- 1Department of Anaesthesia and Intensive Care, Nordland Hospital Trust, Bodø, Norway
- 2Department of Clinical Medicine, Faculty of Health Sciences, UiT The Arctic University of Norway, Tromsø, Norway
- 3Research Laboratory, Nordland Hospital Trust, Bodø, Norway
- 4Brain Physics Laboratory, Department of Clinical Neurosciences, University of Cambridge, Cambridge, United Kingdom
- 5Faculty of Nursing and Health Sciences, Nord University, Bodø, Norway
- 6Faculty of Medicine, Institute of Clinical Medicine, University of Oslo, Oslo, Norway
- 7Faculté de médecine Lyon-Est, Université de Lyon, Lyon, France
- 8Department of Anaesthesia and Intensive Care, University Hospital of North Norway, Tromsø, Norway
Introduction: Positive end-expiratory pressure (PEEP) and prone positioning can improve gas exchange by promoting uniform lung aeration. However, elevated ventilation pressures may increase intracranial pressure (ICP) and disrupt cerebral autoregulation. This study investigated the effects of PEEP on ICP and cerebral autoregulation in a porcine model with healthy lungs and normal ICP, comparing prone and supine positions. Cerebral autoregulation was assessed through cerebrovascular reactivity using the pressure reactivity index (PRx). We also explored whether other baseline variables influenced potential variances in ICP and PRx.
Methodology: Twelve anesthetized pigs were randomized to begin in either supine or prone position, across PEEP of 5, 10, 15, and 20 cmH2O. Continuous monitoring included esophageal pressure to calculate end-inspiratory and end-expiratory transpulmonary pressures. The ICM+® software (University of Cambridge Enterprise, Cambridge, United Kingdom) was used for high-resolution data collection, signal processing and ICP curve analysis. Linear mixed-effects models and ANOVA were used to analyze changes in ICP and PRx and the influence of position. An exploratory correlation analysis was conducted on baseline variables potentially related to the ICP increase.
Results: Mean ICP increase was 1.0 mmHg ± 0.9 at 10 cmH2O PEEP, 2.0 mmHg ± 1.7 at 15 cmH2O PEEP, and 3.1 mmHg ± 1.6 at 20 cmH2O PEEP compared to a baseline PEEP of 5 cmH2O (p < 0.001). The effect of PEEP increase on ICP was not influenced by body position. PRx remained unaffected by PEEP. PEEP-induced increases in ICP were higher in cases of higher baseline ICP, higher central venous pressure, lower respiratory system elastance and lower end-inspiratory and end-expiratory transpulmonary pressures.
Conclusion: Increasing PEEP elevates ICP regardless of body position without adversely affecting cerebral autoregulation in a healthy porcine model. Baseline ICP, central venous pressure, respiratory system elastance and end-inspiratory and end-expiratory transpulmonary pressure may influence the magnitude of ICP changes.
1 Introduction
Invasive positive pressure mechanical ventilation (IPPMV) is a life-saving procedure in patients with acute respiratory failure or severe brain injury, as long as the negative consequences that its settings can entail are minimized (1). To prevent lung injury, lung protective ventilation (LPV) aims to preserve the lung parenchyma from overdistension by reducing tidal volume (VT) and promote homogenisation of aerated lung mass by a judicious level of positive end-expiratory pressure (PEEP) and prone positioning (2–5).
End-inspiratory transpulmonary pressure (TPPei) represents the stress imposed on the lung parenchyma and is generated by PEEP levels and VT (6). Although the effects of IPPMV on the heart-lung interaction have been extensively studied, the complex interplay with other key organs should also be considered to ensure overall safety (7). Among these, the brain is of paramount importance as IPPMV can have deleterious effects on cerebral perfusion, leading to a delay in full recovery in the short-term and neurological sequalae in survivors of brain injury in the long term (8). Although the impact of IPPMV on cerebral perfusion has been studied to some extent, little attention has been paid to the specific effects of PEEP combined with prone position on intracranial pressure (ICP) and cerebral autoregulation (CA) (9). CA has been suggested as a parameter that may guide cerebral perfusion pressure (CPP) targets (10). New tools have emerged to study CA, which represents a crucial defence mechanism for maintaining cerebral blood perfusion, in case of acute changes in perfusion pressure, oxygen supply, carbon dioxide, venous return, or cardiac output. A key method for identifying disturbances in CA is the pressure reactivity index (PRx), which uses the moving correlation coefficient between slow changes in arterial blood pressure (ABP) and ICP (11–13). Negative PRx values signify preserved autoregulation, whereas positive values indicate impairment (14). In addition to PRx, the compensatory reserve index (RAP) is used to evaluate intracranial compliance, where low values indicate sufficient compensatory reserve, and values approaching one reflect reduced compliance (15). PRx has been validated in porcine models (16), but the correlation between PRx, PEEP and body position changes has not been studied previously. Consequently, with the aim of investigating how changes in PEEP in the prone and supine positions affect ICP and PRx in a porcine model with healthy lungs and normal ICP, the present study is the first step in a comprehensive investigation of the lung-brain cross talk in normal as well as diseased condition.
2 Materials and methods
Following the approval of The Norwegian Animal Research Authority (FOTS ID 27107), we conducted a prospective, randomized, controlled animal study. The study was conducted in strict compliance with the Norwegian Laboratory Animal Regulations and the EU Directive 2010/64/EU to ensure the humane treatment of animals. We confirm the use of the ARRIVE guidelines checklist for the reporting of animal research, as appropriate.
2.1 Animal preparation
Fifteen Norwegian domestic landrace pigs (Sus scrofa domesticus) were studied. Twelve pigs, comprising eleven males and one female, were included in the study, with an average weight of 25 kg (range 23–28 kg). Two were excluded due to infection and hypotension, and one was excluded due to technical difficulties with ICP monitoring. The animals were retrieved from a local farm. Immediately after being removed from the pen, they were sedated with an intramuscular injection of 20 mg/kg ketamine, 0.5 mg/kg midazolam, and 1 mg atropine. The animals were directly transported to the animal research facility (ANILAB) at the North University of Bodø. Transportation time was approximately 10 min.
On arrival, they were identified by unique identifiers (e.g., sgb307, sgb311). Bilateral auricular veins were cannulated. Anesthesia was induced with an intravenous infusion comprising morphine (2 mg/kg/h), midazolam (0.15 mg/kg/h), and pentobarbital (4 mg/kg/h). The animals, positioned prone, were intubated with a Portex ID 6.5 to 7.0-mm-inner diameter endotracheal tube from Smiths Medical International Ltd. (Kent, United Kingdom). All animals were regularly checked for the pedal withdrawal reflex, palpebral reflex, and jaw tone, following standard protocol, to ensure that the depth of anesthesia was always adequate.
Mechanical ventilation was initiated using a Datex-Ohmeda Engström Carestation intensive care ventilator (GE Healthcare, Madison, WI) set to a volume-control mode with a VT of 9 mL/kg, fraction of oxygen in air (FiO2) of 0.40, PEEP of 5 cmH2O, inspiratory-to-expiratory time ratio 1:2, inspiratory pause 20%, keeping the respiratory rate between 20–30 breaths/min to maintain adequate ventilation with PaCo2 levels within the range of 35–45 mmHg.
A FluxMed esophageal catheter (MBMED, Martinez, Argentina) was placed and connected to the ventilator to monitor the esophageal pressure (Pes). The correct placement of the probe into the esophagus was ascertained by a ∆Pes/∆Paw ratio close to unity (0.8–1.2) where DPes and DPaw are the change in Pes and airway pressure (Paw), respectively during an expiratory hold (17).
After placing the animals in the supine position, we inserted a MAC™ two-lumen central venous catheter (CVC) with an integrated hemostasis valve (ARROW, Morrisville, United States) via ultrasound guidance into the right external jugular vein. Through this valve, we advanced a 7.5 Fr. Swan-Ganz pulmonary artery catheter (Edwards Lifesciences Corporation, Irvine, CA). Using a sterile cut-down technique, a 4 Fr., 8 cm PiCCO thermodilution catheter (Pulsion/Getinge, Gothenburg, Sweden) was placed in the left femoral artery. These catheters were connected to dedicated pressure transducers and a thermodilution cardiac output monitoring system (Edwards Lifesciences Corporation), interfaced with the Intellivue MP70 Monitor (Philips Healthcare, Cambridge, CA).
To ensure euvolemia, an intravenous fluid bolus of 500 mL of Ringer acetate (Fresenius Kabi, Oberdorf, Switzerland) was administered at a rate of 30 mL/kg/h, followed by a 5 mL/kg/h continuous infusion throughout the study.
A suprapubic catheter was placed via cystotomy for urinary drainage.
2.2 Cerebral monitoring
Subsequent instrumentation in the prone position included the insertion of a Codman MicroSensor ICP Transducer (Medos International SÀRL, Le Locle, Switzerland) into the left frontal hemisphere through a burr hole. The transducer was connected to a Codman ICP Express monitor (Codman & Shurtleff, MA, United States) for real-time ICP monitoring.
2.3 Physiological monitoring and data acquisition
The MP70 Monitor continuously monitored electrocardiography, oxygen saturation via plethysmography (SpO2), end-tidal carbon dioxide (EtCO2), and body temperature. Mean invasive arterial (ABP), central venous (CVP), and pulmonary artery blood pressures (PAP), as well as cardiac output (CO), were continuously measured. Extravascular lung water (EVLW) and stroke volume variation (SVV) were measured intermittently by thermodilution according to the PICCO manufacturer’s guidelines. The ICP signal from the Codman monitor was integrated into the MP70. Respiratory variables were collected by the Engström Carestation ventilator (see below).
The Intensive care monitoring software (ICM+®, University of Cambridge Enterprise, Cambridge, United Kingdom) was used for signal acquisition, waveform analysis, and data summaries. High-resolution data from the MP70 and the ventilator were streamed in real-time to a laptop running the software, providing integration and synchronization of cerebral, respiratory, and hemodynamic variables, as shown in Figure 1.
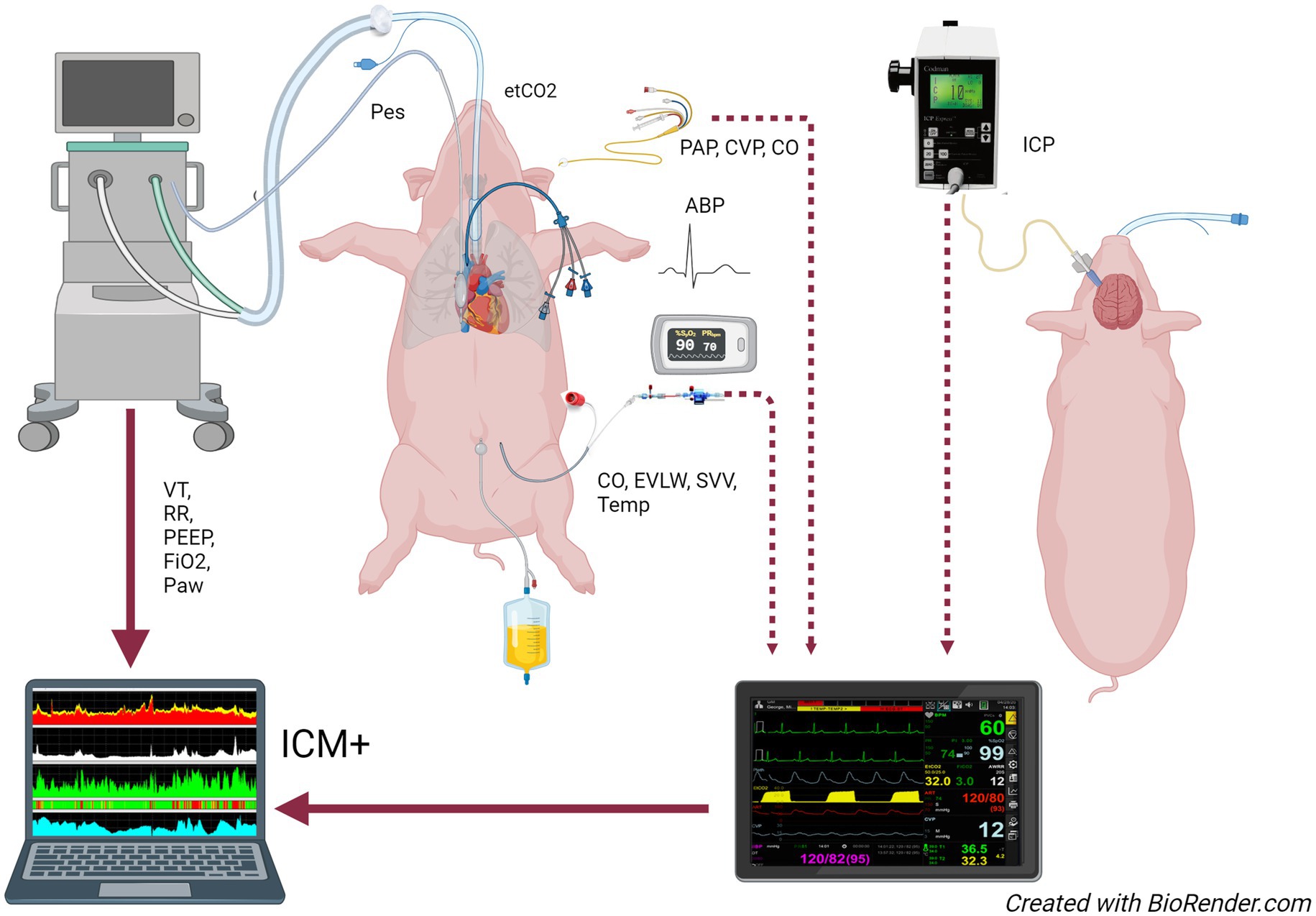
Figure 1. Study setup. The multiparametric monitor and the ventilator are connected to a computer running ICM+ software for data acquisition. ABP, arterial blood pressure; CO, cardiac output; CVP, central venous pressure; etCO2, end-tidal partial pressure of CO2; EVLW, extravascular lung water; FiO2, fraction of inspired oxygen; ICP, intracranial pressure; PAP, pulmonary artery pressure; Paw, airway pressure; Pes, esophageal pressure; PEEP, positive end-expiratory pressure; RR, respiratory rate; SVV, stroke volume variation; Temp, temperature; VT, tidal volume.
Arterial blood was drawn at each PEEP level throughout the experiment to measure pH, partial pressure of oxygen (PaO2), and carbon dioxide (PaCO2).
2.4 Study procedure
Details of the study design are illustrated in Figure 2.
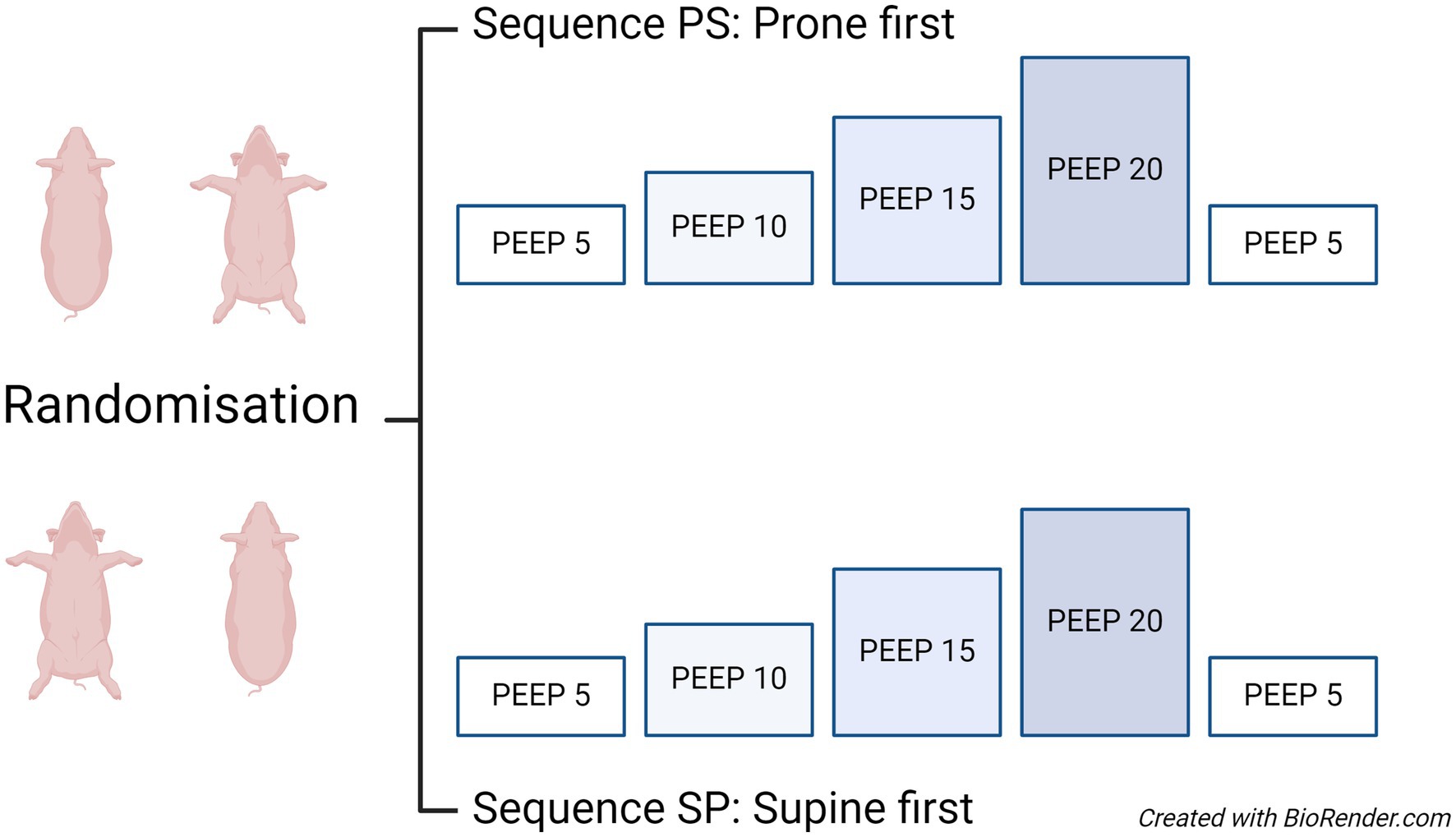
Figure 2. Study design: crossover design and intervention settings. The randomization scheme is shown to the left, and the periods with PEEP levels are shown to the right. Subsequent measurements were conducted at PEEP levels of 10, 15, and 20 cmH₂O, aiming to ventilate the pigs with a tidal volume of 7–8 mL/kg body weight during the PEEP trial. The prone-supine group was first exposed to the PEEP trial in the prone position, followed by the PEEP trial in the supine position. Conversely, the supine-prone group underwent the same interventions, first in the supine position and then turning to the prone position. A washout period with baseline ventilator settings was used to reduce the risk of a carry-over effect from the intervention. PEEP, positive end-expiratory pressure; PS, prone supine; SP, supine prone.
Personnel not involved in the experimental procedures conducted the randomization prior to the pigs’ arrival at the laboratory. The randomization, performed by drawing lots, determined whether each pig would start in the prone or the supine position. According to this plan, the pigs were initially placed in their assigned positions at zero degrees inclination.
Systematic measurements were conducted at increasing PEEP levels of 5 (baseline), 10, 15, and 20 cmH₂O, then back to 5 cmH₂O, where PEEP 5 cmH2O is the baseline. Each PEEP level was maintained for 20 min. Subsequently, the pigs were repositioned to the alternate posture as per the randomization plan and the PEEP trial performed again. The ventilator settings used during the PEEP trial were volume control mode, VT of 7–8 mL/kg body weight, FiO₂ of 0.40, inspiratory: expiratory ratio of 1:2, and an inspiratory pause of 20% of the inspiratory time., and the mean values from these 20 min of measurements were used in the analysis. Additionally, respiratory mechanics was assessed by end-inspiratory and end-expiratory holds towards the end of the measurement period to ensure stable values, and followed by thermodilution measurements. Subsequently, the pigs were repositioned to the opposing posture as per the randomization plan.
2.5 Outcome measures
The primary outcomes were ICP and PRx. We investigated the effect of PEEP level increase on mean ICP and mean PRx and looked at the effect of position on the relationship between PEEP and ICP and Prx.
The secondary outcome was respiratory, cerebral, and hemodynamic variables related to the change in mean ICP, including baseline ICP, RAP, PaO2, PaCO2, VT, respiratory rate (RR), end-inspiratory airway pressure (Pawei), elastance of the respiratory system (Ers), end-expiratory transpulmonary pressure (TPPee), TPPei, lung elastance (El), mechanical power of the respiratory system (MPrs), CVP, PAP and CO.
2.6 Data processing
ICM+ software was used for data preprocessing of the high-resolution recordings before statistical analysis. Two researchers (RH and SF) scrutinized each recording visually and assessed the quality of the signals. The data were first curated manually for significant artifacts. Further, automated artifact markup was applied in ICM+. ICP values below −10 mmHg or above 60 mmHg were rejected, as were the cerebral perfusion pressure (CPP) values below 0 mmHg or above 150 mmHg. All recorded signals were downsampled to 0.1 Hz by coarse graining using 10 s, non-overlapping averages.
The PRx index was calculated using a 5-min moving window of Pearson correlation coefficients between 30 consecutive 10-s averages of ABP and ICP, updated every minute. Similarly, the compensatory reserve index (RAP) was calculated using a 5-min moving window of Pearson correlation coefficients between 30 consecutive 10-s averages of intracranial pulse pressure amplitude (AMP) and ICP, also updated every minute (18). Fisher transformation was applied to PRx and RAP before further analysis. We exported descriptive statistics values of each variable for each animal for each study period, using the event tool implementation in ICM+. Mean values were considered for all variables for further analysis.
2.7 Respiratory mechanics variables
Data from the ventilator was extracted from ICM+ software as one value per period.
The following variables were retrieved as absolute values:
End-inspiratory (Pawei) and total end-expiratory airway pressure (PEEPtot), end-inspiratory and end-expiratory esophageal pressures (Pesei and Pesee respectively) were recorded at zero flow after a 3 s-end-inspiratory and a 3-s end-expiratory hold. Peak pressure of the respiratory system (Ppeakrs) and chest wall (Ppeakcw) were the maximal values of Paw and Pes, respectively. Respiratory rate (RR) and VT were also collected.
The following variables were calculated:
1. Peak pressure across the lung (Ppeakl) = Ppeakrs − Ppeakcw.
2. Respiratory system (Ers), chest wall’s (Ecw), and lung’s (El) elastance was calculated according to the classic formula: Ers = (Pawei − PEEPtot)/VT, Ecw = Pesei − Pesee/VT, and El = Ers − Ecw.
3. Absolute end-expiratory TPP (TPPee) was computed by subtracting Pesee to PEEPtot. Absolute end-inspiratory TPPei is calculated was calculated as the difference between Pawei and Pesei. It was suggested that absolute TPPei reflect lung stress in the most dependent part of the lungs (19). Lung stress was therefore also computed from the TPP elastance (TPPelast) method TPPelast = Pawei × (El/Ers). TPPelast was thought of as reflecting the lung stress in the non-dependent parts of the lungs.
4. MPrs in J/min was calculated with the formula: MPrs = 0.098*VT*RR*(Ppeakrs − (Pawei − Pawee)/2). Mechanical power of the dependent lung (MPlDep) = 0.098*VT*RR*(Ppeakl − (TPPei − TPPee)/2). Mechanical power of the non-dependent lung (MPlnonDep) = 0.098*VT*RR*(Ppeaklung − (TPPelast − TPPee)/2).
2.8 Statistical analysis
The following complete set of physiological variables were considered in the analysis:
1. Cerebral and hemodynamic variables: ICP, PRx, RAP, AMP, ABP, CPP, CVP, PAP, CO, temperature.
2. Gas exchange and respiratory variables: SpO2, PaCO2, PaO2, pH, PaO2/FiO2-ratio, RR, Pawei, Pesei, Pesee, TPPei, TPPee, TPPelast, Ers, Ecw, El, MPrs, MPlDep, MPlnonDep, EVLW, SVV, PEEP, VT, Ppeakrs, Ppeakcw, and Ppeakl.
The normality of continuous variables was assessed with the Shapiro–Wilks test. The homogeneity of variance was also evaluated. Continuous variables were presented as mean and standard deviation (SD) or median and interquartile range (IQR). Outlier analysis was conducted using graphical methods, revealing no outliers in the data after scrutinization during the data preprocessing.
2.9 Statistical analysis strategy
The assumptions of the crossover design (absence of carry-over effect, absence of sequence (prone or supine first) effect, and period of intervention) were tested for change in ICP and PRx with linear mixed effect models using type of intervention (PEEP increase), sequence, and period of intervention (first or second) as a fixed effect, with interaction terms, and the animal and position as random effect variables. Furthermore, the absence of a carry-over effect was tested with a paired t-test (two-tailed) on baseline or washout periods for ICP and PRx.
2.9.1 Primary endpoints
We examined whether different levels of PEEP influence ICP and PRx in prone and supine position.
• The difference between baseline variables in prone and supine positions was tested using two-tailed t-test or the Mann–Whitney test, depending on the data distribution.
• The treatment effects were evaluated by comparing values during the intervention periods (PEEP 10, 15, 20 cmH2O) to the baseline period (PEEP 5 cmH2O).
• After checking for assumptions, two-way ANOVA was performed to see if there was a significant change in ICP between prone and supine across the different PEEP levels.
• If the linear mixed-effects model assumption confirmed that the response in mean ICP and PRx to the intervention (different PEEP levels) did not differ between the positions, the mean changes from baseline to intervention across supine and prone positions were pooled and analyzed through a repeated measure one-way ANOVA.
2.9.2 Secondary endpoints
Using scatterplots and linear mixed models, we investigated the relationships between the changes in ICP induced by the intervention and various baseline neurological, hemodynamic, and respiratory variables. For this analysis, the prone and supine data were pooled since the baseline variables apart from ICP and RAP, were not different between the groups. The covariables were empirically chosen based on clinical relevance to the mechanisms by which PEEP might affect ICP, and not all recorded variables were explored.
We tested the following baseline variables: ICP, RAP, PaO2, PaCO2, VT, RR, Pawei, TPPei, TPPee, Ers, El, MPrs, CVP, PAP, CO.
ICP was included due to prior evidence suggesting that patients with higher baseline ICP may exhibit a different response to PEEP (20). RAP, as a crude index of brain compliance, was considered relevant as reduced compliance might amplify ICP changes in response to increased PEEP.
PaO₂ and PaCO₂ were included due to their impact on cerebral oxygenation and vasoreactivity, with PaCO₂ being a potent regulator of cerebral blood flow, which in turn affects ICP. Pawei, TPPei, TPPee, Ers, El were included because they may affect how thoracic pressures are transmitted to the brain. Similarly, CVP and PAP were examined due to their role in venous return and pulmonary hemodynamics, which can influence cerebral venous drainage and ICP. MPrs, VT and RR was included to evaluate the total mechanical energy transmitted to the lungs, which could impact PEEP’s effects on the brain. Lastly, CO was considered due to its potential influence on cerebral blood flow, as PEEP may reduce venous return and cardiac output. A p-value <0.05 was considered statistically significant. Since we only analyzed clinically relevant variables and did not examine all recorded variables, multiple comparison corrections were not applied.
The statistical analysis was performed with R software version 4.3 (21).
3 Results
3.1 Baseline characteristics
Table 1 presents variables measured during baseline PEEP 5 cmH20 in prone or supine positions before the stepwise increase in PEEP. Mean ICP at baseline was higher in prone (P5) than supine (S5) (p < 0.01). RAP differed at baseline between prone and supine (p < 0.01). No other variables were different between prone and supine before randomization.
Tables with respiratory and hemodynamic variables at different PEEP levels in supine and prone are presented in the Supplementary Tables 2A,B.
3.2 Effects of PEEP on ICP and PRx
Figure 3 shows each animal’s mean ICP during each PEEP level, in prone and supine.
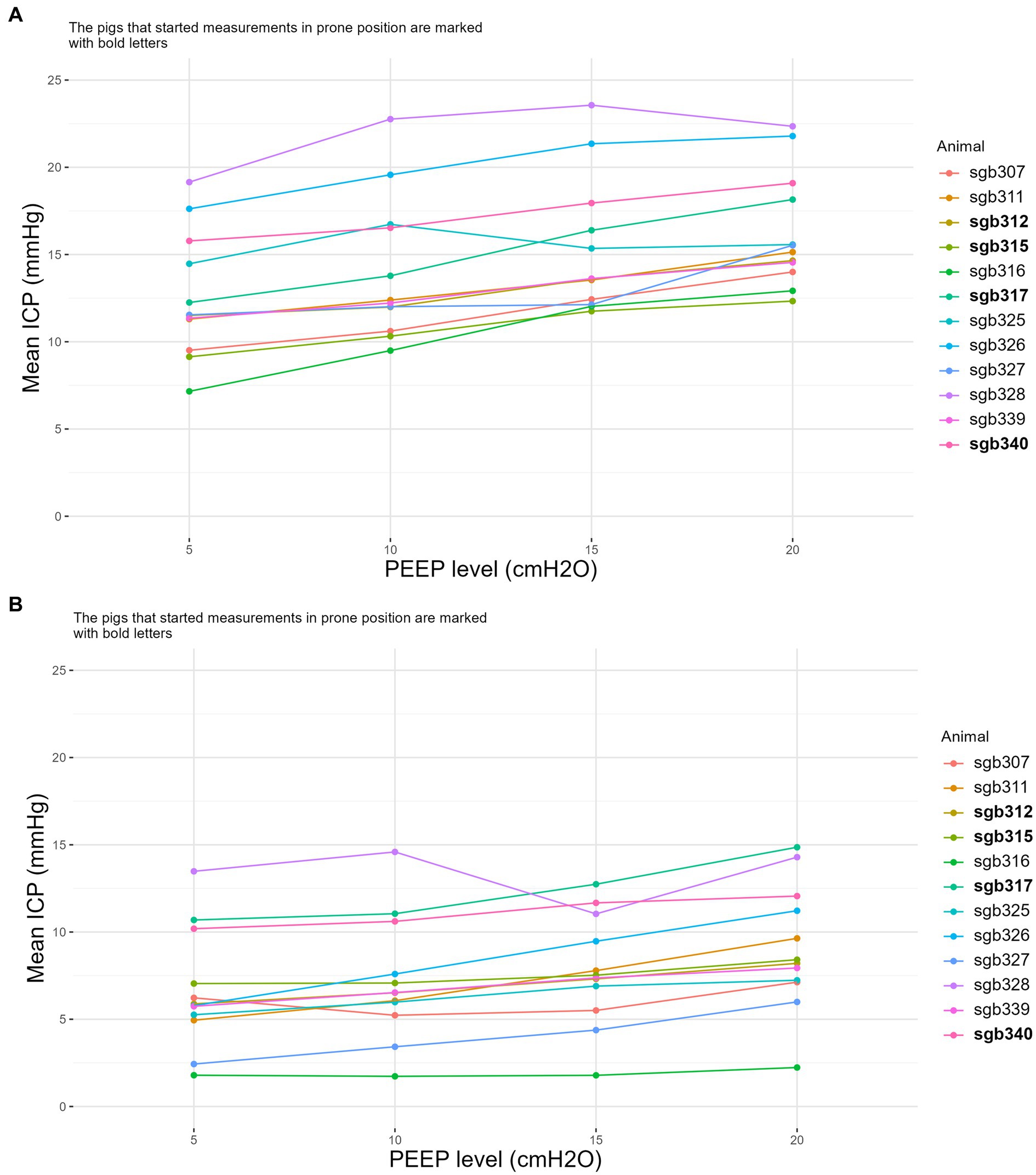
Figure 3. The effect of PEEP and animal position on ICP. Mean intracranial pressure (ICP) in the supine position (A) and in the prone position (B) with PEEP at 5, 10, 15 and 20 cmH2O. Each line represents an individual animal, identified by their unique identifiers (e.g., sgb307, sgb311). The identifiers in bold are the animals that started supine first. ICP, intracranial pressure; PEEP, positive end-expiratory pressure.
The study design assumptions were fulfilled for the analysis of the primary outcome. Change in ICP and PRx had no carry-over, sequence, or period effect.
There was a significant association between PEEP levels and change in mean ICP (p < 0.001) (Figure 4, supine and prone are pooled). Two-way ANOVA showed no interaction between PEEP levels and position (p = 0.66), i.e., the effect of increased PEEP on the absolute rise of ICP was not influenced by position. Position affected change in ICP, regardless of PEEP (p < 0.001) (Supplementary Figures A1, A2). The average ICP changes observed at PEEP settings of 10, 15, and 20 cmH2O, compared to a baseline of 5 cmH2O, were 0.6 mmHg ± 3.5, 1.2 mmHg ± 3.1 in prone, and 2.5 mmHg ± 3.6, and 1.5 mmHg ± 4.1, 2.7 mmHg ± 3.9, and 3.8 mmHg ± 3.3, in supine.
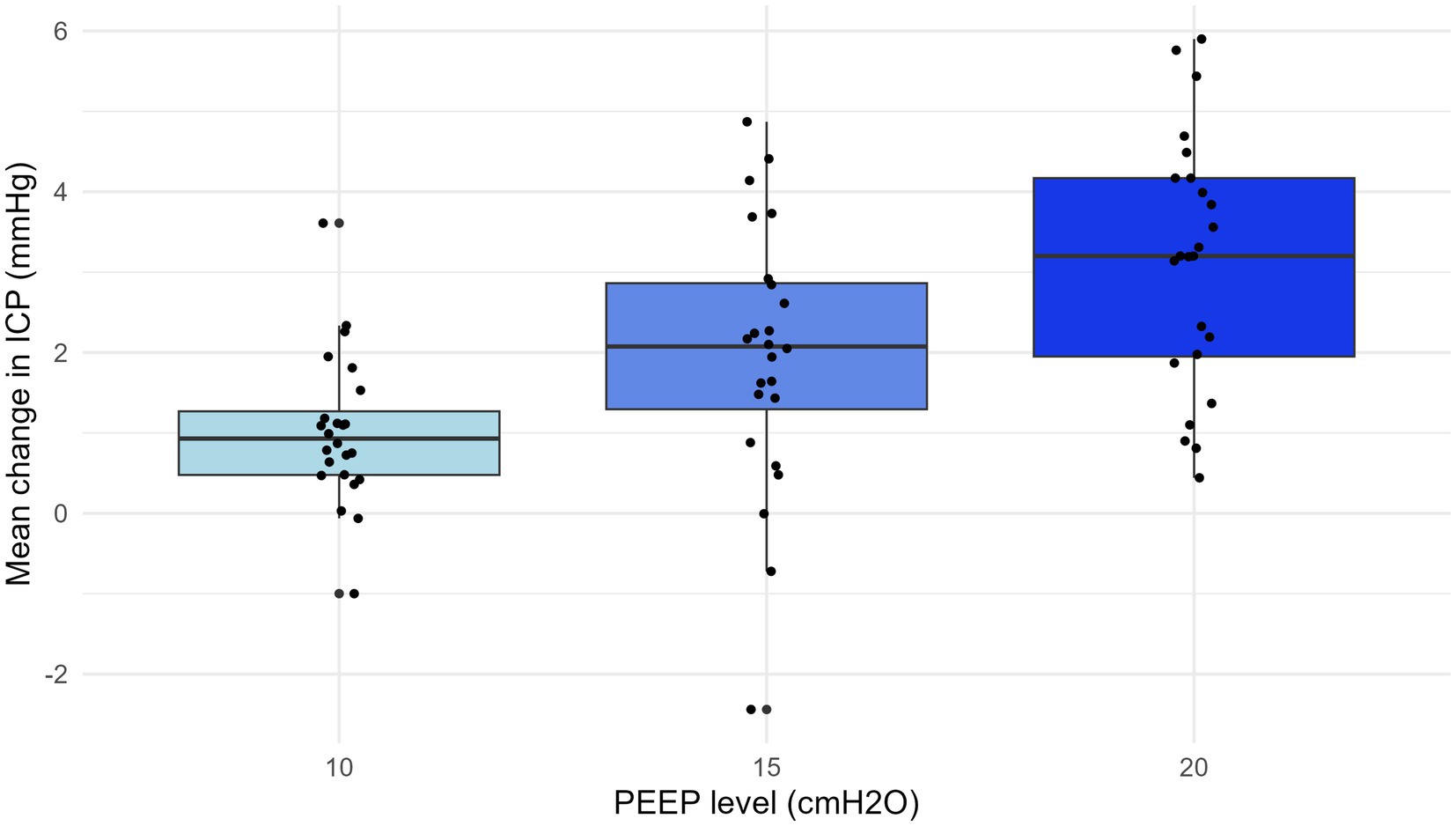
Figure 4. Mean change in ICP with PEEP at 10, 15, and 20 cmH2O. The change in intracranial pressure (ICP) was calculated as the increase from the baseline at positive end-expiratory pressure (PEEP) 5 cmH2O. There was a significant association between PEEP levels and change in mean ICP (r = 0.25, p < 0.001). The prone and supine data are pooled. ICP, intracranial pressure; PEEP, positive end-expiratory pressure.
The PEEP increase did not significantly change mean PRx compared to the baseline value (Figure 5, supine and prone are pooled). The average PRx changes observed at PEEP settings of 10, 15, and 20 cm H2O, compared to a baseline of 5 cmH2O, were 0.1 ± 0.16, 0.0 ± 0.17, and − 0.1 ± 0.28 in prone, and 0.1 ± 0.24, 0.1 ± 0.21, and 0.1 ± 0.24 in supine (Supplementary Figure A3).
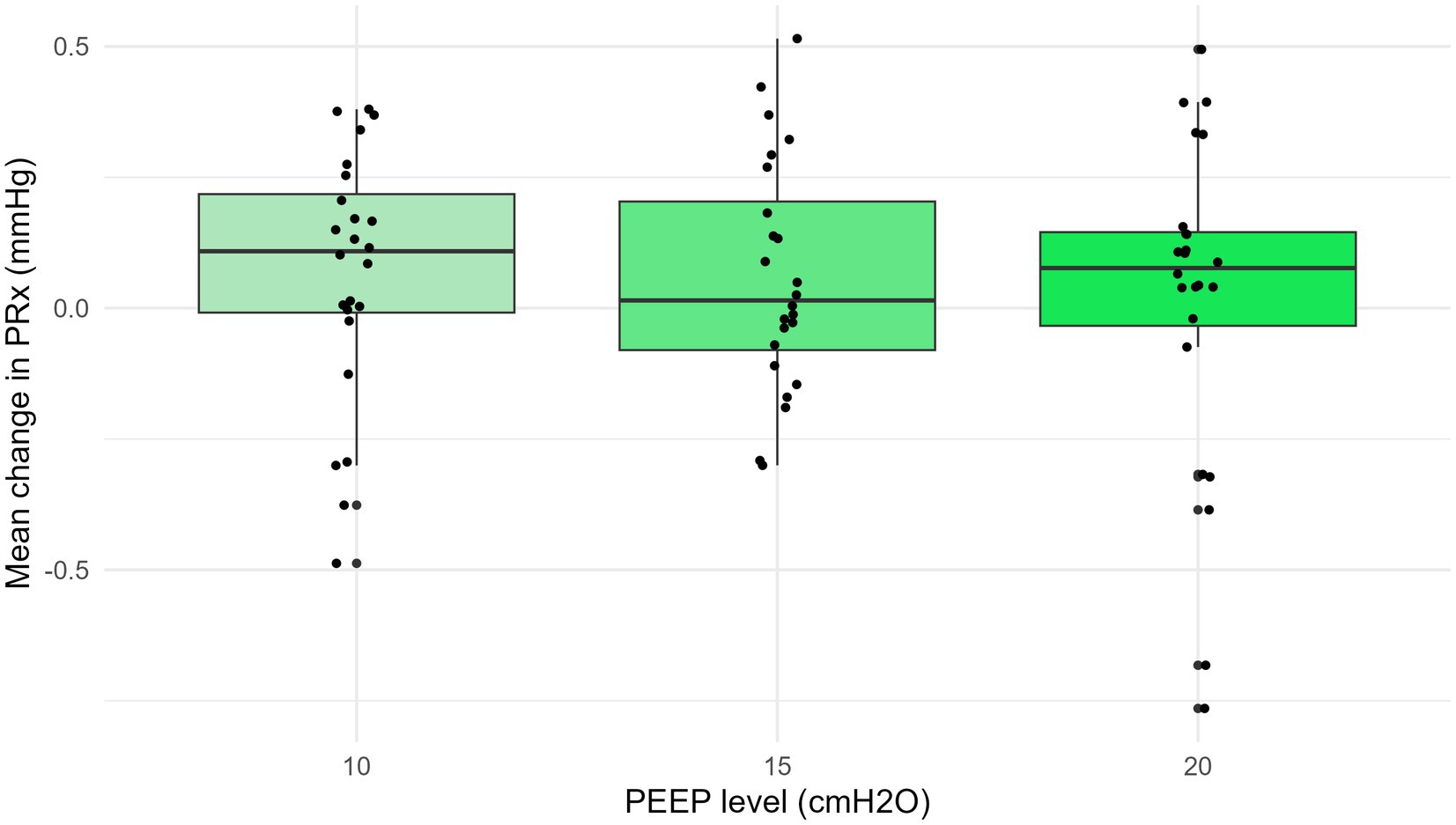
Figure 5. Mean change in PRx with PEEP at 10, 15, and 20 cmH2O. The increase in PEEP did not significantly change the mean pressure reactivity index (PRx) compared to the baseline values (p = 0.7). ICP, intracranial pressure; PEEP, positive end-expiratory pressure; PRx, pressure reactivity index.
3.3 Exploratory analysis
Exploratory analysis looked at baseline variables that could be correlated to changes in ICP. We found that PEEP-induced increases in ICP was higher in cases of lower Ers (p = 0.024, r = −0.21), lower TPPee (p = 0.009, r = −0.20), and lower TPPei (p = 0.006, r = −0.34). In addition, higher CVP (p = 0.0032, r = 0.30) and higher baseline ICP (p = 0.012, r = 0.20) were associated with greater changes in ICP.
RAP, PaO2, PaCO2, VT, RR, Pawei, El, MPrs, PAP, and CO were not significantly correlated with ICP. The correlation plots for the significant correlations are presented in Supplementary Figures B1–B5.
4 Discussion
Our main findings in this experimental porcine model are: (1) increased PEEP levels significantly increase ICP, regardless of position. (2) Both PEEP and position independently affect ICP. (3) PRx remains unaffected by changes in PEEP in supine and prone positions. (4) Baseline ICP, CVP, TPPei, TPPee and Ers are correlated with an elevated ICP response.
4.1 Effect of PEEP on ICP
Our study shows a relatively modest rise in ICP, even with PEEP settings as high as 20 cmH2O. An increase in ICP by 1 mmHg with a 5 cmH2O increase in PEEP appears clinically insignificant.
Other animal studies confirm increases in ICP with elevated PEEP levels. Huseby et al. (22) examined dogs with healthy lungs and normal ICP and reported a mean increase in ICP in dogs of 10 mmHg when PEEP was increased from 0 to 20 cmH₂O. While PaCO₂ was maintained at stable levels in this study, blood pressure was generally allowed to fluctuate without intervention which might have influenced the observed changes in ICP that are greater than ours. Aidinis et al. (23) observed a small, clinically insignificant increase in ICP in cats with intracranial hypertension and healthy lungs when increasing PEEP to 15 cmH₂O.
Sun et al. (24) demonstrated that elevated PEEP increased ICP in dogs with healthy lungs and normal ICP. The mean increase was, similar to our findings, only 5 mmHg with a PEEP increase from 0 to 20 cmH2O. A recent systematic review on the effect of PEEP on ICP (25) states that PEEP can increase ICP, but the extent of this increase varies across different studies and patient populations. Beqiri et al. (20) found that while most patients with ABI and healthy lungs showed a significant but clinically non-relevant increase in ICP, nearly one-quarter of the patients experienced a rise in ICP during the intervention to above 20 mmHg, necessitating its interruption. The effect of PEEP on ICP have also been tested perioperatively during neurosurgery. Ruggieri et al. (26) found no difference in ICP when applying LPV (7 mL/kg and PEEP 5 cmH₂O) and traditional ventilation (9 mL/kg and PEEP 0 cmH₂O) in patients undergoing surgery for brain tumour. Both animal and human studies align with our results, indicating that PEEP can increase ICP. However, the clinical significance of these increases varies widely. This variability underscores the need for careful, individualized management of increasing PEEP.
4.2 Effect of prone versus supine position on ICP
The baseline ICP and RAP index were significantly higher in the supine position compared to the prone position, suggesting slightly reduced cerebral compliance in the supine posture. In humans, ICP is also dependent on position. While human studies typically show higher ICP in the prone position (27, 28), our findings in pigs indicate the opposite, likely due to anatomical differences between species. In pigs, the brain is smaller and positioned more ventrally and caudally, influencing ICP measurements based on the head’s orientation. Specifically, in the prone position, the frontal cerebral parenchyma is located anteriorly, whereas in the supine position, it shifts posteriorly. The placement of the ICP probe in the frontal cerebral parenchyma likely results in increased gravitational pressure when turning from prone to supine, resulting in higher ICP readings. The positional dependence of ICP has been confirmed by other porcine studies (29, 30), though exploring these mechanisms was beyond the scope of our study.
Baseline ICP measurements in the supine position vary across animal studies. For example, studies with healthy pigs have reported ICP values consistent with our findings (29, 31) while others report lower values (32). Our study’s baseline ICP in the prone position is consistent with findings from a study in healthy pigs (33), but to our knowledge, no other studies have compared ICP between prone and supine positions within the same animal.
We found that the absolute mean changes in ICP were significantly greater in the supine position, regardless of PEEP levels, likely due to the higher baseline ICP in this posture. Several human studies indicate increased ICP in the prone position compared to the supine position, independent of PEEP level (28, 34). However, Thelandersson et al. (35) found no increase in ICP in the prone position in patients with reduced intracranial compliance, suggesting variability based on specific patient conditions.
4.3 Effect of PEEP on PRx
While the offset of ICP increased following the increase in PEEP, as indicated by the rise in the mean ICP value, the cerebral arterioles maintained their ability to constrict and dilate in response to changes in perfusion pressure, as reflected by the unchanged PRx. Previous animal studies on PRx have used hyper-/hypotension or ABP oscillations to investigate and validate the use of PRx (16, 36–38). To our knowledge, our study is the first to explore the relationships between PEEP increase and intracranial waveform analysis/autoregulation in a porcine model.
Two recent human studies showed no worsening of PRx with PEEP increase (20, 39). This aligns with our findings. Our findings indicate that PEEP adjustments do not significantly impact PRx, suggesting that cerebrovascular autoregulation remains stable under varying PEEP conditions. This stability implies that cerebral autoregulation mechanisms can withstand ventilatory changes without compromising cerebrovascular integrity.
A recent review (40) highlights the importance of PRx in managing traumatic brain injury, emphasizing how intracranial waveform analysis can improve patient outcomes. Given the scarcity of studies examining PRx in healthy brains, our research holds clinical significance. Understanding the response of a healthy brain to increased PEEP can provide valuable insights into the mechanisms affecting the brain in various pathological conditions where cerebral autoregulation is critical.
4.4 Baseline variables correlated to change in ICP
Our study found a significant positive correlation between changes in mean ICP induced by PEEP and position with baseline values of ICP and CVP, and a negative correlation with Ers and TPPei and TPPee.
The positive correlation between baseline ICP and the ICP response to PEEP changes confirms findings from our group’s previous study conducted on ABI patients (20). However, unlike in the clinical study, the RAP index did not differ between baseline and intervention phases, leaving the role of brain compliance uncertain. These results suggest that patients with elevated baseline ICP are at a higher risk of significant ICP increases when PEEP is adjusted. Therefore, close monitoring and cautious titration of PEEP are warranted.
Higher baseline CVP was associated with increased ICP, possibly due to reduced cerebral venous drainage. Chen et al. (41) also reported a correlation between CVP and increased ICP in animals with normal ICP, though they did not explore the correlation with baseline CVP. This finding suggests that in vulnerable patients, where avoiding ICP increases is critical, monitoring CVP during PEEP adjustments could be beneficial to identify those at higher risk of impaired cerebral venous outflow and subsequent ICP elevation.
Our study observed that the effect of PEEP on ICP was smaller in animals with higher baseline Ers. Contrarily, Chen et al. reported increased ICP in response to PEEP in an experimental pig study when elastance was increased with chest wall strapping. The Ers with chest wall strapping was much lower than in normal lungs, which might explain the difference from our study. They did not report the correlation between ICP increase and baseline Ers in normal lungs. In an observation study on patients, Robba et al. (42) found no correlation between baseline respiratory system compliance and ICP increase with PEEP. Similarly, a clinical intervention study did not find associations between baseline respiratory compliance and the responses of ICP to PEEP (20). Our finding suggests that patients with reduced respiratory compliance may tolerate higher levels of PEEP without significant increases in ICP. However, as previous studies have reported conflicting results, further research is needed to clarify the interaction between respiratory mechanics and cerebral hemodynamics.
Our results suggest that higher baseline TPPei and TPPee predicts a lower ICP response to PEEP. In this study, higher baseline TPPei and TPPee was attributed to variations in intrathoracic pressure (measured as Peso) or compliance, as PEEP and volume were set. To our knowledge, only one study reports the role of transpulmonary pressures on ICP (20). Baseline TPPei and TPPee in patients with ABI but no lung injury did not predict a prominent ICP increase.
4.5 Study strengths and limitations
Our study’s strengths include the use of a controlled experimental model, which allows for precise manipulation of variables and thorough monitoring. This is the first study with multimodal respiratory, hemodynamic and cerebral monitoring that examines the effects of PEEP in both prone and supine positions, providing baseline data for future studies involving pathological models.
Importantly, our study examined the physiological responses to PEEP in animals without ABI, and thus our findings may not reflect the responses in mechanically ventilated ABI patients. However, our findings provide much-needed baseline data for future studies in animals with raised ICP, disturbed cerebrovascular autoregulation, and/or damaged lungs.
Limitations include the use of an animal model, which may not fully replicate human physiology. The anatomical differences might result in baseline ICP values that may not correspond to humans in different positions. The small sample size limits the generalizability of our findings. The skewed randomization may also affect the reliability of our results. Animals were positioned at zero degrees without head elevation, which differs from typical clinical practice in humans. We wanted to minimize the risk of a PEEP effect on MAP, to have the most controlled settings possible for evaluating isolated ICP and PRx responses. Also in clinical practice, head elevation is reduced if it affects MAP and there is no ICP problem. Furthermore, PEEP levels were not randomized, and all animals were exposed to increasing PEEP in the same order, which could influence the results. We chose this approach because the main aim was to examine the ICP and PRx response with incremental PEEP in the prone and supine positions. This approach reflects typical clinical practice in which PEEP is gradually increased to monitor response.
As lung recruitment maneuvers were not performed between PEEP levels, this may potentially have affected the consistency of lung mechanics measurements. We chose not to perform a procedure that could affect ICP and PRx and would require extending the study with a longer time for stability between PEEP levels. Additionally, the short duration of the experiments did not allow for the assessment of the long-term effects of PEEP. A limitation is also that we did not have other cerebral monitoring than ICP.
5 Conclusion
To our knowledge, this study is the first on ICP changes in pigs comparing PEEP increase in prone and supine positions. Our findings suggest that increases in PEEP elevate ICP slightly, independently of position, without negatively impacting cerebral autoregulation (CA) in pigs with normal ICP and healthy lungs. We identified that the baseline variables ICP, CVP, Ers, TPPei and TPPee might predict elevations in ICP, but further investigation is warranted, especially targeting this issue as a primary objective.
Further exploration of the PEEP-ICP-CA relationship, particularly in more complex conditions such as ARDS and intracranial hypertension, is essential. This study provides a basis for such investigations.
Data availability statement
The raw data supporting the conclusions of this article will be made available by the authors, without undue reservation.
Ethics statement
The animal study was approved by Mattilsynet forsøksdyrforvaltningens tilsyns- og søknadssystem FOTS ID 27107. The study was conducted in accordance with the local legislation and institutional requirements.
Author contributions
RH: Data curation, Formal analysis, Funding acquisition, Investigation, Methodology, Visualization, Writing – original draft, Writing – review & editing, Software. EB: Data curation, Formal analysis, Investigation, Methodology, Software, Writing – review & editing, Conceptualization, Supervision, Validation. PS: Conceptualization, Data curation, Formal analysis, Investigation, Methodology, Resources, Software, Supervision, Validation, Writing – review & editing. BS: Writing – review & editing, Methodology, Supervision. EN: Funding acquisition, Resources, Supervision, Writing – review & editing. CG: Conceptualization, Investigation, Methodology, Supervision, Validation, Writing – review & editing, Formal analysis. SF: Conceptualization, Data curation, Formal analysis, Funding acquisition, Investigation, Methodology, Project administration, Resources, Supervision, Validation, Visualization, Writing – original draft, Writing – review & editing.
Funding
The author(s) declare that financial support was received for the research, authorship, and/or publication of this article. The project has received funding from Helse Nord (HNF1580-21) and Nordland Hospital for 2023 (11699) and 2024 (11723).
Acknowledgments
The authors would like to acknowledge Bent Aksel Nilsen for his assistance with the experiments, Renathe Henriksen Grønli for her help with blood sampling and handling and Ola-Jørgen Bøckmann Lie for assistance with ICM+ and ICP setup. The authors also would like to acknowledge the researchers at Brainphysics Lab, Cambridge University, Stefan Bogli, Marina Cherchi, and Ihsane Olakorede for valuable scientific input during the analysis.
Conflict of interest
Peter Smielewski receives part of the licensing fees for ICM+ software, licensed by Cambridge Enterprise Ltd., University of Cambridge, Cambridge.
The remaining authors declare that the research was conducted in the absence of any commercial or financial relationships that could be construed as a potential conflict of interest.
Publisher’s note
All claims expressed in this article are solely those of the authors and do not necessarily represent those of their affiliated organizations, or those of the publisher, the editors and the reviewers. Any product that may be evaluated in this article, or claim that may be made by its manufacturer, is not guaranteed or endorsed by the publisher.
Supplementary material
The Supplementary material for this article can be found online at: https://www.frontiersin.org/articles/10.3389/fmed.2024.1501284/full#supplementary-material
References
1. Martinez-Alejos, R, Rodrigues-Gomes, RM, and Martí, J-D. Respiratory and mechanical ventilation management: avoidance of complications In: C Boulanger and D McWilliams, editors. Passport to successful ICU discharge. Cham: Springer (2020). 15–27.
2. Pinheiro de Oliveira, R, Hetzel, MP, dos Anjos, SM, Dallegrave, D, and Friedman, G. Mechanical ventilation with high tidal volume induces inflammation in patients without lung disease. Crit Care. (2010) 14:R39. doi: 10.1186/cc8919
3. Al-Husinat, L, Azzam, S, Al Sharie, S, Al Sharie, AH, Battaglini, D, Robba, C, et al. Effects of mechanical ventilation on the interstitial extracellular matrix in healthy lungs and lungs affected by acute respiratory distress syndrome: a narrative review. Crit Care. (2024) 28:165–12. doi: 10.1186/s13054-024-04942-y
4. Lumb, AB, and White, A. Breathing in the prone position in health and disease. BJA Educ. (2021) 21:280–3. doi: 10.1016/j.bjae.2021.05.001
5. Guérin, C, Albert, RK, Beitler, J, Gattinoni, L, Jaber, S, Marini, JJ, et al. Prone position in ARDS patients: why, when, how and for whom. Intensive Care Med. (2020) 46:2385–96. doi: 10.1007/s00134-020-06306-w
6. Ball, L, Talmor, D, and Pelosi, P. Transpulmonary pressure monitoring in critically ill patients: pros and cons. Crit Care. (2024) 28:177–12. doi: 10.1186/s13054-024-04950-y
7. Mahmood, SS, and Pinsky, MR. Heart-lung interactions during mechanical ventilation: the basics. Ann Transl Med. (2018) 6:349–9. doi: 10.21037/atm.2018.04.29
8. Frisvold, S, Coppola, S, Ehrmann, S, Chiumello, D, and Guérin, C. Respiratory challenges and ventilatory management in different types of acute brain-injured patients. Crit Care. (2023) 27:247. doi: 10.1186/s13054-023-04532-4
9. Taran, S, Cho, S-M, and Stevens, RD. Mechanical ventilation in patients with traumatic brain injury: is it so different? Neurocrit Care. (2023) 38:178–91. doi: 10.1007/s12028-022-01593-1
10. Chesnut, R, Aguilera, S, Buki, A, Bulger, E, Citerio, G, Cooper, DJ, et al. A management algorithm for adult patients with both brain oxygen and intracranial pressure monitoring: the Seattle International Severe Traumatic Brain Injury Consensus Conference (SIBICC). Intensive Care Med. (2020) 46:919–29. doi: 10.1007/s00134-019-05900-x
11. Zweifel, C, Lavinio, A, Steiner, LA, Radolovich, D, Smielewski, P, Timofeev, I, et al. Continuous monitoring of cerebrovascular pressure reactivity in patients with head injury. Neurosurg Focus. (2008) 25:E2. doi: 10.3171/FOC.2008.25.10.E2
12. Howells, T, Elf, K, Jones, PA, Ronne-Engström, E, Piper, I, Nilsson, P, et al. Pressure reactivity as a guide in the treatment of cerebral perfusion pressure in patients with brain trauma. J Neurosurg. (2005) 102:311–7. doi: 10.3171/jns.2005.102.2.0311
13. Smielewski, P, Beqiri, E, Mataczynski, C, Placek, M, Kazimierska, A, Hutchinson, PJ, et al. Advanced neuromonitoring powered by ICM+ and its place in the brand new AI world, reflections at the 20th anniversary boundary. Brain Spine. (2024) 4:102835. doi: 10.1016/j.bas.2024.102835
14. Bajpai, BK, Preiksaitis, A, Vosylius, S, and Rocka, S. Association between the cerebral autoregulation index (pressure reactivity), patient’s clinical outcome, and quality of ABP(t) and ICP(t) signals for CA monitoring. Medicina. (2020) 56:143. doi: 10.3390/medicina56030143
15. Zeiler, FA, Kim, DJ, Cabeleira, M, Calviello, L, Smielewski, P, and Czosnyka, M. Impaired cerebral compensatory reserve is associated with admission imaging characteristics of diffuse insult in traumatic brain injury. Acta Neurochir. (2018) 160:2277–87. doi: 10.1007/s00701-018-3681-y
16. Liu, X, Hu, X, Brady, KM, Koehler, R, Smielewski, P, Czosnyka, M, et al. Comparison of wavelet and correlation indices of cerebral autoregulation in a pediatric swine model of cardiac arrest. Sci Rep. (2020) 10:5926. doi: 10.1038/s41598-020-62435-8
17. Baydur, A, Behrakis, P, Zin, W, Jaeger, M, and Milic-Emili, J. A simple method for assessing the validity of the esophageal balloon technique. Am Rev Respir Dis. (1982) 126:788–91. doi: 10.1164/arrd.1982.126.5.788
18. Czosnyka, M, Smielewski, P, Timofeev, I, Lavinio, A, Guazzo, E, Hutchinson, P, et al. Intracranial pressure, more than a number? Neurosurg Focus. (2007) 22:1–7. doi: 10.3171/foc.2007.22.5.11
19. Yoshida, T, Amato, MBP, Grieco, DL, Chen, L, Lima, CAS, Roldan, R, et al. Esophageal manometry and regional transpulmonary pressure in lung injury. Am J Respir Crit Care Med. (2018) 197:1018–26. doi: 10.1164/rccm.201709-1806OC
20. Beqiri, E, Smielewski, P, Guérin, C, Czosnyka, M, Robba, C, Bjertnæs, L, et al. Neurological and respiratory effects of lung protective ventilation in acute brain injury patients without lung injury: brain vent, a single centre randomized interventional study. Crit Care. (2023) 27:115. doi: 10.1186/s13054-023-04383-z
21. Dia, M, Wehner, TC, Arellano, C, and Schwarzer, G. RGxE: an r program for genotype × environment interaction analysis. Am J Plant Sci. (2017) 8:1672–98. doi: 10.4236/ajps.2017.87116
22. Huseby, J, Luce, J, Cary, J, Pavlin, E, and Butler, J. Effects of positive end-expiratory pressure on intracranial pressure in mechanically ventilated dogs under hyperbaric oxygenation. Neurosurgery. (1981) 55:704–7. doi: 10.3171/jns.1981.55.5.0704
23. Aidinis, SJ, Lafferty, J, and Shapiro, HM. Intracranial Responses to PEEP. Anesthesiology. (1976) 45:275–85. doi: 10.1097/00000542-197609000-00004
24. Sun, Q, Wu, D, Yu, T, Yang, Y, Wei, L, Lv, F, et al. Effects of positive end-expiratory pressure on intracranial pressure in mechanically ventilated dogs under hyperbaric oxygenation. Undersea Hyperb Med. (2014) 41:273–6.
25. Asehnoune, K, Rooze, P, Robba, C, Bouras, M, Mascia, L, Cinotti, R, et al. Mechanical ventilation in patients with acute brain injury: a systematic review with meta-analysis. Crit Care. (2023) 27:221. doi: 10.1186/s13054-023-04509-3
26. Ruggieri, F, Beretta, L, Corno, L, Testa, V, Martino, EA, and Gemma, M. Feasibility of protective ventilation during elective supratentorial neurosurgery: a randomized, crossover, clinical trial. J Neurosurg Anesthesiol. (2018) 30:246–50. doi: 10.1097/ANA.0000000000000442
27. Leppert, J, Ditz, C, Souayah, N, Behrens, C, Tronnier, VM, and Küchler, J. Limitations of prone positioning in patients with aneurysmal subarachnoid hemorrhage and concomitant respiratory failure. Clin Neurol Neurosurg. (2023) 232:107878. doi: 10.1016/j.clineuro.2023.107878
28. Roth, C, Ferbert, A, Deinsberger, W, Kleffmann, J, Kästner, S, Godau, J, et al. Does prone positioning increase intracranial pressure? A retrospective analysis of patients with acute brain injury and acute respiratory failure. Neurocrit Care. (2014) 21:186–91. doi: 10.1007/s12028-014-0004-x
29. Heuer, JF, Sauter, P, Barwing, J, Herrmann, P, Crozier, TA, Bleckmann, A, et al. Effects of high-frequency oscillatory ventilation on systemic and cerebral hemodynamics and tissue oxygenation: an experimental study in pigs. Neurocrit Care. (2012) 17:281–92. doi: 10.1007/s12028-011-9566-z
30. Yu, D-Y, Cringle, SJ, Darcey, D, Tien, LYH, Vukmirovic, AJ, Yu, PK, et al. Posture-induced changes in intraocular, orbital, cranial, jugular vein, and arterial pressures in a porcine model. Investig Opthalmology Vis Sci. (2023) 64:22. doi: 10.1167/iovs.64.15.22
31. Vammen, L, Johannsen, CM, Magnussen, A, Povlsen, A, Petersen, SR, Azizi, A, et al. Cerebral monitoring in a pig model of cardiac arrest with 48 h of intensive care. Intensive Care Med Exp. (2022) 10:45. doi: 10.1186/s40635-022-00475-2
32. Chen, H, Zhou, J, Lin, Y-Q, Zhou, J-X, and Yu, R-G. Intracranial pressure responsiveness to positive end-expiratory pressure in different respiratory mechanics: a preliminary experimental study in pigs. BMC Neurol. (2018) 18:183. doi: 10.1186/s12883-018-1191-4
33. Muench, E, Bauhuf, C, Roth, H, Horn, P, Phillips, M, Marquetant, N, et al. Effects of positive end-expiratory pressure on regional cerebral blood flow, intracranial pressure, and brain tissue oxygenation. Crit Care Med. (2005) 33:2367–72. doi: 10.1097/01.CCM.0000181732.37319.DF
34. Nekludov, M, Bellander, B-M, and Mure, M. Oxygenation and cerebral perfusion pressure improved in the prone position. Acta Anaesthesiol Scand. (2006) 50:932–6. doi: 10.1111/j.1399-6576.2006.01099.x
35. Thelandersson, A, Cider, Å, and Nellgård, B. Prone position in mechanically ventilated patients with reduced intracranial compliance. Acta Anaesthesiol Scand. (2006) 50:937–41. doi: 10.1111/j.1399-6576.2006.01037.x
36. Brady, KM, Easley, RB, Kibler, K, Kaczka, DW, Andropoulos, D, Fraser, CD, et al. Positive end-expiratory pressure oscillation facilitates brain vascular reactivity monitoring. J Appl Physiol. (2012) 113:1362–8. doi: 10.1152/japplphysiol.00853.2012
37. Klein, SP, Depreitere, B, and Meyfroidt, G. How I monitor cerebral autoregulation. Crit Care. (2019) 23:160. doi: 10.1186/s13054-019-2454-1
38. Klein, SP, Decraene, B, De Sloovere, V, Kempen, B, Meyfroidt, G, and Depreitere, B. The pressure reactivity index as a measure for cerebrovascular autoregulation: validation in a porcine cranial window model. Neurosurgery. (2024) 95:1450–6. doi: 10.1227/neu.0000000000003019
39. Robba, C, Graziano, F, Guglielmi, A, Rebora, P, Galimberti, S, Taccone, FS, et al. Treatments for intracranial hypertension in acute brain-injured patients: grading, timing, and association with outcome. Data from the SYNAPSE-ICU study. Intensive Care Med. (2023) 49:50–61. doi: 10.1007/s00134-022-06937-1
40. Tsigaras, ZA, Weeden, M, McNamara, R, Jeffcote, T, Udy, AA, Anstey, J, et al. The pressure reactivity index as a measure of cerebral autoregulation and its application in traumatic brain injury management. Crit Care Resusc. (2023) 25:229–36. doi: 10.1016/j.ccrj.2023.10.009
41. Chen, H, Zhou, X-F, Zhou, D-W, Zhou, J-X, and Yu, R-G. Effect of increased positive end-expiratory pressure on intracranial pressure and cerebral oxygenation: impact of respiratory mechanics and hypovolemia. BMC Neurosci. (2021) 22:72. doi: 10.1186/s12868-021-00674-9
42. Robba, C, Ball, L, Nogas, S, Battaglini, D, Messina, A, Brunetti, I, et al. Effects of positive end-expiratory pressure on lung recruitment, respiratory mechanics, and intracranial pressure in mechanically ventilated brain-injured patients. Front Physiol. (2021) 12:711273. doi: 10.3389/fphys.2021.711273
Glossary
ABP - Arterial blood pressure
CO - Cardiac output
CPP - Cerebral perfusion pressure
CVP - Central venous pressure
Ecw - Elastance of the chest wall
El - Lung elastance
Ers - Elastance of the respiratory system
EVLW - Extravascular lung water
ICP - Intracranial pressure
MPlDep - Mechanical power of the dependent lung
MPlnonDep - Mechanical power of the non-dependent lung
MPrs - Mechanical power of the respiratory system
PaCO2 - Arterial partial pressure of carbon dioxide
PaO2 - Arterial partial pressure of oxygen
PaO2/FiO2 - Ratio of PaO2 to inspired fraction of oxygen in air
Pawee - End-expiratory airway pressure
Pawei - End-inspiratory airway pressure
PAP - Pulmonary artery pressure
Pesee - End-expiratory esophageal pressure
Pesei - End-inspiratory esophageal pressure
Ppeakcw - Peak inspiratory pressure of the chest wall
Ppeakl - Lung peak pressure
Ppeakrs - Peak inspiratory pressure of the respiratory system
PRx - Pressure reactivity index
RAP - Compensatory reserve index
RR - Respiratory rate
SVV - Stroke volume variation
TPPei - End-inspiratory transpulmonary pressure
TPPee - End-expiratory transpulmonary pressure
TPPelast - Elastance-derived transpulmonary pressure
VT - Tidal volume
Keywords: cerebral autoregulation, ICP, neurocritical care, neuromonitoring, pulmonary issues, ventilation, mechanical ventilation, transpulmonary pressure
Citation: Hammervold R, Beqiri E, Smielewski P, Storm BS, Nielsen EW, Guérin C and Frisvold SK (2025) Positive end-expiratory pressure increases intracranial pressure but not pressure reactivity index in supine and prone positions: a porcine model study. Front. Med. 11:1501284. doi: 10.3389/fmed.2024.1501284
Edited by:
Domenico Luca Grieco, Agostino Gemelli University Polyclinic (IRCCS), ItalyReviewed by:
Mariangela Pellegrini, Uppsala University, SwedenEmanuele Rossetti, Bambino Gesù Children’s Hospital (IRCCS), Italy
Copyright © 2025 Hammervold, Beqiri, Smielewski, Storm, Nielsen, Guérin and Frisvold. This is an open-access article distributed under the terms of the Creative Commons Attribution License (CC BY). The use, distribution or reproduction in other forums is permitted, provided the original author(s) and the copyright owner(s) are credited and that the original publication in this journal is cited, in accordance with accepted academic practice. No use, distribution or reproduction is permitted which does not comply with these terms.
*Correspondence: Shirin K. Frisvold, U2hpcmluLmZyaXN2b2xkQHVpdC5ubw==