- 1Luzhou Longmatan District People's Hospital, Luzhou, China
- 2Department of Orthopedics, The Affiliated Hospital of Southwest Medical University, Luzhou, China
- 3Sichuan Provincial Laboratory of Orthopedic Engineering, Luzhou, China
- 4Department of Clinical Medicine, Southwest Medical University, Luzhou, China
Patients with Osteoarthritis (OA) often also suffer from Sleep Apnea Syndrome (SAS), and many scholars have started to notice this link, although the relationship between the two is still unclear. In this review, we aim to summarize the current literature on these two diseases, integrate evidence of the OA and OSA connection, explore and discuss their potential common mechanisms, and thus identify effective treatment methods for patients with both OA and SAS. Some shared characteristics of the two conditions have been identified, notably aging and obesity as mutual risk factors. Both diseases are associated with various biological processes or molecular pathways, including mitochondrial dysfunction, reactive oxygen species production, the NF-kB pathway, HIF, IL-6, and IL-8. SAS serves as a risk factor for OA, and conversely, OA may influence the progression of SAS. The effects of OA on SAS are underreported in the literature and require more investigation. To effectively manage these patients, timely intervention for SAS is necessary while treating OA, with weight reduction being a primary requirement, alongside combined treatments such as Continuous positive airway pressure (CPAP) and medications. Additionally, numerous studies in drug development are now aimed at inhibiting or clearing certain molecular pathways, including ROS, NF-KB, IL-6, and IL-8. Improving mitochondrial function might represent a viable new strategy, with further research into mitochondrial updates or transplants being essential.
Background
Sleep apnea syndrome (SAS) is a breathing-related disorder during sleep, where breathing frequently pauses or becomes significantly shallow. These pause events may last 10 s or longer and occur more than 5 times per hour. Recent studies show that approximately half of the global population is affected by SAS (1). Research investigating the Asian obese population has discovered a SAS prevalence rate of up to 80.5% (2). Osteoarthritis (OA), a chronic degenerative disease occurring in the joints, has been seeing a rise in prevalence with the aging of the population.
A close relationship exists between SAS and OA. In a cross-sectional study, Asiye Kanbay et al. (3) identified SAS as a risk factor for OA. Furthermore, Andressa Silva et al. (4) observed that patients diagnosed with both OA and SAS exhibited poorer knee joint function than those without SAS. Present evidence suggests the importance of focusing on the connection between OA and SAS. Hence, this review aims to summarize the shared aspects between the two, providing directions for further research into their comorbid issues.
Method
Searches were performed in PubMed and Google Scholar using keywords like “sleep apnea syndrome,” “osteoarthritis,” “obstructive sleep apnea,” and terms such as “arthritis,” “arthropathy,” “joint pain.” By continuously recombining search terms and filtering through titles and abstracts, we identified relevant documents concerning the relationship between SAS and OA. Using the snowballing method, we summarized the content of retrieved literature and identified additional search terms related to OA and SAS, which facilitated further searches. Inclusion criteria included that the full text be in English and published in this century. Excluded were those studies that did not meet the objectives of this review and were unrelated to the topic.
Common risk factors for SAS and OA
Obesity
Obesity is a risk factor for SAS. Compared to individuals of normal weight, those who are obese or overweight are significantly more likely to suffer from SAS, and they tend to experience a higher severity of the condition. As the Body Mass Index (BMI) and the circumference of the neck and waist increase, the incidence of SAS escalates, and sleep quality deteriorates significantly (5–7).
Bariatric surgery demonstrates remarkable outcomes in treating obstructive sleep apnea in obese patients, particularly in cases of morbid obesity. These surgeries are effective in not only reducing apnea-hypopnea events but also in decreasing body weight, and improving oxygen saturation, deep N3 sleep, and REM (Rapid Eye Movement) sleep stages. Moreover, the surgery improves pulmonary function by increasing Forced Vital Capacity (FVC) (8). Additionally, research shows that managing obesity-related body metrics through pharmacological intervention can reduce symptoms of sleep apnea (9). Wyszomirski et al. (10) discovered that sleep apnea can exacerbate obesity. However, research by Caliendo et al. (11) suggests there may not be a direct correlation between obesity and sleep apnea, yet a positive relationship exists between BMI and the severity of AHI. In summary, obesity is a risk factor for SAS, and managing obesity can play a key role in the prevention and control of sleep apnea.
Obesity is closely related to OA. Research shows that both overall and abdominal obesity elevate the risk of knee OA, with the risk peaking when these two types of obesity are present simultaneously (12). In South Korea’s aged population, obesity significantly raises the risk of OA (13). Research in Saudi Arabia has discovered a close relationship between obesity and bilateral knee OA (14). Within an OA animal model, researchers induced obesity in mice with a high-fat diet, thereby speeding up the mice’s OA progression (15). Further research indicates a causal link between obesity and hip OA (16). Interestingly, there is an association between metabolic syndrome and hip OA in women, but in men, this link is not evident. However, for men, obesity is directly associated with hip OA, yet in women, this association is less clear. The mechanical impact of obesity may be the main mechanism behind hip OA in men, while in women, metabolic actions are believed to take precedence (17). Not only do obesity and metabolic syndrome foster knee OA through increased mechanical loading, but they also do so via systemic inflammation caused by obesity (18). Moreover, obesity could intensify OA pain, with class III obese patients suffering from notably greater pain than those who are overweight or have class I or II obesity (19).
OA may in turn worsen obesity. Research indicates a higher incidence of obesity, sarcopenia, and sarcopenic obesity in patients with end-stage knee OA. Patients with bilateral knee OA exhibit a higher rate of sarcopenic obesity compared to those with unilateral knee OA (20), underscoring the significance of focusing on the interplay between obesity and OA. Interestingly, bariatric surgery can alleviate symptoms of OA and even potentially slow its progression (21).
Age
Age has an impact on SAS. With advancing age, the severity of SAS tends to intensify, particularly among men (22). For women, transitioning into menopause significantly raises the risk of developing SAS (23). The prevalence of SAS in elderly women is on the rise, with this increase possibly due to aging causing a decrease in the reactivity of upper airway muscle activity, thereby making the airway more prone to collapse (24).
SAS may accelerate the aging process, acting as a contributing factor to aging-related conditions (25). SAS is associated with aging characteristics such as metabolic syndrome, vascular dysfunction, decline in quality of life and cognitive scores, accelerated brain aging, increased insulin resistance, and elevated levels of plasma hydrogen peroxide, GSH, IL-6, hsCRP, and leptin (26). For those under 50, sleep apnea patients are particularly susceptible to aging-related mechanisms such as altered cell communication, impaired nutrient sensing, telomere shortening, mitochondrial dysfunction, and genomic instability (26). SAS can affect the cognition of the elderly. Research indicates that obstructive sleep apnea is linked to a decline in cognitive abilities, particularly in attention and processing speed, among middle-aged and older people (27, 28). This could be attributed to minor alterations in the local brain structures of individuals with SAS (29).
Age significantly impacts OA. Research analyzing data from 36 Organization for Economic Cooperation and Development countries (OECD) countries identified aging and obesity as critical risk factors for OA (30). Both obesity and age are closely related to the severity of knee OA, with age being an independent risk factor for its severity (31). In studies on aged female mice, increased knee cartilage degeneration was observed, alongside more severe mechanical allodynia, knee joint hyperalgesia, and decreased grip strength (32). Di et al. (33) observed that the incidence of knee OA increased most significantly in the age groups of 35 to 39 and 40 to 44 years. A meta-analysis highlighted age as a risk factor for scapulothoracic OA (34). Furthermore, age also influences the hand strength in patients with hand OA (35).
Common mechanism of SAS and OA
Mitochondrial dysfunction
Mitochondrial dysfunction plays a crucial role in the context of SAS. A diet high in fats causes mitochondrial dysfunction and oxidative stress, which damages the genioglossus muscle and could lead to SAS (36). Moreover, research has shown that in the whole blood DNA of patients with SAS, the copy number of mitochondrial DNA (mtDNA) is reduced, with this reduction being associated with the severity of the patient’s condition (37). However, Lacedonia D et al. found that: in patients, intermittent hypoxia intensifies oxidative stress, resulting in mitochondrial damage, and consequently, a increase in the number of mitochondrial DNA copies (38).
Chronic intermittent hypoxia in patients can lead to mitochondrial dysfunction, triggering neuroinflammation, an increase in reactive oxygen species (ROS) (39). Furthermore, mitochondrial dysfunction is associated with metabolic disorders, and the level of mitochondrial-derived peptide MOTS-c in serum is closely related to SAS. MOTS-c emerges as a new and useful marker for early metabolic disorder in patients (40). Interestingly, enhancing mitochondrial autophagy to remove damaged mitochondria can alleviate tissue cell damage caused by the syndrome. Research has found that silencing NLRP3 in mice activates the PINK1-Parkin pathway to promote mitochondrial autophagy, thereby preventing the neuroinflammation and production of reactive oxygen species induced by intermittent hypoxia (41).
Mitochondrial dysfunction is indeed a significant factor contributing to the development of OA. Cytokine stimulation triggers mitochondrial dysfunction through signaling pathways such as JNK, PI3K/Akt, NF-κB, and p38 MAPK, which is characterized by decreased transmembrane potential, reduced ATP production, and increased ROS, all of which contribute to cartilage cell damage (42). The ensuing ROS chain reaction caused by mitochondrial dysfunction damages mitochondrial DNA (mtDNA) and cardiolipin, leading to a decrease in mitochondrial membrane potential and the opening of mitochondrial permeability transition pores (PTP). This facilitates the influx of calcium ions (Ca2+) and thus triggers an inflammatory response. During this process, the inflammasome NLRP3 is activated, enabling the conversion from pro-Caspase-1 to Caspase-1 and initiating a caspase cascade, ultimately leading to pyroptosis. Moreover, ROS can further activate NF-κB, resulting in changes in gene transcription that lead to cell cycle arrest, cellular senescence, and degradation of the extracellular matrix (43).
Reactive oxygen species (ROS)
Intermittent hypoxia caused by SAS leads to the activation of NADPH oxidase and xanthine oxidase, mitochondrial dysfunction, and uncoupling of nitric oxide synthase (NOS) (44, 45). These reactions result in increased ROS levels, further activating transcription factors such as NF-kB and AP-1, which then trigger the release of inflammatory mediators and pro-inflammatory cytokines (45, 46), ultimately causing tissue and cellular damage (Table 1).
Additionally, intermittent hypoxic conditions during apnea lead to an increase in mtROS and activation of NLRP3 inflammasomes, these inflammatory reactions may cause damage to other organs (41). Hypoxia-induced increase in ROS also promotes cell apoptosis through the activation of the NF-κB/HIF-1α signaling pathway (47).
ROS plays a crucial role in the development mechanism of OA (Table 1). Research indicates that synovitis-induced activation of the ROS/NLRP3 pathway can enhance chondrocyte apoptosis, thereby speeding up the degeneration of joint cartilage in OA (48). Efficiently clearing ROS can alleviate the symptoms of OA. For instance, nitidine chloride, acting as a reactive oxygen species scavenger, can reduce inflammatory responses and cell senescence in a mouse model of OA (49). Moreover, mitochondria-targeted Mn(3)O (4)/UIO-TPP nanozymes restore mitochondrial function by eliminating ROS, thus effectively combating OA (50). Molybdenum-based polyoxometalate (POM) nanoclusters leverage their near-infrared photosensitivity to enhance ROS scavenging capability, markedly improving clinical symptoms of OA (63). Additionally, Nodakenin can mitigate cartilage degeneration and inflammatory responses in a mouse model of OA by modulating the mitochondrial Drp1/ROS/NLRP3 axis (53).
The ROS/NLRP3 pathway also impedes M1 macrophage polarization, leading to OA. By inhibiting the ROS/NLRP3 pathway, the progression of OA can be delayed (54). Researchers have created a nanomaterial NP@Poly(RHAPM) that is capable of significantly lowering ROS levels within cells, thereby leading to the repolarization of M1 macrophages to the M2 phenotype, enhancing the proliferation and vitality of chondrocytes, as well as preventing cell apoptosis (55). Similarly, BTZ@PTK reduces ROS levels, activates apoptosis in M1 macrophages, inhibits M1 macrophage-mediated inflammatory responses, and thus improves OA (64).
ROS regulate the NF-κB and MAPK pathways, thus contributing to OA. The Pt@PCN222-Mn cascaded nanoenzyme, developed by Zhang et al. (57), can delay the progression of temporomandibular joint OA in rat models by inhibiting the ROS-NF-κB and MAPK signaling pathways. Moreover, Dendrobine targets chondrocytes via the ROS/NF-κB axis, inhibiting the expression of aging-related secretory phenotype factors, thus helping to alleviate OA (58). Additionally, Astragalus polysaccharides inhibit apoptosis and improve OA symptoms by suppressing ROS-mediated activation of the ASK1/p38 MAPK signaling pathway, subsequently activating thioredoxins (59).
NF-κB pathway
Chronic hypoxia mediated by SAS can cause damage to multiple organ tissues throughout the body via the NF-κB pathway (Table 2). Hypoxia-induced ROS promotes myoblast apoptosis in obstructive sleep apnea via the NF-κB/HIF-1α signaling pathway (47). Additionally, hypoxia accelerates lung fibrosis in mouse lung injury by regulating the NF-κB/Nrf2 signaling pathway (65). Hypoxia further mediates adipocyte insulin resistance through the RAGE/NF-κB pathway (66). The activation of the TLR4/NF-κB/VEGF pathway enhances vascular dysfunction in the soft palate, aggravating SAS (67).
Intervening in the NF-κB pathway offers a therapeutic avenue for neurological and cardiovascular diseases caused by SAS. Liu et al. (68) demonstrated that inhibiting NF-κB activation can alleviate cognitive impairment in sleep apnea. Similarly, PDTC and Rapa, by inhibiting the mTOR and NF-κB pathways, can prevent damage to hippocampal neurons induced by hypoxia (69). Song et al. (99) showed that inhibiting endothelial NF-κB signaling can alleviate arteriosclerosis induced by chronic intermittent hypoxia in mice. Furthermore, enhanced O-GlcNAcylation can inhibit NFAT and NF-κB activity in mice to attenuate cardiac remodeling induced by intermittent hypoxia (70). Also, overexpressing miR-15b-5p/miR-92b-3p and inhibiting the PTGS1-NF-κB-SP1 signaling pathway provides a potential treatment for depression related to SAS (71).
There’s a significant link between the NF-κB pathway and OA (Table 2); suppressing NF-κB can significantly mitigate OA. Pelagonidin effectively improves OA by inhibiting the NF-κB pathway, thus reducing inflammation and cartilage degeneration (76). Exosomal miR-4738-3p helps relieve mild inflammatory symptoms of OA by regulating COL1A2 through the NF-kB and inflammatory signaling pathways (78). Atractylenolide III effectively alleviates OA and chondrocyte aging by inhibiting the NF-κB signaling pathway (100). Shihuakalin targets cellular aging and OA via the ROS/NF-κB pathway (58). Quercitrin acts to slow down OA by inhibiting the NF-κB signaling pathway and enhancing glucose transport capability (101). Indole-3-propionic acid offers relief to chondrocyte inflammation and OA through the AhR/NF-κB axis (72).
Furthermore, NF-κB is known to promote chondrocyte apoptosis, and inhibiting NF-κB can effectively protect chondrocytes. Paroxetine has been shown to alleviate chondrocyte apoptosis and inhibit osteoclast formation by suppressing NF-κB (85). Stevioside reduces chondrocyte inflammation and apoptosis in vivo via the NF-κB and MAPK pathways, thereby improving OA (86). Forkhead box O3 plays a crucial role in inhibiting chondrocyte ferroptosis and alleviating OA by suppressing the NF-κB/MAPK signaling (87).
NF-κB plays a pivotal role in OA through its influence on macrophages. Suramin effectively ameliorates OA by targeting the Nrf2/HO-1 and NF-κB signaling pathways in chondrocytes and by promoting the M2 polarization of macrophages (88). Additionally, κ-opioid receptor activation can modulate macrophage polarization through the NF-κB pathway and help alleviate osteoarthritic synovitis (89).
Some miRNAs significantly influence OA through the NF-κB pathway. The MicroRNA-15a/β1, 4-galt-i axis is involved in the degeneration of osteoarthritic cartilage via the NF-κB signaling pathway (102). MiR-203a-3p plays a role in alleviating chondrocyte apoptosis by regulating the MYD88/NF-κB pathway (91).
Physical factors also impact OA via the NF-κB pathway. Mechanical stress works to avert chondrocyte apoptosis by suppressing the NF-κB signaling pathway (92). Non-weight-bearing exercise has been shown to reduce rat knee OA through the TLR4/MyD88/NF-κB signaling pathway (93). Tendon adjustments and bone grafting are effective in facilitating the healing of synovitis in rabbit osteoarthritic knees via the TLR4-MyD88-NF-κB pathway (94). Moderate-intensity physical activity can mitigate inflammation and cellular apoptosis by enhancing metrnl release, thereby acting to suppress the PI3K/Akt/NF-κB and NLRP3/caspase-1/GSDMD pathways (95).
Upregulating Nrf2 to inhibit NF-κB activity offers a promising approach to alleviate OA. Li et al. (103) found that the Nrf2/HMGB1/NF-κB axis regulates chondrocyte apoptosis and extracellular matrix degradation in OA. Phillygenin improves OA in mice by inhibiting chondrocyte inflammation via the Nrf2/NF-κB axis (73). Oxymatrine enhances OA treatment in vitro and in vivo via the Nrf2/NF-κB axis (79). Chrysophanol acts to prevent OA inflammation and extracellular matrix degradation via the Nrf2/NF-κB axis (80).
The Sirtuin 6/NF-κB signaling pathway holds significance in OA treatment. Orientin effectively mitigates OA through activation of the SIRT6 signaling pathway and suppression of the NF-κB pathway (74). Pachymic acid targets chondrocyte inflammation by modulating the Sirtuin 6/NF-κB signaling axis (75). Emodin again demonstrates its efficacy in preventing OA inflammation and extracellular matrix degradation in chondrocytes via the Sirt6/NF-κB pathway (80). Ergothioneine is proven to inhibit the progression of OA both in vitro and in vivo via the Sirt6/NF-κB axis (83).
The PI3K/AKT/NF-κB and MAPK signaling pathways are closely associated with the onset and progression of OA. Sakuranetin acts to reduce inflammation and chondrocyte dysfunction in OA by targeting the PI3K/AKT/NF-κB pathway (77). Rutaecarpine demonstrates effectiveness in improving OA by inhibiting PI3K/AKT/NF-κB and MAPK signal transduction through integrin αVβ3 (81). Mulberroside A contributes to alleviating OA by restoring damaged autophagy and inhibiting the MAPK/NF-κB/PI3K-AKT–mTOR signaling pathway (82). Furthermore, Plantamajoside beneficially impacts the development of OA by suppressing the activation of NF-κB and MAPK (84).
HIF (hypoxia-inducible factors)
HIF-1α is recognized as a biomarker for SAS (104). In patients with sleep apnea, the HIF-1α protein expression levels are notably elevated, correlating with the chronic hypoxia state (105). This chronic hypoxia not only leads to other diseases, through HIF-1 (106) but also results in an increase in ROS, which further promotes tissue cells apoptosis via the NF-κB/HIF-1α signaling pathway (47). Moreover, elevated HIF-1α levels are tied to excessive expression of circadian rhythm proteins, thereby increasing the risk of circadian rhythm disturbances in SAS patients (107). SAS may harm other tissues and organs via HIF-1, SAS can accelerate the progression of aortic dissection through the ROS-HIF-1α-MMPs related pathway (108), as well as exacerbate neuroinflammation and apoptosis in early brain injury after subarachnoid hemorrhage via the ASC/HIF-1α pathway (109).
HIF-2α is a subtype of the HIF. HIF-2α increases the expression of Superoxide Dismutase 2 (SOD2), an antioxidant enzyme that can reduce ROS (110). Chronic hypoxia from SAS causes an increase in ROS, subsequently triggering a rise in intracellular calcium levels ([Ca(2+)]i), which stimulates increased synthesis of HIF-1α and enhanced degradation of HIF-2α, as a result, the normal balance between HIF-1α-dependent pro-oxidants and HIF-2α-dependent antioxidants is broken, resulting in an additional increase in ROS, this exacerbates damage to multiple tissues throughout the body (111).
Some studies indicate that HIF-1 plays a protective role in OA (Table 3). HIF-1α may protect articular cartilage by promoting chondrocyte phenotype, maintaining chondrocyte vitality, and supporting metabolic adaptation in hypoxic conditions (128). In hypoxic conditions, the activity of HIF-1α is enhanced, and it accelerates angiogenesis in cartilage through the vascular endothelial growth factor VEGF and Notch signaling pathway (112). Sunli Hu et al. (129) found that HIF-1α-mediated mitochondrial autophagy can alleviate OA. The dysregulation of the HIF-1α/CRAT/miR-144-3p signaling axis has a significant relationship with OA; silencing HIF-1α leads to downregulation of CRAT, which then results in increased expression of miR-144-3p causing dysfunction of peroxisomes and accumulation of long-chain fatty acids, promoting the development of OA (118).
Nonetheless, certain studies refute the protective role of HIF-1 in OA. For instance, in knee OA, elevated HIF-1α may exacerbate synovial fibrosis and fibroblast-like synoviocyte apoptosis (130). Moreover, LncHIFCAR has been shown to positively regulate HIF-1α and its target genes (including VEGF, BNIP3) along with the PI3K/AKT/mTOR pathway, thereby promoting chondrocyte apoptosis (114). Interestingly, Li et al. (115) discovered that Casticin mitigates knee OA through the suppression of HIF-1α/NLRP3 inflammasome signaling. In a similar vein, Agnuside alleviates synovitis and fibrosis in experimental knee OA by inhibiting the accumulation of HIF-1α and the activation of the NLRP3 inflammasome (116). Additionally, Vitexin targets the HIF-1α pathway to reduce inflammatory responses in osteoarthritic chondrocytes (117). Likewise, CircRNA-UBE2G1 modulates chondrocyte damage via the miR-373/HIF-1α pathway (119). Finally, Capsiate metabolites are found to reduce the progression of ferroptosis-associated OA by enhancing SLC2A1 expression, thereby effectively inhibiting HIF-1α expression (120).
HIF-2α is recognized as a crucial regulator of catabolic metabolism and the inflammatory cascade in OA (131). It can directly induce the expression of catabolic metabolic factors in chondrocytes and also enhance the expression of Fas in mature chondrocytes, thus mediating chondrocyte apoptosis and autophagy regulation (128). Moreover, mechanical stress has been found to exacerbate cartilage degradation through NF-κB and HIF-2α. Remarkably, inhibiting p65 has been shown to significantly reduce the expression of HIF-2α, thereby decreasing cartilage degradation and the production of related factors (121). Furthermore, HIF-2α is known to enhance the influx of Zn(2+) in chondrocytes by activating the Zn-ZIP8-MTF1 axis, consequently promoting the expression of matrix metalloproteinases and amplifying the regulatory effect on cartilage destruction in OA (125).
Suppressing HIF-2α offers a therapeutic strategy to relieve OA. Osthole has been demonstrated to alleviate cartilage degeneration by inhibiting the NF-κB and HIF-2α pathways (122). Intra-articular resveratrol injections activate SIRT1, thereby effectively inhibiting HIF-2α and preventing the progression of OA (123). Additionally, chemically modified curcumin targets the NF-κB/Hif-2α axis while stabilizing the extracellular matrix, showing potential in slowing the progression of OA (132). D-Mannose reduces the sensitivity of chondrocytes to ferroptosis by inhibiting HIF-2α, aiming to alleviate OA (124).
Exploring further, additional strategies targeting HIF-2α involve employing IkappaBalpha kinase inhibitors and syndecan-4 inhibitors to address associated pathways. The inhibition of syndecan-4 has been found to reduce cartilage degradation in osteoarthritic mouse models by downregulating HIF-2α via miR-96-5p (126). Similarly, IkappaBalpha kinase inhibitors are reported to reduce cartilage degradation in mouse models by downregulating the NF-κB/HIF-2α axis (127).
IL-6 and IL-8
Cytokines IL-6 and IL-8 play a significant role in sleep apnea. Research has shown increased levels of IL-6, TNF-α, and IL-8 in the serum and plasma of SAS patients, which are positively correlated with disease severity, age, and BMI (133). Furthermore, a consistent conclusion from another study is that IL-6 levels are associated with both glucose metabolism and sleep apnea (134). This increase in IL-6 levels might be linked to the reduced oxygen consumption observed in sleep apnea patients (135). However, Kurt et al. (136) reported no significant variance in plasma IL-6 levels between the SAS group and a control group. As for the levels of IL-8, they are closely associated with obesity. Carpagnano et al. (137) observed a significant increase in the levels of IL-8, ICAM, and neutrophil percentage in induced saliva of obese sleep apnea patients, non-obese sleep apnea patients, and obese non-sleep apnea participants, compared to healthy individuals.
It remains contentious whether treating sleep apnea can influence the levels of IL-6 and IL-8. Previous findings suggest that adenotonsillectomy can improve clinical symptoms and signs, but has a minimal effect on TNF-α and IL-6 inflammatory levels (138). However, recent studies offer a different view, showing a decrease in circulating polymorphonuclear leukocyte levels of IL-6, IL-8, and β2-adrenergic receptor mRNA after adenotonsillectomy in children with obstructive SAS (139). One study found elevated IL-6 levels in patients with SAS, but Continuous positive airway pressure (CPAP) had no significant inhibitory effect on IL-6 levels in adults with SAS (140). In contrast, another study, by comparing untreated SAS patients and a control group, found that untreated SAS patients had significantly higher levels of IL-8 in peripheral blood than the control group. Moreover, this study suggests that nCPAP treatment can reduce hypoxia and the production of inflammatory mediators induced by SAS (141).
IL-6 and IL-8 are associated with OA. Senescent chondroprogenitor cells from the articular cartilage of knee OA patients contribute to the senescence-associated secretory phenotype by releasing IL-6 and IL-8 (142). Notably, IL-6 increases the production of inflammatory cytokines and expression of hypertrophic markers in primary mouse chondrocytes by activating JAK2/STAT3 (143). Blocking IL-6 can enhance Treg function and mitigate the progression of OA (144). Interestingly, IL-6 inhibits the spheroid size of osteophyte cells in OA by inducing apoptosis and reducing extracellular matrix molecules, highlighting its suppressive role in the regulation of pathological osteophyte formation (145).
Long non-coding RNA can influence the expression of IL-6 in OA. In OA patients, long non-coding RNA FER1L4 is downregulated, while IL-6 is upregulated. Importantly, the upregulation of FER1L4 can inhibit IL-6 expression in human chondrocytes (146).
Overexpression of IL-6 can exacerbate OA, and notably, PM2.5 can increase the production of IL-6 in human OA, worsening the condition (147). Moreover, Platelet response protein 2 promotes the production of IL-6 in OA synovial fibroblasts via the PI3K/AKT/NF-κB pathway (148).
Inhibiting IL-6 can alleviate OA. For instance, Tofacitinib relieves OA by upregulating miR-149-5p levels, thereby inhibiting the expression of JAK1/TNF-α/IL-6 (149). Similarly, Achyranthes root demonstrates anti-inflammatory and antioxidative treatment effects on OA and rheumatoid arthritis models by inhibiting the expression of IL-6 mediated matrix metalloproteinase-3 and -13 (150). Additionally, inhibition of the nuclear receptor RORα alleviates cartilage damage in OA by regulating the IL-6/STAT3 pathway (151). Furthermore, Genistein reduces obesity-induced OA in mice by inhibiting IL-6 and MMP-13 (152). Research has shown that fibroblast growth factor 10 delays the progression of OA by inhibiting the IL-6/JAK2/STAT3 signaling pathway, reducing synovial fibrosis (153). Physical therapy can also affect IL-6 expression in OA. Notably, rapid walking exercise significantly improves patients’ daily functioning and physical ability, while notably reducing TNF-α and IL-6 levels (154). Additionally, pulsed electromagnetic fields improve cartilage matrix, chondrocyte apoptosis, and autophagy by inhibiting TNF-α and IL-6 signaling (155). It is also suggested that IL-8 may be a risk factor for OA, with serum IL-8 levels being associated with aggravated knee joint symptoms (156). Moreover, research indicates that IL-8/Kc is highly responsive to mechanical, inflammatory, and metabolic stress (157).
Discussion
SAS and OA not only exhibit numerous common features, epidemiological studies reveal a tight correlation between them. Patients with both OA and SAS exhibit more severe symptoms of knee arthritis than those with only OA. Additional research has identified SAS as a risk factor for OA (3). There are numerous complex mechanisms involved (Figure 1). SAS results in intermittent hypoxia, which over time leads to mitochondrial dysfunction and significant ROS activation that stimulates inflammasomes (NLRP3), causing inflammation. ROS activates the NF-kB pathway, enhancing the production of inflammatory markers like IL-6 and TNF-α, which can expand the inflammatory response in joint synovium and lead to the breakdown of the extracellular matrix in cartilage cells, exacerbating OA.
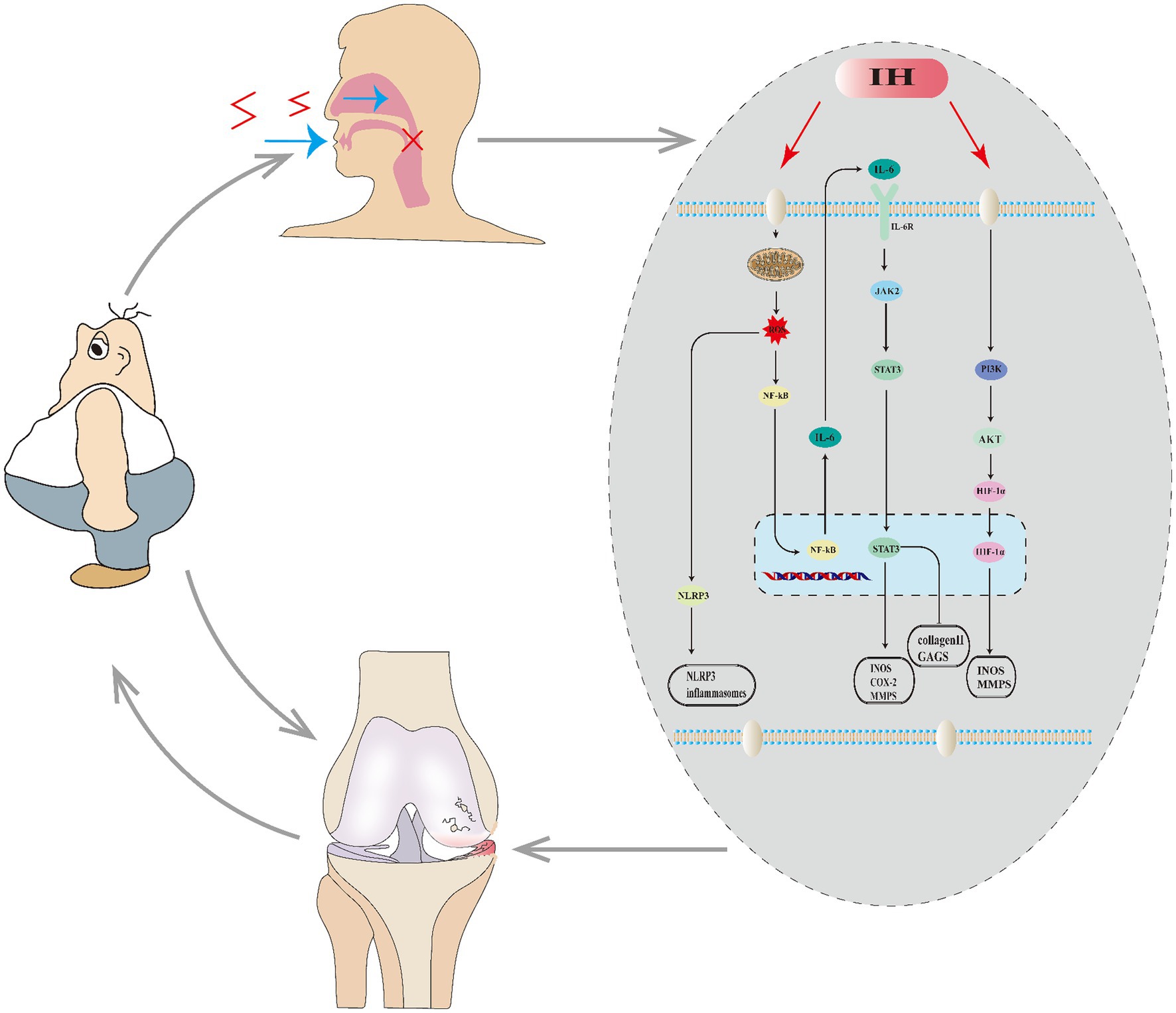
Figure 1. Relationship between OA and SAS. SAS causes intermittent hypoxia (IH) in the body, which affects a series of molecules and pathways, leading to the worsening of OA. OA, in turn, promotes obesity, which can further exacerbate both SAS and OA.
Conversely, is OA a risk factor for SAS? Research in this area is still limited; however, Karla Diaz et al. (159) found a certain correlation between newly diagnosed SAS and prior OA. OA patients suffer from poor proprioception, reduced joint mobility, and weak muscle strength (160), leading to a loss of normal activity. A decrease in physical activity can result in becoming overweight and obese (161). Obesity further aggravates SAS. Overall, there is a bidirectional link between OA and SAS. SAS exacerbates OA, and decreased activity among OA sufferers could lead to obesity, thereby intensifying SAS. Owing to insufficient literature, it remains uncertain whether OA is a risk factor for SAS, but there appears to be a significant relationship between the two. Whether this relationship is causal or merely correlational needs more experimental evidence in the future.
In treating OA patients with SAS, intervention for SAS must not be neglected alongside OA treatment. Reducing weight is both essential and primary, and must be combined with correcting hypoventilation. CPAP is the gold standard for addressing hypoxia in SAS; however, its effectiveness on certain complications of SAS remains debated, potentially due to issues like patient compliance (162). Reduced patient compliance can result in poorer treatment effectiveness. Surgery to enhance hypoventilation is another method; Uvulopalatopharyngoplasty (UPPP) is the most frequently performed surgery for OSA. In traditional UPPP, tissue surrounding the blockage is removed to expand the airway and relieve obstruction (163). The success rate of the surgery is also related to the anatomical staging system; for stage IV patients, the success rate is only 20% (164). Excessive removal of obstructive parts and surrounding tissue to enlarge the airway space can cause complications such as nasopharyngeal narrowing and palatopharyngeal dysfunction (158). Hence, surgery is neither the preferred nor compulsory option, as its effectiveness in relieving small and multiple airway obstructions is limited.
For patients treating OA combined with SAS, relying solely on CPAP is insufficient; medication is also necessary (Tables 1–3), which targets and inhibits certain key molecules or pathways (Tables 1–3) to achieve therapeutic effects. Mitochondrial dysfunction is an upstream factor in these molecular pathways, and improving mitochondrial function might be a more effective method. In the future, treatment for patients with OA combined with SAS may involve improving or renewing mitochondrial function. Currently, a prevalent strategy includes the use of agents such as Urolithin A to boost mitochondrial functionality (165). Additionally, activating mitochondrial autophagy to clear damaged mitochondria can be achieved; for instance, artemisinin reduces TNFSF11 expression in cartilage and inhibits the PI3K/AKT/mTOR signaling pathway, thereby activating mitochondrial autophagy (166). Mitochondrial transplantation is another strategy; Lee et al. (167) have used mitochondrial transplantation to mitigate the progression of OA. Recently, liposomes have emerged as a new tool for transporting mitochondria (168), facilitating mitochondrial regeneration, representing a promising therapeutic strategy. Zhang et al. (169) have discovered that sustained release of melatonin can reactivate mitochondria in cartilage cells. Developing a sustained-release system for melatonin may also be a viable treatment method in the future.
Conclusion
There is a close relationship between OA and SAS. SAS is a risk factor for OA, and OA might influence the progression of SAS, though the specifics of this influence require further experimental investigation. For patients with OA who have SAS, treating OA while also intervening timely in SAS is necessary, with weight reduction being a primary and essential step, alongside combined treatments such as CPAP and medications. Current drug development efforts involve numerous studies aimed at inhibiting or clearing molecular pathways, including ROS, NF-KB pathway, IL-6, and IL-8. Improving mitochondrial function may be an effective new method; renewing or transplanting mitochondria is a promising direction.
Author contributions
LW: Conceptualization, Formal analysis, Project administration, Writing – original draft, Writing – review & editing. YL: Conceptualization, Methodology, Writing – original draft, Writing – review & editing. XL: Software, Visualization, Writing – review & editing. KY: Conceptualization, Data curation, Software, Visualization, Writing – review & editing. QZ: Data curation, Software, Visualization, Writing – review & editing. JT: Data curation, Software, Visualization, Writing – review & editing. YY: Conceptualization, Data curation, Investigation, Methodology, Project administration, Software, Supervision, Validation, Visualization, Writing – review & editing, Writing – original draft.
Funding
The author(s) declare that no financial support was received for the research, authorship, and/or publication of this article.
Conflict of interest
The authors declare that the research was conducted in the absence of any commercial or financial relationships that could be construed as a potential conflict of interest.
Publisher’s note
All claims expressed in this article are solely those of the authors and do not necessarily represent those of their affiliated organizations, or those of the publisher, the editors and the reviewers. Any product that may be evaluated in this article, or claim that may be made by its manufacturer, is not guaranteed or endorsed by the publisher.
References
1. de Araujo, DAB, Goncalves, FM, Martins, AA, Alves, GA, Stechman-Neto, J, Correa, CC, et al. Worldwide prevalence and associated risk factors of obstructive sleep apnea: a meta-analysis and meta-regression. Sleep Breath. (2023) 27:2083–109. doi: 10.1007/s11325-023-02810-7
2. Loo, GH, Rajan, R, Mohd, TA, and Ritza, KN. Prevalence of obstructive sleep apnea in an Asian bariatric population: an underdiagnosed dilemma. Surg Obes Relat Dis. (2020) 16:778–83. doi: 10.1016/j.soard.2020.02.003
3. Kanbay, A, Kokturk, O, Pihtili, A, Ceylan, E, Tulu, S, Madenci, E, et al. Obstructive sleep apnea is a risk factor for osteoarthritis. Tuberk Toraks. (2018) 66:304–11. doi: 10.5578/tt.57403
4. Silva, A, Mello, MT, Serrao, PR, Luz, RP, Ruiz, F, Bittencourt, LR, et al. Influence of obstructive sleep apnea in the functional aspects of patients with osteoarthritis. J Clin Sleep Med. (2018) 14:265–70. doi: 10.5664/jcsm.6950
5. da Silva, NC, da Silva, G, Onofri, SMM, and Pinato, L. Obstructive sleep apnea and orofacial myofunctional aspects in obesity. Sleep Breath. (2023) 27:1351–8. doi: 10.1007/s11325-022-02738-4
6. Lee, S, Ryu, S, Lee, GE, Redline, S, and Morey, BN. Risk of sleep apnea is associated with abdominal Obesity among Asian Americans: comparing waist-to-hip ratio and body mass index. J Racial Ethn Health Disparities. (2024) 11:157–67. doi: 10.1007/s40615-022-01507-z
7. Duksal, F, and Eren, F. Evaluation of thyroid functions and obesity in obstructive sleep apnea syndrome. Rev Assoc Med Bras (1992). (2023) 69:e20230376. doi: 10.1590/1806-9282.20230376
8. Qin, H, Wang, Y, Chen, X, Steenbergen, N, Penzel, T, Zhang, X, et al. The efficacy of bariatric surgery on pulmonary function and sleep architecture of patients with obstructive sleep apnea and co-morbid obesity: a systematic review and meta-analysis. Surg Obes Relat Dis. (2023) 19:1444–57. doi: 10.1016/j.soard.2023.07.007
9. Patial, K, Mishra, HP, Pal, G, Suvvari, TK, Ghosh, T, Mishra, SS, et al. Understanding the association between Obesity and obstructive sleep apnea syndrome: a case-control study. Cureus. (2023) 15:e45843. doi: 10.7759/cureus.45843
10. Wyszomirski, K, Waledziak, M, and Rozanska-Waledziak, A. Obesity bariatric surgery and obstructive sleep apnea-a narrative literature review. Medicina (Kaunas). (2023) 59:1266. doi: 10.3390/medicina59071266
11. Caliendo, C, Femiano, R, Umano, GR, Martina, S, Nucci, L, Perillo, L, et al. Effect of Obesity on the respiratory parameters in children with obstructive sleep apnea syndrome. Children (Basel). (2023) 10:1874. doi: 10.3390/children10121874
12. Park, D, Park, YM, Ko, SH, Hyun, KS, Choi, YH, Min, DU, et al. Association of general and central obesity, and their changes with risk of knee osteoarthritis: a nationwide population-based cohort study. Sci Rep. (2023) 13:3796. doi: 10.1038/s41598-023-30727-4
13. Park, JM . Association between obesity and osteoarthritis in the south Korean older population: a nationwide population-based study. Medicine (Baltimore). (2023) 102:e33455. doi: 10.1097/MD.0000000000033455
14. Altowijri, AA, Alnadawi, AA, Almutairi, JN, Almutairi, AK, Alhawiti, MS, Abu Sinah, AK, et al. The prevalence of knee osteoarthritis and its association with Obesity among individuals in Saudi Arabia. Cureus. (2023) 15:e49625. doi: 10.7759/cureus.49625
15. Ding, C, Yimiti, D, Sanada, Y, Matsubara, Y, Nakasa, T, Matsubara, K, et al. High-fat diet-induced obesity accelerates the progression of spontaneous osteoarthritis in senescence-accelerated mouse prone 8 (SAMP8). Mod Rheumatol. (2023) 34:831–40. doi: 10.1093/mr/road069
16. Yuan, J, Wang, D, Zhang, Y, and Dou, Q. Genetically predicted obesity and risk of hip osteoarthritis. Eat Weight Disord. (2023) 28:11. doi: 10.1007/s40519-023-01538-3
17. Cheng, KY, Strotmeyer, ES, Kado, DM, Schousboe, JT, Schenk, S, Nevitt, M, et al. The Association of Metabolic Syndrome and Obesity with Clinical hip Osteoarthritis in the study of osteoporotic fractures and the osteoporotic fractures in men study Cohorts.ACR open. Rheumatol. (2023) 5:115–23. doi: 10.1002/acr2.11518
18. Shumnalieva, R, Kotov, G, and Monov, S. Obesity-related knee osteoarthritis-current concepts. Life (Basel). (2023) 13:1650. doi: 10.3390/life13081650
19. Messier, SP, Gill, ME, Mihalko, SL, Beavers, DP, Queen, K, Miller, GD, et al. Clinical, health-related quality of life, and gait differences among Obesity classes in adults with knee osteoarthritis. Arthritis Care Res (Hoboken). (2023) 76:503–10. doi: 10.1002/acr.25265
20. Liao, J, Chen, J, Xu, W, Chen, J, Liang, X, Cheng, Q, et al. Prevalence and associations of sarcopenia, obesity and sarcopenic obesity in end-stage knee osteoarthritis patients. J Health Popul Nutr. (2023) 42:108. doi: 10.1186/s41043-023-00438-7
21. Eymard, F, and Aron-Wisnewsky, J. Osteoarthritis in patients with obesity: the bariatric surgery impacts on its evolution. Joint Bone Spine. (2023) 91:105639. doi: 10.1016/j.jbspin.2023.105639
22. Seifen, C, Pordzik, J, Bahr, K, Grosse-Bruggemann, L, Ludwig, K, Hackenberg, B, et al. Accumulating comorbidities may promote increasing severity of obstructive sleep apnea with aging in males but not in females. J Pers Med. (2022) 13:79. doi: 10.3390/jpm13010079
23. Thompson, C, Legault, J, Moullec, G, Baltzan, M, Cross, N, Dang-Vu, TT, et al. A portrait of obstructive sleep apnea risk factors in 27,210 middle-aged and older adults in the Canadian longitudinal study on aging. Sci Rep. (2022) 12:5127. doi: 10.1038/s41598-022-08164-6
24. Qiu, Q, and Mateika, JH. Pathophysiology of obstructive sleep apnea in aging women. Curr Sleep Med Rep. (2021) 7:177–85. doi: 10.1007/s40675-021-00218-x
25. Li, Y, and Wang, Y. Obstructive sleep apnea-hypopnea syndrome as a novel potential risk for aging. Aging Dis. (2021) 12:586–96. doi: 10.14336/AD.2020.0723
26. Tessema, B, Sack, U, Konig, B, Serebrovska, Z, and Egorov, E. Effects of intermittent hypoxia in training regimes and in obstructive sleep apnea on aging biomarkers and age-related diseases: a systematic review. Front Aging Neurosci. (2022) 14:878278. doi: 10.3389/fnagi.2022.878278
27. Legault, J, Thompson, C, Moullec, G, Baril, AA, Martineau-Dussault, ME, Andre, C, et al. Age- and sex-specific associations between obstructive sleep apnea risk and cognitive decline in middle-aged and older adults: a 3-year longitudinal analysis of the Canadian longitudinal study on aging. Sleep Med. (2023) 112:77–87. doi: 10.1016/j.sleep.2023.09.029
28. Thompson, C, Legault, J, Moullec, G, Martineau-Dussault, ME, Baltzan, M, Cross, N, et al. Association between risk of obstructive sleep apnea, inflammation and cognition after 45 years old in the Canadian longitudinal study on aging. Sleep Med. (2022) 91:21–30. doi: 10.1016/j.sleep.2022.02.006
29. Weihs, A, Frenzel, S, Wittfeld, K, Obst, A, Stubbe, B, Habes, M, et al. Associations between sleep apnea and advanced brain aging in a large-scale population study. Sleep. (2021) 44:zsaa204. doi: 10.1093/sleep/zsaa204
30. Kus, G, Yasaci, Z, Boz, C, and Turkmen, E. Association of Osteoarthritis Prevalence with age and Obesity Factors in Organization for Economic Cooperation and Development countries: panel regression model. Am J Phys Med Rehabil. (2023) 102:901–6. doi: 10.1097/PHM.0000000000002244
31. Mustari, MN, Massi, MN, Usman, MA, Fikry, A, Bukhari, A, Idris, I, et al. Dynamic interaction of obesity, age, MCP-1 level, and ACE-1 gene with the severity of knee osteoarthritis: a cross-sectional study. Ann Med Surg (Lond). (2023) 85:3845–51. doi: 10.1097/MS9.0000000000000973
32. Geraghty, T, Obeidat, AM, Ishihara, S, Wood, MJ, Li, J, Lopes, EBP, et al. Age-associated changes in knee osteoarthritis, pain-related behaviors, and dorsal root ganglia Immunophenotyping of male and female mice. Arthritis Rheumatol. (2023) 75:1770–80. doi: 10.1002/art.42530
33. Di, J, Bai, J, Zhang, J, Chen, J, Hao, Y, Bai, J, et al. Regional disparities, age-related changes and sex-related differences in knee osteoarthritis. BMC Musculoskelet Disord. (2024) 25:66. doi: 10.1186/s12891-024-07191-w
34. Prakash, R, Gardner, JE, Petric, UB, Pathak, R, Atem, F, and Jain, NB. Association of age and sex at onset with Glenohumeral osteoarthritis: a systematic review and meta-analysis. Am J Phys Med Rehabil. (2024) 103:611–6. doi: 10.1097/PHM.0000000000002419
35. van Beest, S, Kloppenburg, M, Rosendaal, FR, and van de Stadt, LA. Subluxation of the first carpometacarpal joint and age are important factors in reduced hand strength in patients with hand osteoarthritis. Scand J Rheumatol. (2023) 52:637–44. doi: 10.1080/03009742.2023.2215016
36. Chen, Q, Han, X, Chen, M, Zhao, B, Sun, B, Sun, L, et al. High-fat diet-induced mitochondrial dysfunction promotes genioglossus injury - a potential mechanism for obstructive sleep apnea with Obesity. Nat Sci Sleep. (2021) 13:2203–19. doi: 10.2147/NSS.S343721
37. Kim, YS, Kwak, JW, Lee, KE, Cho, HS, Lim, SJ, Kim, KS, et al. Can mitochondrial dysfunction be a predictive factor for oxidative stress in patients with obstructive sleep apnea?Antioxid redox. Signals. (2014) 21:1285–8. doi: 10.1089/ars.2014.5955
38. Lacedonia, D, Carpagnano, GE, Crisetti, E, Cotugno, G, Palladino, GP, Patricelli, G, et al. Mitochondrial DNA alteration in obstructive sleep apnea. Respir Res. (2015) 16:47. doi: 10.1186/s12931-015-0205-7
39. Seo, YJ, Ju, HM, Lee, SH, Kwak, SH, Kang, MJ, Yoon, JH, et al. Damage of inner ear sensory hair cells via mitochondrial loss in a murine model of sleep apnea with chronic intermittent hypoxia. Sleep. (2017) 40:9. doi: 10.1093/sleep/zsx106
40. Baylan, FA, and Yarar, E. Relationship between the mitochondria-derived peptide MOTS-c and insulin resistance in obstructive sleep apnea. Sleep Breath. (2021) 25:861–6. doi: 10.1007/s11325-020-02273-0
41. Wu, X, Gong, L, Xie, L, Gu, W, Wang, X, Liu, Z, et al. NLRP3 deficiency protects against intermittent hypoxia-induced Neuroinflammation and mitochondrial ROS by promoting the PINK1-Parkin pathway of Mitophagy in a murine model of sleep apnea. Front Immunol. (2021) 12:628168. doi: 10.3389/fimmu.2021.628168
42. Kan, S, Duan, M, Liu, Y, Wang, C, and Xie, J. Role of mitochondria in physiology of chondrocytes and diseases of osteoarthritis and rheumatoid arthritis. Cartilage. (2021) 13:1102S–21S. doi: 10.1177/19476035211063858
43. Jiang, W, Liu, H, Wan, R, Wu, Y, Shi, Z, and Huang, W. Mechanisms linking mitochondrial mechanotransduction and chondrocyte biology in the pathogenesis of osteoarthritis. Ageing Res Rev. (2021) 67:101315. doi: 10.1016/j.arr.2021.101315
44. Liu, P, Zhao, D, Pan, Z, Tang, W, Chen, H, and Hu, K. Identification and validation of ferroptosis-related hub genes in obstructive sleep apnea syndrome. Front Neurol. (2023) 14:1130378. doi: 10.3389/fneur.2023.1130378
45. Lavie, L . Oxidative stress inflammation and endothelial dysfunction in obstructive sleep apnea. Front Biosci (Elite Ed). (2012) 4:1391–403. doi: 10.2741/469
46. Lavie, L . Oxidative stress in obstructive sleep apnea and intermittent hypoxia--revisited--the bad ugly and good: implications to the heart and brain. Sleep Med Rev. (2015) 20:27–45. doi: 10.1016/j.smrv.2014.07.003
47. Yu, LM, Zhang, WH, Han, XX, Li, YY, Lu, Y, Pan, J, et al. Hypoxia-induced ROS contribute to myoblast Pyroptosis during obstructive sleep apnea via the NF-kappaB/HIF-1alpha signaling pathway. Oxidative Med Cell Longev. (2019) 2019:4596368. doi: 10.1155/2019/4596368
48. Liu, X, Li, Y, Zhao, J, Hu, Z, Fang, W, Ke, J, et al. Pyroptosis of chondrocytes activated by synovial inflammation accelerates TMJ osteoarthritis cartilage degeneration via ROS/NLRP3 signaling. Int Immunopharmacol. (2023) 124:110781. doi: 10.1016/j.intimp.2023.110781
49. Lin, C, Ge, L, Tang, L, He, Y, Moqbel, SAA, Xu, K, et al. Nitidine chloride alleviates inflammation and cellular senescence in murine osteoarthritis through scavenging ROS. Front Pharmacol. (2022) 13:919940. doi: 10.3389/fphar.2022.919940
50. Zhang, S, Cai, J, Yao, Y, Huang, L, Zheng, L, and Zhao, J. Mitochondrial-targeting Mn(3)O(4)/UIO-TPP nanozyme scavenge ROS to restore mitochondrial function for osteoarthritis therapy. Regen Biomater. (2023) 10:rbad078. doi: 10.1093/rb/rbad078
51. Wu, J, Qin, Z, Jiang, X, Fang, D, Lu, Z, Zheng, L, et al. ROS-responsive PPGF nanofiber membrane as a drug delivery system for long-term drug release in attenuation of osteoarthritis.NPJ. Regen Med. (2022) 7:66. doi: 10.1038/s41536-022-00254-3
52. Hu, H, Yang, J, Zhong, Y, Wang, J, Cai, J, Luo, C, et al. Polydopamine-Pd nanozymes as potent ROS scavengers in combination with near-infrared irradiation for osteoarthritis treatment. iScience. (2023) 26:106605. doi: 10.1016/j.isci.2023.106605
53. Yi, N, Mi, Y, Xu, X, Li, N, Chen, B, Yan, K, et al. Nodakenin attenuates cartilage degradation and inflammatory responses in a mice model of knee osteoarthritis by regulating mitochondrial Drp1/ROS/NLRP3 axis. Int Immunopharmacol. (2022) 113:109349. doi: 10.1016/j.intimp.2022.109349
54. Sun, H, Sun, Z, Xu, X, Lv, Z, Li, J, Wu, R, et al. Blocking TRPV4 ameliorates osteoarthritis by inhibiting M1 macrophage polarization via the ROS/NLRP3 signaling pathway. Antioxidants (Basel). (2022) 11:2315. doi: 10.3390/antiox11122315
55. Li, H, Yuan, Y, Zhang, L, Xu, C, Xu, H, and Chen, Z. Reprogramming macrophage polarization, depleting ROS by Astaxanthin and Thioketal-containing polymers delivering rapamycin for osteoarthritis treatment. Adv Sci (Weinh). (2023) 11:e2305363. doi: 10.1002/advs.202305363
56. Zhang, Z, Xie, S, Qian, J, Gao, F, Jin, W, Wang, L, et al. Targeting macrophagic PIM-1 alleviates osteoarthritis by inhibiting NLRP3 inflammasome activation via suppressing mitochondrial ROS/cl(−) efflux signaling pathway. J Transl Med. (2023) 21:452. doi: 10.1186/s12967-023-04313-1
57. Zhang, Z, Yuan, L, Liu, Y, Wang, R, Zhang, Y, Yang, Y, et al. Integrated Cascade Nanozyme remodels chondrocyte inflammatory microenvironment in temporomandibular joint osteoarthritis via inhibiting ROS-NF-kappaB and MAPK pathways. Adv Healthc Mater. (2023) 12:e2203195. doi: 10.1002/adhm.202203195
58. Chen, H, Tu, M, Liu, S, Wen, Y, and Chen, L. Dendrobine alleviates cellular senescence and osteoarthritis via the ROS/NF-kappaB Axis. Int J Mol Sci. (2023) 24:2365. doi: 10.3390/ijms24032365
59. Xu, J, Yu, Y, Chen, K, Wang, Y, Zhu, Y, Zou, X, et al. Astragalus polysaccharides ameliorate osteoarthritis via inhibiting apoptosis by regulating ROS-mediated ASK1/p38 MAPK signaling pathway targeting on TXN. Int J Biol Macromol. (2024) 258:129004. doi: 10.1016/j.ijbiomac.2023.129004
60. Zhen, J, Chen, X, Mao, Y, Xie, X, Chen, X, Xu, W, et al. GLX351322, a novel NADPH oxidase 4 inhibitor, attenuates TMJ osteoarthritis by inhibiting the ROS/MAPK/NF-kappaB signaling pathways. Oxidative Med Cell Longev. (2023) 2023:1952348. doi: 10.1155/2023/1952348
61. Huang, YF, Wang, G, Ding, L, Bai, ZR, Leng, Y, Tian, JW, et al. Lactate-upregulated NADPH-dependent NOX4 expression via HCAR1/PI3K pathway contributes to ROS-induced osteoarthritis chondrocyte damage. Redox Biol. (2023) 67:102867. doi: 10.1016/j.redox.2023.102867
62. Ni, S, Yi, N, Yuan, H, Li, D, Chen, X, and Zhuang, C. Angelica sinensis polysaccharide improves mitochondrial metabolism of osteoarthritis chondrocytes through PPARgamma/SOD2/ROS pathways. Phytother Res. (2023) 37:5394–406. doi: 10.1002/ptr.7979
63. Shi, G, Jiang, H, Yang, F, Lin, Z, Li, M, Guo, J, et al. NIR-responsive molybdenum (Mo)-based nanoclusters enhance ROS scavenging for osteoarthritis therapy. Pharmacol Res. (2023) 192:106768. doi: 10.1016/j.phrs.2023.106768
64. Xue, C, Tian, J, Cui, Z, Liu, Y, Sun, D, Xiong, M, et al. Reactive oxygen species (ROS)-mediated M1 macrophage-dependent nanomedicine remodels inflammatory microenvironment for osteoarthritis recession. Bioact Mater. (2024) 33:545–61. doi: 10.1016/j.bioactmat.2023.10.032
65. Kang, HH, Kim, IK, Yeo, CD, Kim, SW, Lee, HY, Im, JH, et al. The effects of chronic intermittent hypoxia in bleomycin-induced lung injury on pulmonary fibrosis via regulating the NF-kappaB/Nrf2 signaling pathway. Tuberc Respir Dis (Seoul). (2020) 83:S63–74. doi: 10.4046/trd.2020.0112
66. Tang, Y, Wang, J, and Cai, W. and Xu J.RAGE/NF-kappaB pathway mediates hypoxia-induced insulin resistance in 3T3-L1 adipocytes. Biochem Biophys Res Commun. (2020) 521:77–83. doi: 10.1016/j.bbrc.2019.10.076
67. Su, T, Li, C, Zhang, Y, Yue, L, Chen, Y, Qian, X, et al. Upregulation of HMGB1 promotes vascular dysfunction in the soft palate of patients with obstructive sleep apnea via the TLR4/NF-kappaB/VEGF pathway. FEBS Open Bio. (2023) 13:246–56. doi: 10.1002/2211-5463.13533
68. Liu, F, Liu, TW, and Kang, J. The role of NF-kappaB-mediated JNK pathway in cognitive impairment in a rat model of sleep apnea. J Thorac Dis. (2018) 10:6921–31. doi: 10.21037/jtd.2018.12.05
69. Zhang, CQ, Yi, S, Chen, BB, Cui, PP, Wang, Y, and Li, Y. Z.mTOR/NF-kappaB signaling pathway protects hippocampal neurons from injury induced by intermittent hypoxia in rats. Int J Neurosci. (2021) 131:994–1003. doi: 10.1080/00207454.2020.1766460
70. Nakagawa, T, Furukawa, Y, Hayashi, T, Nomura, A, Yokoe, S, Moriwaki, K, et al. Augmented O-GlcNAcylation attenuates intermittent hypoxia-induced cardiac remodeling through the suppression of NFAT and NF-kappaB activities in mice. Hypertens Res. (2019) 42:1858–71. doi: 10.1038/s41440-019-0311-x
71. Chen, YC, Hsu, PY, Su, MC, Chen, TW, Hsiao, CC, Chin, CH, et al. MicroRNA sequencing analysis in obstructive sleep apnea and depression: anti-oxidant and MAOA-inhibiting effects of miR-15b-5p and miR-92b-3p through targeting PTGS1-NF-kappaB-SP1 signaling. Antioxidants (Basel). (2021) 10:1854. doi: 10.3390/antiox10111854
72. Zhuang, H, Ren, X, Jiang, F, and Zhou, P. Indole-3-propionic acid alleviates chondrocytes inflammation and osteoarthritis via the AhR/NF-kappaB axis. Mol Med. (2023) 29:17. doi: 10.1186/s10020-023-00614-9
73. Zhang, P, Jin, Y, Xia, W, Wang, X, and Zhou, Z. Phillygenin inhibits inflammation in chondrocytes via the Nrf2/NF-kappaB axis and ameliorates osteoarthritis in mice. J Orthop Translat. (2023) 41:1–11. doi: 10.1016/j.jot.2023.03.002
74. Xia, W, Xiao, J, Tong, C, Lu, J, Tu, Y, Li, S, et al. Orientin inhibits inflammation in chondrocytes and attenuates osteoarthritis through Nrf2/NF-kappaB and SIRT6/NF-kappaB pathway. J Orthop Res. (2023) 41:2405–17. doi: 10.1002/jor.25573
75. Wu, Y, Ying, J, Zhu, X, Xu, C, and Wu, L. Pachymic acid suppresses the inflammatory response of chondrocytes and alleviates the progression of osteoarthritis via regulating the Sirtuin 6/NF-kappaB signal axis. Int Immunopharmacol. (2023) 124:110854. doi: 10.1016/j.intimp.2023.110854
76. Zeng, Z, Li, H, Luo, C, Hu, W, Weng, TJ, and Shuang, F. Pelargonidin ameliorates inflammatory response and cartilage degeneration in osteoarthritis via suppressing the NF-kappaB pathway. Arch Biochem Biophys. (2023) 743:109668. doi: 10.1016/j.abb.2023.109668
77. Deng, X, Qu, Y, Li, M, Wu, C, Dai, J, Wei, K, et al. Sakuranetin reduces inflammation and chondrocyte dysfunction in osteoarthritis by inhibiting the PI3K/AKT/NF-kappaB pathway. Biomed Pharmacother. (2024) 171:116194. doi: 10.1016/j.biopha.2024.116194
78. Xu, J, Zhou, K, Gu, H, Zhang, Y, Wu, L, Bian, C, et al. Exosome miR-4738-3p-mediated regulation of COL1A2 through the NF-kappaB and inflammation signaling pathway alleviates osteoarthritis low-grade inflammation symptoms. Biomol Biomed. (2023) 24:520–36. doi: 10.17305/bb.2023.9921
79. Zhou, K, Liu, D, Jin, Y, Xia, W, Zhang, P, and Zhou, Z. Oxymatrine ameliorates osteoarthritis via the Nrf2/NF-kappaB axis in vitro and in vivo. Chem Biol Interact. (2023) 380:110539. doi: 10.1016/j.cbi.2023.110539
80. Lu, J, Miao, Z, Jiang, Y, Xia, W, Wang, X, Shi, Y, et al. Chrysophanol prevents IL-1beta-induced inflammation and ECM degradation in osteoarthritis via the Sirt6/NF-kappaB and Nrf2/NF-kappaB axis. Biochem Pharmacol. (2023) 208:115402. doi: 10.1016/j.bcp.2022.115402
81. Wan, J, Li, M, Yuan, X, Yu, X, Chen, A, Shao, M, et al. Rutaecarpine ameliorates osteoarthritis by inhibiting PI3K/AKT/NF-kappaB and MAPK signalling transduction through integrin alphaVbeta3. Int J Mol Med. (2023) 52:97. doi: 10.3892/ijmm.2023.5300
82. Lu, R, Wei, Z, Wang, Z, Xu, S, Sun, K, Cheng, P, et al. Mulberroside a alleviates osteoarthritis via restoring impaired autophagy and suppressing MAPK/NF-kappaB/PI3K-AKT-mTOR signaling pathways. iScience. (2023) 26:105936. doi: 10.1016/j.isci.2023.105936
83. Wang, Z, Ma, J, Miao, Z, Sun, Y, Dong, M, Lin, Y, et al. Ergothioneine inhibits the progression of osteoarthritis via the Sirt6/NF-kappaB axis both in vitro and in vivo. Int Immunopharmacol. (2023) 119:110211. doi: 10.1016/j.intimp.2023.110211
84. Lin, S, Lu, J, Chen, Q, Jiang, H, Lou, C, Lin, C, et al. Plantamajoside suppresses the activation of NF-kappaB and MAPK and ameliorates the development of osteoarthritis. Int Immunopharmacol. (2023) 115:109582. doi: 10.1016/j.intimp.2022.109582
85. Zheng, X, Qiu, J, Gao, N, Jiang, T, Li, Z, Zhang, W, et al. Paroxetine attenuates chondrocyte Pyroptosis and inhibits osteoclast formation by inhibiting NF-kappaB pathway activation to delay osteoarthritis progression. Drug Des Devel Ther. (2023) 17:2383–99. doi: 10.2147/DDDT.S417598
86. Cai, T, Ye, H, Jiang, H, Lin, C, Lou, C, Wang, W, et al. Stevioside targets the NF-kappaB and MAPK pathways for inhibiting inflammation and apoptosis of chondrocytes and ameliorates osteoarthritis in vivo. Int Immunopharmacol. (2023) 115:109683. doi: 10.1016/j.intimp.2023.109683
87. Zhao, C, Sun, G, Li, Y, Kong, K, Li, X, Kan, T, et al. Forkhead box O3 attenuates osteoarthritis by suppressing ferroptosis through inactivation of NF-kappaB/MAPK signaling. J Orthop Translat. (2023) 39:147–62. doi: 10.1016/j.jot.2023.02.005
88. Shen, PC, Huang, SH, Liu, ZM, Lu, CC, Chou, SH, and Tien, YC. Suramin ameliorates osteoarthritis by acting on the Nrf2/HO-1 and NF-kappaB signaling pathways in chondrocytes and promoting M2 polarization in macrophages. Int Immunopharmacol. (2023) 120:110295. doi: 10.1016/j.intimp.2023.110295
89. Shi, Y, Tao, H, Li, X, Zhang, L, Li, C, Sun, W, et al. Kappa-opioid receptor activation attenuates osteoarthritis synovitis by regulating macrophage polarization through the NF-kappaB pathway. Acta Biochim Biophys Sin Shanghai. (2023) 56:82–95. doi: 10.3724/abbs.2023223
90. Zhao, P, Ma, G, and Ma, L. miR-181a-5p targets DDX3X to inhibit the progression of osteoarthritis via NF-KappaB signaling pathway. J Orthop Surg Res. (2023) 18:606. doi: 10.1186/s13018-023-04073-0
91. Chen, J, Liu, Z, Sun, H, Liu, M, Wang, J, Zheng, C, et al. MiR-203a-3p attenuates apoptosis and pyroptosis of chondrocytes by regulating the MYD88/NF-kappaB pathway to alleviate osteoarthritis progression. Aging (Albany NY). (2023) 15:14457–72. doi: 10.18632/aging.205373
92. Wang, Y, Jin, Z, Jia, S, Shen, P, Yang, Y, and Huang, Y. Mechanical stress protects against chondrocyte pyroptosis through TGF-beta1-mediated activation of Smad2/3 and inhibition of the NF-kappaB signaling pathway in an osteoarthritis model. Biomed Pharmacother. (2023) 159:114216. doi: 10.1016/j.biopha.2023.114216
93. Wang, K, Zhang, X, Li, X, Li, D, Shan, Z, and Yao, C. Non-weight-bearing exercise attenuates papain-induced knee osteoarthritis in rats via the TLR4/MyD88/NF-kappaB signaling pathway. J Orthop Surg Res. (2023) 18:695. doi: 10.1186/s13018-023-04201-w
94. Jin, X, Yu, Y, Lin, Y, Yang, J, and Chen, Z. Tendon-regulating and bone-setting manipulation promotes the recovery of synovial inflammation in rabbits with knee osteoarthritis via the TLR4-MyD88-NF-κB signaling pathway. Ann Transl Med. (2023) 11:245. doi: 10.21037/atm-22-3039
95. Liu, J, Jia, S, Yang, Y, Piao, L, Wang, Z, Jin, Z, et al. Exercise induced meteorin-like protects chondrocytes against inflammation and pyroptosis in osteoarthritis by inhibiting PI3K/Akt/NF-kappaB and NLRP3/caspase-1/GSDMD signaling. Biomed Pharmacother. (2023) 158:114118. doi: 10.1016/j.biopha.2022.114118
96. Huang, W, Tang, H, Liu, Y, Li, W, Shimu, AS, Li, B, et al. ROR1/STAT3 positive feedback loop facilitates cartilage degeneration in osteoarthritis through activation of NF-kappaB signaling pathway. Int Immunopharmacol. (2023) 121:110433. doi: 10.1016/j.intimp.2023.110433
97. Chen, Y, Guo, W, Lu, W, Guo, X, Gao, W, and Yin, Z. SNIP1 reduces extracellular matrix degradation and inflammation via inhibiting the NF-kappaB signaling pathway in osteoarthritis. Arch Biochem Biophys. (2023) 747:109764. doi: 10.1016/j.abb.2023.109764
98. Cai, D, Zhang, J, Yang, J, Lv, Q, and Zhong, C. Overexpression of FTO alleviates osteoarthritis by regulating the processing of miR-515-5p and the TLR4/MyD88/NF-kappaB axis. Int Immunopharmacol. (2023) 114:109524. doi: 10.1016/j.intimp.2022.109524
99. Song, D, Fang, G, Mao, SZ, Ye, X, Liu, G, Miller, EJ, et al. Selective inhibition of endothelial NF-kappaB signaling attenuates chronic intermittent hypoxia-induced atherosclerosis in mice. Atherosclerosis. (2018) 270:68–75. doi: 10.1016/j.atherosclerosis.2018.01.027
100. Xu, Y, Hu, X, Cai, J, Li, Y, Zou, Y, Wang, Y, et al. Atractylenolide-III alleviates osteoarthritis and chondrocyte senescence by targeting NF-kappaB signaling. Phytother Res. (2023) 37:4607–20. doi: 10.1002/ptr.7929
101. Qiao, S, Zhao, R, He, S, Fu, X, An, J, and Xia, T. Quercitrin attenuates the progression of osteoarthritis via inhibiting NF-kappaB signaling pathways and enhance glucose transport capacity. Exp Cell Res. (2023) 433:113854. doi: 10.1016/j.yexcr.2023.113854
102. Wang, H, Wang, W, Wang, J, Zhang, L, Luo, Y, and Tang, X. MicroRNA-15a/beta1,4-GalT-I axis contributes to cartilage degeneration via NF-kappaB signaling in osteoarthritis. Clinics (São Paulo). (2023) 78:100254. doi: 10.1016/j.clinsp.2023.100254
103. Li, W, Tao, C, Mao, M, and Zhu, K. The Nrf2/HMGB1/NF-kappaB axis modulates chondrocyte apoptosis and extracellular matrix degradation in osteoarthritis. Acta Biochim Biophys Sin Shanghai. (2023) 55:818–30. doi: 10.3724/abbs.2023078
104. Liu, C, Wang, H, Zhu, C, and Wang, S. Plasma expression of HIF-1alpha as novel biomarker for the diagnosis of obstructive sleep apnea-hypopnea syndrome. J Clin Lab Anal. (2020) 34:e23545. doi: 10.1002/jcla.23545
105. Gabryelska, A, Szmyd, B, Szemraj, J, Stawski, R, Sochal, M, and Bialasiewicz, P. Patients with obstructive sleep apnea present with chronic upregulation of serum HIF-1alpha protein. J Clin Sleep Med. (2020) 16:1761–8. doi: 10.5664/jcsm.8682
106. Gabryelska, A, Karuga, FF, Szmyd, B, and Bialasiewicz, P. HIF-1alpha as a mediator of insulin resistance, T2DM, and its complications: potential links with obstructive sleep apnea. Front Physiol. (2020) 11:1035. doi: 10.3389/fphys.2020.01035
107. Gabryelska, A, Sochal, M, Turkiewicz, S, and Bialasiewicz, P. Relationship between HIF-1 and circadian clock proteins in obstructive sleep apnea patients-preliminary Study. J Clin Med. (2020) 9:1599. doi: 10.3390/jcm9051599
108. Liu, W, Zhang, W, Wang, T, Wu, J, Zhong, X, Gao, K, et al. Obstructive sleep apnea syndrome promotes the progression of aortic dissection via a ROS- HIF-1alpha-MMPs associated pathway. Int J Biol Sci. (2019) 15:2774–82. doi: 10.7150/ijbs.34888
109. Xu, J, Li, Q, Xu, CY, Mao, S, Jin, JJ, Gu, W, et al. Obstructive sleep apnea aggravates neuroinflammation and pyroptosis in early brain injury following subarachnoid hemorrhage via ASC/HIF-1alpha pathway. Neural Regen Res. (2022) 17:2537–43. doi: 10.4103/1673-5374.339000
110. Prabhakar, NR, Peng, YJ, and Nanduri, J. Hypoxia-inducible factors and obstructive sleep apnea. J Clin Invest. (2020) 130:5042–51. doi: 10.1172/JCI137560
111. Semenza, GL, and Prabhakar, NR. Neural regulation of hypoxia-inducible factors and redox state drives the pathogenesis of hypertension in a rodent model of sleep apnea. J Appl Physiol (1985). (2015) 119:1152–6. doi: 10.1152/japplphysiol.00162.2015
112. Chen, Y, Zhao, B, Zhu, Y, and Zhao, H. and Ma C.HIF-1-VEGF-notch mediates angiogenesis in temporomandibular joint osteoarthritis. Am J Transl Res. (2019) 11:2969–82.
113. Zeng, CY, Wang, XF, and Hua, F. Z.HIF-1alpha in osteoarthritis: from pathogenesis to therapeutic implications. Front Pharmacol. (2022) 13:927126. doi: 10.3389/fphar.2022.927126
114. Sun, J, Song, X, Su, L, and Cao, S. Long non-coding RNA LncHIFCAR promotes osteoarthritis development via positively regulating HIF-1alpha and activating the PI3K/AKT/mTOR pathway. Int J Clin Exp Pathol. (2018) 11:3000–9.
115. Li, X, Mei, W, Huang, Z, Zhang, L, Zhang, L, Xu, B, et al. Casticin suppresses monoiodoacetic acid-induced knee osteoarthritis through inhibiting HIF-1alpha/NLRP3 inflammasome signaling. Int Immunopharmacol. (2020) 86:106745. doi: 10.1016/j.intimp.2020.106745
116. Zhang, L, Li, X, Zhang, H, Huang, Z, Zhang, N, Zhang, L, et al. Agnuside alleviates synovitis and fibrosis in knee osteoarthritis through the inhibition of HIF-1alpha and NLRP3 Inflammasome. Mediat Inflamm. (2021) 2021:5534614. doi: 10.1155/2021/5534614
117. Yang, H, Huang, J, Mao, Y, Wang, L, Li, R, and Ha, C. Vitexin alleviates interleukin-1beta-induced inflammatory responses in chondrocytes from osteoarthritis patients: involvement of HIF-1alpha pathway. Scand J Immunol. (2019) 90:e12773. doi: 10.1111/sji.12773
118. Song, J, Kang, YH, Yoon, S, Chun, CH, and Jin, E. J.HIF-1alpha:CRAT:miR-144-3p axis dysregulation promotes osteoarthritis chondrocyte apoptosis and VLCFA accumulation. Oncotarget. (2017) 8:69351–61. doi: 10.18632/oncotarget.20615
119. Chen, G, Liu, T, Yu, B, Wang, B, and Peng, Q. CircRNA-UBE2G1 regulates LPS-induced osteoarthritis through miR-373/HIF-1a axis. Cell Cycle. (2020) 19:1696–705. doi: 10.1080/15384101.2020.1772545
120. Guan, Z, Jin, X, Guan, Z, Liu, S, Tao, K, and Luo, L. The gut microbiota metabolite capsiate regulate SLC2A1 expression by targeting HIF-1alpha to inhibit knee osteoarthritis-induced ferroptosis. Aging Cell. (2023) 22:e13807. doi: 10.1111/acel.13807
121. Li, W, Wu, N, Wang, J, Wang, Y, Wu, M, and Wang, H. Role of HIF-2alpha/NF-kappaB pathway in mechanical stress-induced temporomandibular joint osteoarthritis. Oral Dis. (2022) 28:2239–47. doi: 10.1111/odi.13986
122. Chern, CM, Zhou, H, Wang, YH, Chang, CL, Chiou, WF, Chang, WT, et al. Osthole ameliorates cartilage degradation by downregulation of NF-kappaB and HIF-2alpha pathways in an osteoarthritis murine model. Eur J Pharmacol. (2020) 867:172799. doi: 10.1016/j.ejphar.2019.172799
123. Li, W, Cai, L, Zhang, Y, Cui, L, and Shen, G. Intra-articular resveratrol injection prevents osteoarthritis progression in a mouse model by activating SIRT1 and thereby silencing HIF-2alpha. J Orthop Res. (2015) 33:1061–70. doi: 10.1002/jor.22859
124. Zhou, X, Zheng, Y, Sun, W, Zhang, Z, Liu, J, Yang, W, et al. D-mannose alleviates osteoarthritis progression by inhibiting chondrocyte ferroptosis in a HIF-2alpha-dependent manner. Cell Prolif. (2021) 54:e13134. doi: 10.1111/cpr.13134
125. Lee, M, Won, Y, Shin, Y, Kim, JH, and Chun, JS. Reciprocal activation of hypoxia-inducible factor (HIF)-2alpha and the zinc-ZIP8-MTF1 axis amplifies catabolic signaling in osteoarthritis. Osteoarthr Cartil. (2016) 24:134–45. doi: 10.1016/j.joca.2015.07.016
126. Zhou, K, He, S, Yu, H, Pei, F, and Zhou, Z. Inhibition of syndecan-4 reduces cartilage degradation in murine models of osteoarthritis through the downregulation of HIF-2alpha by miR-96-5p. Lab Investig. (2021) 101:1060–70. doi: 10.1038/s41374-021-00595-5
127. Murahashi, Y, Yano, F, Kobayashi, H, Makii, Y, Iba, K, Yamashita, T, et al. Intra-articular administration of IkappaBalpha kinase inhibitor suppresses mouse knee osteoarthritis via downregulation of the NF-kappaB/HIF-2alpha axis. Sci Rep. (2018) 8:16475. doi: 10.1038/s41598-018-34830-9
128. Zhang, FJ, Luo, W, and Lei, GH. Role of HIF-1alpha and HIF-2alpha in osteoarthritis. Joint Bone Spine. (2015) 82:144–7. doi: 10.1016/j.jbspin.2014.10.003
129. Hu, S, Zhang, C, Ni, L, Huang, C, Chen, D, Shi, K, et al. Stabilization of HIF-1alpha alleviates osteoarthritis via enhancing mitophagy. Cell Death Dis. (2020) 11:481. doi: 10.1038/s41419-020-2680-0
130. Zhang, L, Zhang, L, Huang, Z, Xing, R, Li, X, Yin, S, et al. Increased HIF-1alpha in knee osteoarthritis aggravate synovial fibrosis via fibroblast-like Synoviocyte Pyroptosis. Oxidative Med Cell Longev. (2019) 2019:6326517. doi: 10.1155/2019/6326517
131. Wang, K, Li, Y, Dai, Y, Han, L, Zhu, Y, Xue, C, et al. Peptides from Antarctic krill (Euphausia superba) improve osteoarthritis via inhibiting HIF-2alpha-mediated death receptor apoptosis and metabolism regulation in osteoarthritic mice. J Agric Food Chem. (2019) 67:3125–33. doi: 10.1021/acs.jafc.8b05841
132. Zhou, Y, Ming, J, Deng, M, Li, Y, Li, B, Li, J, et al. Chemically modified curcumin (CMC2.24) alleviates osteoarthritis progression by restoring cartilage homeostasis and inhibiting chondrocyte apoptosis via the NF-kappaB/HIF-2alpha axis. J Mol Med (Berl). (2020) 98:1479–91. doi: 10.1007/s00109-020-01972-1
133. Fiedorczuk, P, Olszewska, E, Polecka, A, Walasek, M, Mroczko, B, and Kulczynska-Przybik, A. Investigating the role of serum and plasma IL-6, IL-8, IL-10, TNF-alpha, CRP, and S100B concentrations in obstructive sleep apnea diagnosis. Int J Mol Sci. (2023) 24:13875. doi: 10.3390/ijms241813875
134. Shalitin, S, Deutsch, V, and Tauman, R. Hepcidin, soluble transferrin receptor and IL-6 levels in obese children and adolescents with and without type 2 diabetes mellitus/impaired glucose tolerance and their association with obstructive sleep apnea. J Endocrinol Investig. (2018) 41:969–75. doi: 10.1007/s40618-017-0823-7
135. Lopez-Pascual, A, Lasa, A, Portillo, MP, Aros, F, Mansego, ML, Gonzalez-Muniesa, P, et al. Low oxygen consumption is related to a Hypomethylation and an increased secretion of IL-6 in obese subjects with sleep apnea-hypopnea syndrome. Ann Nutr Metab. (2017) 71:16–25. doi: 10.1159/000478276
136. Kurt, OK, Tosun, M, and Talay, F. Serum cardiotrophin-1 and IL-6 levels in patients with obstructive sleep apnea syndrome. Inflammation. (2013) 36:1344–7. doi: 10.1007/s10753-013-9673-4
137. Carpagnano, GE, Spanevello, A, Sabato, R, Depalo, A, Palladino, GP, Bergantino, L, et al. Systemic and airway inflammation in sleep apnea and obesity: the role of ICAM-1 and IL-8. Transl Res. (2010) 155:35–43. doi: 10.1016/j.trsl.2009.09.004
138. Chu, L, and Li, Q. The evaluation of adenotonsillectomy on TNF-alpha and IL-6 levels in obese children with obstructive sleep apnea. Int J Pediatr Otorhinolaryngol. (2013) 77:690–4. doi: 10.1016/j.ijporl.2013.01.019
139. Ferrari, M, Sica, E, De Bernardi, F, Luini, A, Legnaro, M, Nosetti, L, et al. Reduction of IL-6, IL-8 and beta2-ADRENOCEPTOR mRNA levels in circulating polymorphonuclear leukocytes after adenotonsillectomy in children with obstructive sleep apnea syndrome. Sleep Med. (2024) 114:82–5. doi: 10.1016/j.sleep.2023.12.017
140. Zhong, A, Xiong, X, Shi, M, and Xu, H. Roles of interleukin (IL)-6 gene polymorphisms, serum IL-6 levels, and treatment in obstructive sleep apnea: a meta-analysis. Sleep Breath. (2016) 20:719–31. doi: 10.1007/s11325-015-1288-6
141. Ohga, E, Tomita, T, Wada, H, Yamamoto, H, Nagase, T, and Ouchi, Y. Effects of obstructive sleep apnea on circulating ICAM-1, IL-8, and MCP-1. J Appl Physiol (1985). (2003) 94:179–84. doi: 10.1152/japplphysiol.00177.2002
142. Jacob, J, Aggarwal, A, Aggarwal, A, Bhattacharyya, S, Kumar, V, Sharma, V, et al. Senescent chondrogenic progenitor cells derived from articular cartilage of knee osteoarthritis patients contributes to senescence-associated secretory phenotype via release of IL-6 and IL-8. Acta Histochem. (2022) 124:151867. doi: 10.1016/j.acthis.2022.151867
143. Mima, Z, Wang, K, Liang, M, Wang, Y, Liu, C, Wei, X, et al. Blockade of JAK2 retards cartilage degeneration and IL-6-induced pain amplification in osteoarthritis. Int Immunopharmacol. (2022) 113:109340. doi: 10.1016/j.intimp.2022.109340
144. Keller, LE, Tait Wojno, ED, Begum, L, and Fortier, LA. Regulatory T cells provide chondroprotection through increased TIMP1, IL-10 and IL-4, but cannot mitigate the catabolic effects of IL-1beta and IL-6 in a tri-culture model of osteoarthritis. Osteoarthr Cartil Open. (2021) 3:100193. doi: 10.1016/j.ocarto.2021.100193
145. Negishi, Y, Adili, A, de Vega, S, Momoeda, M, Kaneko, H, Cilek, MZ, et al. IL-6 reduces spheroid sizes of Osteophytic cells derived from osteoarthritis knee joint via induction of apoptosis. Am J Pathol. (2024) 194:135–49. doi: 10.1016/j.ajpath.2023.10.005
146. He, J, Wang, L, Ding, Y, Liu, H, and Zou, G. lncRNA FER1L4 is dysregulated in osteoarthritis and regulates IL-6 expression in human chondrocyte cells. Sci Rep. (2021) 11:13032. doi: 10.1038/s41598-021-92474-8
147. Liu, JF, Chi, MC, Lin, CY, Lee, CW, Chang, TM, Han, CK, et al. PM2.5 facilitates IL-6 production in human osteoarthritis synovial fibroblasts via ASK1 activation. J Cell Physiol. (2021) 236:2205–13. doi: 10.1002/jcp.30009
148. Hou, CH, Tang, CH, Chen, PC, and Liu, JF. Thrombospondin 2 promotes IL-6 production in osteoarthritis synovial fibroblasts via the PI3K/AKT/NF-kappaB Pathway.J. Inflamm Res. (2021) 14:5955–67. doi: 10.2147/JIR.S314747
149. Chiu, YS, Bamodu, OA, Fong, IH, Lee, WH, Lin, CC, Lu, CH, et al. The JAK inhibitor Tofacitinib inhibits structural damage in osteoarthritis by modulating JAK1/TNF-alpha/IL-6 signaling through Mir-149-5p. Bone. (2021) 151:116024. doi: 10.1016/j.bone.2021.116024
150. Zhao, X, Kim, D, Suminda, GGD, Min, Y, Yang, J, Kim, M, et al. Inhibitory effects of IL-6-mediated matrix Metalloproteinase-3 and -13 by Achyranthes japonica Nakai root in osteoarthritis and rheumatoid arthritis mice models. Pharmaceuticals (Basel). (2021) 14:776. doi: 10.3390/ph14080776
151. Liang, T, Chen, T, Qiu, J, Gao, W, Qiu, X, Zhu, Y, et al. Inhibition of nuclear receptor RORalpha attenuates cartilage damage in osteoarthritis by modulating IL-6/STAT3 pathway. Cell Death Dis. (2021) 12:886. doi: 10.1038/s41419-021-04170-0
152. Lopez, J, Al-Nakkash, L, Broderick, TL, Castro, M, Tobin, B, and Plochocki, JH. Genistein suppresses IL-6 and MMP-13 to attenuate osteoarthritis in obese diabetic mice. Meta. (2023) 13:1014. doi: 10.3390/metabo13091014
153. Su, W, Zheng, X, Zhou, H, Yang, S, and Zhu, X. Fibroblast growth factor 10 delays the progression of osteoarthritis by attenuating synovial fibrosis via inhibition of IL-6/JAK2/STAT3 signaling in vivo and in vitro. Mol Immunol. (2023) 159:46–57. doi: 10.1016/j.molimm.2023.04.001
154. Lin, Z, Liu, T, Hu, Z, Que, W, Qiu, H, and Chen, L. Effects of different running intensity on serum levels of IL-6 and TNF-alpha in patients with early knee osteoarthritis. J Coll Physicians Surg Pak. (2022) 32:899–903. doi: 10.29271/jcpsp.2022.07.899
155. Yang, X, Guo, H, Ye, W, Yang, L, and He, C. Pulsed electromagnetic field attenuates osteoarthritis progression in a murine destabilization-induced model through inhibition of TNF-alpha and IL-6 signaling. Cartilage. (2021) 13:1665S–75S. doi: 10.1177/19476035211049561
156. Ruan, G, Xu, J, Wang, K, Zheng, S, Wu, J, Bian, F, et al. Associations between serum IL-8 and knee symptoms, joint structures, and cartilage or bone biomarkers in patients with knee osteoarthritis. Clin Rheumatol. (2019) 38:3609–17. doi: 10.1007/s10067-019-04718-8
157. Chauffier, K, Laiguillon, MC, Bougault, C, Gosset, M, Priam, S, Salvat, C, et al. Induction of the chemokine IL-8/kc by the articular cartilage: possible influence on osteoarthritis. Joint Bone Spine. (2012) 79:604–9. doi: 10.1016/j.jbspin.2011.12.013
158. Katsantonis, GP, Friedman, WH, Krebs, FJ, and Walsh, JK. Nasopharyngeal complications following uvulopalatopharyngoplasty. Laryngoscope. (1987) 97:309–14. doi: 10.1288/00005537-198703000-00009
159. Diaz, K, Faverio, P, Hospenthal, A, Restrepo, MI, Amuan, ME, and Pugh, MJ. Obstructive sleep apnea is associated with higher healthcare utilization in elderly patients. Ann Thorac Med. (2014) 9:92–8. doi: 10.4103/1817-1737.128854
160. Shen, P, Li, S, Li, L, Fong, DTP, Mao, D, and Song, Q. Balance control is sequentially correlated with proprioception, joint range of motion, strength, pain, and plantar tactile sensation among older adults with knee osteoarthritis. Sports Med Open. (2024) 10:70. doi: 10.1186/s40798-024-00735-3
161. Curran, F, Davis, ME, Murphy, K, Tersigni, N, King, A, Ngo, N, et al. Correlates of physical activity and sedentary behavior in adults living with overweight and obesity: a systematic review. Obes Rev. (2023) 24:e13615. doi: 10.1111/obr.13615
162. Reutrakul, S, and Mokhlesi, B. Obstructive sleep apnea and diabetes: a state of the art review. Chest. (2017) 152:1070–86. doi: 10.1016/j.chest.2017.05.009
163. Simmons, FB, Guilleminault, C, and Silvestri, R. Snoring, and some obstructive sleep apnea, can be cured by oropharyngeal surgery. Arch Otolaryngol. (1983) 109:503–7. doi: 10.1001/archotol.1983.00800220009003
164. Li, HY, Wang, PC, Lee, LA, Chen, NH, and Fang, TJ. Prediction of uvulopalatopharyngoplasty outcome: anatomy-based staging system versus severity-based staging system. Sleep. (2006) 29:1537–41. doi: 10.1093/sleep/29.12.1537
165. D'Amico, D, Olmer, M, Fouassier, AM, Valdes, P, Andreux, PA, Rinsch, C, et al. Urolithin a improves mitochondrial health, reduces cartilage degeneration, and alleviates pain in osteoarthritis. Aging Cell. (2022) 21:e13662. doi: 10.1111/acel.13662
166. Li, J, Jiang, M, Yu, Z, Xiong, C, Pan, J, Cai, Z, et al. Artemisinin relieves osteoarthritis by activating mitochondrial autophagy through reducing TNFSF11 expression and inhibiting PI3K/AKT/mTOR signaling in cartilage. Cell Mol Biol Lett. (2022) 27:62. doi: 10.1186/s11658-022-00365-1
167. Lee, AR, Woo, JS, Lee, SY, Na, HS, Cho, KH, Lee, YS, et al. Mitochondrial transplantation ameliorates the development and progression of osteoarthritis. Immune Netw. (2022) 22:e14. doi: 10.4110/in.2022.22.e14
168. Kim, HR, Cho, HB, Lee, S, Park, JI, Kim, HJ, and Park, KH. Fusogenic liposomes encapsulating mitochondria as a promising delivery system for osteoarthritis therapy. Biomaterials. (2023) 302:122350. doi: 10.1016/j.biomaterials.2023.122350
Keywords: sleep apnea syndrome, osteoarthritis, obesity, age, comorbidity
Citation: Weng L, Luo Y, Luo X, Yao K, Zhang Q, Tan J and Yin Y (2024) The common link between sleep apnea syndrome and osteoarthritis: a literature review. Front. Med. 11:1401309. doi: 10.3389/fmed.2024.1401309
Edited by:
Peng Li, University of Michigan, United StatesReviewed by:
Luiz Menezes-Junior, Universidade Federal de Ouro Preto, BrazilZoya Serebrovska, National Academy of Sciences of Ukraine, Ukraine
Jihyun Song, The University of Utah, United States
Copyright © 2024 Weng, Luo, Luo, Yao, Zhang, Tan and Yin. This is an open-access article distributed under the terms of the Creative Commons Attribution License (CC BY). The use, distribution or reproduction in other forums is permitted, provided the original author(s) and the copyright owner(s) are credited and that the original publication in this journal is cited, in accordance with accepted academic practice. No use, distribution or reproduction is permitted which does not comply with these terms.
*Correspondence: Yiran Yin, eWlyYW55aW5Ac3dtdS5lZHUuY24=
†These authors have contributed equally to this work