence of robust in vivo data supporting the notion of electrostatic enhancement in deposition (Fraser and Hill, 1966; Biology, 1983; Prodi and Mularoni, 1985; Cohen et al., 1998; Kwok et al., 2021). The lung tissue exhibits an electrical conductivity and a dielectric constant (ε) similar to that of conducting saline water (Bailey et al., 1998). When a particle with charge
As a final remark, one must recognize that none of the forces acting on an inhaled airborne particle is specifically oriented to enhance airway deposition. For example, the gravitational force is directed toward the Earth’s center, diffusion involves a random walk and impaction depends on the particle’s flight trajectory (Hinds and Zhu, 2022). In contrast, electrostatic charge is at the source of the only mechanism that actively encourages a particle to deposit along its shortest trajectory to the airway surface (Bailey et al., 1998).
Non-dimensional analysis
Despite the above simplification to quantify the electrostatic force (i.e., induced image force), the particle transport equations (in Newton’s second law) nevertheless remain a challenging nonlinear second-order ordinary differential equation with no simple analytical solution. In turn, much research in the field has benefited from numerical approaches, most notably computational fluid dynamics (CFD) (Osman et al., 2015; Koullapis et al., 2016). Yet, much insight can be immediately gained via non-dimensional analysis in assessing the relative importance of each contributing force, as highlighted below.
We recall that the lungs encompass a complex multiscale problem, spanning approximately twenty or so bifurcating airway generations (z). At the trachea (z=0), the characteristic length
Following traditional dimensional analysis for airborne particle (Bailey, 1993), we recover four non-dimensional groups summarized in Table 1 (see Supplementary Material for further details), underlining impaction (Stk), sedimentation (H), diffusion (Pe), and electrostatics (Inc). We may assess their relative importance by plotting corresponding phase maps as a function of aerosol diameter and net electric charge for common inhalers across the lung regions (Swarbrick, 2006; Sznitman, 2013; Hofemeier and Sznitman, 2015), as shown in Figure 2. Distinct areas in the phase map highlight regions where one group dominates over the others, whereas lines between regions delineate when two groups have equal magnitude signifying the superimposition of their effects.
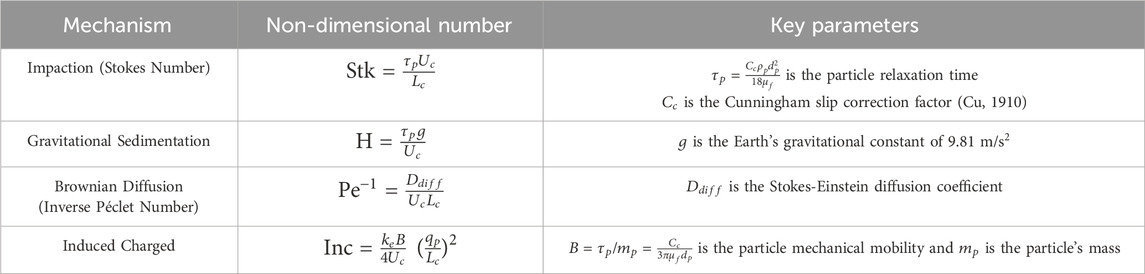
TABLE 1. Summary of the leading mechanisms contributing to aerosol deposition within the lungs, presented as non-dimensional numbers.
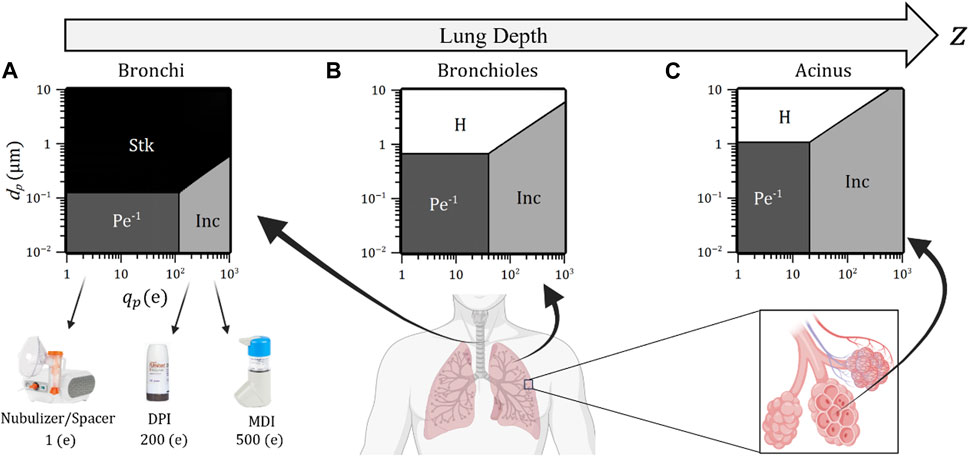
FIGURE 2. Phase maps depicting the leading dimensionless numbers (Stk, H, Pe−1 and Inc.) at various depths of the lungs: (A) bronchi, (B) bronchioles, and (C) in the acinus. The dimensionless numbers are plotted against units of elementary charge
Role of electrostatics in the conducting regions of the lungs
As a general rule, in the extrathoracic and upper airway regions of the lungs (Figure 2A) the main deposition mechanism for particles larger than 5 µm is often attributed to impaction (corresponding to large values of Stk). Nevertheless, sedimentation still plays a significant role for particles larger than approximately 1 µm in the main conducting airways. This phenomenon largely overshadows diffusion and electrostatic effects. However, for high amounts of charge (Bailey et al., 1998) or in the case of ultrafine particles (
In the small bronchioles, with the rapid decrease in airflow velocities the Stk number becomes less dominant than the gravity number H. Two interesting phenomena may be observed. Firstly, due to the smaller characteristic length
When considering airway sizes and anatomy, the case of pediatric patients is of particular relevance, where deposition efficiency (DE) is often underwhelming, with typical results reaching only around 30% of the provided dosage and dropping as low as 5% depending on age and condition (Schueepp et al., 2009). Recent reviews continue to highlight this issue (Amirav and Newhouse, 2012; Schüepp et al., 2004), pointing out the rule of thumb that “the younger the patient, the worse the targeting efficiency" (Oakes et al., 2023). Contrary to common intuition, smaller lungs in children do not imply weaker airflows, since children’s lungs have narrow airways (i.e., smaller cross-sectional areas) but faster breathing rates, leading to higher velocities (Das et al., 2018; Oakes et al., 2023; Oakes et al., 2018). We recall that
To the best of our knowledge, the smallest models used to explore electrostatic effects in vitro have been hollow casts of adult human airways that span down to the sixth generation (Cohen et al., 1998). For infants, despite the considerations we described, research has largely focused on the upper airways (Azhdarzadeh et al., 2014b) and the effect of neutralizing charge via spacers (Osman et al., 2015; Finlay, 2021), underlining the need for further research.
Role of electrostatics in the deep acinar regions
Gravitational sedimentation (H) and Brownian diffusion (
We recall that aerosols in the size range of 5–10 μm are mostly susceptible to deposition in proximal airway regions (i.e., extra-thoracic and upper airways), regardless of charge. Even for smaller particles, such as those between 1–5 μm, a substantial proportion will not successfully reach the deeper regions of the lungs without careful adjustment of inhalation flow rates (Koullapis et al., 2016; Heyder et al., 1986; Kleinstreuer et al., 2008; Kleinstreuer and Zhang, 2010; Islam et al., 2017). For example, the broad deposition efficiency of 2 μm particles is less than ∼15% in the acinar regions (Piérart et al., 1999; Prodi and Mularoni, 1985). Yet, such assessments are typically based on spherical particles and alternative particle shapes could be considered (Kleinstreuer et al., 2008; Kleinstreuer and Zhang, 2010; Islam et al., 2017). For example, long straight fibers align with the flow due to fluid shear stress, enabling them to penetrate deeper into the lungs than spherical particles with the same diameter as the fiber length (Asgharian and Yu, 1988; Harris and Timbrell, 1975). Fibers experience stronger drag forces that hinder gravitational sedimentation, leading to longer air retention compared to spherical particles of the same mass (Shachar-Berman et al., 2018). Asbestos fibers, for instance, with lengths of 50–200 μm, yield significant deposition in the alveolar space, causing a dramatic decline in health conditions (Timbrell, 1965; Donaldson et al., 1989; Kamp, 2009; Barlow et al., 2017; Tsuda et al., 2013; Stahlhofen et al., 1989; Velkov et al., 2015; Sznitman et al., 2016). In vivo, studies have shown that charged asbestos fibers exhibit much more acinar deposition than neutralized ones (Davis et al., 1988). Hence, the combination of fiber particles with electrostatics offers a potential strategy for targeted drug delivery to the acinar regions. This is particularly relevant for therapeutic agents, which when deposited in the upper airways, can cause important side effects. For instance, pentamidine, an effective antifungal medication used in chemotherapy, can only be prescribed to individuals allergic to alternatives due to its harmful side effects in the upper airways (Hofemeier and Sznitman, 2015; Ari and Fink, 2020). Such considerations embody the potential of combining shape and electrostatic charge to design airborne carriers tailored to reach the deep lungs.
As a final remark, we recall that the deep respiratory regions encompass ∼90% of the total lung volume (Knudsen and Ochs, 2018). Therefore, when examining the phase map in the acinar space (Figure 2C), it becomes even more striking that from a non-dimensional perspective the vast majority of the lung space is susceptible to electrostatic effects.
Conclusion
In this short perspective, we revisited the significance of electrostatics in inhalation therapy with special consideration to deposition in the distal regions of the lungs. Our non-dimensional analysis reveals the crucial importance of this mechanism, showing its potential for improved pulmonary delivery strategies. Until now, little attention has been paid to how electrostatic mechanisms alter aerosol deposition at the smallest scales of the lungs, while there has been extensive research on such phenomenon in the upper airways (Kwok and Chan, 2010). Most notably, the well-known ICPR reference curves entirely overlook the role of electrostatic and charge (ICRP, 1994). The quest to identify the electrostatic window of opportunity should inspire drug delivery researchers to engineer novel deposition strategies for both higher drug efficiency and targeted delivery within the lungs. Integrating charge as a key design parameter, alongside more traditional factors such as particle size, shape, characteristic airflow velocity, can provide a more comprehensive framework to enhance the current state of pulmonary drug delivery.
Data availability statement
The original contributions presented in the study are included in the article/Supplementary Material, further inquiries can be directed to the corresponding author.
Author contributions
RB: Conceptualization, Data curation, Formal Analysis, Investigation, Visualization, Writing–original draft. JS: Conceptualization, Funding acquisition, Methodology, Project administration, Supervision, Writing–original draft.
Funding
The author(s) declare financial support was received for the research, authorship, and/or publication of this article. This research was supported by the Israel Science Foundation (Grant no. 1840/21).
Conflict of interest
The authors declare that the research was conducted in the absence of any commercial or financial relationships that could be construed as a potential conflict of interest.
The author(s) declared that they were an editorial board member of Frontiers, at the time of submission. This had no impact on the peer review process and the final decision.
Publisher’s note
All claims expressed in this article are solely those of the authors and do not necessarily represent those of their affiliated organizations, or those of the publisher, the editors and the reviewers. Any product that may be evaluated in this article, or claim that may be made by its manufacturer, is not guaranteed or endorsed by the publisher.
Supplementary material
The Supplementary Material for this article can be found online at: https://www.frontiersin.org/articles/10.3389/fmede.2023.1298251/full#supplementary-material
References
Amirav, I., and Newhouse, M. T. (2012). Deposition of small particles in the developing lung. Paediatr. Respir. Rev. 13 (2), 73–78. doi:10.1016/j.prrv.2011.05.006
Ari, A., and Fink, J. B. (2020). Recent advances in aerosol devices for the delivery of inhaled medications. Expert Opin. Drug Deliv. 17 (2), 133–144. doi:10.1080/17425247.2020.1712356
Asgharian, B., and Yu, C. P. (1988). Deposition of inhaled fibrous particles in the human lung. J. Aerosol Med. 1 (1), 37–50. doi:10.1089/jam.1988.1.37
Azhdarzadeh, M., Olfert, J. S., Vehring, R., and Finlay, W. H. (2014a). Effect of electrostatic charge on oral-extrathoracic deposition for uniformly charged monodisperse aerosols. J. Aerosol Sci. 68, 38–45. doi:10.1016/j.jaerosci.2013.11.002
Azhdarzadeh, M., Olfert, J. S., Vehring, R., and Finlay, W. H. (2014b). Effect of induced charge on deposition of uniformly charged particles in a pediatric oral-extrathoracic airway. Aerosol Sci. Technol. 48 (5), 508–514. doi:10.1080/02786826.2014.896989
Azhdarzadeh, M., Olfert, J. S., Vehring, R., and Finlay, W. H. (2015). Effect of electrostatic charge on deposition of uniformly charged monodisperse particles in the nasal extrathoracic airways of an infant. J. Aerosol Med. Pulm. Drug Deliv. 28 (1), 30–34. doi:10.1089/jamp.2013.1118
Bailey, A. G., Hashish, A. H., and Williams, T. J. (1998). Drug delivery by inhalation of charged particles. J. Electrost. 44 (1–2), 3–10. doi:10.1016/S0304-3886(98)00017-5
Bailey, A. G. (1993). Charging of solids and powders. J. Electrost. 30 (6), 167–180. doi:10.1016/0304-3886(93)90072-f
Balachandran, W., Machowski, W., Gaura, E., Hudson, C., and Systems, E. (1997). Control of drug aerosol in human airways using electrostatic forces. J. Electrost. 40-41 (97), 579–584. doi:10.1016/s0304-3886(97)00106-x
Bansil, R., and Turner, B. S. (2018). The biology of mucus: composition, synthesis and organization. Adv. Drug Deliv. Rev. 124, 3–15. doi:10.1016/j.addr.2017.09.023
Barlow, C. A., Grespin, M., and Best, E. A. (2017). Asbestos fiber length and its relation to disease risk. Inhal. Toxicol. 29 (12–14), 541–554. doi:10.1080/08958378.2018.1435756
Biology, R. (1983). The Effect of Aerosol Charge on the Deposition and Clearance of TiOp Particles in Rats chamber atmosphere was sampled through a cylindrical condenser in series with a second Sinclair-Phoenix photometer. Relat. amount aerosol deposited con 151, 148–151. doi:10.1016/0013-9351(83)90071-3
Byron, P. R., Peart, J., and Staniforth, J. N. (1997). Aerosol electrostatics I: properties of fine powders before and after aerosolization by dry powder inhalers. Pharm. Res. 14 (6), 698–705. doi:10.1023/A:1012181818244
Carter, P. A., Rowley, G., Roughley, N., and Suggett, J. (2011). Electrostatic charge accumulation and decay in pharmaceutical polymer materials used in metered dose inhalers. J. Pharm. Pharmacol. 50 (Suppl. ment_9), 55. doi:10.1111/j.2042-7158.1998.tb02255.x
Chi Lip Kwok, P., Noakes, T., and Chan, H.-K. (2008). Effect of moisture on the electrostatic charge properties of metered dose inhaler aerosols. J. Aerosol Sci. 39 (3), 211–226. doi:10.1016/j.jaerosci.2007.11.004
Choi, J., LeBlanc, L. J., Choi, S., Haghighi, B., Hoffman, E. A., O’Shaughnessy, P., et al. (2019). Differences in particle deposition between members of imaging-based asthma clusters. J. Aerosol Med. Pulm. Drug Deliv. 32 (4), 213–223. doi:10.1089/jamp.2018.1487
Cohen, B. S., Xiong, J. Q., Asgharian, B., and Ayres, L. (1995). Deposition of inhaled charged ultrafine particles in a simple tracheal model. J. Aerosol Sci. 26 (7), 1149–1160. doi:10.1016/0021-8502(95)00039-f
Cohen, B. S., Xiong, J. Q., Fang, C.-P., and Li, W. (1998). Deposition of charged particles on lung airways. Health Phys. 74 (5), 554–560. doi:10.1097/00004032-199805000-00002
Cu, E. (1910). On the velocity of steady fall of spherical particles through fluid medium. Proc. R. Soc. Lond. Ser. A, Contain. Pap. a Math. Phys. Character 83 (563), 357–365. doi:10.1098/RSPA.1910.0024
Darquenne, C. (2012). Aerosol deposition in health and disease. J. Aerosol Med. Pulm. Drug Deliv. 25 (3), 140–147. doi:10.1089/jamp.2011.0916
Das, P., Nof, E., Amirav, I., Kassinos, S. C., and Sznitman, J. (2018). Targeting inhaled aerosol delivery to upper airways in children: insight from computational fluid dynamics (CFD). PLoS One 13 (11), e0207711. doi:10.1371/journal.pone.0207711
Davis, J. M. G., Bolton, R. E., Douglas, A. N., Jones, A. D., and Smith, T. (1988). Effects of electrostatic charge on the pathogenicity of chrysotile asbestos. Occup. Environ. Med. 45 (5), 292–299. doi:10.1136/oem.45.5.292
de Boer, A. H., Hagedoorn, P., Hoppentocht, M., Buttini, F., Grasmeijer, F., and Frijlink, H. W. (2017). Dry powder inhalation: past, present and future. Expert Opin. Drug Deliv. 14 (4), 499–512. doi:10.1080/17425247.2016.1224846
Diamond, G., Legarda, D., and Ryan, L. K. (2000). The innate immune response of the respiratory epithelium. Immunol. Rev. 173, 27–38. doi:10.1034/j.1600-065X.2000.917304.x
Donaldson, K., Brown, G. M., Brown, D. M., Bolton, R. E., and Davis, J. M. G. (1989). Inflammation generating potential of long and short fibre amosite asbestos samples. Occup. Environ. Med. 46 (4), 271–276. doi:10.1136/oem.46.4.271
England, S. J., Lihou, K., and Robert, D. (2023). Static electricity passively attracts ticks onto hosts. Curr. Biol. 33, 3041–3047.e4. doi:10.1016/j.cub.2023.06.021
Finlay, W. H. (2021). Deposition of aerosols in the lungs: particle characteristics. J. Aerosol Med. Pulm. Drug Deliv. 34 (4), 213–216. doi:10.1089/jamp.2021.29040.whf
Fraser, D. A., and Hill, C. (1966). The deposition of unipolar charged particles in the lungs of animals,” Archives Environ. Health Int. J. 13 (2), 152–157. doi:10.1080/00039896.1966.10664527
Haefeli-Bleuer, B., and Weibel, E. R. (1988). Morphometry of the human pulmonary acinus. Anatomical Rec. 220 (4), 401–414. doi:10.1002/ar.1092200410
Halliday, D., Resnick, R., and Walker, J. (2013). Fundamentals of physics. Hoboken, New Jersey: Wiley.
Harris, R., and Timbrell, V. (1975). The influence of fibre shape in lung deposition-mathematical estimates.
Heyder, J., Gebhart, J., Rudolf, G., Schiller, C. F., and Stahlhofen, W. (1986). Deposition of particles in the human respiratory tract in the size range 0.005–15 μm. J. Aerosol Sci. 17 (5), 811–825. doi:10.1016/0021-8502(86)90035-2
Hinds, W. C., and Zhu, Y. (2022). “Electrical properties,” in Aerosol technology: properties, behavior, and measurement of airborne particles (Hoboken, New Jersey: Wiley), 277–304.
Hofemeier, P., and Sznitman, J. (2015). Revisiting pulmonary acinar particle transport: convection, sedimentation, diffusion, and their interplay. J. Appl. Physiology 118 (11), 1375–1385. doi:10.1152/japplphysiol.01117.2014
ICRP (1994). Human respiratory tract model for radiological protection. PCRP Publ. 66. Ann. ICRP 24, 1–3. doi:10.1093/rpd/53.1-4.107
Islam, M. S., Saha, S. C., Sauret, E., Gemci, T., and Gu, Y. T. (2017). Pulmonary aerosol transport and deposition analysis in upper 17 generations of the human respiratory tract. J. Aerosol Sci. 108, 29–43. doi:10.1016/j.jaerosci.2017.03.004
Janssens, H. M., Devadason, S. G., Hop, W. C. J., Lesouëf, P. N., De Jongste, J. C., and Tiddens, H. A. W. M. (1999). Variability of aerosol delivery via spacer devices in young asthmatic children in daily life. Eur. Respir. J. 13 (4), 787–791. doi:10.1034/j.1399-3003.1999.13d15.x
Janssens, H. M., Heijnen, E., de Jong, V., Hop, W., Holland, W., de Jongste, J., et al. (2000). Aerosol delivery from spacers in wheezy infants: a daily life study. Eur. Respir. J. 16 (5), 850–856. doi:10.1183/09031936.00.16585000
Ji, C. M., Cardoso, W. V., Gebremichael, A., Philpot, R. M., Buckpitt, A. R., Plopper, C. G., et al. (1995). Pulmonary cytochrome P-450 monooxygenase system and Clara cell differentiation in rats. Am. J. Physiology-Lung Cell. Mol. Physiology 269 (3), L394–L402. doi:10.1152/ajplung.1995.269.3.L394
Kamp, D. W. (2009). Asbestos-induced lung diseases: an update. Transl. Res. 153 (4), 143–152. doi:10.1016/j.trsl.2009.01.004
King, M. (2006). Physiology of mucus clearance. Paediatr. Respir. Rev. 7 (Suppl. 1), 212–214. doi:10.1016/j.prrv.2006.04.199
Kleinstreuer, C., and Zhang, Z. (2010). Airflow and particle transport in the human respiratory system. Annu. Rev. Fluid Mech. 42, 301–334. doi:10.1146/annurev-fluid-121108-145453
Kleinstreuer, C., Zhang, Z., and Li, Z. (2008). Modeling airflow and particle transport/deposition in pulmonary airways. Respir. Physiology Neurobiol. 163 (1–3), 128–138. doi:10.1016/j.resp.2008.07.002
Knudsen, L., and Ochs, M. (2018). The micromechanics of lung alveoli: structure and function of surfactant and tissue components. Histochem. Cell Biol. 150 (6), 661–676. doi:10.1007/s00418-018-1747-9
Koullapis, P. G., Kassinos, S. C., Bivolarova, M. P., and Melikov, A. K. (2016). Particle deposition in a realistic geometry of the human conducting airways: effects of inlet velocity profile, inhalation flowrate and electrostatic charge. J. Biomechanics 49 (11), 2201–2212. doi:10.1016/j.jbiomech.2015.11.029
Kwok, P. C. L., and Chan, H. K. (2008). Effect of relative humidity on the electrostatic charge properties of dry powder inhaler aerosols. Pharm. Res. 25 (2), 277–288. doi:10.1007/s11095-007-9377-2
Kwok, P. C. L., and Chan, H.-K. (2010). Electrostatics of pharmaceutical inhalation aerosols. J. Pharm. Pharmacol. 61 (12), 1587–1599. doi:10.1211/jpp/61.12.0002
Kwok, P. C. L., Collins, R., and Chan, H. K. (2006). Effect of spacers on the electrostatic charge properties of metered dose inhaler aerosols. J. Aerosol Sci. 37 (12), 1671–1682. doi:10.1016/j.jaerosci.2006.08.008
Kwok, P. C. L., Glover, W., and Chan, H. K. (2005). Electrostatic charge characteristics of aerosols produced from metered dose inhalers. J. Pharm. Sci. 94 (12), 2789–2799. doi:10.1002/jps.20395
Kwok, P. C. L., Li, D. D., Tang, P., Fincher, L., Browne, E., Finlay, W. H., et al. (2021). Generation and characterization of electrostatically charged radiolabelled aerosols for lung scintigraphy. Aerosol Sci. Technol. 55 (6), 640–652. doi:10.1080/02786826.2021.1878095
Laube, B. L. (2005). The expanding role of aerosols in systemic drug delivery, gene therapy, and vaccination: discussion. Respir. Care 50 (9), 1174–1176. doi:10.1186/2213-0802-2-3
Majid, H., Madl, P., Hofmann, W., and Alam, K. (2012). Implementation of charged particles deposition in stochastic lung model and calculation of enhanced deposition. Aerosol Sci. Technol. 46 (5), 547–554. doi:10.1080/02786826.2011.645957
Melandri, C., Tarroni, G., Prodi, V., De Zaiacomo, T., Formignani, M., and Lombardi, C. (1983). Deposition of charged particles in the human airways. J. Aerosol Sci. 14 (5), 657–669. doi:10.1016/0021-8502(83)90070-8
Newman, S. P. (2004). Spacer devices for metered dose inhalers. Clin. Pharmacokinet. 43 (6), 349–360. doi:10.2165/00003088-200443060-00001
Oakes, J. M., Amirav, I., and Sznitman, J. (2023). Pediatric inhalation therapy and the aerodynamic rationale for age-based aerosol sizes. Expert Opin. Drug Deliv. 20 (00), 1037–1040. doi:10.1080/17425247.2023.2209314
Oakes, J. M., Roth, S. C., and Shadden, S. C. (2018). Airflow simulations in infant, child, and adult pulmonary conducting airways. Ann. Biomed. Eng. 46 (3), 498–512. doi:10.1007/s10439-017-1971-9
Osman, H., Castle, G. P., and Adamiak, K. (2015). Numerical study of particle deposition in electrostatic painting near a protrusion or indentation on a planar surface. J. Electrost. 77, 58–68. doi:10.1016/j.elstat.2015.07.005
Patton, J. S. (1996). Mechanisms of macromolecule absorption by the lungs. Adv. Drug Deliv. Rev. 19 (1), 3–36. doi:10.1016/0169-409x(95)00113-l
Piérart, F., Wildhaber, J. H., Vrancken, I., Devadason, S. G., and Le Souëf, P. N. (1999). Washing plastic spacers in household detergent reduces electrostatic charge and greatly improves delivery. Eur. Respir. J. 13 (3), 673–678. doi:10.1183/09031936.99.13367399
Prodi, V., and Mularoni, A. (1985). Electrostatic lung deposition experiments with humans and animals. Ann. Occup. Hyg. 29 (2), 229–240. doi:10.1093/annhyg/29.2.229
Sasaki, K., Porter, E., Rashed, E. A., Farrugia, L., and Schmid, G. (2022). Measurement and image-based estimation of dielectric properties of biological tissues —past, present, and future—. Phys. Med. Biol. 67 (14), 14TR01. doi:10.1088/1361-6560/ac7b64
Schueepp, K. G., Devadason, S. G., Roller, C., Minocchieri, S., Moeller, A., Hamacher, J., et al. (2009). Aerosol delivery of nebulised budesonide in young children with asthma. Respir. Med. 103 (11), 1738–1745. doi:10.1016/j.rmed.2009.04.029
Schüepp, K. G., Straub, D., Möller, A., and Wildhaber, J. H. (2004). Deposition of aerosols in infants and children. J. Aerosol Med. 17 (2), 153–156. doi:10.1089/0894268041457228
Schwab, J. A., and Zenkel, M. (1998). Filtration of particulates in the human nose. Laryngoscope 108 (1), 120–124. doi:10.1097/00005537-199801000-00023
Shachar-Berman, L., Ostrovski, Y., De Rosis, A., Kassinos, S., and Sznitman, J. (2018). Transport of ellipsoid fibers in oscillatory shear flows: implications for aerosol deposition in deep airways, Eur. J. Pharm. Sci. 113. 145–151. doi:10.1016/j.ejps.2017.09.023
Stahlhofen, W., Rudolf, G., and James, A. C. (1989). Intercomparison of experimental regional aerosol deposition data. J. Aerosol Med. 2 (3), 285–308. doi:10.1089/jam.1989.2.285
Stein, S. W., and Thiel, C. G. (2017). The history of therapeutic aerosols: a chronological review. J. Aerosol Med. Pulm. Drug Deliv. 30 (1), 20–41. doi:10.1089/jamp.2016.1297
Swarbrick, J. (2006). Encyclopedia of pharmaceutical technology. Third Edition. Milton Park, in Oxfordshire: Taylor & Francis.
Sznitman, J., Hofemeier, P., and Hofemeier, P. (2016). Augmenting regional and targeted delivery in the pulmonary acinus using magnetic particles. Int. J. Nanomedicine 11, 3385–3395. doi:10.2147/IJN.S102138
Sznitman, J. (2013). Respiratory microflows in the pulmonary acinus. J. Biomechanics 46 (2), 284–298. doi:10.1016/j.jbiomech.2012.10.028
Timbrell, V. (1965). Human exposure to asbestos: dust controls and standards. The inhalation of fibrous dusts. Ann. N. Y. Acad. Sci. 132 (1), 255–273. doi:10.1111/j.1749-6632.1965.tb41107.x
Tsuda, A., Henry, F. S. S., and Butler, J. P. P. (2013). Particle transport and deposition: basic physics of particle kinetics. Compr. Physiol. 3 (4), 1437–1471. doi:10.1002/cphy.c100085
Vaaraslahti, K., Laitinen, A., and Keskinen, J. (2002). Spray charging of droplets in a wet scrubber. J. Air & Waste Manag. Assoc. 52 (2), 175–180. doi:10.1080/10473289.2002.10470776
Velkov, T., Abdul Rahim, N., Zhou, Q. T., Chan, H. K., and Li, J. (2015). Inhaled anti-infective chemotherapy for respiratory tract infections: successes, challenges and the road ahead. Adv. Drug Deliv. Rev. 85, 65–82. doi:10.1016/j.addr.2014.11.004
Waterer, G. W., Wildhaber, J. H., McWilliams, A., and Summers, Q. A. (1998). Reducing electrostatic charge in spacer devices improves bronchodilator response. Chest 114 (4 Suppl. L), 277–280. doi:10.1046/j.1365-2125.2000.00251.x
Wilson, I. B. (1947). The deposition of charged particles in tubes, with reference to the retention of therapeutic aerosols in the human lung. J. Colloid Sci. 2 (2), 271–276. doi:10.1016/0095-8522(47)90028-7
Xi, J., Si, X., and Longest, W. (2014). Electrostatic charge effects on pharmaceutical aerosol deposition in human nasal-laryngeal airways. Pharmaceutics 6 (1), 26–35. doi:10.3390/pharmaceutics6010026
Yatsuzuka, K., Higashiyama, Y., and Asano, K. (1996). Electrification of polymer surface caused by sliding ultrapure water. IEEE Trans. Industry Appl. 32 (4), 825–831. doi:10.1109/28.511638
Yatsuzuka, K., Mizuno, Y., and Asano, K. (1994). Electrification phenomena of pure water droplets dripping and sliding on a polymer surface. J. Electrost. 32 (2), 157–171. doi:10.1016/0304-3886(94)90005-1
Yeung, T., Gilbert, G. E., Shi, J., Silvius, J., Kapus, A., and Grinstein, S. (2008). Membrane phosphatidylserine regulates surface charge and protein localization. Science 319 (5860), 210–213. doi:10.1126/science.1152066
Young, P. M., Sung, A., Traini, D., Kwok, P., Chiou, H., and Chan, H. K. (2007). Influence of humidity on the electrostatic charge and aerosol performance of dry powder inhaler carrier based systems. Pharm. Res. 24 (5), 963–970. doi:10.1007/s11095-006-9218-8
Keywords: electrostatic forces, drug delivery, pulmonary administration, respiratory medicine, charged aerosols
Citation: Bessler R and Sznitman J (2024) The potential of leveraging electrostatics for improved inhaled drug delivery to the lungs. Front. Med. Eng. 1:1298251. doi: 10.3389/fmede.2023.1298251
Received: 21 September 2023; Accepted: 28 December 2023;
Published: 15 January 2024.
Edited by:
Chiara Tonda-Turo, Polytechnic University of Turin, ItalyReviewed by:
Tim Corcoran, University of Pittsburgh, United StatesCopyright © 2024 Bessler and Sznitman. This is an open-access article distributed under the terms of the Creative Commons Attribution License (CC BY). The use, distribution or reproduction in other forums is permitted, provided the original author(s) and the copyright owner(s) are credited and that the original publication in this journal is cited, in accordance with accepted academic practice. No use, distribution or reproduction is permitted which does not comply with these terms.
*Correspondence: Josué Sznitman, c3puaXRtYW5AYm0udGVjaG5pb24uYWMuaWw=