- 1Experimental Ophthalmo-Biology Group, Department of Cell Biology and Histology, University of Basque Country UPV/EHU, Leioa, Spain
- 2Begiker-Ophthalmology Research Group, BioCruces Health Research Institute, Cruces Hospital, Barakaldo, Spain
The retina is a highly heterogeneous tissue, both cell-wise but also regarding its extracellular matrix (ECM). The stiffness of the ECM is pivotal in retinal development and maturation and has also been associated with the onset and/or progression of numerous retinal pathologies, such as glaucoma, proliferative vitreoretinopathy (PVR), age-related macular degeneration (AMD), epiretinal membrane (ERM) formation or uveitis. Nonetheless, much remains unknown about the biomechanical milieu of the retina, and specifically the role that Müller glia play as principal mechanosensors and major producers of ECM constituents. So far, new approaches need to be developed to further the knowledge in the field of retinal mechanobiology for ECM-target applications to arise. In this review, we focus on the involvement of Müller glia in shaping and altering the retinal ECM under both physiological and pathological conditions and look into various biomaterial options to more accurately replicate the impact of matrix stiffness in vitro.
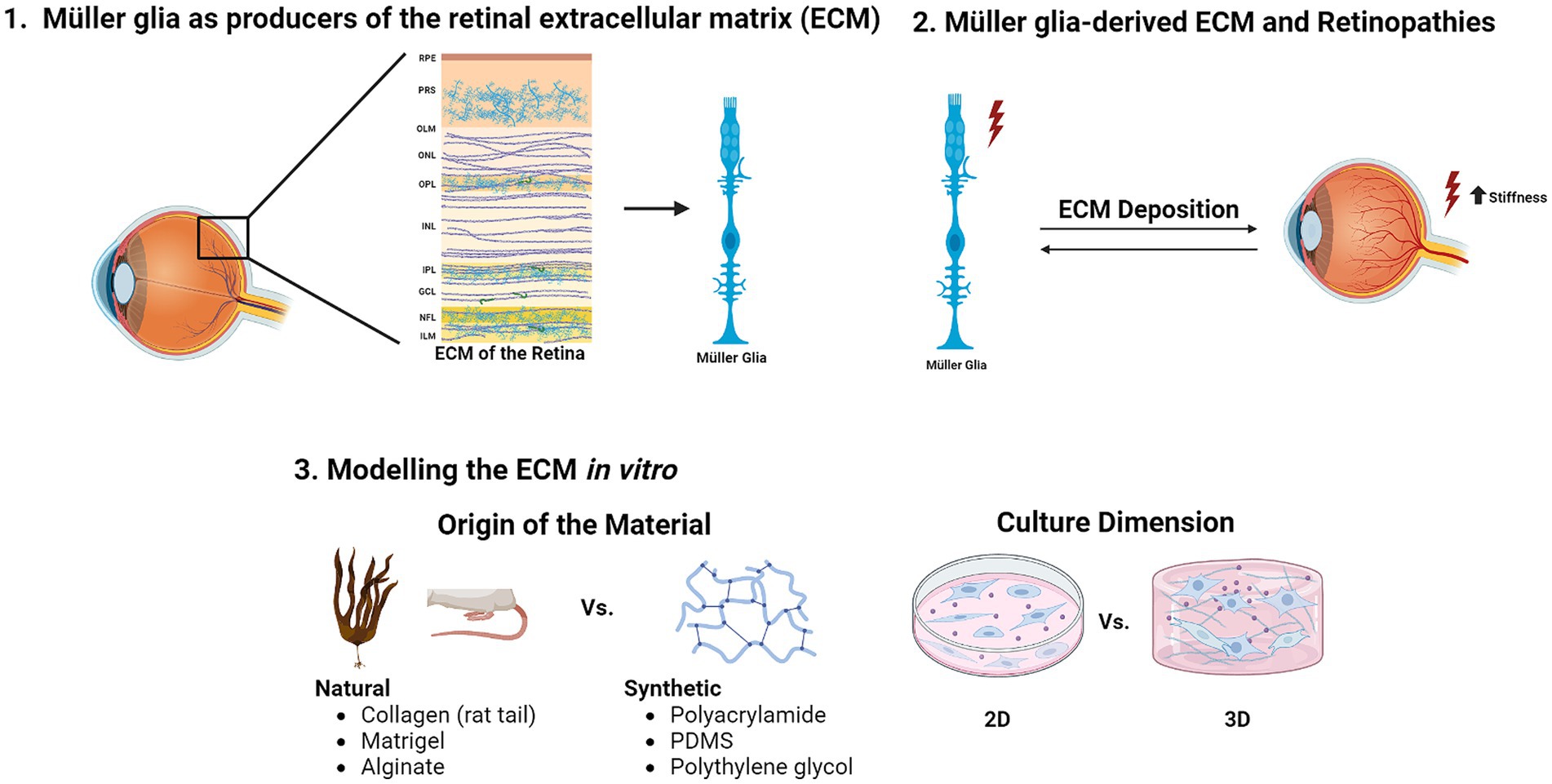
Graphical Abstract. Visual summary illustrating the key sections discussed in this review. Created with BioRender.com.
1 Introduction
Over the last decades, the focus has been put on deciphering how biochemical cues control tissue formation and homeostasis. However, the significance of the physical microenvironment itself has been overlooked. Therefore, by harnessing the unique properties of biomaterials to create in vitro models, research is taking a further step in understanding how biomechanical factors influence tissue behaviour, particularly in those subjected to intense mechanical stimuli, such as the retina.
The retina is a tissue belonging to the central nervous system (CNS), responsible for the reception of light stimuli and their transformation into electrical signals (1). Due to its location at the back of the eye, where it is only attached to the eyeball at the ora serrata and at the optic nerve head, it is constantly exposed to physical stresses.
The retina endures both static pressures, i.e., the negative pressure pulling on the outer retinal surface exerted by the retinal pigment epithelium (RPE) pumping fluid from the retina to the choroid and the positive pressure on the inner retinal surface created by the tamponade effect of the vitreous body. However, it also suffers a more dynamic pressure; since the vitreous body is attached to both the lens and the retina, it exerts mechanical forces at the retinal surface and at their adhesion sites as the result of every eye movement (2).
Regarding the tissue itself, the retina consists of different layers of intricately shaped cells, extracellular matrix (ECM) and blood vessels, where variations in their composition and distribution suggest a highly mechanically heterogeneous environment (3). For example neurons, including retinal ganglion cells (RGCs), which are the neurons in charge of transmitting the visual information to the brain, while stiff cells themselves require a more compliant environment — i.e. a soft substrate—for their neurites to grow (4, 5). Conversely, Müller glia, the main glial cell type in the retina, despite being softer than RGCs favour a less compliant substrate (3, 5) since it allows them to stretch and be in contact with the different retinal layers. Indeed, Müller glia have been found to be an optimal substrate for RGCs to grow on (6, 7), also promoting neuritogenesis among different species via secretion of neuroprotective factors [for a more comprehensive review of the neuroprotective role of Müller glia see García and Vecino (8) and Vecino et al. (9)].
In this context, the retinal ECM, the acellular part of the tissue on which cells grow, plays a key role in the homeostais of the retina (10). Specifically its rigidity or stiffness (i.e., its capability to resist deformation in response to an applied force) (11) emerges as a determining factor under both retinal physiological and pathologic conditions. In retinal development and homeostasis the ECM rigidity allows the correct organization of the different cell layers and cell differentiation and maturation (12–14). However, in many retinal pathologies, such as diabetic retinopathy (15), age-related macular degeneration (16), proliferative vitreoretinopathy (17) or the formation of epiretinal membranes (18), ECM remodelling and abnormal deposition is involved. Thus, the control of the ECM stiffness plays a key role in regulating the morphology, gene expression, differentiation and overall health of the different retinal cell types (19).
Over the last 20 years, many protein families that respond to mechanical forces (compression, tension, stiffness…) have been discovered. Particularly, several members of the TRP (transient receptor potential) superfamily, first discovered in 1969 (20), were later on found to be mechanosensitive (21). Likewise, in 2010, the Patapoutian group discovered two transmembrane cation channels, Piezo1 and Piezo 2, which were mechanically activated and involved in pressure sensing (22). Focusing on the retina, different studies show that both the tissue itself and adjoining tissues express multiple types of mechanosensitive TRP, particularly TRPV4 and TRPV1 and both Piezo channels (23–26).
Müller glia display long processes and side branches, spanning the entire neural retinal thickness, and thus being in contact with all retinal neuron cell types. Thanks to their privileged location and morphology, not only they provide the necessary tensile strength to maintain tissue integrity (27, 28) but they are also able to sense and respond to even minimal mechanical changes in the retinal structure. Indeed, Müller glia express both pressure/stretch-activated mechanoreceptors TRPV4, Piezo1, and to a lesser extent Piezo 2 (29), which upon activation elicit both a fast response via intracellular calcium signaling and a slow response via changes in protein expression, adapting the environment for their neighbouring neurons (30). Thus, Müller glia are the main retinal mechanosensors and also the major producers of ECM constituents (13, 31). The ECM plays a vital role in Müller glia activation and subsequent gliotic process (32). For this reason, Müller glia should be more closely studied to better understand the mechanical behaviour of the retina.
Although in vivo studies ideally would be better to look into the retinal ECM, and indeed several disease animal models have been used to that end (33, 34), in this type of studies there are many variables that difficult the study of a particular mechanical parameter. Thus, biomaterials emerge as a good alternative for in vitro models that allow a tight control of the substrate stiffness while more closely recapitulating physiological ECM conditions than traditional cultures.
In this comprehensive review, we aim to deepen our understanding of the retinal physical environment. We will provide context on the distinct characteristics of the retinal ECM and elucidate the impact of matrix stiffening on the onset and progression of common retinal pathologies, focusing on the role played by Müller glia as major mechanosensors and ECM contributors. The characteristics of different biomaterials suitable for in vitro modelling of the retinal ECM to further advance our understanding of its biomechanical properties will be explored.
2 The ECM of the retina
The ECM forms a complex yet organized network that surrounds the cells and confers structural and mechanical support to tissues. It is a highly dynamic structure in constant change which creates a complex milieu for the cells, regulating cellular homeostasis and signaling both through biochemical signals (e.g., hormones, growth factors and diffusible morphogens) and mechanical cues (e.g., matrix stiffness; tensile, compressive and shear forces; topographical strain) (13, 35, 36).
The ECM transmits mechanical cues to resident retinal cells and guides their fate by modulating cell growth and differentiation (37). Meanwhile, the cells, primarily Müller glia and astrocytes, can change the composition and macromolecular network structure by secreting matrix components and matrix metalloproteinases (MMPs) or exerting mechanical forces to regulate the arrangement of the ECM (18) (Figure 1).
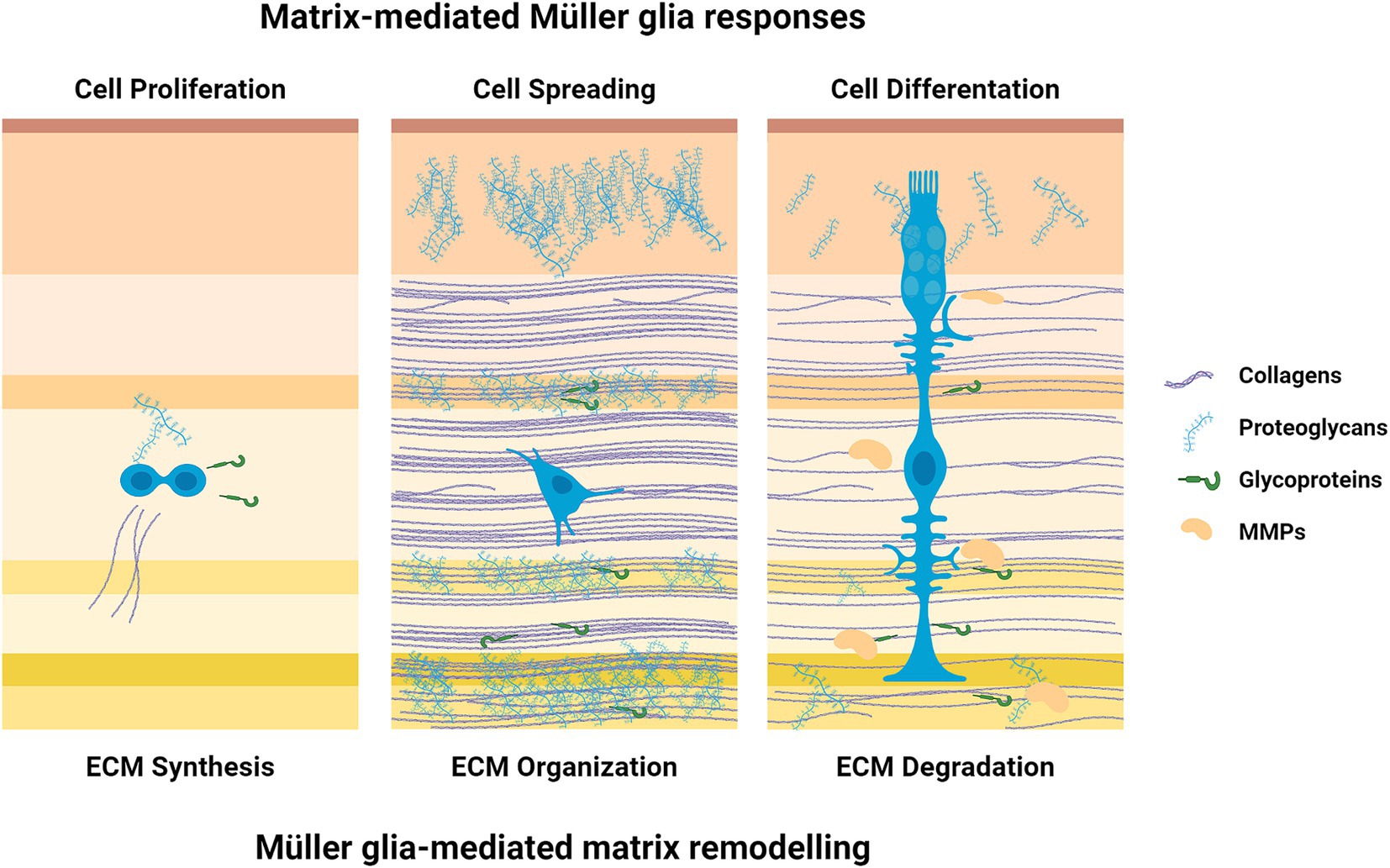
Figure 1. Cell-matrix interactions. There is a two-way communication between the ECM and Müller glia; ECM influences the celular outcome while those same cells organize the ECM. Created with BioRender.com.
Müller glia, established as the retina main mechanosensors (30) respond to shifts in the stiffness of the substrate through a mechanical–chemical process that consists in the mechanosensation, mechanotransduction, and downstream mechanoresponses to rigidity stimulus (38). At first, focal adhesions, nanoscaled mechanosensors located on the cell membrane, are formed to link the Müller glia cytoskeleton to the substrate. Then, the rigidity of the microenvironment is sensed and transduced into biochemical signals, triggering changes in cell behaviours, including cell morphology and differentiation (12, 39, 40), and the shuttling of cytoplasmic proteins, such as YAP (yes-associated protein) and TAZ (tafazzin; YAP co-activator), to the nucleus to further regulate cell response has also been linked to Müller glia mechanoresponse (15, 17). Upon increased substrate stiffness, Müller glia activate actin filament extension, leading to cell hypertrophy, and upregulate the production of ECM proteins (41).
2.1 Components of the retinal ECM secreted by Müller glia
In the retina, ECM components have been mainly associated with basal membranes (BMs), but also with non-BMs (42). The retinal ECM is composed mainly of collagens, proteoglycans and glycoproteins (Figure 2) as seen summarized in Table 1.
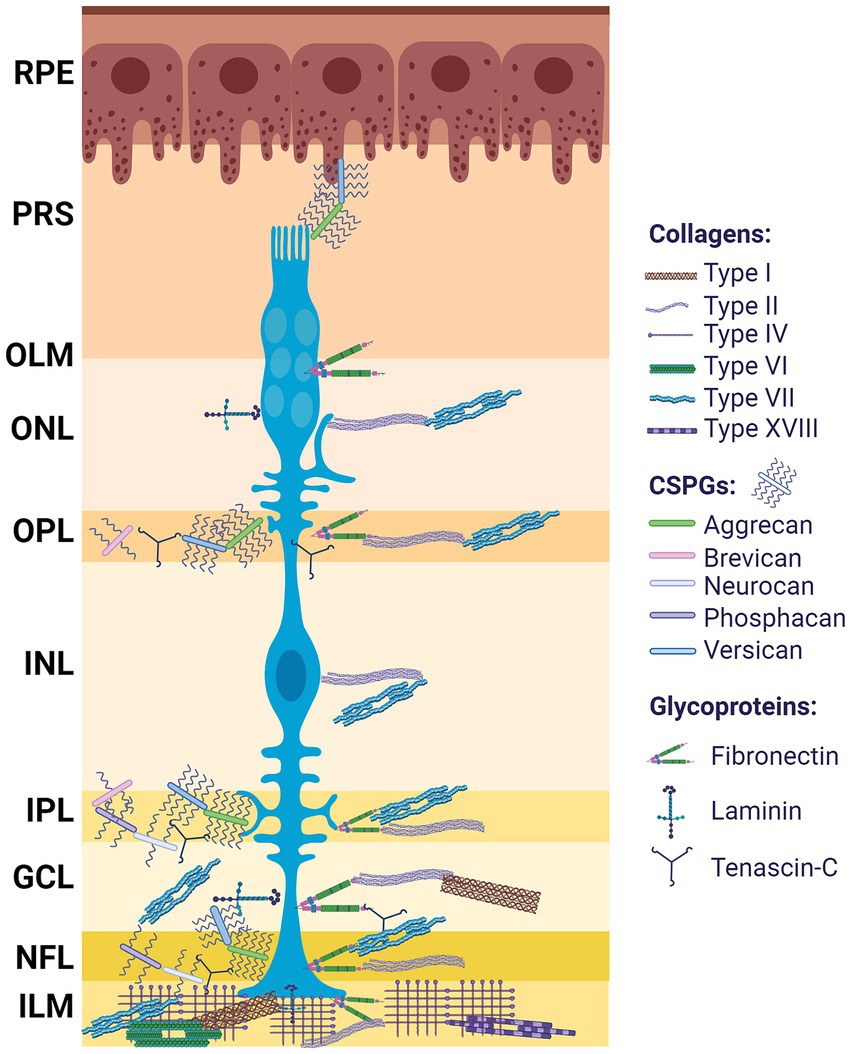
Figure 2. ECM distribution in the retina. Differential distribution of ECM components among the retinal layers; from outermost to innermost: retinal pigment epithelium (RPE), photoreceptor segment (PRS), outer limiting membrane (OLM), outer nuclear layer (ONL), outer plexiform layer (OPL), inner nuclear layer (INL), inner plexiform layer (IPL), ganglion cell layer (GCL), nerve fiber layer (NFL), optic nerve (ON). Müller glia (blue). Created with BioRender.com.
2.1.1 Collagens
Collagens are the main components of the ECM of most soft tissues, including the retina, where they form a fibrillar net in charge of maintaining its structural strength, its attachment to the vitreous and the retinal vasculature (43). The main collagens in the retina secreted by Müller glia are collagen types I-VII and XVIII.
Collagen I is the most common fibril-forming collagen in vertebrates. It is both synthesised by retinal astrocytes in direct contact with RGCs and Müller glia (44), thus being an integral part of the ganglion cell layer (GCL) and the inner limiting membrane (ILM), a membrane mostly comprise of Müller glia end-feet that separates the vitreous from the retina. Upregulation of collagen I has been linked as a response to IOP elevation (45) and as a major component of the glial scar in the wound healing process (46).
Collagen II has been found mainly around retinal blood vessels, as part of the ECM sheath that encapsulates retinal vasculature (47) but also as isolated deposits in the retina, which have been suggested to intervene in the remodelling of the ILM and the collagen intraretinal network by associating with Müller glia via integrin α1β1 and α2β1 (48, 49). Although in the ILM collagen II is mainly generated by the vitreous-originated hyalocytes (50), Müller glia express the transcription factor Sox9, which directly regulates the gene encoding collagen II (51).
Collagen IV is secreted by Müller glia and is the principal ECM component of the ILM of the retina, comprising approximately 60% of its total proteins (45, 52). Due to its structural role, it has been reported to participate in RGCs survival by maintaining ILM integrity, as well as in retinal angiogenesis, where it is part of the collagen network surrounding new vessels (31, 47, 53). It has also been found in the Bruch’s membrane, anchoring the RPE and intervening in its differentiation and wound healing (54, 55).
Collagens VI, VII, and XVIII are secreted by Müller glia in the ILM of the retina which suggest a role in vitreoretinal attachment due to their matrix anchoring function (56). Collagen VI has also been described in retinal blood vessels and collagen VII is found in most retina layers (NFL, GCL, IPL, INL, OPL, and ONL), especially at the optic nerve head. Furthermore, endostatin, proteolytically derived from Collagen XVIII, has been associated with a protection against neovascularization and maintaining RPE integrity (57).
2.1.2 Proteoglycans
Proteoglycans are composed of a core protein on which long glycosaminoglycan (GAG) chains are attached (58). Depending on which disaccharide conforms said chains, proteoglycans are classified as: hyaluronan (HPGs), chondroitin sulfate (CSPGs), dermatan sulfate (DSPGs), heparan sulfate (HSPGs) and keratan sulfate (KSPGs).
In retinal tissues, CSPGs are secreted primarily from the retinal cells per se, and constitute the ECM, whereas other types of proteoglycans including HSPGs, that also predominantly expressed in the retina, remain bound to the cell membrane and do not contribute to the formation of the ECM (42). Especially during retinal development, CSPGs represent major constituents of the ECM, being found in all plexiform layers of the retina and contributing to neural network formation by creating inhibitory boundaries that direct RGC axons. In the adult CNS, CSPGs are typically upregulated in response to injury or neurodegeneration, blocking axonal and cell migration and regeneration as part of the glial scar, created by Müller glia in the retina (59, 60). In the CNS aggrecan, versican, brevican, neurocan and phosphacan are the most common CSPGs (58).
Versican and aggrecan are released by both neurons and Müller glia and are located in the inner plexiform layer (IPL) and the GCL of the retina (61), as well as the photoreceptor segment (PRS) and the outer plexiform layer (OPL) (62). Versican plays an important role in the attachment of the neural retina to the RPE (63). The main role of aggrecan is to maintain RGC structure in the IPL and to provide support to photoreceptors (64), which is compromised in gliotic conditions, where Müller glia expression of aggrecan is downregulated (65). Both aggrecan and versican are reported to be more prevalent in ageing and degeneration models, probably due to a higher content of GAG chains compared to other CSPGs that may make up an inhibitory barrier (62).
Brevican is secreted by Müller glia and proliferating astrocytes in development and gliosis in the plexiform layers of the retina, where it stabilizes neural synapsis, and in association with RGC somata (61, 66). In physiological conditions its expression is tightly controlled by Müller glia via the miRNA miR-9, which appears to be essential to maintaining retinal integrity. Indeed, in a genetic model where miR-9 regulation was lost, brevican overexpressing Müller glia aggregated, migrated to abnormal locations in the OPL, the outer nuclear layer (ONL), and the IPL and lost cell polarity, leading to a loss of retinal tensile strength and overall retinal disorganization (67).
Neurocan and phosphacan have been suggested to being involved in the establishment and upkeep of retinal lamination (62). During retinal development, both CSPGs are highly associated with the IPL and nerve fiber layer (NFL) (42), where they display an inhibitory effect on RGCs neurite outgrowth (68). In the adult retina, phosphacan expression is restricted to Müller glia (13), where it has been linked to playing a role in the formation of the glial scar during reactive gliosis as a consequence of RGC damage in a genetic glaucomatous model (33).
2.1.3 Glycoproteins
Fibronectin is a glycoprotein, which N-terminal portion contains a self-assembly domain that allow the formation of a fibronectin matrix. As such, fibronectin functions as a regulator of cellular processes, and directs and maintains tissue organization and ECM composition and remodelling. For example, type I, III, and IV collagens depend on fibronectin for their incorporation into the ECM; it has been observed that although integrins α11β1 and α2β1 are able to promote fibrillogenesis of type I and type III collagens, a collagen network cannot be formed in the abscence of a preconstituted fibronectin matrix and, more so fibronectin is required to maintain the composition of cell–matrix adhesion sites (19). During retinal development, Müller glia and astrocyte-secreted fibronectin has been found in the interstitial matrix of the retina acting as scaffolding, and its disruption has been linked to the dissolution of retinal layers, neuronal cell death and Müller glia activation (69). Fibronectin has also been shown to be key in endothelial radial migration during mouse retinal angiogenesis (70). However, in the adult retina fibronectin seems to be restricted to a structural component of the RPE and retinal vessels (19), as well as act as anchors of Müller glia end-feet to the ILM (71, 72). Interestingly in in vitro studies the fibronectin receptor α5β1 integrin, has been linked to neurite outgrowth of RGCs grown on various ECM substrates as a model for RGC regeneration in the mature retina (73).
Laminins are a family of heterotrimeric glycoproteins, composed of one α, one β and one γ chain, which play an important role in multiple biological processes such as adhesion, differentiation, migration, and resistance to apoptosis via cell membrane receptor signaling (74, 75). Members of the laminin family, mainly γ3, α4 and α5 are major components of the retinal vascular basement membrane and play a functional role in its differentiation and maintenance (76). Laminin has been observed in the ganglion cell layer (GCL) and in close association with Müller glia end-feet in the ILM (73, 77). Müller glia is considered the main producer of laminin in the developing rat retina, and specifically the β2 and γ3 laminin chains are key to the formation and stability of the ILM and retinal lamination during development, while their disruption has been linked to Müller glia disorganization, and consequently RGC apoptosis (53, 77). These two laminin chains have also been connected to photoreceptor synapsis formation and the organization of the outer nuclear layer (ONL) (78). Furthermore, it has been observed that laminin stimulates the motility of Müller glia through the activation of its receptor dystroglycan (79). Additionally, in cultures of both mature RGCs and Müller glia, laminin (plus poli-L-lysine) yielded the greatest survival rate in different substrates (73, 80).
Tenascin-C (tnc) is a glycoprotein expressed by horizontal and amacrine cells in the plexiform layers of the retina, as well as astrocytes in the optic nerve, exhibiting both adhesive and anti-adhesive properties (13, 81). During development of the CNS, tenascin-C regulates neurite outgrowth and glial cell differentiation (82, 83), and it also acts as a barrier in the optic nerve preventing oligodendrocyte precursors from migrating to the retina (84). However, upon retinal damage the expression of tenascin-C by Müller glia is upregulated, which has been linked to contribute to ECM deposition, retinal mechanical stiffness and a gliotic environment, photoreceptor degeneration and neuroinflammation (40, 85–87). This molecule can interact with other ECM components such as fibronectin and proteoglycans such as aggrecan and neurocan (88), and it has been proposed to promote the generation of stable focal adhesions between Müller glia and the ECM (89), being implicated in the morphology of Müller glia. Interestingly, the effect of tenascin-C on Müller glia mediated neurite outgrowth depends on which Tnc-derived fibronectin type III (TNfn) domains they express, with TNfn domain D being required to promote retinal outgrowth by Müller glia (90, 91). Additionally, tenascin-c has been found to increase Müller glia sensitivity to fibroblast growth factor 2 (FGF2), a factor capable of inducing the dedifferentiation of Müller glia in vitro, and tenascin-c knock-out has been reported to impair FGF2 effect on the dedifferentiation state of Müller glia (89).
2.2 Remodelling of the ECM
Glial cells are constantly remodelling the ECM through synthesis, degradation, reassembly and chemical modification (92). The main process in ECM remodelling is the cleavage of ECM components, which regulates ECM abundance, composition and structure, and leads to the release of biologically active molecules (e.g., growth factors) that can in turn influence ECM architecture and cell behaviour (93). Different families of proteases can cleave the retinal ECM, the main being matrix metalloproteinase and the adamalysin families.
2.2.1 Matrix metalloproteinases
Matrix metalloproteinases (MMPs) are calcium-dependent endopeptidases that cleave structural motifs of their substrates (e.g., collagens, CSPGs) and have major roles in ECM remodelling, neovascularization and wound healing (94). Depending on their substrate and structural domains, MMPs are classified into: collagenases (MMP-1, −8, −13, −18); gelatinases (MMP-2 and MMP-9); stromelysins (MMP-3, −10, −11); matrilysins (MMP-7, −26); membrane-type matrix metalloproteinases (MT1/2/3/4/5/6-MMP); and unclassified MMPs (MMP-12, −19, −20, −21, −23, −27, −28) (95). Their expression levels in healthy tissue are generally low, and are characterized by an autoinhibitory prodomain that keeps the MMP in an inactive state (96). They are also tightly regulated by the expression of endogenoues tissue inhibitors of metalloproteinases (TIMPs). In the retina, the main MMPs expressed are MMP-2, −3 and-9 (97).
MMP-2 is the main gelatinase in the retina, where it is constitutively expressed by Müller glia (98) and is activated by the release of MT1-MMP (also known as MMP-14) by RGCs. Different functional studies have identified MMP-2 as a key player in axonal regeneration both via resolution of the glial scar and/or proteolysis of molecular cues that guide axon outgrowth. Indeed, in a retinal progenitor cell (RPC) transplantation study, it was observed that neurite outgrowth was dependent on the activation of MMP-2, which cleaves the CSPG neurocan, thus disrupting its inhibitory effect (99). This was further confirmed in a mouse retinal explant model, where MMP-2 was found to act at the growth cone via a β1-integrin-dependent pathway (100). Furthermore, in a mouse optic nerve crush model, it has been proposed that MMP-2 produced by inflammatory myeloid cells activates Müller glia, which in turn produce anti-inflammatory molecules, such as ciliary neurotrophic factor (CNTF) and interleukin-6 (IL-6), as well as increased levels of MMP-2 that could activate certain intrinsic growth-inducing pathways in the RGCs (101), although the specific mechanisms are yet to be elucidated. MMP-2 is also capable of degrading collagen IV (102), thus altering the integrity of the ILM.
MMP-3, mainly expressed by the microglia and in loaded vesicles across Müller glia (97). Its expression is tightly connected to retinal vasculature homeostasis, although its involvement is controversial. While some studies report that MMP-3 contributes to blood-retinal barrier (BRB) disruption and neuroinflammation by degrading tight junction proteins and stimulating the activation of cytokines (103, 104), a more recent study showed that upon inflammation MMP-3 expression at the glia limitans (a barrier formed by the end feet of astrocytes and Müller glia that surround the BRB) is upregulated by Müller glia and promotes the expression of astrocyte-specific tight junction proteins, which in turn tighten the glia limitans reducing leukocyte infiltration and adhesion to the retina (105). Moreover, MMP-3 appears to cleave and inactivate the chemokine CCL2 further restricting leukocyte infiltration.
MMP-9, as well as its endogenous inhibitor TIMP-1, is expressed in the nuclei of Müller glia. In healthy retinas, MMP-9 and TIMP-1 form an axis in which TIMP-1 tightly regulates the expression of active MMP-9 (97). MMP-9 primarily cleaves collagen IV and the MMP-9/TIMP-1 axis appears to be key in maintaining normal retinal blood vessel structure and basement membrane integrity (97, 106). However, it has been reported that upon inflammation TIMP-1 tends to aggregate in the cytoplasm of Müller glia, impairing its secretion and reducing TIMP-1 abundance to bind to and inhibit MMP-9 in vitro (107). In this context, the overactivation of MMP-9 plays an important role in the promotion of detachment-induced RGC death by excessive degradation of collagen IV, and indeed, its expression has been observed in apoptosing RGCs (97). Furthermore, MMP-9 have been found to cleave both RPE tight junction proteins (108) and blood vessel tight junction protein ZO-2 (106), contributing to the disruption of the BRB integrity and the progression of chronic inflammation. Interestingly, upon photoreceptor damage in a zebrafish model, MMP-9 has been shown to be required for the survival of regenerated cones by modulating the inflammatory response and negatively regulating Müller glia-derived progenitors overproliferation (109).
2.2.2 Adamalysins
Adamalysins are a family of zinc-dependent metallopeptidases conformed by transmembrane-A-disintegrin and metalloproteinases (ADAMs), and secreted A-disintegrin and metalloproteinase with thrombospondin type I motifs (ADAMTSs) that play a key role in ECM remodelling (110).
Regarding membrane bound ADAMs, ADAM-10 and ADAM-17 are the best characterized members of this proteinase family due to their ubiquitous expression and involvement in tissue development (111). Both proteinases have been associated with early retinal development via NOTCH1 receptor cleavage, which facilitates neurogenesis by maintaining RPCs in an undifferentiated state, and N-cadherin cleavage, which allows RPC migration and proper retinal lamination (112). Although they are membrane-bound proteases, ADAM-10 and-17 can be released to the ECM by ADAM-15 and-8, respectively, where they are capable of cleaving collagen IV and fibronectin (111). In the developed retina, they are mainly expressed by Müller glia and have been reported to be upregulated in a neovascularization and pro-inflammatory context (113). In the outer limiting membrane (OLM), a tight regulation of ADAM-10 by the Wnt signaling modulator SFRP1, also expressed by Müller glia helps maintain OLM integrity and photoreceptor homeostasis (114). ADAM-10 is also expressed by RGCs and is required for axon guidance and formation of the optic projection (115).
ADAMTSs can be classified by their substrate as: procollagen N-propeptidases, proteoglycanases, fibrillin/fribronectin-associated peptidases and angiogenesis regulators (116). In the retina, Müller glia constitutively express ADAMTS-1, −2, −4, −5, and −13 (117). Especially ADAMTS-1 and-4, and to a lesser extent also ADAMTS-5, have been found to promote synaptogenesis by cleaving the CSPGs neurocan and phosphacan, which inhibit RGCs neurite outgrowth (68, 118, 119), while ADAMTS-4 thrombospondin repeats may also induce neurite extension independent of CSPG cleavage (120). Meanwhile, ADAMTS-2 has been reported to cleave fibrillar collagen precursos (type I-III), thus contributing to the maturation and formation of collagen fibers within the ECM (121). As for ADAMTS-13, it cleaves the von Willebrand factor, a proteoglycan that participates in platelet aggregation (122); interestingly, while by itself promotes angiogenesis, in the presence of vascular endothelial growth factor (VEGF), a potent vascular permeability and angiogenesis factor, ADAMTS-13 become anti-angiogenic (123).
3 Müller glia ECM deposition and retinopathies
It is necessary a balance between the synthesis and degradation of ECM components for tissue homeostasis (92). However, when this equilibrium is disrupted it leads to an abnormal deposition of ECM molecules and changes in the overall stiffness of the tissue. In the retina, several pathological responses cause local changes in the tissue stiffness, exacerbating the process and creating a fibrotic environment.
Fibrosis is a complex biological process that is activated in a tissue after it is wounded. In normal wound healing, myofibroblasts, a cell type key in ECM (mainly collagens) deposition and wound contraction, usually undergo apoptosis once the tissue integrity is restored. However, in fibrotic diseases, myofibroblasts are persistently activated, resulting in the excessive deposition of collagens and severe tissue contraction (i.e., scarring) (124). It has been suggested that ECM stiffness regulates transforming growth factor β (TGFβ)–induced myofibroblast formation in a variety of fibrotic processes in heart, liver, and ocular tissue (125–127), indicating that the increased matrix stiffness caused by the fibrotic process itself promotes myofibroblast formation and further stimulates the fibrotic process. In the retina, the formation of scars has been linked to loss of visual acuity and neurodegeneration (128).
Upon damage, zebrafish Müller glia are capable to re-differentiate into neuronal progenitors, effectively regenerating the damaged retina (129). However, mammalian Müller glia undergo reactive gliosis, characterized by activation, proliferation and hypertrophy. Initially, Müller glia reactivity generate neurotrophic factors to promote the protection of neurons, mainly RGCs (130, 131), but if gliosis persist, they contribute to degeneration and block tissue regeneration (132).
The YAP/TAZ complex, a downstream effector of the Hippo signaling pathway that has been shown to affect cellular apoptosis, proliferation and Müller glia reprogramming (133), can be modulated by ECM stiffness (134). A feed-forward loop is settled, in which matrix stiffness promotes YAP translocation to the nucleus and activation, which in turn increases cell proliferation and collagen deposition, thus enhancing substrate stiffness even more (135) (Figure 3). In addition, YAP activation has been linked to TGFβ signaling.
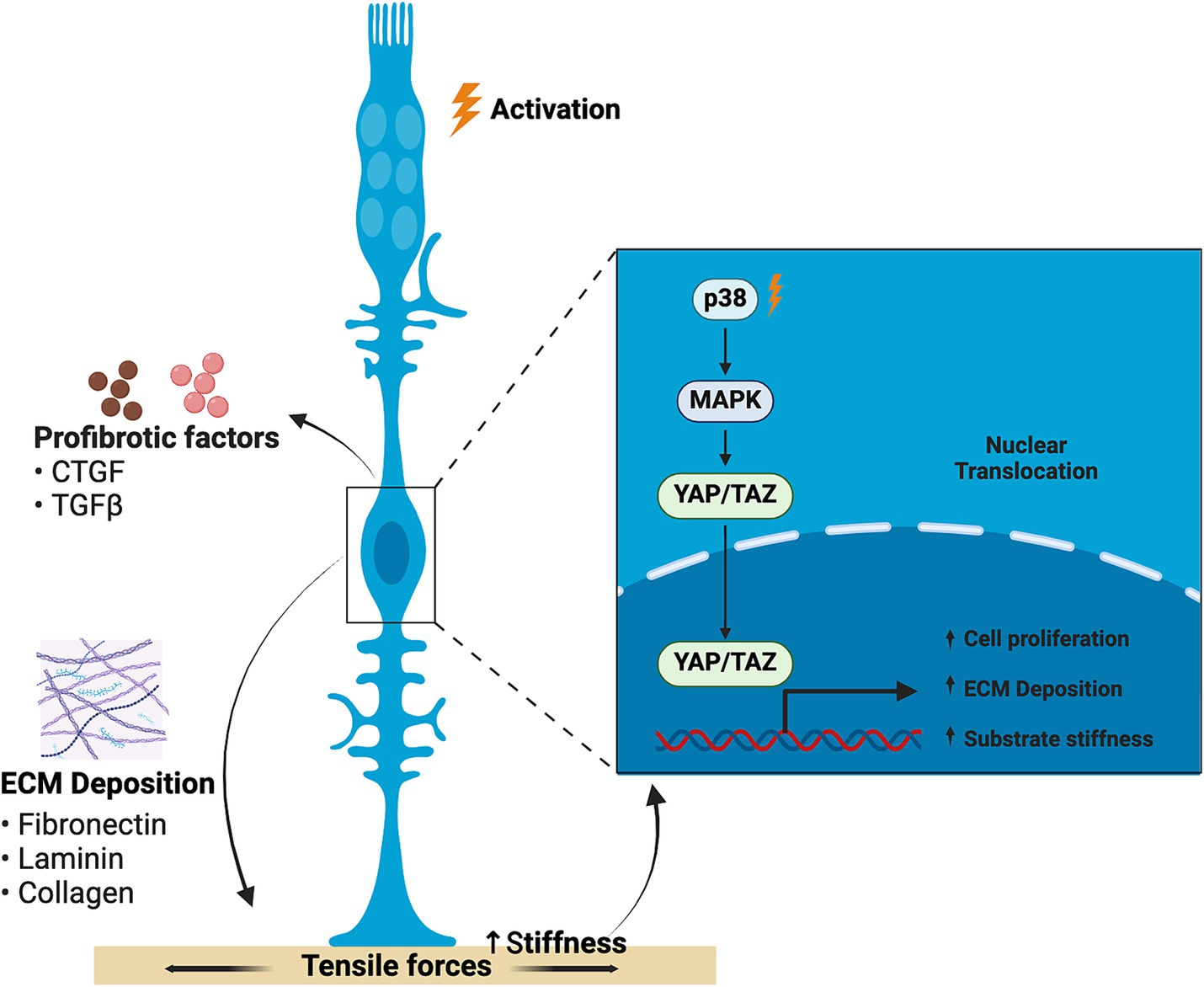
Figure 3. Schematic representation of the feed-forward loop generated by ECM stiffness in retinal fibrosis. Matrix stiffness promotes YAP nucleus translocation and activation, thus increasing cell proliferation and collagen deposition, increasing substrate stiffness in a feed-forward loop. Created with BioRender.com.
TGFβ signaling is essential to the wound healing process, both in scar formation and tissue-specific regeneration (136). It has been observed that the activation of either canonical or non-canonical TGFβ pathway is associated with the differential injury response in zebrafish and mammals (137). The TGFβ3 isoform, in combination with Smad protein, was the only one upregulated upon damage in zebrafish Müller glia and promoted retinal regeneration via canonical signaling; furthermore, TGFβ3 has been connected to anti-fibrotic wound healing (138). On the other hand, mammal (murine) Müller glia expressed the TGFβ1 and TGFβ2 isoforms, which mediate the non-canonical pathway via p38MAPK (137). Both isoforms promote fibronectin and collagen deposition and stiffening (139, 140). Furthermore, the Notch signaling pathway also promotes Müller glia ECM overexpression working in an additive way with TFGβ, as has been observed both in vitro and in an in vivo mouse model (141).
Interestingly, the expression of TGFβ1 together with the transcription factor SNAIL has been found to induce the upregulation of several glial-to-mesenchymal transition (GMT) related molecular markers in Müller glia, and the downregulation of glutamine synthetase (142). Also, the interplay between TFGβ and Notch pathways has been linked to the dedifferentiation of Müller glia to an epithelial lineage (143), which correlates to changes in their morphology and their detrimental contribution to retinal fibrosis in chronic gliosis.
Although fibrosis is a common aspect of different retinopathies, the specific cues driving this process and the ECM components preferentially deregulated are disease-dependent. Therefore, in this section we will discuss the particular mechanisms and ECM components involved in different retinal diseases (Figure 4).
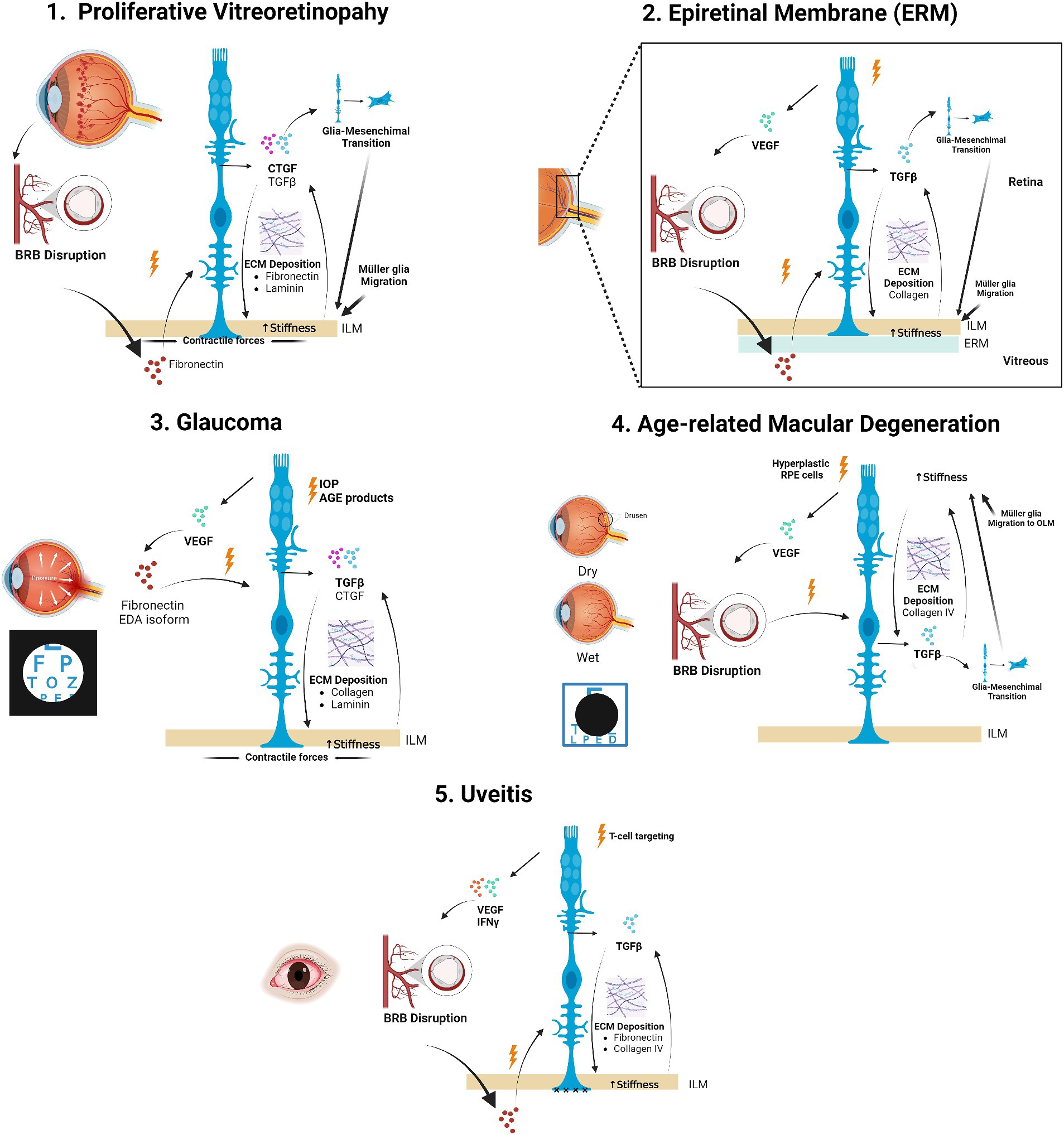
Figure 4. Müller glia-driven alterations in retinal ECM stiffness involvement in retinal diseases. Schematic representation of different retinal pathologies in which ECM deposition and increased stiffness via activated Müller glia is involved and known mechanisms. Created with BioRender.com.
3.1 Proliferative vitreoretinopathy
Proliferative vitreoretinopathy (PVR) consists in the formation of fibrocellular membranes on the retinal and posterior hyaloid surfaces following either rhegmatogenous or post-surgery retinal detachment, being the main reason for its failure. The major cell types involved in PVR have a retinal origin (Müller glia and RPE) (144). Although RPE proliferation has been considered for a long time to be the major player in the development of this pathology, in recent years Müller glia have emerged as key in the pathogenesis of PVR (41). Following BRB disruption in PVR, fibronectin in the plasma enters the subretinal space and acts as a chemical attractant, leading to the proliferation and migration of Müller glia into the vitreous, where they synthesize ECM proteins and contract onto the retina, eventually forming a subretinal glial scar (34, 145, 146).
Connective tissue growth factor (CTGF) is thought to be the major mediator of retinal fibrosis in PVR, promoting the synthesis of fibronectin, laminin, and MMP-2 (147), and its expression by Müller glia peaks during late stages of the pathology (148). Müller glia expression of CTGF on substrates of different stiffness was shown to increase on soft substrates (17, 40). This suggests that, when the retina becomes too soft after retinal detachment (since it loses its anchoring points), Müller glia upregulate CTGF in order to stiffen the tissue, which if persisting, leads to PVR.
Apart from synthetizing ECM proteins, in the context of PVR Müller glia themselves develop myofibroblast-like features (141, 149), determined by the expression of smooth-muscle actin. These transdifferentiated myofibroblasts are key in PVR membrane contractility, leading to retinal wrinkling, formation of retinal breaks and reopening of previously sealed ones. They exert this effect on the retina both by contracting themselves and by remodelling the surrounding ECM via TGFβ, contributing to the fibrocellular membrane formation.
3.2 Epiretinal membranes
Epiretinal membranes (ERM) are transparent membranes formed at the limit between the vitreous and the retina either as a consequence of diseases (e.g., diabetes, retinal tears), or without any apparent reason – i.e. idiopathic ERMs (iERM) (150, 151). ERMs consist of reactive cellular elements, vitreous structures, and fibrotic components, and can seriously affect vision when developing and retracting in front of the macula (152). Furthermore, the formation and retraction of ERMs increase the rigidity of the retina.
It has been proposed that a main mechanism that leads to ERM formation is the activation of Müller glia by the persisting contact of their processes in the ILM with blood-borne substances that migrate to the vitreous after the disruption of the BRB (18). Therefore, ERMs have been associated with the proliferation of activated Müller glia, which create stronger adhesion between the ERM/ILM complex and the inner retinal tissue, difficulting ERM removal during vitrectomy (153). Furthermore, Müller glia are responsible for newly formed collagens type I, III, and IV in the ERMs, increasing the adhesion to the retina and the stiffness of the ERM and reducing collagen digestibility. Moreover, the upregulation of the intermediate filament GFAP (glial fibrilary acid protein), increases the stiffness of Müller glia, which can also transdifferentiate into myofibroblasts via TGF-β1/2/SNAIL–initiated Müller GMT, contributing to the mechanical properties of ERMs (142, 154). Both collagen deposition and myofibroblast transdifferentiated Müller glia make a rigid and degradation-resistant fibrotic tissue.
The traction exerted by contractile ERM also produce degenerative lamellar holes due to disruption either of the Müller glia cone in the foveola or the connection between the cone and the foveal walls of Müller glia (155). These facts lead to the degeneration of photoreceptors, bipolar cells and horizontal cells in the INL and ONL of the retina.
3.3 Glaucoma
Glaucoma is a neurodegenerative disease characterized by the selective death of RGCs, mainly due to an increase in the intraocular pressure (IOP), which leads to a progressive loss of vision, representing the second leading cause of irreversible blindness worldwide (156).
Increased stiffness in the ECM of surrounding tissues such as the trabecular meshwork (TM) has been linked to the progression of glaucoma, where elevated IOP results from increased resistance to aqueous humour outflow as a result of impaired TM cell function (i.e., remodelling of cytoskeleton, increased cell stiffness) and increased ECM deposition (157). Both processes contribute to TM stiffening (158) which affects cell function and increases IOP in a feed-forward cycle. Similarly, in the lamina cribrosa, repeated cycles of increased strain and stiffnening coupled with profibrotic ECM deposition have been associated with glaucoma progression and optic nerve head cupping (159).
In the glaucomatous retina, the enhanced accumulation of advanced glycation end products (AGE) and the expression of their receptor, RAGE, is upregulated, mainly by Müller glia (160). The binding of AGE to Müller glia RAGEs induces the activation of these cells and their release of profibrotic cytokines, such as TGFβ, and an increased expression of ECM proteins (161), increasing retinal stiffness. AGE-binding also induces collagen crosslinking which has been correlated with decreased degradation, loss of tissue compliance and therefore, a higher susceptibility of RGCs to damage (162).
Furthermore, AGE binding to Müller glia have been linked to promoting inflammation and retinal vascularization via the release of VEGF, contributing to the contraction of the fibril matrix and further retinal stiffening (163). VEGF has been associated with the induction of profibrotic growth factors, such as TGFβ1 and CTGF, and ECM components in the early stages of retinopathies (32). Specifically, VEGF may induce the upregulation of cellular fibronectin extra-domain (EDA) isoform, an endogenous ligand of the Toll-like receptor 4 (TLR4) (33). EDA has been shown to be upregulated in glaucoma and contribute to TGFβ2 induced ocular hypertension and ECM deposition (164, 165). AGE accumulation also modifies retinal ECM laminin, which reduces Müller glia Kir4.1 channels expression, thus reducing potassium buffering and leading to RGCs apoptosis (166).
As in other retinal diseases, YAP is heavily involved in the mechanotransduction process. Recently, a genome-wide meta-analysis identified YAP as a potential genetic glaucoma risk factor (167); therefore YAP may play a prominent role in glaucoma pathogenesis, driving the establishment of Müller glia TGFβ activation-retinal stiffening feed-forward loop (135).
3.4 Age-related macular degeneration
Age-related macular degeneration (AMD) is a progressive, multi-factorial neurodegenerative disease that is the leading cause of vision loss in western elderly population (168). AMD primarily affects the macula, a small structure within the central retina containing cells of the neuroretina (neurons and glial cells), and RPE attached to the Bruch’s membrane (a pentalaminar ECM that connects the RPE to the choriocapillaris) (169). The macula has the highest density of photoreceptors in the retina, and photoreceptor degeneration is one of the main consequences of AMD (170).
Stress-relaxation studies of retinal explants have shown that AMD retinas are stiffer than healthy ones (171), while genome-wide association studies (GWAS) linked a dysregulation of the collagen IV ECM pathway to the pathogenesis of AMD (172). Early stages of AMD are characterized by the accumulation of drusen, extracellular deposits of protein and lipid aggregates, which appear as yellowish dots (173). More advanced forms of the disease include the “dry” or atrophic form characterized by the progressive dysfunction of the RPE, that anchors the outer retina, and underlying choriocapillaris resulting in loss of photoreceptors and retinal degeneration. “Wet” or neovascular form, less frequent, is characterized by choroidal neovascularization (CNV) that produce leakage into the retina, Müller glia activation and RPE detachments (169, 174, 175).
In CNV, hyperplastic RPE cells, i.e., their proliferation is abnormally upregulated, and the vascular leak and subsequent development of subretinal fibrosis leads to the disruption of Müller glia, which activate the TGFβ1/Smad3 signaling pathway, associated to the stiffening of the matrix (176). Additionaly, endoglin (a co-receptor for the TGFβ family) is strongly expressed on fibro-neovascular tissue and hyperplastic RPE cells, promoting fibro-neovascularization through Müller–derived VEGF and could be also involved in Müller glia GMT.
Interestingly, upon photoreceptor loss Müller glia both extend their processes and proliferate beyond the outer limiting membrane (OLM) creating a dense gliotic membrane and “sealing the gaps” in atrophic areas (177). This Müller glia migration is stimulated by the accumulation of drusen attached to the choroid and their interaction with the RPE resulting in the production of tumour necrosis factor α (TNFα), TGFβ, and VEGF by both cell types, further driving photoreceptor degeneration.
GWAS analysis also identified a highly penetrant missense rare variant in the coagulation factor II thrombin receptor-like 2 (F2RL2), expressed by Müller glia of human and pig retinas (172). F2RL2 has been associated to fibrotic, neovascularized areas in advanced AMD, suggesting a role in the progression of the disease.
3.5 Uveitis
Uveitis comprises a diverse group of intraocular inflammatory diseases of both the uvea (i.e., the iris, ciliary body, and choroid) but also adjacent tissues including the retina. Uveitis is mostly idiopathic but could also developed as a result of infectious and autoimmune processes (178).
In the retina, uveitis mostly displays an autoimmune aetiology, where CD4+ T-cells recurrently target the tissue, as a result of the breakdown of the BRB. In this context, Müller glia have been shown to contribute to the disease by becoming gliotic and upregulating the expression of pro-inflammatory cytokine interferon gamma (IFNγ) and downregulating the neurotrophic factor pigment epithelium-derived protein (PEDF), which under physiologic conditions counteracts the activity of angiogenesis inducers (179). Furthermore, they also contribute to BRB breakdown by releasing VEGF (180).
Uveitis has been associated with the disintegration of the ILM due to the disruption of the fibronectin matrix. Müller glia upregulate fibronectin, which changes its expression pattern from a continuous band to a spotted one. This change, in addition to alterations to Müller glia morphology consequence of the gliotic process, leads to the loss of Müller glia end-feet connection at the ILM, and thus, its disruption (72).
In addition, in infection-derived uveitis the p38MAPK pathway is activated in Müller glia, which has been associated to the expression of pro-inflammatory factors (e.g., IL-6, ICAM-1, CXCL1, CXCL10, CCL2 and CCL5 and CCL7), ECM deposition (mainly fibronectin and collagen IV) and tissue stiffening (137, 181).
4 Modelling the retinal ECM
Although in vitro research cannot fully recapitulate in vivo conditions, it presents many advantages over in vivo studies. Mainly, it allows a tight control of the chemico-physical environment, and displays higher throughput, reduced cost and restricted animal-use.
Traditionally, cell cultures are performed on materials such as glass and polystyrene (plastic). However, the stiffness of these materials is in the range of gigapascals (GPa) while the retinal stiffness ranges between 1.3 to 25.9 kPa (182). Therefore, the cellular behaviour observed on glass/plastic might not correlate with what is actually happening in vivo, where the ECM comprises a complex, changing milieu. Nonetheless, in vitro studies of Müller glia grown on substrates displaying physiological stiffnesses are somewhat lacking. Thus, in this section we will discuss biomaterial alternatives to culture Müller glia.
In recent years, different substrates have been developed aiming to bridge the gap between in vitro and in vivo models. Biomaterials allow controlling their mechanical, compositional, and structural properties, thus more closely resembling the native tissue. They not only provide more physiological culture conditions, but could also be employed in new therapeutic approaches focusing on the mechanical environment of the retina. Among the different biomaterial systems developed, hydrogels—aqueous-swollen crosslinked polymeric networks —have emerged as the most promising option for cell culture. By varying the hydrogel composition and the gelation conditions it is possible to tune their biophysical and biochemical properties (stiffness, pore size, fiber alignment), enabling them to support cell adhesion and protein sequestration (183–185). Hydrogels are derived either from natural or synthetic materials, providing a different set of advantages and disadvantages that will be explored.
4.1 Natural hydrogels
Natural materials for hydrogel fabrication are mostly isolated from native ECM. Due to their origin, they present many similarities to the physiological environment. Furthermore, they contain adhesive sites for cell attachment, not requiring additional functionalization nor surface modifications (186) (Table 2).
4.1.1 Collagen hydrogels
Collagen is the main component of the ECM of most soft tissues. Thanks to its natural origin, collagen gels are biocompatible and biodegradable and present low cytotoxicity, while promoting cell adhesion, proliferation and motility (187) via α1β1 and α2β1 integrin-binding sites (188). Furthermore, collagen hydrogels are highly porous allowing free diffusion of ions and molecules to support cell growth through the polymer matrix (189).
Since collagen type I comprises 90% of fibrillar collagens (190), it is used preferably to prepare hydrogels, although collagen type IV can also be used. Collagen used as polymer material is usually derived from solubilized type I collagen obtained from rat tail tendon and gelation of these solutions is achieved by raising the temperature and the pH to initiate collagen fibril self-assembly (191). Interestingly collagen hydrogel stiffness can be tuned by increasing the pH (above pH 10.0) while decreasing polymerization temperature; this changes augment fibril packaging thus increasing overall matrix stiffness (192, 193).
Collagen hydrogels have been sparsely used for culturing retinal cells such as RPE cells in an AMD model (194), while their use in Müller glia culture has been mostly restricted to contractility essays (195). In cultures of cerebellar granule neuron-glial spheroids, collagen type I, but not type IV, hydrogels have been shown to stimulate astrocyte proliferation to a certain extent but fail to induce neuritogenesis and Calcium signaling activity (196), which suggest that collagen hydrogels might be a poor candidate for CNS tissue mimicking and thus to culture retinal cells. Indeed, previous studies in which the influence of ECM in the neurite branching and regrowth of RGCs was addressed and only a discrete effect was found (73).
In general, collagen hydrogels present some important drawbacks including limited long-term stability and batch-to-batch variability. These hydrogels display low stiffness, requiring extensive chemical crosslinking to achieve stiffnesses higher than 1 kPa (191). This crosslinking leads to significant matrix contraction and altered degradability, restricting the culturing time. Furthermore, cells cultured may alter the intrinsic mechanical properties of the hydrogel (197).
4.1.2 Matrigel
Matrigel is a commercial preparation of ECM components obtained from Engelbreth–Holm–Swarm (EHS) mouse sarcoma tumours; it is composed primarily of laminin, collagen type IV, and entactin, with various other constituents including proteoglycans, such as perlecan, metalloproteinases and growth factors (including the TGFβ family) (198). Matrigel is considered a reconstituted basement membrane that undergoes gelation at temperatures in the range 22–37°C, when entactin acts as a crosslinker between the laminin and collagen IV to create the hydrogel (199).
Matrigel ability to maintain self-renewal and pluripotency due to its tumorigenic origin makes it a good candidate for culturing stem cells. Indeed, as a thin gel coating, Matrigel has been used to culture and expand human pluripotent stem cells (hPSCs) (200) and neuronal stem cells (201), while thicker coatings have been used to investigate angiogenesis (202).
Matrigel has been used as substrate to develop retinal organoids, where different groups have achieved the differentiation of human induced Pluripotent Stem Cells into Müller glia and further expand the obtained cells; these cells were functional and expressed typical Müller glia markers (203, 204), which suggested that Matrigel’s enriched ECM composition favoured Müller glia differentiation. Likewise, Matrigel was also used to differentiate mouse Embryonic Stem Cells into RGC-like cells (205) and human Embryonic Stem Cells into RGCs and Müller glia precursors (206).
Due to its origin, Matrigel is not well-defined and present high batch-to-batch variability and xenogenic contaminants (191, 199), which leads to a significant level of uncertainty to experimental results. Furthermore, Matrigel stiffness is relatively low (around 400 Pa) and due to its temperature sensitivity drops to lower stiffnesses if it is not kept at 37°C during experimentation (207); although it can be tuned by increasing overall protein concentration, this leads to alterations in its biological functionality.
4.1.3 Alginate
Alginate is a polysaccharide typically obtained from brown algae that presents biocompatibility and low toxicity for biomedical applications. It is formed by α-L-guluronate (G) and β-D-mannuronate (M) residues, and hydrogels are formed by the interaction of G residues with different divalent cations (mainly calcium and barium) (11).
The stiffness of the gel depends on the overall G section (208) and the cation used for gelation (209). Since mammalian cells lack receptors for alginate, and alginate gels show low protein adsorption, their coupling to specific peptides is required for proper cellular adhesion (210). The most commonly used peptide is arginine-glycine-aspartic acid (RGD), which concentration required for cell adhesion and growth is cell-type dependent.
RGD-alginate gels have been found to display compatibility with CNS tissue, promoting the outgrowth of regenerating axons and the elongation of astrocytic processes of the spinal cord (211) and favouring the expansion of hipoccampus neural progenitor cells in vitro (212). These hydrogels have also been shown to promote the generation of retinal progenitor cells from iPSC-and hESC-derived embryoid bodies (213). Although there are no studies of Müller glia grown on alginate hydrogels, this biomaterial has been used in brain astrocyte activation studies where different alginate concentrations elicited a different stiffness-mediated response (214, 215); as Müller glia take up the mechanosensor function in the retina that astrocyte carry out in the brain and both cell types are glial cells of the CNS these results suggest that alginate hydrogels could be a good alternative to study the effect of substrate stiffness on Müller glia.
However, as in other natural hydrogels, alginate shows poor long-term stability, and batch-to-batch variability in their degradation and mechanical properties (216). Furthermore, native alginate gels display low mechanical integrity, as a result of their swelling behaviour in the aqueous culture environment, requiring the incorporation of a compatible reinforcing agent (e.g., cellulose, polylactic acid) within the alginate structure (217).
4.2 Synthetic hydrogels
As opposed to natural hydrogels, synthetic hydrogels are well-defined and their chemical–physical properties can be easily tuned (218), which may be more suitable for cell culture and native ECM modelling, although they do not have any inherent bioactivity (Table 3).
4.2.1 Polyacrylamide gels
Polyacrylamide is a cyto-compatible, bioinert material, formed by the synthetic polymer acrylamide and its crosslinker (bis-acrylamide), gelation being activated by ammonium persulfate (APS) and tetramethylethylenediamine (TEMED). Polyacrylamide gels exhibit a strong homogeneity in surface topography, mechanical properties, and coating density, displaying high reproducibility (219).
Polyacrylamide hydrogels stiffness can be easily tuned over several orders of magnitude within the physiological range, by varying the concentration of the constituents (219, 220). Although the gel elastic modulus increases with increasing cross-link concentration, the formation of highly cross-linked clusters add heterogeneity to the network structure, leading to an inflection point, after which gel stiffness decreases under high cross-linking conditions (221). Therefore, crosslinking should be tightly controlled when fabricating the hydrogel. Due to their bioinert characteristics, polyacrylamide hydrogels need to be functionalised by the tethering of ECM components in order to support cell adhesion, including poly-L-lysine (222), laminin (14) or collagen I (223), among others. This tethering is UV-dependent.
One of the major disadvantages observed in polyacrylamide gels is the change in porosity dependent on the gel formulation (224). However, Wen et al. (225) have shown that porosity and stiffness can be independently tuned. Furthermore, when they cultured mesenchymal stem cells on polyacrylamide gels of different stiffnesses and porosities they observed that differentiation into either adipocytes or osteocytes did not depend on porosity or protein tethering but only on the substrate stiffness, which makes polyacrylamide gels good candidates for studying substrate stiffness as an isolated parameter.
Polyacrylamide gels are ideal for CNS mimicking. Cultures of adult neural stem cells on soft polyacrylamide gels promote neural expansion while stiffer ones favoured the appearance of glial cells of astrocytic phenotype (226, 227). Likewise, the survival and proliferation of oligodendrocyte progenitor cells has been shown to be modulated by substrate stiffness (228).
Thanks to polyacrylamide capacity to tune its rigidity over a wide range of stiffnesses, they can be used to model pathologic contexts, where stiffness is heightened. For example, a stiff polyacrylamide matrix led to impaired myosin activity and branching inhibition in an oligodendrocyte differentiation study (229).
In the study of retinal fibrotic diseases, Müller glia cultured on polyacrylamide gels showed that on stiff substrates there is an upregulation of stress fibers and reorganization of the cytoeskeletal integrity, changes in gene regulation and a morphologic transition to a myofibroblast-like phenotype (39, 40). This is due to stiffening-stimulated YAP activation by the TGFβ1-PI3K/Akt pathway (15) and the expression of CTFG, implicated in the gliotic process (17).
Polyacrylamide gels manufacturing parameters such as UV activation intensity and exposure time, gel thickness, and acrylamide/bis-acrylamide concentration should be tightly controlled to achieve accurate, reproducible results (219).
4.2.2 Polydimethylsiloxane gels
Polydimethylsiloxane (PDMS) is a silicon-containing polymeric material. PDMS hydrogels are formed by an elastomer base (PDMS oligomers with vinyl-terminated groups, a platinum catalyst and dimethylvinylated/trimethylated silica) and a curing agent (dimethyl methylhydrogen siloxane and tetramethyl tetravinyl cyclotetrasiloxane) (11). PDMS hydrogels display biocompatibility, high oxygen permeability, thermal stability, a high protein binding capability for cell expansion and tunable mechanical properties (230, 231). Substrate stiffness can be easily tuned by curing temperature and time and changing the base-to-curing agent ratio (232), where an increased ratio correlates to augmented stiffness.
The elastic modulus of PDMS is typically in the order of megapascals (233). However different strategies, i.e., altering the geometrical organization by fabricating microposts of different heights (234), have been developed to decrease the effective stiffness of PDMS hydrogels, making them apt for mimicking soft tissues such as the CNS.
PDMS hydrogels have also been used to study mechanotransduction in different glial cells of the CNS. Thanks to their elastic properties they have been applied as a stretchable material to test the effects of tensile forces on oligodendrocyte progenitor cells (235), which have been shown to favour cell expansion and differentiation. In other studies, tuning their rigidity have been used to study the effect of mechanical stiffness on cortical astrocyte activation and progression of astrogliosis (236), which could be extrapolated to Müller glia. Also for Schwann cell proliferation and transcription of basal lamina receptor genes in axonal sorting and myelination which is promoted by the substrate stiffness activation of the YAP/TAZ complex (237). Likewise, PDMS have been used as substrate for the developing of retinal organoids, where mESCs were differentiated mainly into photoreceptors, but also RGCs, amacrine cells, horizontal cells, bipolar cells and Müller glia (238, 239).
However, PDMS being a synthetic material, is bioinert and require the adsorption of charge enhancers, e.g., poly-L-lysine (236), and/or the binding of adhesive ECM proteins, e.g., laminin, fibronectin (240, 241), for proper cell attachment and integrin signaling. Thus, the nature of the generated interactions and the coating density are critical for cell adhesion and spreading.
PDMS hydrogels are preferentially used when looking for larger structural stiffness, while in starting studies of compliant substrates polyacrylamide gels are usually chosen (219).
4.2.3 Polyethylene glycol gels
Polyethylene glycol (PEG) is a hydrophilic polymer of ethylene oxide that display a variety of end groups (e.g., alcohol, methyl ether, amine, N-hydroxysuccinimidyl (NHS) ester) which are cross-linked for hydrogel formation (242).
PEG is hydrophilic, present biocompatibility and low toxicity, is non-adhesive toward proteins and cells and its stiffness can be tuned by varying the polymer concentration. As with other synthetic hydrogels PEG is bioinert and as such requires functionalization by ECM protein (e.g., vitronectin, fibronectin) binding for cell attachment (243–245). PEG hydrogels can mimic soft substrates; however, softer gels (~1 kPa) are somewhat limited in the amount of protein that can be coupled to the gel substrate, which can pose a problem for cells with weak adhesion forces (246). Alternatively, PEG cross-linking to natural-based materials, such as gelatin, and natural-based gelatin cross-linking has been shown to enhance the biophysical properties, including gelation time, and biocompatibility of PEG hydrogels improving cell long-term viability (247).
Although less frequently than polyacrylamide or PDMS gels, PEG hydrogels have been utilized to study neural cell biomechanical behaviour, increasing PEG composition and consequential increase in stiffness has been associated with the expansion of neural progenitor cell cultures and differentiation to glial cells (248). PEG hydrogels have also been fabricated for retinal organoid culturing, their intrinsic inertness and hydrophilicity allowing for a good diffusion of metabolites as well as factors, favouring organoid development and colony formation in this type of essay (238). Although PEG hydrogels (fused with hyaluronan acid) have been proved to be non-citotoxic to Müller glia in vitro (249), no further studies have been carried out.
Interestingly, RGCs grown on PEG hydrogels of different stiffnesses, coupled with poly-L-lysine, attached better in hydrogels with an stiffness range of 3.8–5.7 kPa than on expected optimal stiffness hydrogels (0.9–1.8 kPa), due to the ratio of free amines to hydroxyls (250), which suggests that stiffness cannot be measured as an isolated parameter in PEG hydrogels.
4.3 Two-dimensional vs. three-dimensional culture
Traditionally, cell cultures have been performed on two-dimensional (2D) conditions. Although these approaches have significantly advanced our understanding of cell behaviour, cell bioactivity on 2D systems not always correlates with what happens in vivo, where cells are embedded in the three-dimensional (3D) native ECM. In fact, cell encapsulation in 3D systems demonstrate that increasing the dimensionality can impact cell proliferation, differentiation, mechanoresponse, and cell survival, making them good candidates for tissue-engineering (251, 252).
Cells grown in 2D monolayers rely on their adherence to a flat surface and have unrestricted access to nutrients and growth factors present in the culture medium, which results in homogenous growth and proliferation (253). This allows for simple and efficient cell culturing, but lacks important mechanical cues since cells in vivo receive stiffness signals from all over their surface. Additionally, cells display forced apical-basal polarity that does not occur in vivo (254). Meanwhile, 3D cultures allow the formation of more cell–cell and cell-matrix interactions that more closely resembles native tissues, but could also display oxygen and nutrient restrictions, reducing their viability (255).
In retinal cells, a study in which perinatal Müller glia were investigated for neuron cell replacement therapy, culture in 2D conditions and encapsulating spheroids showed differential neuronal gene expression (256). For example, expression of Mdk and Sox3, regulators of neurogenesis, was upregulated only in 2D cultures. In contrast, Mef2c, involved in early neuronal differentiation, was robustly expressed only in 3D conditions. They also observed some genes associated with dendrite formation, neurite outgrowth and synaptic refinement, such as Bmp2, Ptn and Nptx1, upregulated only in 3D conditions.
Regarding the production of retinal organoids, although different groups have been able to successfully differentiate iPSCs or ESCs into different retinal cell types (213, 238, 257), the small size and the heterogeneity found pose significant technical problems such as lower sensitivity or the difficulty to distinguish the origin of the measured signals.
Assessing the mechanical response of cells to substrate stiffness is significantly easier in 2D cultures (258), where cells adhere their basal surface along a planar surface and are able to detect stiffness over the length of a single cell (259). However, in 3D systems, the whole cell is in contact with other cells and/or the ECM. Furthermore, ECM stiffness can vary depending on the ECM ligand density and pore size, and intra-and extra-fibril crosslinking and alignment. Therefore, it is possible for an individual cell to locally sense part of a matrix as stiff or soft depending on whether tension is generated parallel or perpendicular to a particular fiber. Regarding the materials employed, hydrogels used for 3D culture have shown to facilitate matrix production/degradation and other cell bioactivities, that highly affect matrix stiffness and viscoelastic properties, which has been deemed complex to control and isolate (254).
While 3D cultures better recapitulate in vivo overall ECM conditions, their complexity difficult the independent study of mechanical parameters, such as ECM stiffness. Conversely, in 2D cultures the effect of these parameters can be effectively isolated from one another.
5 Conclusions and future directions
The retina, due to its location, is constantly exposed to mechanical changes. Furthermore, the tissue itself is very heterogeneous, displaying cells with different stiffnesses and ECM layer-dependent composition. The mechanical state of the retina, and specifically its resistance of deformation or stiffness, is key in its development and maturation, and aberrant ECM deposition and increased stiffness is associated with the progression of different retinal diseases, including retinal fibrosis, PVR, glaucoma, AMD, uveitis and the formation of ERMs.
Müller glia, as the main retinal glial cell type, are capable of both sensing mechanical changes in the whole retinal thickness and deposit ECM constituents. During retinal diseases, Müller glia become gliotic and hypertrophic, promoting a tissue stiffening feed-forward loop by secreting profibrotic and proinflammatory factors and ECM components, and transitioning to a myofibroblast state.
The interplay between retinal stiffness and the biological response of the mechanosensitive Müller glia is a research area of growing interest. As more sophisticated in vitro models are being developed, this would lead to new findings into the effect of substrate stiffness in retinal physiology and disease, and promote the elucidation of novel diagnostic targets. To better mimic the effect of matrix stiffness in vitro, 2D cultures in synthetic materials appear to be ideal candidates for retinal ECM modelling. However, most Müller glia and other retinal cells in vitro studies where these biomaterials have been used were focused on retinal organoid differentiation and expansion. So far, Müller glia in vitro studies focusing on the stiffness of the substrate have been mainly been done on polyacrylamide hydrogels, which display high biocompatibility and stability, low toxicity and are bioinert. Additionally, their stiffness can be tuned over a wide physiological range and allow for the study of the substrate stiffness effect on Müller glia behaviour independent of other mechanical parameters. Nonetheless the culture of other glial cell types in different biomaterials show promising results that could be extrapolated to Müller glia. To more closely resemble the native ECM complexity, in future, hydrogel 3D cultures would be a better option, but oxygen and nutrient restrictions as well as effectively decoupling the stiffness from other mechanical parameters need to be overcome first.
This opens up a field of mechanical therapeutic approaches to retinal disease focusing on the modulation of the ECM stiffness via Müller glia to tackle the fibrotic component present in many of these pathologies. Furthermore, improved, more native-like hydrogels could help advance the field of cell transplant therapies by providing a compliant environment for the cells to grow and differentiate. With this review, we aim to emphasize the role of Müller glia in the ECM stiffness of the retina and its importance in retinal homeostasis, and offer an insight into different hydrogel systems to model the ECM stiffness in vitro, which has great research value and translational potential in the treatment of retinal diseases.
Author contributions
LP-L: Investigation, Writing – original draft, Writing – review & editing. XP: Supervision, Visualization, Writing – original draft, Writing – review & editing. EV: Funding acquisition, Investigation, Supervision, Visualization, Writing – original draft, Writing – review & editing.
Funding
The author(s) declare financial support was received for the research, authorship, and/or publication of this article. This research was funded by ELKARTEK (KK-2019/00086), Gobierno Vasco (IT1510-22), MINECO-Retos MICIU/AEI/10.13039/501100011033 (PID2023-152778OB-I00), and PIBA (2020_1_0026).
Conflict of interest
The authors declare that the research was conducted in the absence of any commercial or financial relationships that could be construed as a potential conflict of interest.
Publisher’s note
All claims expressed in this article are solely those of the authors and do not necessarily represent those of their affiliated organizations, or those of the publisher, the editors and the reviewers. Any product that may be evaluated in this article, or claim that may be made by its manufacturer, is not guaranteed or endorsed by the publisher.
References
2. Marmor, M. Mechanisms of Normal retinal adhesion. Prog Retin Res. (1993) 12:179–204. doi: 10.1016/0278-4327(93)90009-I
3. Franze, K, Francke, M, Günter, K, Christ, AF, Körber, N, Reichenbach, A, et al. Spatial mapping of the mechanical properties of the living retina using scanning force microscopy. Soft Matter. (2011) 7:3147. doi: 10.1039/c0sm01017k
4. Franze, K. Integrating chemistry and mechanics: the forces driving axon growth. Annu Rev Cell Dev Biol. (2020) 36:61–83. doi: 10.1146/annurev-cellbio-100818-125157
5. Lu, Y-B, Franze, K, Seifert, G, Steinhäuser, C, Kirchhoff, F, Wolburg, H, et al. Viscoelastic properties of individual glial cells and neurons in the CNS. Proc Natl Acad Sci. (2006) 103:17759–64. doi: 10.1073/pnas.0606150103
6. García, M, Forster, V, Hicks, D, and Vecino, E. Effects of Müller glia on cell survival and Neuritogenesis in adult porcine retina in vitro. Invest Ophthalmol Vis Sci. (2002) 43:3735–43.
7. Ruzafa, N, and Vecino, E. Effect of Müller cells on the survival and neuritogenesis in retinal ganglion cells. Arch Soc Esp Oftalmol. (2015) 90:522–6. doi: 10.1016/j.oftale.2015.08.027
8. García, M, and Vecino, E. Role of Müller glia in neuroprotection and regeneration in the retina. Histol Histopathol. (2003) 18:1205–18. doi: 10.14670/HH-18.1205
9. Vecino, E, Rodriguez, FD, Ruzafa, N, Pereiro, X, and Sharma, SC. Glia–neuron interactions in the mammalian retina. Prog Retin Eye Res. (2016) 51:1–40. doi: 10.1016/j.preteyeres.2015.06.003
10. Vecino, E, and Kwok, JCF. The extracellular matrix in the nervous system: the good and the bad aspects, composition and function of the extracellular matrix in the human body London (UK): IntechOpen (2016).
11. Yi, B, Xu, Q, and Liu, W. An overview of substrate stiffness guided cellular response and its applications in tissue regeneration. Bioact Mater. (2022) 15:82–102. doi: 10.1016/j.bioactmat.2021.12.005
12. Barriga, EH, Franze, K, Charras, G, and Mayor, R. Tissue stiffening coordinates morphogenesis by triggering collective cell migration in vivo. Nature. (2018) 554:523–7. doi: 10.1038/nature25742
13. Reinhard, J, Joachim, SC, and Faissner, A. Extracellular matrix remodeling during retinal development. Exp Eye Res. (2015) 133:132–40. doi: 10.1016/j.exer.2014.07.001
14. Segel, M, Neumann, B, Hill, MFE, Weber, IP, Viscomi, C, Zhao, C, et al. Niche stiffness underlies the ageing of central nervous system progenitor cells. Nature. (2019) 573:130–4. doi: 10.1038/s41586-019-1484-9
15. Zhang, W, and Kong, Y. YAP is essential for TGF-β-induced retinal fibrosis in diabetic rats via promoting the fibrogenic activity of Müller cells. J Cell Mol Med. (2020) 24:12390–400. doi: 10.1111/jcmm.15739
16. Chen, K, and Weiland, JD. Discovery of retinal elastin and its possible role in age-related macular degeneration. Ann Biomed Eng. (2014) 42:678–84. doi: 10.1007/s10439-013-0936-x
17. Davis, JT, Foster, WJ, Foster, WJ, and Foster, WJ. Substrate stiffness influences the time dependence of CTGF protein expression in Müller cells. Int Physiol J. (2018) 1:1–7. doi: 10.14302/issn.2578-8590.ipj-17-1910
18. Bringmann, A, and Wiedemann, P. Involvement of Müller glial cells in epiretinal membrane formation. Graefes Arch Clin Exp Ophthalmol. (2009) 247:865–83. doi: 10.1007/s00417-009-1082-x
19. Miller, CG, Budoff, G, Prenner, JL, and Schwarzbauer, JE. Minireview: fibronectin in retinal disease. Exp Biol Med. (2017) 242:1–7. doi: 10.1177/1535370216675245
20. Cosens, DJ, and Manning, A. Abnormal electroretinogram from a drosophila mutant. Nature. (1969) 224:285–7. doi: 10.1038/224285a0
21. Howard, J, and Bechstedt, S. Hypothesis: a helix of ankyrin repeats of the NOMPC-TRP ion channel is the gating spring of mechanoreceptors. Curr Biol. (2004) 14:R224–6. doi: 10.1016/j.cub.2004.02.050
22. Coste, B, Mathur, J, Schmidt, M, Earley, TJ, Ranade, S, Petrus, MJ, et al. Piezo1 and Piezo2 are essential components of distinct mechanically activated cation channels. Science. (2010) 330:55–60. doi: 10.1126/science.1193270
23. Phuong, TTT, Redmon, SN, Yarishkin, O, Winter, JM, Li, DY, and Križaj, D. Calcium influx through TRPV4 channels modulates the adherens contacts between retinal microvascular endothelial cells. J Physiol. (2017) 595:6869–85. doi: 10.1113/JP275052
24. Ryskamp, DA, Frye, AM, Phuong, TTT, Yarishkin, O, Jo, AO, Xu, Y, et al. TRPV4 regulates calcium homeostasis, cytoskeletal remodeling, conventional outflow and intraocular pressure in the mammalian eye. Sci Rep. (2016) 6:30583. doi: 10.1038/srep30583
25. Sappington, RM, Sidorova, T, Ward, NJ, Chakravarthy, R, Ho, KW, and Calkins, DJ. Activation of transient receptor potential vanilloid-1 (TRPV1) influences how retinal ganglion cell neurons respond to pressure-related stress. Channels. (2015) 9:102–13. doi: 10.1080/19336950.2015.1009272
26. Yarishkin, O, Phuong, TTT, Bretz, CA, Olsen, KW, Baumann, JM, Lakk, M, et al. TREK-1 channels regulate pressure sensitivity and calcium signaling in trabecular meshwork cells. J Gen Physiol. (2018) 150:1660–75. doi: 10.1085/jgp.201812179
27. MacDonald, RB, Randlett, O, Oswald, J, Yoshimatsu, T, Franze, K, and Harris, WA. Müller glia provide essential tensile strength to the developing retina. J Cell Biol. (2015) 210:1075–83. doi: 10.1083/jcb.201503115
28. Taylor, L, Moran, D, Arnér, K, Warrant, E, and Ghosh, F. Stretch to see: lateral tension strongly determines cell survival in long-term cultures of adult porcine retina. Invest Ophthalmol Vis Sci. (2013) 54:1845–56. doi: 10.1167/iovs.12-11420
29. Jo, YH, Choi, GW, Kim, M-L, and Sung, KR. Statins inhibit the gliosis of MIO-M1, a Müller glial cell line induced by TRPV4 activation. Int J Mol Sci. (2022) 23:5190. doi: 10.3390/ijms23095190
30. Lindqvist, N, Liu, Q, Zajadacz, J, Franze, K, and Reichenbach, A. Retinal glial (Müller) cells: sensing and responding to tissue stretch. Invest Ophthalmol Vis Sci. (2010) 51:1683–90. doi: 10.1167/iovs.09-4159
31. Song, Y, Overmass, M, Fan, J, Hodge, C, Sutton, G, Lovicu, FJ, et al. Application of collagen I and IV in bioengineering transparent ocular tissues. Front Surg. (2021) 8:639500. doi: 10.3389/fsurg.2021.639500
32. Kuiper, EJ, Hughes, JM, Van Geest, RJ, Vogels, IMC, Goldschmeding, R, Van Noorden, CJF, et al. Effect of VEGF-A on expression of profibrotic growth factor and extracellular matrix genes in the retina. Invest Ophthalmol Vis Sci. (2007) 48:4267–76. doi: 10.1167/iovs.06-0804
33. Reinhard, J, Wiemann, S, Hildebrandt, S, and Faissner, A. Extracellular matrix Remodeling in the retina and optic nerve of a novel glaucoma mouse model. Biology. (2021) 10:169. doi: 10.3390/biology10030169
34. Zhang, W, and Han, H. Targeting matrix stiffness-induced activation of retinal pigment epithelial cells through the RhoA/YAP pathway ameliorates proliferative vitreoretinopathy. Exp Eye Res. (2021) 209:108677. doi: 10.1016/j.exer.2021.108677
35. Frantz, C, Stewart, KM, and Weaver, VM. The extracellular matrix at a glance. J Cell Sci. (2010) 123:4195–200. doi: 10.1242/jcs.023820
36. Kreeger, PK, Strong, LE, and Masters, KS. Engineering approaches to study cellular decision making. Annu Rev Biomed Eng. (2018) 20:49–72. doi: 10.1146/annurev-bioeng-062117-121011
37. Thompson, AJ, Pillai, EK, Dimov, IB, Foster, SK, Holt, CE, and Franze, K. Rapid changes in tissue mechanics regulate cell behaviour in the developing embryonic brain. eLife. (2019) 8:e39356. doi: 10.7554/eLife.39356
38. Kennedy, KM, Bhaw-Luximon, A, and Jhurry, D. Cell-matrix mechanical interaction in electrospun polymeric scaffolds for tissue engineering: implications for scaffold design and performance. Acta Biomater. (2017) 50:41–55. doi: 10.1016/j.actbio.2016.12.034
39. Bu, S-C, Kuijer, R, van der Worp, RJ, van Putten, SM, Wouters, O, and Li, XR. Substrate elastic modulus regulates the morphology, focal adhesions, and α-smooth muscle actin expression of retinal Müller cells. Invest Ophthalmol Vis Sci. (2015) 56:5974–82. doi: 10.1167/iovs.14-15969
40. Davis, JT, Wen, Q, Janmey, PA, Otteson, DC, and Foster, WJ. Müller cell expression of genes implicated in proliferative vitreoretinopathy is influenced by substrate elastic modulus. Invest Ophthalmol Vis Sci. (2012) 53:3014–9. doi: 10.1167/iovs.11-8450
41. Bringmann, A, Iandiev, I, Pannicke, T, Wurm, A, Hollborn, M, Wiedemann, P, et al. Cellular signaling and factors involved in Müller cell gliosis: neuroprotective and detrimental effects. Prog Retin Eye Res. (2009) 28:423–51. doi: 10.1016/j.preteyeres.2009.07.001
42. Inatani, M, and Tanihara, H. Proteoglycans in retina. Prog Retin Eye Res. (2002) 21:429–47. doi: 10.1016/S1350-9462(02)00009-5
43. Ihanamäki, T, Pelliniemi, LJ, and Vuorio, E. Collagens and collagen-related matrix components in the human and mouse eye. Prog Retin Eye Res. (2004) 23:403–34. doi: 10.1016/j.preteyeres.2004.04.002
44. Burke, JM, and Kower, HS. Collagen synthesis by rabbit neural retina in vitro and in vivo. Exp Eye Res. (1980) 31:213–26. doi: 10.1016/0014-4835(80)90079-2
45. Guo, L, Moss, SE, Alexander, RA, Ali, RR, Fitzke, FW, and Cordeiro, MF. Retinal ganglion cell apoptosis in glaucoma is related to intraocular pressure and IOP-induced effects on extracellular matrix. Invest Ophthalmol Vis Sci. (2005) 46:175–82. doi: 10.1167/iovs.04-0832
46. Hara, M, Kobayakawa, K, Ohkawa, Y, Kumamaru, H, Yokota, K, Saito, T, et al. Interaction of reactive astrocytes with type I collagen induces astrocytic scar formation through the integrin-N-cadherin pathway after spinal cord injury. Nat Med. (2017) 23:818–28. doi: 10.1038/nm.4354
47. Jerdan, JA, and Glaser, BM. Retinal microvessel extracellular matrix: an immunofluorescent study. Invest Ophthalmol Vis Sci. (1986) 27:194–203.
48. Bu, SC, Kuijer, R, van der Worp, RJ, Li, XR, Hooymans, JMM, and Los, LI. The ultrastructural localization of type II, IV, and VI collagens at the vitreoretinal Interface. PLoS One. (2015) 10:e0134325. doi: 10.1371/journal.pone.0134325
49. Ponsioen, TL, van der Worp, RJ, van Luyn, MJA, Hooymans, JMM, and Los, LI. Packages of vitreous collagen (type II) in the human retina: an indication of postnatal collagen turnover? Exp Eye Res. (2005) 80:643–50. doi: 10.1016/j.exer.2004.11.014
50. Kobuch, K, Wild, B, Eckert, E, Fischbach, C, and Gabel, V. Expression of collagen type II by hyalocytes in vitro-first steps towards a biological vitreous substitute. Invest Ophthalmol Vis Sci. (2002) 43:3495.
51. Wang, X, Fan, J, Zhang, M, Ni, Y, and Xu, G. Upregulation of SOX9 in glial (Müller) cells in retinal light damage of rats. Neurosci Lett. (2013) 556:140–5. doi: 10.1016/j.neulet.2013.10.005
52. Candiello, J, Cole, GJ, and Halfter, W. Age-dependent changes in the structure, composition and biophysical properties of a human basement membrane. Matrix Biol. (2010) 29:402–10. doi: 10.1016/j.matbio.2010.03.004
53. Halfter, W, Willem, M, and Mayer, U. Basement membrane–dependent survival of retinal ganglion cells. Invest Ophthalmol Vis Sci. (2005) 46:1000–9. doi: 10.1167/iovs.04-1185
54. Gong, J, Sagiv, O, Cai, H, Tsang, SH, and Del Priore, LV. Effects of extracellular matrix and neighboring cells on induction of human embryonic stem cells into retinal or retinal pigment epithelial progenitors. Exp Eye Res. (2008) 86:957–65. doi: 10.1016/j.exer.2008.03.014
55. Tezel, TH, Del Priore, LV, and Kaplan, HJ. Reengineering of aged Bruch’s membrane to enhance retinal pigment epithelium repopulation. Invest Ophthalmol Vis Sci. (2004) 45:3337–48. doi: 10.1167/iovs.04-0193
56. Ponsioen, TL, van Luyn, MJA, van der Worp, RJ, van Meurs, JC, Hooymans, JMM, and Los, LI. Collagen distribution in the human vitreoretinal Interface. Invest Ophthalmol Vis Sci. (2008) 49:4089–95. doi: 10.1167/iovs.07-1456
57. Marneros, AG, Keene, DR, Hansen, U, Fukai, N, Moulton, K, Goletz, PL, et al. Collagen XVIII/endostatin is essential for vision and retinal pigment epithelial function. EMBO J. (2004) 23:89–99. doi: 10.1038/sj.emboj.7600014
58. Smith, PD, Coulson-Thomas, VJ, Foscarin, S, Kwok, JCF, and Fawcett, JW. “GAG-ing with the neuron”: the role of glycosaminoglycan patterning in the central nervous system. Exp Neurol. (2015) 274:100–14. doi: 10.1016/j.expneurol.2015.08.004
59. Barber, AC, Hippert, C, Duran, Y, West, EL, Bainbridge, JWB, Warre-Cornish, K, et al. Repair of the degenerate retina by photoreceptor transplantation. Proc Natl Acad Sci USA. (2013) 110:354–9. doi: 10.1073/pnas.1212677110
60. Fawcett, JW, Oohashi, T, and Pizzorusso, T. The roles of perineuronal nets and the perinodal extracellular matrix in neuronal function. Nat Rev Neurosci. (2019) 20:451–65. doi: 10.1038/s41583-019-0196-3
61. Pearson, CS, Solano, AG, Tilve, SM, Mencio, CP, Martin, KR, and Geller, HM. Spatiotemporal distribution of chondroitin sulfate proteoglycans after optic nerve injury in rodents. Exp Eye Res. (2020) 190:107859. doi: 10.1016/j.exer.2019.107859
62. Matsuyama, A, Kalargyrou, AA, Smith, AJ, Ali, RR, and Pearson, RA. A comprehensive atlas of aggrecan, Versican, Neurocan and Phosphacan expression across time in wildtype retina and in retinal degeneration. Sci Rep. (2022) 12:7282. doi: 10.1038/s41598-022-11204-w
63. Dillinger, AE, Bauer, N, Schneider, M, Seitz, R, Fuchshofer, R, and Tamm, ER. Versican GAG-α domain deficiency causes rosette formation and detachment of the sensory retina in the mouse eye. Invest Ophthalmol Vis Sci. (2022) 63:1961–F0379.
64. Chen, L-F, Fitz Gibbon, T, He, J-R, and Yin, ZQ. Localization and developmental expression patterns of CSPG-cs56 (aggrecan) in normal and dystrophic retinas in two rat strains. Exp Neurol. (2012) 234:488–98. doi: 10.1016/j.expneurol.2012.01.023
65. Eastlake, K, Banerjee, PJ, Angbohang, A, Charteris, DG, Khaw, PT, and Limb, GA. Müller glia as an important source of cytokines and inflammatory factors present in the gliotic retina during proliferative vitreoretinopathy. Glia. (2016) 64:495–506. doi: 10.1002/glia.22942
66. Reinhard, J, Renner, M, Wiemann, S, Shakoor, DA, Stute, G, Dick, HB, et al. Ischemic injury leads to extracellular matrix alterations in retina and optic nerve. Sci Rep. (2017) 7:43470. doi: 10.1038/srep43470
67. Wohl, SG, Jorstad, NL, Levine, EM, and Reh, TA. Müller glial microRNAs are required for the maintenance of glial homeostasis and retinal architecture. Nat Commun. (2017) 8:1603. doi: 10.1038/s41467-017-01624-y
68. Inatani, M, Honjo, M, Otori, Y, Oohira, A, Kido, N, Tano, Y, et al. Inhibitory effects of Neurocan and Phosphacan on neurite outgrowth from retinal ganglion cells in culture. Invest Ophthalmol Vis Sci. (2001) 42:1930–8.
69. Taylor, L, Arnér, K, Engelsberg, K, and Ghosh, F. Scaffolding the retina: the interstitial extracellular matrix during rat retinal development. Int J Dev Neurosci. (2015) 42:46–58. doi: 10.1016/j.ijdevneu.2015.03.002
70. Stenzel, D, Lundkvist, A, Sauvaget, D, Busse, M, Graupera, M, van der Flier, A, et al. Integrin-dependent and-independent functions of astrocytic fibronectin in retinal angiogenesis. Development. (2011) 138:4451–63. doi: 10.1242/dev.071381
71. Chen, W, Mo, W, Sun, K, Huang, X, Zhang, Y, and Song, H. Microplasmin degrades fibronectin and laminin at vitreoretinal interface and outer retina during enzymatic vitrectomy. Curr Eye Res. (2009) 34:1057–64. doi: 10.3109/02713680903308487
72. Deeg, CA, Eberhardt, C, Hofmaier, F, Amann, B, and Hauck, SM. Osteopontin and fibronectin levels are decreased in vitreous of autoimmune uveitis and retinal expression of both proteins indicates ECM re-Modeling. PLoS One. (2011) 6:e27674. doi: 10.1371/journal.pone.0027674
73. Vecino, E, Heller, JP, Veiga-Crespo, P, Martin, KR, and Fawcett, JW. Influence of extracellular matrix components on the expression of integrins and regeneration of adult retinal ganglion cells. PLoS One. (2015) 10:e0125250. doi: 10.1371/journal.pone.0125250
74. Domogatskaya, A, Rodin, S, and Tryggvason, K. Functional diversity of laminins. Annu Rev Cell Dev Biol. (2012) 28:523–53. doi: 10.1146/annurev-cellbio-101011-155750
75. Paulsson, M. The role of laminin in attachment, growth, and differentiation of cultured cells: a brief review. Cytotechnology. (1992) 9:99–106. doi: 10.1007/BF02521736
76. Edwards, MM, and Lefebvre, O. Laminins and retinal vascular development. Cell Adhes Migr. (2013) 7:82–9. doi: 10.4161/cam.22480
77. Libby, RT, Champliaud, M-F, Claudepierre, T, Xu, Y, Gibbons, EP, Koch, M, et al. Laminin expression in adult and developing retinae: evidence of two novel CNS laminins. J Neurosci. (2000) 20:6517–28. doi: 10.1523/JNEUROSCI.20-17-06517.2000
78. Dorgau, B, Felemban, M, Sharpe, A, Bauer, R, Hallam, D, Steel, DH, et al. Laminin γ3 plays an important role in retinal lamination, photoreceptor organisation and ganglion cell differentiation. Cell Death Dis. (2018) 9:615–3. doi: 10.1038/s41419-018-0648-0
79. Méhes, E, Czirók, A, Hegedüs, B, Szabó, B, Vicsek, T, Satz, J, et al. Dystroglycan is involved in laminin-1-stimulated motility of Müller glial cells: combined velocity and directionality analysis. Glia. (2005) 49:492–500. doi: 10.1002/glia.20135
80. Pereiro, X, Ruzafa, N, Acera, A, Urcola, A, and Vecino, E. Optimization of a method to isolate and culture adult porcine, rats and mice Müller glia in order to study retinal diseases. Front Cell Neurosci. (2020) 14:7. doi: 10.3389/fncel.2020.00007
81. Reinhard, J, Roll, L, and Faissner, A. Tenascins in retinal and optic nerve neurodegeneration. Front Integr Neurosci. (2017) 11:11. doi: 10.3389/fnint.2017.00030
82. Faissner, A, Roll, L, and Theocharidis, U. Tenascin-C in the matrisome of neural stem and progenitor cells. Mol Cell Neurosci. (2017) 81:22–31. doi: 10.1016/j.mcn.2016.11.003
83. Garwood, J, Garcion, E, Dobbertin, A, Heck, N, Calco, V, Ffrench-Constant, C, et al. The extracellular matrix glycoprotein tenascin-C is expressed by oligodendrocyte precursor cells and required for the regulation of maturation rate, survival and responsiveness to platelet-derived growth factor. Eur J Neurosci. (2004) 20:2524–40. doi: 10.1111/j.1460-9568.2004.03727.x
84. Kiernan, BW, Garcion, E, Ferguson, J, Frost, EE, Torres, EM, Dunnett, SB, et al. Myelination and behaviour of tenascin-C null transgenic mice. Eur J Neurosci. (1999) 11:3082–92. doi: 10.1046/j.1460-9568.1999.00729.x
85. Eastlake, K, Heywood, WE, Banerjee, P, Bliss, E, Mills, K, Khaw, PT, et al. Comparative proteomic analysis of normal and gliotic PVR retina and contribution of Müller glia to this profile. Exp Eye Res. (2018) 177:197–207. doi: 10.1016/j.exer.2018.08.016
86. Wiemann, S, Reinhard, J, Reinehr, S, Cibir, Z, Joachim, SC, and Faissner, A. Loss of the extracellular matrix molecule tenascin-C leads to absence of reactive gliosis and promotes anti-inflammatory cytokine expression in an autoimmune glaucoma mouse model. Front Immunol. (2020) 11:11. doi: 10.3389/fimmu.2020.566279
87. Wiemann, S, Yousf, A, Joachim, SC, Peters, C, Mueller-Buehl, AM, Wagner, N, et al. Knock-out of tenascin-C ameliorates ischemia-induced rod-photoreceptor degeneration and retinal dysfunction. Front Neurosci. (2021) 15:642176. doi: 10.3389/fnins.2021.642176
88. Roll, L, and Faissner, A. Tenascins in CNS lesions. Semin Cell Dev Biol. (2019) 89:118–24. doi: 10.1016/j.semcdb.2018.09.012
89. Besser, M, Jagatheaswaran, M, Reinhard, J, Schaffelke, P, and Faissner, A. Tenascin C regulates proliferation and differentiation processes during embryonic retinogenesis and modulates the de-differentiation capacity of Müller glia by influencing growth factor responsiveness and the extracellular matrix compartment. Dev Biol. (2012) 369:163–76. doi: 10.1016/j.ydbio.2012.05.020
90. Siddiqui, S, Horvat-Bröcker, A, and Faissner, A. The glia-derived extracellular matrix glycoprotein tenascin-C promotes embryonic and postnatal retina axon outgrowth via the alternatively spliced fibronectin type III domain TNfnD. Neuron Glia Biol. (2008) 4:271–83. doi: 10.1017/S1740925X09990020
91. Siddiqui, S, Horvat-Broecker, A, and Faissner, A. Comparative screening of glial cell types reveals extracellular matrix that inhibits retinal axon growth in a chondroitinase ABC-resistant fashion. Glia. (2009) 57:1420–38. doi: 10.1002/glia.20860
92. Lu, P, Takai, K, Weaver, VM, and Werb, Z. Extracellular matrix degradation and Remodeling in development and disease. Cold Spring Harb Perspect Biol. (2011) 3:a005058. doi: 10.1101/cshperspect.a005058
93. Bonnans, C, Chou, J, and Werb, Z. Remodelling the extracellular matrix in development and disease. Nat Rev Mol Cell Biol. (2014) 15:786–801. doi: 10.1038/nrm3904
94. Page-McCaw, A, Ewald, AJ, and Werb, Z. Matrix metalloproteinases and the regulation of tissue remodelling. Nat Rev Mol Cell Biol. (2007) 8:221–33. doi: 10.1038/nrm2125
95. Boguszewska-Czubara, A, Budzynska, B, Skalicka-Wozniak, K, and Kurzepa, J. Perspectives and new aspects of metalloproteinases’ inhibitors in the therapy of CNS disorders: from chemistry to medicine. Curr Med Chem. (2019) 26:3208–24. doi: 10.2174/0929867325666180514111500
96. De Groef, L, Van Hove, I, Dekeyster, E, Stalmans, I, and Moons, L. MMPs in the neuroretina and optic nerve: modulators of glaucoma pathogenesis and repair? Invest Ophthalmol Vis Sci. (2014) 55:1953–64. doi: 10.1167/iovs.13-13630
97. De Groef, L, Andries, L, Lemmens, K, Van Hove, I, and Moons, L. Matrix metalloproteinases in the mouse retina: a comparative study of expression patterns and MMP antibodies. BMC Ophthalmol. (2015) 15:187. doi: 10.1186/s12886-015-0176-y
98. Limb, GA, Daniels, JT, Pleass, R, Charteris, DG, Luthert, PJ, and Khaw, PT. Differential expression of matrix metalloproteinases 2 and 9 by glial Müller cells. Am J Pathol. (2002) 160:1847–55. doi: 10.1016/S0002-9440(10)61131-5
99. Zhang, Y, Klassen, HJ, Tucker, BA, Perez, M-TR, and Young, MJ. CNS progenitor cells promote a permissive environment for neurite outgrowth via a matrix Metalloproteinase-2-dependent mechanism. J Neurosci. (2007) 27:4499–506. doi: 10.1523/JNEUROSCI.0200-07.2007
100. Gaublomme, D, Buyens, T, De Groef, L, Stakenborg, M, Janssens, E, Ingvarsen, S, et al. Matrix metalloproteinase 2 and membrane type 1 matrix metalloproteinase co-regulate axonal outgrowth of mouse retinal ganglion cells. J Neurochem. (2014) 129:966–79. doi: 10.1111/jnc.12703
101. Andries, L, Masin, L, Salinas-Navarro, M, Zaunz, S, Claes, M, Bergmans, S, et al. MMP2 modulates inflammatory response during axonal regeneration in the murine visual system. Cells. (2021) 10:1672. doi: 10.3390/cells10071672
102. Monaco, S, Sparano, V, Gioia, M, Sbardella, D, Di Pierro, D, Marini, S, et al. Enzymatic processing of collagen IV by MMP-2 (gelatinase A) affects neutrophil migration and it is modulated by extracatalytic domains. Protein Sci. (2006) 15:2805–15. doi: 10.1110/ps.062430706
103. Rosenberg, GA. Matrix metalloproteinases and their multiple roles in neurodegenerative diseases. Lancet Neurol. (2009) 8:205–16. doi: 10.1016/S1474-4422(09)70016-X
104. Van Hove, I, Lefevere, E, De Groef, L, Sergeys, J, Salinas-Navarro, M, Libert, C, et al. MMP-3 deficiency alleviates endotoxin-induced acute inflammation in the posterior eye segment. Int J Mol Sci. (2016) 17:1825. doi: 10.3390/ijms17111825
105. Lefevere, E, Salinas-Navarro, M, Andries, L, Noterdaeme, L, Etienne, I, Van Wonterghem, E, et al. Tightening the retinal glia limitans attenuates neuroinflammation after optic nerve injury. Glia. (2020) 68:2643–60. doi: 10.1002/glia.23875
106. George, AK, Homme, RP, Majumder, A, Tyagi, SC, and Singh, M. Effect of MMP-9 gene knockout on retinal vascular form and function. Physiol Genomics. (2019) 51:613–22. doi: 10.1152/physiolgenomics.00041.2019
107. Lee, E-J, Zheng, M, Craft, CM, and Jeong, S. Matrix metalloproteinase-9 (MMP-9) and tissue inhibitor of metalloproteinases 1 (TIMP-1) are localized in the nucleus of retinal Müller glial cells and modulated by cytokines and oxidative stress. PLoS One. (2021) 16:e0253915. doi: 10.1371/journal.pone.0253915
108. Cao, L, Wang, H, and Wang, F. Amyloid-β-induced matrix metalloproteinase-9 secretion is associated with retinal pigment epithelial barrier disruption. Int J Mol Med. (2013) 31:1105–12. doi: 10.3892/ijmm.2013.1310
109. Silva, NJ, Nagashima, M, Li, J, Kakuk-Atkins, L, Ashrafzadeh, M, Hyde, DR, et al. Inflammation and matrix metalloproteinase 9 (Mmp-9) regulate photoreceptor regeneration in adult zebrafish. Glia. (2020) 68:1445–65. doi: 10.1002/glia.23792
110. Rocks, N, Paulissen, G, El Hour, M, Quesada, F, Crahay, C, Gueders, M, et al. Emerging roles of ADAM and ADAMTS metalloproteinases in cancer. Biochimie. (2008) 90:369–79. doi: 10.1016/j.biochi.2007.08.008
111. Scharfenberg, F, Helbig, A, Sammel, M, Benzel, J, Schlomann, U, Peters, F, et al. Degradome of soluble ADAM10 and ADAM17 metalloproteases. Cell Mol Life Sci. (2020) 77:331–50. doi: 10.1007/s00018-019-03184-4
112. Toonen, JA, Ronchetti, A, and Sidjanin, DJ. A Disintegrin and Metalloproteinase10 (ADAM10) regulates NOTCH Signaling during early retinal development. PLoS One. (2016) 11:e0156184. doi: 10.1371/journal.pone.0156184
113. Abu El-Asrar, AM, Nawaz, MI, Ahmad, A, De Zutter, A, Siddiquei, MM, Blanter, M, et al. Evaluation of Proteoforms of the transmembrane chemokines CXCL16 and CX3CL1, their receptors, and their processing metalloproteinases ADAM10 and ADAM17 in proliferative diabetic retinopathy. Front Immunol. (2021) 11:601639. doi: 10.3389/fimmu.2020.601639
114. Cisneros, E, di Marco, F, Rueda-Carrasco, J, Lillo, C, Pereyra, G, Martín-Bermejo, MJ, et al. Sfrp1 deficiency makes retinal photoreceptors prone to degeneration. Sci Rep. (2020) 10:5115. doi: 10.1038/s41598-020-61970-8
115. Chen, Y, Hehr, CL, Atkinson-Leadbeater, K, Hocking, JC, and McFarlane, S. Targeting of retinal axons requires the metalloproteinase ADAM10. J Neurosci. (2007) 27:8448–56. doi: 10.1523/JNEUROSCI.1841-07.2007
116. Rose, KWJ, Taye, N, Karoulias, SZ, and Hubmacher, D. Regulation of ADAMTS proteases. Front Mol Biosci. (2021) 8:8. doi: 10.3389/fmolb.2021.701959
117. Abu El-Asrar, AM, Nawaz, MI, Allegaert, E, Siddiquei, MM, Ahmad, A, Gikandi, P, et al. Differential expression and localization of ADAMTS proteinases in proliferative diabetic retinopathy. Molecules. (2022) 27:5977. doi: 10.3390/molecules27185977
118. Cua, RC, Lau, LW, Keough, MB, Midha, R, Apte, SS, and Yong, VW. Overcoming neurite-inhibitory chondroitin sulfate proteoglycans in the astrocyte matrix. Glia. (2013) 61:972–84. doi: 10.1002/glia.22489
119. Mohamedi, Y, Fontanil, T, Cobo, T, Cal, S, and Obaya, AJ. New insights into ADAMTS metalloproteases in the central nervous system. Biomol Ther. (2020) 10:403. doi: 10.3390/biom10030403
120. Hamel, MG, Mayer, J, Leonardo, CC, Zuo, F, Sandy, JD, and Gottschall, PE. Multimodal Signaling by the ADAMTSs (a disintegrin and metalloproteinase with thrombospondin motifs) promote neurite extension. Exp Neurol. (2008) 210:428–40. doi: 10.1016/j.expneurol.2007.11.014
121. Bekhouche, M, and Colige, A. The procollagen N-proteinases ADAMTS2, 3 and 14 in pathophysiology. Matrix Biol. (2015) 44–46:46–53. doi: 10.1016/j.matbio.2015.04.001
122. Levi, M, Scully, M, and Singer, M. The role of ADAMTS-13 in the coagulopathy of sepsis. J Thromb Haemost. (2018) 16:646–51. doi: 10.1111/jth.13953
123. Lee, M, Rodansky, ES, Smith, JK, and Rodgers, GM. ADAMTS13 promotes angiogenesis and modulates VEGF-induced angiogenesis. Microvasc Res. (2012) 84:109–15. doi: 10.1016/j.mvr.2012.05.004
124. Gabbiani, G. The myofibroblast in wound healing and fibrocontractive diseases. J Pathol. (2003) 200:500–3. doi: 10.1002/path.1427
125. Galie, PA, Westfall, MV, and Stegemann, JP. Reduced serum content and increased matrix stiffness promote the cardiac myofibroblast transition in 3D collagen matrices. Cardiovasc Pathol. (2011) 20:325–33. doi: 10.1016/j.carpath.2010.10.001
126. Guvendiren, M, Perepelyuk, M, Wells, RG, and Burdick, JA. Hydrogels with differential and patterned mechanics to study stiffness-mediated myofibroblastic differentiation of hepatic stellate cells. J Mech Behav Biomed Mater. (2014) 38:198–208. doi: 10.1016/j.jmbbm.2013.11.008
127. Hoerster, R, Muether, PS, Vierkotten, S, Hermann, MM, Kirchhof, B, and Fauser, S. Upregulation of TGF-ß1 in experimental proliferative vitreoretinopathy is accompanied by epithelial to mesenchymal transition. Graefes Arch Clin Exp Ophthalmol. (2014) 252:11–6. doi: 10.1007/s00417-013-2377-5
128. Bringmann, A, and Wiedemann, P. Müller glial cells in retinal disease. Ophthalmologica. (2012) 227:1–19. doi: 10.1159/000328979
129. Wan, J, and Goldman, D. Retina regeneration in zebrafish. Curr Opin Genet Dev. (2016) 40:41–7. doi: 10.1016/j.gde.2016.05.009
130. Bringmann, A, Pannicke, T, Biedermann, B, Francke, M, Iandiev, I, Grosche, J, et al. Role of retinal glial cells in neurotransmitter uptake and metabolism. Neurochem Int. (2009) 54:143–60. doi: 10.1016/j.neuint.2008.10.014
131. Bringmann, A, Pannicke, T, Grosche, J, Francke, M, Wiedemann, P, Skatchkov, S, et al. Müller cells in the healthy and diseased retina. Prog Retin Eye Res. (2006) 25:397–424. doi: 10.1016/j.preteyeres.2006.05.003
132. Roche, SL, Ruiz-Lopez, AM, Moloney, JN, Byrne, AM, and Cotter, TG. Microglial-induced Müller cell gliosis is attenuated by progesterone in a mouse model of retinitis pigmentosa. Glia. (2018) 66:295–310. doi: 10.1002/glia.23243
133. Rueda, EM, Hall, BM, Hill, MC, Swinton, PG, Tong, X, Martin, JF, et al. The hippo pathway blocks mammalian retinal Müller glial cell reprogramming. Cell Rep. (2019) 27:1637–1649.e6. doi: 10.1016/j.celrep.2019.04.047
134. Gui, Y, Li, J, Lu, Q, Feng, Y, Wang, M, He, W, et al. Yap/Taz mediates mTORC2-stimulated fibroblast activation and kidney fibrosis. J Biol Chem. (2018) 293:16364–75. doi: 10.1074/jbc.RA118.004073
135. Liang, M, Yu, M, Xia, R, Song, K, Wang, J, Luo, J, et al. Yap/Taz deletion in Gli+ cell-derived myofibroblasts attenuates fibrosis. J Am Soc Nephrol. (2017) 28:3278–90. doi: 10.1681/ASN.2015121354
136. Gilbert, RWD, Vickaryous, MK, and Viloria-Petit, AM. Signalling by transforming growth factor Beta isoforms in wound healing and tissue regeneration. J Dev Biol. (2016) 4:21. doi: 10.3390/jdb4020021
137. Conedera, FM, Quintela Pousa, AM, Presby, DM, Mercader, N, Enzmann, V, and Tschopp, M. Diverse Signaling by TGFβ isoforms in response to focal injury is associated with either retinal regeneration or reactive gliosis. Cell Mol Neurobiol. (2021) 41:43–62. doi: 10.1007/s10571-020-00830-5
138. Ferguson, MWJ, Duncan, J, Bond, J, Bush, J, Durani, P, So, K, et al. Prophylactic administration of avotermin for improvement of skin scarring: three double-blind, placebo-controlled, phase I/II studies. Lancet. (2009) 373:1264–74. doi: 10.1016/S0140-6736(09)60322-6
139. Ignotz, RA, and Massagué, J. Transforming growth factor-beta stimulates the expression of fibronectin and collagen and their incorporation into the extracellular matrix. J Biol Chem. (1986) 261:4337–45. doi: 10.1016/S0021-9258(17)35666-1
140. Wordinger, RJ, Sharma, T, and Clark, AF. The role of TGF-β2 and bone morphogenetic proteins in the trabecular meshwork and glaucoma. J Ocul Pharmacol Ther. (2014) 30:154–62. doi: 10.1089/jop.2013.0220
141. Fan, J, Shen, W, Lee, S-R, Mathai, AE, Zhang, R, Xu, G, et al. Targeting the Notch and TGF-β signaling pathways to prevent retinal fibrosis in vitro and in vivo. Theranostics. (2020) 10:7956–73. doi: 10.7150/thno.45192
142. Kanda, A, Noda, K, Hirose, I, and Ishida, S. TGF-β-SNAIL axis induces Müller glial-mesenchymal transition in the pathogenesis of idiopathic epiretinal membrane. Sci Rep. (2019) 9:673. doi: 10.1038/s41598-018-36917-9
143. Conedera, FM, Pousa, AMQ, Mercader, N, Tschopp, M, and Enzmann, V. The TGFβ/Notch axis facilitates Müller cell-to-epithelial transition to ultimately form a chronic glial scar. Mol Neurodegener. (2021) 16:69. doi: 10.1186/s13024-021-00482-z
144. Hiscott, P, and Wong, D. Proliferative vitreoretinopathy. In: DA Dartt, editor. Encyclopedia of the eye. Oxford: Academic Press (2010). 526–34.
145. Benner, JD, Dao, D, Butler, JW, and Hamill, KI. Intravitreal methotrexate for the treatment of proliferative vitreoretinopathy. BMJ Open Ophthalmol. (2019) 4:e000293. doi: 10.1136/bmjophth-2019-000293
146. Fisher, SK, and Lewis, GP. Müller cell and neuronal remodeling in retinal detachment and reattachment and their potential consequences for visual recovery: a review and reconsideration of recent data. Vis Res. (2003) 43:887–97. doi: 10.1016/S0042-6989(02)00680-6
147. Nagai, N, Klimava, A, Lee, W-H, Izumi-Nagai, K, and Handa, JT. CTGF is increased in basal deposits and regulates matrix production through the ERK (p42/p44mapk) MAPK and the p38 MAPK Signaling pathways. Invest Ophthalmol Vis Sci. (2009) 50:1903–10. doi: 10.1167/iovs.08-2383
148. Cui, JZ, Chiu, A, Maberley, D, Ma, P, Samad, A, and Matsubara, JA. Stage specificity of novel growth factor expression during development of proliferative vitreoretinopathy. Eye (Lond). (2007) 21:200–8. doi: 10.1038/sj.eye.6702169
149. Morescalchi, F, Duse, S, Gambicorti, E, Romano, MR, Costagliola, C, and Semeraro, F. Proliferative vitreoretinopathy after eye injuries: an overexpression of growth factors and cytokines leading to a retinal keloid. Mediat Inflamm. (2013) 2013:269787:1–12. doi: 10.1155/2013/269787
150. Flaxel, CJ, Adelman, RA, Bailey, ST, Fawzi, A, Lim, JI, Vemulakonda, GA, et al. Idiopathic epiretinal membrane and vitreomacular traction preferred practice pattern®. Ophthalmology. (2020) 127:P145–83. doi: 10.1016/j.ophtha.2019.09.022
151. Folk, JC, Adelman, RA, Flaxel, CJ, Hyman, L, Pulido, JS, and Olsen, TW. Idiopathic epiretinal membrane and vitreomacular traction preferred practice pattern(®) guidelines. Ophthalmology. (2016) 123:P152–81. doi: 10.1016/j.ophtha.2015.10.048
152. Altera, A, Tosi, GM, Regoli, M, De Benedetto, E, and Bertelli, E. The extracellular matrix complexity of idiopathic epiretinal membranes and the bilaminar arrangement of the associated internal limiting membrane in the posterior retina. Graefes Arch Clin Exp Ophthalmol. (2021) 259:2559–71. doi: 10.1007/s00417-021-05156-6
153. Bu, S-C, Kuijer, R, van der Worp, RJ, Huiskamp, EA, Renardel de Lavalette, VW, Li, X-R, et al. Glial cells and collagens in epiretinal membranes associated with idiopathic macular holes. Retina. (2014) 34:897–906. doi: 10.1097/IAE.0000000000000013
154. Krishna Chandran, AM, Coltrini, D, Belleri, M, Rezzola, S, Gambicorti, E, Romano, D, et al. Vitreous from idiopathic epiretinal membrane patients induces glial-to-mesenchymal transition in Müller cells. Biochim Biophys Acta Mol Basis Dis. (2021) 1867:166181. doi: 10.1016/j.bbadis.2021.166181
155. Bringmann, A, Unterlauft, JD, Wiedemann, R, Rehak, M, and Wiedemann, P. Morphology of partial-thickness macular defects: presumed roles of Müller cells and tissue layer interfaces of low mechanical stability. Int J Retina Vitreous. (2020) 6:28. doi: 10.1186/s40942-020-00232-1
157. Li, H, Raghunathan, V, Stamer, WD, Ganapathy, PS, and Herberg, S. Extracellular matrix stiffness and TGFβ2 regulate YAP/TAZ activity in human trabecular meshwork cells. Front Cell Dev Biol. (2022) 10:844342. doi: 10.3389/fcell.2022.844342
158. Wang, K, Read, AT, Sulchek, T, and Ethier, CR. Trabecular meshwork stiffness in glaucoma. Exp Eye Res. (2017) 158:3–12. doi: 10.1016/j.exer.2016.07.011
159. Hopkins, AA, Murphy, R, Irnaten, M, Wallace, DM, Quill, B, and O’Brien, C. The role of lamina cribrosa tissue stiffness and fibrosis as fundamental biomechanical drivers of pathological glaucoma cupping. Am J Physiol Cell Physiol. (2020) 319:C611–23. doi: 10.1152/ajpcell.00054.2020
160. Tezel, G, Luo, C, and Yang, X. Accelerated aging in glaucoma: immunohistochemical assessment of advanced glycation end products in the human retina and optic nerve head. Invest Ophthalmol Vis Sci. (2007) 48:1201–11. doi: 10.1167/iovs.06-0737
161. Tezel, G. Oxidative stress in glaucomatous neurodegeneration: mechanisms and consequences. Prog Retin Eye Res. (2006) 25:490–513. doi: 10.1016/j.preteyeres.2006.07.003
162. Vasan, S, Foiles, P, and Founds, H. Therapeutic potential of breakers of advanced glycation end product–protein crosslinks. Arch Biochem Biophys. (2003) 419:89–96. doi: 10.1016/j.abb.2003.08.016
163. Yuan, C, Mo, Y, Yang, J, Zhang, M, and Xie, X. Influences of advanced glycosylation end products on the inner blood–retinal barrier in a co-culture cell model in vitro. Open Life Sci. (2020) 15:619–28. doi: 10.1515/biol-2020-0067
164. Hernandez, H, Medina-Ortiz, WE, Luan, T, Clark, AF, and McDowell, CM. Crosstalk between transforming growth factor Beta-2 and toll-like receptor 4 in the trabecular meshwork. Invest Ophthalmol Vis Sci. (2017) 58:1811–23. doi: 10.1167/iovs.16-21331
165. Mavlyutov, TA, Myrah, JJ, Chauhan, AK, Liu, Y, and McDowell, CM. Fibronectin extra domain A (FN-EDA) causes glaucomatous trabecular meshwork, retina, and optic nerve damage in mice. Cell Biosci. (2022) 12:72. doi: 10.1186/s13578-022-00800-y
166. Thompson, K, Chen, J, Luo, Q, Xiao, Y, Cummins, TR, and Bhatwadekar, AD. Advanced glycation end (AGE) product modification of laminin downregulates Kir4.1 in retinal Müller cells. PLoS One. (2018) 13:e0193280. doi: 10.1371/journal.pone.0193280
167. Gharahkhani, P, Jorgenson, E, Hysi, P, Khawaja, AP, Pendergrass, S, Han, X, et al. Genome-wide meta-analysis identifies 127 open-angle glaucoma loci with consistent effect across ancestries. Nat Commun. (2021) 12:1258. doi: 10.1038/s41467-020-20851-4
168. Wong, WL, Su, X, Li, X, Cheung, CMG, Klein, R, Cheng, C-Y, et al. Global prevalence of age-related macular degeneration and disease burden projection for 2020 and 2040: a systematic review and meta-analysis. Lancet Glob Health. (2014) 2:e106–16. doi: 10.1016/S2214-109X(13)70145-1
169. Blasiak, J, Sobczuk, P, Pawlowska, E, and Kaarniranta, K. Interplay between aging and other factors of the pathogenesis of age-related macular degeneration. Ageing Res Rev. (2022) 81:101735. doi: 10.1016/j.arr.2022.101735
170. Malek, G, and Lad, EM. Emerging roles for nuclear receptors in the pathogenesis of age-related macular degeneration. Cell Mol Life Sci. (2014) 71:4617–36. doi: 10.1007/s00018-014-1709-x
171. Zhang, ZH, Pan, MX, Cai, JT, Weiland, JD, and Chen, K. Viscoelastic properties of the posterior eye of Normal subjects, patients with age-related macular degeneration, and pigs: viscoelastic properties of normal and AMD posterior eye. J Biomed Mater Res A. (2018) 106:2151–7. doi: 10.1002/jbm.a.36417
172. Huan, T, Cheng, S-Y, Tian, B, Punzo, C, Lin, H, Daly, M, et al. Identifying novel genes and variants in immune and coagulation pathways associated with macular degeneration. Ophthalmol Sci. (2023) 3:100206. doi: 10.1016/j.xops.2022.100206
173. Boyer, DS, Schmidt-Erfurth, U, van Lookeren Campagne, M, Henry, EC, and Brittain, C. The pathophysiology of geographic atrophy secondary to age-related macular degeneration and the complement pathway as a therapeutic target. Retina. (2017) 37:819–35. doi: 10.1097/IAE.0000000000001392
174. Caicedo, A, Espinosa-Heidmann, DG, Piña, Y, Hernandez, EP, and Cousins, SW. Blood-derived macrophages infiltrate the retina and activate muller glial cells under experimental choroidal neovascularization. Exp Eye Res. (2005) 81:38–47. doi: 10.1016/j.exer.2005.01.013
175. Fleckenstein, M, Mitchell, P, Freund, KB, Sadda, S, Holz, FG, Brittain, C, et al. The progression of geographic atrophy secondary to age-related macular degeneration. Ophthalmology. (2018) 125:369–90. doi: 10.1016/j.ophtha.2017.08.038
176. Shen, W, Lee, S-R, Yam, M, Zhu, L, Zhang, T, Pye, V, et al. A combination therapy targeting endoglin and VEGF-A prevents subretinal fibro-neovascularization caused by induced Müller cell disruption. Invest Ophthalmol Vis Sci. (2018) 59:6075–88. doi: 10.1167/iovs.18-25628
177. Edwards, MM, McLeod, DS, Bhutto, IA, Grebe, R, Duffy, M, and Lutty, GA. Subretinal glial membranes in eyes with geographic atrophy. Invest Ophthalmol Vis Sci. (2017) 58:1352–67. doi: 10.1167/iovs.16-21229
178. Guly, CM, and Forrester, JV. Investigation and management of uveitis. BMJ. (2010) 341:c4976–6. doi: 10.1136/bmj.c4976
179. Hauck, SM, Schoeffmann, S, Amann, B, Stangassinger, M, Gerhards, H, Ueffing, M, et al. Retinal Mueller glial cells trigger the Hallmark inflammatory process in autoimmune uveitis. J Proteome Res. (2007) 6:2121–31. doi: 10.1021/pr060668y
180. Wang, J-J, Zhu, M, and Le, Y-Z. Functions of Müller cell-derived vascular endothelial growth factor in diabetic retinopathy. World J Diabetes. (2015) 6:726–33. doi: 10.4239/wjd.v6.i5.726
181. Zhu, S, Luo, H, Liu, H, Ha, Y, Mays, ER, Lawrence, RE, et al. p38MAPK plays a critical role in induction of a pro-inflammatory phenotype of retinal Müller cells following zika virus infection. Antivir Res. (2017) 145:70–81. doi: 10.1016/j.antiviral.2017.07.012
182. Qu, Y, He, Y, Zhang, Y, Ma, T, Zhu, J, Miao, Y, et al. Quantified elasticity mapping of retinal layers using synchronized acoustic radiation force optical coherence elastography. Biomed Opt Express. (2018) 9:4054–63. doi: 10.1364/BOE.9.004054
183. Li, X, Sun, Q, Li, Q, Kawazoe, N, and Chen, G. Functional hydrogels with Tunable structures and properties for tissue engineering applications. Front Chem. (2018) 6:499. doi: 10.3389/fchem.2018.00499
184. Lienemann, PS, Rossow, T, Mao, AS, Vallmajo-Martin, Q, Ehrbar, M, and Mooney, DJ. Single cell-laden protease-sensitive microniches for long-term culture in 3D. Lab Chip. (2017) 17:727–37. doi: 10.1039/C6LC01444E
185. Tibbitt, MW, and Anseth, KS. Hydrogels as extracellular matrix mimics for 3D cell culture. Biotechnol Bioeng. (2009) 103:655–63. doi: 10.1002/bit.22361
186. Catoira, MC, Fusaro, L, Di Francesco, D, Ramella, M, and Boccafoschi, F. Overview of natural hydrogels for regenerative medicine applications. J Mater Sci Mater Med. (2019) 30:115. doi: 10.1007/s10856-019-6318-7
187. Hesse, E, Hefferan, TE, Tarara, JE, Haasper, C, Meller, R, Krettek, C, et al. Collagen type I hydrogel allows migration, proliferation and osteogenic differentiation of rat bone marrow stromal cells. J Biomed Mater Res A. (2010) 94:442–9. doi: 10.1002/jbm.a.32696
188. Knight, CG, Morton, LF, Peachey, AR, Tuckwell, DS, Farndale, RW, and Barnes, MJ. The collagen-binding A-domains of integrins α1β1 and α2β1Recognize the same specific amino acid sequence, GFOGER, in native (triple-helical) collagens *. J Biol Chem. (2000) 275:35–40. doi: 10.1074/jbc.275.1.35
189. Evans, MG, Al-Shakli, A, and Chari, DM. Electrophysiological properties of neurons grown on soft polymer scaffolds reveal the potential to develop neuromimetic culture environments. Integr Biol. (2019) 11:395–403. doi: 10.1093/intbio/zyz033
190. Shoulders, MD, and Raines, RT. Collagen structure and stability. Annu Rev Biochem. (2009) 78:929–58. doi: 10.1146/annurev.biochem.77.032207.120833
191. Caliari, SR, and Burdick, JA. A practical guide to hydrogels for cell culture. Nat Methods. (2016) 13:405–14. doi: 10.1038/nmeth.3839
192. Achilli, M, and Mantovani, D. Tailoring mechanical properties of collagen-based scaffolds for vascular tissue engineering: the effects of pH, temperature and ionic strength on gelation. Polymers. (2010) 2:664–80. doi: 10.3390/polym2040664
193. Walters, BD, and Stegemann, JP. Strategies for directing the structure and function of 3D collagen biomaterials across length scales. Acta Biomater. (2014) 10:1488–501. doi: 10.1016/j.actbio.2013.08.038
194. Lu, JT, Lee, CJ, Bent, SF, Fishman, HA, and Sabelman, EE. Thin collagen film scaffolds for retinal epithelial cell culture. Biomaterials. (2007) 28:1486–94. doi: 10.1016/j.biomaterials.2006.11.023
195. da Silva, RA, Roda, VM, De, P, Akamine, PS, Da Silva, DS, Siqueira, PV, et al. Blockade of the TGF-β pathway by galunisertib inhibits the glial-mesenchymal transition in Müller glial cells. Exp Eye Res. (2023) 226:109336. doi: 10.1016/j.exer.2022.109336
196. Balion, Z, Svirskienė, N, Svirskis, G, Inokaitis, H, Cėpla, V, Ulčinas, A, et al. Comparison of microglial morphology and function in primary cerebellar cell cultures on collagen and collagen-mimetic hydrogels. Biomedicines. (2022) 10:1023. doi: 10.3390/biomedicines10051023
197. Bacakova, M, Pajorova, J, Broz, A, Hadraba, D, Lopot, F, Zavadakova, A, et al. A two-layer skin construct consisting of a collagen hydrogel reinforced by a fibrin-coated polylactide nanofibrous membrane. Int J Nanomedicine. (2019) 14:5033–50. doi: 10.2147/IJN.S200782
198. Kleinman, HK, and Martin, GR. Matrigel: basement membrane matrix with biological activity. Semin Cancer Biol. (2005) 15:378–86. doi: 10.1016/j.semcancer.2005.05.004
199. Aisenbrey, EA, and Murphy, WL. Synthetic alternatives to Matrigel. Nat Rev Mater. (2020) 5:539–51. doi: 10.1038/s41578-020-0199-8
200. Xu, C, Inokuma, MS, Denham, J, Golds, K, Kundu, P, Gold, JD, et al. Feeder-free growth of undifferentiated human embryonic stem cells. Nat Biotechnol. (2001) 19:971–4. doi: 10.1038/nbt1001-971
201. Lee, S-W, Lee, HJ, Hwang, HS, Ko, K, Han, DW, and Ko, K. Optimization of Matrigel-based culture for expansion of neural stem cells. Anim Cells Syst. (2015) 19:175–80. doi: 10.1080/19768354.2015.1035750
202. Ponce, ML. Tube formation: an in vitro matrigel angiogenesis assay. Methods Mol Biol. (2009) 467:183–8. doi: 10.1007/978-1-59745-241-0_10
203. Eastlake, K, Wang, W, Jayaram, H, Murray-Dunning, C, Carr, AJF, Ramsden, CM, et al. Phenotypic and functional characterization of Müller glia isolated from induced pluripotent stem cell-derived retinal organoids: improvement of retinal ganglion cell function upon transplantation. Stem Cells Transl Med. (2019) 8:775–84. doi: 10.1002/sctm.18-0263
204. Ning, R, Zheng, D, Xie, B, Gao, G, Xu, J, Xu, P, et al. Spatial and temporal development of Müller glial cells in hiPSC-derived retinal organoids facilitates the cell enrichment and transcriptome analysis. Front Cell Neurosci. (2022) 16:820396. doi: 10.3389/fncel.2022.820396
205. Pereiro, X, Miltner, AM, La Torre, A, and Vecino, E. Effects of adult Müller cells and their conditioned media on the survival of stem cell-derived retinal ganglion cells. Cells. (2020) 9:1759. doi: 10.3390/cells9081759
206. Bonilla-Pons, SÀ, Nakagawa, S, Bahima, EG, Fernández-Blanco, Á, Pesaresi, M, D’Antin, JC, et al. Müller glia fused with adult stem cells undergo neural differentiation in human retinal models. EBioMedicine. (2022) 77:103914. doi: 10.1016/j.ebiom.2022.103914
207. Soofi, SS, Last, JA, Liliensiek, SJ, Nealey, PF, and Murphy, CJ. The elastic modulus of Matrigel as determined by atomic force microscopy. J Struct Biol. (2009) 167:216–9. doi: 10.1016/j.jsb.2009.05.005
208. Huang, X, Hang, R, Wang, X, Lin, N, Zhang, X, and Tang, B. Matrix stiffness in three-dimensional systems effects on the behavior of C3A cells. Artif Organs. (2013) 37:166–74. doi: 10.1111/j.1525-1594.2012.01546.x
209. David, A, Day, J, and Shikanov, A. Immunoisolation to prevent tissue graft rejection: current knowledge and future use. Exp Biol Med. (2016) 241:955–61. doi: 10.1177/1535370216647129
210. Lee, KY, and Mooney, DJ. Alginate: properties and biomedical applications. Prog Polym Sci. (2012) 37:106–26. doi: 10.1016/j.progpolymsci.2011.06.003
211. Ashton, RS, Banerjee, A, Punyani, S, Schaffer, DV, and Kane, RS. Scaffolds based on degradable alginate hydrogels and poly (lactide-co-glycolide) microspheres for stem cell culture. Biomaterials. (2007) 28:5518–25. doi: 10.1016/j.biomaterials.2007.08.038
212. Kataoka, K, Suzuki, Y, Kitada, M, Hashimoto, T, Chou, H, Bai, H, et al. Alginate enhances elongation of early regenerating axons in spinal cord of Young rats. Tissue Eng. (2004) 10:493–504. doi: 10.1089/107632704323061852
213. Hunt, NC, Hallam, D, Karimi, A, Mellough, CB, Chen, J, Steel, DHW, et al. 3D culture of human pluripotent stem cells in RGD-alginate hydrogel improves retinal tissue development. Acta Biomater. (2017) 49:329–43. doi: 10.1016/j.actbio.2016.11.016
214. Hu, Y, Huang, G, Tian, J, Qiu, J, Jia, Y, Feng, D, et al. Matrix stiffness changes affect astrocyte phenotype in an in vitro injury model. NPG Asia Mater. (2021) 13:1–15. doi: 10.1038/s41427-021-00304-0
215. Rocha, DN, Ferraz-Nogueira, JP, Barrias, CC, Relvas, JB, and Pêgo, AP. Extracellular environment contribution to astrogliosis—lessons learned from a tissue engineered 3D model of the glial scar. Front Cell Neurosci. (2015) 9:377. doi: 10.3389/fncel.2015.00377
216. Gheorghita Puscaselu, R, Lobiuc, A, Dimian, M, and Covasa, M. Alginate: from food industry to biomedical applications and Management of Metabolic Disorders. Polymers. (2020) 12:2417. doi: 10.3390/polym12102417
217. Kuo, CK, and Ma, PX. Maintaining dimensions and mechanical properties of ionically crosslinked alginate hydrogel scaffolds in vitro. J Biomed Mater Res A. (2008) 84A:899–907. doi: 10.1002/jbm.a.31375
218. Lin, C-C, and Metters, AT. Hydrogels in controlled release formulations: network design and mathematical modeling. Adv Drug Deliv Rev. (2006) 58:1379–408. doi: 10.1016/j.addr.2006.09.004
219. Marinval, N, and Chew, SY. Mechanotransduction assays for neural regeneration strategies: A focus on glial cells. APL Bioeng. (2021) 5:021505. doi: 10.1063/5.0037814
220. Labouesse, C, Tan, BX, Agley, CC, Hofer, M, Winkel, AK, Stirparo, GG, et al. StemBond hydrogels control the mechanical microenvironment for pluripotent stem cells. Nat Commun. (2021) 12:6132. doi: 10.1038/s41467-021-26236-5
221. Denisin, AK, and Pruitt, BL. Tuning the range of polyacrylamide gel stiffness for mechanobiology applications. ACS Appl Mater Interfaces. (2016) 8:21893–902. doi: 10.1021/acsami.5b09344
222. Tanaka, A, Fujii, Y, Kasai, N, Okajima, T, and Nakashima, H. Regulation of neuritogenesis in hippocampal neurons using stiffness of extracellular microenvironment. PLoS One. (2018) 13:e0191928. doi: 10.1371/journal.pone.0191928
223. Pogoda, K, Bucki, R, Byfield, FJ, Cruz, K, Lee, T, Marcinkiewicz, C, et al. Soft substrates containing hyaluronan mimic the effects of increased stiffness on morphology, motility, and proliferation of glioma cells. Biomacromolecules. (2017) 18:3040–51. doi: 10.1021/acs.biomac.7b00324
224. Trappmann, B, Gautrot, JE, Connelly, JT, Strange, DGT, Li, Y, Oyen, ML, et al. Extracellular-matrix tethering regulates stem-cell fate. Nat Mater. (2012) 11:642–9. doi: 10.1038/nmat3339
225. Wen, JH, Vincent, LG, Fuhrmann, A, Choi, YS, Hribar, KC, Taylor-Weiner, H, et al. Interplay of matrix stiffness and protein tethering in stem cell differentiation. Nat Mater. (2014) 13:979–87. doi: 10.1038/nmat4051
226. Keung, AJ, de Juan-Pardo, EM, Schaffer, DV, and Kumar, S. Rho GTPases mediate the mechanosensitive lineage commitment of neural stem cells. Stem Cells. (2011) 29:1886–97. doi: 10.1002/stem.746
227. Saha, K, Keung, AJ, Irwin, EF, Li, Y, Little, L, Schaffer, DV, et al. Substrate modulus directs neural stem cell behavior. Biophys J. (2008) 95:4426–38. doi: 10.1529/biophysj.108.132217
228. Jagielska, A, Norman, AL, Whyte, G, Vliet, KJV, Guck, J, and Franklin, RJM. Mechanical environment modulates biological properties of oligodendrocyte progenitor cells. Stem Cells Dev. (2012) 21:2905–14. doi: 10.1089/scd.2012.0189
229. Urbanski, MM, Kingsbury, L, Moussouros, D, Kassim, I, Mehjabeen, S, Paknejad, N, et al. Myelinating glia differentiation is regulated by extracellular matrix elasticity. Sci Rep. (2016) 6:33751. doi: 10.1038/srep33751
230. Wang, P-Y, Tsai, W-B, and Voelcker, NH. Screening of rat mesenchymal stem cell behaviour on polydimethylsiloxane stiffness gradients. Acta Biomater. (2012) 8:519–30. doi: 10.1016/j.actbio.2011.09.030
231. Wolf, MP, Salieb-Beugelaar, GB, and Hunziker, P. PDMS with designer functionalities—properties, modifications strategies, and applications. Prog Polym Sci. (2018) 83:97–134. doi: 10.1016/j.progpolymsci.2018.06.001
232. Lee, JN, Jiang, X, Ryan, D, and Whitesides, GM. Compatibility of mammalian cells on surfaces of poly(dimethylsiloxane). Langmuir. (2004) 20:11684–91. doi: 10.1021/la048562+
233. Mata, A, Fleischman, AJ, and Roy, S. Characterization of polydimethylsiloxane (PDMS) properties for biomedical micro/Nanosystems. Biomed Microdevices. (2005) 7:281–93. doi: 10.1007/s10544-005-6070-2
234. Fu, J, Wang, Y-K, Yang, MT, Desai, RA, Yu, X, Liu, Z, et al. Mechanical regulation of cell function with geometrically modulated elastomeric substrates. Nat Methods. (2010) 7:733–6. doi: 10.1038/nmeth.1487
235. Makhija, E, Jagielska, A, Zhu, L, Bost, AC, Ong, W, Chew, SY, et al. Mechanical strain alters cellular and nuclear dynamics at early stages of oligodendrocyte differentiation. Front Cell Neurosci. (2018) 12:59. doi: 10.3389/fncel.2018.00059
236. Wilson, CL, Hayward, SL, and Kidambi, S. Astrogliosis in a dish: substrate stiffness induces astrogliosis in primary rat astrocytes. RSC Adv. (2016) 6:34447–57. doi: 10.1039/C5RA25916A
237. Poitelon, Y, Lopez-Anido, C, Catignas, K, Berti, C, Palmisano, M, Williamson, C, et al. YAP and TAZ control peripheral myelination and the expression of laminin receptors in Schwann cells. Nat Neurosci. (2016) 19:879–87. doi: 10.1038/nn.4316
238. Decembrini, S, Hoehnel, S, Brandenberg, N, Arsenijevic, Y, and Lutolf, MP. Hydrogel-based milliwell arrays for standardized and scalable retinal organoid cultures. Sci Rep. (2020) 10:10275. doi: 10.1038/s41598-020-67012-7
239. Sun, X, Cui, Z, Liang, Y, Duan, C, Chan, HF, Mao, S, et al. One-stop assembly of adherent 3D retinal organoids from hiPSCs based on 3D-printed derived PDMS microwell platform. Biofabrication. (2023) 15:035005. doi: 10.1088/1758-5090/acc761
240. Chen, W-H, Cheng, S-J, Tzen, JTC, Cheng, C-M, and Lin, Y-W. Probing relevant molecules in modulating the neurite outgrowth of hippocampal neurons on substrates of different stiffness. PLoS One. (2013) 8:e83394. doi: 10.1371/journal.pone.0083394
241. Pathak, MM, Nourse, JL, Tran, T, Hwe, J, Arulmoli, J, Le, DTT, et al. Stretch-activated ion channel Piezo1 directs lineage choice in human neural stem cells. Proc Natl Acad Sci. (2014) 111:16148–53. doi: 10.1073/pnas.1409802111
242. Harris, JM, and Zalipsky, S eds. Poly(ethylene glycol): chemistry and biological applications, ACS symposium series. Washington, DC: American Chemical Society (1997).
243. M Jonker, A, A Bode, S, H Kusters, A, van Hest, JC, and Löwik, DW. Soft PEG-hydrogels with independently Tunable stiffness and RGDS-content for cell adhesion studies: soft PEG-hydrogels for cell adhesion studies. Macromol Biosci. (2015) 15:1338–47. doi: 10.1002/mabi.201500110
244. Missirlis, D, and Spatz, JP. Combined effects of PEG hydrogel elasticity and cell-adhesive coating on fibroblast adhesion and persistent migration. Biomacromolecules. (2014) 15:195–205. doi: 10.1021/bm4014827
245. Zhu, J. Bioactive modification of poly(ethylene glycol) hydrogels for tissue engineering. Biomaterials. (2010) 31:4639–56. doi: 10.1016/j.biomaterials.2010.02.044
246. Gobaa, S, Hoehnel, S, Roccio, M, Negro, A, Kobel, S, and Lutolf, MP. Artificial niche microarrays for probing single stem cell fate in high throughput. Nat Methods. (2011) 8:949–55. doi: 10.1038/nmeth.1732
247. Carthew, J, Frith, JE, Forsythe, JS, and Truong, VX. Polyethylene glycol–gelatin hydrogels with tuneable stiffness prepared by horseradish peroxidase-activated tetrazine–norbornene ligation. J Mater Chem B. (2018) 6:1394–401. doi: 10.1039/C7TB02764H
248. Lampe, KJ, Mooney, RG, Bjugstad, KB, and Mahoney, MJ. Effect of macromer weight percent on neural cell growth in 2D and 3D nondegradable PEG hydrogel culture. J Biomed Mater Res A. (2010) 94A:1162–71. doi: 10.1002/jbm.a.32787
249. Ilochonwu, BC, Mihajlovic, M, Maas-Bakker, RF, Rousou, C, Tang, M, Chen, M, et al. Hyaluronic acid-PEG-based Diels-Alder in situ forming hydrogels for sustained intraocular delivery of bevacizumab. Biomacromolecules. (2022) 23:2914–29. doi: 10.1021/acs.biomac.2c00383
250. Hertz, J, Robinson, R, Valenzuela, DA, Lavik, EB, and Goldberg, JL. A tunable synthetic hydrogel system for culture of retinal ganglion cells and amacrine cells. Acta Biomater. (2013) 9:7622–9. doi: 10.1016/j.actbio.2013.04.048
251. Baker, BM, and Chen, CS. Deconstructing the third dimension – how 3D culture microenvironments alter cellular cues. J Cell Sci. (2012) 125:3015–24. doi: 10.1242/jcs.079509
252. Bonnier, F, Keating, ME, Wróbel, TP, Majzner, K, Baranska, M, Garcia-Munoz, A, et al. Cell viability assessment using the Alamar blue assay: A comparison of 2D and 3D cell culture models. Toxicol In Vitro. (2015) 29:124–31. doi: 10.1016/j.tiv.2014.09.014
253. Edmondson, R, Broglie, JJ, Adcock, AF, and Yang, L. Three-dimensional cell culture systems and their applications in drug discovery and cell-based biosensors. Assay Drug Dev Technol. (2014) 12:207–18. doi: 10.1089/adt.2014.573
254. Duval, K, Grover, H, Han, L-H, Mou, Y, Pegoraro, AF, Fredberg, J, et al. Modeling physiological events in 2D vs. 3D cell culture. Physiology. (2017) 32:266–77. doi: 10.1152/physiol.00036.2016
255. Tse, HM, Gardner, G, Dominguez-Bendala, J, and Fraker, CA. The importance of proper oxygenation in 3D culture. Front Bioeng Biotechnol. (2021) 9:634403. doi: 10.3389/fbioe.2021.634403
256. Phillips, MJ, and Otteson, DC. Differential expression of neuronal genes in Müller glia in two-and three-dimensional cultures. Invest Ophthalmol Vis Sci. (2011) 52:1439–49. doi: 10.1167/iovs.10-6400
257. Afanasyeva, TAV, Corral-Serrano, JC, Garanto, A, Roepman, R, Cheetham, ME, and Collin, RWJ. A look into retinal organoids: methods, analytical techniques, and applications. Cell Mol Life Sci. (2021) 78:6505–32. doi: 10.1007/s00018-021-03917-4
258. Koch, TM, Münster, S, Bonakdar, N, Butler, JP, and Fabry, B. 3D traction forces in cancer cell invasion. PLoS One. (2012) 7:e33476. doi: 10.1371/journal.pone.0033476
Keywords: extracellular matrix, stiffness, Müller glia, retina, hydrogels
Citation: Prieto-López L, Pereiro X and Vecino E (2024) The mechanics of the retina: Müller glia role on retinal extracellular matrix and modelling. Front. Med. 11:1393057. doi: 10.3389/fmed.2024.1393057
Edited by:
Maria Llorian-Salvador, Vall d’Hebron Research Institute (VHIR), SpainReviewed by:
Paola Bagnoli, University of Pisa, ItalyYi-Rong Peng, University of California, Los Angeles, United States
Copyright © 2024 Prieto-López, Pereiro and Vecino. This is an open-access article distributed under the terms of the Creative Commons Attribution License (CC BY). The use, distribution or reproduction in other forums is permitted, provided the original author(s) and the copyright owner(s) are credited and that the original publication in this journal is cited, in accordance with accepted academic practice. No use, distribution or reproduction is permitted which does not comply with these terms.
*Correspondence: Xandra Pereiro, eGFuZHJhLnBlcmVpcm9AZWh1LmV1cw==; Elena Vecino, ZWxlbmEudmVjaW5vQGVodS5ldXM=