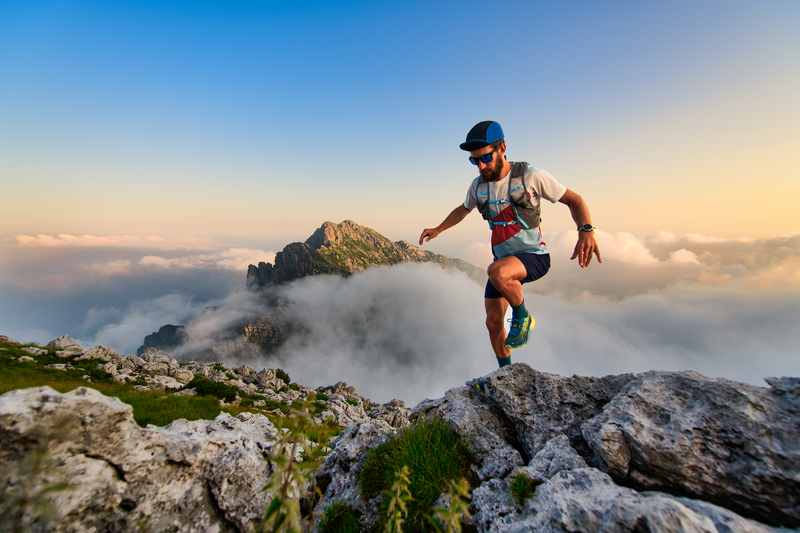
95% of researchers rate our articles as excellent or good
Learn more about the work of our research integrity team to safeguard the quality of each article we publish.
Find out more
MINI REVIEW article
Front. Med. , 27 August 2024
Sec. Hematology
Volume 11 - 2024 | https://doi.org/10.3389/fmed.2024.1448654
High altitude polycythemia is a maladaptation of highlanders exposed to hypoxic environment, leading to high blood viscosity and severe cardiorespiratory dysfunction. Prolonged hypoxia causes respiratory depression and severe hypoxemia, and further mediates changes in genetic and molecular mechanisms that regulate erythropoiesis and apoptosis, ultimately resulting in excessive erythrocytosis (EE). This updated review investigated the maladaptive mechanisms of EE, including respiratory chemoreceptor passivation, sleep-related breathing disorders, sex hormones, iron metabolism, and hypoxia-related factors and pathways.
Over 140 million people reside in highlands globally, among whom some people develop hypoxemia due to insufficient oxygen supply, leading to excessive erythrocytosis (EE) and increased blood viscosity, so-called high altitude polycythemia (HAPC), which is the most important contributor to chronic mountain sickness (CMS), a well-known maladaptive syndrome in highlands (1, 2).
Different populations show varied morbidity and adaptation. The incidence of EE was 2.39% in Lhasa, Tibet (3650 m) (3), whereas it increased to 4.5% among Peruvians in the Andes (3825 m) (4) and peaked at 44% in La Rinconada, Peru (5200 m) (5). Tibetans have been living in the Qinghai-Tibet Plateau for ten thousand years and successfully evolved with adaptive genetic mechanisms, thus showing a lower hematocrit (Hct) and incidence of EE than other highlanders living at the same altitude (6). Gene variations in EGLN1 and EPAS1 that regulated the pathways associated with hypoxia-inducible factor (HIF) were demonstrated to exert a significant influence on Tibetan adaptation (7, 8). Noticeably, distinct genetic adaptations to highlands were also revealed in other populations. However, the frequency of adaptive mutations such as the EPAS1 variant (rs570553380) was relatively low in Andean highlanders (9, 10), which resulted in a particular maladaptation to the hypoxic environment and more severe clinical manifestations (Table 1).
The maladaptive mechanisms that accelerate EE development are catching more attentions as the pathogenesis of EE has not been fully elucidated. Long-term hypoxic exposure stimulates the increase of erythrocyte count to enhance the transportation capacity of oxygen in blood, but promote the development of EE at the same time. In addition to the genetic adaptation, the maladaptive mechanisms of EE have been reported, including respiratory chemoreceptor passivation, sleep-related breathing disorders, sex hormones, iron metabolism, and hypoxia-related factors and pathways (11–17), which were reviewed with updates in this study, and prospects regarding new therapeutic strategies for HAPC were discussed as well.
Blunted chemoreceptor sensitivity means a decreased respiratory response to hypoxia, which has been observed in highlanders, leading to severe hypoxemia and EE. The chemoreflex drives of breathing were stimulated by sustained hypoxia to regulate ventilation response for the stabilization of arterial PO2 and were commonly evaluated by the hypoxic ventilatory response (HVR) and hypercapnic ventilatory response (HCVR) (18, 19).
Previous studies have documented that Han-Tibetan immigrants and Andeans with prolonged high-altitude living exhibited progressively weaker HVR and lower ventilation than Tibetans (20, 21). The discrepancy mainly accounted for a loss-of-function allele in Tibetans named EGLN1 that played a pivotal role in enhancing HVR (8). Additionally, Menuet et al. (22) also observed blunted HCVR in respiratory recordings of Tg6 mice with high erythropoietin (EPO) level in brain and plasma, and concluded that the high level of plasma EPO, but not cerebral EPO, acted on erythropoiesis and regulation of the blunted HCVR (22), possibly due to the reduced depletion of O2 and CO2, rather than the effect of EPO on CO2 chemosensitivity (23).
Nocturnal periodic breathing (nPB) and nocturnal hypoxemia are frequent manifestations for sleep disorders in highlanders (12), which are attributed to insufficient ventilation and apnea caused by hypoxia in highlands (24). The nocturnal hypoxemia, represented by lower nocturnal blood oxygen saturation (SpO2), has been demonstrated to be relevant to higher Hct (25). Moreover, the apnea-hypopnea index (AHI), an indicator of nPB severity, was revealed to be negatively related to nocturnal SpO2, suggesting that more severe nBP correlated with lower nocturnal SpO2 (12). However, it is still doubtful whether nPB is only a clinical manifestation caused by nocturnal hypoxemia, since no univariate relevance between nPB and EE has been detected (26). In male Andeans, Hct was observed to be forecasted only by average sleep SpO2, AHI, and obstructive apnea index (OAI) (27), which indicated that the direct effect of nPB on Hct might be limited. Furthermore, a recent study suggested that ameliorating nPB did not improve the disordered sleep structure of highlanders, and thus more valuable indicators are needed to evaluate the relationship between sleep pattern and EE occurrence (24).
The prevalence of HAPC is higher in men and postmenopausal women due to sex hormone regulation (4). Higher serum testosterone level was detected in male EE patients and up to 45% of postmenopausal women had high CMS scores with increased Hct in highlands, increased by 23% compared to premenopausal women (27–29). Azad et al. (17) collected samples from Andeans at an altitude of 4338m and established an induced pluripotent stem cell line to find that estrogen significantly decreased hypoxia-induced erythropoiesis in a dose-dependent manner (17). The estrogen directly reduced the expression level of GATA1, a transcription factor regulating erythroid differentiation, and inhibited its downstream targets, including Alas2, Bcl-xL, and erythropoietin receptor (EpoR), to decrease hypoxia-induced erythropoiesis and increase erythrocyte apoptosis (17). Moreover, the mRNA expressions of GATA1, vascular endothelial growth factor (VEGF), and HIF-1 were distinctly restrained by estrogen beta signaling that was identified as a dominant pathway involved in inhibiting EE progression (17).
Iron is an important component of hemoglobin and is involved in EE occurrence. EE patients among Han immigrants living in the Qinghai-Tibet Plateau showed higher concentrations of serum iron, Hct, serum soluble transferrin receptor, and serum ferritin than healthy highlanders, indicating increased iron availability and reserves (30). The increased expression of HIF-2α in the intestine of EE mice upregulated the hub gene expression related to iron metabolism, such as Dmt1, Dcytb, Fpn, Tfrc, and Fth, to enhance iron availability and thus promote erythropoiesis (13). Hepcidin, a peptide hormone, plays a crucial role in iron homeostasis by inhibiting excessive iron mobilization. In the hypoxic environment, the hepcidin expression level was decreased, followed by stored iron releasing and consequent erythropoiesis, which was involved in downregulation of STAT3 signaling pathway (30). Moreover, red pulp macrophages (RPMs) in the spleen serve as a cleaner for the removal of abnormal erythrocytes, and the ferroptosis of RPMs due to lipid peroxidation was observed in C58BL/6 mice with hypoxia, resulting in decreased RPMs and erythrocytic clearance (31). Besides, in a recent study, men living in the highest cities of the world indicated that their iron stores remained stable regardless of whether they suffered from CMS, which implied the highlanders might have a unique mechanism for maintaining an efficient balance between iron absorption/storage and its consumption (32).
EPO, mainly generated by the kidneys, is induced in anoxic environment and binds to EpoR, then activating its downstream signals to further promote erythropoiesis and reduce erythrocyte apoptosis (33). HIF is a regulator of EPO, including HIF-1α and HIF-2α, and involved in acute and chronic hypoxia respectively, which mediates erythrocyte formation by affecting the transcription and synthesis of EPO (34). Recent studies have suggested that HIF-2α/EPO-related pathway plays a dominant role in hypoxia-induced erythrocytosis (35).
VHL is a tumor suppressor involved in the ubiquitylation and degradation of HIFs (36, 37). Hypoxia promoted methylation of the VHL promoter by increasing the expression of DNA methyltransferase, DNMT3α and DNMT3β, leading to inhibition of VHL expression (14, 38). The downregulated VHL protected the HIF-2α from degradation and contributed to erythropoiesis (14). Moreover, the mRNA expression of HIF-2α, but not HIF-1α, was upregulated in the bone marrow cells of patients with EE (14). Overall, hypoxia inhibits VHL to reduce the degradation of HIFs and promotes HIF-2α/EPO-related pathway, resulting in EE.
EPO regulates erythropoiesis through the EPO/EpoR pathway (39), and soluble EpoR (sEpoR) is a protein produced by alternative splicing of EpoR mRNA and can reduce EPO availability by competitively combining with EPO (40). Noticeably, only part of EE patients exhibited raised EPO levels, while other patients had normal EPO levels, suggesting, in addition to EPO, sEpoR may play a role in pathogenesis of EE (41). Hypoxia-induced downregulation of sEpoR was observed to modulate respiratory status and increase oxygen transport in mice (42). Further, sEpoR was decreased in both normal and high EPO groups of Andeans with EE at an altitude of 4340m while EPO/sEpoR was positively correlated with Hct and negatively correlated with SpO2 (41). Another study reported a higher EPO/sEpoR ratio during the night and early morning in Andean highlanders, despite EPO concentration showing no obvious change over time (43). The proportion of lower nocturnal SpO2 ( ≤ 80%) was higher in CMS patients, leading to a decrease in sEpoR and an increase in nocturnal EPO/sEpoR ratio and erythropoiesis (43). Overall, the EPO/sEpoR ratio rather than EPO may play a key role in EE.
SENP1 is a nuclear small ubiquitin-related modifier protein (SUMO) protease. Previous studies demonstrated that SENP1 was involved in the pathogenesis of CMS and regulated hypoxia-stimulating erythropoiesis (44). A decrease in the number of erythroid colony-forming units (CFU-e) was observed in SENP1 knockout mice due to EPO downregulation and increased apoptosis of erythroid progenitor cells (45). SENP1 regulated EPO production and erythrocyte metabolism through HIF and GATA-1 in the hypoxic environment (15, 45). HIF is sumoylated intracellularly and then degraded by SUMO-targeted ubiquitin ligases. SENP1 protects sumoylated HIF from degradation by desumoylation and increases the downstream effects of HIF under hypoxic conditions (45, 46), then further regulates the production of EPO and erythrocytes. GATA1, a transcription factor that regulates erythroid differentiation, interacts with a variety of hematopoietic transcription factors and is involved in the regulation of erythropoiesis genes such as heme biosynthetic enzymes, hemoglobin, EpoR, and anti-apoptotic protein (Bcl-xL) (47–51), and GATA1-deficient mice exhibited severe anemia and eventual death (48). SENP1 can upregulate the expression of GATA1 and its downstream factor Bcl-xL during hypoxia, then contributing to erythrocyte proliferation and apoptosis inhibition (15, 48). Overall, under hypoxic environment, SENP1 upregulates the expression of HIF and GATA1 to increase EPO and erythropoiesis.
long-chain noncoding RNAs (lncRNAs) are transcripts that can regulate gene transcription, having a vital role in cardiovascular, neurological, endocrine systems, cancer occurrence, and so on (52), which was recently revealed to be correlated with EE (53). An RNA-Seq analysis was conducted to investigate the effect of gene transcription of erythroid cells on EE patients and significant differences in the expression of lncRNAs were discovered between EE and non-EE subjects (53). Among these, the lncRNA showing the most notable change was named hypoxia-induced kinase-mediated erythropoietic regulator (HIKER)/LINC02228, which was upregulated in vitro with EE phenotype and proved to be significant for regulating erythroid progenitors under hypoxic conditions by increasing the expression of casein kinase 2β unit (CSNK2B) (53), a downstream factor of HIKER/LINC02228. CSNK2B was suggested to promote erythrocyte growth and upregulation of GATA1 (54), and CSNK2B knockout mice showed severe anemia (53). Overall, hypoxia upregulates the HIKER/LINC02228 in EE patients to increase CSNK2B expression, which further promotes the GATA1 level and thus erythrocyte growth.
This updated review suggested that the pathogenesis of HAPC was involved in not only lack of the adaptive genetic background, but also complicated maladaptive mechanisms that were discussed above. However, the advances in HAPC treatment are slow. In addition to recommendation of migration to low-altitude areas, the conventional treatments for HAPC include phlebotomy and hemodilution (55, 56), which often result in hypovolemia, iron deficiency and even exacerbation of pulmonary hypertension (57), but recently, erythrocytapheresis has been widely used to reduce Hct in HAPC by seperating erythrocytes from the circulation with hemodynamic stability (58).
However, targeting on the maladaptive mechanisms could be more effective for treatment of HAPC. Six-month administration of acetazolamide, a carbonic anhydrase inhibitor, stimulated respiratory response and rectify hypoxemia, and thus reduced Hct, EPO and pulmonary vascular resistance and even improved sleep-related breathing disorders (59, 60). Noticeably, as a methylated lipophilic analog of acetazolamide, methazolamide was shown to reduce Hct and blood viscosity dose-dependently in a rat model of EE, but with fewer side effects than acetazolamide, indicating a promising prospect for methazolamide in HAPC treatment (61, 62).
Maca is a plant growing in Peru, and long-term use of maca reduced the Hct in EE patients (63), which was implicated in regulation of sex hormone and its related pathways (64). Likewise, as a traditional Chinese medicinal plant, astragalus membranaceus, decreased erythropoiesis by inhibiting the differentiation of hematopoietic stem cells into erythroid lineage and downregulating the expression of HIF-1a, EPO and GATA-1 in HAPC mice (65).
Interestingly, it was demonstrated that arginine could inhibit the development of EE by reducing EPO/EpoR via upregulation of miR-144-5p in a rat model of CMS (66). Moreover, HIF-2α antagonists, CSNK2B inhibitors and DNMT inhibitors were reported to improve hypoxia-induced EE in experimental studies, suggesting potential pharmaceutical values (13, 14, 53).
Taken together, although the treatment of HAPC is dependent on the conventional methods, new strategies targeting on the maladaptive mechanisms may lead to better therapeutic effects in the forthcoming future.
ST: Writing – original draft. WZ: Writing – original draft. LiC: Writing – original draft. HY: Writing – review and editing. LeC: Writing – review and editing. FL: Writing – review and editing.
The author(s) declare financial support was received for the research, authorship, and/or publication of the article. This study was supported in part by grants GYYX24001 and GYYX24002 from 1.3.5 Project of Center for High Altitude Medicine, West China Hospital, Sichuan University.
The authors declare that the research was conducted in the absence of any commercial or financial relationships that could be construed as a potential conflict of interest.
All claims expressed in this article are solely those of the authors and do not necessarily represent those of their affiliated organizations, or those of the publisher, the editors and the reviewers. Any product that may be evaluated in this article, or claim that may be made by its manufacturer, is not guaranteed or endorsed by the publisher.
1. Tremblay J, Hoiland R, Howe C, Coombs G, Vizcardo-Galindo G, Figueroa-Mujíca R, et al. Global REACH 2018: High blood viscosity and hemoglobin concentration contribute to reduced flow-mediated dilation in high-altitude excessive erythrocytosis. Hypertension. (2019) 73:1327–35. doi: 10.1161/HYPERTENSIONAHA.119.12780
2. Soria R, Egger M, Scherrer U, Bender N, Rimoldi S. Pulmonary arterial pressure at rest and during exercise in chronic mountain sickness: A meta-analysis. Eur Respir J. (2019) 53:1802040. doi: 10.1183/13993003.02040-2018
3. Chen S. Investigation on the incidence of high altitude polycythemia and its hemoglobin characteristics in a Tibetan population. Zhongguo Yi Xue Ke Xue Yuan Xue Bao. (1992) 14:237–43.
4. De Ferrari A, Miranda J, Gilman R, Dávila-Román V, León-Velarde F, Rivera-Ch M, et al. Prevalence, clinical profile, iron status, and subject-specific traits for excessive erythrocytosis in andean adults living permanently at 3,825 meters above sea level. Chest. (2014) 146:1327–36. doi: 10.1378/chest.14-0298
5. Hancco I, Bailly S, Baillieul S, Doutreleau S, Germain M, Pépin J, et al. Excessive erythrocytosis and chronic mountain sickness in dwellers of the highest city in the world. Front Physiol. (2020) 11:773. doi: 10.3389/fphys.2020.00773
6. Beall C. Andean, Tibetan, and Ethiopian patterns of adaptation to high-altitude hypoxia. Integr Comp Biol. (2006) 46:18–24. doi: 10.1093/icb/icj004
7. Lorenzo F, Huff C, Myllymäki M, Olenchock B, Swierczek S, Tashi T, et al. A genetic mechanism for Tibetan high-altitude adaptation. Nat Genet. (2014) 46:951–6. doi: 10.1038/ng.3067
8. Song D, Navalsky B, Guan W, Ingersoll C, Wang T, Loro E, et al. Tibetan PHD2, an allele with loss-of-function properties. Proc Natl Acad Sci USA. (2020) 117:12230–8. doi: 10.1073/pnas.1920546117
9. Brutsaert T, Kiyamu M, Elias Revollendo G, Isherwood J, Lee F, Rivera-Ch M, et al. Association of EGLN1 gene with high aerobic capacity of Peruvian Quechua at high altitude. Proc Natl Acad Sci USA. (2019) 116:24006–11. doi: 10.1073/pnas.1906171116
10. Lawrence E, Gu W, Bohlender R, Anza-Ramirez C, Cole A, Yu J, et al. Functional EPAS1/HIF2A missense variant is associated with hematocrit in Andean highlanders. Sci Adv. (2024) 10:eadj5661. doi: 10.1126/sciadv.adj5661
11. Brutsaert T. Population genetic aspects and phenotypic plasticity of ventilatory responses in high altitude natives. Respir Physiol Neurobiol. (2007) 158:151–60. doi: 10.1016/j.resp.2007.03.004
12. Rexhaj E, Rimoldi S, Pratali L, Brenner R, Andries D, Soria R, et al. Sleep-disordered breathing and vascular function in patients with chronic mountain sickness and healthy high-altitude dwellers. Chest. (2016) 149:991–8. doi: 10.1378/chest.15-1450
13. Zhou S, Yan J, Song K, Ge R. High-altitude hypoxia induces excessive erythrocytosis in mice via upregulation of the intestinal HIF2a/iron-metabolism pathway. Biomedicines. (2023) 11:2992. doi: 10.3390/biomedicines11112992
14. Yang M, Zhu M, Song K, Wuren T, Yan J, Ge R, et al. VHL gene methylation contributes to excessive erythrocytosis in chronic mountain sickness rat model by upregulating the HIF-2α/EPO pathway. Life Sci. (2021) 266:118873. doi: 10.1016/j.lfs.2020.118873
15. Azad P, Zhao H, Cabrales P, Ronen R, Zhou D, Poulsen O, et al. Senp1 drives hypoxia-induced polycythemia via GATA1 and Bcl-xL in subjects with Monge’s disease. J Exp Med. (2016) 213:2729–44. doi: 10.1084/jem.20151920
16. Vizcardo-Galindo G, León-Velarde F, Villafuerte F. High-altitude hypoxia decreases plasma erythropoietin soluble receptor concentration in lowlanders. High Alt Med Biol. (2020) 21:92–8. doi: 10.1089/ham.2019.0118
17. Azad P, Villafuerte F, Bermudez D, Patel G, Haddad G. Protective role of estrogen against excessive erythrocytosis in Monge’s disease. Exp Mol Med. (2021) 53:125–35. doi: 10.1038/s12276-020-00550-2
18. Duffin J. The chemoreflex control of breathing and its measurement. Can J Anaesth. (1990) 37:933–42. doi: 10.1007/BF03006641
19. Slessarev M, Prisman E, Ito S, Watson R, Jensen D, Preiss D, et al. Differences in the control of breathing between Himalayan and sea-level residents. J Physiol. (2010) 588:1591–606. doi: 10.1113/jphysiol.2009.185504
20. Curran L, Zhuang J, Sun S, Moore L. Ventilation and hypoxic ventilatory responsiveness in Chinese-Tibetan residents at 3,658 m. J Appl Physiol (1985). (1997) 83:2098–104. doi: 10.1152/jappl.1997.83.6.2098
21. Beall C. Tibetan and Andean patterns of adaptation to high-altitude hypoxia. Hum Biol. (2000) 72:201–28.
22. Menuet C, Khemiri H, de la Poëze d’Harambure T, Gestreau C. Polycythemia and high levels of erythropoietin in blood and brain blunt the hypercapnic ventilatory response in adult mice. Am J Physiol Regul Integr Comp Physiol. (2016) 310:R979–91. doi: 10.1152/ajpregu.00474.2015
23. Laouafa S, Elliot-Portal E, Revollo S, Schneider Gasser E, Joseph V, Voituron N, et al. Hypercapnic ventilatory response is decreased in a mouse model of excessive erythrocytosis. Am J Physiol Regul Integr Comp Physiol. (2016) 311:R940–7. doi: 10.1152/ajpregu.00226.2016
24. Ibrahim A, Stefani A, Cesari M, Roche J, Gatterer H, Holzknecht E, et al. Effects of periodic breathing on sleep at high altitude: A randomized, placebo-controlled, crossover study using inspiratory CO2. J Physiol. (2024). doi: 10.1113/JP285397
25. Perger E, Baillieul S, Esteve F, Pichon A, Bilo G, Soranna D, et al. Nocturnal hypoxemia, blood pressure, vascular status and chronic mountain sickness in the highest city in the world. Ann Med. (2022) 54:1884–93. doi: 10.1080/07853890.2022.2091791
26. Spicuzza L, Casiraghi N, Gamboa A, Keyl C, Schneider A, Mori A, et al. Sleep-related hypoxaemia and excessive erythrocytosis in Andean high-altitude natives. Eur Respir J. (2004) 23:41–6. doi: 10.1183/09031936.03.00000703
27. Heinrich E, Orr J, Gilbertson D, Anza-Ramirez C, DeYoung P, Djokic M, et al. Relationships between chemoreflex responses, sleep quality, and hematocrit in andean men and women. Front Physiol. (2020) 11:437. doi: 10.3389/fphys.2020.00437
28. Gonzales G, Tapia V, Gasco M, Rubio J, Gonzales-Castañeda C. High serum zinc and serum testosterone levels were associated with excessive erythrocytosis in men at high altitudes. Endocrine. (2011) 40:472–80. doi: 10.1007/s12020-011-9482-1
29. León-Velarde F, Ramos M, Hernández J, De Idiáquez D, Muñoz L, Gaffo A, et al. The role of menopause in the development of chronic mountain sickness. Am J Physiol. (1997) 272:R90–4. doi: 10.1152/ajpregu.1997.272.1.R90
30. Liu Y, Huang H, Zhou S, Tian H, Li P. Excessive iron availability caused by disorders of interleukin-10 and interleukin-22 contributes to high altitude polycythemia. Front Physiol. (2018) 9:548. doi: 10.3389/fphys.2018.00548
31. Yang W, Li M, Ding J, Li J, Wu G, Liu B, et al. High-altitude hypoxia exposure inhibits erythrophagocytosis by inducing macrophage ferroptosis in the spleen. Elife. (2024) 12:R87496. doi: 10.7554/eLife.87496
32. Cairo G, Champigneulle B, Correnti M, Gammella E, Recalcati S, Girelli D, et al. Excessive erythrocytosis is not associated with altered iron homeostasis in men from the world’s highest city. Hemasphere. (2023) 7:e849. doi: 10.1097/HS9.0000000000000849
33. Franke K, Gassmann M, Wielockx B. Erythrocytosis: The HIF pathway in control. Blood. (2013) 122:1122–8. doi: 10.1182/blood-2013-01-478065
34. Semenza G. Regulation of erythropoiesis by the hypoxia-inducible factor pathway: Effects of genetic and pharmacological perturbations. Annu Rev Med. (2023) 74:307–19. doi: 10.1146/annurev-med-042921-102602
35. Lappin T, Lee F. Update on mutations in the HIF: EPO pathway and their role in erythrocytosis. Blood Rev. (2019) 37:100590. doi: 10.1016/j.blre.2019.100590
36. Cockman M, Masson N, Mole D, Jaakkola P, Chang G, Clifford S, et al. Hypoxia inducible factor-alpha binding and ubiquitylation by the von Hippel-Lindau tumor suppressor protein. J Biol Chem. (2000) 275:25733–41. doi: 10.1074/jbc.M002740200
37. Krieg M, Haas R, Brauch H, Acker T, Flamme I, Plate K. Up-regulation of hypoxia-inducible factors HIF-1alpha and HIF-2alpha under normoxic conditions in renal carcinoma cells by von Hippel-Lindau tumor suppressor gene loss of function. Oncogene. (2000) 19:5435–43. doi: 10.1038/sj.onc.1203938
38. Chen T, Ueda Y, Dodge J, Wang Z, Li E. Establishment and maintenance of genomic methylation patterns in mouse embryonic stem cells by Dnmt3a and Dnmt3b. Mol Cell Biol. (2003) 23:5594–605. doi: 10.1128/MCB.23.16.5594-5605.2003
39. Todokoro K, Kuramochi S, Nagasawa T, Abe T, Ikawa Y. Isolation of a cDNA encoding a potential soluble receptor for human erythropoietin. Gene. (1991) 106:283–4. doi: 10.1016/0378-1119(91)90213-u
40. Kuramochi S, Ikawa Y, Todokoro K. Characterization of murine erythropoietin receptor genes. J Mol Biol. (1990) 216:567–75. doi: 10.1016/0022-2836(90)90384-x
41. Villafuerte F, Macarlupú J, Anza-Ramírez C, Corrales-Melgar D, Vizcardo-Galindo G, Corante N, et al. Decreased plasma soluble erythropoietin receptor in high-altitude excessive erythrocytosis and chronic mountain sickness. J Appl Physiol (1985). (2014) 117:1356–62. doi: 10.1152/japplphysiol.00619.2014
42. Soliz J, Gassmann M, Joseph V. Soluble erythropoietin receptor is present in the mouse brain and is required for the ventilatory acclimatization to hypoxia. J Physiol. (2007) 583:329–36. doi: 10.1113/jphysiol.2007.133454
43. Villafuerte F, Corante N, Anza-Ramírez C, Figueroa-Mujíca R, Vizcardo-Galindo G, Mercado A, et al. Plasma soluble erythropoietin receptor is decreased during sleep in Andean highlanders with chronic mountain sickness. J Appl Physiol (1985). (2016) 121:53–8. doi: 10.1152/japplphysiol.00107.2016
44. Stobdan T, Akbari A, Azad P, Zhou D, Poulsen O, Appenzeller O, et al. New insights into the genetic basis of Monge’s disease and adaptation to high-altitude. Mol Biol Evol. (2017) 34:3154–68. doi: 10.1093/molbev/msx239
45. Cheng J, Kang X, Zhang S, Yeh ETH. SUMO-specific protease 1 is essential for stabilization of HIF1alpha during hypoxia. Cell. (2007) 131:584–95. doi: 10.1016/j.cell.2007.08.045
46. van Hagen M, Overmeer R, Abolvardi S, Vertegaal A. RNF4 and VHL regulate the proteasomal degradation of SUMO-conjugated Hypoxia-Inducible Factor-2alpha. Nucleic Acids Res. (2010) 38:1922–31. doi: 10.1093/nar/gkp1157
47. Papadopoulos G, Karkoulia E, Tsamardinos I, Porcher C, Ragoussis J, Bungert J, et al. GATA-1 genome-wide occupancy associates with distinct epigenetic profiles in mouse fetal liver erythropoiesis. Nucleic Acids Res. (2013) 41:4938–48. doi: 10.1093/nar/gkt167
48. Yu L, Ji W, Zhang H, Renda M, He Y, Lin S, et al. SENP1-mediated GATA1 deSUMOylation is critical for definitive erythropoiesis. J Exp Med. (2010) 207:1183–95. doi: 10.1084/jem.20092215
49. Gregory T, Yu C, Ma A, Orkin S, Blobel G, Weiss MJ. GATA-1 and erythropoietin cooperate to promote erythroid cell survival by regulating bcl-xL expression. Blood. (1999) 94:87–96.
50. Chiba T, Ikawa Y, Todokoro K. GATA-1 transactivates erythropoietin receptor gene, and erythropoietin receptor-mediated signals enhance GATA-1 gene expression. Nucleic Acids Res. (1991) 19:3843–8. doi: 10.1093/nar/19.14.3843
51. Lacombe C, Mayeux P. The molecular biology of erythropoietin. Nephrol Dial Transplant. (1999) 14:22–8. doi: 10.1093/ndt/14.suppl_2.22
52. Ferrer J, Dimitrova N. Transcription regulation by long non-coding RNAs: Mechanisms and disease relevance. Nat Rev Mol Cell Biol. (2024) 25:396–415. doi: 10.1038/s41580-023-00694-9
53. Azad P, Zhou D, Tu H, Villafuerte F, Traver D, Rana T, et al. Long noncoding RNA HIKER regulates erythropoiesis in Monge’s disease via CSNK2B. J Clin Invest. (2023) 133:e165831. doi: 10.1172/JCI165831
54. Quotti Tubi L, Canovas Nunes S, Carrino M, Gianesin K, Manni S, Filhol-Cochet O, et al. Hematopoietic-specific CSNK2B loss in mice causes impaired erythropoiesis. Blood. (2017) 130:82. doi: 10.1182/blood.V130.Suppl_1.82.82
55. Winslow R, Monge C, Brown E, Klein H, Sarnquist F, Winslow N, et al. Effects of hemodilution on O2 transport in high-altitude polycythemia. J Appl Physiol (1985). (1985) 59:1495–502. doi: 10.1152/jappl.1985.59.5.1495
56. Cruz J, Diaz C, Marticorena E, Hilario V. Phlebotomy improves pulmonary gas exchange in chronic mountain polycythemia. Respiration. (1979) 38:305–13. doi: 10.1159/000194097
57. Smith T, Talbot N, Privat C, Rivera-Ch M, Nickol A, Ratcliffe P, et al. Effects of iron supplementation and depletion on hypoxic pulmonary hypertension: Two randomized controlled trials. JAMA. (2009) 302:1444–50. doi: 10.1001/jama.2009.1404
58. Niu M, Singh S, Mi M, Bian P, Deji Z, Mima D, et al. Safety and efficacy of therapeutic erythrocytapheresis treatment in chronic mountain sickness patients in Shigatse, Tibet, China. Med Sci Monit. (2020) 26:e927853. doi: 10.12659/MSM.927853
59. Richalet J, Rivera-Ch M, Maignan M, Privat C, Pham I, Macarlupu J, et al. Acetazolamide for Monge’s disease: Efficiency and tolerance of 6-month treatment. Am J Respir Crit Care Med. (2008) 177:1370–6. doi: 10.1164/rccm.200802-196OC
60. Richalet J, Rivera M, Bouchet P, Chirinos E, Onnen I, Petitjean O, et al. Acetazolamide: A treatment for chronic mountain sickness. Am J Respir Crit Care Med. (2005) 172:1427–33. doi: 10.1164/rccm.200505-807OC
61. Zhang Z, Xiao Z, Deng B, Liu X, Liu W, Nie H, et al. Therapeutic efficacy of methazolamide against intermittent hypoxia-induced excessive erythrocytosis in rats. High Alt Med Biol. (2018) 19:69–80. doi: 10.1089/ham.2017.0044
62. Lu H, Zhang H, Jiang Y. Methazolamide in high-altitude illnesses. Eur J Pharm Sci. (2020) 148:105326. doi: 10.1016/j.ejps.2020.105326
63. Gonzales-Arimborgo C, Yupanqui I, Montero E, Alarcón-Yaquetto D, Zevallos-Concha A, Caballero L, et al. Acceptability, safety, and efficacy of oral administration of extracts of black or red maca (Lepidium meyenii) in adult human subjects: A randomized, double-blind, placebo-controlled study. Pharmaceuticals (Basel). (2016) 9:49. doi: 10.3390/ph9030049
64. Gonzales G, Gasco M, Lozada-Requena I. Role of maca (Lepidium meyenii) consumption on serum interleukin-6 levels and health status in populations living in the Peruvian Central Andes over 4000 m of altitude. Plant Foods Hum Nutr. (2013) 68:347–51. doi: 10.1007/s11130-013-0378-5
65. Liu X, Zhang H, Yan J, Li X, Li J, Hu J, et al. Deciphering the efficacy and mechanism of Astragalus membranaceus on high altitude polycythemia by integrating network pharmacology and in vivo experiments. Nutrients. (2022) 14:4968. doi: 10.3390/nu14234968
66. Zhang L, Liu X, Wei Q, Zou L, Zhou L, Yu Y, et al. Arginine attenuates chronic mountain sickness in rats via microRNA-144-5p. Mamm Genome. (2023) 34:76–89. doi: 10.1007/s00335-023-09980-5
67. Xiang K, Ouzhuluobu, Peng Y, Yang Z, Zhang X, Cui C, et al. Identification of a Tibetan-specific mutation in the hypoxic gene EGLN1 and its contribution to high-altitude adaptation. Mol Biol Evol. (2013) 30:1889–98. doi: 10.1093/molbev/mst090
68. Simonson T, Yang Y, Huff C, Yun H, Qin G, Witherspoon D, et al. Genetic evidence for high-altitude adaptation in Tibet. Science. (2010) 329:72–5. doi: 10.1126/science.1189406
69. Zhao Y, Zhang Z, Liu L, Zhang Y, Fan X, Ma L, et al. Associations of high altitude polycythemia with polymorphisms in EPAS1, ITGA6 and ERBB4 in Chinese Han and Tibetan populations. Oncotarget. (2017) 8:86736–46. doi: 10.18632/oncotarget.21420
70. Fan X, Ma L, Zhang Z, Li Y, Hao M, Zhao Z, et al. Associations of high-altitude polycythemia with polymorphisms in PIK3CD and COL4A3 in Tibetan populations. Hum Genom. (2018) 12:37. doi: 10.1186/s40246-018-0169-z
71. Zhou D, Udpa N, Ronen R, Stobdan T, Liang J, Appenzeller O, et al. Whole-genome sequencing uncovers the genetic basis of chronic mountain sickness in Andean highlanders. Am J Hum Genet. (2013) 93:452–62. doi: 10.1016/j.ajhg.2013.07.011
72. Sharma S, Koshy R, Kumar R, Mohammad G, Thinlas T, Graham B, et al. Hypobaric hypoxia drives selection of altitude-associated adaptative alleles in the Himalayan population. Sci Total Environ. (2024) 913:169605. doi: 10.1016/j.scitotenv.2023.169605
Keywords: excessive erythrocytosis, high altitude polycythemia, hypoxemia, maladaptive mechanisms, review
Citation: Tang S, Zhou W, Chen L, Yan H, Chen L and Luo F (2024) High altitude polycythemia and its maladaptive mechanisms: an updated review. Front. Med. 11:1448654. doi: 10.3389/fmed.2024.1448654
Received: 13 June 2024; Accepted: 12 August 2024;
Published: 27 August 2024.
Edited by:
Ahmet Emre Eskazan, Istanbul University-Cerrahpasa, TürkiyeReviewed by:
Sinan Mersin, Dr. Ersin Arslan Education and Research Hospital, TürkiyeCopyright © 2024 Tang, Zhou, Chen, Yan, Chen and Luo. This is an open-access article distributed under the terms of the Creative Commons Attribution License (CC BY). The use, distribution or reproduction in other forums is permitted, provided the original author(s) and the copyright owner(s) are credited and that the original publication in this journal is cited, in accordance with accepted academic practice. No use, distribution or reproduction is permitted which does not comply with these terms.
*Correspondence: Lei Chen, lchens@126.com; Hui Yan, yanhui@wchscu.com
†These authors have contributed equally to this work and share first authorship
Disclaimer: All claims expressed in this article are solely those of the authors and do not necessarily represent those of their affiliated organizations, or those of the publisher, the editors and the reviewers. Any product that may be evaluated in this article or claim that may be made by its manufacturer is not guaranteed or endorsed by the publisher.
Research integrity at Frontiers
Learn more about the work of our research integrity team to safeguard the quality of each article we publish.