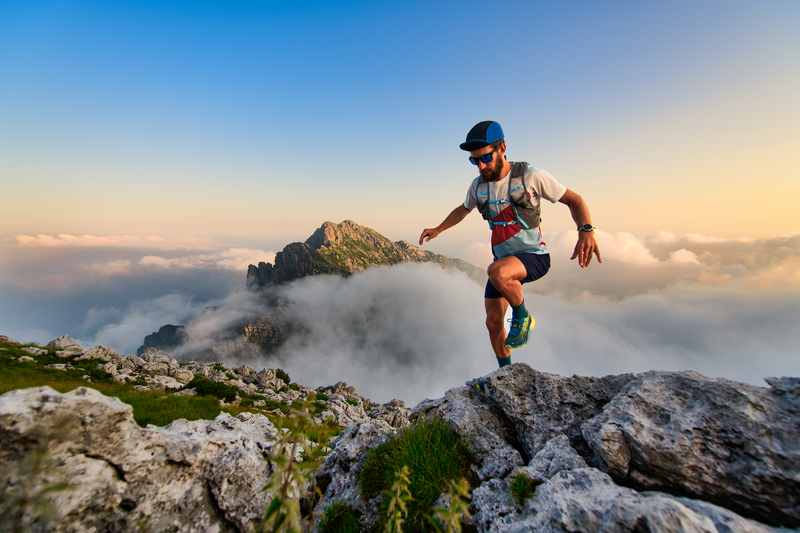
94% of researchers rate our articles as excellent or good
Learn more about the work of our research integrity team to safeguard the quality of each article we publish.
Find out more
CLINICAL TRIAL article
Front. Med. , 10 September 2024
Sec. Intensive Care Medicine and Anesthesiology
Volume 11 - 2024 | https://doi.org/10.3389/fmed.2024.1426969
This article is part of the Research Topic Personalized Therapy in ARDS - Volume II View all 8 articles
Background: The aim of this study is the evaluation of a closed-loop oxygen control system in pediatric patients undergoing invasive mechanical ventilation (IMV).
Methods: Cross-over, multicenter, randomized, single-blind clinical trial. Patients between the ages of 1 month and 18 years who were undergoing IMV therapy for acute hypoxemic respiratory failure (AHRF) were assigned at random to either begin with a 2-hour period of closed-loop oxygen control or manual oxygen titrations. By using closed-loop oxygen control, the patients' SpO2 levels were maintained within a predetermined target range by the automated adjustment of the FiO2. During the manual oxygen titration phase of the trial, healthcare professionals at the bedside made manual changes to the FiO2, while maintaining the same target range for SpO2. Following either period, the patient transitioned to the alternative therapy. The outcomes were the percentage of time spent in predefined SpO2 ranges ±2% (primary), FiO2, total oxygen use, and the number of manual adjustments.
Findings: The median age of included 33 patients was 17 (13–55.5) months. In contrast to manual oxygen titrations, patients spent a greater proportion of time within a predefined optimal SpO2 range when the closed-loop oxygen controller was enabled (95.7% [IQR 92.1–100%] vs. 65.6% [IQR 41.6–82.5%]), mean difference 33.4% [95%–CI 24.5–42%]; P < 0.001). Median FiO2 was lower (32.1% [IQR 23.9–54.1%] vs. 40.6% [IQR 31.1–62.8%]; P < 0.001) similar to total oxygen use (19.8 L/h [IQR 4.6–64.8] vs. 39.4 L/h [IQR 16.8–79]; P < 0.001); however, median SpO2/FiO2 was higher (329.4 [IQR 180–411.1] vs. 246.7 [IQR 151.1–320.5]; P < 0.001) with closed–loop oxygen control. With closed–loop oxygen control, the median number of manual adjustments reduced (0.0 [IQR 0.0–0.0] vs. 1 [IQR 0.0–2.2]; P < 0.001).
Conclusion: Closed-loop oxygen control enhances oxygen therapy in pediatric patients undergoing IMV for AHRF, potentially leading to more efficient utilization of oxygen. This technology also decreases the necessity for manual adjustments, which could reduce the workloads of healthcare providers.
Clinical Trial Registration: This research has been submitted to ClinicalTrials.gov (NCT05714527).
When it comes to the treatment of respiratory failure of any type, oxygen is a fantastic drug to use. The Pediatric Mechanical Ventilation Consensus Conference (PEMVECC) recommends that all ventilated children should have their peripheral oxygen saturation (SpO2) monitored using pulse oximetry, and that patients with moderate to severe conditions should have their partial arterial oxygen pressure (PaO2) measured (1). This is done in order to prevent hypoxemia and hyperoxemia. On the other hand, this may need the manual adjustment of inspired oxygen, which may be an annoyance during time periods of high demand, such as the current epidemic of COVID-19. Furthermore, the pandemic has brought to light the need of increasing oxygen utilization in hospitals. This is due to the fact that it is probable that oxygen might become a scarce resource during times of such high demand (2, 3). The monitoring of SpO2 is the fundamental metric that is used to guide the treatment of acute respiratory failure in patients of all ages, including neonates, children, and adults. All of the recommendations that are now in place include specific sections on SpO2 monitoring, as well as ranges that are often imprecise but are up for debate based on the severity of the condition and the patient's age (1, 4, 5).
Both hypoxemia and hyperoxemia are conditions that pediatric intensivists often want to steer clear of (6–9). This precaution is rooted in previous research data has shown that there is a connection between excess or insufficient oxygen utilization and mortality in pediatric intensive care unit patients who have received oxygen treatment (10–14). Although the partial pressure of oxygen in the arterial system (PaO2) and the saturation of arterial oxygen (SaO2) are usually the measures that are used in the process of titrating oxygen, it is sometimes challenging to keep track of these values in pediatric patients. Pulse oximetry, often known as SpO2, is a potentially appealing option since it provides the benefit of ongoing tracking.
On November 29, 2023, a search was conducted in Embase, MEDLINE, CINAHL, and Web of Science using the keywords “closed-loop” or “automatic” and “oxygen” or “oxygen therapy.” There were no constraints placed on the search based on the publication date or language. The search resulted in the identification of 45 clinical investigations, of which 38 were randomized clinical trials. According to the findings of all of the investigations, SpO2 may be used by closed-loop oxygen systems in order to automatically modify the FiO2. The majority of these studies were conducted in neonates, with the remaining focus on adults; however, only two studies were carried out in pediatric patients. None of these research, on the other hand, investigated the effects of closed-loop oxygen regulation in pediatric patients while they were undergoing different modes of mechanical ventilation (15–56).
There is a current gap in research evaluating the effectiveness and safety of closed-loop oxygen systems in pediatric patients undergoing invasive mechanical ventilation for acute hypoxemic respiratory failure (AHRF), regardless of the applied ventilation mode. To address this gap, we conducted a randomized crossover study aimed at assessing the performance of a closed-loop oxygen control system integrated into a mechanical ventilator concerning the quality of oxygen therapy in pediatric patients. Our investigation also encompassed an evaluation of safety, determination of total oxygen consumption, and a comparison of manual adjustments between closed-loop oxygen control and manual oxygen titration. Our hypothesis postulated that the utilization of this closed-loop oxygen system would result in an increased duration within predefined optimal SpO2 ranges.
This study adopts a multicentre, single-blinded, randomized, crossover design to compare closed-loop oxygen control with manual oxygen titrations in the pediatric patient population across four medical facilities in Turkey. The eligible participants were carefully screened for inclusion in the PICUs at Dr Behcet Uz Children's Research and Training Hospital in Izmir, Aydin Obstetrics and Children Hospital in Aydin, Erzurum Territorial Training and Research Hospital in Erzurum, and Cam Sakura Research and Training Hospital in Istanbul. The enrolment period spanned from June 2022 to October 2022. Ethical approval was obtained from the Institutional Ethical Committee (Approval ID: 750/2022/29-09), and the study adhered to the principles outlined in the Declaration of Helsinki. Registration details for this study can be found on ClinicalTrials.gov (study identifier NCT05714527). Also the protocol including statistical plan was published online (57).
Patients were eligible if they were (1) aged 1 month to 18 years and (2) receiving IMV with FiO2 > 25% to maintain SpO2 within clinician-defined parameters. (3) We excluded patients who had diseases or conditions that could potentially impact the measurement of transcutaneous SpO2, such as chronic or acute dyshemoglobinemia (including methemoglobinemia), carbon monoxide (CO) poisoning, and sickle cell disease. Additionally, we excluded patients who required a continuous infusion of epinephrine or norepinephrine at rates exceeding 1 mg/h. The exclusion criteria for this study included patients who had an immediate need for non-invasive ventilation or high-flow oxygen therapy whether foreseeable or unforeseeable, those with poor quality SpO2 measurements using finger and ear sensors, individuals with severe acidosis, pregnant women, patients at high risk for needing non-invasive mechanical ventilation or transportation to another unit or hospital, those with a formalized ethical decision to withhold or withdraw life support, patients participating in another research study, and patients who had previously been enrolled in the study during a previous episode of acute respiratory failure. The establishment of these criteria was aimed at guaranteeing the study's integrity and dependability, as well as the safety and wellbeing of the participants.
Participants who were already intubated and receiving IMV due to the natural course and treatment of their disease, were assigned randomly to either begin with a 2-h session of closed-loop oxygen control or a 2-h session of manual oxygen titration. Subsequently, patients were transitioned to the alternative treatment. The randomization was conducted in a 1:1 ratio, using blocks of 4 and sealed opaque envelopes. The intervention design precluded the possibility of blinding healthcare professionals. Nonetheless, patients remained blinded about the specific techniques employed to regulate their oxygen levels.
The patients underwent intubation using an endotracheal tube of appropriate size, which was inserted properly. Throughout the trial, the patients were maintained in a semi–recumbent position. Invasive mechanical ventilation was performed using a pediatric ventilator which included a closed-loop oxygen controller (Hamilton–C1 or C6, Hamilton Medical AG, Bonaduz, Switzerland). Patients were administered sedatives as required, achieving an appropriate amount of sedation for each individual. The amount of sedation-analgesia remained constant during the whole course of the study. Continuous patient care and routine tasks, such as suctioning secretions or providing nutrition, were carried out without interruption, and randomly throughout both periods. At the research locations, the doctor to patient ratios during daytime and night-time shifts were roughly 6:1 and 12:1, respectively. Similarly, the nurse to patient ratios were around 2:1 and 3:1 during daytime and night-time shifts, respectively. The overall setting remained equivalent throughout the trial, meaning that these ratios did not alter between the two crossover periods. Furthermore, there was an absence of additional research staff throughout these two phases.
Following randomization, the attending pediatric intensivist determined the optimum range of oxygen saturation (SpO2) for each patient, taking into account their specific clinical condition and medical background. The term “optimal SpO2 target” does not represent a universal optimal for all patients. Instead, the optimal range is the ideal SpO2 level tailored to the patient's particular condition, taking into account factors such as lung compliance, driving pressure (ΔP), plateau pressure (Pplat), and positive end-expiratory pressure (PEEP). This approach ensures sufficient oxygenation while mitigating the risks associated with excessively high or low oxygen levels. Prior to moving on to the second 2-h session using the alternative oxygen titration approach, a 30-min washout interval was instituted after the first 2 h with the initial oxygen titration approach (Supplementary Figure 1). By using closed-loop oxygen control, the patients' SpO2 levels were maintained within a predetermined target range by the automated adjustment of the FiO2. During the manual oxygen titration phase of the trial, healthcare professionals at the bedside made manual changes to the FiO2, while maintaining the same target range for SpO2. For the two crossover stages of the study, the ventilation settings were unchanged. The SpO2 target range was established by determining four thresholds: an upper and lower threshold for the “optimum” range, and an upper and lower threshold for the “suboptimal” range. The optimum thresholds ranged from 94% to 98%, 93% to 97%, 92% to 96%, or 88% to 92%. The respective suboptimal thresholds were reported in Supplementary Table 1. The operational concepts of the closed-loop control are elaborated in Supplementary Table 2 and in the protocol paper (57).
Case report forms (CRFs) were used to record clinical and epidemiologic data. Using the ventilator's RS-232 interface connector, a Memory Box (Hamilton Medical AG) was attached to record ventilation data, including FiO2, SpO2, waveforms, alarms, and manual titrations, breath by breath. Patients' SpO2 was meticulously monitored using a Masimo Set sensor, specifically the Masimo RD model (Masimo Corp., Irvine, CA, USA), attached to their finger. This sensor provided the signal utilized by the closed-loop controller to ensure precise and automated FiO2 adjustments.
Each SpO2 reading was categorized as either optimal if it fell within the patient's predetermined range, suboptimal high or low if it fell outside of the optimal range but still within the suboptimal cut-offs, or unacceptable if it fell outside of the suboptimal range (Supplementary Table 1).
The main aim of the research was to evaluate the efficacy of closed-loop oxygen control in the context of invasive ventilation for pediatric patients. Hence, the main objective was to determine the percentage of time spent within certain predetermined SpO2 goal ranges throughout each 2-h interval. The secondary outcomes included the percentage of time spent in suboptimal and unacceptable SpO2 ranges, the FiO2 and SpO2/FiO2 ratio, the frequency of manual oxygen changes, and the number of alarms.
The goal of the research was to assess the effectiveness of closed-loop oxygen control in the context of invasive ventilation for pediatric patients. Hence, we selected the primary objective to ascertain the proportion of time spent within predetermined SpO2 goal ranges during each 2-h interval. Secondary outcomes encompassed the percentage of time spent in suboptimal and unacceptable SpO2 ranges, the FiO2 and PaO2/FiO2 ratio, the frequency of manual oxygen adjustments, and the number of alarms. Furthermore, we evaluated the percentage of time with an available SpO2 signal to gauge the reliability of the oxygen monitoring system. Additionally, the study examined total oxygen consumption to provide insights into overall oxygen utilization during the research period.
The determination of the sample size was based on data recorded from the pilot study involving seven patients (7 × 4 h = 28 h). This initial investigation aimed to assess the same disparity in the percentage of time allocated to optimal SpO2 target ranges during closed-loop vs. manual oxygen titrations. Utilizing the data from this pilot study, G*Power analysis indicated the necessity of including an additional 32 patients to achieve a statistical power of 95% (with a two-tailed α of 0.05) for detecting an effect size of Cohen's d = 0.68 in a Wilcoxon signed–rank test (58).
To accommodate potential dropouts, defined as instances where patients required extubation or noninvasive ventilation support, withdrew consent, experienced poor SpO2 readings for over 1 h during either study phase, or encountered technical recording issues, we opted for a final sample size of 35 patients.
The normality of data distribution was assessed using Shapiro–Wilk, skewness, and kurtosis tests. Continuous data were presented as mean and standard deviation (SD) or median and interquartile range [IQR], depending on the nature of the distribution.
Statistical analysis involved the use of either a paired samples t-test or Wilcoxon test, selected based on appropriateness for the data. Specifically, the Wilcoxon signed–rank test was employed to compare the percentage of time spent within the target SpO2 range with manual FiO2 adjustments against the percentage with closed–loop FiO2 control.
A significance level of <0.05 was deemed statistically meaningful for all comparisons. Data were processed using MATLAB (version 2021b) by The MathWorks, Inc., Natick, Massachusetts, United States, while XLSTAT (version 2016) by Addinsoft, Paris, France, was utilized for statistical testing. Visual representations were generated using JASP (version 2022) by the JASP Team, Amsterdam, The Netherlands, and GraphPad PRISM (version 9) in San Diego, California, USA.
Between June 2023 and December 2023, a total of 206 patients underwent screening. Out of these, 81 patients were found to be eligible, but 46 of them were excluded due to meeting one or more exclusion criteria. Ultimately, 35 patients were included in the study, with 2 patients dropping out. Therefore, a total of 33 patients were analyzed (Figure 1). Table 1 displays basic characteristics. The majority of patients were under 1.5 years old, weighed <15 kilograms, and in nearly half of the cases, AHRF was caused by a respiratory infection.
When the oxygen controller was enabled, patients spent a significantly higher amount of time within the ideal SpO2 ranges compared to manual oxygen titrations (95.7% [IQR 92.1%−100%] vs. 65.6% [IQR 41.6%−82.5%], mean difference 33.4% [95%–CI 24.5 to 42]); P < 0.001) (Table 2; Figures 2, 3).
Figure 3. Effect of closed-loop control on time spent in optimum SpO2 zone, total oxygen use, manual FiO2 adjustments and SpO2/FiO2. The left panels collectively illustrate the effectiveness of the closed-loop system compared to manual adjustments across several parameters. The time spent in the optimum SpO2 zone is generally higher and more consistent with the closed-loop system, as indicated by the individual data points and box plots. Additionally, total oxygen use is lower with the closed-loop system, reflecting its efficiency in oxygen utilization. The need for manual FiO2 adjustments is significantly reduced when using the closed-loop system, highlighting its automation advantage. Furthermore, the SpO2/FiO2 ratio is higher and more stable with the closed-loop system, demonstrating better oxygenation efficiency. These findings suggest that the closed-loop system provides superior control and management of oxygen levels in patients. The right panels depict the differences between the closed-loop and manual methods for each parameter through density plots and scatter plots. For time spent in the optimum SpO2 zone, the closed-loop system shows a higher and more consistent distribution compared to manual adjustments. The total oxygen use is clearly lower with the closed-loop system, indicating its greater efficiency. The need for manual FiO2 adjustments is considerably fewer with the closed-loop system, as shown by the shift toward fewer adjustments. Lastly, the SpO2/FiO2 ratio is maintained at a higher level with the closed-loop system, reflecting better overall oxygenation. These visualizations confirm the advantages of the closed-loop system in providing efficient and effective oxygen management.
Upon activating the oxygen controller, patients spent considerably less time in the total unacceptably and suboptimal SpO2 ranges (Table 2, Figure 2). Also, this activation significantly reduced the duration patients were exposed to SpO2 levels considered unacceptably high and sub-optimally high (Table 2, Figure 2).
The adoption of closed-loop oxygen controller led to a significant reduction in both the mean fraction of inspired oxygen (FiO2) and total oxygen utilization (Table 2). Moreover, the SpO2/FiO2 ratio was significantly increased under the closed-loop oxygen controller (Table 2; Figure 3). Closed-loop controller also markedly decreased the frequency of manual interventions required (Table 2; Figures 2, 3).
This multicenter randomized controlled crossover trial, focusing on pediatric patients treated with invasive mechanical ventilation for AHRF, reveals the following outcomes: The implementation of a closed-loop oxygen controller within a mechanical ventilator, as opposed to manual oxygen titration, brought about numerous significant benefits: The closed-loop system substantially increased the time that patients' SpO2 levels remained what we pre-defined as the optimal range. This precise regulation ensures that patients receive oxygen to maintain a reasonable range of oxygenation and avoid hypoxemia and hyperoxia. The closed-loop controller effectively reduced the periods during which patients' SpO2 levels were outside the physician predefined optimal range. This reduction in time spent at suboptimal levels means that patients are less likely to experience the adverse effects associated with inadequate or excessive oxygenation. The system's ability to swiftly respond to changes in the patient's condition ensures a higher level of care and reduces potential complications. One of the standout advantages of the closed-loop system is its efficiency in oxygen use. By delivering oxygen more precisely and only when necessary, the system reduces overall oxygen consumption. This not only has economic benefits but also lessens the burden on oxygen supply systems, making it particularly valuable in resource-limited settings. The meticulous management of FiO2 means that no excess oxygen is wasted, contributing to more sustainable healthcare practices. The closed-loop oxygen controller significantly lowered the need for manual adjustments by healthcare providers. This automation allows medical staff to focus more on other critical aspects of patient care, enhancing overall efficiency within the clinical environment. The reduction in manual intervention also means that there is less room for human error, thereby improving the safety and reliability of oxygen therapy. This feature is particularly beneficial in busy or understaffed medical settings, where it can greatly enhance the quality of patient management. In summary, the introduction of a closed-loop oxygen controller in mechanical ventilators offers a transformative approach to oxygen therapy, ensuring precise, efficient, and safe management of SpO2 levels. Its ability to maintain optimal oxygen saturation, reduce suboptimal periods, lower oxygen consumption, and minimize manual interventions makes it a superior alternative to traditional manual oxygen titration methods. Our research has several benefits, both conceptually and in terms of execution. We used a crossover approach to compare the effectiveness of closed-loop oxygen control and manual oxygen titrations for each participant, making our findings statistically more robust. We conducted the study across multiple centers, including universities and teaching hospitals, to increase the generalizability of our results. We adhered to a published protocol and used randomization mechanisms to minimize the risk of bias. Additionally, we established an analytic strategy before finalizing the database, which involved predefining optimal, suboptimal, and unacceptable SpO2 values based on previous consensus. This ensured the objectivity and reliability of our results. To the extent of our knowledge, this study is the inaugural effort to examine the effectiveness of closed-loop oxygen management in pediatric patients receiving invasive mechanical ventilation, regardless of ventilation mode reliance.
The results of our investigation are consistent with previous research studies that have evaluated the efficacy of closed-loop oxygen regulation in groups of premature infants, pediatric and adult patients undergoing either invasive or non-invasive mechanical ventilation for acute respiratory failure caused by various factors (15–56, 59–67).
Throughout these investigations, closed-loop oxygen control consistently exhibited superior efficacy compared to manual oxygen titration by healthcare personnel. This was demonstrated by improved adherence to target peripheral oxygen saturation (SpO2) ranges and decreased duration spent within potentially dangerous SpO2 levels. Our study enhances the current understanding of the effectiveness of closed-loop oxygen control in pediatric patients with acute hypoxemic respiratory failure (AHRF). It clarifies that closed-loop oxygen control is superior to manually adjusting the FiO2 in cases where there is clinical instability. This demonstrated superiority is particularly significant because to its potential to alleviate the onerous tasks carried by healthcare workers in the intensive care unit (ICU), whose workload is sometimes exacerbated by the need of patient stabilization (68, 69).
Our findings are also consistent with previous studies investigating closed-loop oxygen controllers in invasively ventilated neonates (19, 59–61). Similar results have been reported in the pediatric population (53) and adult patients (62–67). This body of research demonstrates the superiority of closed-loop oxygen control compared to manual titration methods in patients receiving respiratory support. Notably, oxygenation in these patients is influenced not only by FiO2, but also by factors such as delivered tidal volumes and airway pressures. Collectively, these findings suggest a broad applicability of closed-loop oxygen control for critically ill hypoxemic patients receiving various forms of respiratory support. This includes both passive and active breathers, too. Not all of our patients were under assisted mechanical ventilation; some were in a passive state. This variation in patient ventilation modes could be perceived as a limitation of our study, as it introduces a level of heterogeneity that might affect the generalizability of our findings. Additionally, the generalizability of our results is limited to similar settings, and this should be duly noted. It is widely recognized that medical professionals specializing in intensive care, including physicians and nurses, diligently prevent both hypoxemia and hyperoxemia due to numerous justifiable concerns. This approach is especially pertinent among healthcare providers who manage critically ill neonatal and pediatric patients (6–9). This strategy necessitates not only proficient health care providers but also a significant number of intensive care unit (ICU) staff directly attending to the patient. Minimizing hypoxemia can only be achieved if there is a consistent presence of a nurse who can do manual oxygen adjustments (59, 60). This condition can be deemed both unfeasible and costly, and may not be regularly fulfilled.
Recent investigations, including an older Canadian study and a more recent one from the Netherlands, have highlighted an asymmetrical approach by physicians in managing SpO2 levels beyond the optimal zones. While physicians strive to avert both hypoxemia and hyperoxemia, there is a greater focus on preventing hypoxemia. Consequently, this often leads to extended periods within suboptimal or high SpO2 ranges when manually adjusting oxygen levels (70, 71). In contrast, the implementation of a closed-loop oxygen control system in our experimental arm, demonstrates its efficacy by equally preventing deviations into both lower and higher SpO2 ranges, thus maintaining a more stable patient condition.
The observation that SpO2/FiO2 ratios were greater in the context of closed-loop oxygen control indicates that this method not only averts hypoxemic and hyperoxemic deterioration, but also enhances overall oxygenation. Concurrently, it achieves the same or better levels of oxygenation with reduced oxygen consumption, which might be crucial in contexts with limited resources or during times of increased demand, such as a pandemic. This finding aligns with several previous studies that have shown patients receiving closed-loop control of FiO2 got a lower quantity of FiO2 than those who underwent manual titration (51, 53, 60, 62, 63, 72). Moreover, the utility of automatizing oxygen delivery is especially significant in low- and middle-income countries (LMICs), where the lack of personnel and the high consumption of oxygen are prominent challenges. Automated systems can ensure consistent and precise oxygen delivery, thereby alleviating the burden on limited healthcare staff and conserving essential oxygen supplies. Even in high-resource countries, replacing the nursing workforce at the bedside has become increasingly difficult post-pandemic. The implementation of closed-loop oxygen controllers can therefore play a pivotal role in both high- and low-resource settings by optimizing oxygen use and reducing the dependency on manual titration, ultimately enhancing patient care and resource management.
We selected the same SpO2 target range for both the closed-loop group and the manual group. However, in the control arm, patients spent more time in the suboptimal high SpO2 range, indicating that the mean SpO2 was higher during the manual titration period. This discrepancy in SpO2/FiO2 ratios could potentially be attributed to denitrogenation atelectasis, as more than 25% of the patients in the manual arm were exposed to FiO2 levels exceeding 60% at some point during the study (73). Nevertheless, we lack concrete evidence to substantiate the occurrence of this type of atelectasis within our study group. Moreover, no significant changes were observed in driving pressure or tidal volume (TV) during the two phases of the study to support this hypothesis.
Surprisingly, we saw far fewer manual adjustments, yet a statistically significant reduction in the number of hourly alarms with closed-loop oxygen regulation. This may potentially result in decreased workloads, since our research indicates that the implementation of a closed-loop oxygen controller requires trained healthcare provider adjustments less than manual oxygen titration (74). Increased ICU staff workloads are correlated with higher mortality rates. Furthermore, our findings indicate a modification in the intensity of alarms toward a more tolerable level, thereby enhancing patient comfort and sleep hygiene while concurrently mitigating the likelihood of delirium (75, 76).
The study reported here has certain limitations that should be taken into account. Initially, the time allocated for both the manual and automated oxygen titration procedures was limited to a mere 2 h, which is not enough to cover the whole spectrum of everyday activities that patients go through. The restricted duration was selected to ensure uniformity in patient circumstances throughout both phases of the crossover trial, which is critical due to the fast fluctuations that may occur in pediatric patients. In addition, both stages were intentionally planned to be carried out inside a single shift, which unavoidably limits the thorough examination of each case within these time periods.
The crossover design of the study also restricts our ability to assess the effects of closed-loop oxygen control on pertinent clinical outcomes, such as the duration of invasive mechanical ventilation, the shift from invasive to non-invasive ventilation, and the process of gradually reducing respiratory support for patients. Furthermore, the nature of the intervention precluded the blinding of healthcare workers involved in the study. Nevertheless, in order to adhere to regular clinical procedures, predetermined SpO2 zones were used, mirroring the zones to which ICU nurses often adapt FiO2.
We did not include patients with skin types darker than Fitzpatrick scale 4 in this study. However, we acknowledge that there is a significant difference in melanin levels between Fitzpatrick skin types 1–2 and type 4. Consequently, it is possible that adjustments for skin pigmentation could be necessary even within the range of Fitzpatrick skin types included in our study. This represents a limitation of our study, as the continuum of melanin levels across the full Fitzpatrick scale, or more accurately, the Monk Skin Tone Scale, should be considered to better account for variations in skin pigmentation in future research It is important to note that pulse oximetry can overestimate true SaO2 in individuals with darker skin tones. While the clinical relevance of this bias remains unclear, its magnitude is likely to be more significant when SaO2 is lower (77). Consequently, future research should take into account skin color when defining personal optimal targets to ensure accuracy and efficacy in oxygen therapy across diverse patient populations.
Future research should prioritize investigating these clinical goals to improve comprehension of the consequences of closed-loop oxygen titration approaches in pediatric intensive and emergency care.
In many liberal vs. limited oxygen trials, such as the most recent OXY-PICU trial, researchers have struggled to maintain low SpO2 levels in the restricted arm. Although the restricted arm in the OXY-PICU study aimed for an SpO2 level of 88 to 92%, they could only achieve a median SpO2 of 94% (IQR 93–96) for the conservative group, compared to 97% (96–98) for the liberal oxygenation group (78). Therefore, closed-loop oxygen controllers may play a very important potential role in these types of studies in the future, ensuring more precise maintenance of target SpO2 levels. One significant limitation of our study is the use of the terms “optimal” and “ideal oxygenation” without sufficient nuance. Currently, there is insufficient data to definitively determine what constitutes ideal or optimal oxygenation. Furthermore, the impact of different oxygenation levels on clinical outcomes has not been evaluated in this study. Future research is needed to establish clear guidelines and evidence-based practices for optimal oxygenation to improve patient outcomes. Until such data are available, the terms “optimal” and “ideal” should be interpreted with caution, and our findings should be viewed as preliminary in this context.
In conclusion, when evaluating the efficacy of closed-loop oxygen control vs. manual oxygen titrations in pediatric patients undergoing invasive mechanical ventilation for AHRF, significant benefits are observed with the closed-loop system. Notably, it enhances the duration that patients remain within optimal SpO2 ranges and diminishes the periods spent in SpO2 zones that may pose risks. Furthermore, closed-loop oxygen control not only improves overall oxygenation but also conserves oxygen resources and reduces the necessity for manual adjustments. These findings suggest that implementing closed-loop systems could offer substantial clinical advantages in managing pediatric AHRF.
The raw data supporting the conclusions of this article will be made available by the authors, without undue reservation.
The studies involving humans were approved by Institutional Review Board, Dr. Behcet Uz Children's Research and Training Hospital, Izmir, Turkey. The studies were conducted in accordance with the local legislation and institutional requirements. Written informed consent for participation in this study was provided by the participants' legal guardians/next of kin.
GA: Conceptualization, Data curation, Formal analysis, Funding acquisition, Investigation, Methodology, Supervision, Validation, Writing – original draft, Writing – review & editing. GC: Conceptualization, Data curation, Formal analysis, Funding acquisition, Investigation, Methodology, Project administration, Resources, Software, Supervision, Validation, Visualization, Writing – original draft, Writing – review & editing. OS: Conceptualization, Data curation, Formal analysis, Funding acquisition, Investigation, Methodology, Validation, Writing – original draft, Writing – review & editing. ES: Conceptualization, Data curation, Formal analysis, Validation, Writing – original draft, Writing – review & editing. PH: Data curation, Investigation, Methodology, Writing – original draft, Writing – review & editing. MC: Investigation, Methodology, Validation, Writing – original draft, Writing – review & editing. JZ: Software, Validation, Visualization, Writing – original draft, Writing – review & editing, Data curation. DN: Conceptualization, Project administration, Writing – original draft, Writing – review & editing. UK: Writing – original draft, Writing – review & editing. ST: Investigation, Writing – original draft, Writing – review & editing. HA: Conceptualization, Formal analysis, Funding acquisition, Project administration, Resources, Supervision, Writing – original draft, Writing – review & editing.
The author(s) declare financial support was received for the research, authorship, and/or publication of this article. The participating centers received only material support from Hamilton Medical AG, Bonaduz, Switzerland. They received mechanical ventilators and memory boxes for collecting the respiratory support data for the duration of this study.
We thank the trial participants, their families, and all investigators involved in this study.
GC, DN, and JZ works at Hamilton Medical AG in the Department of Medical Research.
The remaining authors declare that the research was conducted in the absence of any commercial or financial relationships that could be construed as a potential conflict of interest.
All claims expressed in this article are solely those of the authors and do not necessarily represent those of their affiliated organizations, or those of the publisher, the editors and the reviewers. Any product that may be evaluated in this article, or claim that may be made by its manufacturer, is not guaranteed or endorsed by the publisher.
The Supplementary Material for this article can be found online at: https://www.frontiersin.org/articles/10.3389/fmed.2024.1426969/full#supplementary-material
Supplementary Figure 1 | Trial flow diagram.
Supplementary Table 1 | SpO2 predefined targets.
Supplementary Table 2 | Principles of Closed-loop oxygen controller.
1. Kneyber MCJ, de Luca D, Calderini E, Jarreau P-H, Javouhey E, Lopez-Herce J, et al. Recommendations for mechanical ventilation of critically ill children from the Paediatric Mechanical Ventilation Consensus Conference (PEMVECC). Intensive Care Med. (2017) 43:1764–80. doi: 10.1007/s00134-017-4920-z
2. Shrestha GS, Lamsal R. Rational Use of Oxygen in COVID-19 Pandemic - Are We Doing Enough? JNMA J Nepal Med Assoc. (2021) 59:429–31. doi: 10.31729/jnma.6479
3. Sindwani G, Suri A. Acute hospital oxygen shortage during COVID-19 pandemic surge: how can we prevent the apocalypse? Brazilian J Anesthesiol. (2022) 72:311–2. doi: 10.1016/j.bjane.2021.10.003
4. Group TPALICC. Pediatric acute respiratory distress syndrome: consensus recommendations from the pediatric acute lung injury consensus conference*. Pediatr Crit Care Med. (2015) 16:428–39. doi: 10.1097/PCC.0000000000000350
5. Emeriaud G, López-Fernández YM, Iyer NP, Bembea MM, Agulnik A, Barbaro RP, et al. Executive summary of the second international guidelines for the diagnosis and management of pediatric acute respiratory distress syndrome (PALICC-2). Pediatr Crit Care Med. (2023) 24:143–68.
6. Singer M, Young PJ, Laffey JG, Asfar P, Taccone FS, Skrifvars MB, et al. Dangers of hyperoxia. Critical Care. (2021) 25:440. doi: 10.1186/s13054-021-03815-y
7. Palmer E, Post B, Klapaukh R, Marra G, MacCallum NS, Brealey D, et al. The association between supraphysiologic arterial oxygen levels and mortality in critically ill patients. A multicenter observational cohort study. Am J Respir Crit Care Med. (2019) 200:1373–80. doi: 10.1164/rccm.201904-0849OC
8. de Jonge E, Peelen L, Keijzers PJ, Joore H, de Lange D, van der Voort PH, et al. Association between administered oxygen, arterial partial oxygen pressure and mortality in mechanically ventilated intensive care unit patients. Crit Care. (2008) 12:R156. doi: 10.1186/cc7150
9. Young PJ, Hodgson CL, Rasmussen BS. Oxygen targets. Intensive Care Med. (2022). doi: 10.1007/s00134-022-06714-0
10. Pelletier JH, Ramgopal S, Au AK, Clark RSB, Horvat CM. Maximum Pao2 in the first 72 hours of intensive care is associated with risk-adjusted mortality in pediatric patients undergoing mechanical ventilation. Crit Care Explor. (2020) 2:e0186. doi: 10.1097/CCE.0000000000000186
11. Peters MJ, Jones GA, Wiley D, Wulff J, Ramnarayan P, Ray S, et al. Conservative versus liberal oxygenation targets in critically ill children: the randomised multiple-centre pilot Oxy-PICU trial. Intensive Care Med. (2018) 44:1240–8. doi: 10.1007/s00134-018-5232-7
12. Page D, Ablordeppey E, Wessman BT, Mohr NM, Trzeciak S, Kollef MH, et al. Emergency department hyperoxia is associated with increased mortality in mechanically ventilated patients: a cohort study. Crit Care. (2018) 22:9. doi: 10.1186/s13054-017-1926-4
13. Stenson BJ, Tarnow-Mordi WO, Darlow BA, Simes J, Juszczak E, Askie L. Oxygen saturation and outcomes in preterm infants. N Engl J Med. (2013) 368:2094–104.
14. Lilien TA, Groeneveld NS, van Etten-Jamaludin F, Peters MJ, Buysse CM, Ralston SL, et al. Association of arterial hyperoxia with outcomes in critically ill children: a systematic review and meta-analysis. JAMA Netw Open. (2022) 5:e2142105. doi: 10.1001/jamanetworkopen.2021.42105
15. Mitra S, Singh B, El-Naggar W, McMillan DD. Automated versus manual control of inspired oxygen to target oxygen saturation in preterm infants: a systematic review and meta-analysis. J Perinatol. (2018) 38:351–60. doi: 10.1038/s41372-017-0037-z
16. Reynolds PR, Miller TL, Volakis LI, Holland N, Dungan GC, Roehr CC, et al. Randomised cross-over study of automated oxygen control for preterm infants receiving nasal high flow. Arch Dis Child Fetal Neonatal Ed. (2019) 104:F366–f71. doi: 10.1136/archdischild-2018-315342
17. Waitz M, Schmid MB, Fuchs H, Mendler MR, Dreyhaupt J, Hummler HD. Effects of automated adjustment of the inspired oxygen on fluctuations of arterial and regional cerebral tissue oxygenation in preterm infants with frequent desaturations. J Pediatr. (2015) 166:240–4.e1. doi: 10.1016/j.jpeds.2014.10.007
18. Bourassa S, Bouchard P-A, Dauphin M, Lellouche F. Oxygen conservation methods with automated titration. Respir Care. (2020) 65:1433–42. doi: 10.4187/respcare.07240
19. Kaam AH, Hummler HD, Wilinska M, Swietlinski J, Lal MK, Pas AB. Automated versus manual oxygen control with different saturation targets and modes of respiratory support in preterm infants. J Pediatr. (2015) 167:545–50. doi: 10.1016/j.jpeds.2015.06.012
20. Lal M, Tin W, Sinha S. Automated control of inspired oxygen in ventilated preterm infants: crossover physiological study. Acta Paediatr. (2015) 104:1084–9. doi: 10.1111/apa.13137
21. Dijkman KP, Mohns T, Dieleman JP, van Pul C, Goos TG, Reiss IK, et al. Predictive Intelligent Control of Oxygenation (PRICO) in preterm infants on high flow nasal cannula support: a randomised cross-over study. Arch Dis Childh Fetal Neonatal. (2021) 106:621–6. doi: 10.1136/archdischild-2020-320728
22. Dani C, Pratesi S, Luzzati M, Petrolini C, Montano S, Remaschi G, et al. Cerebral and splanchnic oxygenation during automated control of inspired oxygen (FiO2) in preterm infants. Pediatr Pulmonol. (2021) 56:2067–72. doi: 10.1002/ppul.25379
23. Das A, Mhanna M, Teleron-Khorshad A, Houdek J, Kumar N, Gunzler D, et al. A comparison of manual versus automated saturation of peripheral oxygenation in the neonatal intensive care unit. J Matern Fetal Neonatal Med. (2016) 29:1631–5. doi: 10.3109/14767058.2015.1057493
24. Dargaville PA, Fathabadi OS, Plottier GK, Lim K, Wheeler KI, Jayakar R, et al. Development and preclinical testing of an adaptive algorithm for automated control of inspired oxygen in the preterm infant. Arch Dis Child Fetal Neonatal Ed. (2017) 102:F31–f6. doi: 10.1136/archdischild-2016-310650
25. Zapata J, Gómez JJ, Araque Campo R, Matiz Rubio A, Sola A. A randomised controlled trial of an automated oxygen delivery algorithm for preterm neonates receiving supplemental oxygen without mechanical ventilation. Acta Paediatr. (2014) 103:928–33. doi: 10.1111/apa.12684
26. Van Zanten HA, Kuypers K, Stenson BJ, Bachman TE, Pauws SC, Te Pas AB. The effect of implementing an automated oxygen control on oxygen saturation in preterm infants. Arch Dis Child Fetal Neonatal Ed. (2017) 102:F395–f9. doi: 10.1136/archdischild-2016-312172
27. Sturrock S, Ambulkar H, Williams EE, Sweeney S, Bednarczuk NF, Dassios T, et al. A randomised crossover trial of closed loop automated oxygen control in preterm, ventilated infants. Acta Paediatr. (2021) 110:833–7. doi: 10.1111/apa.15585
28. Kaltsogianni O, Dassios T, Greenough A. Does closed-loop automated oxygen control reduce the duration of mechanical ventilation? A randomised controlled trial in ventilated preterm infants. Trials. (2022) 23:276. doi: 10.1186/s13063-022-06222-y
29. Morozoff E, Smyth JA, Saif M. Applying computer models to realize closed-loop neonatal oxygen therapy. Anesth Analg. (2017) 124:95–103. doi: 10.1213/ANE.0000000000001367
30. Gajdos M, Waitz M, Mendler MR, Braun W, Hummler H. Effects of a new device for automated closed loop control of inspired oxygen concentration on fluctuations of arterial and different regional organ tissue oxygen saturations in preterm infants. Arch Dis Child Fetal Neonatal Ed. (2019) 104:F360–f5. doi: 10.1136/archdischild-2018-314769
31. Schwarz CE, Kidszun A, Bieder NS, Franz AR, König J, Mildenberger E, et al. Is faster better? A randomised crossover study comparing algorithms for closed-loop automatic oxygen control. Arch Dis Child Fetal Neonatal Ed. (2020) 105:369–74. doi: 10.1136/archdischild-2019-317029
32. Dargaville PA, Marshall AP, Ladlow OJ, Bannink C, Jayakar R, Eastwood-Sutherland C, et al. Automated control of oxygen titration in preterm infants on non-invasive respiratory support. Arch Dis Child Fetal Neonatal Ed. (2022) 107:39–44. doi: 10.1136/archdischild-2020-321538
33. Ali SK, Jayakar RV, Marshall AP, Gale TJ, Dargaville PA. Preliminary study of automated oxygen titration at birth for preterm infants. Arch Dis Child Fetal Neonatal Ed. (2022). doi: 10.1136/archdischild-2021-323486
34. Plottier GK, Wheeler KI, Ali SKM, Fathabadi OS, Jayakar R, Gale TJ, et al. Clinical evaluation of a novel adaptive algorithm for automated control of oxygen therapy in preterm infants on non-invasive respiratory support. Arch Dis Child Fetal Neonatal Ed. (2017) 102:F37–f43. doi: 10.1136/archdischild-2016-310647
35. Salverda HH, Cramer SJ, Witlox RS, Gale TJ, Dargaville PA, Pauws SC, et al. Comparison of two devices for automated oxygen control in preterm infants: a randomised crossover trial. Arch Dis Child Fetal Neonatal Ed. (2022) 107:20–5. doi: 10.1136/archdischild-2020-321387
36. Schwarz CE, Kreutzer KB, Langanky L, Wolf NS, Braun W, O'Sullivan MP, et al. Randomised crossover trial comparing algorithms and averaging times for automatic oxygen control in preterm infants. Arch Dis Child Fetal Neonatal Ed. (2021) 107:425–30. doi: 10.1136/archdischild-2021-322096
37. Roca O, Caritg O, Santafé M, Ramos FJ, Pacheco A, García-de-Acilu M, et al. Closed-loop oxygen control improves oxygen therapy in acute hypoxemic respiratory failure patients under high flow nasal oxygen: a randomized cross-over study (the HILOOP study). Crit Care. (2022) 26:108. doi: 10.1186/s13054-022-03970-w
38. L'Her E, Jaber S, Verzilli D, Jacob C, Huiban B, Futier E, et al. Automated closed-loop <em>versus </em> standard manual oxygen administration after major abdominal or thoracic surgery: an international multicentre randomised controlled study. Eur Respir J. (2021) 57:2000182. doi: 10.1183/13993003.00182-2020
39. Harper JC, Kearns NA, Maijers I, Bird GE, Braithwaite I, Shortt NP, et al. Closed-loop oxygen control using a novel nasal high-flow device: a randomized crossover trial. Respir Care. (2021) 66:416–24. doi: 10.4187/respcare.08087
40. Kofod LM, Westerdahl E, Kristensen MT, Brocki BC, Ringbæk T, Hansen EF. Effect of automated oxygen titration during walking on dyspnea and endurance in chronic hypoxemic patients with COPD: a randomized crossover trial. J Clin Med. (2021) 10:4820. doi: 10.3390/jcm10214820
41. Malli F, Boutlas S, Lioufas N, Gourgoulianis KI. Automated oxygen delivery in hospitalized patients with acute respiratory failure: a pilot study. Canadian Respir J. (2019) 2019:4901049. doi: 10.1155/2019/4901049
42. Lellouche F, L'Her E. Automated oxygen flow titration to maintain constant oxygenation. Respir Care. (2012) 57:1254–62. doi: 10.4187/respcare.01343
43. Hansen EF, Hove JD, Bech CS, Jensen JS, Kallemose T, Vestbo J. Automated oxygen control with O2matic(®) during admission with exacerbation of COPD. Int J Chron Obstruct Pulmon Dis. (2018) 13:3997–4003. doi: 10.2147/COPD.S183762
44. Lellouche F, Bouchard PA, Roberge M, Simard S, L'Her E, Maltais F, et al. Automated oxygen titration and weaning with FreeO2 in patients with acute exacerbation of COPD: a pilot randomized trial. Int J Chron Obstruct Pulmon Dis. (2016) 11:1983–90. doi: 10.2147/COPD.S112820
45. Poder TG, Kouakou CR, Bouchard PA, Tremblay V, Blais S, Maltais F, et al. Cost-effectiveness of FreeO(2) in patients with chronic obstructive pulmonary disease hospitalised for acute exacerbations: analysis of a pilot study in Quebec. BMJ Open. (2018) 8:e018835. doi: 10.1136/bmjopen-2017-018835
46. Cirio S, Nava S. Pilot study of a new device to titrate oxygen flow in hypoxic patients on long-term oxygen therapy. Respir Care. (2011) 56:429–34. doi: 10.4187/respcare.00983
47. Trottier M, Bouchard PA, L'Her E, Lellouche F. Automated oxygen titration during CPAP and noninvasive ventilation in healthy subjects with induced hypoxemia. Respir Care. (2023) 68:1553–60. doi: 10.4187/respcare.09866
48. Sandau C, Hansen EF, Ringbæk TJ, Kallemose T, Bove DG, Poulsen I, et al. Automated oxygen administration alleviates dyspnea in patients admitted with acute exacerbation of COPD: a randomized controlled trial. Int J Chron Obstruct Pulmon Dis. (2023) 18:599–614. doi: 10.2147/COPD.S397782
49. Kaltsogianni O, Dassios T, Jenkinson A, Greenough A. Does closed-loop automated oxygen control reduce the duration of supplementary oxygen treatment and the amount of time spent in hyperoxia? A randomised controlled trial in ventilated infants born at or near term. Trials. (2023) 24:404. doi: 10.1186/s13063-023-07415-9
50. Kaltsogianni O, Dassios T, Harris C, Jenkinson A, Lee RA, Sugino M, et al. Closed-loop oxygen system in late preterm/term, ventilated infants with different severities of respiratory disease. Acta Paediatr. (2023) 112:1185–9. doi: 10.1111/apa.16678
51. Soydan E, Ceylan G, Topal S, Hepduman P, Atakul G, Colak M, et al. Automated closed–loop FiO2 titration increases the percentage of time spent in optimal zones of oxygen saturation in pediatric patients–A randomized crossover clinical trial. Front. Med. (2022) 9:969218. doi: 10.3389/fmed.2022.969218
52. Sandau C, Poulsen I, Nørholm V, Hansen EF, Ringbaek TJ, Suppli Ulrik C, et al. Patients' perspective on automated oxygen administration during hospitalization for acute exacerbation of chronic obstructive pulmonary disease: a qualitative study nested in a randomized controlled trial. COPD. (2022) 19:345–52. doi: 10.1080/15412555.2022.2141620
53. Sandal O, Ceylan G, Topal S, Hepduman P, Colak M, Novotni D, et al. Closed–loop oxygen control improves oxygenation in pediatric patients under high–flow nasal oxygen—A randomized crossover study. Front Med. (2022) 9:1046902. doi: 10.3389/fmed.2022.1046902
54. Salverda HH, Beelen DML, Cramer SJE, Pauws SC, Schalij-Delfos N, Te Pas AB. Clinical outcomes of preterm infants while using automated controllers during standard care: comparison of cohorts with different automated titration strategies. Arch Dis Child Fetal Neonatal Ed. (2022) 108:26–30. doi: 10.1136/archdischild-2021-323690
55. Nair V, Lal MK, Gillone J, Kannan Loganathan P, Bachman TE. Comparison of volume guarantee and volume-controlled ventilation both using closed loop inspired oxygen in preterm infants: a randomised crossover study (CLIO-VG study). Arch Dis Child Fetal Neonatal Ed. (2022) 107:161–5. doi: 10.1136/archdischild-2021-321712
56. Kaltsogianni O, Dassios T, Belbal R, Greenough A. Survey of closed-loop automated oxygen control systems in neonatal intensive care units. Acta Paediatr. (2022) 111:1002–3. doi: 10.1111/apa.16239
57. Atakul G, Ceylan G, Sandal O, Soydan E, Topal S, Colak M, et al. Closed-loop O2 use during invasive mechanical ventilation of pediatric patients (CLOUDIMPP): a randomized cross-over study. Res Square. (2023) 1:2908224. doi: 10.21203/rs.3.rs-2908224/v1
58. Faul F, Erdfelder E, Lang A-G, Buchner A. G*Power 3: a flexible statistical power analysis program for the social, behavioral, and biomedical sciences. Behav Res Methods. (2007) 39:175–91. doi: 10.3758/BF03193146
59. Claure N, Gerhardt T, Everett R, Musante G, Herrera C, Bancalari E. Closed-loop controlled inspired oxygen concentration for mechanically ventilated very low birth weight infants with frequent episodes of hypoxemia. Pediatrics. (2001) 107:1120–4. doi: 10.1542/peds.107.5.1120
60. Claure N, D'Ugard C, Bancalari E. Automated adjustment of inspired oxygen in preterm infants with frequent fluctuations in oxygenation: a pilot clinical trial. J Pediatr. (2009) 155:640–5. doi: 10.1016/j.jpeds.2009.04.057
61. Hallenberger A, Poets CF, Horn W, Seyfang A, Urschitz MS. Closed-loop automatic oxygen control (CLAC) in preterm infants: a randomized controlled trial. Pediatrics. (2014) 133:e379–85. doi: 10.1542/peds.2013-1834
62. Johannigman JA, Branson R, Lecroy D, Beck G. Autonomous control of inspired oxygen concentration during mechanical ventilation of the critically injured trauma patient. J Trauma. (2009) 66:386–92. doi: 10.1097/TA.0b013e318197a4bb
63. Arnal J-M, Wysocki M, Novotni D, Demory D, Lopez R, Donati S, et al. Safety and efficacy of a fully closed-loop control ventilation (IntelliVent-ASV(R)) in sedated ICU patients with acute respiratory failure: a prospective randomized crossover study. Inten Care Med. (2012) 38:781–7. doi: 10.1007/s00134-012-2548-6
64. Lellouche F, Bouchard P-A, Simard S, L'Her E, Wysocki M. Evaluation of fully automated ventilation: a randomized controlled study in post-cardiac surgery patients. Intens Care Med. (2013) 39:463–71. doi: 10.1007/s00134-012-2799-2
65. Johannigman JA, Branson RD, Edwards MG. Closed loop control of inspired oxygen concentration in trauma patients. J Am Coll Surg. (2009) 208:763–8. doi: 10.1016/j.jamcollsurg.2009.01.033
66. Saihi K, Richard J-CM, Gonin X, Krüger T, Dojat M, Brochard L. Feasibility and reliability of an automated controller of inspired oxygen concentration during mechanical ventilation. Crit Care. (2014) 18:R35-R. doi: 10.1186/cc13734
67. De Bie AJ, Neto AS, van Meenen DM, Bouwman AR, Roos AN, Lameijer JR, et al. Fully automated postoperative ventilation in cardiac surgery patients: a randomised clinical trial. Br J Anaesth. (2020) 125:739–49. doi: 10.1016/j.bja.2020.06.037
68. van Zanten HA, Pauws SC, Beks EC, Stenson BJ, Lopriore E, Te Pas AB. Improving manual oxygen titration in preterm infants by training and guideline implementation. Eur J Pediatr. (2017) 176:99–107. doi: 10.1007/s00431-016-2811-x
69. Armbruster J, Schmidt B, Poets CF, Bassler D. Nurses' compliance with alarm limits for pulse oximetry: qualitative study. J Perinatol. (2010) 30:531–4. doi: 10.1038/jp.2009.189
70. Mao C, Wong DT, Slutsky AS, Kavanagh BP, A. quantitative assessment of how Canadian intensivists believe they utilize oxygen in the intensive care unit. Crit Care Med. (1999) 27:2806–11. doi: 10.1097/00003246-199912000-00033
71. Grim CCA, Cornet AD, Kroner A, Meiners AJ, Brouwers AJBW, Reidinga AC, et al. Attitudes of Dutch intensive care unit clinicians towards oxygen therapy. Neth J Med. (2020) 78:167–74.
72. Roué JM, Delpeut J, d‘Hennezel A, Tierrie T, Barzic A, L'Her E, et al. Automatic oxygen flow titration in spontaneously breathing children: An open-label randomized controlled pilot study. Pediatr Pulmonol. (2020) 55:3180–8. doi: 10.1002/ppul.25035
73. Aboab J, Louis B, Jonson B, Brochard L. Relation between PaO2/FIO2 ratio and FIO2: a mathematical description. Intensive Care Med. (2006) 32:1494–7. doi: 10.1007/s00134-006-0337-9
74. Tarnow-Mordi WO, Hau C, Warden A, Shearer AJ. Hospital mortality in relation to staff workload: a 4-year study in an adult intensive-care unit. Lancet. (2000) 356:185–9. doi: 10.1016/S0140-6736(00)02478-8
75. Darbyshire JL, Young JD. An investigation of sound levels on intensive care units with reference to the WHO guidelines. Crit Care. (2013) 17:R187. doi: 10.1186/cc12870
76. Smith HAB, Besunder JB, Betters KA, Johnson PN, Srinivasan V, Stormorken A, et al. 2022 society of critical care medicine clinical practice guidelines on prevention and management of pain, agitation, neuromuscular blockade, and delirium in critically ill pediatric patients with consideration of the ICU environment and early mobility. Pediatr Crit Care Med. (2022) 23:e74–e110. doi: 10.1097/PCC.0000000000002873
77. Martin D, Johns C, Sorrell L, Healy E, Phull M, Olusanya S, et al. Effect of skin tone on the accuracy of the estimation of arterial oxygen saturation by pulse oximetry: a systematic review. Br J Anaesth. (2024) 132:945–56. doi: 10.1016/j.bja.2024.01.023
Keywords: hypoxemia, oxygen therapy, invasive mechanical ventilation, automation, closed-loop, oxygen controller, intensive care, pediatrics
Citation: Atakul G, Ceylan G, Sandal O, Soydan E, Hepduman P, Colak M, Zimmermann JM, Novotni D, Karaarslan U, Topal S and Aǧin H (2024) Closed–loop oxygen usage during invasive mechanical ventilation of pediatric patients (CLOUDIMPP): a randomized controlled cross-over study. Front. Med. 11:1426969. doi: 10.3389/fmed.2024.1426969
Received: 02 May 2024; Accepted: 13 August 2024;
Published: 10 September 2024.
Edited by:
Claude E. Guérin, Hospices Civils de Lyon, FranceReviewed by:
Michele Bertoni, University of Brescia, ItalyCopyright © 2024 Atakul, Ceylan, Sandal, Soydan, Hepduman, Colak, Zimmermann, Novotni, Karaarslan, Topal and Aǧin. This is an open-access article distributed under the terms of the Creative Commons Attribution License (CC BY). The use, distribution or reproduction in other forums is permitted, provided the original author(s) and the copyright owner(s) are credited and that the original publication in this journal is cited, in accordance with accepted academic practice. No use, distribution or reproduction is permitted which does not comply with these terms.
*Correspondence: Gokhan Ceylan, ZHJnb2toYW5jZXlsYW5AZ21haWwuY29t
Disclaimer: All claims expressed in this article are solely those of the authors and do not necessarily represent those of their affiliated organizations, or those of the publisher, the editors and the reviewers. Any product that may be evaluated in this article or claim that may be made by its manufacturer is not guaranteed or endorsed by the publisher.
Research integrity at Frontiers
Learn more about the work of our research integrity team to safeguard the quality of each article we publish.