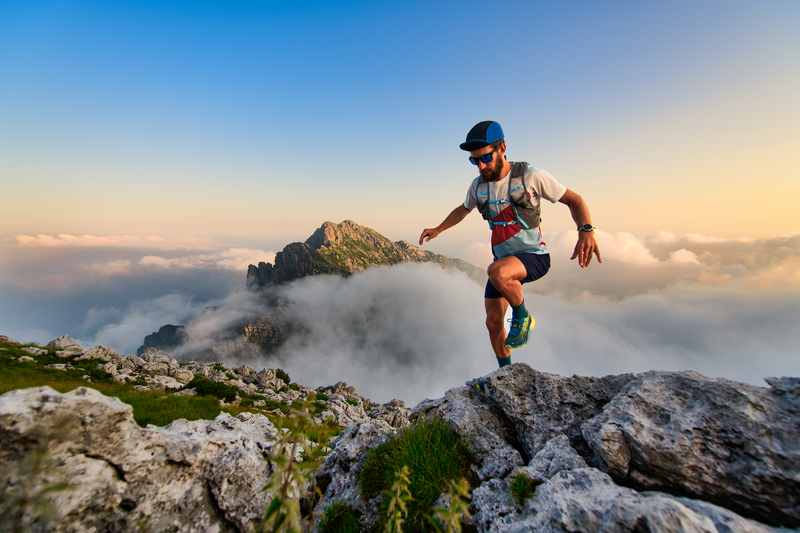
94% of researchers rate our articles as excellent or good
Learn more about the work of our research integrity team to safeguard the quality of each article we publish.
Find out more
REVIEW article
Front. Med. , 30 July 2024
Sec. Gastroenterology
Volume 11 - 2024 | https://doi.org/10.3389/fmed.2024.1406547
This article is part of the Research Topic Biology of Mesenchymal Stromal Cells -Emerging New Concepts to Improve Function and Scalability View all 10 articles
A correction has been applied to this article in:
Corrigendum: Therapeutic role of extracellular vesicles from human umbilical cord mesenchymal stem cells and their wide therapeutic implications in inflammatory bowel disease and other inflammatory disorder
The chronic immune-mediated inflammatory condition known as inflammatory bowel disease (IBD) significantly affects the gastrointestinal system. While the precise etiology of IBD remains elusive, extensive research suggests that a range of pathophysiological pathways and immunopathological mechanisms may significantly contribute as potential factors. Mesenchymal stem cells (MSCs) have shown significant potential in the development of novel therapeutic approaches for various medical conditions. However, some MSCs have been found to exhibit tumorigenic characteristics, which limit their potential for medical treatments. The extracellular vesicles (EVs), paracrine factors play a crucial role in the therapeutic benefits conferred by MSCs. The EVs consist of proteins, microRNAs, and lipids, and are instrumental in facilitating intercellular communication. Due to the ease of maintenance, and decreased immunogenicity, tumorigenicity the EVs have become a new and exciting option for whole cell treatment. This review comprehensively assesses recent preclinical research on human umbilical cord mesenchymal stem cell (hUC-MSC)-derived EVs as a potential IBD therapy. It comprehensively addresses key aspects of various conditions, including diabetes, cancer, dermal injuries, neurological disorders, cardiovascular issues, liver and kidney diseases, and bone-related afflictions.
Inflammatory bowel disease (IBD) refers to a group of chronic inflammatory disorders of the gastrointestinal tract, with two primary subtypes: Crohn’s disease (CD) and ulcerative colitis (UC) (1). These disorders result from an abnormal immune response in genetically susceptible individuals, triggered by environmental factors. The characteristic symptoms include abdominal pain, diarrhea, rectal bleeding, weight loss, and fatigue (2). The chronic and relapsing nature of IBD often leads to a diminished quality of life and a higher risk of complications such as bowel strictures, abscesses, and even colorectal cancer. Over the years, various treatment modalities have been developed to treat patient with IBD including anti-inflammatory medications, immunosuppressant, biological therapies, and surgical interventions (3). While these treatments have been effective for many patients, they are not without limitations. Long-term use of immunosuppressive drugs can lead to increased susceptibility to infections, and biologic therapies often come with a high financial burden. Moreover, a significant proportion of IBD patients do not respond adequately to existing treatments, highlighting the urgent need for novel therapeutic strategies (4).
The complexities of IBD, including its multifactorial etiology and heterogeneity in disease presentation, pose a challenge for clinicians seeking to tailor treatment approaches to individual patients (5). Currently, a significant proportion of the etiology and pathology underlying this condition remains elusive to the scientific community. Nevertheless, it is widely acknowledged that the condition is characterized by a polygenic and multifactorial nature (6). Incorporating the insights obtained from numerous recent studies, the fundamental elements contributing to the onset of IBD involve genetic interactions, dysregulated mucosal immune responses prompted by environmental factors, and disruptions in the regulation of the gut microbiota (6). Currently, several treatment protocols, including immunomodulatory, thiopurine agents, and monoclonal antibodies targeting tumor necrosis factor (anti-TNF), are employed for the management of IBD. However, these treatments have been found to lack the attainment of sufficiently favorable therapeutic outcomes (7). Consequently, researchers are actively exploring the development of advanced clinical techniques and strategies for the treatment of IBD. In this context, the search for safer and more effective therapies has led researchers to explore the regenerative potential of mesenchymal stem cells (MSCs) and their extracellular vesicles (EVs) (8, 9). MSCs are multipotent, adult stem cells found in various tissues, including bone marrow, adipose tissue, and the umbilical cord (10). These cells have garnered immense interest in the field of regenerative medicine due to their remarkable self-renewal capabilities and their ability to differentiate into multiple cell lineages, including osteocytes, adipocytes, and chondrocytes (11, 12). Moreover, the quantity of stem cells and their capacity for proliferation and differentiation exhibited a marked decline with advancing age (13), thereby imposing limitations on the application of these cells in clinical trials (14). The morphological characteristics, immunophenotype, proliferation rate, multi-directional differentiation capacity, and their potential to induce hematopoietic stem cell (HSC) differentiation in umbilical cord-derived mesenchymal stem cells (UC-MSCs) closely resemble those observed in bone marrow-derived mesenchymal stem cells (BM-MSCs) (15), but, it is noteworthy that UC-MSCs display a heightened proliferative capacity and lower levels of human leukocyte antigen (HLA)-ABC and HLA-DR expression in comparison to BM-MSCs (16). Furthermore, it is worth noting that UC-MSCs exhibit a diverse array of stem cell types, making them readily available and easily collectable resource that can be efficiently preserved (17, 18). Consequently, UC-MSCs assume a distinctive role in diminishing both the frequency and severity of graft-versus-host disease (GVHD), a complication that affects over 50% of patients undergoing hematopoietic stem cell transplantation (HSCT) (18). Moreover, owing to their inherent migratory potential towards cancer cells, numerous studies have suggested the utilization of UC-MSCs in cell-based therapies aimed at targeting tumors and facilitating the localized delivery of anti-cancer agents (19). Nonetheless, several facets of research concerning UC-MSCs are still in their early stages of development. UC-MSCs are considered optimal candidate cells for cell replacement therapy, primarily due to their minimal immunogenicity, robust proliferative potential, and capacity for differentiation (20).
The therapeutic effectiveness of MSCs do not exclusively hinge on their ability to differentiate into various cell types. Instead, their paracrine effects, which involve the release of trophic factors and EVs, assume a central role in promoting tissue repair and modulating the immune response (21). MSCs can orchestrate a coordinated response by influencing local cell populations, reducing inflammation, and promoting tissue regeneration (22). The secretion of EVs, in particular, has gained significant attention for their role in intercellular communication and their potential as therapeutic agents (23). EVs are small membranous vesicles released by virtually all cell types, including MSCs. They are involved in cell-to-cell communication and serve as vehicles for the transfer of bioactive molecules, including proteins, lipids, and nucleic acids, between cells (24, 25). EVs are classified into several subtypes, including exosomes, macrovesicles, and apoptotic bodies, based on their biogenesis and size. Among these, exosomes, typically ranging from 30 to 150 nanometers in diameter, have garnered significant interest for the therapeutic potential (26). Exosomes are released into the extracellular space through the fusion of multivesicular bodies with the plasma membrane (27). Extensive investigations have revealed the secretion of exosomes by a wide range of cell types, including mast cells, dendritic cells (27), B cells (28), T cells (29), tumor cells (30), and epithelial cells. Exosomes have also been found in numerous kinds of body fluids, such as saliva, urine, breast milk, and plasma (31, 32) urine (33, 34). Exosomes, like their parent cells, carry a cargo of bioactive molecules that can modulate various cellular processes (35, 36). These molecules include growth factors, cytokines, microRNAs, and lipids, all of which can influence recipient cells’ behavior (37, 38). This cargo is carefully packaged within the exosome’s lipid bilayer, protecting it from degradation and ensuring its efficient delivery to target cells (39). This unique characteristic makes exosomes ideal candidates for therapeutic interventions, as they can harness the regenerative power of their parent cells in a more controlled and targeted manner (40, 41). Exosomes originating from diverse sources exert an influence on the etiology of IBD (42). The significance of intercellular communication in maintaining homeostasis in multicellular organisms has been well-documented previously. Consequently, exosomes, which are secreted by a majority of cells, play a critical role in forming a network and actively participating in intracellular signaling (43). They facilitate the transfer of bioactive components including, lipids, nucleic acids, and proteins from one cell to another, thereby initiating biological responses in the recipient cells (44). Exosomes have demonstrated a greater efficacy than their parent cells and can be stored without compromising their functionality, making them an appealing focus of research. Recently, there has been an increasing interest in utilizing exosome administration as a novel therapeutic strategy to expedite preclinical research endeavors (45, 46). Furthermore, exosome-delivered miRNAs contribute to lymphangiogenesis and play a role in IBD. Exosomes derived from adipose tissue-derived MSCs modulate the miRNA-132/TGF-β pathway, thereby promoting VEGF-C-dependent lymphangiogenesis (47). MSC-derived exosomes have been substantiated to augment angiogenesis in endothelial cells by transporting miR-125a (48). Conversely, BM-MSCs promote lymphangiogenesis through the secretion of VEGF-A, which stimulates lymphatic endothelial cells (LECs) to activate the VEGFR-2 pathway (49).
The therapeutic potential of EVs, including those derived from MSCs, extends beyond their immunomodulatory effects (50, 51). Studies have demonstrated that MSC-derived EVs can promote tissue repair and regeneration in various disease models, including myocardial infarction, stroke, and cartilage injury (52). The cargo carried by these vesicles plays a pivotal role in modulating the recipient cell’s behavior, promoting angiogenesis, reducing fibrosis, and enhancing tissue remodeling (52). The isolation and characterization of human umbilical cord mesenchymal stem cell (hUC-MSC)-derived EVs represent a critical step in harnessing their therapeutic potential (53). Researchers have developed various methods to isolate and purify these vesicles, including ultracentrifugation, size exclusion chromatography, and immunoaffinity-based techniques (54).
These methods ensure the enrichment of exosomes and other EV subtypes from hUC-MSC culture supernatants, allowing for their subsequent analysis and utilization (55). One of the most striking features of hUC-MSC-derived EVs is their ability to modulate the immune response (56). These vesicles can suppress the activation of pro-inflammatory immune cells while promoting the expansion of regulatory T cells and M2 macrophages, thus shifting the immune milieu towards an anti-inflammatory and tissue-healing phenotype (57). This immunomodulatory capacity has profound implications for the treatment of immune-mediated disorders like IBD (58). Recent preclinical studies and early-phase clinical trials have provided compelling evidence of the therapeutic potential of hUC-MSC-derived EVs in the management of IBD (59). These studies have shown that the administration of hUC-MSC-derived EVs can ameliorate disease symptoms, reduce inflammation, and promote mucosal healing in animal models and human patients with IBD. The mechanisms underlying these effects involve the immunomodulatory properties of the vesicles, as well as their ability to enhance epithelial barrier function and promote tissue repair (60, 61). The therapeutic potential of hUC-MSC-derived EVs extends beyond gastrointestinal disorders. Researchers are exploring their use in various neurological disorders, such as Parkinson’s disease, Alzheimer’s disease, and spinal cord injury (SCI) (62, 63). These vesicles have shown promise in promoting neuroprotection, reducing inflammation, and enhancing neural tissue repair in preclinical models (64). Cardiovascular diseases, including myocardial infarction and heart failure, are leading causes of morbidity and mortality worldwide. The hUC-MSC-derived EVs have emerged as potential candidates for cardiac regeneration and repair. Their ability to stimulate angiogenesis, reduce oxidative stress, and modulate immune responses has made them attractive for the treatment of cardiovascular disorders (65, 66). Musculoskeletal disorders, such as osteoarthritis and bone fractures, present significant challenges in the field of regenerative medicine. The hUC-MSC-derived EVs have shown promise in promoting bone and cartilage regeneration by enhancing the proliferation and differentiation of osteoblasts and chondrocytes. These vesicles may offer a minimally invasive and cell-free alternative to traditional treatments (67).
While the therapeutic potential of hUC-MSC-derived EVs is promising, several challenges must be addressed before their widespread clinical adoption. Standardization of isolation and characterization methods, determination of optimal dosing regimens, and long-term safety assessments are crucial steps in the path to clinical translation (54). Moreover, regulatory approvals and manufacturing scalability need to be addressed to ensure the accessibility of these therapies to a broader patient population. The heterogeneity of diseases like IBD underscores the importance of personalized medicine. Identifying biomarkers that can predict patient responses to hUC-MSC-derived EV therapy is a critical research area. Biomarker discovery will enable clinicians to select the most appropriate patients for treatment and tailor therapy regimens accordingly, maximizing therapeutic outcomes (68). As the field of regenerative medicine advances, ethical and regulatory considerations become increasingly important (69). Ensuring the ethical sourcing of umbilical cord tissue and transparent reporting of research findings is essential. Regulatory agencies must also develop clear guidelines to govern the production and clinical use of hUC-MSC-derived EVs, balancing innovation with patient safety (61). The therapeutic role of hUC-MSC-derived EVs represent a promising frontier in the treatment of IBD and a wide array of other disorders (70). With their ability to modulate the immune response, promote tissue repair, and enhance regenerative processes, hUC-MSC-derived EVs hold the potential to revolutionize the way we approach the management of chronic and debilitating conditions (71).
This study has undertaken an exploration of the complexities surrounding IBD, delving into the regenerative capabilities of MSCs, revealing the therapeutic potential inherent in EVs, and engaging in a discussion concerning the promising prospects of hUC-MSC-EVs within the context of IBD. The journey towards fully harnessing the therapeutic potential of these minuscule communicators is an ongoing endeavor, replete with a myriad of challenges and opportunities that await both researchers and clinicians. As we continue to venture deeper into the realm of regenerative medicine, we stand at the precipice of uncovering innovative solutions that have the potential to transform the lives of individuals grappling with chronic and presently incurable diseases. This review underscores the paramount importance of sustained research and clinical progress within this promising field, emphasizing the potential of hUC-MSC-EVs as an exceptional therapeutic avenue not only for IBD but also for a spectrum of inflammatory conditions.
The release of EVs is a fundamental biological process in both prokaryotic and eukaryotic cells, occurring under normal physiological conditions as well as in aberrant situations. Despite being written off in the past as little more than biological waste with little significance, recent studies have highlighted their crucial function as bioactive transporters. These vesicles mediate a wide range of biological processes and act as transporters of many cellular components, enabling complex cellular communications (72). Proteins such as cell surface receptors, signaling proteins, transcription factors, enzymes, and extracellular matrix proteins are among the diverse cargo carried by EVs (73). Additionally, they have lipids and nucleic acids (DNA, mRNA, and miRNA) that can be transferred from donor to recipient cells to facilitate molecular transfer and intercellular communication (74). It has been discovered that EVs are linked to pathological conditions like cancer, heart disease, and neurological illnesses (75). EVs comprise a range of subtypes that are categorized based on their mechanisms of synthesis and release, such as exosomes, apoptotic blebs, and other EV subgroups (76). Additionally, they can be categorized according to the type of cell from which they originated, such as endothelium or platelet-derived cells, or according to the physiological state of the cells, such as “prostasomes” coming from the prostate and “oncosomes” originating from cancer cells. The primary components of EVs include apoptotic bodies, exosomes, and microvesicles (77). However, other forms have been discovered recently, including membrane particles, large oncosomes, migrasomes (78), ectosomes (78), exomeres (79) and supermeres (Table 1).
Recently, EVs have emerged as a promising therapeutic option for a range of diseases and conditions, including BD (91). In animal models of IBD, studies have provided evidence showing that EVs derived from various sources, including MSCs, possess the ability to mitigate inflammation and facilitate tissue healing (92). Using EVs as a therapeutic alternative presents several challenges that need to be addressed. In the preparation of EVs, standardization of isolation and characterization is a significant challenge in maintaining the purity and quality of EVs (93). The immunogenicity potential and pro-coagulant effects are two additional safety concerns associated with EV-based medicines (94). Despite these obstacles, EV-based medicines are still being clinically researched and developed, demonstrating significant potential for the treatment of various inflammatory illnesses including IBD. Herein, the MSC-derived-exosomes are the main focus of the study to provide comprehensive use, specifically in the treatment of IDB and other pathological inflammatory conditions in general.
Exosomes, a type of EVs, which originate from endosomes, typically exhibit an average diameter of approximately 50–100 nm (95). The plasma membrane undergoes a sequential process of invagination, leading to the eventual formation of multivesicular bodies (MVBs). These MVBs have the ability to interact with other intracellular vesicles and organelles, thereby contributing to the diversity of components found in exosomes. Depending on the specific cell they originate from, exosomes, can encompass a wide range of cellular constituents, such as DNA, RNA, lipids, metabolites, as well as cytosolic and cell-surface proteins (96).
Exosomes, in particular, have emerged as crucial mediators of cellular communication, playing significant roles in both normal physiological processes, such as lactation (97), immune response (34) and neuronal function (97), and also in the development and progression of diseases, such as liver disease (97), neurodegenerative diseases (98) and cancer. Exosomes are increasingly recognized as promising therapeutic agents for gastrointestinal conditions, IBD, using a cell-free therapeutic strategy (98, 99).
Numerous diseases associated with IBD, characterized by disruptions in mucosal immune responses, compromised intestinal barrier integrity, and alterations in the balance of intestinal microbial populations, are orchestrated through pathways involving exosomal intercellular communication (100). Exosomes, being complex molecules, are discharged into human serum and other bodily fluids, and their functional contents exhibit variations between IBD patients and healthy individuals (101). Consequently, exosomes may have the potential to serve as diagnostic biomarkers that reflect the current state of IBD (101, 102).
Exosomes originate from endosomal vesicles within the cell and exhibit distinctive characteristics that set them apart from other cell-secreted microvesicles (103). They are enclosed by a phospholipid bilayer architecture, with a size ranging from 30 to 150 nanometers and a density falling within the range of 1.13 to 1.19 grams per milliliter (104, 105). When observed through transmission electron microscopy (TEM), exosomes present a cup-shaped morphology, further confirming their identity. Moreover, the presence of specific proteins, including tetraspanins (e.g., CD63, CD9, and CD81) and β-actin, serves as additional markers to differentiate exosomes from other vesicular structures (101, 104). To emphasize their prevalence, it is noteworthy that approximately 1 × 1012 exosomes can be found in just 1 milliliter of blood (105). These distinct characteristics and abundance make exosomes a subject of significant interest in various fields of research and clinical applications (106).
Exosome biogenesis is a highly regulated process that occurs in various cell types under both pathological and physiological conditions. The secretion of exosomes is governed by the modulation of Rab27a and Rab27b expression (107). A diverse array of cell types, including lymphocytes, dendritic cells, fibroblasts, erythrocytes, platelets, mast cells, tumor cells, stem cells, monocytes, macrophages, natural killer (NK) cells, B lymphocytes, and T lymphocytes, are known to synthesize exosomes (108).
The process of exosome biogenesis commences with the internalization of the cell membrane, leading to the formation of small intracellular structures referred to as early endosomes (103–107). Early endosome development involves a progressive maturation process that culminates in the generation of intraluminal vesicles (ILVs). On the other hand, late endosomes, known as multivesicular bodies (MVBs), are formed through the inward folding of segments of the endosomal membrane (106). The fusion of MVBs with the plasma membrane results in the exocytotic release of ILVs into the extracellular space, where they ultimately undergo transformation into exosomes (99–102, 105). This intricate process is visually depicted in Figure 1.
Figure 1. Schematic representation of exosome biogenesis. The process of exosome biogenesis is illustrated schematically in this figure. During exosome biogenesis, multivesicular endosomes (MVEs) initially undergo invagination, resulting in the formation of small intracellular vesicles. These vesicles encapsulate a diverse array of cytoplasmic cargo molecules, including proteins, messenger RNAs (mRNAs), and microRNAs (miRNAs). Notably, MVEs demonstrate two distinct fusion pathways: one involves fusion with the cellular membrane, facilitating the exocytosis of the enclosed exosomes (referred to as intravesicular vesicles). The other pathway entails fusion with lysosomes, leading to the degradation of the contents within MVEs. Consequently, exosomes gain entry into recipient cells through two distinct mechanisms: initially, they traverse the endocytic route, followed by internalization by the recipient cell. Secondly, they can merge directly with the recipient cell’s plasma membrane, subsequently releasing their cargo into the cytoplasm. It’s important to note that cells possess the capacity to generate membrane-derived vesicles that bud directly from the plasma membrane. These vesicles serve as vehicles for transporting functional proteins, RNAs, and other bioactive molecules.
Notably, exosomes are present in a variety of physiological fluids, including serum, as well as in saliva, amniotic fluid, and breast milk (106). Their presence in these fluids underscores the ubiquity of exosomes in biological systems and their potential significance in various biological and clinical contexts.
Exosomes, which are minuscule in size and invisible to the naked eye and standard light microscopes, can only be observed using electron microscopy. Their morphology is characterized by flattened spheres, which can result from the dehydration process required for electron microscopy preparation, leading to their collapse (108, 109). Exosomes are complex structures composed of proteins, particularly those derived from the plasma or endosomal membrane, lipids, and various cytosolic components (Figure 2). It’s important to note that exosomes lack proteins originating from the Golgi apparatus, nuclear pore complex, mitochondria, and endoplasmic reticulum (Figure 2). Exosomes lack proteins of the Golgi apparatus, nuclear pore complex, mitochondria and endoplasmic reticulum (110). Data on exosomal content are systematically curated and updated in databases such as ExoCarta, Vesiclepedia, and EVpedia (111). Although initial investigations suggest the presence of proteins from the cytosol, endosomes, and plasma membrane in exosomes, it is essential to acknowledge that cellular organelles such as mitochondria, the Golgi apparatus, or the nucleus do not exclusively consist of proteins. The establishment of exosomal protein databases has been a collaborative effort among multiple research teams. Exosome repositories like ExoCarta1 and Vesiclepedia2 have cataloged a total of 9,769 proteins, 1,116 lipids, 3,408 mRNAs, and 2,838 miRNAs within exosomes originating from various cellular sources and organisms up to the present day (110). Exosome protein sorting, a newly explored field of study, relies, at least in part, on the endosomal sorting complex required for transport (ESCRT) machinery and protein ubiquitylation (112). During exosome biogenesis, ESCRT orchestrates the formation of intricate structures on the plasma membrane, resembling membranous necks. These structures encompass conical funnels, tubular arrangements, planar spirals, and filaments, which are hypothesized to regulate membrane remodeling processes.
Exosomes encompass a substantial number of transport proteins, including tubulin, actin, and actin-binding molecules (110), in addition to various proteins intricately involved in specific roles within secretory cells (113). Exosomal proteins are categorized into diverse groups based on their family, function, and subcellular localization (114). The most frequently encountered protein categories in exosomes include those related to (i) the formation of multivesicular bodies (MVBs), (ii) transmembrane proteins acting as targeting or adhesion molecules, such as tetraspanins like CD9, CD63, and CD81, which play roles in membrane fusion, (iii) signal transduction proteins such as annexin and 14-3-3 proteins, (iv) cytoskeletal proteins including actin, syntenin, and myosin, (v) chaperones like HSPA8 and HSP90, and (vi) metabolic enzymes, such as GAPDH, LDHA, PGK1, aldolase, and PKM (111, 113). Each individual exosome also contains MHC class I molecules, among distinct components (114), and heat shock proteins (112). These proteins participate in antigen presentation and antigenic peptide attachment to MHC class I molecules (113). Tetraspanin family members CD9, CD63, CD81, and CD82 interact with other transmembrane proteins to facilitate antigen presentation and adhesion. The interaction of CD9 and CD82 with integrins can inhibit the migration and invasion of tumor cells (111). Exosomes exhibit distinctive lipid compositions in addition to their protein content (113). Intriguingly, they are deficient in lysobisphosphatidic acid and intraluminal vesicle (ILV) lipids (115) but contain high levels of sphingomyelin, phosphatidylserine, ceramide, and cholesterol.
Exosomes are recognized for their substantial content of DNA and RNA, alongside lipids and proteins. The term “EV-DNA” refers to DNA enclosed within EVs, spanning a size range of 100 base pairs to 2.5 kilobases (115). Analysis of complete RNA sequencing data from EVs isolated from serum suggests that RNA repeats constitute approximately 50% of the total EV-RNA content. Additionally, miRNAs and tRNAs are estimated to account for up to 15% of the EV-RNA content (115, 116). Certain RNAs are found in higher concentrations in exosomes than in the originating cells, particularly in exosomes from MSCs (116). Numerous studies have provided evidence that RNA can indeed be transferred between cells via exosomes (117). However, the extent to which transferred RNA retains its functionality in recipient cells, as well as the proportion that undergoes fragmentation and subsequent transport, remains an area of ongoing investigation.
MSCs have gained significant prominence in experimental cell-based therapeutic approaches for a variety of human ailments. They are widely employed due to their proven efficacy in numerous disease-related animal models and their excellent safety record in clinical settings. MSCs offer great potential for treating human illnesses because of their ability to differentiate, self-renew, and modulate the immune response (118). While MSCs have garnered attention as a potential cellular treatment for various medical conditions, emerging evidence suggests that their therapeutic benefits are primarily mediated through EVs produced via paracrine processes (119). These EVs play a crucial role in conveying the therapeutic advantages of MSCs. The hUC-MSCs have been the subject of numerous research projects that have explored their potential use for the treatments of various medical problems, including diabetes (120), cancer (121), liver (122), bone (123), cartilage (124), brain (125), cardiovascular issues (126), and IBD (127), and Figure 3 illustrates the therapeutic effects of hUC-MSC, both in in vivo and in vitro environments. The administration of hUC-MSCs demonstrates marked amelioration in pivotal parameters, encompassing the disease activity index, fluctuations in body weight, alterations in colonic length, and histopathological assessments of colitis, predominantly through the mitigation of inflammation. A discernible correlation exists between the dosage of hUC-MSCs and the extent of therapeutic response as these parameters serve as standard indicators in IBD evaluations, reflecting both disease severity and the effectiveness of therapeutic interventions (126–128).
The subcortical endothelium of the umbilical cord, Wharton’s jelly (WJ), and the perivascular area are the primary locations where UC-MSCs are commonly found. Wharton’s Jelly has a structure primarily resembling a sponge, with collagen fibers, proteoglycans, and stromal cells intertwined within it (20). When analyzing UC-MSCs from Wharton’s Jelly using flow cytometry, it was discovered that CD24 and CD108 were abundantly expressed, while the dermal fibroblast marker CD40 and fibroblast-specific markers (FAP and FSP) were not discernible, which highlights the abundance of MSCs in the Wharton’s Jelly region (128, 129). UC-MSCs can exhibit distinctive markers corresponding to various cell lineages, exemplifying their pluripotent nature (130). In contrast to hematopoietic stem cells, UC-MSCs exhibit the expression of MSC-specific markers such as CD105, CD90, and CD73, along with adhesion molecule markers including CD54, CD13, CD29, and CD44. Conversely, UC-MSCs shows reduced or absent expression of surface antigens CD31, CD14, CD34, and CD45 (131). Additionally, these cells are deficient in immune response-related antigens necessary for T lymphocyte activation, including CD80, CD86, CD40, and CD40L, as well as the MHC class II antigen HLA-DR. (132) UC-MSCs exhibit reduced immunogenicity compared to BM-derived cells due to their considerably lower expression levels of CD106 and HLA-ABC (133).
WJ-MSCs, which exhibited the highest proliferation rate, outperformed adipose tissue and BM-MSCs by a factor of three to four in terms of proliferation (134). Based on the inquiry conducted by the Mennan research team, no significant disparity in the rate of proliferation was observed. The population of UC-MSCs exhibited an average twofold increase between passages P0 and P3 within a span of 2–3 days. This rate of expansion significantly exceeds the duration observed for BM-MSCs (135). UC-MSCs have the capacity for multidirectional differentiation, enabling them to differentiate into various tissues such as bone, fat, cartilage, and others (136). Therefore, in the field of regenerative medicine, these cells are considered ideal seed cells due to their potential to facilitate the healing of various tissues and organs (137). According to studies, chemokines are produced by biological tissues that undergo damage due to ischemia-anoxia or persistent inflammation (138). These chemokines aid in recruiting MSCs to the injury site and subsequently regulate their migration to facilitate their differentiation into various cell lineages (139). Under specific conditions in an in vitro setting, UC-MSCs have been observed to exhibit the ability to undergo “trans-differentiation,” resulting in their differentiation into osteoblast-like mesodermal cell types (140), endothelial cells (141), cardiomyocytes (142), as well as ectoderm-derived hepatocytes with potential for neuronal transformation (143), and pancreatic cells (144), bridging the germinal layers within the endoderm.
To maximize the therapeutic effects of EVs, it is essential for researchers to isolate and characterize them. The isolation and characterization of hUC-MSC-EVs hold great importance for their potential therapeutic use in IBD and other associated illnesses (145). However, the extraction of EVs from biological fluids, such as serum or conditioned media, can be challenging due to the heterogeneity of EV populations and the presence of impurities (93). In response to this challenge, various methodologies have been developed to achieve optimal purity and cost-effectiveness of EVs. These techniques include ultracentrifugation, density gradient centrifugation, and size-exclusion chromatography, all aiming to achieve high levels of purity (146). Ultracentrifugation, are commonly used technique for EV extraction, involves differential centrifugation at high speeds, reaching up to 100,000 x g, to pellet EVs (147). This method can be further optimized by utilizing sucrose or iodixanol gradients to separate EVs from other subcellular components (148). Another technique, density gradient centrifugation, employs a continuous gradient to separate EVs based on their buoyant density. This method is often used for isolating specific EV subpopulations, such as exosomes (149). Size-exclusion chromatography (SEC) is another method used for EV isolation, where EVs are separated based on their size characteristics. This technology is useful for removing unwanted contaminants, such as proteins and lipoproteins, which may be present during the isolation process (150). Once the isolation process is complete, hUC-MSC-EVs can be characterized by analyzing their physical and biochemical properties. Physical characterization includes assessing various attributes of EVs, such as their dimensions, structure, and abundance. Techniques such as dynamic light scattering (DLS), transmission electron microscopy (TEM), and nanoparticle tracking analysis (NTA) are commonly employed for this purpose (151). Biochemical characterization involves the detection and analysis of specific EV markers, including CD9, CD63, and CD81, using techniques like western blotting, flow cytometry, or immunogold labeling (152). Furthermore, the cargo of hUC-MSC-EVs can be characterized through proteomics, RNA sequencing, and lipidomics, providing insights into their functional properties and potential therapeutic targets (153).
It is important to acknowledge the inherent challenges associated with the extraction and characterization of EVs, which stem from the diverse nature of EV populations and the potential risk of contamination (154). Therefore, it is crucial to employ a range of isolation and characterization methodologies to ensure the integrity and homogeneity of hUC-MSC-EVs for their potential clinical application. By improving separation and characterization techniques and tailoring the cargo of EVs to therapeutic targets, researchers can develop novel IBD medications that are more effective and exhibit fewer side effects than current treatments. For a comprehensive overview of different techniques for exosome isolation and characterization, please refer to Table 2.
MSC-derived EVs have been shown in numerous studies to be an effective treatment for experimentally induced colitis in mice. One study documented and examined the weight loss, stool viscosity, and hematochezia in mice with DSS-induced colitis treated with MSCs, MSC-Exs, and placebo in distinct groups. The findings demonstrated that MSCs and MSC-Exs both had an equal anti-inflammatory impact and could treat colitis in mice (160). Additionally, studies on MSCs’ immediate and long-term protective effects on experimental colitis have shown that MSCs produced from human adipose tissue not only temporarily relieve colitis but also have positive long-term regulatory effects on IBD (161) (Table 3).
It has been documented that the MSC-Exos have the ability to home in on areas of intestinal inflammation, interact with immune cells like macrophages, T lymphocytes, and DCs, and modulate the characteristics and activities of immune cells by releasing bioactive substances, such as cytokines, to regulate irregular immune responses and suppress inflammatory reactions (153), as outlined in Figure 4.
Furthermore, MSC-Exos possess the capability to influence intestinal epithelial cells (IECs), facilitate the restoration of the intestinal epithelial barrier (IEB), mitigate oxidative stress, and alleviate colon fibrosis. Therefore, exhibit potential in the treatment of IBD (171). The therapeutic utilization of MSC-derived EVs in the treatment of IBD are given in Table 4.
It has been determined that macrophages are the primary cells responsible for causing colon inflammation (184, 185), as depicted in Figure 5. Upon activation by pro-inflammatory stimuli, macrophages are mobilized to inflamed areas and undergo differentiation into macrophages with distinct polarities under the influence of chemokines and inflammatory factors. M1 macrophages release proinflammatory cytokines (IL-1β, IL-6, TNF-α, and IL-12) as well as Th1 chemokines (CXCL9, CXCL10, and CXCL11), which are involved in antigen presentation, T cell activation, and the initiation of an adaptive immune response. M2 macrophages release suppressive cytokines like IL-10 and TGF-β, which serve to dampen immune reactions and counteract inflammatory responses (186, 187). Anomalous polarization of macrophages contributes to immune irregularities within the intestinal mucosa and mediates the onset of intestinal inflammation, a key triggering factor in the development of IBD (188). Research indicates that the regulation of macrophage polarization and the balance between M1 and M2 macrophages is crucial in the immunotherapeutic approach to IBD (187–190). Both laboratory studies and animal trials have demonstrated that when lipopolysaccharide (LPS)-activated macrophages are co-cultured with BM-derived MSC-Exos, fluorescently labeled MSC-Exos are observed within the macrophages after 24 h. This interaction leads to the modulation of macrophage polarization towards the M2 phenotype, resulting in a decreased M1/M2 ratio and reduced expression of IL-6, IL-7, TNF-α, and IL-12, along with diminished macrophage infiltration in colon tissue (165, 173). Additionally, further research has indicated that adipose-derived MSC-Exos harbor an anti-inflammatory agent, TNF-α stimulated gene-6 (TSG-6), plays a pivotal role in orchestrating the polarization of M2 macrophages (191). The potential pathways through which MSC-Exos regulate macrophages include:
Figure 5. The MSC-EVs mitigate ulcerative colitis is through modulating the phenotype and function of colonic macrophages. MSC-EVs reduce the cleavage of caspase-3, -8, and -9 and lessen the release of damage-associated molecular patterns (DAMPs) from damaged gut epithelial cells, leading to decreased activation of the NF-κB signaling pathway in colon macrophages. MSC-EVs deliver miR-146a, which inhibits the expression of TNF receptor-associated factor 6 (TRAF6) and IL-1 receptor-associated kinase 1 (IRAK1), reduces phosphorylation of NF-κB p65, and suppresses the generation of inflammatory M1 macrophages. This is evidenced by decreased inducible nitric oxide synthase (iNOS) expression, significantly lower production of nitric oxide (NO), inflammatory cytokines (TNF-α, IL-1β, IL-6), and chemokines (CCL-17, CCL-24), resulting in reduced recruitment of neutrophils, monocytes, and lymphocytes to the inflamed gut. Additionally, MSC-EVs promote the polarization of colon macrophages to an anti-inflammatory M2 phenotype, marked by increased secretion of immunosuppressive cytokines TGF-β and IL-10, thus alleviating colitis.
At the site of intestinal inflammation, MSC-Exos bind to macrophages and release encapsulated miRNA, which in turn selectively modulates the mRNA within macrophages and influences the polarization of M2 macrophages. Research has demonstrated that the ExomiRNAs of MSCs, including miR-146a, can downregulate the expression of TNF receptor-related factor 6 (TRAF-6) and IL-1 receptor-related kinase 1 (IRAK-1), thereby suppressing the release of proinflammatory cytokines and promoting the expression of the anti-inflammatory factor IL-10 (192).
MSC-Exo contains a multitude of proteins, notably metallothionein-2 (MT-2), renowned for its capacity to suppress colitis activity. This protein plays a pivotal role in mitigating the intestinal inflammatory response by upholding the integrity of the intestinal barrier and fostering the polarization of M2b macrophages (179).
MSC-Exos penetrate macrophages, potentially triggering the myeloid differentiation primary response gene 88 (MyD88)-dependent signaling pathway in macrophages upon recognition of TLR3 by the enclosed dsRNA. This activation leads to the induction of M2 polarization in macrophages, the release of anti-inflammatory factors, and the inhibition of the inflammatory response (193).
In the presence of inflammatory stimuli, the interaction between CC chemokine receptor 2 (CCR2) expressed on the surface of monocytes and CC chemokine ligand 2 expressed on the surface of macrophages triggers the migration of monocytes to inflammatory sites, where they transform into macrophages, thereby intensifying the inflammatory response and exacerbating tissue damage. Notably, MSC-Exos exhibit high expression of CCR2, which competitively binds to the chemokine ligand 2 on the surface of macrophages. This competitive binding inhibits the recruitment and activation of monocytes, prevents the polarization of M1 macrophages, and reduces the expression of proinflammatory cytokines, such as IL-1β, IL-6, and TNF-α, thereby effectively restraining inflammatory responses (194).
Firstly, MSC-Exos derived from MSCs, induced by specific inflammatory factors, encapsulate numerous anti-inflammatory cytokines and chemokines. Upon uptake by macrophages, these components are released into the surrounding environment. Secondly, the aforementioned pathways, once activated, govern or initiate the polarization of M2 macrophages, leading to the upregulation of anti-inflammatory cytokines and the downregulation of proinflammatory cytokines. This orchestration serves to sustain the equilibrium of anti-inflammatory factors at inflammatory sites and effectively suppress excessive inflammatory responses (195).
The continual exposure of antigens to CD4+ T cells by immune cells in the intestinal mucosa prompts the differentiation of primitive CD4+ T cells (Th0) into various helper T cell subtypes (predominantly Th1, Th2, and Th17) and regulatory T (Treg) cells, under the influence of antigen presentation and cytokine regulation. The trajectory of their differentiation plays a crucial role in preserving intestinal immune equilibrium and modulating inflammatory responses within the intestine (196). Research indicates that the co-cultivation of T lymphocytes with MSC-Exos results in the downregulation of cyclinD-2 and the upregulation of P27KIP-1, thereby impeding T lymphocytes from entering the S phase and inhibiting their growth and proliferation. This suggests that MSC-Exos have the capacity to modulate the proliferation and differentiation of T lymphocytes. Notably, MSC-Exos primarily regulate the equilibrium between Th1 and Th2, as well as Treg and Th17 cells (196).
Th1 and Th2 cells represent the two primary subtypes of Th0 differentiation. Th1 cells primarily express IFN-γ and IL-12, while Th2 cells predominantly express IL-4 (196, 197). The dysregulation of Th1/Th2 subsets is intricately linked to the chronic inflammation observed in IBD (197, 198). The direction of differentiation is largely influenced by the concentration of IL-12 in the environment and the activation status of antigen-presenting cells. Through the regulation of DCs and macrophages, MSC-Exos induce the polarization of M2 macrophages, impede the antigen presentation of DCs, and diminish the release of proinflammatory cytokines, such as IL-12. This environment is conducive to the transition of T cells into Th2 cells. Studies have demonstrated that MSC-Exos can markedly diminish the expression of IL-12, hinder the differentiation and proliferation of Th1 cells, and facilitate the transition of T cells into Th2 cells following co-cultivation with T cells activated by phytohemagglutinin (199, 200). Furthermore, research has revealed that the TSG-6 protein detected in hUC-MSC-exo regulates the immune response of Th2 and Th17 cells in mesenteric lymph nodes (MLN), downregulates proinflammatory cytokines in colon tissue, and upregulates anti-inflammatory cytokines, thereby safeguarding the integrity of the intestinal barrier (162).
Treg cells play a pivotal role in inhibiting the transformation of effector T cells and adaptive mucosal immunity in the intestine, thereby reducing immune-related tissue damage in IBD. A study has revealed that MSC-Exos induce T lymphocyte apoptosis and modulate the differentiation and proliferation of Treg cells through programmed death ligand and galactosin-1 (201). Treg cells express anti-inflammatory cytokines, such as IL-10 and TGF-β, to achieve immunosuppressive effects (193, 202, 203). In contrast, Th17 cells express proinflammatory cytokines that contribute to the inflammatory activity in IBD. Notably, the ratio of Th17/Treg in the peripheral blood of IBD patients has been found to be significantly increased, as demonstrated (204). Furthermore, Chen et al. (133) discovered a significant increase in the ratio of Th17/Treg cells in the mesenteric lymphoid tissue of colitis rats. Conversely, the ratio of Th17/Treg cells was markedly decreased, leading to a notable amelioration of colitis after the injection of MSC-Exos via the caudal vein (180, 205). Additionally, it was found that adipose-MSC-Exos (AD-MSC-Exos) can restore the proportion of Treg cells in the spleen of the IBD mouse model to the baseline level, akin to that of normal mice, and also improve the inflammation of dextran sulfate sodium (DSS)-induced colitis (166).
In the intestinal mucosa, DC are the main antigen-presenting cells. They produce and secrete proinflammatory cytokines including IL-6 and IL-12, which exacerbate the inflammatory response in IBD (refer to Figure 4) (206). Additionally, their production of reactive oxygen species (ROS) contributes to the destruction of the intestinal mucosal barrier and participates in the tissue damage observed in IBD (1). The potential mechanisms through which MSC-Exos regulate DCs encompass the following: (1) MSC-Exo-treated DCs result in the downregulation of IL-4 and IL-12, coupled with the upregulation of TGF-β, thus inhibiting the maturation and differentiation of DCs (200, 207, 208). This process also induces the differentiation of T cells, affects the balance of Th1/Th2 transformation (209), and inhibits the intestinal inflammatory response (210). Furthermore, (2) MSC-Exos suppress the differentiation and maturation of DCs by modulating the TLR-NF-κB signaling pathway. In summary, MSC-Exos exert a potent immunomodulatory effect (211). In the context of IBD treatment, MSC-Exos carrying immunosuppressive factors influence M2 macrophage polarization, inhibit the proliferation of Th1 and Th17 cells, promote the differentiation of Treg cells, and induce antigen-presenting cells (212).
The intestinal barrier encompasses the IEB, mucosal innate immune system, intestinal mucus, and intestinal microbiota. The IEB, constituted by the tight junctions of IECs, forms a crucial mechanical barrier and represents the most pivotal component of the intestinal barrier. Dysregulation and disturbances in various aspects, including mucosal immunity, surface mucus, intestinal microbiota, and oxidative stress, can compromise the integrity of the IEB, leading to the necrosis and apoptosis of IECs and an escalation in wall permeability. These changes underlie the pathological alterations observed in IBD (213–216). MSC-Exos exhibit the capacity to repair IEC injury, inhibit IEC apoptosis, preserve the equilibrium of oxidative stress, and diminish intestinal wall permeability, thereby facilitating the restoration of the IEB, refer to Figure 6.
Mao et al. (173) observed a significant reduction in immune-mediated damage to IECs in colitis mice at 12 h post-intravenous injection of hUC-MSC-exo, indicating the therapeutic potential of exosomes in immune regulation. Additionally, Wang et al. (47) demonstrated that ADSCs-Exos treated with vascular endothelial growth factor C (VEGF-C) upregulate miR-132 and modulate the Smad-7 gene and TGF-β/Smad signaling pathways, thereby promoting the proliferation, migration, and lymphangiogenesis of lymphatic endothelial cells (LECs). Furthermore, in a DSS-induced colitis model, hUC-MSC-exo were found to regulate the balance between ubiquitination and deubiquitination of functional proteins through miR-326, facilitating the repair of IEC damage and maintenance of IEB integrity by inhibiting neddylation (181). Although the specific regulatory process and molecular mechanism require further elucidation.
It has been identified in numerous apoptotic bodies in the colon mucosa of UC patients (214). Subsequent studies revealed that the lack of NF-κB in IECs is a critical factor leading to their entry into the apoptosis program (215). Yang et al. (182) observed a high expression of caspase 3, caspase 8, and caspase 9 in the cysteinyl aspartate-specific protein (caspase) family in the colon tissue of rats with colitis. Following the injection of MSC-derived EVs into the tail vein, the expressions of these caspases were altered, indicating the potential of MSC-EVs to inhibit IEC apoptosis and repair the IEB. Similarly, Yang et al. (182) obtained similar results, further affirming the ability of MSC-EVs to mitigate the apoptosis of IECs and contribute to IEB repair.
Oxidative stress plays a crucial role in the onset and progression of IBD. Typically, colonic tissue cells maintain a dynamic equilibrium between oxidation and antioxidation. However, chronic colonic inflammation disrupts this balance, leading to excessive production of reactive ROS by IEC and macrophages, which results in colonic tissue damage (217, 218). Elevated levels of ROS have been detected in the colonic mucosa of IBD patients and animal models, with a positive correlation to disease severity and increased peroxidation products, such as peroxidized lipid molecules and proteins (218–221). Overproduction of ROS directly damages nucleic acids, lipids, and proteins in colon epithelial cells, inducing necrosis or apoptosis of IECs and compromising the integrity of the IEB (222). While oxidative stress aids the immune system in pathogen clearance, excessive oxidative stress stimulates macrophages to produce proinflammatory cytokines, exacerbating intestinal inflammation. Research indicates that mesenchymal stem cell-derived exosomes (MSC-Exos) can repair various injuries caused by oxidative stress (223–225).
However, most studies have focused on tissues such as the heart, lungs, liver, and kidneys, with limited research on MSC-Exos in treating colitis (48). Nevertheless, MSC-Exos might repair oxidative stress-damaged IECs and help maintain the intestinal mucosal barrier. Additionally, MSC-Exos could mitigate oxidative stress injuries through immune regulation. Further research is needed to determine whether MSC-Exos can reduce intestinal ROS production and restore the balance between oxidation and antioxidation in the intestine.
It was discovered that the incidence of necrotizing enterocolitis (NEC) and intestinal wall permeability significantly decreased in NEC model rats treated with intraperitoneal injections of BM MSCs or BM MSC-Exos (226). Furthermore, McCulloh et al. (227) verified that MSC-Exos can reduce intestinal wall permeability, though the precise molecular mechanisms underlying this effect remain unclear.
Colonic fibrosis is a chronic complication of IBD, with more than 30% of CDpatients developing fibrosis, which rapidly leads to intestinal stenosis and adversely impacts patient prognosis (228, 229). Pathological stimuli such as chronic intestinal inflammation, oxidative stress, damage to and repair of the IEB, promote epithelial-mesenchymal transition (EMT). This transition involves the loss of epithelial cell polarity and intercellular connections, morphological and functional transformation, and significant extracellular matrix (ECM) accumulation, culminating in intestinal fibrosis (230). During tissue repair or response, fibroblast activation is crucial for fibrosis development (230). The TGF-β signaling pathway has been identified as a key activator of fibroblasts, inducing their differentiation into myofibroblasts, which is central to the pathogenesis of intestinal fibrosis (231). Yang et al. (232) demonstrated that BM-MSC-Exos can significantly reverse EMT in TGF-β1-treated (IEC-6) through miR-200b, thereby mitigating intestinal fibrosis. Various studies have shown that MSCs from different sources regulate EMT by activating TGF-β and Wnt signaling pathways, reducing ECM aggregation, and exerting antifibrotic effects (233). While MSCs have been shown to reduce fibrosis in the heart, lungs, liver, and kidneys, research on their effects on intestinal fibrosis remains limited (234). Choi et al. (234) hUCMSC-Exos suppress TGF-β1-induced fibroblast activation by inhibiting the Rho/MRTF/SRF pathway, thereby reducing intestinal fibrosis. Additionally, Duan and Cao (235) discovered that human placental MSC-Exos can decrease collagen deposition in the intestinal wall by reducing collagen production and promoting its degradation through inhibition of TGF-β1 protein expression.
Instead of being solely associated with IBD, hUC-MSC derived EVs have demonstrated promising potential in the treatment and management of diverse pathological conditions.
The induction of M1 to M2 phenotypic transition in bone marrow-derived macrophages (BM-DM) can be effectively achieved through the utilization of hUC-MSC-derived exosomes, which are characterized by an average particle size of 70 nm. In vivo studies have provided compelling evidence that hUC-MSC-derived exosomes facilitate the process of functional recuperation subsequent to SCI through the downregulation of inflammatory cytokines, including TNF-α, MIP-1, IL-6, and IFN-γ (61). The role of hUC-MSC-exo in the transformation of macrophages from the M1 to the M2 phenotype has been established (236). Intravenous administration of these exosomes shows promise as a therapeutic approach for mitigating inflammation and facilitating the restoration of locomotor function following SCI. These findings highlight the potential of exosomes to significantly contribute to the future of SCI therapy (237).
In the treatment of SCI mice, hUC-MSC transplantation has been shown to greatly enhance the survival and regeneration of myelin and nerve cells in the injured area of the spinal cord (238). This transplantation approach also leads to a significant improvement in the motor function of the animals. These positive therapeutic outcomes may be attributed, at least in part, to the effects of hUC-MSC transplantation, which include reducing the production of IL-7 at the site of injury, enhancing the activation of M2 macrophages, and preventing inflammatory infiltration (239). A study conducted in 2022 suggests that the ability of hUC-MSCs to modulate the inflammatory response following nerve injury plays a critical role in their effectiveness in treating acute SCI. This information may guide future applications of hUC-MSCs and enhance the effectiveness of their clinical translation (240). Moreover, hUC-MSC-EVs, acting through the miR-29b-3p/PTEN/Akt/mTOR axis, have demonstrated the ability to reduce pathological alterations, enhance motor function, and promote nerve function repair in SCI rats (241).
Globally, traumatic brain injury (TBI) is a leading cause of fatalities and long-term impairment (242). TBI treatments have recently gained significant attention. However, the therapeutic application of hUC-MSC transplantation in TBI has been limited by challenges such as immunological rejection, ethical considerations, and the potential for tumorigenicity. Notably, hUC-MSC-exo have demonstrated the ability to enhance neurological performance, reduce cerebral edema, and decrease lesion volume following TBI (243). According to a study, hUC-MSC-exo may provide neuroprotection against TBI by exerting inhibitory effects on cell death processes, including apoptosis, pyroptosis, and ferroptosis, through the PINK1/Parkin-mediated mitophagy pathway (238). Recent studies have shed light on the emerging significance of pyroptosis in the context of brain damage. It has been demonstrated that pyroptosis plays a significant role in the development of neonatal cerebral ischemia-reperfusion (I/R) injury, exerting a substantial influence on the pathological processes involved (244). hUC-MSC-exo possesses the potential to mitigate apoptosis following TBI, reduce neuroinflammation, and promote neurogenesis (245). In order to evaluate the efficacy of exosome therapy in facilitating neurological recovery, a rat model of TBI was established. Subsequent analysis revealed a significant improvement in sensorimotor function and spatial learning in rats upon administration of hUC-MSC-exo (246). Moreover, through the inhibition of the NF-kB signaling pathway, hUC-MSC-exo exhibited a substantial reduction in the synthesis of proinflammatory cytokines. Furthermore, noteworthy observations were made regarding the neuroprotective effects of hUC-MSC-exos, including the prevention of neuronal apoptosis, attenuation of inflammation, and facilitation of neuronal regeneration within the injured cortex of rats subjected to TBI (247).
A myocardial infarction (MI) is a significant inflammatory disorder triggered by an imbalance in substrate and oxygen supply versus demand, leading to ischemia or cellular demise (248, 249). Despite the frequent utilization of early revascularization and the widespread implementation of quality measures, notable variations exist at the local and regional levels regarding the management and outcomes of MI (250, 251). The therapeutic effectiveness of stem cell-based therapy in MI for the purposes of heart repair and regeneration has been substantiated through preclinical investigations as well as clinical trials (252). A potential therapeutic approach for MI involves the utilization of MSC-EVs. To investigate the effects of hUC-MSC-EVs loaded with miR-223 in the context of MI, experiments were conducted employing both an in vitro cellular model of oxidative stress and cardiac fibrosis, as well as in vivo rat models of MI. The transfer of miR-223 via EVs demonstrated improved cardiac function in MI rat models, along with reduced fibrosis and inflammation (253). The pathogenesis of MI is primarily attributed to the presence of inflammation, wherein the detrimental effects of MI are intricately associated with the orchestrated activation of multiple inflammatory cascades and the recruitment of inflammatory cells (254). Before the widespread implementation of cardiomyocyte regeneration in clinical trials, substantial further research and development are required. In the realm of cell therapy, extensive investigations have been conducted to explore the differentiating capabilities of (hUC-MSCs). It is widely recognized that the utilization of 5-Azacytidine (5-Aza) effectively triggers the differentiation of hUC-MSCs into cardiomyocytes, resulting in morphological changes and the expression of cardiac-specific proteins, irrespective of the presence of basic fibroblast growth factor (bFGF) (255). An additional investigation has revealed that 5-Azacytidine (5-Aza) may facilitate the in vitro differentiation of hUC-MSCs into cardiomyocytes through the sustained phosphorylation of extracellular signal-regulated kinase (ERK) (256). Recent scientific investigations have demonstrated that the administration of injected hUC-MSCs exerts beneficial effects on cardiac function in rats with dilated cardiomyopathy (DCM) through the attenuation of myocardial fibrosis and dysfunction. These effects are achieved by the downregulation of Transforming Growth Factor-β1 (TGF-β1) and TNF-α production (257). Furthermore, emerging scientific evidence suggests that exosomes possess potential protective properties in the context of acute myocardial infarction. These exosomes have been shown to potentially facilitate cell repair mechanisms by modulating the expression of Smad7 in cardiomyocytes (257).
Depending on the concentration of serum creatinine (Scr) or glomerular filtration rate (GFR), nephrologists have classified kidney failure into two distinct syndromes: acute renal failure and chronic renal failure (258). Currently, clinical treatments for kidney injury primarily involve medication, surgery, and renal transplantation (259). As stem cell research has advanced, the preventive effects of hUC-MSCs and hUC-MSC-exo on renal tissue injury have been demonstrated (260). Acute kidney injury (AKI) refers to the clinical condition that arises from a rapid loss of renal function caused by various factors (260). Pre-renal acute kidney injury commonly arises from diminished blood volume caused by fluid loss and bleeding from various etiologies, as well as a decrease in effective arterial blood volume and alterations in intrarenal hemodynamics. Recent research has focused on the role of sepsis in causing AKI. To investigate this, a sepsis model was induced using cecal ligation and puncture (CLP), followed by treatment with hUC-MSCs (261). The data presented in the study provided evidence that the administration of hUC-MSCs resulted in substantial improvement in renal function, reduction in tissue damage, and significant enhancement of overall health in mice with sepsis (262, 263). According to the data reported, pre-treatment of hUC-MSCs with IL-1 exhibited a statistically significant augmentation in their capacity to modulate the immune system (264). In response to IL-1 stimulation, exosomes selectively encapsulated a widely recognized anti-inflammatory microRNA, MiR-146a. Subsequent delivery of exosomal MiR-146a to macrophages induced M2 polarization, leading to a reduction in kidney damage in septic mice (265). According to a study, the administration of hUC-MSCs have been demonstrated to effectively ameliorate renal ischemia-reperfusion injury (IRI) in mice. This beneficial effect is achieved through a dual mechanism involving a reduction in the infiltration of macrophages into the injured kidneys and a concomitant increase in the population of M2-like macrophages during the healing process (265). Through the modulation of inflammatory cytokine production and promotion of renal tubular cell proliferation, hUC-MSCs have been observed to expedite the recovery of renal function. Additionally, it was discovered that hUC-MSC transplantation resulted in a reduction in the levels of malondialdehyde (MDA) in renal tissues, indicating a potential protective effect of hUC-MSCs against oxidative damage and mitochondrial dysfunction in renal cells (266). Subsequent investigations revealed that the underlying mechanism responsible for cisplatin-induced nephrotoxicity was primarily attributed to the induction of oxidative stress. However, this detrimental effect can be mitigated by the administration of hUC-MSC-exo, which exert their protective effects by suppressing the activation of the p38 mitogen-activated protein kinase (MAPK) pathway (267). Renal fibrosis is a common pathway in the progression of chronic kidney disease (CKD) that often culminates in end-stage renal failure. Huang et al. provided insights into the potential therapeutic role of MSCs in mitigating obstructive chronic progressive renal interstitial fibrosis (RIF) in the context of kidney failure. Their study demonstrated that infused MSCs could effectively reach the damaged kidney tissues, offering a promising approach for addressing the underlying mechanisms involved in renal fibrosis (268).
Inflammatory, proliferative, and remodeling stages are just a few of the many phases that make up the complex process of cutaneous wound healing (268). Recent years have witnessed extensive exploration into benefits of direct stem cell infusion for tissue regeneration (269, 270). A degradable, dual-sensitive hydrogel incorporating exosomes extracted from hUC-MSC was synthesized. Afterwards, the in vivo wound healing potential, exosome identification and material properties of the hydrogel were assessed. The exosome-loaded hydrogel demonstrated substantial improvements in wound closure rates, re-epithelialization rates and collagen deposition at the wound sites, as evidenced by the in vivo results (269). The administration of exosomes-loaded hydrogel to the wounded sites resulted in a higher density of skin appendages, indicating a potential for achieving full skin regeneration (271).
Clinicians persistently encounter challenges in managing diabetic wounds due to the prevalence of multiple bacterial infections and oxidative damage (272). Exosomes have been widely employed as a promising nanodrug delivery strategy for the treatment of diabetic (273). To ascertain their participation in the modulation of diabetic wound healing, a co-culture of human umbilical vein endothelial cells (HU-VECs) and hUC-MSCs was established, and hUC-MSC-exo were subsequently utilized in both in vitro and in vivo experiments (274). The findings demonstrated that hUC-MSCs possess the ability to mitigate oxidative stress-induced damage to endothelial cells via exosomal mechanisms, thereby accelerating the healing process of diabetic cutaneous wounds in an in vitro setting (273). In vivo setting, it was observed that wounds treated with hUC-MSC-exo exhibited significantly accelerated re-epithelialization and elevated expression levels of CK19, PCNA, and collagen I (in contrast to collagen III) (274).
Acute lung injury (ALI) leads to the early onset of lung inflammation, capillary rupture, destruction of endothelial and epithelial cells, and breakdown of tight epithelial junctions. These factors increase alveolar epithelial permeability, causing significant pulmonary edema and, in severe cases, even death (275). In animal models, transplantation of MSCs effectively enhances the recovery from ALI (276). In the LPS-induced ALI rat model, hUC-MSCs can enhance the activity of antioxidant enzymes in lung tissue (277). Based on this, photobiomodulation (PBM) was utilized to modulate the release of pro-inflammatory chemicals, reduce their levels to a certain extent, and enhance the balance of oxidative stress at the site of injury (278). Bronchopulmonary dysplasia (BPD) is a dangerous chronic lung condition characterized by a high rate of morbidity and mortality in premature neonates (279). Transplantation of MSCs is a promising strategy for treating BPD. In a rat model of BPD, the transplantation of hUC-MSC-EVs resulted in the improvement of pulmonary hypertension, restoration of lung function, and alveolar structure (280). In a BPD model treated with hUC-MSC-EVs, the proportion of Ki-67-positive lung cells increased, indicating an upregulation of cell proliferation, whereas the proportion of TUNEL-positive lung cells decreased, suggesting a downregulation of cell apoptosis (263). Furthermore, in a hyperoxia-induced BPD model treated with hUC-MSC-EVs, there was an increase in SP-C staining, a marker of type II alveolar epithelial cells (TIIAECs), as well as CD31 staining, a marker of pulmonary vascular endothelial cells (PVECs) (281). Another study has demonstrated that intervention with PBM in hUC-MSCs naturally reduces the thickness of the alveolar septum, suppresses excessive secretion of inflammatory factors, and alleviates in vivo conditions of bleeding, edema, and fibrosis (278). PBM is a physical intervention that enhances the therapeutic effect of hUC-MSCs and shows promise as a therapy for the treatment of ALI. Furthermore, the use of hUC-MSCs may potentially reduce bleomycin-induced mouse mortality and attenuate lung collagen buildup (282). The transplantation of hUC-MSCs induced the proliferation of alveolar type 2 (AT2) cells, while concurrently suppressing the proliferation of lung fibroblasts (283). After the transplantation of hUC-MSCs, there is an induction of AT2 cell proliferation, concomitant with the suppression of lung fibroblast proliferation. Additionally, this transplantation leads to increased release of CXCL9 and CXCL10 by interferon-stimulated cells (IFNSMs), thereby attracting additional Treg cells to the injured lung (284). In a study, hUC-MSCs were administered, and lung morphometry was successfully enhanced (285). Further research has revealed the crucial role of exosome-resident miR-377-3p in controlling autophagy, thereby preventing LPS-induced ALI (286).
The liver, which is widely considered to be the most critical organ in the body, plays a pivotal role in the detoxification, secretion, and metabolism of medications and toxins (287). Acute liver failure (ALF), a pathological condition resulting from rapid deterioration in hepatic function, and is clinically characterized by jaundice, coagulopathy, and encephalopathy (288). In recent years, increasing scientific research has indicated that the transplantation of MSCs holds promise as a potential therapeutic strategy for the treatment of ALF (289, 290). Findings of ta study revealed that the administration of hUC-MSC-exo via a single tail vein injection resulted in a significant improvement in the survival rate, prevention of hepatocyte death, and enhancement of liver function in an experimental mouse model of ALF induced by APAP (291). Furthermore, the administration of hUC-MSCs effectively mitigated APAP-induced hepatocyte apoptosis by modulating oxidative stress markers (292). This was evident through the downregulation of glutathione (GSH) and superoxide dismutase (SOD) levels, as well as the attenuation of MDA production. Additionally, the excessive expression of cytochrome P450 E1 (CYP2E1) and 4-hydroxynonenal (4-HNE), which are known contributors to oxidative stress, was significantly suppressed. These findings highlight the ability of hUC-MSC-exo to regulate oxidative stress pathways, thereby conferring protection against APAP-induced hepatocyte death in the context of acute liver failure (293). In a study, mouse models of acute and chronic liver damage, as well as liver tumors, were generated by intraperitoneal injection of carbon tetrachloride (CCl4) followed by the intravenous infusion of hUC-MSC-exo. The results of the study demonstrated the hepatoprotective effect of hUC-MSC-exo, as evidenced by a significant reduction in liver damage (294). hUC-MSC-exo treatment has been shown to mitigate the expression of the NLRP3 inflammasome and associated inflammatory factors (295), offering a potential therapeutic approach for the management of acute liver failure. Furthermore, in lipopolysaccharide (LPS)-stimulated RAW 264.7 macrophages, hUC-MSC-exo demonstrated inhibitory effects on the expression of NLRP3, caspase-1, IL-1β, and IL-6. These findings highlight the immunomodulatory properties of hUC-MSC-exo, which could contribute to the attenuation of inflammatory responses in various pathological conditions (296). The proteolytic activation of pro-IL-1β is facilitated by activated caspase-1. Caspase-1, IL-1β, and NLRP3 play pivotal roles in the development of acute pancreatic and hepatic injury, inflammation, and subsequent organ damage. These factors are crucial in the pathophysiological processes associated with inflammatory responses and tissue injury in the pancreas and liver (297). The primary outcome of a recent study revealed that T-Exo treatment effectively reduces circulating levels of alanine aminotransferase (ALT), aspartate aminotransferase (AST), and proinflammatory cytokines. Additionally, T-Exo therapy attenuates the activation of proteins involved in the NLRP3 inflammation-associated pathway, thereby mitigating the pathological liver damage associated with acute ALF (298). Notably, TNF stimulation of MSCs leads to the selective packaging of anti-inflammatory microRNA-299-3p into exosomes, which can be utilized for exosomal therapy purposes (299).
Diabetes mellitus (DM), a collective term referring to a group of metabolic disorders, is distinguished by the presence of elevated blood glucose levels, commonly known as hyperglycemia (300). Over the preceding half-century, the dynamics of lifestyles and the process of globalization have imparted profound ramifications on political, environmental, societal, and human behavioral domains. DM represents a complex disorder influenced by a combination of genetic predisposition and environmental factors, leading to compromised insulin secretion or insulin resistance (IR). These physiological aberrations contribute to the characteristic pathophysiology of DM and its associated metabolic dysregulation (300, 301). In recent years, obesity and physical inactivity have become more common, and rapid population expansion, aging, and urbanization have all contributed to the worldwide health issue that is DM (302). To maintain physiological equilibrium within the body’s ecosystem, it is imperative to regulate the homeostasis of blood glucose, which is contingent upon the management of vital life processes (303). In the year 2018, a team of researchers conducted an investigation into the correlation between hUC-MSC-exo and hyperglycemia induced by type 2 diabetes mellitus (T2DM) (304). The findings unequivocally indicate that hUC-MSC-exo consistently mitigated blood glucose levels in T2DM-afflicted rats subjected to a high-fat diet (HFD) and streptozotocin (STZ). Notably, hUC-MSC-exo enhanced the uptake of the fluorescent glucose analogue 2-NBDG in myotubes and hepatocytes, thereby substantiating their role in facilitating glucose absorption. Additionally, hUC-MSC-exo promoted glucose uptake in the muscles, upregulated the expression of the glucose-responsive transporter (GLUT4) in T2DM rats, and reinstated hepatic glucose homeostasis through the activation of insulin signaling. The activation of the insulin/AKT signaling pathway further corroborated the potential of hUC-MSC-exo to enhance insulin sensitivity in both in vivo and in vitro settings. Moreover, an evidence demonstrated that hUC-MSC-exo facilitated insulin secretion and islet regeneration by preventing STZ-induced caspase-3 alterations that lead to cellular apoptosis (305).
Clinical trials are currently underway to evaluate the safety and efficacy of MSC-EVs in the management of IBD and its related conditions (306). Significantly, a series of phase I and II trials have been conducted, resulting in promising results. Significantly, the administration of hUC-MSC-exosomes demonstrated protective effects against weight loss, without eliciting adverse effects on liver or renal functions (307, 308). Furthermore, the versatility of hUC-MSC-exosomes was highlighted through diverse evaluations, encompassing assessments for hemolysis, activation of vascular and muscular systems, systemic anaphylaxis, pyrogenicity, and hematological markers (309). In another phase I clinical trial, four individuals exhibited a response to therapy 6 months after the initiation of treatment. Three out of the patients who underwent exosome injections achieved full recovery, while one patient reported no improvement and had active discharge from the fistula site. Furthermore, all five patients (100%) reported no occurrence of local or systemic side effects (310). In a similar vein, a team of scientists has developed a comprehensive systemic quality control system and robust testing methods to ensure the safety and efficacy of hUC-MSCs, based on a minimal set of requirements for MSC-based products. The validity of this system for quality control and assessment of hUC-MSCs as a cell-based product has been verified, as none of the qualified hUC-MSCs demonstrated any serious adverse reactions during the 1-year follow-up period, even after testing for multiple indications (311). However, to ascertain the safety and effectiveness of hUC-MSC-EVs in treating IBD and related illnesses, larger, multicenter clinical trials are necessary. Furthermore, long-term follow-up research is required to assess the durability of treatment response and the presence of any potential side effects.
Given the variation of MSC-based therapies across countries and regions, adherence to regulations is crucial. The development of hUC-MSC-EVs as a therapeutic option for IBD and related disorders necessitates meticulous consideration of regulatory and manufacturing aspects. Considering the scalability and cost-effectiveness of production procedures is imperative to enable the widespread clinical utilization of hUC-MSCs. To successfully integrate EV-based therapies into clinical practice, it is crucial to address multiple challenges associated with scalability, standardization, and characterization of EV products. Establishing standardized manufacturing processes is essential to guarantee the reproducibility, safety, and quality of the ultimate therapeutic product (312, 313). Current efforts focus on developing strategies, such as the utilization of bioreactors and microfluidic systems, for the large-scale production of EVs (314, 315). To effectively integrate the use of hUC-MSC-EVs into clinical applications, adherence to regulatory and manufacturing factors is imperative.
The European Medicines Agency (EMA) has issued guidelines regarding the utilization of MSCs in clinical studies (316). To comply with regulatory standards, it is necessary to ensure adherence to Good Manufacturing Practices (GMP) during the production of MSC-based products. In accordance with regulatory requirements, it is mandatory to submit a Clinical Trial Application (CTA) prior to the initiation of any clinical trials.
Similarly, In the United States, the Food and Drug Administration (FDA) has established recommendations for the utilization of MSCs in clinical trials. Based on the stipulations outlined in these rules, MSCs are categorized as biological entities and, as a result, are subject to regulatory oversight by the Food and Drug Administration (FDA). In accordance with the established protocols, it is necessary to complete and submit an Investigational New Drug (IND) application prior to initiating clinical trials (317). Additionally, for the production of MSC-based products, strict adherence to Good Manufacturing Practices (GMP) is mandatory.
Various characteristics of hUC-MSCs, including their anti-inflammatory, immunomodulatory, and regenerative properties, have been extensively investigated both in-vivo and in-vitro. These findings suggest that hUC-MSCs hold promise for mitigating inflammation and promoting the healing of damaged tissues in individuals affected by IBD and related conditions. Consequently, hUC-MSCs may serve as a potential therapeutic intervention in the treatment of IBD and its associated ailments. The development of standardized protocols for the isolation and purification of EVs will be essential to ensure their quality, safety, and efficacy in clinical applications. Further research is needed to optimize the isolation, characterization, and administration of hUC-MSC-EVs. Furthermore, further studies are required to elucidate the molecular mechanisms and signaling pathways that underlie the therapeutic effects of hUC-MSC-EVs. This will enhance our comprehension of their mode of action and guide the development of more precise therapies. To fully realize the potential of this innovative therapy, it will be crucial to address the regulatory and manufacturing challenges as clinical trials progress. Ensuring the safety and effectiveness of hUC-MSC-EVs in human patients will be crucial, and carefully planned clinical trials will be essential in determining the therapeutic usefulness of these vesicles. Furthermore, the development of scalable manufacturing processes that can produce large quantities of high-quality hUC-MSC-EVs will be essential for meeting the demands of clinical use. There are also several challenges associated with the administration of hUC-MSC-EVs, such as determining the optimal dosage, route of administration, and frequency of treatment. Future studies should explore these parameters in order to maximize the therapeutic potential of hUC-MSC-EVs and minimize potential side effects. In addition, the development of biomarkers for patient stratification and monitoring treatment response could help to personalize and optimize hUC-MSC-EV-based therapies for individual patients.
In conclusion, hUC-MSC-EVs represent a promising and novel therapeutic approach for IBD and other related disorders. The growing body of preclinical evidence supporting their therapeutic potential, coupled with advancements in our understanding of their biogenesis, molecular mechanisms, and clinical translation, offers hope for more effective and targeted treatments for patients suffering from these debilitating conditions. By addressing the current challenges and building upon the existing knowledge, researchers and clinicians can work together to harness the full potential of hUC-MSC-EVs and bring this innovative therapy to the forefront of IBD treatment.
MD: Conceptualization, Writing – original draft. AW: Conceptualization, Writing – original draft. YC: Writing – review & editing, Funding acquisition. JZ: Writing – original draft, Conceptualization. YY: Conceptualization, Writing – review & editing. ZX: Visualization, Writing – review & editing.
The author(s) declare that financial support was received for the research, authorship, and/or publication of this article. This work was supported by the Grant of the Scientific Project of Jiangsu Health Commission (Z2020038); the Grant of the Open Project of Jiangsu Key Laboratory of New Drug Research and Clinical Pharmacy (XZSYSKF2020032); Jiangsu 333 High-Level Talents Training Project, Changzhou High-Level Medical Talents Training Project (2022CZBJ111) and Zhenjiang Key Research and Development Plan (Social Development) (Grant No. SH2022062).
We extend our sincere appreciation to Professor Mao Fei for his invaluable and comprehensive guidance throughout the entire manuscript writing process. Additionally, we would like to express our sincere appreciation and recognition to Dr. Arif Rashid, School of Food and Biological Engineering, Jiangsu University, for his diligent and meticulous review of this article.
The authors declare that the research was conducted in the absence of any commercial or financial relationships that could be construed as a potential conflict of interest.
All claims expressed in this article are solely those of the authors and do not necessarily represent those of their affiliated organizations, or those of the publisher, the editors and the reviewers. Any product that may be evaluated in this article, or claim that may be made by its manufacturer, is not guaranteed or endorsed by the publisher.
1. Prakash, S, Tanaka, T, and Ashat, D. A nationwide study of patients hospitalized with indeterminate colitis: a comparison with Crohn’s disease and ulcerative colitis. Int J Color Dis. (2023) 38:223. doi: 10.1007/s00384-023-04515-5
2. Hemmer, A, Forest, K, Rath, J, and Bowman, J. Inflammatory bowel disease: a concise review. S D Med. (2023) 76:416–23.
3. Roda, G, Chien Ng, S, Kotze, PG, Argollo, M, Panaccione, R, Spinelli, A, et al. Crohn’s disease. Nat Rev Dis Primers. (2020) 6:22. doi: 10.1038/s41572-020-0156-2
4. Li, Y, Chen, J, Bolinger, AA, Chen, H, Liu, Z, Cong, Y, et al. Target-based small molecule drug discovery towards novel therapeutics for inflammatory bowel diseases. Inflamm Bowel Dis. (2021) 27:S38–62. doi: 10.1093/ibd/izab190
5. Gajendran, M, Loganathan, P, Catinella, AP, and Hashash, JG. A comprehensive review and update on Crohn’s disease. Dis Mon. (2018) 64:20–57. doi: 10.1016/j.disamonth.2017.07.001
6. Loddo, I, and Romano, C. Inflammatory bowel disease: genetics, epigenetics, and pathogenesis. Front Immunol. (2015) 6:551. doi: 10.3389/fimmu.2015.00551
7. Uranga, JA, López-Miranda, V, Lombó, F, and Abalo, R. Food, nutrients and nutraceuticals affecting the course of inflammatory bowel disease. Pharmacol Rep. (2016) 68:816–26. doi: 10.1016/j.pharep.2016.05.002
8. Danese, S, Allez, M, van Bodegraven, AA, Dotan, I, Gisbert, JP, Hart, A, et al. Unmet medical needs in ulcerative colitis: an expert group consensus. Dig Dis. (2019) 37:266–83. doi: 10.1159/000496739
9. Armuzzi, A, and Liguori, G. Quality of life in patients with moderate to severe ulcerative colitis and the impact of treatment: a narrative review. Dig Liver Dis. (2021) 53:803–8. doi: 10.1016/j.dld.2021.03.002
10. Ding, D-C, Shyu, W-C, and Lin, S-Z. Mesenchymal stem cells. Cell Transplant. (2011) 20:5–14. doi: 10.3727/096368910X
11. Najera, J, and Hao, J. Recent advance in mesenchymal stem cells therapy for atopic dermatitis. J Cell Biochem. (2023) 124:181–7. doi: 10.1002/jcb.30365
12. Zhu, X, Xu, X, Shen, M, Wang, Y, Zheng, T, Li, H, et al. Transcriptomic heterogeneity of human mesenchymal stem cells derived from bone marrow, dental pulp, adipose tissue, and umbilical cord. Cell Reprogram. (2023) 25:162–70. doi: 10.1089/cell.2023.0019
13. Marędziak, M, Marycz, K, Tomaszewski, KA, Kornicka, K, and Henry, BM. The influence of aging on the regenerative potential of human adipose derived mesenchymal stem cells. Stem Cells Int. (2016) 2016:2152435. doi: 10.1155/2016/2152435
14. Kern, S, Eichler, H, Stoeve, J, Klüter, H, and Bieback, K. Comparative analysis of mesenchymal stem cells from bone marrow, umbilical cord blood, or adipose tissue. Stem Cells. (2006) 24:1294–301. doi: 10.1634/stemcells.2005-0342
15. Thaweesapphithak, S, Tantrawatpan, C, Kheolamai, P, Tantikanlayaporn, D, Roytrakul, S, and Manochantr, S. Human serum enhances the proliferative capacity and immunomodulatory property of MSCs derived from human placenta and umbilical cord. Stem Cell Res Ther. (2019) 10:79. doi: 10.1186/s13287-019-1175-3
16. Shang, Y, Guan, H, and Zhou, F. Biological characteristics of umbilical cord mesenchymal stem cells and its therapeutic potential for hematological disorders. Front Cell Dev Biol. (2021) 9:570179. doi: 10.3389/fcell.2021.570179
17. Jovic, D, Yu, Y, Wang, D, Wang, K, Li, H, Xu, F, et al. A brief overview of global trends in MSC-based cell therapy. Stem Cell Rev Rep. (2022) 18:1525–45. doi: 10.1007/s12015-022-10369-1
18. Zhao, L, Chen, S, Yang, P, Cao, H, and Li, L. The role of mesenchymal stem cells in hematopoietic stem cell transplantation: prevention and treatment of graft-versus-host disease. Stem Cell Res Ther. (2019) 10:723–9. doi: 10.3727/096368912X655217
19. Bajetto, A, Pattarozzi, A, Corsaro, A, Barbieri, F, Daga, A, Bosio, A, et al. Different effects of human umbilical cord mesenchymal stem cells on glioblastoma stem cells by direct cell interaction or via released soluble factors. Front Cell Neurosci. (2017) 11:312. doi: 10.3389/fncel.2017.00312
20. Watson, N, Divers, R, Kedar, R, Mehindru, A, Mehindru, A, Borlongan, MC, et al. Discarded Wharton jelly of the human umbilical cord: a viable source for mesenchymal stromal cells. Cytotherapy. (2015) 17:18–24. doi: 10.1016/j.jcyt.2014.08.009
21. Katsuda, T, Kosaka, N, Takeshita, F, and Ochiya, T. The therapeutic potential of mesenchymal stem cell-derived extracellular vesicles. Proteomics. (2013) 13:1637–53. doi: 10.1002/pmic.201200373
22. Balaji, S, Keswani, SG, and Crombleholme, TM. The role of mesenchymal stem cells in the regenerative wound healing phenotype. Adv Wound Care. (2012) 1:159–65.
23. van Niel, G, Carter, DRF, Clayton, A, Lambert, DW, Raposo, G, and Vader, P. Challenges and directions in studying cell–cell communication by extracellular vesicles. Nat Rev Mol Cell Biol. (2022) 23:369–82. doi: 10.1038/s41580-022-00460-3
24. Mentkowski, KI, Snitzer, JD, Rusnak, S, and Lang, JK. Therapeutic potential of engineered extracellular vesicles. AAPS J. (2018) 20:50. doi: 10.1208/s12248-018-0211-z
25. Lazar, S, Mor, S, Chen, J, Hao, D, and Wang, A. Bioengineered extracellular vesicle-loaded bioscaffolds for therapeutic applications in regenerative medicine. Extracell Vesicles Circ Nucl Acids. (2021) 2:175. doi: 10.20517/evcna.2021.10
26. Alzhrani, GN, Alanazi, ST, Alsharif, SY, Albalawi, AM, Alsharif, AA, Abdel-Maksoud, MS, et al. Exosomes: isolation, characterization, and biomedical applications. Cell Biol Int. (2021) 45:1807–31. doi: 10.1002/cbin.11620
27. Chaput, N, Taïeb, J, Schartz, NEC, André, F, Angevin, E, and Zitvogel, L. Exosome-based immunotherapy. Cancer Immunol Immunother. (2004) 53:234–9. doi: 10.1007/s00262-003-0472-x
28. Raposo, G, Nijman, HW, Stoorvogel, W, Liejendekker, R, Harding, CV, Melief, CJ, et al. B lymphocytes secrete antigen-presenting vesicles. J Exp Med. (1996) 183:1161–72. doi: 10.1084/jem.183.3.1161
29. Zhang, B, Shen, L, Shi, H, Pan, Z, Wu, L, Yan, Y, et al. Exosomes from human umbilical cord mesenchymal stem cells: identification, purification, and biological characteristics. Stem Cells Int. (2016) 2016:1929536. doi: 10.1155/2016/1929536
30. Andre, F, Schartz, NEC, Movassagh, M, Flament, C, Pautier, P, Morice, P, et al. Malignant effusions and immunogenic tumour-derived exosomes. Lancet. (2002) 360:295–305. doi: 10.1016/S0140-6736(02)09552-1
31. Admyre, C, Grunewald, J, Thyberg, J, Gripenbäck, S, Tornling, G, Eklund, A, et al. Exosomes with major histocompatibility complex class II and co-stimulatory molecules are present in human BAL fluid. Eur Respir J. (2003) 22:578–83. doi: 10.1183/09031936.03.00041703
32. Caby, M-P, Lankar, D, Vincendeau-Scherrer, C, Raposo, G, and Bonnerot, C. Exosomal-like vesicles are present in human blood plasma. Int Immunol. (2005) 17:879–87. doi: 10.1093/intimm/dxh267
33. Palanisamy, V, Sharma, S, Deshpande, A, Zhou, H, Gimzewski, J, and Wong, DT. Nanostructural and transcriptomic analyses of human saliva derived exosomes. PLoS One. (2010) 5:e8577. doi: 10.1371/journal.pone.0008577
34. Admyre, C, Johansson, SM, Qazi, KR, Filén, J-J, Lahesmaa, R, Norman, M, et al. Exosomes with immune modulatory features are present in human breast milk. J Immunol. (2007) 179:1969–78. doi: 10.4049/jimmunol.179.3.1969
35. Lee, TH, D’Asti, E, Magnus, N, Al-Nedawi, K, Meehan, B, and Rak, J. Microvesicles as mediators of intercellular communication in cancer—the emerging science of cellular ‘debris’. Semin Immunopathol. (2011) 33:455–67. doi: 10.1007/s00281-011-0250-3
36. Tai, Y, Chen, K, Hsieh, J, and Shen, T. Exosomes in cancer development and clinical applications. Cancer Sci. (2018) 109:2364–74. doi: 10.1111/cas.13697
37. Ohyashiki, JH, Umezu, T, and Ohyashiki, K. Extracellular vesicle-mediated cell–cell communication in haematological neoplasms. Philos Trans R Soc B. (2018) 373:20160484. doi: 10.1098/rstb.2016.0484
38. Safdar, A, and Tarnopolsky, MA. Exosomes as mediators of the systemic adaptations to endurance exercise. Cold Spring Harb Perspect Med. (2018) 8:a029827. doi: 10.1101/cshperspect.a029827
39. Tenchov, R, Sasso, JM, Wang, X, Liaw, W-S, Chen, C-A, and Zhou, QA. Exosomes—nature’s lipid nanoparticles, a rising star in drug delivery and diagnostics. ACS Nano. (2022) 16:17802–46. doi: 10.1021/acsnano.2c08774
40. Kooijmans, SAA, Schiffelers, RM, Zarovni, N, and Vago, R. Modulation of tissue tropism and biological activity of exosomes and other extracellular vesicles: new nanotools for cancer treatment. Pharmacol Res. (2016) 111:487–500. doi: 10.1016/j.phrs.2016.07.006
41. Liu, H, Deng, S, Han, L, Ren, Y, Gu, J, He, L, et al. Mesenchymal stem cells, exosomes and exosome-mimics as smart drug carriers for targeted cancer therapy. Colloids Surf B. (2022) 209:112163. doi: 10.1016/j.colsurfb.2021.112163
42. Wang, X, Zhou, G, Zhou, W, Wang, X, Wang, X, and Miao, C. Exosomes as a new delivery vehicle in inflammatory bowel disease. Pharmaceutics. (2021) 13:1644. doi: 10.3390/pharmaceutics13101644
43. Carrière, J, Barnich, N, and Nguyen, HTT. Exosomes: from functions in host-pathogen interactions and immunity to diagnostic and therapeutic opportunities. Rev Physiol Biochem Pharmacol. (2016) 172:39–75. doi: 10.1007/112_2016_7
44. Toh, WS, Lai, RC, Hui, JHP, and Lim, SK. MSC exosome as a cell-free MSC therapy for cartilage regeneration: implications for osteoarthritis treatment. Semin Cell Dev Biol. (2017) 67:56–64. doi: 10.1016/j.semcdb.2016.11.008
45. Guo, S, Perets, N, Betzer, O, Ben-Shaul, S, Sheinin, A, Michaelevski, I, et al. Intranasal delivery of mesenchymal stem cell derived exosomes loaded with phosphatase and tensin homolog si RNA repairs complete spinal cord injury. ACS Nano. (2019) 13:10015–28. doi: 10.1021/acsnano.9b01892
46. Elahi, FM, Farwell, DG, Nolta, JA, and Anderson, JD. Preclinical translation of exosomes derived from mesenchymal stem/stromal cells. Stem Cells. (2020) 38:15–21. doi: 10.1002/stem.3061
47. Wang, X, Wang, H, Cao, J, and Ye, C. Exosomes from adipose-derived stem cells promotes VEGF-C-dependent lymphangiogenesis by regulating miRNA-132/TGF-β pathway. Cell Physiol Biochem. (2018) 49:160–71. doi: 10.1159/000492851
48. Liang, X, Zhang, L, Wang, S, Han, Q, and Zhao, RC. Exosomes secreted by mesenchymal stem cells promote endothelial cell angiogenesis by transferring miR-125a. J Cell Sci. (2016) 129:2182–9. doi: 10.1242/jcs.170373
49. Maertens, L, Erpicum, C, Detry, B, Blacher, S, Lenoir, B, Carnet, O, et al. Bone marrow-derived mesenchymal stem cells drive lymphangiogenesis. PLoS One. (2014) 9:e106976. doi: 10.1371/journal.pone.0106976
50. Ferreira, JR, Teixeira, GQ, Santos, SG, Barbosa, MA, Almeida-Porada, G, and Gonçalves, RM. Mesenchymal stromal cell secretome: influencing therapeutic potential by cellular pre-conditioning. Front Immunol. (2018) 9:2837. doi: 10.3389/fimmu.2018.02837
51. Kronstadt, SM, Pottash, AE, Levy, D, Wang, S, Chao, W, and Jay, SM. Therapeutic potential of extracellular vesicles for sepsis treatment. Adv Ther. (2021) 4:2000259. doi: 10.1002/adtp.202000259
52. Keshtkar, S, Azarpira, N, and Ghahremani, MH. Mesenchymal stem cell-derived extracellular vesicles: novel frontiers in regenerative medicine. Stem Cell Res Ther. (2018) 9:63. doi: 10.1186/s13287-018-0791-7
53. Hassanzadeh, A, Rahman, HS, Markov, A, Endjun, JJ, Zekiy, AO, Chartrand, MS, et al. Mesenchymal stem/stromal cell-derived exosomes in regenerative medicine and cancer; overview of development, challenges, and opportunities. Stem Cell Res Ther. (2021) 12:297. doi: 10.1186/s13287-021-02378-7
54. Guo, Y, Hu, D, Lian, L, Zhao, L, Li, M, Bao, H, et al. Stem cell-derived extracellular vesicles: a promising nano delivery platform to the brain? Stem Cell Rev Rep. (2023) 19:285–308. doi: 10.1007/s12015-022-10455-4
55. Gimona, M, Pachler, K, Laner-Plamberger, S, Schallmoser, K, and Rohde, E. Manufacturing of human extracellular vesicle-based therapeutics for clinical use. Int J Mol Sci. (2017) 18:1190. doi: 10.3390/ijms18061190
56. Cargnoni, A, Papait, A, Masserdotti, A, Pasotti, A, Stefani, FR, Silini, AR, et al. Extracellular vesicles from perinatal cells for anti-inflammatory therapy. Front Bioeng Biotechnol. (2021) 9:637737. doi: 10.3389/fbioe.2021.637737
57. Sun, W, Yan, S, Yang, C, Yang, J, Wang, H, Li, C, et al. Mesenchymal stem cells-derived exosomes ameliorate lupus by inducing M2 macrophage polarization and regulatory T cell expansion in MRL/lpr mice. Immunol Investig. (2022) 51:1785–803. doi: 10.1080/08820139.2022.2055478
58. Dias, IE, Pinto, PO, Barros, LC, Viegas, CA, Dias, IR, and Carvalho, PP. Mesenchymal stem cells therapy in companion animals: useful for immune-mediated diseases? BMC Vet Res. (2019) 15:358. doi: 10.1186/s12917-019-2087-2
59. Valade, G, Libert, N, Martinaud, C, Vicaut, E, Banzet, S, and Peltzer, J. Therapeutic potential of mesenchymal stromal cell-derived extracellular vesicles in the prevention of organ injuries induced by traumatic hemorrhagic shock. Front Immunol. (2021) 12:749659. doi: 10.3389/fimmu.2021.749659
60. Phan, J, Kumar, P, Hao, D, Gao, K, Farmer, D, and Wang, A. Engineering mesenchymal stem cells to improve their exosome efficacy and yield for cell-free therapy. J Extracell Vesicles. (2018) 7:1522236. doi: 10.1080/20013078.2018.1522236
61. Fang, Y, Lao, P, Tang, L, Chen, J, Chen, Y, Sun, L, et al. Mechanisms of potential therapeutic utilization of mesenchymal stem cells in COVID-19 treatment. Cell Transplant. (2023) 32:09636897231184611. doi: 10.1177/09636897231184611
62. Lazar, SV, Mor, S, Wang, D, Goldbloom-Helzner, L, Clark, K, Hao, D, et al. Engineering extracellular vesicles for Alzheimer’s disease: an emerging cell-free approach for earlier diagnosis and treatment. WIREs Mech Dis. (2022) 14:e1541. doi: 10.1002/wsbm.1541
63. You, B, Jin, C, Zhang, J, Xu, M, Xu, W, Sun, Z, et al. MSC-derived extracellular vesicle-delivered L-PGDS inhibit gastric cancer progression by suppressing cancer cell stemness and STAT3 phosphorylation. Stem Cells Int. (2022) 2022:9668239. doi: 10.1155/2022/9668239
64. Heris, RM, Shirvaliloo, M, Abbaspour-Aghdam, S, Hazrati, A, Shariati, A, Youshanlouei, HR, et al. The potential use of mesenchymal stem cells and their exosomes in Parkinson’s disease treatment. Stem Cell Res Ther. (2022) 13:371. doi: 10.1186/s13287-022-03050-4
65. Ramasubramanian, L, Du, S, Gidda, S, Bahatyrevich, N, Hao, D, Kumar, P, et al. Bioengineering extracellular vesicles for the treatment of cardiovascular diseases. Adv Biol. (2022) 6:e2200087. doi: 10.1002/adbi.202200087
66. Wang, X, Tang, Y, Liu, Z, Yin, Y, Li, Q, Liu, G, et al. The application potential and advance of mesenchymal stem cell-derived exosomes in myocardial infarction. Stem Cells Int. (2021) 2021:5579904. doi: 10.1155/2021/5579904
67. Zhang, Y, Zhuang, H, Ren, X, Jiang, F, and Zhou, P. Therapeutic effects of different intervention forms of human umbilical cord mesenchymal stem cells in the treatment of osteoarthritis. Front Cell Dev Biol. (2023) 11:1246504. doi: 10.3389/fcell.2023.1246504
68. Kang, Y, Song, Y, Luo, Y, Song, J, Li, C, Yang, S, et al. Exosomes derived from human umbilical cord mesenchymal stem cells ameliorate experimental non-alcoholic steatohepatitis via Nrf 2/NQO-1 pathway. Free Radic Biol Med. (2022) 192:25–36. doi: 10.1016/j.freeradbiomed.2022.08.037
69. Mason, C, and Dunnill, P. A brief definition of regenerative medicine. Reg Med. (2008) 3:1–5. doi: 10.2217/17460751.3.1.1
70. Merino-González, C, Zuñiga, FA, Escudero, C, Ormazabal, V, Reyes, C, Nova-Lamperti, E, et al. Mesenchymal stem cell-derived extracellular vesicles promote angiogenesis: potencial clinical application. Front Physiol. (2016) 7:24. doi: 10.3389/fphys.2016.00024
71. Lu, J, Zhang, Y, Yang, X, and Zhao, H. Harnessing exosomes as cutting-edge drug delivery systems for revolutionary osteoarthritis therapy. Biomed Pharmacother. (2023) 165:115135. doi: 10.1016/j.biopha.2023.115135
72. Johnstone, RM, Adam, M, Hammond, JR, Orr, L, and Turbide, C. Vesicle formation during reticulocyte maturation. Association of plasma membrane activities with released vesicles (exosomes). J Biol Chem. (1987) 262:9412–20. doi: 10.1016/S0021-9258(18)48095-7
73. Chang, W-H, Cerione, RA, and Antonyak, MA. Extracellular vesicles and their roles in cancer progression. Methods Mol Biol. (2021) 2174:143–70. doi: 10.1007/978-1-0716-0759-6_10
74. Xie, F, Zhou, X, Fang, M, Li, H, Su, P, Tu, Y, et al. Extracellular vesicles in cancer immune microenvironment and cancer immunotherapy. Adv Sci. (2019) 6:1901779. doi: 10.1002/advs.201901779
75. Abhange, K, Makler, A, Wen, Y, Ramnauth, N, Mao, W, Asghar, W, et al. Small extracellular vesicles in cancer. Bioact Mater. (2021) 6:3705–43. doi: 10.1016/j.bioactmat.2021.03.015
76. Shah, R, Patel, T, and Freedman, JE. Circulating extracellular vesicles in human disease. N Engl J Med. (2018) 379:958–66. doi: 10.1056/NEJMra1704286
77. Ono, R, Yoshioka, Y, Furukawa, Y, Naruse, M, Kuwagata, M, Ochiya, T, et al. Novel hepatotoxicity biomarkers of extracellular vesicle (EV)-associated miRNAs induced by CCl4. Toxicol Rep. (2020) 7:685–92. doi: 10.1016/j.toxrep.2020.05.002
78. Anand, S, Samuel, M, and Mathivanan, S. Exomeres: a new member of extracellular vesicles family. Subcell Biochem. (2021) 97:89–97. doi: 10.1007/978-3-030-67171-6_5
79. Lötvall, J, Hill, AF, Hochberg, F, Buzás, EI, Di Vizio, D, Gardiner, C, et al. Minimal experimental requirements for definition of extracellular vesicles and their functions: a position statement from the International Society for Extracellular Vesicles. J Extracell Vesicles. (2014) 3:26913. doi: 10.3402/jev.v3.26913
80. Yáñez-Mó, M, Siljander, PR-M, Andreu, Z, Bedina Zavec, A, Borràs, FE, Buzas, EI, et al. Biological properties of extracellular vesicles and their physiological functions. J Extracell Vesicles. (2015) 4:27066. doi: 10.3402/jev.v4.27066
81. Mathieu, M, Martin-Jaular, L, Lavieu, G, and Théry, C. Specificities of secretion and uptake of exosomes and other extracellular vesicles for cell-to-cell communication. Nat Cell Biol. (2019) 21:9–17. doi: 10.1038/s41556-018-0250-9
82. Yang, Y, Li, C-W, Chan, L-C, Wei, Y, Hsu, J-M, Xia, W, et al. Exosomal PD-L1 harbors active defense function to suppress T cell killing of breast cancer cells and promote tumor growth. Cell Res. (2018) 28:862–4. doi: 10.1038/s41422-018-0060-4
83. Ma, L, Li, Y, Peng, J, Wu, D, Zhao, X, Cui, Y, et al. Discovery of the migrasome, an organelle mediating release of cytoplasmic contents during cell migration. Cell Res. (2015) 25:24–38. doi: 10.1038/cr.2014.135
84. Akers, JC, Gonda, D, Kim, R, Carter, BS, and Chen, CC. Biogenesis of extracellular vesicles (EV): exosomes, microvesicles, retrovirus-like vesicles, and apoptotic bodies. J Neuro-Oncol. (2013) 113:1–11. doi: 10.1007/s11060-013-1084-8
85. Hristov, M, Erl, W, Linder, S, and Weber, PC. Apoptotic bodies from endothelial cells enhance the number and initiate the differentiation of human endothelial progenitor cells in vitro. Blood. (2004) 104:2761–6. doi: 10.1182/blood-2003-10-3614
86. Zhang, H, Freitas, D, Kim, HS, Fabijanic, K, Li, Z, Chen, H, et al. Identification of distinct nanoparticles and subsets of extracellular vesicles by asymmetric flow field-flow fractionation. Nat Cell Biol. (2018) 20:332–43. doi: 10.1038/s41556-018-0040-4
87. Meehan, B, Rak, J, and Di Vizio, D. Oncosomes—large and small: what are they, where they came from? J Extracell Vesicles. (2016) 5:33109. doi: 10.3402/jev.v5.33109
88. Minciacchi, VR, Freeman, MR, and Di Vizio, D. Extracellular vesicles in cancer: exosomes, microvesicles and the emerging role of large oncosomes. Semin Cell Dev Biol. (2015) 40:41–51. doi: 10.1016/j.semcdb.2015.02.010
89. Zhang, Q, Jeppesen, DK, Higginbotham, JN, Graves-Deal, R, Trinh, VQ, Ramirez, MA, et al. Supermeres are functional extracellular nanoparticles replete with disease biomarkers and therapeutic targets. Nat Cell Biol. (2021) 23:1240–54. doi: 10.1038/s41556-021-00805-8
90. Tosar, JP, Cayota, A, and Witwer, K. Exomeres and supermeres: monolithic or diverse? J Extracell Biol. (2022) 1:e45. doi: 10.1002/jex2.45
91. Pallio, G. Novel therapeutic approaches in inflammatory bowel diseases. Biomedicines. (2023) 11:2466. doi: 10.3390/biomedicines11092466
92. Varderidou-Minasian, S, and Lorenowicz, MJ. Mesenchymal stromal/stem cell-derived extracellular vesicles in tissue repair: challenges and opportunities. Theranostics. (2020) 10:5979. doi: 10.7150/thno.40122
93. Witwer, KW, Buzás, EI, Bemis, LT, Bora, A, Lässer, C, Lötvall, J, et al. Standardization of sample collection, isolation and analysis methods in extracellular vesicle research. J Extracell Vesicles. (2013) 2:20360. doi: 10.3402/jev.v2i0.20360
94. Buzas, EI, György, B, Nagy, G, Falus, A, and Gay, S. Emerging role of extracellular vesicles in inflammatory diseases. Nat Rev Rheumatol. (2014) 10:356–64. doi: 10.1038/nrrheum.2014.19
95. Gibbings, DJ, Ciaudo, C, Erhardt, M, and Voinnet, O. Multivesicular bodies associate with components of miRNA effector complexes and modulate miRNA activity. Nat Cell Biol. (2009) 11:1143–9. doi: 10.1038/ncb1929
96. Kalluri, R, and LeBleu, VS. The biology, function, and biomedical applications of exosomes. Science. (2020) 367:eaau6977. doi: 10.1126/science.aau6977
97. Harding, C, Heuser, J, and Stahl, P. Receptor-mediated endocytosis of transferrin and recycling of the transferrin receptor in rat reticulocytes. J Cell Biol. (1983) 97:329–39. doi: 10.1083/jcb.97.2.329
98. Vella, LJ, Sharples, RA, Nisbet, RM, Cappai, R, and Hill, AF. The role of exosomes in the processing of proteins associated with neurodegenerative diseases. Eur Biophys J. (2008) 37:323–32. doi: 10.1007/s00249-007-0246-z
99. Baghaei, K, Tokhanbigli, S, Asadzadeh, H, Nmaki, S, Reza Zali, M, and Hashemi, SM. Exosomes as a novel cell-free therapeutic approach in gastrointestinal diseases. J Cell Physiol. (2019) 234:9910–26. doi: 10.1002/jcp.27934
100. Carrière, J, Bretin, A, Darfeuille-Michaud, A, Barnich, N, and Nguyen, HTT. Exosomes released from cells infected with Crohn’s disease-associated adherent-invasive Escherichia coli activate host innate immune responses and enhance bacterial intracellular replication. Inflamm Bowel Dis. (2016) 22:516–28. doi: 10.1097/MIB.0000000000000635
101. Mitsuhashi, S, Feldbrügge, L, Csizmadia, E, Mitsuhashi, M, Robson, SC, and Moss, AC. Luminal extracellular vesicles (EVs) in inflammatory bowel disease (IBD) exhibit proinflammatory effects on epithelial cells and macrophages. Inflamm Bowel Dis. (2016) 22:1587–95. doi: 10.1097/MIB.0000000000000840
102. Zheng, X, Chen, F, Zhang, Q, Liu, Y, You, P, Sun, S, et al. Salivary exosomal PSMA7: a promising biomarker of inflammatory bowel disease. Protein Cell. (2017) 8:686–95. doi: 10.1007/s13238-017-0413-7
103. Hovhannisyan, L, Czechowska, E, and Gutowska-Owsiak, D. The role of non-immune cell-derived extracellular vesicles in allergy. Front Immunol. (2021) 12:702381. doi: 10.3389/fimmu.2021.702381
104. Gross, JC, Chaudhary, V, Bartscherer, K, and Boutros, M. Active Wnt proteins are secreted on exosomes. Nat Cell Biol. (2012) 14:1036–45. doi: 10.1038/ncb2574
105. Natasha, G, Gundogan, B, Tan, A, Farhatnia, Y, Wu, W, Rajadas, J, et al. Exosomes as immunotheranostic nanoparticles. Clin Ther. (2014) 36:820–9. doi: 10.1016/j.clinthera.2014.04.019
106. Tran, T-H, Mattheolabakis, G, Aldawsari, H, and Amiji, M. Exosomes as nanocarriers for immunotherapy of cancer and inflammatory diseases. Clin Immunol. (2015) 160:46–58. doi: 10.1016/j.clim.2015.03.021
107. Boukouris, S, and Mathivanan, S. Exosomes in bodily fluids are a highly stable resource of disease biomarkers. Proteomics Clin Appl. (2015) 9:358–67. doi: 10.1002/prca.201400114
108. Ostrowski, M, Carmo, NB, Krumeich, S, Fanget, I, Raposo, G, Savina, A, et al. Rab 27a and Rab 27b control different steps of the exosome secretion pathway. Nat Cell Biol. (2010) 12:19–30. doi: 10.1038/ncb2000
109. Greening, DW, Gopal, SK, Xu, R, Simpson, RJ, and Chen, W. Exosomes and their roles in immune regulation and cancer. Semin Cell Dev Biol. (2015) 40:72–81. doi: 10.1016/j.semcdb.2015.02.009
110. Théry, C, Boussac, M, Véron, P, Ricciardi-Castagnoli, P, Raposo, G, Garin, J, et al. Proteomic analysis of dendritic cell-derived exosomes: a secreted subcellular compartment distinct from apoptotic vesicles. J Immunol. (2001) 166:7309–18. doi: 10.4049/jimmunol.166.12.7309
111. Mathivanan, S, Fahner, CJ, Reid, GE, and Simpson, RJ. ExoCarta 2012: database of exosomal proteins, RNA and lipids. Nucleic Acids Res. (2012) 40:D1241–4. doi: 10.1093/nar/gkr828
112. Li, P, Kaslan, M, Lee, SH, Yao, J, and Gao, Z. Progress in exosome isolation techniques. Theranostics. (2017) 7:789. doi: 10.7150/thno.18133
113. Théry, C, Zitvogel, L, and Amigorena, S. Exosomes: composition, biogenesis and function. Nat Rev Immunol. (2002) 2:569–79. doi: 10.1038/nri855
114. Gebert, LFR, and Mac Rae, IJ. Regulation of microRNA function in animals. Nat Rev Mol Cell Biol. (2019) 20:21–37. doi: 10.1038/s41580-018-0045-7
115. Matsuo, H, Chevallier, J, Mayran, N, Le Blanc, I, Ferguson, C, Fauré, J, et al. Role of LBPA and Alix in multivesicular liposome formation and endosome organization. Science. (2004) 303:531–4. doi: 10.1126/science.1092425
116. Bellingham, SA, Coleman, BM, and Hill, AF. Small RNA deep sequencing reveals a distinct miRNA signature released in exosomes from prion-infected neuronal cells. Nucleic Acids Res. (2012) 40:10937–49. doi: 10.1093/nar/gks832
117. Eirin, A, Riester, SM, Zhu, X-Y, Tang, H, Evans, JM, O’Brien, D, et al. MicroRNA and mRNA cargo of extracellular vesicles from porcine adipose tissue-derived mesenchymal stem cells. Gene. (2014) 551:55–64. doi: 10.1016/j.gene.2014.08.041
118. Ren, H, Sang, Y, Zhang, F, Liu, Z, Qi, N, and Chen, Y. Comparative analysis of human mesenchymal stem cells from umbilical cord, dental pulp, and menstrual blood as sources for cell therapy. Stem Cells Int. (2016) 2016:3516574. doi: 10.1155/2016/3516574
119. Zhou, J, Benito-Martin, A, Mighty, J, Chang, L, Ghoroghi, S, Wu, H, et al. Retinal progenitor cells release extracellular vesicles containing developmental transcription factors, microRNA and membrane proteins. Sci Rep. (2018) 8:2823. doi: 10.1038/s41598-018-20421-1
120. Bi, S, Nie, Q, Wang, W, Zhu, Y, Ma, X, Wang, C, et al. Human umbilical cord mesenchymal stem cells therapy for insulin resistance: a novel strategy in clinical implication. Curr Stem Cell Res Ther. (2018) 13:658–64. doi: 10.2174/1574888X13666180810154048
121. Xiong, Z-H, Wei, J, Lu, M-Q, Jin, M-Y, and Geng, H-L. Protective effect of human umbilical cord mesenchymal stem cell exosomes on preserving the morphology and angiogenesis of placenta in rats with preeclampsia. Biomed Pharmacother. (2018) 105:1240–7. doi: 10.1016/j.biopha.2018.06.032
122. Guo, G, Zhuang, X, Xu, Q, Wu, Z, Zhu, Y, Zhou, Y, et al. Peripheral infusion of human umbilical cord mesenchymal stem cells rescues acute liver failure lethality in monkeys. Stem Cell Res Ther. (2019) 10:84. doi: 10.1186/s13287-019-1184-2
123. Day, AGE, Francis, WR, Fu, K, Pieper, IL, Guy, O, and Xia, Z. Osteogenic potential of human umbilical cord mesenchymal stem cells on coralline hydroxyapatite/calcium carbonate microparticles. Stem Cells Int. (2018) 2018:4258613. doi: 10.1155/2018/4258613
124. Wu, K-C, Chang, Y-H, Liu, H-W, and Ding, D-C. Transplanting human umbilical cord mesenchymal stem cells and hyaluronate hydrogel repairs cartilage of osteoarthritis in the minipig model. Tzu Chi Med J. (2019) 31:11–9. doi: 10.4103/tcmj.tcmj_87_18
125. Tanaka, E, Ogawa, Y, Mukai, T, Sato, Y, Hamazaki, T, Nagamura-Inoue, T, et al. Dose-dependent effect of intravenous administration of human umbilical cord-derived mesenchymal stem cells in neonatal stroke mice. Front Neurol. (2018) 9:133. doi: 10.3389/fneur.2018.00133
126. Mirza, A, Khan, I, Salim, A, Husain, M, and Herzig, JW. Role of Wnt/β-catenin pathway in cardiac lineage commitment of human umbilical cord mesenchymal stem cells by zebularine and 2′-deoxycytidine. Tissue Cell. (2022) 77:101850. doi: 10.1016/j.tice.2022.101850
127. Mao, F, Wu, Y, Tang, X, Wang, J, Pan, Z, Zhang, P, et al. Human umbilical cord mesenchymal stem cells alleviate inflammatory bowel disease through the regulation of 15-LOX-1 in macrophages. Biotechnol Lett. (2017) 39:929–38. doi: 10.1007/s10529-017-2315-4
128. Li, Y, Ma, K, Zhang, L, Xu, H, and Zhang, N. Human umbilical cord blood derived-mesenchymal stem cells alleviate dextran sulfate sodium-induced colitis by increasing regulatory T cells in mice. Front Cell Dev Biol. (2020) 8:604021. doi: 10.3389/fcell.2020.604021
129. Subramanian, A, Fong, C-Y, Biswas, A, and Bongso, A. Comparative characterization of cells from the various compartments of the human umbilical cord shows that the Wharton’s jelly compartment provides the best source of clinically utilizable mesenchymal stem cells. PLoS One. (2015) 10:e0127992. doi: 10.1371/journal.pone.0127992
130. Rizano, A, Margiana, R, Supardi, S, and Narulita, P. Exploring the future potential of mesenchymal stem/stromal cells and their derivatives to support assisted reproductive technology for female infertility applications. Hum Cell. (2023) 36:1604–19. doi: 10.1007/s13577-023-00941-3
131. Bárcia, RN. What makes umbilical cord tissue‐derived mesenchymal stromal cells superior immunomodulators when compared to bone marrow derived mesenchymal stromal cells? Stem Cells Intern. (2015) 1:583984.
132. Stefańska, K, Ożegowska, K, Hutchings, G, Popis, M, Moncrieff, L, Dompe, C, et al. Human Wharton’s jelly—cellular specificity, stemness potency, animal models, and current application in human clinical trials. J Clin Med. (2020) 9:1102. doi: 10.3390/jcm9041102
133. Chen, K, Wang, D, Du, WT, Han, Z-B, Ren, H, Chi, Y, et al. Human umbilical cord mesenchymal stem cells hUC-MSCs exert immunosuppressive activities through a PGE2-dependent mechanism. Clin Immunol. (2010) 135:448–58. doi: 10.1016/j.clim.2010.01.015
134. Kim, J-H, Jo, CH, Kim, H-R, and Hwang, Y. Comparison of immunological characteristics of mesenchymal stem cells from the periodontal ligament, umbilical cord, and adipose tissue. Stem Cells Int. (2018) 2018:8429042. doi: 10.1155/2018/8429042
135. Mennan, C, Wright, K, Bhattacharjee, A, Balain, B, Richardson, J, and Roberts, S. Isolation and characterisation of mesenchymal stem cells from different regions of the human umbilical cord. Biomed Res Int. (2013) 2013:916136. doi: 10.1155/2013/916136
136. Jo, CH, Lim, H-J, and Yoon, KS. Characterization of tendon-specific markers in various human tissues, tenocytes and mesenchymal stem cells. Tissue Eng Regen Med. (2019) 16:151–9. doi: 10.1007/s13770-019-00182-2
137. Wang, Z, He, Z, Liang, S, Yang, Q, Cheng, P, and Chen, A. Comprehensive proteomic analysis of exosomes derived from human bone marrow, adipose tissue, and umbilical cord mesenchymal stem cells. Stem Cell Res Ther. (2020) 11:511. doi: 10.1186/s13287-020-02032-8
138. Monov, D, and Pashanova, O. Experimental substantiation of the use of phenibut combinations with salicylic, nicotinic, and glutamic acids in cerebral ischemia. Neurocrit Care. (2023) 39:464–77. doi: 10.1007/s12028-023-01719-z
139. Kidd, S, Spaeth, E, Dembinski, JL, Dietrich, M, Watson, K, Klopp, A, et al. Direct evidence of mesenchymal stem cell tropism for tumor and wounding microenvironments using in vivo bioluminescent imaging. Stem Cells. (2009) 27:2614–23. doi: 10.1002/stem.187
140. Xue, Z-L, Meng, Y-L, and Ge, J-H. Upregulation of miR-132 attenuates osteoblast differentiation of UC-MSCs. Eur Rev Med Pharmacol Sci. (2018) 22:1580–7. doi: 10.26355/eurrev_201808_15643
141. Motawea, SM, Noreldin, RI, and Naguib, YM. Potential therapeutic effects of endothelial cells trans-differentiated from Wharton’s jelly-derived mesenchymal stem cells on altered vascular functions in aged diabetic rat model. Diabetol Metab Syndr. (2020) 12:40. doi: 10.1186/s13098-020-00546-y
142. Wu, KH, Wang, SY, Xiao, QR, Yang, Y, Huang, NP, Mo, XM, et al. Efficient generation of functional cardiomyocytes from human umbilical cord-derived virus-free induced pluripotent stem cells. Cell Tissue Res. (2018) 374:275–83. doi: 10.1007/s00441-018-2875-1
143. Zhou, X, Cui, L, Zhou, X, Yang, Q, Wang, L, Guo, G, et al. Induction of hepatocyte-like cells from human umbilical cord-derived mesenchymal stem cells by defined microRNAs. J Cell Mol Med. (2017) 21:881–93. doi: 10.1111/jcmm.13027
144. Van Pham, P, Nguyen, PT-M, Nguyen, AT-Q, Pham, VM, Bui, AN-T, Dang, LT-T, et al. Improved differentiation of umbilical cord blood-derived mesenchymal stem cells into insulin-producing cells by PDX-1 mRNA transfection. Differentiation. (2014) 87:200–8. doi: 10.1016/j.diff.2014.08.001
145. Ortiz, GGR, Zaidi, NH, Saini, RS, Coronel, AAR, Alsandook, T, Lafta, MH, et al. The developing role of extracellular vesicles in autoimmune diseases: special attention to mesenchymal stem cell-derived extracellular vesicles. Int Immunopharmacol. (2023) 122:110531. doi: 10.1016/j.intimp.2023.110531
146. Lener, T, Gimona, M, Aigner, L, Börger, V, Buzas, E, Camussi, G, et al. Applying extracellular vesicles based therapeutics in clinical trials—an ISEV position paper. J Extracell Vesicles. (2015) 4:30087. doi: 10.3402/jev.v4.30087
147. Théry, C, Amigorena, S, Raposo, G, and Clayton, A. Isolation and characterization of exosomes from cell culture supernatants and biological fluids. Curr Protoc Cell Biol. (2006) 30:3–22. doi: 10.1002/0471143030.cb0322s30
148. Lobb, RJ, Becker, M, Wen Wen, S, Wong, CSF, Wiegmans, AP, Leimgruber, A, et al. Optimized exosome isolation protocol for cell culture supernatant and human plasma. J Extracell Vesicles. (2015) 4:27031. doi: 10.3402/jev.v4.27031
149. Gardiner, C, Di Vizio, D, Sahoo, S, Théry, C, Witwer, KW, Wauben, M, et al. Techniques used for the isolation and characterization of extracellular vesicles: results of a worldwide survey. J Extracell Vesicles. (2016) 5:32945. doi: 10.3402/jev.v5.32945
150. Lozano-Ramos, I, Bancu, I, Oliveira-Tercero, A, Armengol, MP, Menezes-Neto, A, Del Portillo, HA, et al. Size-exclusion chromatography-based enrichment of extracellular vesicles from urine samples. J Extracell Vesicles. (2015) 4:27369. doi: 10.3402/jev.v4.27369
151. Arraud, N, Gounou, C, Turpin, D, and Brisson, AR. Fluorescence triggering: a general strategy for enumerating and phenotyping extracellular vesicles by flow cytometry. Cytometry A. (2016) 89:184–95. doi: 10.1002/cyto.a.22669
152. Chen, C, Luo, Y, He, W, Zhao, Y, Kong, Y, Liu, H, et al. Exosomal long noncoding RNA LNMAT2 promotes lymphatic metastasis in bladder cancer. J Clin Invest. (2020) 130:404–21. doi: 10.1172/JCI130892
153. Kusuma, GD, Barabadi, M, Tan, JL, Morton DA, V, Frith, JE, and Lim, R. To protect and to preserve: novel preservation strategies for extracellular vesicles. Front Pharmacol. (2018) 9:1199. doi: 10.3389/fphar.2018.01199
154. Llorente, A, van Deurs, B, and Sandvig, K. Cholesterol regulates prostasome release from secretory lysosomes in PC-3 human prostate cancer cells. Eur J Cell Biol. (2007) 86:405–15. doi: 10.1016/j.ejcb.2007.05.001
155. Diaz, G, Bridges, C, Lucas, M, Cheng, Y, Schorey, JS, Dobos, KM, et al. Protein digestion, ultrafiltration, and size exclusion chromatography to optimize the isolation of exosomes from human blood plasma and serum. J Vis Exp. (2018):e57467. doi: 10.3791/57467-v
156. Shtam, T, Evtushenko, V, Samsonov, R, Zabrodskaya, Y, Kamyshinsky, R, Zabegina, L, et al. Evaluation of immune and chemical precipitation methods for plasma exosome isolation. PLoS One. (2020) 15:e0242732. doi: 10.1371/journal.pone.0242732
157. Li, K, Wong, DK, Hong, KY, and Raffai, RL. Cushioned-density gradient ultracentrifugation (C-DGUC): a refined and high performance method for the isolation, characterization, and use of exosomes. Methods Mol Biol. (2018) 1740:69–83. doi: 10.1007/978-1-4939-7652-2_7
158. Sidhom, K, Obi, PO, and Saleem, A. A review of exosomal isolation methods: is size exclusion chromatography the best option? Int J Mol Sci. (2020) 21:6466. doi: 10.3390/ijms21186466
159. Wang, J, Ma, P, Kim, DH, Liu, B-F, and Demirci, U. Towards microfluidic-based exosome isolation and detection for tumor therapy. Nano Today. (2021) 37:101066. doi: 10.1016/j.nantod.2020.101066
160. Li, Y, Altemus, J, and Lightner, AL. Mesenchymal stem cells and acellular products attenuate murine induced colitis. Stem Cell Res Ther. (2020) 11:515. doi: 10.1186/s13287-020-02025-7
161. Alves, VBF, de Sousa, BC, Fonseca, MTC, Ogata, H, Caliari-Oliveira, C, Yaochite, JNU, et al. A single administration of human adipose tissue-derived mesenchymal stromal cells (MSC) induces durable and sustained long-term regulation of inflammatory response in experimental colitis. Clin Exp Immunol. (2019) 196:139–54. doi: 10.1111/cei.13262
162. Yang, S, Liang, X, Song, J, Li, C, Liu, A, Luo, Y, et al. A novel therapeutic approach for inflammatory bowel disease by exosomes derived from human umbilical cord mesenchymal stem cells to repair intestinal barrier via TSG-6. Stem Cell Res Ther. (2021) 12:315. doi: 10.1186/s13287-021-02404-8
163. Song, W-J, Li, Q, Ryu, M-O, Ahn, J-O, Bhang, DH, Jung, YC, et al. TSG-6 released from intraperitoneally injected canine adipose tissue-derived mesenchymal stem cells ameliorate inflammatory bowel disease by inducing M2 macrophage switch in mice. Stem Cell Res Ther. (2018) 9:91. doi: 10.1186/s13287-018-0841-1
164. Sala, E, Genua, M, Petti, L, Anselmo, A, Arena, V, Cibella, J, et al. Mesenchymal stem cells reduce colitis in mice via release of TSG6, independently of their localization to the intestine. Gastroenterology. (2015) 149:163–76. doi: 10.1053/j.gastro.2015.03.013
165. Cao, L, Xu, H, Wang, G, Liu, M, Tian, D, and Yuan, Z. Extracellular vesicles derived from bone marrow mesenchymal stem cells attenuate dextran sodium sulfate-induced ulcerative colitis by promoting M2 macrophage polarization. Int Immunopharmacol. (2019) 72:264–74. doi: 10.1016/j.intimp.2019.04.020
166. Heidari, N, Abbasi-Kenarsari, H, Namaki, S, Baghaei, K, Zali, MR, Ghaffari Khaligh, S, et al. Adipose-derived mesenchymal stem cell-secreted exosome alleviates dextran sulfate sodium-induced acute colitis by Treg cell induction and inflammatory cytokine reduction. J Cell Physiol. (2021) 236:5906–20. doi: 10.1002/jcp.30275
167. Qi, L, Wu, J, Zhu, S, Wang, X, Lv, X, Liu, C, et al. Mesenchymal stem cells alleviate inflammatory bowel disease via Tr1 cells. Stem Cell Rev Rep. (2022) 18:2444–57. doi: 10.1007/s12015-022-10353-9
168. Li, P, Zhang, H, Gao, J, Du, W, Tang, D, Wang, W, et al. Mesenchymal stem cells-derived extracellular vesicles containing miR-378a-3p inhibit the occurrence of inflammatory bowel disease by targeting GATA2. J Cell Mol Med. (2022) 26:3133–46. doi: 10.1111/jcmm.17176
169. Xu, J, Wang, X, Chen, J, Chen, S, Li, Z, Liu, H, et al. Embryonic stem cell-derived mesenchymal stem cells promote colon epithelial integrity and regeneration by elevating circulating IGF-1 in colitis mice. Theranostics. (2020) 10:12204. doi: 10.7150/thno.47683
170. Liu, P, Xie, X, Wu, H, Li, H, Chi, J, Liu, X, et al. Mesenchymal stem cells promote intestinal mucosal repair by positively regulating the Nrf 2/Keap 1/ARE signaling pathway in acute experimental colitis. Dig Dis Sci. (2023) 68:1835–46. doi: 10.1007/s10620-022-07722-2
171. Lou, G, Chen, Z, Zheng, M, and Liu, Y. Mesenchymal stem cell-derived exosomes as a new therapeutic strategy for liver diseases. Exp Mol Med. (2017) 49:e346–6. doi: 10.1038/emm.2017.63
172. Shen, Z, Huang, W, Liu, J, Tian, J, Wang, S, and Rui, K. Effects of mesenchymal stem cell-derived exosomes on autoimmune diseases. Front Immunol. (2021) 12:749192. doi: 10.3389/fimmu.2021.749192
173. Mao, F, Wu, Y, Tang, X, Kang, J, Zhang, B, Yan, Y, et al. Exosomes derived from human umbilical cord mesenchymal stem cells relieve inflammatory bowel disease in mice. Biomed Res Int. (2017) 2017:5356760. doi: 10.1155/2017/5356760
174. Cai, X, Zhang, Z, Yuan, J, Ocansey, DKW, Tu, Q, Zhang, X, et al. hucMSC-derived-derived exosomes attenuate colitis by regulating macrophage pyroptosis via the miR-378a-5p/NLRP3 axis. Stem Cell Res Ther. (2021) 12:416. doi: 10.1186/s13287-021-02492-6
175. Yu, H, Yang, X, Xiao, X, Xu, M, Yang, Y, Xue, C, et al. Human adipose mesenchymal stem cell-derived exosomes protect mice from DSS-induced inflammatory bowel disease by promoting intestinal-stem-cell and epithelial regeneration. Aging Dis. (2021) 12:1423. doi: 10.14336/AD.2021.0601
176. Xu, Y, Zhang, L, Ocansey, DKW, Wang, B, Hou, Y, Mei, R, et al. HucMSC-Ex alleviates inflammatory bowel disease via the lnc78583-mediated miR3202/HOXB13 pathway. J Zhejiang Univ Sci B. (2022) 23:423–31. doi: 10.1631/jzus.B2100793
177. Sun, D, Cao, H, Yang, L, Lin, L, Hou, B, Zheng, W, et al. MiR-200b in heme oxygenase-1-modified bone marrow mesenchymal stem cell-derived exosomes alleviates inflammatory injury of intestinal epithelial cells by targeting high mobility group box 3. Cell Death Dis. (2020) 11:480. doi: 10.1038/s41419-020-2685-8
178. Yang, R, Huang, H, Cui, S, Zhou, Y, Zhang, T, and Zhou, Y. IFN-γ promoted exosomes from mesenchymal stem cells to attenuate colitis via miR-125a and miR-125b. Cell Death Dis. (2020) 11:603. doi: 10.1038/s41419-020-02788-0
179. Liu, H, Liang, Z, Wang, F, Zhou, C, Zheng, X, Hu, T, et al. Exosomes from mesenchymal stromal cells reduce murine colonic inflammation via a macrophage-dependent mechanism. JCI Insight. (2019) 4:e131273. doi: 10.1172/jci.insight.131273
180. Tian, J, Zhu, Q, Zhang, Y, Bian, Q, Hong, Y, Shen, Z, et al. Olfactory ecto-mesenchymal stem cell-derived exosomes ameliorate experimental colitis via modulating Th1/Th17 and Treg cell responses. Front Immunol. (2020) 11:598322. doi: 10.3389/fimmu.2020.598322
181. Wu, Y, Qiu, W, Xu, X, Kang, J, Wang, J, Wen, Y, et al. Exosomes derived from human umbilical cord mesenchymal stem cells alleviate inflammatory bowel disease in mice through ubiquitination. Am J Transl Res. (2018) 10:2026.
182. Yang, J, Liu, X-X, Fan, H, Tang, Q, Shou, Z-X, Zuo, D-M, et al. Extracellular vesicles derived from bone marrow mesenchymal stem cells protect against experimental colitis via attenuating colon inflammation, oxidative stress and apoptosis. PLoS One. (2015) 10:e0140551. doi: 10.1371/journal.pone.0145800
183. Zhang, N, Chen, Y, Huang, C, Wei, M, Li, T, Lv, Y, et al. Adipose-derived mesenchymal stem cells may reduce intestinal epithelial damage in ulcerative colitis by communicating with macrophages and blocking inflammatory pathways: an analysis in silico. Aging. (2022) 14:2665. doi: 10.18632/aging.203964
184. Baumgart, DC, and Carding, SR. Inflammatory bowel disease: cause and immunobiology. Lancet. (2007) 369:1627–40. doi: 10.1016/S0140-6736(07)60750-8
185. Lee, SH, Eun Kwon, J, and Cho, M-L. Immunological pathogenesis of inflammatory bowel disease. Intest Res. (2018) 16:26–42. doi: 10.5217/ir.2018.16.1.26
186. Mosser, DM, and Edwards, JP. Exploring the full spectrum of macrophage activation. Nat Rev Immunol. (2008) 8:958–69. doi: 10.1038/nri2448
187. Atri, C, Guerfali, FZ, and Laouini, D. Role of human macrophage polarization in inflammation during infectious diseases. Int J Mol Sci. (2018) 19:1801. doi: 10.3390/ijms19061801
188. Na, YR, Stakenborg, M, Seok, SH, and Matteoli, G. Macrophages in intestinal inflammation and resolution: a potential therapeutic target in IBD. Nat Rev Gastroenterol Hepatol. (2019) 16:531–43. doi: 10.1038/s41575-019-0172-4
189. Vergadi, E, Ieronymaki, E, Lyroni, K, Vaporidi, K, and Tsatsanis, C. Akt signaling pathway in macrophage activation and M1/M2 polarization. J Immunol. (2017) 198:1006–14. doi: 10.4049/jimmunol.1601515
190. Laskin, DL, Sunil, VR, Gardner, CR, and Laskin, JD. Macrophages and tissue injury: agents of defense or destruction? Annu Rev Pharmacol Toxicol. (2011) 51:267–88. doi: 10.1146/annurev.pharmtox.010909.105812
191. An, J-H, Li, Q, Ryu, M-O, Nam, A-R, Bhang, D-H, Jung, Y-C, et al. TSG-6 in extracellular vesicles from canine mesenchymal stem/stromal is a major factor in relieving DSS-induced colitis. PLoS One. (2020) 15:e0220756. doi: 10.1371/journal.pone.0220756
192. Wang, J, Pei, B, Yan, J, Xu, X, Fang, A-N, Ocansey, DKW, et al. hucMSC-derived exosomes alleviate the deterioration of colitis via the miR-146a/SUMO1 axis. Mol Pharm. (2022) 19:484–93. doi: 10.1021/acs.molpharmaceut.1c00450
193. Zhang, B, Yin, Y, Lai, RC, Tan, SS, Choo, ABH, and Lim, SK. Mesenchymal stem cells secrete immunologically active exosomes. Stem Cells Dev. (2014) 23:1233–44. doi: 10.1089/scd.2013.0479
194. Shen, B, Liu, J, Zhang, F, Wang, Y, Qin, Y, Zhou, Z, et al. CCR2 positive exosome released by mesenchymal stem cells suppresses macrophage functions and alleviates ischemia/reperfusion-induced renal injury. Stem Cells Int. (2016) 2016:1240301. doi: 10.1155/2016/1240301
195. Willis, GR, Fernandez-Gonzalez, A, Anastas, J, Vitali, SH, Liu, X, Ericsson, M, et al. Mesenchymal stromal cell exosomes ameliorate experimental bronchopulmonary dysplasia and restore lung function through macrophage immunomodulation. Am J Respir Crit Care Med. (2018) 197:104–16. doi: 10.1164/rccm.201705-0925OC
196. Kane, S, and Lemieux, N. The role of breastfeeding in postpartum disease activity in women with inflammatory bowel disease. Am J Gastroenterol. (2005) 100:102–5. doi: 10.1111/j.1572-0241.2005.40785.x
197. Fuss, IJ, Heller, F, Boirivant, M, Leon, F, Yoshida, M, Fichtner-Feigl, S, et al. Nonclassical CD1d-restricted NK T cells that produce IL-13 characterize an atypical Th2 response in ulcerative colitis. J Clin Invest. (2004) 113:1490–7. doi: 10.1172/JCI19836
198. Kanai, T, Kawamura, T, Dohi, T, Makita, S, Nemoto, Y, Totsuka, T, et al. TH1/TH2-mediated colitis induced by adoptive transfer of CD4+ CD45RBhigh T lymphocytes into nude mice. Inflamm Bowel Dis. (2006) 12:89–99. doi: 10.1097/01.MIB.0000197237.21387.mL
199. Lotfinejad, P, Shamsasenjan, K, Baradaran, B, Safarzadeh, E, Kazemi, T, and Movassaghpour, AA. Immunomodulatory effect of human umbilical cord blood-derived mesenchymal stem cells on activated T-lymphocyte. Iran J Allergy Asthma Immunol. (2021) 20:711–20. doi: 10.18502/ijaai.v20i6.8022
200. Jiang, X-X, Zhang, YI, Liu, B, Zhang, S-X, Wu, Y, Yu, X-D, et al. Human mesenchymal stem cells inhibit differentiation and function of monocyte-derived dendritic cells. Blood. (2005) 105:4120–6. doi: 10.1182/blood-2004-02-0586
201. Rabinovich, GA, Alonso, CR, Sotomayor, CE, Durand, S, Bocco, JL, and Riera, CM. Molecular mechanisms implicated in galectin-1-induced apoptosis: activation of the AP-1 transcription factor and downregulation of Bcl-2. Cell Death Differ. (2000) 7:747–53. doi: 10.1038/sj.cdd.4400708
202. Francisco, LM, Salinas, VH, Brown, KE, Vanguri, VK, Freeman, GJ, Kuchroo, VK, et al. PD-L1 regulates the development, maintenance, and function of induced regulatory T cells. J Exp Med. (2009) 206:3015–29. doi: 10.1084/jem.20090847
203. Del Fattore, A, Luciano, R, Pascucci, L, Goffredo, BM, Giorda, E, Scapaticci, M, et al. Immunoregulatory effects of mesenchymal stem cell-derived extracellular vesicles on T lymphocytes. Cell Transplant. (2015) 24:2615–27. doi: 10.3727/096368915X687543
204. Eastaff-Leung, N, Mabarrack, N, Barbour, A, Cummins, A, and Barry, S. Foxp 3+ regulatory T cells, Th17 effector cells, and cytokine environment in inflammatory bowel disease. J Clin Immunol. (2010) 30:80–9. doi: 10.1007/s10875-009-9345-1
205. Galley, JD, Parry, NM, Ahmer, BMM, Fox, JG, and Bailey, MT. The commensal microbiota exacerbate infectious colitis in stressor-exposed mice. Brain Behav Immun. (2017) 60:44–50. doi: 10.1016/j.bbi.2016.09.010
206. Morel, PA, and Butterfield, LH. Dendritic cell control of immune responses. Front Immunol. (2015) 6:42. doi: 10.3389/fimmu.2015.00042
207. Nakamura, Y, Miyaki, S, Ishitobi, H, Matsuyama, S, Nakasa, T, Kamei, N, et al. Mesenchymal-stem-cell-derived exosomes accelerate skeletal muscle regeneration. FEBS Lett. (2015) 589:1257–65. doi: 10.1016/j.febslet.2015.03.031
208. La Rocca, G, Anzalone, R, Corrao, S, Magno, F, Loria, T, Lo Iacono, M, et al. Isolation and characterization of Oct-4+/HLA-G+ mesenchymal stem cells from human umbilical cord matrix: differentiation potential and detection of new markers. Histochem Cell Biol. (2009) 131:267–82. doi: 10.1007/s00418-008-0519-3
209. Bang, OY, and Kim, EH. Mesenchymal stem cell-derived extracellular vesicle therapy for stroke: challenges and progress. Front Neurol. (2019) 10:211. doi: 10.3389/fneur.2019.00211
210. Zhao, Q, Yang, W-R, Wang, X-H, Li, G-Q, Xu, L-Q, Cui, X, et al. Clostridium butyricum alleviates intestinal low-grade inflammation in TNBS-induced irritable bowel syndrome in mice by regulating functional status of lamina propria dendritic cells. World J Gastroenterol. (2019) 25:5469. doi: 10.3748/wjg.v25.i36.5469
211. Qian, X, An, N, Ren, Y, Yang, C, Zhang, X, and Li, L. Immunosuppressive effects of mesenchymal stem cells-derived exosomes. Stem Cell Rev Rep. (2021) 17:411–27. doi: 10.1007/s12015-020-10040-7
212. Grégoire, C, Lechanteur, C, Briquet, A, Baudoux, É, Baron, F, Louis, E, et al. Mesenchymal stromal cell therapy for inflammatory bowel diseases. Aliment Pharmacol Ther. (2017) 45:205–21. doi: 10.1111/apt.13864
213. Chi, L, Khan, I, Lin, Z, Zhang, J, Lee, MYS, Leong, W, et al. Fructo-oligosaccharides from Morinda officinalis remodeled gut microbiota and alleviated depression features in a stress rat model. Phytomedicine. (2020) 67:153157. doi: 10.1016/j.phymed.2019.153157
214. He, Z, Cui, B-T, Zhang, T, Li, P, Long, C-Y, Ji, G-Z, et al. Fecal microbiota transplantation cured epilepsy in a case with Crohn’s disease: the first report. World J Gastroenterol. (2017) 23:3565. doi: 10.3748/wjg.v23.i19.3565
215. Llewellyn, SR, Britton, GJ, Contijoch, EJ, Vennaro, OH, Mortha, A, Colombel, J-F, et al. Interactions between diet and the intestinal microbiota alter intestinal permeability and colitis severity in mice. Gastroenterology. (2018) 154:1037–46. doi: 10.1053/j.gastro.2017.11.030
216. Holmberg, FEO, Pedersen, J, Jørgensen, P, Soendergaard, C, Jensen, KB, and Nielsen, OH. Intestinal barrier integrity and inflammatory bowel disease: stem cell-based approaches to regenerate the barrier. J Tissue Eng Regen Med. (2018) 12:923–35. doi: 10.1002/term.2506
217. Bhattacharyya, A, Chattopadhyay, R, Mitra, S, and Crowe, SE. Oxidative stress: an essential factor in the pathogenesis of gastrointestinal mucosal diseases. Physiol Rev. (2014) 94:329–54. doi: 10.1152/physrev.00040.2012
218. Tanida, S, Mizoshita, T, Mizushima, T, Sasaki, M, Shimura, T, Kamiya, T, et al. Involvement of oxidative stress and mucosal addressin cell adhesion molecule-1 (MAdCAM-1) in inflammatory bowel disease. J Clin Biochem Nutr. (2011) 48:112–6. doi: 10.3164/jcbn.10-41
219. Pravda, J. Radical induction theory of ulcerative colitis. World J Gastroenterol. (2005) 11:2371. doi: 10.3748/wjg.v11.i16.2371
220. Pavlick, KP, Laroux, FS, Fuseler, J, Wolf, RE, Gray, L, Hoffman, J, et al. Role of reactive metabolites of oxygen and nitrogen in inflammatory bowel disease. Free Radic Biol Med. (2002) 33:311–22. doi: 10.1016/S0891-5849(02)00853-5
221. Hatsugai, M, Kurokawa, MS, Kouro, T, Nagai, K, Arito, M, Masuko, K, et al. Protein profiles of peripheral blood mononuclear cells are useful for differential diagnosis of ulcerative colitis and Crohn’s disease. J Gastroenterol. (2010) 45:488–500. doi: 10.1007/s00535-009-0183-y
222. Rachmilewitz, D, Stamler, JS, Karmeli, F, Mullins, ME, Singel, DJ, Loscalzo, J, et al. Peroxynitrite-induced rat colitis—a new model of colonic inflammation. Gastroenterology. (1993) 105:1681–8. doi: 10.1016/0016-5085(93)91063-N
223. Li, X, Fang, P, Mai, J, Choi, ET, Wang, H, and Yang, X. Targeting mitochondrial reactive oxygen species as novel therapy for inflammatory diseases and cancers. J Hematol Oncol. (2013) 6:19. doi: 10.1186/1756-8722-6-19
224. Lawlor, KE, and Vince, JE. Ambiguities in NLRP3 inflammasome regulation: Is there a role for mitochondria? Biochim Biophys Acta. (2014) 1840:1433–40. doi: 10.1016/j.bbagen.2013.08.014
225. Cristani, M, Speciale, A, Saija, A, Gangemi, S, Lucia Minciullo, P, and Cimino, F. Circulating advanced oxidation protein products as oxidative stress biomarkers and progression mediators in pathological conditions related to inflammation and immune dysregulation. Curr Med Chem. (2016) 23:3862–82. doi: 10.2174/0929867323666160902154748
226. Rager, TM, Olson, JK, Zhou, Y, Wang, Y, and Besner, GE. Exosomes secreted from bone marrow-derived mesenchymal stem cells protect the intestines from experimental necrotizing enterocolitis. J Pediatr Surg. (2016) 51:942–7. doi: 10.1016/j.jpedsurg.2016.02.061
227. McCulloh, CJ, Olson, JK, Wang, Y, Zhou, Y, Tengberg, NH, Deshpande, S, et al. Treatment of experimental necrotizing enterocolitis with stem cell-derived exosomes. J Pediatr Surg. (2018) 53:1215–20. doi: 10.1016/j.jpedsurg.2018.02.086
228. Rieder, F, Fiocchi, C, and Rogler, G. Mechanisms, management, and treatment of fibrosis in patients with inflammatory bowel diseases. Gastroenterology. (2017) 152:340–50. doi: 10.1053/j.gastro.2016.09.047
229. Hayashi, Y, and Nakase, H. The molecular mechanisms of intestinal inflammation and fibrosis in Crohn’s disease. Front Physiol. (2022) 13:845078. doi: 10.3389/fphys.2022.845078
230. Frangogiannis, NG. Fibroblast—extracellular matrix interactions in tissue fibrosis. Curr Pathobiol Rep. (2016) 4:11–8. doi: 10.1007/s40139-016-0099-1
231. Lamouille, S, Xu, J, and Derynck, R. Molecular mechanisms of epithelial–mesenchymal transition. Nat Rev Mol Cell Biol. (2014) 15:178–96. doi: 10.1038/nrm3758
232. Yang, J, Zhou, C, Zhu, R, Fan, H, Liu, X, Duan, X, et al. miR-200b-containing microvesicles attenuate experimental colitis associated intestinal fibrosis by inhibiting epithelial-mesenchymal transition. J Gastroenterol Hepatol. (2017) 32:1966–74. doi: 10.1111/jgh.13797
233. Yao, Y, Chen, R, Wang, G, Zhang, Y, and Liu, F. Exosomes derived from mesenchymal stem cells reverse EMT via TGF-β1/Smad pathway and promote repair of damaged endometrium. Stem Cell Res Ther. (2019) 10:225. doi: 10.1186/s13287-019-1332-8
234. Choi, YJ, Koo, JB, Kim, HY, Seo, JW, Lee, EJ, Kim, WR, et al. Umbilical cord/placenta-derived mesenchymal stem cells inhibit fibrogenic activation in human intestinal myofibroblasts via inhibition of myocardin-related transcription factor A. Stem Cell Res Ther. (2019) 10:291. doi: 10.1186/s13287-019-1385-8
235. Duan, L, and Cao, X. Human placenta mesenchymal stem cells-derived extracellular vesicles regulate collagen deposition in intestinal mucosa of mice with colitis. Chin J Tissue Eng Res. (2021) 25:1026.
236. Pan, Y, Wu, W, Jiang, X, and Liu, Y. Mesenchymal stem cell-derived exosomes in cardiovascular and cerebrovascular diseases: from mechanisms to therapy. Biomed Pharmacother. (2023) 163:114817. doi: 10.1016/j.biopha.2023.114817
237. Sun, G, Li, G, Li, D, Huang, W, Zhang, R, Zhang, H, et al. hucMSC derived exosomes promote functional recovery in spinal cord injury mice via attenuating inflammation. Mater Sci Eng C. (2018) 89:194–204. doi: 10.1016/j.msec.2018.04.006
238. Pang, Q-M, Deng, K-Q, Zhang, M, Wu, X-C, Yang, R-L, Fu, S-P, et al. Multiple strategies enhance the efficacy of MSCs transplantation for spinal cord injury. Biomed Pharmacother. (2023) 157:114011. doi: 10.1016/j.biopha.2022.114011
239. Bao, CS, Li, XL, Liu, L, Wang, B, Yang, FB, and Chen, LG. Transplantation of human umbilical cord mesenchymal stem cells promotes functional recovery after spinal cord injury by blocking the expression of IL-7. Eur Rev Med Pharmacol Sci. (2018) 22:6436–47. doi: 10.26355/eurrev_201810_16056
240. Zhu, X, Wang, Z, Sun, YE, Liu, Y, Wu, Z, Ma, B, et al. Neuroprotective effects of human umbilical cord-derived mesenchymal stem cells from different donors on spinal cord injury in mice. Front Cell Neurosci. (2022) 15:768711. doi: 10.3389/fncel.2021.768711
241. Xiao, X, Li, W, Rong, D, Xu, Z, Zhang, Z, Ye, H, et al. Human umbilical cord mesenchymal stem cells-derived extracellular vesicles facilitate the repair of spinal cord injury via the miR-29b-3p/PTEN/Akt/mTOR axis. Cell Death Discov. (2021) 7:212. doi: 10.1038/s41420-021-00572-3
242. GBD 2016 Traumatic Brain Injury and Spinal Cord Injury Collaborators. Global, regional, and national burden of traumatic brain injury and spinal cord injury, 1990-2016: a systematic analysis for the Global Burden of Disease Study 2016. Lancet Neurol. (2019) 18:56–87. doi: 10.1016/S1474-4422(18)30415-0
243. Zhang, L, Lin, Y, Bai, W, Sun, L, and Tian, M. Human umbilical cord mesenchymal stem cell-derived exosome suppresses programmed cell death in traumatic brain injury via PINK1/Parkin-mediated mitophagy. CNS Neurosci Ther. (2023) 29:2236–58. doi: 10.1111/cns.14159
244. Xia, P, Marjan, M, Liu, Z, Zhou, W, Zhang, Q, Cheng, C, et al. Chrysophanol postconditioning attenuated cerebral ischemia-reperfusion injury induced NLRP3-related pyroptosis in a TRAF6-dependent manner. Exp Neurol. (2022) 357:114197. doi: 10.1016/j.expneurol.2022.114197
245. Nazari, S, Pourmand, SM, Motevaseli, E, and Hassanzadeh, G. Mesenchymal stem cells (MSCs) and MSC-derived exosomes in animal models of central nervous system diseases: targeting the NLRP3 inflammasome. IUBMB Life. (2023) 75:794–810. doi: 10.1002/iub.2759
246. Yang, H-C, Zhang, M, Wu, R, Zheng, H-Q, Zhang, L-Y, Luo, J, et al. CC chemokine receptor type 2-overexpressing exosomes alleviated experimental post-stroke cognitive impairment by enhancing microglia/macrophage M2 polarization. World J Stem Cells. (2020) 12:152. doi: 10.4252/wjsc.v12.i2.152
247. Zhang, Z-W, Wei, P, Zhang, G-J, Yan, J-X, Zhang, S, Liang, J, et al. Intravenous infusion of the exosomes derived from human umbilical cord mesenchymal stem cells enhance neurological recovery after traumatic brain injury via suppressing the NF-κB pathway. Open Life Sci. (2022) 17:189–201. doi: 10.1515/biol-2022-0022
248. Hofmann, R, James, SK, Jernberg, T, Lindahl, B, Erlinge, D, Witt, N, et al. Oxygen therapy in suspected acute myocardial infarction. N Engl J Med. (2017) 377:1240–9. doi: 10.1056/NEJMoa1706222
249. Diebold, S, Moellmann, J, Kahles, F, Haj-Yehia, E, Liehn, EA, Nickel, A, et al. Myocardial infarction is sufficient to increase GLP-1 secretion, leading to improved left ventricular contractility and mitochondrial respiratory capacity. Diabetes Obes Metab. (2018) 20:2911–8. doi: 10.1111/dom.13472
250. Vallabhajosyula, S, Patlolla, SH, Dunlay, SM, Prasad, A, Bell, MR, Jaffe, AS, et al. Regional variation in the management and outcomes of acute myocardial infarction with cardiogenic shock in the United States. Circ Heart Fail. (2020) 13:e006661. doi: 10.1161/CIRCHEARTFAILURE.120.007154
251. Hao, D, Swindell, HS, Ramasubramanian, L, Liu, R, Lam, KS, Farmer, DL, et al. Extracellular matrix mimicking nanofibrous scaffolds modified with mesenchymal stem cell-derived extracellular vesicles for improved vascularization. Front Bioeng Biotechnol. (2020) 8:633. doi: 10.3389/fbioe.2020.00633
252. Chen, J, Zhan, Y, Wang, Y, Han, D, Tao, B, Luo, Z, et al. Chitosan/silk fibroin modified nanofibrous patches with mesenchymal stem cells prevent heart remodeling post-myocardial infarction in rats. Acta Biomater. (2018) 80:154–68. doi: 10.1016/j.actbio.2018.09.013
253. Yang, M, Liao, M, Liu, R, Zhang, Q, Zhang, S, He, Y, et al. Human umbilical cord mesenchymal stem cell-derived extracellular vesicles loaded with miR-223 ameliorate myocardial infarction through P53/S100A9 axis. Genomics. (2022) 114:110319. doi: 10.1016/j.ygeno.2022.110319
254. Zhou, R, Gao, J, Xiang, C, Liu, Z, Zhang, Y, Zhang, J, et al. Salvianolic acid A attenuated myocardial infarction-induced apoptosis and inflammation by activating Trx. Naunyn Schmiedeberg’s Arch Pharmacol. (2020) 393:991–1002. doi: 10.1007/s00210-019-01766-4
255. Hollweck, T, Hartmann, I, Eblenkamp, M, Wintermantel, E, Reichart, B, Uberfuhr, P, et al. Cardiac differentiation of human Wharton’s jelly stem cells–experimental comparison of protocols. Open Tissue Eng Regen Med J. (2011) 4:95–102. doi: 10.2174/1875043501104010095
256. Qian, Q, Qian, H, Zhang, X, Zhu, W, Yan, Y, Ye, S, et al. 5-Azacytidine induces cardiac differentiation of human umbilical cord-derived mesenchymal stem cells by activating extracellular regulated kinase. Stem Cells Dev. (2012) 21:67–75. doi: 10.1089/scd.2010.0519
257. Zhang, C, Zhou, G, Chen, Y, Liu, S, Chen, F, Xie, L, et al. Human umbilical cord mesenchymal stem cells alleviate interstitial fibrosis and cardiac dysfunction in a dilated cardiomyopathy rat model by inhibiting TNF-α and TGF-β1/ERK1/2 signaling pathways. Mol Med Rep. (2018) 17:71–8. doi: 10.3892/mmr.2017.7882
258. Chao, C-T, Lin, Y-F, Tsai, H-B, Wu, V-C, and Ko, W-J. Acute kidney injury network staging in geriatric postoperative acute kidney injury patients: shortcomings and improvements. J Am Coll Surg. (2013) 217:240–50. doi: 10.1016/j.jamcollsurg.2013.03.024
259. Barbano, B, Sardo, L, Gigante, A, Ludovica Gasperini, M, Liberatori, M, Di Lazzaro Giraldi, G, et al. Pathophysiology, diagnosis and clinical management of hepatorenal syndrome: from classic to new drugs. Curr Vasc Pharmacol. (2014) 12:125–35. doi: 10.2174/157016111201140327163930
260. Yaghoubi, Y, Movassaghpour, A, Zamani, M, Talebi, M, Mehdizadeh, A, and Yousefi, M. Human umbilical cord mesenchymal stem cells derived-exosomes in diseases treatment. Life Sci. (2019) 233:116733. doi: 10.1016/j.lfs.2019.116733
261. Muiru, AN, Hsu, JY, Zhang, X, Appel, LJ, Chen, J, Cohen, DL, et al. Risk for chronic kidney disease progression after acute kidney injury: findings from the chronic renal insufficiency cohort study. Ann Intern Med. (2023) 176:961–8. doi: 10.7326/M22-3617
262. Al-Dhalimy, AMB, Salim, HM, Shather, AH, Naser, IH, Hizam, MM, and Alshujery, MK. The pathological and therapeutically role of mesenchymal stem cell (MSC)-derived exosome in degenerative diseases; particular focus on LncRNA and microRNA. Pathol Res Pract. (2023):154778. doi: 10.1016/j.prp.2023.154778
263. Huang, F, He, Y, Zhang, M, Luo, K, Li, J, Li, J, et al. Progress in research on stem cells in neonatal refractory diseases. J Pers Med. (2023) 13:1281. doi: 10.3390/jpm13081281
264. Wang, Y, Li, H, Li, X, Su, X, Xiao, H, and Yang, J. Hypoxic preconditioning of human umbilical cord mesenchymal stem cells is an effective strategy for treating acute lung injury. Stem Cells Dev. (2021) 30:128–34. doi: 10.1089/scd.2020.0174
265. Li, W, Zhang, Q, Wang, M, Wu, H, Mao, F, Zhang, B, et al. Macrophages are involved in the protective role of human umbilical cord-derived stromal cells in renal ischemia–reperfusion injury. Stem Cell Res. (2013) 10:405–16. doi: 10.1016/j.scr.2013.01.005
266. Peng, X, Xu, H, Zhou, Y, Wang, B, Yan, Y, Zhang, X, et al. Human umbilical cord mesenchymal stem cells attenuate cisplatin-induced acute and chronic renal injury. Exp Biol Med. (2013) 238:960–70. doi: 10.1177/1477153513497176
267. Zhou, Y, Xu, H, Xu, W, Wang, B, Wu, H, Tao, Y, et al. Exosomes released by human umbilical cord mesenchymal stem cells protect against cisplatin-induced renal oxidative stress and apoptosis in vivo and in vitro. Stem Cell Res Ther. (2013) 4:34. doi: 10.1186/scrt194
268. Huang, D, Yi, Z, He, X, Mo, S, Dang, X, and Wu, X. Distribution of infused umbilical cord mesenchymal stem cells in a rat model of renal interstitial fibrosis. Ren Fail. (2013) 35:1146–50. doi: 10.3109/0886022X.2013.815109
269. Hsu, L, Peng, B, Chen, M, Thalib, B, Ruslin, M, Tung, TDX, et al. The potential of the stem cells composite hydrogel wound dressings for promoting wound healing and skin regeneration: in vitro and in vivo evaluation. J Biomed Mater Res B. (2019) 107:278–85. doi: 10.1002/jbm.b.34118
270. Ju, Z, Ma, J, Wang, C, Yu, J, Qiao, Y, and Hei, F. Exosomes from iPSCs delivering siRNA attenuate intracellular adhesion molecule-1 expression and neutrophils adhesion in pulmonary microvascular endothelial cells. Inflammation. (2017) 40:486–96. doi: 10.1007/s10753-016-0494-0
271. Li, Q, Gong, S, Yao, W, Yang, Z, Wang, R, Yu, Z, et al. Exosome loaded genipin crosslinked hydrogel facilitates full thickness cutaneous wound healing in rat animal model. Drug Deliv. (2021) 28:884–93. doi: 10.1080/10717544.2021.1912210
272. Greenhalgh, DG. Wound healing and diabetes mellitus. Clin Plast Surg. (2003) 30:37–45. doi: 10.1016/S0094-1298(02)00066-4
273. Yan, C, Xv, Y, Lin, Z, Endo, Y, Xue, H, Hu, Y, et al. Human umbilical cord mesenchymal stem cell-derived exosomes accelerate diabetic wound healing via ameliorating oxidative stress and promoting angiogenesis. Front Bioeng Biotechnol. (2022) 10:829868. doi: 10.3389/fbioe.2022.829868
274. Conese, M, and Portincasa, A. Mesenchymal stem cells, secretome and biomaterials in in-vivo animal models: regenerative medicine application in cutaneous wound healing. Biocell. (2022) 46:1815–26. doi: 10.32604/biocell.2022.019448
275. Jiang, Y, and Huang, Z. Ulinastatin alleviates pulmonary edema by reducing pulmonary permeability and stimulating alveolar fluid clearance in a rat model of acute lung injury. Iran J Basic Med Sci. (2022) 25:1002. doi: 10.22038/IJBMS.2022.64655.14230
276. McIntyre, LA, Moher, D, Fergusson, DA, Sullivan, KJ, Mei, SHJ, Lalu, M, et al. Efficacy of mesenchymal stromal cell therapy for acute lung injury in preclinical animal models: a systematic review. PLoS One. (2016) 11:e0147170. doi: 10.1371/journal.pone.0147170
277. Chen, J, Li, C, Liang, Z, Li, C, Li, Y, Zhao, Z, et al. Human mesenchymal stromal cells small extracellular vesicles attenuate sepsis-induced acute lung injury in a mouse model: the role of oxidative stress and the mitogen-activated protein kinase/nuclear factor kappa B pathway. Cytotherapy. (2021) 23:918–30. doi: 10.1016/j.jcyt.2021.05.009
278. Chen, H, Cai, Y, Sun, S, Pan, Z, Han, Z, Liu, P, et al. Repair effect of photobiomodulation combined with human umbilical cord mesenchymal stem cells on rats with acute lung injury. J Photochem Photobiol B. (2022) 234:112541. doi: 10.1016/j.jphotobiol.2022.112541
279. Levy, PT, Levin, J, Leeman, KT, Mullen, MP, Hansmann, G, and Kourembanas, S. Diagnosis and management of pulmonary hypertension in infants with bronchopulmonary dysplasia. Semin Fetal Neonatal Med. (2022):101351. doi: 10.1016/j.siny.2022.101351
280. Ai, D, Shen, J, Sun, J, Zhu, Z, Gao, R, Du, Y, et al. Mesenchymal stem cell-derived extracellular vesicles suppress hyperoxia-induced transdifferentiation of rat alveolar type 2 epithelial cells. Stem Cells Dev. (2022) 31:53–66. doi: 10.1089/scd.2021.0256
281. You, J, Zhou, O, Liu, J, Zou, W, Zhang, L, Tian, D, et al. Human umbilical cord mesenchymal stem cell-derived small extracellular vesicles alleviate lung injury in rat model of bronchopulmonary dysplasia by affecting cell survival and angiogenesis. Stem Cells Dev. (2020) 29:1520–32. doi: 10.1089/scd.2020.0156
282. Guo, Z, Zhang, Y, and Yan, F. Potential of mesenchymal stem cell-based therapies for pulmonary fibrosis. DNA Cell Biol. (2022) 41:951–65. doi: 10.1089/dna.2022.0327
283. Ahani-Nahayati, M, Niazi, V, Moradi, A, Pourjabbar, B, Roozafzoon, R, Keshel, SH, et al. Umbilical cord mesenchymal stem/stromal cells potential to treat organ disorders; an emerging strategy. Curr Stem Cell Res Ther. (2022) 17:126–46. doi: 10.2174/1574888X16666210907164046
284. Tang, Z, Gao, J, Wu, J, Zeng, G, Liao, Y, Song, Z, et al. Human umbilical cord mesenchymal stromal cells attenuate pulmonary fibrosis via regulatory T cell through interaction with macrophage. Stem Cell Res Ther. (2021) 12:397. doi: 10.1186/s13287-021-02469-5
285. Liu, J, Xing, F, Fu, Q, He, B, Jia, Z, Du, J, et al. hUC-MSCs exosomal miR-451 alleviated acute lung injury by modulating macrophage M2 polarization via regulating MIF-PI3K-AKT signaling pathway. Environ Toxicol. (2022) 37:2819–31. doi: 10.1002/tox.23639
286. Wei, X, Yi, X, Lv, H, Sui, X, Lu, P, Li, L, et al. MicroRNA-377-3p released by mesenchymal stem cell exosomes ameliorates lipopolysaccharide-induced acute lung injury by targeting RPTOR to induce autophagy. Cell Death Dis. (2020) 11:657. doi: 10.1038/s41419-020-02857-4
287. Wu, C-T, Deng, J-S, Huang, W-C, Shieh, P-C, Chung, M-I, and Huang, G-J. Salvianolic acid C against acetaminophen-induced acute liver injury by attenuating inflammation, oxidative stress, and apoptosis through inhibition of the Keap 1/Nrf2/HO-1 signaling. Oxid Med Cell Longev. (2019) 2019:9056845. doi: 10.1155/2019/9056845
288. Lin, M, Li, S, Yang, L, Ye, D, Xu, L, Zhang, X, et al. Plasma membrane vesicles of human umbilical cord mesenchymal stem cells ameliorate acetaminophen-induced damage in HepG2 cells: a novel stem cell therapy. Stem Cell Res Ther. (2020) 11:225. doi: 10.1186/s13287-020-01738-z
289. Hua, D, Ju, Z, Gan, X, Wang, Q, Luo, C, Gu, J, et al. Human amniotic mesenchymal stromal cells alleviate acute liver injury by inhibiting the pro-inflammatory response of liver resident macrophage through autophagy. Ann Transl Med. (2019) 7:392. doi: 10.21037/atm.2019.08.83
290. Liu, Z, Meng, F, Li, C, Zhou, X, Zeng, X, He, Y, et al. Human umbilical cord mesenchymal stromal cells rescue mice from acetaminophen-induced acute liver failure. Cytotherapy. (2014) 16:1207–19. doi: 10.1016/j.jcyt.2014.05.018
291. Guo, G, Tan, Z, Liu, Y, Shi, F, and She, J. The therapeutic potential of stem cell-derived exosomes in the ulcerative colitis and colorectal cancer. Stem Cell Res Ther. (2022) 13:138. doi: 10.1186/s13287-022-02811-5
292. Chen, P, Yao, L, Yuan, M, Wang, Z, Zhang, Q, Jiang, Y, et al. Mitochondrial dysfunction: a promising therapeutic target for liver diseases. Genes Dis. (2023) 11:101115. doi: 10.1016/j.gendis.2023.101115
293. Wu, H-Y, Zhang, X-C, Jia, B-B, Cao, Y, Yan, K, Li, J-Y, et al. Exosomes derived from human umbilical cord mesenchymal stem cells alleviate acetaminophen-induced acute liver failure through activating ERK and IGF-1R/PI3K/AKT signaling pathway. J Pharmacol Sci. (2021) 147:143–55. doi: 10.1016/j.jphs.2021.06.008
294. Jiang, W, Tan, Y, Cai, M, Zhao, T, Mao, F, Zhang, X, et al. Human umbilical cord MSC-derived exosomes suppress the development of CCl4-induced liver injury through antioxidant effect. Stem Cells Int. (2018) 2018:6079642. doi: 10.1155/2018/6079642
295. Che, J, Wang, H, Dong, J, Wu, Y, Zhang, H, Fu, L, et al. Human umbilical cord mesenchymal stem cell-derived exosomes attenuate neuroinflammation and oxidative stress through the NRF2/NF-κB/NLRP3 pathway. CNS Neurosci Ther. (2024) 30:e14454. doi: 10.1111/cns.14454
296. Jiang, L, Zhang, S, Hu, H, Yang, J, Wang, X, Ma, Y, et al. Exosomes derived from human umbilical cord mesenchymal stem cells alleviate acute liver failure by reducing the activity of the NLRP3 inflammasome in macrophages. Biochem Biophys Res Commun. (2019) 508:735–41. doi: 10.1016/j.bbrc.2018.11.189
297. Hoque, R, Farooq, A, Ghani, A, Gorelick, F, and Mehal, WZ. Lactate reduces liver and pancreatic injury in toll-like receptor-and inflammasome-mediated inflammation via GPR81-mediated suppression of innate immunity. Gastroenterology. (2014) 146:1763–74. doi: 10.1053/j.gastro.2014.03.014
298. Wang, Z, Yao, L, Hu, X, Yuan, M, Chen, P, Liu, P, et al. Advancements in mesenchymal stem cell therapy for liver cirrhosis: unveiling origins, treatment mechanisms, and current research frontiers. Tissue Cell. (2023) 84:102198. doi: 10.1016/j.tice.2023.102198
299. Zhang, S, Jiang, L, Hu, H, Wang, H, Wang, X, Jiang, J, et al. Pretreatment of exosomes derived from hUCMSCs with TNF-α ameliorates acute liver failure by inhibiting the activation of NLRP3 in macrophage. Life Sci. (2020) 246:117401. doi: 10.1016/j.lfs.2020.117401
300. He, L-Y, Li, Y, Niu, S-Q, Bai, J, Liu, S-J, and Guo, J-L. Polysaccharides from natural resource: ameliorate type 2 diabetes mellitus via regulation of oxidative stress network. Front Pharmacol. (2023) 14:1184572. doi: 10.3389/fphar.2023.1184572
301. He, X, Ou, C, Xiao, Y, Han, Q, Li, H, and Zhou, S. LncRNAs: key players and novel insights into diabetes mellitus. Oncotarget. (2017) 8:71325. doi: 10.18632/oncotarget.19921
302. Li, X, Liu, R, Chen, Y, Han, Y, Wang, Q, Xu, Y, et al. Patterns and trends in mortality associated with and due to diabetes mellitus in a transitioning region with 3.17 million people: observational study. JMIR Public Health Surveill. (2023) 9:e43687. doi: 10.2196/43687
303. Suh, S-H, Paik, I-Y, and Jacobs, KA. Regulation of blood glucose homeostasis during prolonged exercise. Mol Cells. (2007) 23:272–9. doi: 10.1016/S1016-8478(23)10717-5
304. Yang, M, Chen, J, and Chen, L. The roles of mesenchymal stem cell-derived exosomes in diabetes mellitus and its related complications. Front Endocrinol. (2022) 13:1027686. doi: 10.3389/fendo.2022.1027686
305. Sun, Y, Shi, H, Yin, S, Ji, C, Zhang, X, Zhang, B, et al. Human mesenchymal stem cell derived exosomes alleviate type 2 diabetes mellitus by reversing peripheral insulin resistance and relieving β-cell destruction. ACS Nano. (2018) 12:7613–28. doi: 10.1021/acsnano.7b07643
306. Lee, B-C, Kang, I, and Yu, K-R. Therapeutic features and updated clinical trials of mesenchymal stem cell (MSC)-derived exosomes. J Clin Med. (2021) 10:711. doi: 10.3390/jcm10040711
307. Marcheque, J, Bussolati, B, Csete, M, and Perin, L. Concise reviews: stem cells and kidney regeneration: an update. Stem Cells Transl Med. (2019) 8:82–92. doi: 10.1002/sctm.18-0115
308. Bruno, S, Kholia, S, Deregibus, MC, and Camussi, G. The role of extracellular vesicles as paracrine effectors in stem cell-based therapies. Adv Exp Med Biol. (2019) 1201:175–93. doi: 10.1007/978-3-030-31206-0_9
309. Sun, LI, Xu, R, Sun, X, Duan, Y, Han, Y, Zhao, Y, et al. Safety evaluation of exosomes derived from human umbilical cord mesenchymal stromal cell. Cytotherapy. (2016) 18:413–22. doi: 10.1016/j.jcyt.2015.11.018
310. Nazari, H, Alborzi, F, Heirani-Tabasi, A, Hadizadeh, A, Asbagh, RA, Behboudi, B, et al. Evaluating the safety and efficacy of mesenchymal stem cell-derived exosomes for treatment of refractory perianal fistula in IBD patients: clinical trial phase I. Gastroenterol Rep. (2022) 10:goac075. doi: 10.1093/gastro/goac075
311. Xie, Y, Liu, W, Liu, S, Wang, L, Mu, D, Cui, Y, et al. The quality evaluation system establishment of mesenchymal stromal cells for cell-based therapy products. Stem Cell Res Ther. (2020) 11:176. doi: 10.1186/s13287-020-01696-6
312. Agrahari, V, Agrahari, V, Burnouf, P-A, Chew, CH, and Burnouf, T. Extracellular microvesicles as new industrial therapeutic frontiers. Trends Biotechnol. (2019) 37:707–29. doi: 10.1016/j.tibtech.2018.11.012
313. Liguori, GL, and Kisslinger, A. Standardization and reproducibility in EV research: the support of a Quality Management System. Adv Biomembr Lipid Self-Assem. (2021) 33:175–206. doi: 10.1016/bs.abl.2020.05.005
314. Grangier, A, Branchu, J, Volatron, J, Piffoux, M, Gazeau, F, Wilhelm, C, et al. Technological advances towards extracellular vesicles mass production. Adv Drug Deliv Rev. (2021) 176:113843. doi: 10.1016/j.addr.2021.113843
315. Giancaterino, S, and Boi, C. Alternative biological sources for extracellular vesicles production and purification strategies for process scale-up. Biotechnol Adv. (2023) 63:108092. doi: 10.1016/j.biotechadv.2022.108092
316. Ghanem, B, Seoane-Vazquez, E, Brown, L, and Rodriguez-Monguio, R. Analysis of the gene therapies authorized by the United States Food and Drug Administration and the European Medicines Agency. Med Care. (2023) 61:438–47. doi: 10.1097/MLR.0000000000001840
Keywords: inflammatory bowel disease, human umbilical mesenchymal stem cells, exosomes, genes, extracellular vesicles
Citation: Din MAU, Wan A, Chu Y, Zhou J, Yan Y and Xu Z (2024) Therapeutic role of extracellular vesicles from human umbilical cord mesenchymal stem cells and their wide therapeutic implications in inflammatory bowel disease and other inflammatory disorder. Front. Med. 11:1406547. doi: 10.3389/fmed.2024.1406547
Received: 25 March 2024; Accepted: 18 July 2024;
Published: 30 July 2024.
Edited by:
Willem Fibbe, Leiden University Medical Center (LUMC), NetherlandsReviewed by:
Eiji Kawamoto, Mie University, JapanCopyright © 2024 Din, Wan, Chu, Zhou, Yan and Xu. This is an open-access article distributed under the terms of the Creative Commons Attribution License (CC BY). The use, distribution or reproduction in other forums is permitted, provided the original author(s) and the copyright owner(s) are credited and that the original publication in this journal is cited, in accordance with accepted academic practice. No use, distribution or reproduction is permitted which does not comply with these terms.
*Correspondence: Yongmin Yan, eXltQHdqcm15eS5jbg==; Zhiliang Xu, Y2xpbmxhYkB3anJteXkuY24=
†These authors have contributed equally to this work
Disclaimer: All claims expressed in this article are solely those of the authors and do not necessarily represent those of their affiliated organizations, or those of the publisher, the editors and the reviewers. Any product that may be evaluated in this article or claim that may be made by its manufacturer is not guaranteed or endorsed by the publisher.
Research integrity at Frontiers
Learn more about the work of our research integrity team to safeguard the quality of each article we publish.