- Section of Emergency Medicine, Department of Medicine, LSU School of Medicine, New Orleans, LA, United States
For short periods, even without the presence of red blood cells, hyperbaric oxygen can safely allow plasma to meet the oxygen delivery requirements of a human at rest. By this means, hyperbaric oxygen, in special instances, may be used as a bridge to lessen blood transfusion requirements. Hyperbaric oxygen, applied intermittently, can readily avert oxygen toxicity while meeting the body's oxygen requirements. In acute injury or illness, accumulated oxygen debt is shadowed by adenosine triphosphate debt. Hyperbaric oxygen efficiently provides superior diffusion distances of oxygen in tissue compared to those provided by breathing normobaric oxygen. Intermittent application of hyperbaric oxygen can resupply adenosine triphosphate for energy for gene expression and reparative and anti-inflammatory cellular function. This advantageous effect is termed the hyperbaric oxygen paradox. Similarly, the normobaric oxygen paradox has been used to elicit erythropoietin expression. Referfusion injury after an ischemic insult can be ameliorated by hyperbaric oxygen administration. Oxygen toxicity can be averted by short hyperbaric oxygen exposure times with air breaks during treatments and also by lengthening the time between hyperbaric oxygen sessions as the treatment advances. Hyperbaric chambers can be assembled to provide everything available to a patient in modern-day intensive care units. The complication rate of hyperbaric oxygen therapy is very low. Accordingly, hyperbaric oxygen, when safely available in hospital settings, should be considered as an adjunct for the management of critically injured or ill patients with disabling anemia.
Preclinical introduction
The clinical use of hyperbaric oxygen therapy (HBOT) to address the absence of sufficient hemoglobin levels began with the work of Dutch surgeon, I. Boerema, in the late 1950s. He rapidly exsanguinated swine to hemoglobin levels as low as 1 g per deciliter and then resuscitated them by intravenous volume repletion with a Ringer's lactate–dextran 6%–dextrose water 5% solution. Next, he pressurized the unconscious, collapsed but still breathing, swine to three atmospheres of pressure in a hyperbaric chamber and made them breathe 100% oxygen. At three atmospheres of pressure, inhaled oxygen of 100% provided a surface equivalent fraction of inhaled oxygen of 300% (SEFIO2 300%). He kept the swine at three atmospheres of pressure for 15 min and then re-transfused them with their shed blood and depressurized the chamber to the surface, whereupon the swine walked off unimpaired. He published these results in an article entitled “Life Without Blood” (1).
These results were replicated in a laboratory in the United States in 2010 at the LSU Health Sciences Center in New Orleans in an Institutional Animal Care Utilization Committee (IACUC)-approved pilot study. An acutely anesthetized, exsanguinated swine was monitored by a polarographic oxygen tension probe through a cranial burr hole (2). The swine, breathing normobaric room air, had a baseline brain tissue pO2 level of 30 mmHg. After a rapid exsanguination involving the removal of 40% of the blood volume, the swine's brain tissue pO2 dropped to 0 mmHg even while the swine was being ventilated with normobaric 100% oxygen. For volume replacement, the swine received intravenous Ringers' D5W solution. Next, the animal was pressurized inside a hyperbaric chamber while being kept on 100% oxygen inhalation at three atmospheres of pressure. At this pressure, the oxygen inhalation provided SEFIO2 of 300% oxygen. The brain tissue pO2 rose back to 30 mmHg, and the animal remained pressurized for 50 min. Before ascent to the surface, the swine was transfused with its shed blood. Upon reaching the surface at ambient pressure, the animal was recovered from anesthesia, and monitoring access catheters were removed. The swine walked off unimpaired and was returned to a rescue ranch for a long life (3). Table 1 shows a summary of published animal experiments investigating the use of HBOT in severe anemia. The tabular summary includes a thumbnail of evidence-based analysis using three different criteria (AHA/NCI-PDQ/BMJ) (4).
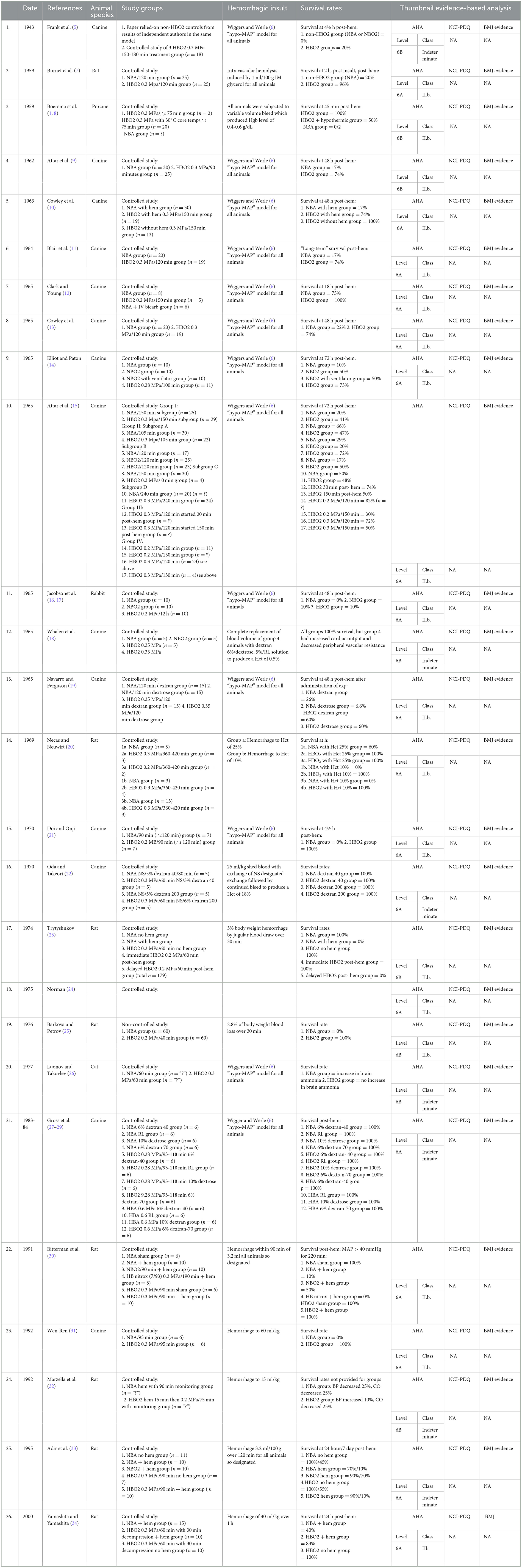
Table 1. Summary of published animal experiments investigating the use of hyperbaric oxygen therapy in severe anemia.
The clinical use of hyperbaric oxygen
Hyperbaric oxygen (HBO) may be used as a bridging therapy in the Advanced Trauma Life Support (ATLS) and Advanced Cardiac Life Support (ACLS) resuscitation of a precariously anemic patient to prevent multiunit transfusion until damage control surgical efforts can be implemented. The initial damage control surgery aims at preventing continued blood loss to allow the patient to retain transfused blood (35).
Likewise, HBO may be used as a bridging therapy for patients who refuse blood transfusions due to religious or philosophical reasons. Tincture of time could then allow the provision of hematinic nutrients and pharmaceuticals to support hematopoiesis to endogenously provide red blood cell replacement (36). If hemoglobin's ability to transport oxygen by carbon monoxide, cyanide, or hydrogen sulfide is impaired, HBO can be used acutely to treat these conditions to assist in patient recovery from the chemical hypoxia imposed by the poisoning (37–41).
In yet another clinical instance, HBO may be used if an anticipated complication of a blood transfusion precludes further transfusion (42):
1. Blood group incompatibility.
2. Febrile non-hemolytic transfusion reaction (FNHTR).
3. Both delayed amnestic and primary hemolytic anemia.
4. Allergy from urticaria to anaphylaxis.
5. Transfusion-associated graft-versus-host disease (TAGVHD).
6. Acute radiation-induced anemia in disasters with a supply shortage.
7. Transfusion-transmitted infections (TTI).
8. Both red blood cell and human leukocyte antigen (HLA) allosensitization.
9. Confounding severe congestive heart failure with profound anemia until stabilization, providing the safety of transfusion.
10. Stacking hemosiderosis from multiple transfusions by lessening the number of transfusions.
11. The prevention of long-term transfusion immunomodulation by lessening the number of transfusions.
12. The prevention of short-term induction of multiorgan failure by red blood cell-associated lipids and cytokines.
13. Transfusion-related lung injury (TRALI).
HBOT has been documented to ameliorate the adult respiratory syndrome induced by trauma or infection in severely anemic patients (43–46). This effect of HBOT may also be found to be an additional advantage of HBO as a bridging treatment until safe transfusions are possible in a patient with TRALI or acute respiratory distress (ARDS) in SARS-CoV2 patients with severe anemia (47). Research into this area is necessary. A randomized, controlled study has been published evidencing the use of HBOT to perform this (44). Table 2 shows human case studies and series for use in the treatment of severe anemia. The tabular summary includes a thumbnail, evidence-based analysis of the published papers using three different criteria (AHA/NCI-PDQ/BMJ) (4). More recently, a randomized, controlled trial of HBOT used in severe anemia has been published (57).
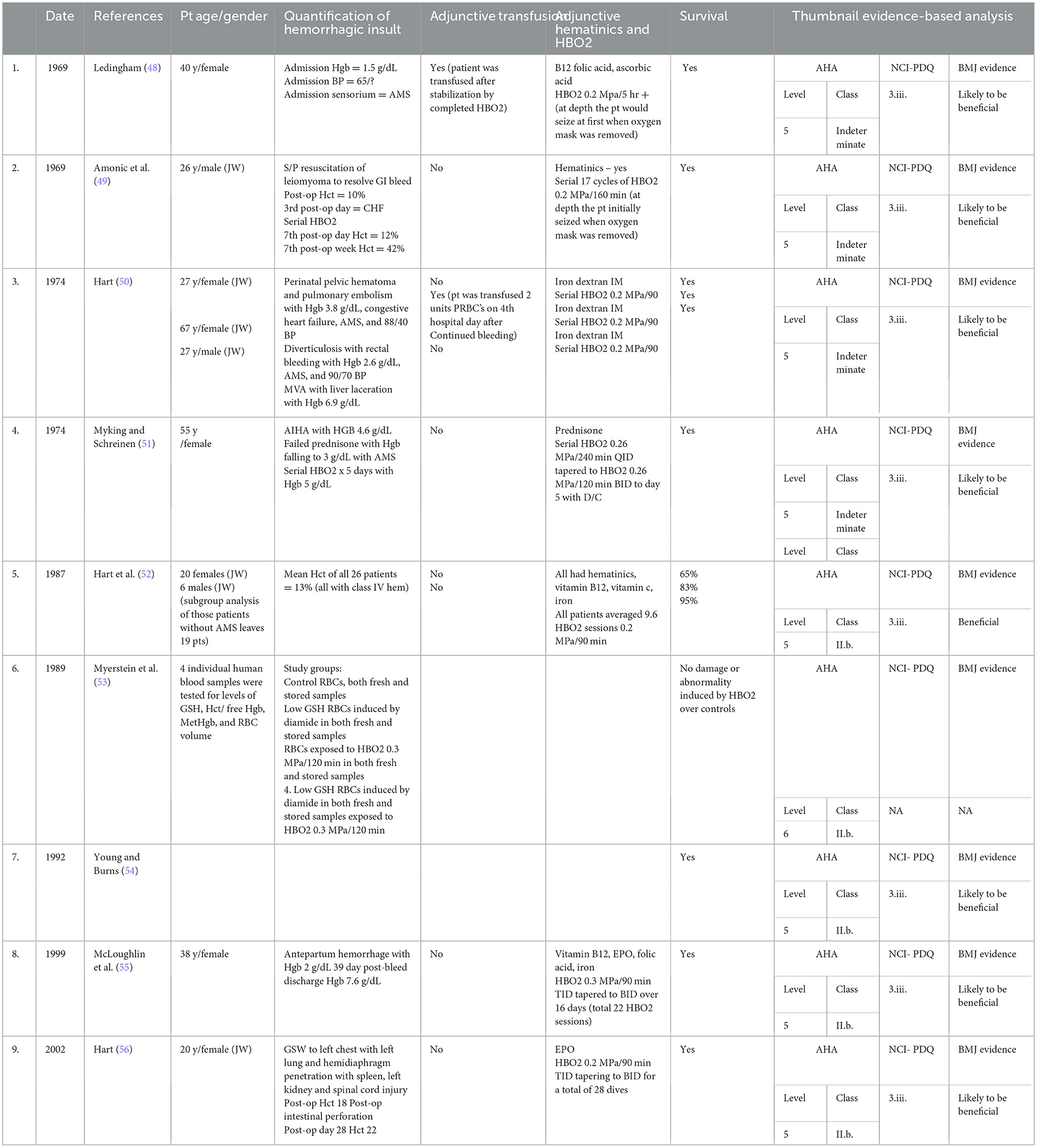
Table 2. Human case reports and series for use of hyperbaric oxygen therapy in treatment of severe anemia.
When considering the use of HBOT in cases of severe anemia, the clinician should consult a hyperbaric physician specialist to determine whether HBOT would be helpful for the individual patient. Figure 1 demonstrates the treatment course recommended by the UHMS in their 2023 edition of the Hyperbaric Medicine Indications Manual. The patient would undergo HBOT at two–three ATA for 60–90 min with one–two intermittent 5-min air breaks (4).
Discussion
The operational practicality of using HBO as a bridging therapy in a remote non-medical setting was reported in a case of severe exsanguination of a commercial diver while in saturation offshore in the Gulf of Mexico. The patient bled out hemoglobin of 2 g per deciliter acutely when his duodenal artery was eroded by a duodenal ulcer. The requisite decompression to the surface required 3 days. During his recompression, he was kept alive without transfusion by Ringers' D5 solution administered by hypodermoclysis and intermittent HBO breathing periods. At the surface, he was then transfused with intravenous packed red blood cells (58). Many years before, three cases of patients with severe blood loss who each refused transfusion for religious belief were reported in the medical literature. The cases were successfully treated with intermittently administered HBO in the same way at a naval dock in a hyperbaric chamber (50).
High concentrations of continuously administered oxygen have been reported to be deleterious when used in patient's resuscitative management (59, 60). This observation has remained consistent regardless of whether the patients enrolled in clinical trials have had high, normal, or low hemoglobin levels, whether acute or chronic (61). How could HBO provided by ventilation with SEFIO2 of inhaled oxygen of 150%−300% not be deleterious? For one, the inhaled oxygen under these conditions is not continuous but is intermittent, with administered air breaks incorporated during HBOT sessions (62). Additionally, as the series of HBO treatment sessions progresses and the patient's condition improves, the patient becomes increasingly tolerant of the off-oxygen periods. This allows the HBOT to be spread out with longer periods between treatments (49). During the HBO breathing periods, enough oxygen is dissolved in plasma to allow plasma to deliver oxygen to tissue mitochondria to reduce the previously accumulating oxygen debt, which, in effect, is an adenosine triphosphate (ATP) debt (63–66).
One might say that HBOT, as bridge therapy, serves to resuscitate patients much like the bridging function of veno-venous extracorporeal membrane oxygenation (VV-ECMO) during resuscitative support of critically anemic patients with restrictions on red blood cell transfusion. In the instance of intermittent non-invasive HBOT, the patient can similarly be successfully supported (67). By simile, one might compare VV-ECMO to a continuous weld and short-interval intermittent HBOT to a spot weld (in effect, intermittent HBOT is VV-ECMO-like or “ECMoid” in function). Both therapeutic modalities attempt to hold the metabolic structure of the patient together. ECMO has up to a 30% serious adverse side effect incidence (68). Hyperbaric oxygen has, on average, one in 10,000 incidences of serious side effects including pneumothorax, oxygen toxicity seizure, fire or explosion, and arterial gas embolism (69–71). The ECMO hospital facility support fee is often US $50,000 per day (72) and a hospital-based HBOT series of 30 treatments includes a facility charge of US $7,500 (73, 74). In almost all instances, 30 HBOT treatments would be more than enough to bridge a patient through an anemic crisis. In the United States, the cost of a unit of packed red blood cells, along with its administration, is comparable to the cost of one HBOT treatment (4).
The tolerance to high-dose oxygen administration by intermittent application has been well-documented with oxygen administered at one atmosphere pressure as well as at increased atmospheric pressure (62, 75). There is more than just oxygen tolerance provided by the intermittency of use; this is the effect of intermittency itself. ATP resupply occurs when the mitochondrial intermembrane space minimally attains 1.5–2.0 mmHg of oxygen, which is a requisite for the unimpaired production of ATP by the mitochondrial respiratory chain of enzymes (76). In a severely anemic patient, equally important is the return of tissue hypoxia after the completion of an HBOT treatment. It is hypoxia that incites ATP-dependent reparative cytokine tissue release and antioxidant production. For the reparative and anti-inflammatory cytokines to work at cellular receptor sites, ATP is needed. The anteceding HBOT would have supplied the needed ATP for this to occur. The ensuing tissue hypoxia between treatments induces the following energy-dependent or ATP-dependent activity:
1. Antioxidant productions and functions to include catalase and peroxidase (77), glutathione (78), superoxide dismutase (79), and ATP itself as an antioxidant (80).
2. Support of genomic activity (81).
3. Support of epigenomic activity (82).
4. Support of proteomic activity (83) and protein folding (84).
5. Support of lipidomic activity (85).
6. Support of anti-inflammatory and reparative cytokines/chemokines (86).
7. Leukocyte function (87, 88).
8. Adaptive function in hypoxia: (erythropoietin) (89, 90) (heat shock protein) (91) (nitric oxide) (92) (hypoxia-inducible factor) (93).
This oscillation between hyperoxia and hypoxia may be graphically depiected as a sinusoidal timeline by Figure 2 and is the crux of the oxygen paradox.
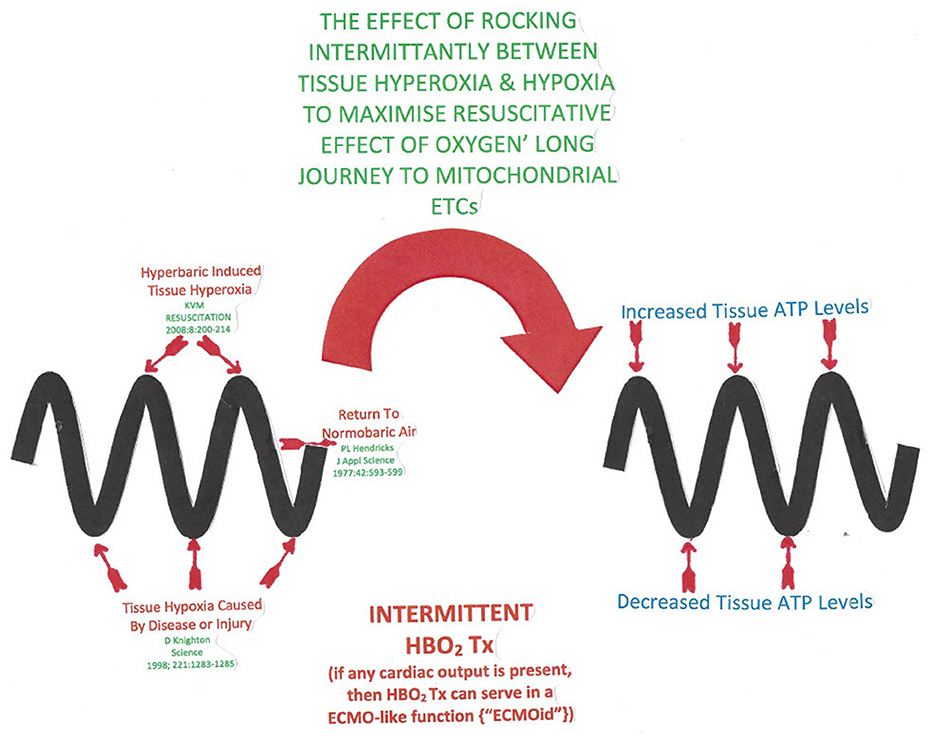
Figure 2. The sinusoidal horizontal timeline depicted by the thick wavy lines in the diagram represents an intermittent hyperbaric oxygen treatment course. The wave peaks represent a hyperbaric oxygen treatment producing ATP resupply (93). Post treatment when the tissue oxygen tension drops, energy requiring cytokine and antioxidant release occurs (85).
To provide ATP resupply in the instance of an acute hypoxic state, pulsed high-dose HBO inhalation can be used to diffuse minimally 1.5–2.0 mmHg of oxygen into the mitochondrial intermembrane space (94). At best, when a red blood cell gets to its destination in capillaries, it must offload a portion of its remaining oxygen content back into the plasma. As the patient inhales 100% oxygen at one atmosphere of pressure, the plasma can maximally contain only 2.3 volumes% of dissolved oxygen. In contrast, the patient in a hyperbaric chamber at three atmospheres of pressure would inhale a SEFIO2 of 300%, thereby delivering to the capillaries 6.6 volume% of dissolved oxygen with a five-fold diffusion distance outside of the capillary over that of a subject inhaling an FIO2 of 100% oxygen at one atmosphere of pressure (95–97). This concept was reported over 60 years ago by W. Brummelkamp when he reported that during an HBOT treatment, “drenching of the tissue with dissolved oxygen” occurred by way of immersing plasma with oxygen (98).
HBOT inhalation can only be accomplished safely when the entire patient is pressurized above ambient pressure in an enclosure (i.e., a hyperbaric chamber). The spectrum of potential treatment doses of oxygen using HBO pressure incorporates the pharmacologic effect of the gases at increased pressure and the physiologic effect of pressure itself (99).
A measure of the safety of HBO can best be described by Pascal's Law, where in a confined space, any contained fluid will transmit the pressure evenly throughout the fluid non-destructively. The human skin envelope contains the fluid of all the body's tissue (gas is not a problem in sinus spaces if vented by an open ostia and in the middle ear if vented by a patient's functioning Eustachian tube) (100). By virtue of the principle of Pascal's law, a patient may be ventilated without barotrauma by pressurized gas at the same pressure as that of hyperbaric chamber pressurization. Ventilators have been developed to do this safely, and chambers can be fitted with all the functions of critical care hospital units (101, 102).
Henry's Gas Law states that the concentration of a solute gas in a solution is directly proportional to the partial pressure of the gas over the solution. Inhalation of HBO at three atmospheres of pressure allows enough dissolved oxygen (6.6 volumes%) in plasma to supply the metabolic extraction rate of most of the tissue in a human body at rest (103).
The operational safety of hyperbaric medicine units has evolved through adherence to developing safety guidelines. This has allowed a remarkable safety record for hospital-based units for equipment, patients, and healthcare providers for both multiplace and monoplace chamber facilities (104, 105).
At the 21% oxygen content of air in one atmosphere, hemoglobin makes up for plasma's inability to deliver adequate oxygen to tissue. This is because a subject breathing air would have, at maximum, a 0.48 volume% of plasma dissolved oxygen, which clearly would not be enough to support human life (0.003 ml × 21% × 760 mmHg, where 0.003 ml is the amount at one atmosphere of oxygen dissolved in plasma for each mmHg of pressure, 21% is the oxygen content of air, and 760 mmHg is the pressure for each mmHg of pressure in the atmosphere at sea level) (106). Using the same equation for breathing 100% oxygen at one atmosphere, the maximum amount of dissolved oxygen in plasma would be 2.3 volume%. As mentioned, this would be far below the average oxygen extraction rate of most human tissue with the body at rest. To get around this problem, hemoglobin serves as a powerful gas clathrate, especially for oxygen. When a red blood cell picks up oxygen in the lung and discharges it in the periphery, the maximum oxygen conceivably dissolved in plasma would be 2.3 volume% at both ends of the line.
Plasma delivers the oxygen from the red blood cells to the endothelium, where it diffuses into the interstitial fluid, then diffuses through cellular membranes into the cytosol, and finally passes into the intermembrane space (IMS) of mitochondria. HBO administered at three atmospheres of pressure (SEFIO2 300%) allows 6.6 volume% of oxygen to be dissolved in plasma. It is this concentration that begins its journey by diffusion through the capillary endothelium, ultimately filling the IMS of mitochondria minimally with the 1.5–2.0 mmHg of dissolved oxygen requisite for the electron transport chain along with ATP synthase to produce ATP (107). Figures 3, 4 demonstrate this process.
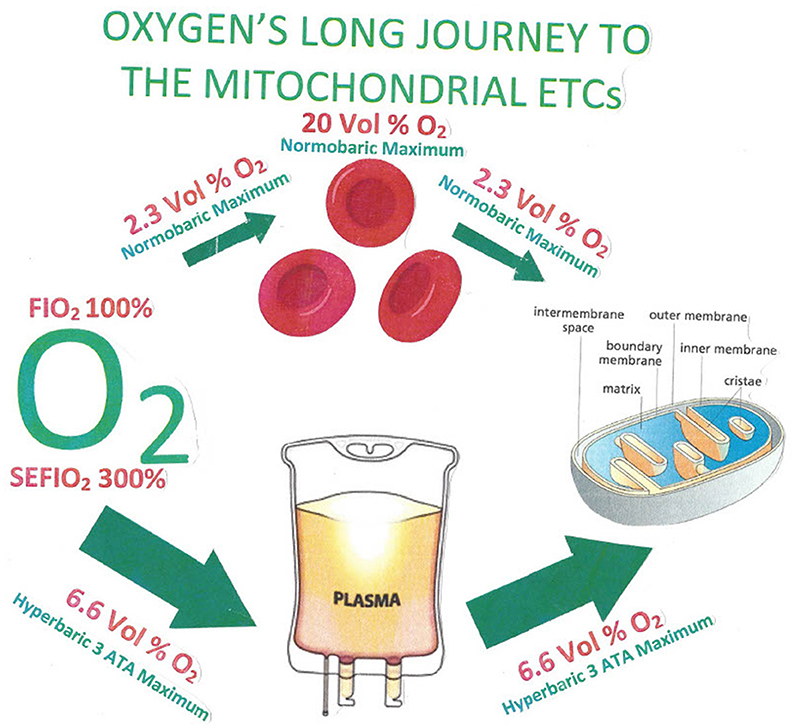
Figure 3. The most oxygen that can conceivably be dissolved in plasma by a subject who breathes 100% oxygen is 2.3 volume%, and the plasma level enters the red blood cells in the lung capillary, where the blood content can be boosted as high as 20 volume% by the presence of the gas-clathrate-like function of hemoglobin. When the red blood cell gets to its destination in a capillary of distant tissue, the highest possible concentration as the oxygen unloads from the red blood cell into the plasma possible at normobaric pressure is again at the very highest, 2.3 volume%. Under hyperbaric conditions at three atmospheres of pressure, the equivalent amount of oxygen possible in plasma during the circulatory route all the way to distal capillaries at the very highest would be 6.6 volume% (108).
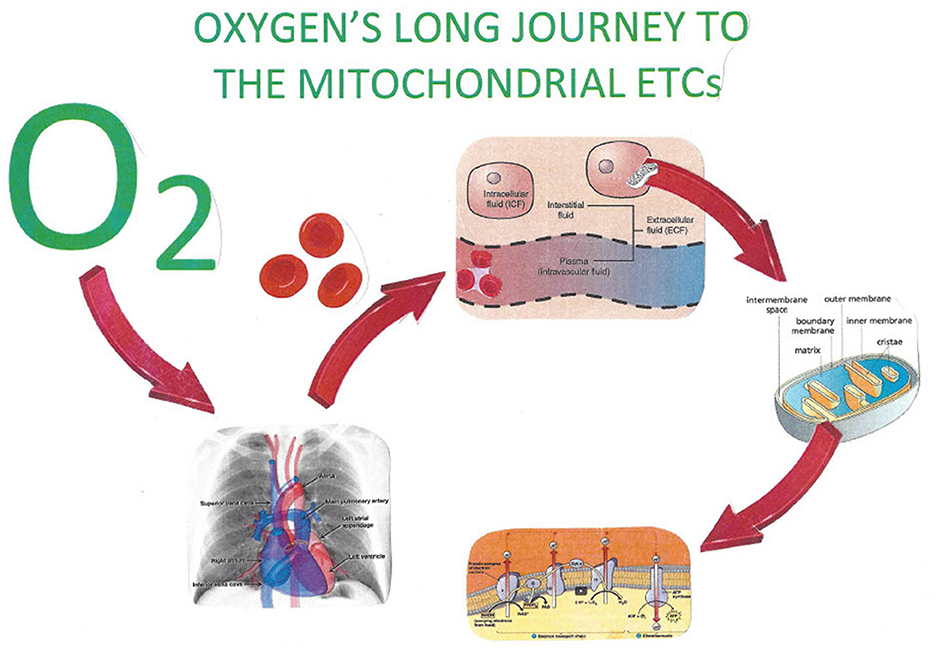
Figure 4. The increased ability of hyperbaric oxygen allows for an increased quantity of dissolved oxygen in body fluids. The facilitated delivery of oxygen thereby to the IMS of mitochondria throughout the body provides for necessary oxidative phosphorylation. Oxygen, by attaching to the cytochrome 3 oxidase enzyme of the mitochondrial electron transport chain, produces the necessary supply of hydronium ions for ATP.
The increased diffusivity of oxygen in tissue afforded by hyperbaric pressure is important. Krogh has described the diffusion distance of oxygen from plasma through the capillary endothelium (95, 97). This has been further expounded upon to include the added effect of the diffusivity of oxygen in the hyperbaric environment (99) as demonstrated in Figure 5.
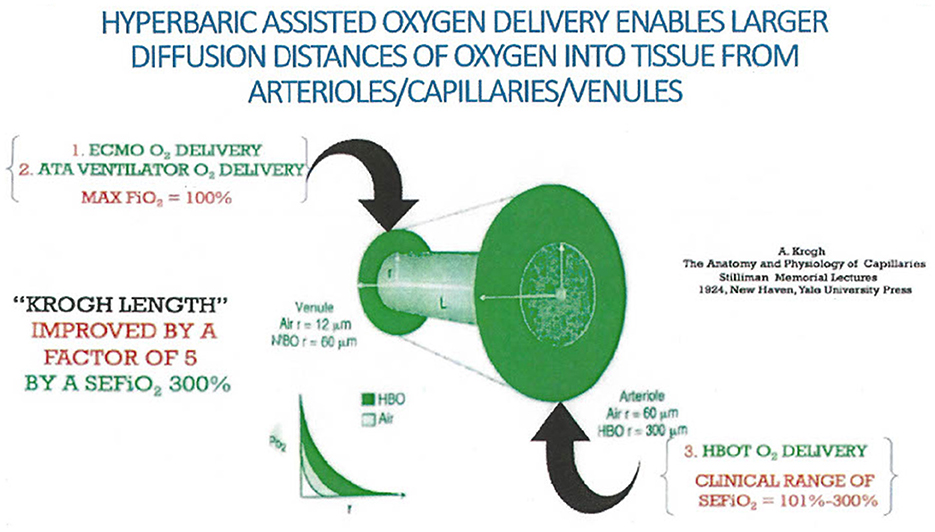
Figure 5. Krogh calculated the diffusion distance of oxygen outside of capillaries into tissue when a human breathed normobaric air. Extrapolation incorporating three atmospheres of pressure provided by hyperbaric treatment would provide a fivefold improvement in diffusion distance (94, 110).
Tissue oxygen capacitance increases during and after an HBOT treatment. The oxygen that is onloaded into the tissue during HBOT is, in part, slowly off-gassed, much like an inert gas with tissue elimination half-lives supplemented by the additional elimination of oxygen by metabolic consumption (109). Furthermore, some oxygen is retained in tissue by attaching to cellular gas clathrates [i.e., neuroglobin (110), cytoglobin (111), and myoglobin (112)]. With serial HBOT treatment, tissue oxygen capacitance increases (113).
The red blood cell, as a biconcave disk, has a shape that maximizes its surface area. As a short-lived bag of hemoglobin, the mature red blood cell does not have mitochondria or a nucleus. An important mission of the red blood cell is to overcome the poor solubility of oxygen in plasma at one atmosphere in order to adequately get a supply of oxygen to mitochondria. The use of HBOT, especially in remote settings, has compelled some tertiary urban trauma medical staff to consider the development of a hyperbaric ambulance to mimic the success of the deck decompression chambers on operational sites to address injury of commercial divers (114). Figure 6 demonstrates this point.
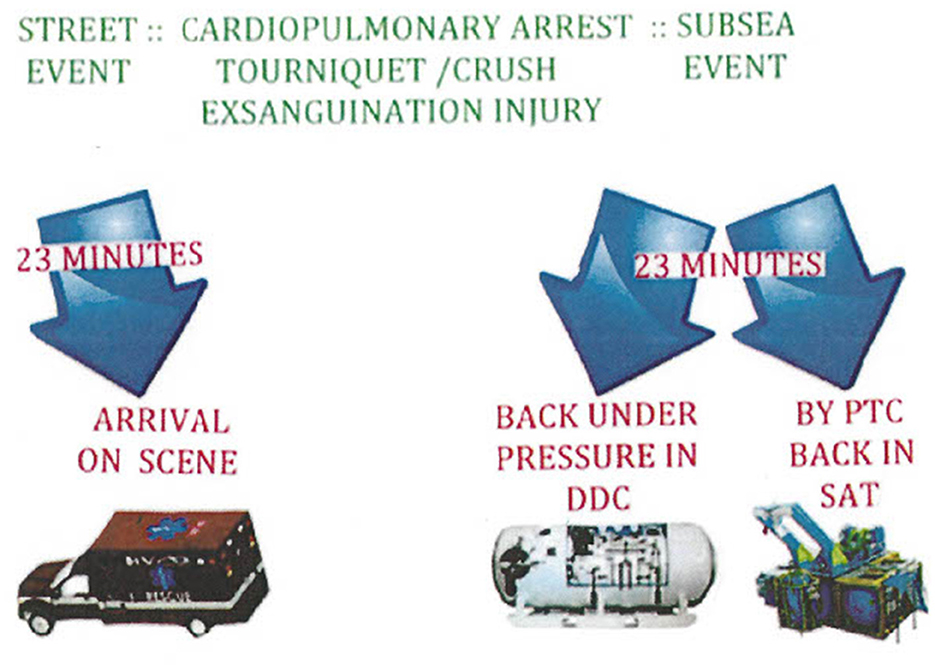
Figure 6. Having a hyperbaric ambulance to bring immediate hyperbaric oxygen treatment to severely anemic, injured, or ill patients in transit to a hospital emergency department increases the chance of having the same success rate as commercial diving operations, which require a hyperbaric chamber on site for accidents.
A consideration of the potential toxic properties of a prolonged administration of O2 in almost all cases under normobaric, hyperbaric, or hypobaric exposures is a certainty (115–117). A judicious use of short-tie exposures of 60–90 min with intermittency of 5 min air breaks during administration and with gradual spreading of time intervals between treatments has thoroughly been documented to be safe, allowing the “hyperoxic–hypoxic paradox” prevail to the patient's benefit (118–120).
Conclusion
Red blood cells play an important role in the chain of oxygen delivery to the mitochondrial IMS. Finally, in the IMS, oxygen attaches to cytochrome c oxidase in the electron transport chain of enzymes embedded in the inner IMS (121). Reacting with the oxygen and hydrogen ions, cytochrome c oxidase expels the by-product of water. The hydrogen ions in the IMS fall down the molecular shoot of the ATP synthase nanomachine, and by rotary catalysis, Pi ions join with ADP to form ATP. It is an evolutionary wonder that the red blood cell without mitochondria carts oxygen to mitochondria in all the body's cells to provide the energy for the homeostasis of life.
HBOT, if used promptly, can serve as a bridge therapy to alleviate illness and injury when transfusion of red blood cells is necessary, otherwise requiring massive transfusion protocols. In other instances, HBOT could address severely anemic patients burdened with complicating comorbidities that otherwise would preclude the desirability of transfusion of red blood cells in any amount or by transfusion altogether. Hypoxic stress simulates recovery, and recovery requires energy provided by ATP.
Emerging ways that HBOT can safely and quickly be available are currently in existence (122). Hyperbaric units can be parts of emergency departments (123), intensive care units (124), and ambulances (114). Figure 7 shows schematics for a designed hyperbaric ambulance. The cost of an HBOT treatment is equal to the cost of a unit of blood and its administration (4). HBOT does not need type and crossing, or IV access, as the systemic dose of oxygen is administered via breathing or through a patient ventilator.
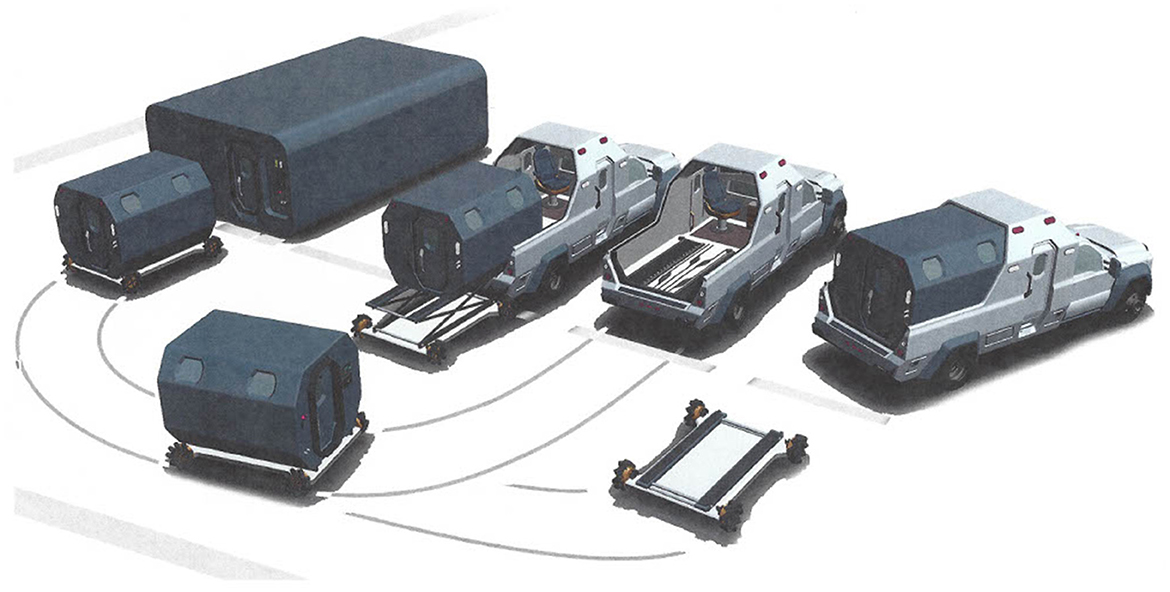
Figure 7. The best of all possibilities would be to have a hyperbaric ambulance system that deploys to the street to transport a severely anemic, ill, or injured patient under pressure with oxygen administration. Upon arrival at the hospital, the pressurized patient compartment would hydraulically depart the ambulance chaise and roll into the hospital emergency department to continue conventional normobaric resuscitation. The option could be for the detached patient compartment of the ambulance to mate with a hyperbaric intensive care multiplace chamber for continued hyperbaric resuscitation (113).
Author contributions
KV: Writing – original draft, Writing – review & editing.
Funding
The author(s) declare that no financial support was received for the research, authorship, and/or publication of this article.
Conflict of interest
The author declares that the research was conducted in the absence of any commercial or financial relationships that could be construed as a potential conflict of interest.
Publisher's note
All claims expressed in this article are solely those of the authors and do not necessarily represent those of their affiliated organizations, or those of the publisher, the editors and the reviewers. Any product that may be evaluated in this article, or claim that may be made by its manufacturer, is not guaranteed or endorsed by the publisher.
References
1. Boerema I, Meyne NG, Brummelkamp WK, Mensch MH, Kamermans F, Stern Hanf M, et al. Life without blood: a study of the influence of high atmospheric pressure and hypothermia on dilution of the blood. J Cardiovasc Surg. (1960) 1:133–46.
2. Maloney-Wilensky E, Le Roux P. The physiology behind direct oxygen monitors and practical aspects of their use. Childs Nerv Syst. (2010) 26:419–30. doi: 10.1007/s00381-009-1037-x
3. Van Meter K. Hyperbaric oxygen use in exceptional blood loss anemia. In: Whelan HT, Kindwall EP, editors. Hyperbaric Medicine Practice, 4th Edn. North Palm Beach, FL: Best Publishing (2017), p. 691–705.
4. Van Meter K. Severe anemia. In: Huang ET, editor. Undersea and Hyperbaric Medical Society Hyperbaric Medicine Indications Manual, 15th Edn. North Palm Beach, FL: Best Publishing Company (2023), p. 295–304.
5. Frank HA, Fine J. Traumatic shock V: a study of the effect of oxygen on hemorrhagic shock. J Clin Invest. (1943) 22:305–14. doi: 10.1172/JCI101396
6. Wiggers CJ, Werle JM. Exploration of method for standardizing hemorrhagic shock. Proc Soc Exper Biol Med. (1942) 49:601. doi: 10.3181/00379727-49-13645
7. Burnet W, Clark RG, Duthie HL, Smith AN. The treatment of shock by oxygen under pressure. Scot Med J. (1959) 4:535–8. doi: 10.1177/003693305900401105
8. Boerema I, Meyne NG, Brummelkamp WH, Bouma S, Mensch MH, Kamermans F, et al. Life without blood. Arch Chir Neerl. (1959) 11:70–84.
9. Attar S. Esmond WH, Cowley RA. Hyperbaric oxygenation in vascular collapse. J Thoracic Cardiovasc Surg. (1962) 44:759–70. doi: 10.1016/S0022-5223(19)32911-3
10. Cowley RA, Attar S, Esmond WJ, Blair E, Hawthorne I. Electrocardiographic and biochemical study in hemorrhagic shock in dogs treated with hyperbaric oxygen. Circulation. (1963) 27:670–5. doi: 10.1161/01.CIR.27.4.670
11. Blair E, Henning G, Esmond WG, Attar S, Cowley RA, Michaelis M. The effect of hyperbaric oxygenation (OHP) on three forms of shock – traumatic, hemorrhagic, and septic. J Trauma. (1964) 4:652–63. doi: 10.1097/00005373-196409000-00009
12. Clark RG, Young DG. Effects of hyperoxygenation and sodium bicarbonate in hemorrhagic hypotension. Brit J Surg. (1965) 52:704–8. doi: 10.1002/bjs.1800520918
13. Cowley RA, Attar S, Blair E, Esmond WG, Michaelis M, Olloartr. Prevention and treatment of shock by hyperbaric oxygenation. Ann NY Acad Sci. (1965) 117:673–83. doi: 10.1111/j.1749-6632.1964.tb56314.x
14. Elliot DP, Paton BC. Effect of 100% oxygen at 1 and 3 atmospheres on dogs subjected to hemorrhagic hypotension. Surgery. (1965) 57:401–8.
15. Attar S, Scanlan E, Cowley RA. Further evaluation of hyperbaric oxygen in hemorrhagic shock. In: Brown IW, Cox B, editors. Proceedings of the Third International Congress on Hyperbaric Medicine. Washington DC: NAS/NRC (1965), p. 417–24.
16. Jacobson YG, Keller MI, Mundth ED, DeFalco AJ, McClenehan JE. Hyperbaric oxygen therapy in experimental hemorrhagic shock. In: Brown IW, Cox B, editors. Proceedings of the third international congress on hyperbaric medicine. Washington DC: NAS/NRC (1965), p. 93–6.
17. Jacobson YG, Keller MI, Mundth ED, Defalco AJ, McClenethan JE. Hemorrhagic shock: influence of hyperbaric oxygen on metabolic parameters. Calif Med. (1966) 105:93–6.
18. Whalen RE, Moor GF, Mauney FM, Brown IW, McIntosh HD. Hemodynamic responses to “Life without blood.” In: Brown IW, Cox B, editors. Proceedings of the Third International Congress on Hyperbaric Medicine. Washington DC: NAS/NRC (1965), p. 402–8.
19. Navarro RU, Ferguson CC. Treatment of experimental hemorrhagic shock by the combined use of hyperbaric oxygen and low-molecular weight dextran. Surg. (1968) 63:775–81.
20. Necas E, Neuwirt J. Lack of erythropoietin in plasma of anemic rats exposed to hyperbaric oxygen. Life Sci. (1969) 8:1221–8. doi: 10.1016/0024-3205(69)90178-7
21. Doi Y, Onji Y. Oxygen deficit in hemorrhagic shock under hyperbaric oxygen. In: Wada J, Iwa JT, editors. Proceedings of the fourth international congress on hyperbaric oxygen. Baltimore, MD: Williams and Wilkins (1970), p. 181–4.
22. Oda T, Takeori M. Effect of viscosity of the blood on increase in cardiac output following acute hemodilation. In: Wada J, Iwa JT, editors. Proceedings of the Fourth International Congress on Hyperbaric Oxygen. Baltimore, MD: Williams and Wilkins (1970), p. 191–6.
23. Trytyshnikov IM. Effect of acute massive blood loss during hyperbaric oxygen therapy on nucleic and metabolism in the albino rat liver. Biull Eksp Biol Med. (1974) 77:611–2. doi: 10.1007/BF00789978
24. Norman JN. Hemodynamic studies in total blood replacement. Biblio Haema. (1975) 41:203–8. doi: 10.1159/000398118
25. Barkova EN, Petrov AV. The effect of oxygen barotherapy on erythropoiesis in the recuperative period following hemorrhagic collapse. Biull Eksp Bio Med. (1976) 81:156–8. doi: 10.1007/BF00801054
26. Luenov AN, Takovlev VN. Role of cerebral nitrogen metabolism in the mechanisms of the therapeutic action of oxygen under elevated pressure in hemorrhagic shock. Biull Eksp Biol Med. (1977) 83:418–20. doi: 10.1007/BF00807483
27. Gross DR, Moreau PM, Jabor M, Welch DW, Fife WP. Hemodynamic effects of dextran-40 on hemorrhagic shock during hyperbaria and hyperbaric hyperoxia. Aviat Space Environ Med. (1983) 54:413–9.
28. Gross DR, Moreau PM, Chaikin BN, Welch DW, Jabor M, Fife WP. Hemodynamic effects of lactated Ringers' solution on hemorrhagic shock during exposure to hyperbaric air and hyperbaric hyperoxia. Aviat Space Environ Med. (1983) 54:701–8.
29. Gross DR, Dodd KT, Welch KW, Fife WP. Hemodynamic effects of 10% dextrose and of dextran-70 on hemorrhagic shock during exposure to hyperbaric air and hyperbaric hyperoxia. Aviat Space Environ Med. (1984) 55:1118–28.
30. Bitterman H, Reissman P, Bitterman N, Melamed Y, Cohen L. Oxygen therapy in hemorrhagic shock. Circ Shock. (1991) 33:183–91.
31. Wen-Ren L. Resection of aortic aneurysms under 3 ATA of hyperbaric oxygenation. In: Bakker DJ, Cramer JS, editors. Proceedings of the Tenth International Congress of Hyperbaric Medicine. Flagstaff, AZ: Best Publishing Co (1992), p. 94–5.
32. Marzella L, Yin A, Darlington D, et al. Hemodynamic responses to hyperbaric oxygen administration in a cat model of hemorrhagic shock. Circ Shock. (1992) 37:12.
33. Adir Y, Bitterman N, Katz E, Melamed Y, Bitterman H. Salutary consequences of oxygen therapy or long-term outcome of hemorrhagic shock in awake, unrestrained rats. Undersea Hyperb Med. (1995) 22:23–30.
34. Yamashita M, Yamashita M. Hyperbaric oxygen treatment attenuates cytokine induction after massive hemorrhage. Am J Physiol Endocrin Metab. (2000) 278:E811–6. doi: 10.1152/ajpendo.2000.278.5.E811
35. Van Meter K. Hyperbaric oxygen therapy as an adjunct to pre-hospital advanced trauma life support. Surg Technol Int. (2011) 21:61–73.
36. Graffeo C, Dishong W. Severe blood loss anemia in a Jehovah's Witness treated with adjunctive hyperbaric oxygen therapy. Am J Emerg Med. (2013) 31:756e3–4. doi: 10.1016/j.ajem.2012.11.013
37. Weaver LK, Hopkins RO, Chan KJ, Churchill S, Elliott CG, Clemmer TP, et al. Hyperbaric oxygen for acute carbon monoxide poisoning. N Engl J Med. (2002) 347:1057–67. doi: 10.1056/NEJMoa013121
38. Takano T, Miyazaki Y, Nashimoto I, Kobayashi K. Effect of hyperbaric oxygen on cyanide intoxication: in situ changes in intracellular oxidation reduction. Undersea Biomed Res. (1980) 7:191–7.
39. Hansen MB, Olson NV, Hyldegaard O. Combined administration of hyperbaric oxygen and hydroxocobalamin improves cerebral metabolism after acute cyanide poisoning in rats. J Appl Physiol. (2013) 115:1254–61. doi: 10.1152/japplphysiol.00516.2013
40. Hanley ME, Murphy-Lavoie HM. Hyperbaric Evaluation and Treatment of Cyanide Toxicity. Treasure Island, FL: StatPearls Publishing (2023).
41. Smilkstein MJ, Bronstein AC, Pickett HM, Rumack BH. Hyperbaric oxygen therapy for severe hydrogen sulfide poisoning. J Emerg Med. (1985) 3:27–30. doi: 10.1016/0736-4679(85)90216-1
42. Van Meter KW. A systematic review of the application of hyperbaric oxygen in the treatment of severe anemia: an evidence-based approach. Undersea Hyperb Med. (2005) 32:61–83.
43. Rogatsky GG, Shifrin EG, Mayevsky A. Acute respiratory distress syndrome in patients after blunt thoracic trauma: the influence of hyperbaric oxygen therapy. Adv Exp Med Biol. (2003) 540:77–85. doi: 10.1007/978-1-4757-6125-2_12
44. Cannellotto M, Duarte M, Keller G, Larrea R, Cunto E, Chediack V, et al. Hyperbaric oxygen as an adjuvant treatment for patients with COVID-19 severe hypoxaemia: a randomised, controlled trial. Emerg Med J. (2002) 39:88–93. doi: 10.1136/emermed-2021-211253
45. Senniappan K, Jeyabalan S, Rangappa P, Kanchi M. Hyperbaric oxygen therapy: can it be a novel supportive therapy in COVID-19? Indian J Anaesth. (2020) 64:835–41. doi: 10.4103/ija.IJA_613_20
46. Koch A, Kahler W, Klapa S, Grams B, van Ooij PAM. The conundrum of using hyperoxia in COVID-19 treatment strategies: may intermittent therapeutic hyperoxia play a helpful role in the expression of the surface receptors ACE2 and furin in lung tissue via triggering of HIF-1a. Intensive Care Med Exp. (2020) 8:53. doi: 10.1186/s40635-020-00323-1
47. Tao Z, Xu J, Chen W, Yang Z, Xu X, Liu L, et al. Anemia is associated with severe illness in COVID-19: a retrospective cohort study. J Med Virol. (2021) 93:1478–88. doi: 10.1002/jmv.26444
48. Ledingham IM. Hyperbaric oxygen in shock. Int Anes Clin. (1969) 7:819–39. doi: 10.1097/00004311-196907040-00007
49. Amonic RS, Cockett ATK, Lonhan PH, Thompson JC. Hyperbaric oxygen therapy in chronic hemorrhagic shock. JAMA. (1969) 208:2051–4. doi: 10.1001/jama.208.11.2051
50. Hart GB. Exceptional blood loss anemia: treatment with hyperbaric oxygen. JAMA. (1974) 228:1028–9. doi: 10.1001/jama.228.8.1028
51. Myking O, Schreinen A. Hyperbaric oxygen in hemolytic crisis. JAMA. (1974) 227:1161–2. doi: 10.1001/jama.227.10.1161
52. Hart GB. Lennon PA, Strauss MB. Hyperbaric oxygen in exceptional acute blood loss anemia. J Hyperbar Med. (1987) 2:205–10.
53. Meyerstein N, Mazor D, Tsach T, et al. Resistance of human red blood cells to hyperbaric oxygen under therapeutic conditions. J Hyperbar Med. (1989) 4:1–5.
54. Young BA, Burns JR. Management of the severely anemic Jehovah's Witness. Ann Int Med. (1992) 119:170. doi: 10.7326/0003-4819-119-2-199307150-00020
55. McLoughlin PL, Cope TM, Harrison JC. Hyperbaric oxygen therapy in management of severe acute anemia in a Jehovah's witness. Anesthesia. (1999) 54:891–5. doi: 10.1046/j.1365-2044.1999.01004.x
56. Hart GB. Hyperbaric oxygen and exceptional blood loss anemia. In: Kindwall EP, Whelan HT, editors. Hyperbaric Medicine Practice, 2nd Edn. Flagstaff, AZ: Best Publishing Co. (2002), p. 741–51.
57. Ueno S, Sakoda M, Kurahara H, Iino S, Minami K, Ando K, et al. Safety and efficacy of early postoperative hyperbaric oxygen therapy with restriction of transfusions in patients with HCC who have undergone partial hepatectomy. Langenbecks Arch Surg. (2011) 396:99–106. doi: 10.1007/s00423-010-0725-z
58. Van Meter, KW. Hyperbaric oxygen in resuscitation. In: Jain KK, , editor. Textbook of Hyperbaric Medicine. New York, NY: Springer International (2017), p. 551–66. doi: 10.1007/978-3-319-47140-2_42
59. Hafner S. Beloncle F, Koch A, Radermacher P, Asfar P. Hyperoxia in intensive care, emergency and peri-operative medicine: Dr. Jekyll or Mr. Hyde? A 2015 update. Ann Intensive Care. (2015) 5:42. doi: 10.1186/s13613-015-0084-6
60. Schmidt H, Kjaergaard J, Hassager C, Mølstrøm S, Grand J, Borregaard B, et al. Oxygen targets in comatose survivors of cardiac arrest. N Engl J Med. (2022) 387:1467–76. doi: 10.1056/NEJMoa2208686
61. Llitjos JF, Mira JP, Duranteau J, Cariou A. Hyperoxia toxicity after cardiac arrest: what is the evidence? Ann Intensive Care. (2016) 6:23. doi: 10.1186/s13613-016-0126-8
62. Hendricks PL, Hall DA, Hunter WL, Haley PJ. Extension of pulmonary oxygen tolerance in man at 2 ATA by intermittent oxygen exposure. J Appl Physiol Respir Environ Exerc Physiol. (1977) 42:593–9. doi: 10.1152/jappl.1977.42.4.593
63. Balestra C, Germonpre P, Poortmans JR, Marroni A. Serum erythropoietin levels in healthy humans after a short period of normobaric and hyperbaric oxygen breathing: the “normobaric oxygen paradox”. J Appl Physiol. (2006) 100:512–8. doi: 10.1152/japplphysiol.00964.2005
64. Van Meter KW. Hyperbaric oxygen and exceptional blood loss anemia. In: Kindwall EP, Whelen HT, editors. Hyperbaric Medicine Practice. Flagstaff, AZ: Best Publishing (2002), p. 741–51.
65. Shoemaker WC, Appel PL, Kram HB. Tissue oxygen debt as a determinant of lethal and nonlethal postoperative organ failure. Crit Care Med. (1988) 16:1117–20. doi: 10.1097/00003246-198811000-00007
66. Van Meter KW. Hyperbaric oxygen clinical application in resuscitation from insult from acute severe anemia. Wound Care Hyperbar Med. (2014) 5:27–32.
67. Vaquer S, de Haro C, Peruga P, Oliva JC, Artigas A. Systematic review and meta-analysis of complications and mortality of veno-venous extracorporeal membrane oxygenation for refractory acute respiratory arrest distress syndrome. Ann Intensive Care. (2017) 7:51–64. doi: 10.1186/s13613-017-0275-4
68. Heyboer M, Sharma D, Santiago W, McCulloch N. Hyperbaric oxygen therapy: side effects defined and quantified. Advan Wound Care. (2017) 6:210–24. doi: 10.1089/wound.2016.0718
70. Plafki C, Peters P, Avmeling M, Welslau W, Busch R. Complications and side effects of hyperbaric oxygen therapy. Aviat Space Environ Med. (2000) 71:119–24.
71. Hayanga JWA, Aboagye J, Bush E, Canner J, Hayanga HK, Klingbeil A, et al. Contemporary analysis of changes and mortality in the use of extracorporeal membrane oxygenation: a cautionary tale. HTCVS Open. (2020) 1:61–70. doi: 10.1016/j.xjon.2020.02.003
72. Gomez-Castillo JD, Bennett MH. The cost of hyperbaric therapy at the Prince of Wales Hospital, Sidney. SPUMS J. (2005) 35:194–8.
73. Hajhosseini B, Kuehlmann BA, Bonham CA, Kamperman KJ, Gurtner GC. Hyperbaric oxygen therapy: descriptive review of the technology and current application in chronic wounds. Plast Reconstr Surg Glob Open. (2020) 8:e3136. doi: 10.1097/GOX.0000000000003136
74. Wojan F, Stray-Gundersen S, Nagel MJ, Lalande S. Short exposure to intermittent hypoxia increases erythropoietin level in healthy individuals. J Appl Physiol. (2021) 130:1955–60. doi: 10.1152/japplphysiol.00941.2020
75. Waypa GB, Smith KA, Schumacker PT. Oxygen sensing, mitochondria, and ROS signaling: the fog is lifelong. Mol Aspects Med. (2016) 47–48:76–89. doi: 10.1016/j.mam.2016.01.002
76. Avashalumov MV, Chen BT, Koos T, Tepper JM, Rice ME. Endogenous hydrogen peroxide regulates the excitability of midbrain dopamine neurons via ATP-sensitive potassium channels. J Neurosci. (2005) 25:4222–31. doi: 10.1523/JNEUROSCI.4701-04.2005
77. Ribas V, Garcia Ruiz C, Fernandez-Checa JC. Glutathione and mitochondria. Front Pharmacol. (2014) 5:151. doi: 10.3389/fphar.2014.00151
78. Chen HB, Chan YT, Hung AC, Tsai YC, Sun SH. Elucidation of ATP-stimulated stress protein expression of RBA-2 type-2 astrocytes: ATP potentiate HSP60 and Cu/Zn S0D expression and stimulates pI shift of peroxiredoxin II. J Cell Biochem. (2006) 97:314–26. doi: 10.1002/jcb.20547
79. Shi Y, Tang M, Sun C, Pan Y, Liu L, Long Y, Zheng Z. ATP mimics pH-dependent dual peroxidase-catalase activities driving H2O2 decomposition. CCS Chem. (2019) 1:373–83. doi: 10.31635/ccschem.019.20190017
80. Hargreaves DC, Crabtree GR. ATP-dependent chromatin remodeling: genetics, genomics, and mechanisms. Cell Res. (2011) 21:396–420. doi: 10.1038/cr.2011.32
81. Runge JS, Raab JR, Magnuson T. Epigenetic regulation by ATP-dependent chromatin remodeling enzymes: SNF-ing out crosstalk. Curr Top Dev Biol. (2016) 117:1–13. doi: 10.1016/bs.ctdb.2015.10.009
82. Jewatt M, Miller ML, Chen Y, Swartz JR. Continued protein synthesis at low [ATP] and [GTP] enables cell adaptation during energy limitation. J Bacteriol. (2009) 191:1083–91. doi: 10.1128/JB.00852-08
83. Ou X, Lao Y, Xu J, Wutthinitikornkit Y, Shi R, Chen X, et al. ATP can efficiently stabilize protein through a unique mechanism. JACS Au. (2021) 1:1766–77. doi: 10.1021/jacsau.1c00316
84. Verma DD, Levchenko TS, Bernstein EA, Torchilin VP. ATP-loaded liposomes effectively protect mechanical functions of the myocardium from global ischemia in an isolated rat heart model. J Control Release. (2005) 108:460–71. doi: 10.1016/j.jconrel.2005.08.029
85. Mo Y, Sarojini H, Wan R, Zhang Q, Wang J, Eichenberger S, et al. Intracellular ATP delivery causes rapid tissue regeneration via upregulation of cytokines, chemokines and stem cells. Front Pharmacol. (2020) 10:1502. doi: 10.3389/fphar.2019.01502
86. Eltzchig HK, Eckle T, Mager A, Küper N, Karcher C, Weissmüller T, et al. ATP release from activated neutrophils occurs via connexin 43 and modulates adenosine-dependent endothelial cell function. Circ Res. (2006) 99:1100–8. doi: 10.1161/01.RES.0000250174.31269.70
87. Thom SR, Mendiguren I, Hardy K, Bolotin T, Fisher D, Nebolon M, et al. Inhibition of human neutrophil beta-2-ntegrin-dependent adherence by hyperbaric oxygen. Am J Physiol. (1997) 272:C770–7. doi: 10.1152/ajpcell.1997.272.3.C770
88. Yilmaz TU, Yazihan N, Dalgic A, Kaya EE, Salman B, Kocak M, et al. Role of ATP-dependent K channels in the effects of erythropoietin in renal ischaemia injury. Indian J Med Res. (2015) 141:807–15. doi: 10.4103/0971-5916.160713
89. Goto T, Ubukawa I, Kobayashi I, Sugawara K, Asanuma K, Sasaki Y, et al. ATP produced by anaerobic glycolysis is essential for enucleation of human erythroblasts. Exp Hematol. (2019) 72:14–26.e1. doi: 10.1016/j.exphem.2019.02.004
90. Mallouk Y, Vayssier-Tassat M, Bonventre JV, Polla BS. Heat shock protein 70 and ATP as partners in cell homeostasis. Int J Mol Med. (1999) 4:463–74. doi: 10.3892/ijmm.4.5.463
91. Brown GC, Borutaite V. Nitric oxide and mitochondrial respiration in the heart. Cardiovas Res. (2007) 75:283–90. doi: 10.1016/j.cardiores.2007.03.022
92. Cerychova R, Pavlinkova G. HIF-1, metabolism, and diabetes in the embryonic and adult heart. Front Endocrinol. (2018) 9:460. doi: 10.3389/fendo.2018.00460
93. Wilson DF, Erecinska M, Drown C, Silver IA. The oxygen dependence of cellular energy metabolism. Arch Biochem Biophys. (1979) 195:485–93. doi: 10.1016/0003-9861(79)90375-8
94. Krogh A. The number and distribution of capillaries in muscles with calculations of the oxygen pressure head necessary for supplying the tissue. J Physiol. (1919) 52:409–15. doi: 10.1113/jphysiol.1919.sp001839
95. Tissue oxygen measurements. In: David JC, Hunt TK, editors. Problem Wounds: The Role of Oxygen. New York, NY: Elsevier Science Publishing (1977), p. 17–51.
96. Brummelkamp WH, Hogendijk J, Boerema I. Treatment of anaerobic infections (clostridial myositis) by drenching the tissues with oxygen under high atmospheric pressure. Surg. (1961) 49:299–302.
97. Gjedde A. Diffusive insights: on the disagreement of Christian Bohr and August Krogh at the Centennial of the Seven Little Devils. Adv Physiol Educ. (2010) 34:174–85. doi: 10.1152/advan.00092.2010
98. Harch PG. New scientific definitions: hyperbaric therapy and hyperbaric oxygen therapy. Med Gas Res. (2023) 13:92–3. doi: 10.4103/2045-9912.356475
99. O'Neill OJ, Smykowski E, Marker JA, Perez L, Gurash S, Sullivan J. Proof of concept study using modified Politzer inflation device as a rescue modality for treating Eustachian tube dysfunction during hyperbaric oxygen treatment in a multiplace (Class A) chamber. Undersea Hyperb Med. (2019) 46:55–61. doi: 10.22462/01.03.2019.6
100. Kronlund P, Lind F, Olsson D. Hyperbaric critical care patient data management system. Diving Hyperb Med. (2012) 42:85–7.
101. Bessereau J, Aboab J, Hullin T, Huon-Bessereau A, Bourgeois JL, Brun PM, et al. Safety of hyperbaric oxygen therapy in mechanically ventilated patients. Int Marit Health. (2017) 68:46–51. doi: 10.5603/IMH.2017.0008
102. Nichols D, Nielsen ND. Oxygen delivery and consumption: a macrocirculatory perspective. Crit Care Clin. (2010) 26:239–53. doi: 10.1016/j.ccc.2009.12.003
103. Clarke R. Monoplace chamber treatment of decompression illness: review and commentary. Diving Hyperb Med. (2020) 50:264–72. doi: 10.28920/dhm50.3.264-272
104. Clarke R. Health care worker decompression sickness: incidence, risk and mitigation. Undersea Hyperb Med. (2017) 44:509–19. doi: 10.22462/11.12.2017.2
105. McLellan SA, Walsh TS. Oxygen delivery and hemoglobin. Cont Educ in Anaes Crit Care Pain. (2004) 4:123–6. doi: 10.1093/bjaceaccp/mkh033
106. Mik EG, Balestra GM, Harms FA. Monitoring mitochondrial pO2: the next step. Curr Opin Crit Care. (2020) 26:289–95. doi: 10.1097/MCC.0000000000000719
107. Tzameli I. The evolving role of mitochondria in metabolism. Trends Endocrinol Metab. (2012) 23:417–9. doi: 10.1016/j.tem.2012.07.008
108. Tornroth-Horsefield S, Neutz R. Opening and closing the metabolite gate. Proc Natl Acad Sci USA. (2008) 105:19565–6. doi: 10.1073/pnas.0810654106
109. Burmester T, Hankeln T. Neuroglobin: a respiratory protein of the nervous system. News Physiol Sci. (2004) 19:110–3. doi: 10.1152/nips.01513.2003
110. Yoshizato K, Thuy LTT, Shiota G, Kawata N. Discovery of cytoglobin and its roles in physiology and pathology of hepatic stellate cells. Proc Jpn Acad Ser B Phys Biol Sci. (2016) 92:77–87. doi: 10.2183/pjab.92.77
111. Endeward V, Gros G, Jurgens KD. Significance of myoglobin as an oxygen store and oxygen transporter in the intermittently perfused heart: a model study. Cardiovas Res. (2010) 87:22–9. doi: 10.1093/cvr/cvq036
112. Siddiqi A, Davidson JD, Mustoe TA. Ischemic tissue oxygen capacitance after hyperbaric oxygen therapy: a new physiologic concept. Plast Resconst Surg. (1992) 99:148–55. doi: 10.1097/00006534-199701000-00023
113. Van Meter KW. Hyperbaric transport: New Orleans could host the first hyperbaric ambulance concept. Underwater. (2021) 2:16−9.
114. Leveque C, Mrakic-Sposta S. Lafere, Vezzoli A, Germonpré P, Beer A, et al. Oxidative stress response's kinetics after 60 minutes at different (30% or 100%) normobaric hyperoxia exposures. Int J Mol Sci. (2022) 24:664. doi: 10.3390/ijms24010664
115. Leveque C, Sposta SM, Theunissen S, Germonpré P, Lambrechts K, Vezzoli A. et al. Oxidative stress response kinetics after 60 minutes at different (14 ATA and 25 ATA) hyperbaric hyperoxia exposures. Int J Mol Sci. (2023) 23:12361. doi: 10.3390/ijms241512361
116. Leveque C. Mrakic-Sposta S, Theunissen S, Theunissen S, Germonpré P, Lambrechts K, et al. Oxidative stress response kinetics after 60 minutes at different levels (10% or 15%) of normobaric hypoxia exposure. Int J Mol Sci. (2023) 24:10188. doi: 10.3390/ijms241210188
117. Hadanny A, Efrati S. The hyperoxic-hypoxic paradox. Biomolecules. (2020) 10:958. doi: 10.3390/biom10060958
118. Lafere P, Schubert T, De Bels D, Germonpré P, Balestra C. Can the normobaric oxygen paradox (NOP) increase reticulocyte count after traumatic hip surgery? J Clin Anesth. (2013) 25:129–34. doi: 10.1016/j.jclinane.2012.06.021
119. De Bels D, Corazza F, Germonpre P, Balestra C. The normobaric oxygen paradox: a novel way to administer oxygen as an adjuvant treatment for cancer? Med Hypotheses. (2011) 76:467–70. doi: 10.1016/j.mehy.2010.11.022
120. Burk R. Oxygen breathing may be a cheaper and safer alternative to exogenous erythropoietin (EPO). Med Hypotheses. (2007) 69:1200–4. doi: 10.1016/j.mehy.2007.03.015
121. Van Meter KW, Weiss LD, Harch PG. Hyperbaric oxygen in emergency medicine. In: Jain KK, editors. Textbook of Hyperbaric Medicine, 3rd Edn. Seattle, WA: Hogrefe & Huber (1999), p. 557–88.
122. Kot J, Sicko Z, Doboszynski T. The extended oxygen window concept for programming saturation decompressions using air and nitrox. PLoS ONE. (2015) 10:e130835. doi: 10.1371/journal.pone.0130835
123. Tibbles P, Edelsberg JD. Hyperbaric oxygen therapy. N Engl J Med. (1996) 334:1642–8. doi: 10.1056/NEJM199606203342506
124. Kjellbert A, Douglass J, Pawlik MT, Kraus M, Oscarsson N, Zheng X, et al. Randomised, controlled, open label, multicentre clinical trial to explore safety and efficacy of hyperbaric oxygen for preventing ICU admission, morbidity and mortality in adult patients with COVID-19. BMJ Open. (2021) 11:e046738. doi: 10.1136/bmjopen-2020-046738
Keywords: anemia, normobaric oxygen, hyperbaric oxygen, adenosine triphosphate, advanced cardiac life support, advanced trauma life support, oxygen debt, normobaric oxygen paradox
Citation: Van Meter KW (2024) Hyperbaric oxygen therapy in the ATLS/ACLS resuscitative management of acutely ill or severely injured patients with severe anemia: a review. Front. Med. 11:1408816. doi: 10.3389/fmed.2024.1408816
Received: 28 March 2024; Accepted: 19 August 2024;
Published: 08 October 2024.
Edited by:
Carmine Siniscalchi, University of Parma, ItalyReviewed by:
Lucia Prezioso, University Hospital of Parma, ItalyAlfredo Caturano, University of Campania Luigi Vanvitelli, Italy
Costantino Balestra, Haute École Bruxelles-Brabant (HE2B), Belgium
Copyright © 2024 Van Meter. This is an open-access article distributed under the terms of the Creative Commons Attribution License (CC BY). The use, distribution or reproduction in other forums is permitted, provided the original author(s) and the copyright owner(s) are credited and that the original publication in this journal is cited, in accordance with accepted academic practice. No use, distribution or reproduction is permitted which does not comply with these terms.
*Correspondence: Keith W. Van Meter, a3Zhbm1lJiN4MDAwNDA7bHN1aHNjLmVkdQ==