- 1Center of Regenerative Medicine, Department of Stomatology Renmin Hospital of Wuhan University, Wuhan, China
- 2Department of Orthopaedics, Shanghai Sixth People's Hospital Affiliated to Shanghai Jiao Tong University School of Medicine, Shanghai, China
- 3Sydney Dental School, Faculty of Medicine and Health, University of Sydney, Sydney, NSW, Australia
- 4Institute of Regenerative and Translational Medicine, Tianyou Hospital of Wuhan University of Science and Technology, Wuhan, China
- 5Department of Oral and Maxillofacial Surgery, Massachusetts General Hospital, Harvard Medical School, Boston, MA, United States
Bone defects resulting from severe trauma, tumors, inflammation, and other factors are increasingly prevalent. Stem cell-based therapies have emerged as a promising alternative. Dental pulp stem cells (DPSCs), sourced from dental pulp, have garnered significant attention owing to their ready accessibility and minimal collection-associated risks. Ongoing investigations into DPSCs have revealed their potential to undergo osteogenic differentiation and their capacity to secrete a diverse array of ontogenetic components, such as extracellular vesicles and cell lysates. This comprehensive review article aims to provide an in-depth analysis of DPSCs and their secretory components, emphasizing extraction techniques and utilization while elucidating the intricate mechanisms governing bone regeneration. Furthermore, we explore the merits and demerits of cell and cell-free therapeutic modalities, as well as discuss the potential prospects, opportunities, and inherent challenges associated with DPSC therapy and cell-free therapies in the context of bone regeneration.
1 Introduction
Bone defects resulting from severe trauma, tumors, inflammation, and other factors have become increasingly prevalent (1). Bone undergoes remodeling, growth, and development and have remarkable regenerative capabilities following injury (2). Bone defect of critical size exceeds the regenerative ability of ones’ leading to impaired healing and nonunion.
Ideal bone grafts should be osteoconductive, osteoinductive, biocompatible, mechanically compatible, and potentially degradable. To treat critical size bone defect, autologous bone grafting remains the gold standard in current practice (3), but is subject to several limitations, such as low availability and collection risk.
Stem cell-based therapies have garnered increasing attention in bone regeneration. Mesenchymal stem cells (MSCs), initially isolated by Friedenstein from bone marrow in 1976 (4), have been found to differentiate into various lineages, including adipocytes, chondrocytes, and osteoblasts. MSC possess immunomodulatory, anti-inflammatory, anti-apoptotic, and paracrine abilities (5). Dental pulp stem cells (DPSCs) are a type of MSC derived from dental pulp that exhibit MSC-like properties and have exceptional capabilities in nerve and bone regeneration (6), secreting various active components, including extracellular vesicles, growth factors, cytokines, and extracellular matrix (7). These cell-free components derived from diverse procedures and amenable to various formulations, such as cell lysates, extracellular vesicles, conditioned media. Several studies have shown that cell-free components of MSCs’ could offer superior therapeutic effect over MSCs treating diseases. In contrast to the substantial body of research on MSCs, DPSC-based regenerative medicine and tissue engineering applications is limited but fast growing, especially in the context of their cell-free therapeutic modalities designed for bone regeneration.
In this review, we provide a detailed overview of the latest progress about the research update on DPSCs in bone regeneration. We believe this review will serve as a guidance for researchers and practitioners to establish a clear understanding on the research update of DPSCs in bone regeneration.
2 Characteristics of bone regeneration
2.1 General properties of bone
Bone matrix mainly consists of the inorganic component, hydroxyapatite, and the organic component, type I collagen, which is a calcified intercellular matrix (8). These components constitute the infrastructure of the mechanical properties of bone, e.g., tensile and compressive strength. Four primary cell populations present in bone tissue are osteoprogenitor cells, osteoblasts, osteoclasts, and osteocytes (9). Osteocytes are predominantly located within the bone matrix, while the other three cell types are found at the edge of bone tissue. The maintenance of bone homeostasis hinges on the dynamic balance between the osteoclast and osteoblast.
Osteoblasts are mainly involved in the development of new bone and undergo three differentiation stages: osteoprogenitor cells, preosteoblasts, and osteoblasts. Osteoblasts are derived from MSCs through multiple differentiation pathways. Initially, in osteoblast cell lines, MSCs differentiate into osteoprogenitor cells (10). The expression of Sox family transcription factors marks the primary differentiation of osteoblasts. Sox4 and Sox11 (SOXC group) promote the survival of osteoprogenitor cells (11), while Sox9 indicates differentiation toward chondrocytes (12). Osteoblasts could transform into osteocytes, which interact with nerves, blood vessels, and tissue fluid to form osteons that provide structural support for the skeleton.
In addition, bone morphogenetic proteins (BMPs) also play a critical role in bone generation and repair (13), attracting the aggregation of preosteoblasts at the injured site and differentiating them into osteoblasts (14). Recombinant human bone morphogenetic protein-2 (rhBMP-2) was approved by the FDA in 2002 for use in anterior lumbar interbody fusion. However, complications have been identified, such as excessive osteogenesis, osteolysis, inflammation, edema, and carcinogenicity.
Osteoclasts originate from hematopoietic stem cells, and their function is mainly to absorb and resorb bone. Hematopoietic stem cells can differentiate into osteoblasts, macrophages, dendritic cells, and other cell types (15). Macrophage colony-stimulating factor (M-CSF) and receptor activator of NF-κB ligand (RANKL), which can be produced by stromal cells, osteoblasts, and immune system cells, are essential in the differentiation and survival of osteoclasts (16). Interestingly, they are highly expressed in osteoblasts (17). M-CSF binds to the CSF-1 receptor to activate downstream signaling and further upregulate RANK expression, thereby promoting the differentiation of hematopoietic stem cells into osteoblast precursor cells (18). When activated by RANKL-RANK signaling, osteoclast precursor cells differentiate, fuse, and interact with osteoblasts to become mature osteoclasts (19). Osteoprotegerin (OPG) is also involved in this process. OPG, produced mainly by osteoblasts, is a soluble RANKL decoy receptor that inhibits osteoclast formation and bone resorption by preventing RANKL-RANK receptor interactions (20). M-CSF can stimulate cell survival signaling through activation of thymoma virus proto-oncogene 1 (commonly known as AKT) via phosphatidylinositol 3 kinase (PI3K), but it most notably activates extracellular signal-regulated kinase (ERK) via growth factor receptor binding protein 2 [Grb-2; (21)]. All these factors can indirectly induce osteoblast precursor cells to differentiate into mature osteoblasts (Figure 1).
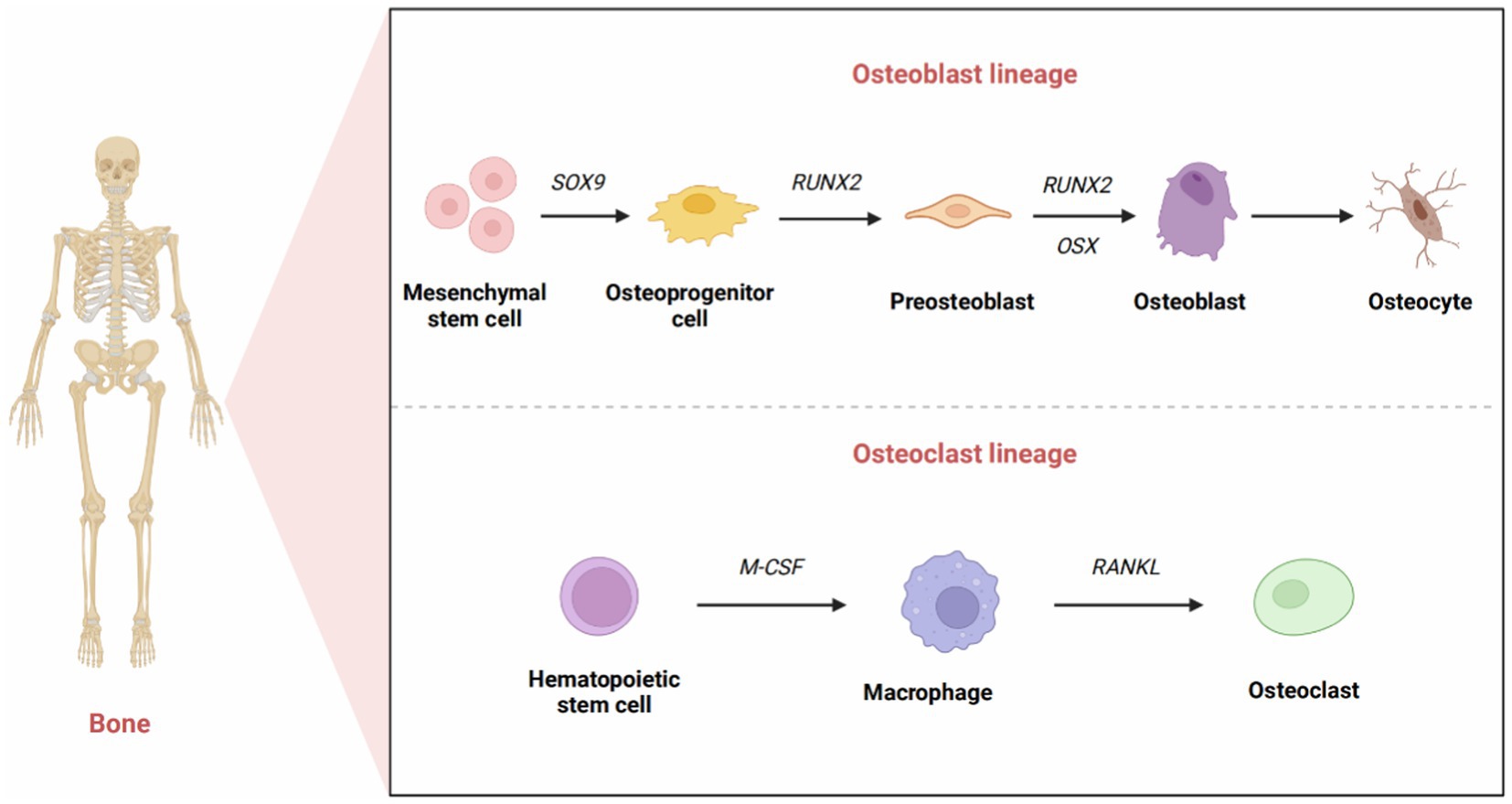
Figure 1. The development of osteoblasts and osteoclasts. The formation of mature osteoblasts has three differentiation stages: osteoprogenitor cells, preosteoblasts, and osteoblasts. Originally, the expression of Sox9 transcription factors marked the differentiation of osteoprogenitor cells. Runx2 signals the formation of preosteoblasts. The high expression of Osx reflects the differentiation of preosteoblasts into osteoblasts. Eventually, a portion of osteoblasts transformed into osteocytes that interact and combine with nerves, blood vessels, and tissue fluid to form osteons that provide structural support for the skeleton. Osteoclasts originate from hematopoietic stem cells. M-CSF promotes the proliferation of osteoclast precursors, and RANKL promotes the differentiation of mature osteoclasts.
The growth and development of human bone involve a continuous process of bone remodeling. In this process, osteoclasts absorb old bone matrix, and osteoblasts deposit sedimentary new bone matrix (8). However, bone regeneration after damage caused by trauma, tumors, or inflammation is a distinct regenerative process. This process commences with the onset of inflammation and the upregulation of proinflammatory factors at the site of damage, including tumor necrosis factor-α (TNF-α), interleukin-1 (IL-1), interleukin-6 (IL-6), and interleukin-11 (IL-11) among others (22). Subsequently, neutrophils and macrophages accumulate at injury site. The bone remodeling process occurs in four stages (19): recruitment and activation of osteoclasts at the site of injury; resorption of broken bone matrix by mature osteoclasts; differentiation of osteoprogenitor cells into mature osteoblasts; and aggregation of osteoblasts to form new bone matrix and mineralization. These stages are distinct and overlap with each other.
2.2 Pathways associated with bone regeneration
The mechanisms underlying bone regeneration have been extensively investigated, leading to the identification of various signaling pathways involved in this process (23). These pathways, such as the Hedgehog signaling (Hh) pathway, Notch signaling pathway, WNT signaling pathway, BMP/TGF-β and MAPK signaling pathway, IGF signaling pathway, and other signaling pathways (FGF signaling pathway, PI3K/Akt/mTOR signaling pathway, et al.), interact with each other to co-regulate bone regeneration (24) (Figures 2–5).
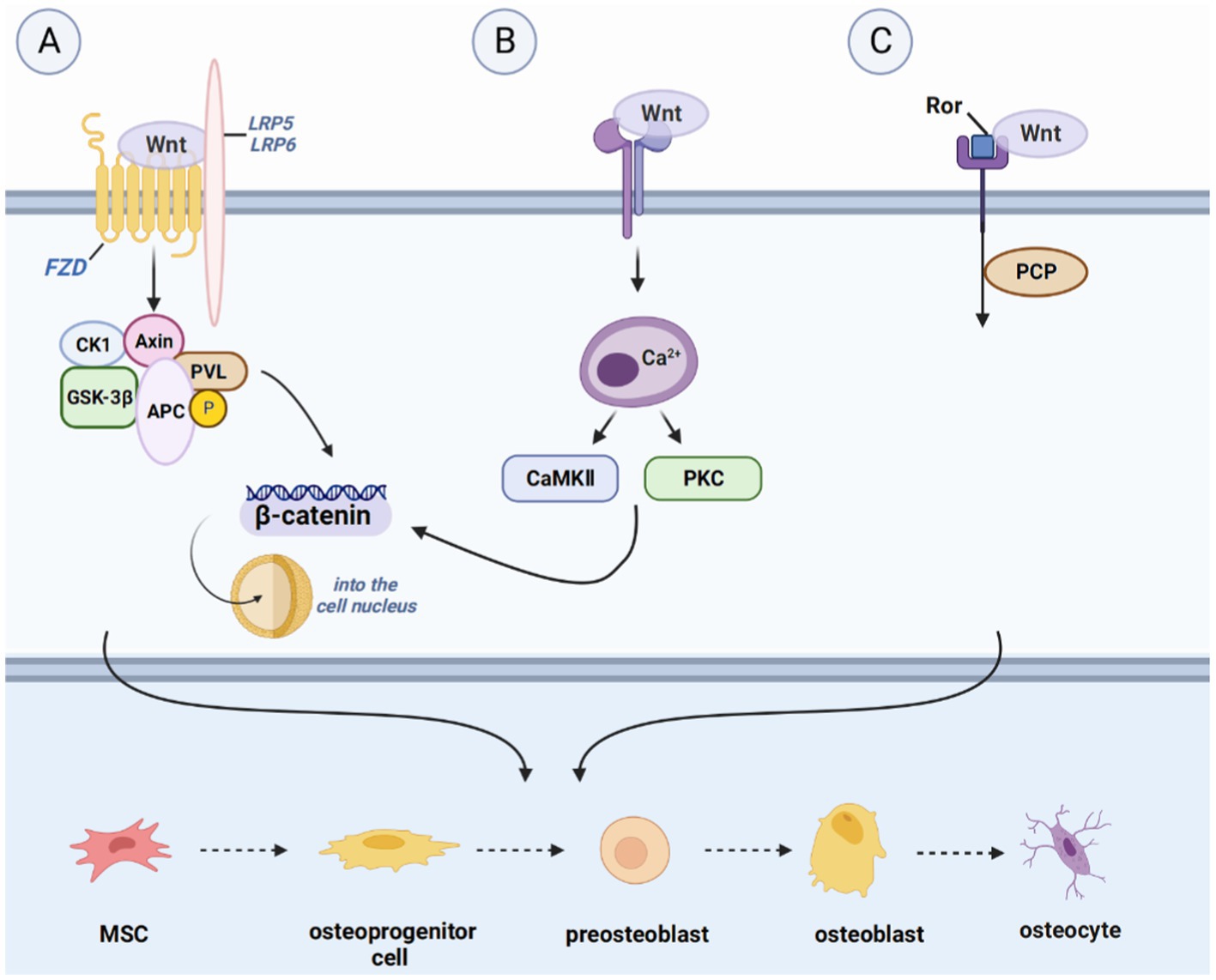
Figure 2. Introduction of pathways related to bone regeneration, like WNT signaling pathway. (A) IHH binds to Smoothened homolog (SMO) to activate GLI2 activator (GLI2A) and to prevent the cleavage of GLI3-to-GLI3 repressor (GLI3R), thus leading to the formation of osteocytes. (B) Hydrolysis of Notch proteins is achieved by Notch binding to JAG or DLL, followed by Notch intracellular structural domain (NCID) binding to EGF repeats present in the ligand to affect the expression of downstream target genes, including the split hairy enhancer (Hes) and Hes associated with the YRPW motif (Hey). Hes1 and Hey1 prevent osteoblast differentiation and maturation and promote bone resorption by inhibiting Runx2 activity, where the expression of Hes1 appears to be a key determinant of bone mass. (C) The FGF pathway activation begins with FGF binding to FGFR, with subsequent phosphorylation of tyrosine residues in the intracellular domain of FGFR, recruitment of various substrates, and activation of downstream pathways. This ultimately leads to cell proliferation, differentiation, and apoptosis.
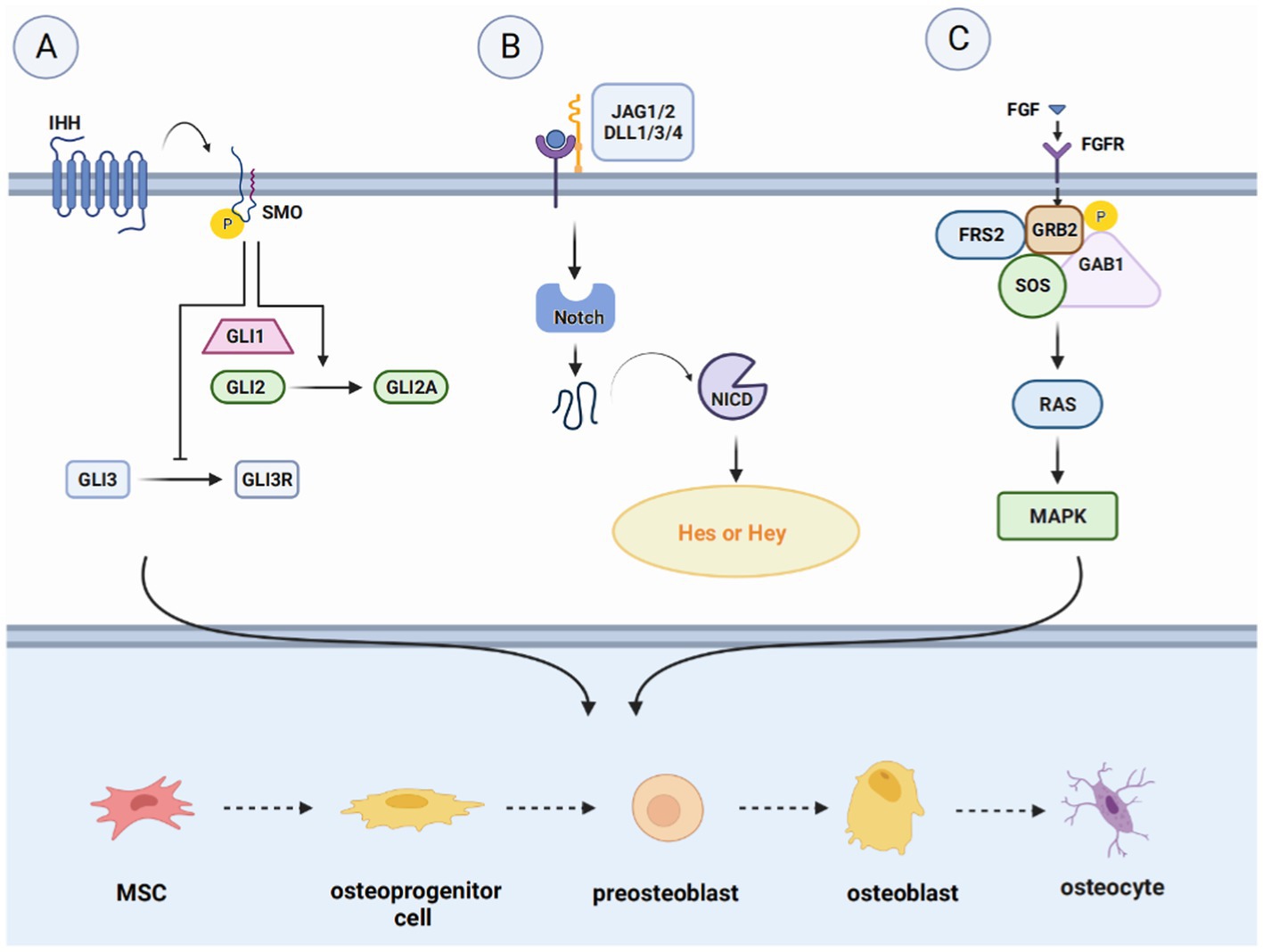
Figure 3. Introduction of pathways related to bone regeneration, like Hedgehog signaling pathway, Notch signaling pathway and FGF signaling pathway. (A) The WNT signaling pathway, divided into the WNT/β-catenin pathway, the WNT/Ca2+-dependent pathway, and the WNT/planar cell polarity (PCP) pathway. Upon binding ligands such as Wnt1 to the FZD receptor and LRP5/6 complex, Axin down-regulates and inactivates GSK-3β, inducing the accumulation of β-catenin in the cytoplasm and translocation to the nucleus to induce the expression of target genes. (B) In the Wnt/Ca2+ pathway, the increase in intracellular Ca2+ concentration activates CaMK II and protein kinase C (PKC) and facilitates the translocation of β-catenin to the nucleus. (C) Wnt activates WNT/PCP signaling through tyrosine kinase-like orphan receptor (Ror) proteins, promoting the formation of osteocytes and activation of downstream signaling pathways.
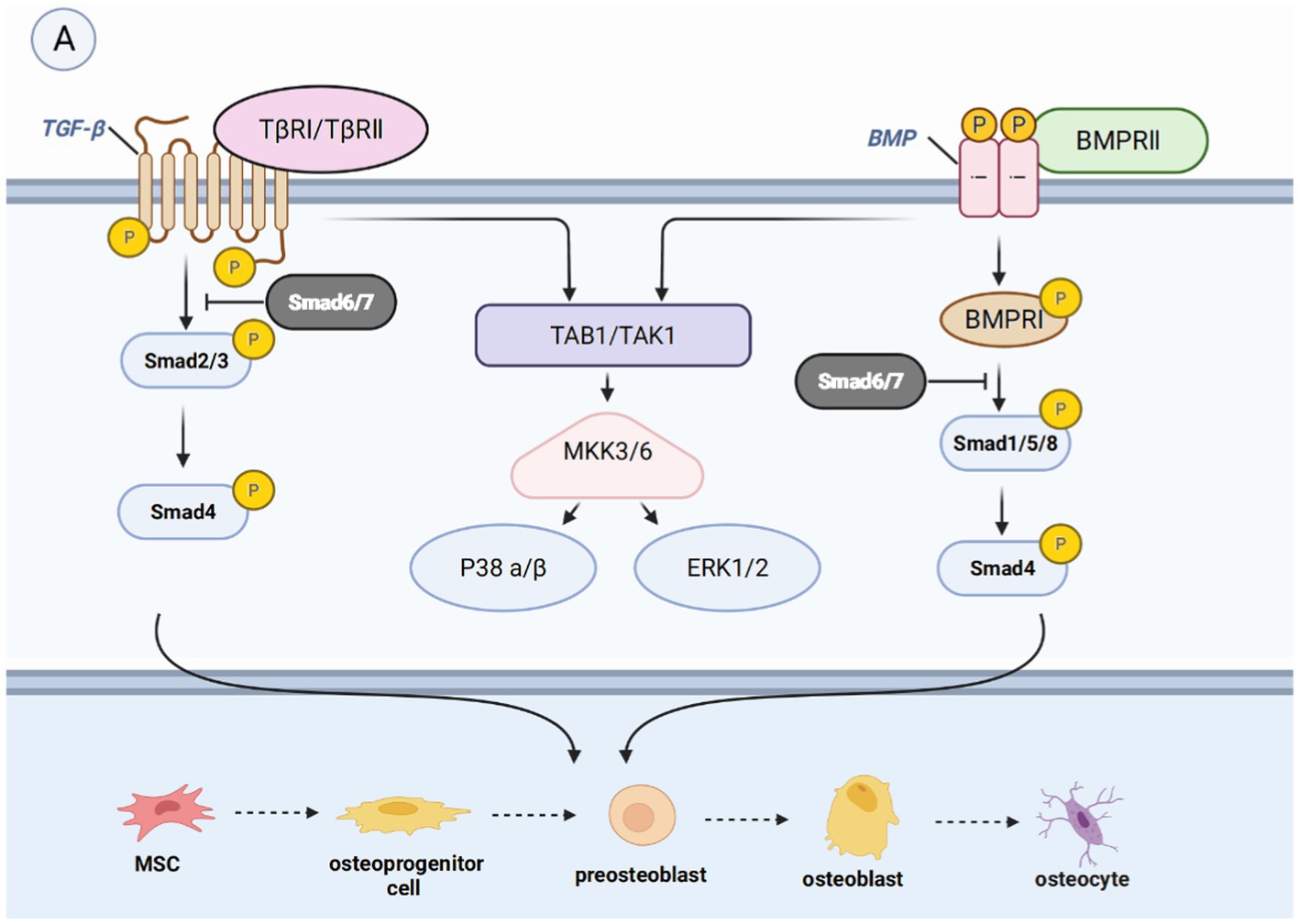
Figure 4. Introduction of pathways related to bone regeneration, like BMP/TGF-βsignaling pathway. (A) Binding of BMP leads to the phosphorylation of Smad1, Smad5, or Smad8. The binding of TGF-β leads to the phosphorylation of Smad2 or Smad3. They form a complex with Smad4, which then moves the nucleus to control gene expression and enable the transformation of mature osteoblasts. They can also activate non-smad-dependent pathways, including PI3K/AKT, TAK1, and MAPK signaling pathways, which are cascades of signaling events.
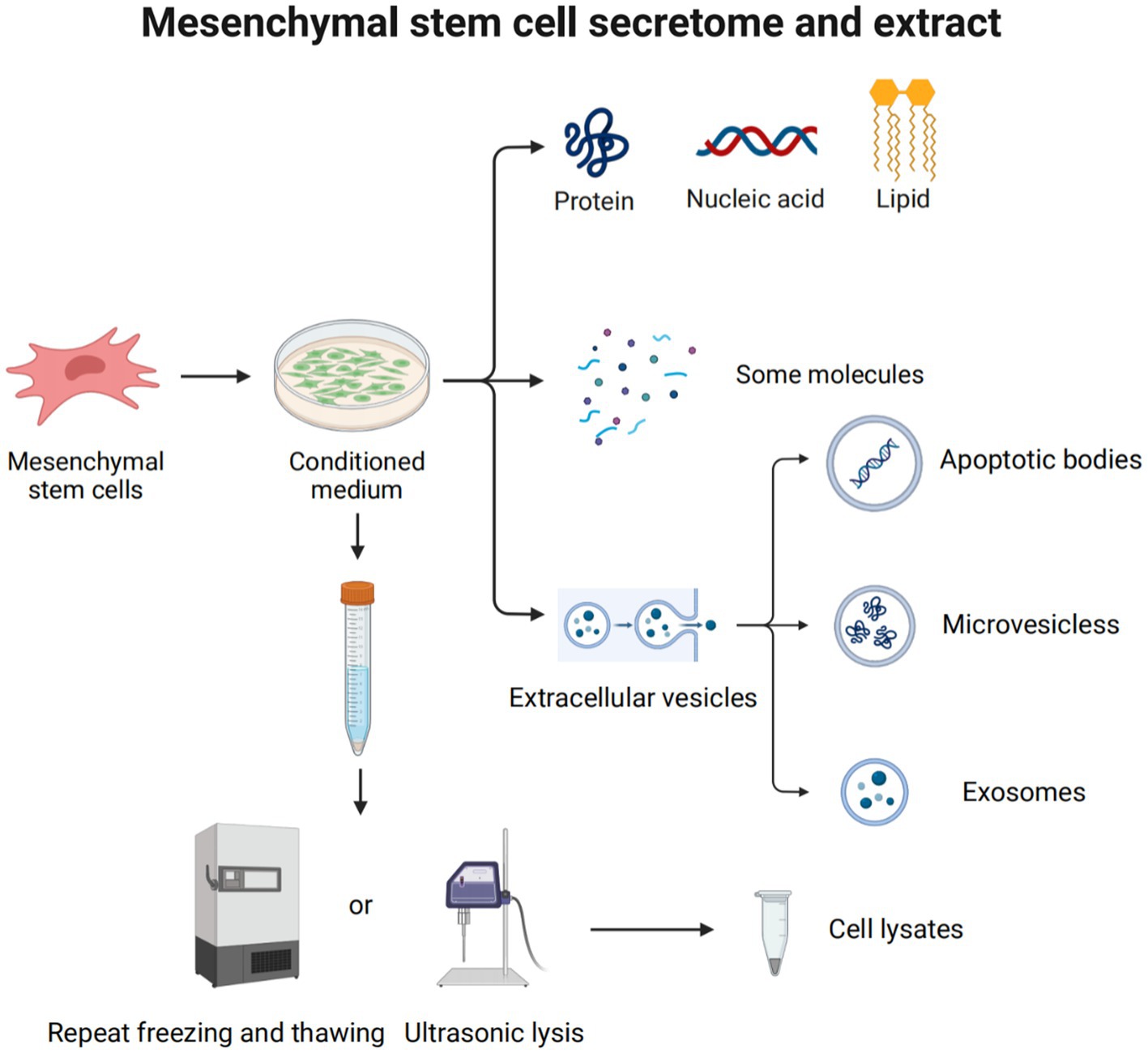
Figure 5. Mesenchymal stem cell secretome and extract. The MSC secretome, or CM, plays a major role in the use of MSCs to treat diseases. It is a variety of molecules and extracellular vehicles (EVs) secreted by MSCs into the extracellular space. These molecules include soluble proteins (such as growth factors, chemokines, enzymes, adhesion molecules, hormones, antimicrobial peptides, etc.), free nucleic acids, lipids, etc. EVs can be classified into apoptotic bodies (1–5 μm), microvesicles (250–400 nm), and exosomes (30–150 nm), according to their size and origin. Cell lysate is obtained by lysing cells through ultrasonolysis or freeze–thaw technology.
2.2.1 Hedgehog signaling pathway
The differentiation of osteoblast lineages is regulated by multiple signaling pathways, with the Hh pathway being of critical importance in bone formation. Shh is a major morphogenetic factor in limb formation, regulating patterns and growth during early limb development. Takebe et al. found that Runx2 and Osx co-localized Shh and Gli1 on the surface of bone matrix and chondrocytes 7 days after fracture in SD rats, suggesting that Shh is involved in intramembranous ossification and endochondral ossification during fracture healing (25). On the other hand, Ihh is involved in several aspects of cartilage endosteal bone development. It is upregulated during the early stages of bone regeneration, expressed in prehypertrophic and hypertrophic chondrocytes, and indirectly regulates osteoblast differentiation by controlling cartilage development.
The Gli family is an important transcription factor involved in the Hh pathway, which includes Gli1, Gli2, and Gli3 (25). As a transcriptional repressor, Gli3 appears to play a dominant role in the control of chondrocyte proliferation and hypertrophy, while Gli2 activation may have a more prominent role in angiogenesis (26). The absence of these transcription factors can lead to abnormal skeletal development or postnatal death in mice (27). Gli1 acts synergistically with Gli2 and Gli3, and there is an inhibitory effect of Gli3 inhibitors on osteoblast differentiation, which is partially mediated through inhibition of Gli1 (28).
2.2.2 Notch signaling pathway
The Notch signaling pathway plays a crucial role in regulating cell proliferation and differentiation in various tissues and organs, including skeletal development, bone metabolism, and regeneration (29). Four Notch receptors have been identified so far; they are Notch1-4 and have been identified in humans and mice. Studies have demonstrated that Notch1 overexpression leads to decreased expression of alkaline phosphatase transcripts, type I collagen, osteocalcin, and other proteins associated with bone regeneration during the differentiation of ST-2 stromal cells into osteoblasts. Additionally, Notch2 selective inhibition has been shown to hinder RANKL-induced osteoclast genes during osteoclast differentiation, while ectopic expression of Notch2 enhances NFATc1 promoter activity and promotes osteoclastogenesis (30). Notch3 activation in prostate cancer bone metastases has been shown to induce and secrete matrix metalloproteinase-3 (MMP-3), which subsequently inhibits osteoclast differentiation and enhances osteoblast proliferation (31). Pulsed electromagnetic field (PEMF) treatment has been shown to activate Notch pathways, stimulate all osteogenic markers, and increase the expression of Hey1, Dll4, and Notch4 in osteogenic media (32). This suggests that Notch4 plays a positive regulatory role in Notch signaling. It is worth noting that the stimulation or inhibition of Notch signaling in osteoblasts and osteoprogenitor cells may depend on the cellular environment, the differentiation status of the cell, or the developmental stage of bone formation (33).
Delta-like protein-1/3/4 and Jagged1/2 are five membrane-bound ligands that have been identified as activators of Notch signaling (34). Mechanistically, the binding of Notch to Jagged1/2 or Delta-like protein-1/3/4 leads to hydrolytic cleavage of Notch proteins, allowing the Notch intracellular structural domain (NCID) to bind to EGF repeats present in the ligand and affect the expression of downstream target genes. These genes include the hairy enhancer (Hes) and the Hes associated with the YRPW motif (Hey). Hes1 and Hey1 inhibit Runx2 activity, thereby preventing osteoblast differentiation and maturation and promoting bone resorption (35). Notably, the expression of Hes1 appears to be a key determinant of bone mass (36). Shen et al. have reported that in osteoblasts, Hes-1 enhances 1,25-Dihydroxyvitamin D3 (1, 25-(OH)2-D3)-induced osteopontin transcription and that this enhancement is inhibited by inhibitors of Runx2 (37).
2.2.3 WNT signaling pathway
The WNT signaling pathway is a complex and versatile pathway that is present in all major systems. WNT signaling pathway, divided into the WNT/β-catenin pathway and two non-classical pathways, the WNT/Ca2+-dependent pathway and the WNT/planar cell polarity (PCP) pathway (38). Binding of extracellular WNT ligands to the Frizzled family of transmembrane receptors as well as lipoprotein receptor-related protein-5 (LRP5) and lipoprotein receptor-related protein-6 (LRP6) co-receptors activate the classical pathway (39). Wnt ligands are a class of secretive proteins that act through autocrine or paracrine mechanisms, and 19 different Wnt ligands are present in the human body. Wnt1, Wnt2b, Wnt3, Wnt7a, and Wnt8 are primarily involved in the WNT/β-catenin pathway.
Under normal conditions, cytoplasmic β-catenin is phosphorylated by a complex of glycogen synthase-3β (GSK-3β), adenomatous polyposis coli, and Axin and subsequently rapidly degraded by the ubiqui-tin-proteasome system. However, upon Wnt stimulation, Axin down-regulates and inactivates GSK-3β, leading to the accumulation of β-catenin in the cytoplasm, which translocates to the nucleus and induces target gene epistasis via TCF/LEF1 and CBP. In vitro studies have indicated that Wnt ligands promote the differentiation of MSCs into osteoblasts by activating the WNT/β-catenin pathway, thereby enhancing osteogenic bone mass, suggesting that the WNT/β-catenin pathway is essential in the osteogenic differentiation system (40).
The non-classical pathway includes the ligands Wnt4, Wnt5a, Wnt5b, Wnt7b, and Wnt11. Ligand binding to the receptor does not induce intracellular β-catenin accumulation, but it does participate in osteoblast and osteoclast differentiation. Maeda et al. found that Wnt5a activates WNT/PCP signaling through the tyrosine kinase-like orphan receptor (Ror) protein, thereby enhancing osteoclastogenesis and promoting bone resorption (41). In the Wnt/Ca2+ pathway, an increase in intracellular Ca2+ concentration leads to the activation of calmodulin-dependent protein kinase II (CaMK II) and protein kinase C (PKC), which in turn facilitates β-catenin translocation to the nucleus (42). Kuhl et al. showed that CaMK II and PKC activated by the Wnt/Ca2+ pathway block the Wnt/β-catenin pathway upstream of β-catenin and phosphorylate the intracellular protein (43). However, its downstream mechanisms are unclear.
2.2.4 BMP/TGF-β signaling pathway
In most of the studies, it is believed that the BMP/TGF-β signaling pathway is crucial to bone biogenesis. The transcriptional growth factor beta (TGFβ) is synthesized by osteoblasts and embedded in the mineralized matrix. It is one of the most abundant cytokines present in the bone matrix, and it is more abundant than BMP in vivo. The BMP family constitutes the largest subfamily of the TGFβ superfamily. BMP is a cytokine essential for fetal tissue development and fracture repair and is considered a key factor in the lineage of MSCs to bone progenitor cells (44). The major intracellular mediators of the BMP/TGF-β signaling pathway are Smad molecules. Nine Smads molecules have been identified and defined, classified as receptor-regulated Smads (R-Smads), co-mediator Smads (Co-Smads), and inhibitory Smads (I-Smads). R-Smads consist of Smad1/2/3/5/8/9, among which Smad2/3 is mainly involved in the TGF-β signaling pathway, while Smad1/5/8/9 is mainly involved in the BMP signaling pathway. Co-Smad, also known as Smad4, is involved in the two pathways (45). I-Smad, which is divided into Smad6/7, exerts a negative regulatory effect on the TGF-β signaling pathway. In the typical Smad-dependent TGF-β or BMP signaling pathway (46), TGF-β binds to type II and type I TGF-β receptors, or BMP binds to BMP receptor II to form a heterotetrameric receptor complex. This complex activates phosphorylated R-Smads, which forms a complex with Smad4 and is subsequently recruited to the nucleus (47, 48). In the nucleus, the complex promotes the expression of osteogenic-related genes. In the presence of I-Smads, R-Smads cannot be phosphorylated to form complexes with Co-Smads, leading to the inhibition of this signaling pathway (45).
The non-Smad-dependent pathways include TAK1, MAPKs, and PI3K/AKT signaling pathways (49), which constitute cascades of signaling events. For example, phosphorylated TAK1 initiates the MKK-p38 MAPK or MKK-ERK1/2 signaling cascade by recruiting TAB1 (50, 51). Subsequently, Runx2, Dlx5, and Osx are phosphorylated to promote their transcriptional activity (52). However, some studies have demonstrated that these pathways may inhibit the osteogenic effect, indicating a dual role (53).
2.2.5 Other signaling pathways
In recent years, there has been growing evidence indicating that the fibroblast growth factor (FGF) signaling pathway holds promise as a key regulator of bone regeneration. Studies have shown that FGF stimulates the proliferation and differentiation of MSCs and osteoblasts, contributing to bone formation (54). For instance, Nakajima et al. demonstrated that the injection of basic fibroblast growth factor (bFGF) into a rat model of fracture healing accelerates the rate of fracture healing and promotes bone regeneration (55).
The regulation of the PI3K/Akt/mTOR pathway in pathways that promote bone repair has not been extensively studied. Inhibiting the PI3K/Akt/mTOR pathway can lead to osteoclast formation; activating the pathway promotes cell differentiation toward osteoblasts (56). In a study by Peng et al., Akt1 and Akt2 gene-deficient mice showed severe skeletal muscle atrophy and impaired skeletal development (57).
2.3 Cross-talk between these pathways
Bone development and regeneration involve a complex interplay of various signaling pathways that coordinate and crosstalk with each other (49). Despite the distinct roles of these pathways, they function cooperatively to ensure proper bone formation and repair. The interaction between the TGF-β/BMP pathway and the Wnt pathway is critical for early development and tissue stabilization (58). TGF-β can prevent hyperphosphate-induced osteogenesis by inhibiting the Wnt/β-catenin pathway and reducing nuclear translocation of Smad 1/5/8 in the Smad-dependent BMP signaling pathway (59). Furthermore, Wnt3a inhibits Smad1 phosphorylation via GSK3 and stabilizes Smad1 (60). At Ser-204 and Ser-208 (61), GSK3 mediates Smad3 phosphorylation and inhibits its activity. Axin promotes GSK3β-mediated Smad3 phosphorylation at Thr66, leading to Smad3 ubiquitination and degradation (62). TGF-β regulates Hes-1 transcription in a Notch-dependent manner. The intracellular domain of Notch1 (NICD) can interact with Smad3, enhancing cascade signaling (63).
Although numerous pathways not listed here are also involved in bone regeneration and development, the mechanisms underlying skeletal development and repair are primarily a result of cross-talk between these pathways.
3 DPSCs therapeutic potential in bone regeneration
Stem cell-based therapies have been a major area of focus in the fields of tissue engineering and regenerative medicine. MSCs have been isolated from a variety of human tissues, including bone marrow, peripheral blood, umbilical cord synovial fluid, dental pulp, adipose tissue, and endometrial tissue (64). MSCs are characterized by their immunomodulatory properties, multi-directional differentiation potential, and high self-renewal capacity. These cells have been demonstrated to participate in diverse processes such as immune regulation (65), neuroprotection, anti-inflammatory, anti-fibrotic, anti-oxidant, and angiogenic processes (66). They also demonstrate advantages in the treatment of bone regeneration, degenerative diseases, diabetes, wound healing, and other areas (67). While theoretically, MSCs can be isolated from any human tissue, there are limitations due to the availability of the source tissue, the invasive nature of the isolation procedure, and the different characteristics of the donor. Obtaining bone marrow mesenchymal stem cells (BMMSCs), for example, may present risks of pain, bleeding, or infection, making it challenging and ethically controversial (68). Therefore, it is crucial to select a suitable cell source, assess the difficulty of obtaining samples, and consider the rejection of allogeneic cell transplantation.
3.1 The unique characteristics of DPSCs
DPSCs have emerged as a prospective cell source for stem cell-based therapies that can be readily available from third molars, deciduous teeth, or permanent teeth (69). Third molars are usually discarded as medical waste, and two sets of teeth germinate during a person’s lifetime, indicating that DPSCs are abundant and easily accessible. DPSCs have MSC-like properties, such as multidirectional differentiation and the ability to self-renew. Compared with other sources of MSCs or progenitor cells, DPSCs are not only easy to obtain but also have low immunogenicity and avoid ethical concerns. More importantly, DPSCs can be collected without injury to the donor or invasive surgical procedures (70).
Because of their origin in the cranial neural crest lineage, DPSCs have significant neural differentiation potential, making them suitable in treatment of neurological problems (71). However, their advantages in bone regeneration are also unquestionable. DPSCs exhibit higher fibroblast colony-forming units and proliferation rates, similar gene expression profiles of mineralization-related genes, and differential osteogenic, paracrine, and immunomodulatory capacity as compared to BMMSCs (72). The osteoblasts in the craniofacial region are also derived from neural crest cells, which are derived from neural ectoderm. During the embryonic period, the neural ectoderm develops into the tissues of the craniofacial region, including the facial bones, the skull, and the dentin of the teeth (23). Therefore, DPSCs have great osteogenic potential (73).
3.2 Bone regeneration and immunomodulatory properties of DPSCs
In the past few years, the majority of scholars have shifted their focus to inducing bone regeneration through the transplantation of BMMSCs, adipose-derived mesenchymal stem cells (ADSCs), and umbilical cord-derived mesenchymal stem cells (UCMSCs). Firstly, bone marrow mesenchymal stem cells are heterogenous cell populations located in the medullary stroma of bone marrow, originating from the early development of the mesoderm and outer layer. They are the earliest-discovered and extracted type of mesenchymal stem cells. BMMSCs possess a strong ability to differentiate into bone cells and can be used for repairing bone defects (74). By implanting BMMSCs into the defective area, it is possible to promote bone tissue growth and reconstruction (75). However, due to their low proliferation capacity, high collection risks, and the painful collection process, researchers have been searching for alternative sources of mesenchymal stem cells (76). Subsequently, adipose stem cells and umbilical cord mesenchymal stem cells have been applied to treat bone defects. ADSCs, compared to other stem cells, exhibit excellent proliferation and differentiation potential (77), capable of promoting tissue regeneration and functional recovery. UCMSCs possess strong immune regulation and anti-inflammatory abilities (78), are able to alleviate inflammatory reactions and promote tissue recovery, but have a relatively weak osteogenic capability and are associated with ethical controversies (79).
Like other types of stem cells, DPSCs exert their therapeutic effects primarily through paracrine signaling. Specifically, DPSCs secrete a wide range of bioactive molecules, including regulatory factors, growth factors, cytokines, and signal peptides (80). DPSCs also release secretory proteomes, including exosomes, microvesicles, apoptotic vesicles, and other extracellular factors (81), which act in the body to provide a suitable environment for immune regulation and anti-apoptosis, maintaining overall body homeostasis. Importantly, DPSCs exhibit low immunogenicity (82), and the expression of histocompatibility complex class II antigen does not exist on DPSCs. These unique properties have led to the development of various therapeutic applications for DPSCs in treating neurological, circulatory, diabetic, liver, eye, immune, and oral diseases (83).
The regenerative potential of dental pulp stem cells (DPSCs) in the field of craniofacial bone repair is not to be underestimated. Gaus et al. conducted a study using data from the Gene Expression Omnibus database, which revealed 16 overlapping differentially expressed miRNAs and shared regulators associated with bone differentiation between DPSCs and BMMSCs, suggesting a common genetic and epigenetic mechanism for bone differentiation in both cell types (84). An in vitro study has demonstrated that DPSCs exhibit superior osteogenic potential compared to other MSCs, including BMMSCs, gingival mesenchymal stem cells (GMSCs), and adipose-derived stem cells (ADSCs), as evidenced by various assays such as fluorescence-activated cell sorting, flow cytometry, quantitative polymerase chain reaction for osteogenic gene expression, alizarin red staining, and micro-computed tomography analysis (85). However, other conflicting results have been reported (72, 86–94), which may be attributed to differences in MSCs donors and isolation techniques. Table 1 summarizes the inconsistent findings on the osteogenic potential of DPSCs. Nevertheless, DPSCs show advantages in terms of proliferation rates, cell utilization, and cell numbers compared to BMMSCs (90, 92). These advantages are reflected in Table 2. DPSCs also exhibit remarkable immunomodulatory, paracrine, anti-apoptotic, and angiogenic properties, which contribute to bone differentiation and reduce inflammation (91, 96, 98, 101). Anderson et al. showed that DPSCs reduce inflammation and induce M2 polarization in bone marrow cells (102). The same conclusion was drawn in vivo. In a rabbit cranial defect model, inoculation of BMSC or DPSC in a Bio-Oss stent and implantation into a 6-mm cranial defect promoted bone regeneration and improved osteogenesis-related protein expression at the defect site, and the bone regeneration efficacy of the two cells was shown to be compatible (94). In a temporomandibular joint arthritis rat model, local injection of DPSCs was found to alleviate inflammation and pain in the joint cavity, promote bone regeneration, and inhibit the STAT1 signaling pathway (103).
The ability of DPSCs to promote bone regeneration is mediated by various mechanisms. Ferutinin, a phytoestrogen, promotes osteogenic differentiation of the Wnt/β-catenin signaling pathway by activating H3K9 acetylation and H3K4 trimethylation in the promoter regions of Wnt3a and DVL3 (104). Similarly, adenosine A1 receptors have been shown to promote DPSCs osteogenesis through the Wnt/Dvl pathway, as evidenced by increased expression of the osteogenic markers RUNX-2 and ALP (105). AR-A014418, a glycogen synthase kinase 3β (GSK3β) inhibitor, promotes not only the proliferation and migration of DPSCs but also their osteogenic differentiation, which is achieved through the activation of the β-catenin/PI3K/Akt signaling pathway (106). DPSCs also inhibit osteoclasts by inactivating the AKT pathway through the secretion of OPG, as demonstrated by Kanji et al. (107). Melatonin affected the osteogenic differentiation ability of DPSCs through COX-2/NF-κB and p38/ERK MAPK signaling pathways, which was also verified in the rabbit calvarial defect model (108). In addition, some drugs, enzymes, hormones, or trace elements can induce DPSCs to express osteogenic-related genes through the BMP4/Smad pathway, the Erk1/2 pathway, the p38 MAPK pathway, and other pathways to promote bone regeneration and repair (109, 110). Notably, the formation and connectivity of blood vessels are crucial for osteogenesis (111), and DPSCs have been shown to possess a higher angiogenic potential than other MSCs (112).
4 Cell-free therapies based on DPSCs for bone regeneration
Cell-free therapy has emerged as a promising approach in regenerative medicine due to its potential to address some of the drawbacks and limitations linked to the use of MSCs. While the ability of DPSCs in osteogenesis is well established, there is growing evidence to suggest that the therapeutic effect of MSCs may be derived from their paracrine bioactive factors, which have a critical role in the regulation of cellular processes (67). Adverse reactions have been reported with the use of MSCs, either intravenously or topically, such as mild fever, headache, dizziness, venous obstruction, and adverse reflux (113). The survival time of MSCs after entering the body is short and is affected by the microenvironment. Furthermore, the long-term culture of MSCs may lead to a loss of phenotypic stability (80, 114). Consequently, attention has shifted toward safer and more practical cell-free therapies.
The MSC secretome, also known as conditioned medium, is a secretory molecule of MSCs that has a variety of biological activities, is actively involved in regulating cellular processes, and can be used for various therapeutic applications (115). These molecules include soluble proteins (such as growth factors, chemokines, enzymes, adhesion molecules, hormones, antimicrobial peptides, etc.), free nucleic acids, lipids, some vesicles, etc. (116). Many of these have immunomodulatory, anti-apoptotic, anti-fibrotic, and tissue-regenerative functions. Among them, extracellular vehicles (EVs) are considered important mediators of intracellular communication and are involved in the regulation of multiple signaling pathways (117). EVs can be classified into apoptotic bodies (1–5 μm), microvesicles (250–400 nm), and exosomes (30–150 nm), according to their size and origin (118). Apoptotic bodies, containing histone and DNA fragments, are the largest extracellular vesicles that split from cells during apoptosis. Microvesicles are produced by the plasma membrane budding and are rich in sphingomyelin and ceramides. Exosomes are budded from the interior of the multivesicular body, which fuses with the plasma membrane and releases exosomes outward, containing a series of evolutionarily conserved proteins like tetrameric proteins and heat shock proteins (119).
In summary, cell-free therapies in the form of the MSC secretome, or CM, could offer a safer and more practical alternative to cell therapy, with the potential for greater efficacy in the treatment of bone defects (73). The EVs within the secretome are particularly important in regulating cellular processes and communication and hold promise as therapeutic agents in their own right (67, 120).
4.1 Conditioned medium
MSCs are known to secrete a variety of substances, including numerous proteins, peptides, RNA, and lipid mediators, as well as an abundance of extracellular vesicles (EVs). Mesenchymal Stem Cell Conditioned Medium (MSC-CM) (121) is derived from the supernatant of cultured MSCs and is a mixture of hundreds to thousands of different enzymes, growth factors, cytokines, and proteins that can be concentrated, frozen, or even lyophilized without loss of activity. MSC-CM can be collected in a simple and efficient manner. Cells are inoculated in a culture dish and cultured with serum-containing medium until the density is approximately 70%, after which serum-free medium is added, and the culture continues for 48 h. The supernatant is then collected and filtered with a sterile 0.22 μm filter to remove cell debris and bacterial microorganisms. The final supernatant is referred to as conditioned medium for MSCs and can be stored at −80°C until use or further concentrated and stored at ultra-low temperatures (122).
MSC-CM from different sources has been identified to have diverse and beneficial effects on the receptor, including anti-inflammatory, immunomodulatory, and angiogenic effects (123). Huang et al. used periodontal membrane-derived stem cell-conditioned medium, concentrated 20-fold, to culture chondrocytes, synovial cells, and meniscus cells isolated from IL-1β-treated porcine knees. The results validated increased expression of anti-inflammatory factors as well as decreased expression of inflammatory factors, confirming the anti-inflammatory effect of MSC-CM (124). Gharaei et al. analyzed multiple pro- and anti-angiogenic factors in DPSC-conditioned medium (DPSC-CM), cultured with human umbilical vein endothelial cells (HUVEC). They reported that these factors affected cellular migration and proliferation, stimulated tubulogenesis and promoted angiogenesis such as the number of nodes, meshes, and total tubular length (125). Proteomic analysis has uncovered a total of 1,533 proteins in CM derived from ADSCs, BMMSCs, and DPSCs, which have regenerative potential in areas such as cell migration, angiogenesis, inflammatory response, ossification, and organ survival. Moreover, the expression of multiple cytokines associated with odontoblast differentiation and anti-inflammatory cytokines was significantly higher in DPSC-CM (126). Paschalidis et al. found that DPSC-CM enhanced the viability, migration, and mineralization potential of DPSCs and even counteracted TEGDMA-induced cytotoxicity (127). Through in vivo and in vitro studies, Fujio et al. showed that anti-inflammatory, angiogenesis, and osteogenic-related factors were more highly expressed in DPSC-CM under hypoxia and promoted osteogenesis and accelerated bone healing in mouse tibia (128). DPSC-CM also increases mineralization potential through TGF-β1 expression, thereby triggering new bone formation and improving osteoblast and chondrogenic markers (128). New blood vessels are particularly important in the bone regeneration process, allowing better reconnection of new bone to its own bone. Ishizaka et al. showed that in vivo DPSC-CM exhibited higher immunomodulatory capacity as well as higher angiogenic and anti-apoptotic capacity in vivo compared to BMMSCs-CM (96).
4.2 Extracellular vesicles
All mammalian cell types studied so far, including neuronal cells, endothelial cells, MSCs, and epithelial cells, have been found to release EVs. EVs can be found in all kinds of body fluids like saliva, synovial fluid, urine, and blood. While EVs were previously considered to be cellular waste, recent research has shown that they have a major role in regulating cellular signaling pathways in target cells, including tumor cell growth, cell migration, cell communication, and angiogenesis (129).
EVs are small membranous vesicles that are released into the extracellular matrix by cells via the plasma membrane during the budding process (117). EVs contain a variety of substances, including phosphatidylserine, cytoplasmic proteins, mRNA, miRNA, DNA, and other molecules. Exosomes (Exos) are one group of the smallest and most extensively studied MSC-derived EVs. The genesis of Exos occurs through the endocytic exocytosis pathway, with early endosomes maturing, extending to late endosomes, and budding inward at the late endosomal membrane of multivesicular bodies. After the fusion of these multivesicular bodies with the cell membranes, MSC-derived Exos are released into the extracellular environment. These Exos carry important signaling molecules and exert biological effects in target cells (67, 130).
Various methods are available for the isolation of EVs and Exos, including ultracentrifugation, density gradient centrifugation, exosome kits, ultrafiltration, immunoprecipitation, and acoustic nanofilters (131). Ultracentrifugation is the gold standard method for Exos extraction, which involves multiple rounds of centrifugation at varying speeds to collect Exos from cell culture supernatants (132, 133). Density gradient centrifugation, on the other hand, separates Exos with less contamination but is more complex and time-consuming (134). Recently, commercial kits based on polymer coprecipitation have been developed for Exos extraction, such as ExoQuick, which is simple to use and has a high yield but may contain impurities (135). While each method has its advantages and disadvantages, ultracentrifugation is considered to be the most reliable and efficient technique for Exos extraction. However, this method can be expensive, time-consuming, and require large sample volumes. In contrast, commercial kits are simpler and faster but may have a higher level of impurities. Density gradient centrifugation offers higher purity, but the preparation is more complicated and time-consuming, and Exos cannot be completely separated from proteins. Overall, the choice of isolation method should depend on the specific research needs and resources available.
Exos have emerged as promising candidates for bone defect regeneration (136, 137). Studies have shown that Exos can bind to scaffolds or growth factors and modulate both osteoblast and osteoclast functions (138). Exos have also been found to induce osteogenic differentiation and bone regeneration by increasing osteoblast differentiation-related miRNA expression and inhibiting Axin1-activated Wnt signaling (139). High-throughput miRNA sequencing has revealed that Exos secrete 41 miRNAs that are differentially expressed after osteogenesis induction. These miRNAs have been implicated in bone differentiation and development, including osteoclast differentiation, the PI3K-AKT signaling pathway, the MAPK signaling pathway, and the mTOR signaling pathway. Among these miRNAs, hsa-mir-328-3p and hsa-mir-2110 have been identified as potentially the most important osteogenic regulatory miRNAs in Exos (140). In addition, Wei et al. prepared exosome-treated titanium nanotubes to enhance the osteogenic potential of BMP2 via natural nanocarriers (141).
Moreover, exosomes from human exfoliated deciduous teeth (SHED-Exos) have been shown to stimulate BMMSCs to express more osteogenic-related genes, such as Runx2 and ALP. Surprisingly, they also down-regulate lipopolysaccharide (LPS)-induced expression of inflammation-related factors in BMMSCs.
Although less research has been conducted on the use of dental pulp stem cell-derived exosomes (DPSC-Exos) in bone regeneration, their advantages are evident. DPSC-Exos have been shown to regulate target cells by translocating mRNA, miRNA, proteins, and other molecules to receptor cells. Hu et al. analyzed the microRNA profile of DPSC-Exos and verified that miR-27a-5p of DPSC-Exos promotes odontogenic differentiation of DPSCs by downregulating LTBP1 (one of the suppressor molecules of TGFβ1 signaling) to regulate the TGFβ1/Smads signaling pathway and thus express more osteogenic-related genes (142). DPSC-Exos have also been proven to enhance the proliferation and migration of haplotype homo dental pulp cells and mouse osteoblasts, inhibit the formation of mouse osteoclasts, and effectively reduce bone loss caused by periodontitis (143). Combining DPSC-Exos and DPSCs with β-tricalcium phosphate, hydroxyapatite, or collagen in a rat skull defect model has been found to accelerate bone regeneration and promote more extensive angiogenesis at the defect site. Notably, DPSC-Exos and DPSCs were found to have almost identical effects at the site of bone defects (144). Although DPSC-Exos has been shown to have bone tissue repair effects, the mechanisms behind these effects are not fully understood.
4.3 Cell lysate
Cell lysate, a product of cell lysis, is a promising source of bioactive compounds for regenerative medicine and tissue engineering applications. Although the specific components of the cell lysate remain uncertain, it is known to contain several soluble nutrients, including growth factors, EVs, Exos, and other proteins. To obtain the cell lysate, cells are first cultured and then lysed using trypsin digestion, followed by centrifugation and resuspension in ultra-pure water, and finally subjected to ultrasound or freeze–thaw technology. At this stage, cell releases various proteins and soluble nutrients, including growth factors like EGF and IGF. It can be used in the same way as MSCs, CMs, EVs, Exos, etc. to treat diseases.
While there is limited literature on the utilization of cell lysate for treating bone defects, several studies have demonstrated its anti-inflammatory and regenerative properties in other applications. For example, Jiao et al. were surprised to find that MSC-conditioned medium (MSC-CM) or MSC lysate (MSC Ly) can substantially enhance IL-10 secretion by peripheral blood mononuclear cells (PBMCs) in vitro. Simultaneously, it can significantly increase serum IL-10 levels in two animal models and reproduce the effects of an MSC graft in vivo (145). Ward et al. used the classical hind-paw edema model to simulate temporomandibular joint osteoarthritis, after treatment with human umbilical perivascular mesenchymal cells and their lysates, there were significantly lower concentrations of myeloperoxidase and TNF-α at 48 h. Treated osteoarthritis demonstrated lower concentrations of leukocytes in the synovium compared to controls and histologic evidence in the peri-articular tissue of reduced inflammation (146). Similarly, lysates of olfactory mucosa tissue-derived mesenchymal stem cells (OM-MSCs), when cultured with LPS-stimulated normal human liver cells (LO-2), inhibited the inflammatory process and promoted the proliferation rate of LO-2. Similarly, in a mouse model of LPS-induced acute liver injury, OM-MSCs lysate treatment attenuated inflammation and reduced liver enzyme release, thus alleviating liver injury (147). Khubutiya et al. reported that a mixture of BMMSC-conditioned medium (BMMSC-CM) and MSCs lysate significantly enhanced liver regeneration and reduced injury in a mouse model of acetaminophen-induced acute liver injury (148). Moreover, the administration of filtrated adipose tissue-derived mesenchymal stem cell lysate for three consecutive days mitigated inflammation and inhibited cell apoptosis in a mouse model of acute colitis, leading to improved survival rates, reduced weight loss, and clinical signs (149). Erectile dysfunction (ED) remains a major complication after radical prostatectomy. Albersen et al. found that penile injection of both ADSC and ADSC-derived lysate can improve recovery of erectile function in a rat model of neurogenic erectile dysfunction (150). Our research team has found that the lysates of DPSCs can be used for anti-photoaging treatment, and ZIF-8, as a safe and effective protein delivery system, can improve the skin bioavailability of DPSC lysates, demonstrating significantly enhanced cell uptake and skin retention ability (151). The cell lysate extraction process is rapid, simple, and produces a diverse range of precipitated components with excellent efficacy, thus holding great potential for future applications in regenerative medicine and tissue engineering.
4.4 Advantages and limitations of cell-free therapy
Recent experimental and clinical studies have shown that the use of the MSC secretome is a highly successful therapeutic strategy. MSC-CM and MSC-derived EVs have demonstrated similar therapeutic efficacy to MSC transplantation in bone and cartilage defects (152). The replacement of cell therapy with cell-free therapy eliminates the undesirable side effects associated with live cell transplantation, including immune rejection, emboli formation, tumorigenicity, arrhythmias, calcified ossification, and disease transmission. The secretome can be stored at ultra-low temperatures, such as −80°C, for prolonged periods of time. Cryopreservation or freeze-drying does not compromise the effectiveness of the cell secretome. Whereas cell cryopreservation necessitates the use of potentially toxic cryopreservation agents and harsh cryopreservation temperatures. In emergency situations, the secretome can be rapidly thawed for immediate use. Lastly, following clinical translation, the cell secretome can be standardized in terms of dose and potency, akin to a clinical drug (153).
Despite all the benefits of cell-free therapy, there are some limitations to its application. For instance, there are currently no standardized protocols for producing large quantities of EVs or extracellular vesicles. Furthermore, soluble factors secreted by MSC have not been fully elucidated. And it is unclear whether the use of CM, EVs, or cell lysate is safe for treating various diseases. Most significantly, the legal regulations surrounding cell-free therapy have not yet been fully established, given the rapid evolution of this field. Cell-free therapy remains in its nascent stages, and additional research is required to fully understand its potential as a therapeutic strategy (154).
5 Conclusion and future prospects
Critical size bone defect is a difficult problem to solve in modern medicine. In recent years, tissue engineering and regenerative medicine have shown good results in using DPSCs to treat bone defects. Since the first isolation of DPSCs, DPSCs have been identified to promote proliferation, differentiation, immune regulation, anti-inflammatory, anti-apoptosis, and paracrine signaling. DPSCs demonstrate good therapeutic effects when applied to various systemic diseases. It is noteworthy that they also exhibit strong regenerative capabilities for bone defects.
Cell-free therapy as a new therapeutic approach was discovered in the fields of regenerative medicine and tissue engineering, utilizing. The secretome of DPSCs could effectively avoid the risks in using stem cells (155). In the future, DPSCs secretome or its combination with biomaterials would enhance bone regenerative (156).
In summary, the use of DPSCs as a treatment for bone defects has shown promise but is still in the pre-clinical stages. The emergence of cell-free therapies has opened up possibilities for rapid access to therapeutic solutions for DPSC application.
Author contributions
YeL: Conceptualization, Writing – original draft, Writing – review & editing. WX: Conceptualization, Writing – original draft, Writing – review & editing. JL: Supervision, Writing – review & editing. HF: Supervision, Writing – review & editing. YoL: Writing – review & editing. HZ: Writing – review & editing. LD: Writing – review & editing. DF: Writing – review & editing. CX: Methodology, Supervision, Writing – review & editing. YH: Methodology, Supervision, Writing – review & editing. QY: Conceptualization, Supervision, Methodology, Writing – review & editing.
Funding
The author(s) declare financial support was received for the research, authorship, and/or publication of this article. This work was supported by the State Key Project Ministry of Science and Technology of China (2022YFC2504200 from QY) and Chu tian Researcher Project (X22020024 from YH), the key R&D Program of Hubei Province of China (YFXM2022000264 from QY).
Conflict of interest
The authors declare that the research was conducted in the absence of any commercial or financial relationships that could be construed as a potential conflict of interest.
The reviewer YZ declared a shared affiliation with the author CX to the handling editor at the time of review.
Publisher’s note
All claims expressed in this article are solely those of the authors and do not necessarily represent those of their affiliated organizations, or those of the publisher, the editors and the reviewers. Any product that may be evaluated in this article, or claim that may be made by its manufacturer, is not guaranteed or endorsed by the publisher.
References
1. Ambrosi, TH, Longaker, MT, and Chan, CKF. A revised perspective of skeletal stem cell biology. Front Cell Dev Biol. (2019) 7:189. doi: 10.3389/fcell.2019.00189
2. Galen, T. Usefulness of parts of the body. Clin Orthop Relat Res. (1997) 337:3–12. doi: 10.1097/00003086-199704000-00002
3. Schmidt, AH. Autologous bone graft: is it still the gold standard? Injury. (2021) 52:S18–s22. doi: 10.1016/j.injury.2021.01.043
4. Friedenstein, AJ, Piatetzky, S II, and Petrakova, KV. Osteogenesis in transplants of bone marrow cells. J Embryol Exp Morphol. (1966) 16:381–90.
5. Andrzejewska, A, Lukomska, B, and Janowski, M. Concise review: mesenchymal stem cells: from roots to boost. Stem cells (Dayton, Ohio). (2019) 37:855–64. doi: 10.1002/stem.3016
6. Ogata, K, Moriyama, M, Matsumura-Kawashima, M, Kawado, T, Yano, A, and Nakamura, S. The therapeutic potential of secreted factors from dental pulp stem cells for various diseases. Biomedicine. (2022) 10:1049. doi: 10.3390/biomedicines10051049
7. Li, J, Law, HK, Liu, YL, and Chan, GC. Effect of cisplatin, topotecan, daunorubicin and hydroxyurea on human mesenchymal stem cells. Zhongguo Shi Yan Xue Ye Xue Za Zhi. (2010) 18:991–6.
9. Klar, RM. The induction of bone formation: the translation enigma. Front Bioeng Biotechnol. (2018) 6:74. doi: 10.3389/fbioe.2018.00074
10. Ducy, P, Schinke, T, and Karsenty, G. The Osteoblast: A Sophisticated Fibroblast under Central Surveillance. Science. (2000) 289:1501–4. doi: 10.1126/science.289.5484.1501
11. Lefebvre, V. Roles and regulation of SOX transcription factors in skeletogenesis. Curr Top Dev Biol. (2019) 133:171–93. doi: 10.1016/bs.ctdb.2019.01.007
12. Haseeb, A, Kc, R, Angelozzi, M, de Charleroy, C, Rux, D, Tower, RJ, et al. SOX9 keeps growth plates and articular cartilage healthy by inhibiting chondrocyte dedifferentiation/osteoblastic redifferentiation. Proc Natl Acad Sci U S A. (2021) 118:e 2019152118. doi: 10.1073/pnas.2019152118
13. Tsuji, K, Bandyopadhyay, A, Harfe, BD, Cox, K, Kakar, S, Gerstenfeld, L, et al. BMP2 activity, although dispensable for bone formation, is required for the initiation of fracture healing. Nat Genet. (2006) 38:1424–9. doi: 10.1038/ng1916
14. Kolambkar, YM, Boerckel, JD, Dupont, KM, Bajin, M, Huebsch, N, Mooney, DJ, et al. Spatiotemporal delivery of bone morphogenetic protein enhances functional repair of segmental bone defects. Bone. (2011) 49:485–92. doi: 10.1016/j.bone.2011.05.010
15. Jacome-Galarza, CE, Lee, SK, Lorenzo, JA, and Aguila, HL. Identification, characterization, and isolation of a common progenitor for osteoclasts, macrophages, and dendritic cells from murine bone marrow and periphery. J Bone Miner Res Off J Am Soc Bone Miner Res. (2013) 28:1203–13. doi: 10.1002/jbmr.1822
16. Walsh, MC, Kim, N, Kadono, Y, Rho, J, Lee, SY, Lorenzo, J, et al. Osteoimmunology: interplay between the immune system and bone metabolism. Annu Rev Immunol. (2006) 24:33–63. doi: 10.1146/annurev.immunol.24.021605.090646
17. Chen, X, Wang, Z, Duan, N, Zhu, G, Schwarz, EM, and Xie, C. Osteoblast-osteoclast interactions. Connect Tissue Res. (2018) 59:99–107. doi: 10.1080/03008207.2017.1290085
18. Yu, J, and Canalis, E. Notch and the regulation of osteoclast differentiation and function. Bone. (2020) 138:115474. doi: 10.1016/j.bone.2020.115474
19. Kim, JM, Lin, C, Stavre, Z, Greenblatt, MB, and Shim, JH. Osteoblast-osteoclast communication and bone homeostasis. Cell. (2020) 9. doi: 10.3390/cells9092073
20. Udagawa, N, Koide, M, Nakamura, M, Nakamichi, Y, Yamashita, T, Uehara, S, et al. Osteoclast differentiation by RANKL and OPG signaling pathways. J Bone Miner Metab. (2021) 39:19–26. doi: 10.1007/s00774-020-01162-6
21. Negishi-Koga, T, and Takayanagi, H. Ca2+-NFATc1 signaling is an essential axis of osteoclast differentiation. Immunol Rev. (2009) 231:241–56. doi: 10.1111/j.1600-065X.2009.00821.x
22. Rundle, CH, Wang, H, Yu, H, Chadwick, RB, Davis, EI, Wergedal, JE, et al. Microarray analysis of gene expression during the inflammation and endochondral bone formation stages of rat femur fracture repair. Bone. (2006) 38:521–9. doi: 10.1016/j.bone.2005.09.015
23. Salhotra, A, Shah, HN, Levi, B, and Longaker, MT. Mechanisms of bone development and repair. Nat Rev Mol Cell Biol. (2020) 21:696–711. doi: 10.1038/s41580-020-00279-w
24. Majidinia, M, Sadeghpour, A, and Yousefi, B. The roles of signaling pathways in bone repair and regeneration. J Cell Physiol. (2018) 233:2937–48. doi: 10.1002/jcp.26042
25. Joeng, KS, and Long, F. The Gli2 transcriptional activator is a crucial effector for Ihh signaling in osteoblast development and cartilage vascularization. Development. (2009) 136:4177–85. doi: 10.1242/dev.041624
26. Ohba, S. Hedgehog signaling in skeletal development: roles of Indian hedgehog and the mode of its action. Int J Mol Sci. (2020) 21:6665. doi: 10.3390/ijms21186665
27. Park, HL, Bai, C, Platt, KA, Matise, MP, Beeghly, A, Hui, CC, et al. Mouse Gli1 mutants are viable but have defects in SHH signaling in combination with a Gli2 mutation. Development. (2000) 127:1593–605. doi: 10.1242/dev.127.8.1593
28. Hojo, H, Ohba, S, Yano, F, Saito, T, Ikeda, T, Nakajima, K, et al. Gli1 protein participates in hedgehog-mediated specification of osteoblast lineage during endochondral ossification. J Biol Chem. (2012) 287:17860–9. doi: 10.1074/jbc.M112.347716
29. Zanotti, S, and Canalis, E. Notch signaling in skeletal health and disease. Eur J Endocrinol. (2013) 168:R95–R103. doi: 10.1530/EJE-13-0115
30. Fukushima, H, Nakao, A, Okamoto, F, Shin, M, Kajiya, H, Sakano, S, et al. The association of Notch2 and NF-kappaB accelerates RANKL-induced osteoclastogenesis. Mol Cell Biol. (2008) 28:6402–12. doi: 10.1128/MCB.00299-08
31. Ganguly, SS, Hostetter, G, Tang, L, Frank, SB, Saboda, K, Mehra, R, et al. Notch3 promotes prostate cancer-induced bone lesion development via MMP-3. Oncogene. (2020) 39:204–18. doi: 10.1038/s41388-019-0977-1
32. Bagheri, L, Pellati, A, Rizzo, P, Aquila, G, Massari, L, De Mattei, M, et al. Notch pathway is active during osteogenic differentiation of human bone marrow mesenchymal stem cells induced by pulsed electromagnetic fields. J Tissue Eng Regen Med. (2018) 12:304–15. doi: 10.1002/term.2455
33. Ballhause, TM, Jiang, S, Baranowsky, A, Brandt, S, Mertens, PR, Frosch, KH, et al. Relevance of notch signaling for bone metabolism and regeneration. Int J Mol Sci. (2021) 22:e1325. doi: 10.3390/ijms22031325
34. Xu, C, Dinh, VV, Kruse, K, Jeong, HW, Watson, EC, Adams, S, et al. Induction of osteogenesis by bone-targeted notch activation. Elife. (2022) 11:e60183. doi: 10.7554/eLife.60183
35. Hilton, MJ, Tu, X, Wu, X, Bai, S, Zhao, H, Kobayashi, T, et al. Notch signaling maintains bone marrow mesenchymal progenitors by suppressing osteoblast differentiation. Nat Med. (2008) 14:306–14. doi: 10.1038/nm1716
36. Zanotti, S, Smerdel-Ramoya, A, and Canalis, E. HES1 (hairy and enhancer of split 1) is a determinant of bone mass. J Biol Chem. (2011) 286:2648–57. doi: 10.1074/jbc.M110.183038
37. Shen, Q, and Christakos, S. The vitamin D receptor, Runx2, and the notch signaling pathway cooperate in the transcriptional regulation of osteopontin. J Biol Chem. (2005) 280:40589–98. doi: 10.1074/jbc.M504166200
38. Huybrechts, Y, Mortier, G, Boudin, E, and Van Hul, W. WNT signaling and bone: lessons from skeletal Dysplasias and disorders. Front Endocrinol. (2020) 11:165.
39. Li, F, Chong, ZZ, and Maiese, K. Vital elements of the Wnt-frizzled signaling pathway in the nervous system. Curr Neurovasc Res. (2005) 2:331–40. doi: 10.2174/156720205774322557
40. Oichi, T, Otsuru, S, Usami, Y, Enomoto-Iwamoto, M, and Iwamoto, M. Wnt signaling in chondroprogenitors during long bone development and growth. Bone. (2020) 137:115368. doi: 10.1016/j.bone.2020.115368
41. Maeda, K, Kobayashi, Y, Udagawa, N, Uehara, S, Ishihara, A, Mizoguchi, T, et al. Wnt5a-Ror2 signaling between osteoblast-lineage cells and osteoclast precursors enhances osteoclastogenesis. Nat Med. (2012) 18:405–12. doi: 10.1038/nm.2653
42. Thrasivoulou, C, Millar, M, and Ahmed, A. Activation of intracellular calcium by multiple Wnt ligands and translocation of β-catenin into the nucleus: a convergent model of Wnt/Ca2+ and Wnt/β-catenin pathways. J Biol Chem. (2013) 288:35651–9. doi: 10.1074/jbc.M112.437913
43. Sheldahl, LC, Slusarski, DC, Pandur, P, Miller, JR, Kühl, M, and Moon, RT. Dishevelled activates Ca2+ flux, PKC, and CamKII in vertebrate embryos. J Cell Biol. (2003) 161:769–77. doi: 10.1083/jcb.200211094
44. Li, X, and Cao, X. BMP signaling and skeletogenesis. Ann N Y Acad Sci. (2006) 1068:26–40. doi: 10.1196/annals.1346.006
45. Miyazawa, K, and Miyazono, K. Regulation of TGF-β family signaling by inhibitory Smads. Cold Spring Harb Perspect Biol. (2017) 9:ea022095. doi: 10.1101/cshperspect.a022095
46. Derynck, R, and Budi, EH. Specificity, versatility, and control of TGF-β family signaling. Sci Signal. (2019) 12:eaav5183. doi: 10.1126/scisignal.aav5183
47. Zou, ML, Chen, ZH, Teng, YY, Liu, SY, Jia, Y, Zhang, KW, et al. The Smad dependent TGF-β and BMP signaling pathway in bone remodeling and therapies. Front Mol Biosci. (2021) 8:593310. doi: 10.3389/fmolb.2021.593310
48. Salazar, VS, Gamer, LW, and Rosen, V. BMP signalling in skeletal development, disease and repair. Nat Rev Endocrinol. (2016) 12:203–21. doi: 10.1038/nrendo.2016.12
49. Luo, K. Signaling cross talk between TGF-β/Smad and other signaling pathways. Cold Spring Harb Perspect Biol. (2017) 9:e022137. doi: 10.1101/cshperspect.a022137
50. Wang, G, Wang, F, Zhang, L, Yan, C, and Zhang, Y. miR-133a silencing rescues glucocorticoid-induced bone loss by regulating the MAPK/ERK signaling pathway. Stem Cell Res Ther. (2021) 12:215. doi: 10.1186/s13287-021-02278-w
51. Jo, YJ, Lee, HI, Kim, N, Hwang, D, Lee, J, Lee, GR, et al. Cinchonine inhibits osteoclast differentiation by regulating TAK1 and AKT, and promotes osteogenesis. J Cell Physiol. (2021) 236:1854–65. doi: 10.1002/jcp.29968
52. Gunnell, LM, Jonason, JH, Loiselle, AE, Kohn, A, Schwarz, EM, Hilton, MJ, et al. TAK1 regulates cartilage and joint development via the MAPK and BMP signaling pathways. J Bone and Mineral Res: Official J American Society for Bone and Mineral Res. (2010) 25:1784–97. doi: 10.1002/jbmr.79
53. Tang, L, Wu, M, Lu, S, Zhang, H, Shen, Y, Shen, C, et al. Fgf9 negatively regulates bone mass by inhibiting osteogenesis and promoting Osteoclastogenesis via MAPK and PI3K/AKT signaling. J Bone and Mineral Res: Official J American Society for Bone and Mineral Res. (2021) 36:779–91. doi: 10.1002/jbmr.4230
54. Chen, M, Song, K, Rao, N, Huang, M, Huang, Z, and Cao, Y. Roles of exogenously regulated bFGF expression in angiogenesis and bone regeneration in rat calvarial defects. Int J Mol Med. (2011) 27:545–53. doi: 10.3892/ijmm.2011.619
55. Nakajima, F, Ogasawara, A, Goto, K, Moriya, H, Ninomiya, Y, Einhorn, TA, et al. Spatial and temporal gene expression in chondrogenesis during fracture healing and the effects of basic fibroblast growth factor. J Orthop Res. (2001) 19:935–44. doi: 10.1016/S0736-0266(01)00024-9
56. Zhao, B, Peng, Q, Poon, EHL, Chen, F, Zhou, R, Shang, G, et al. Leonurine promotes the osteoblast differentiation of rat BMSCs by activation of autophagy via the PI3K/Akt/mTOR pathway. Front Bioeng Biotechnol. (2021) 9:615191. doi: 10.3389/fbioe.2021.615191
57. Peng, XD, Xu, PZ, Chen, ML, Hahn-Windgassen, A, Skeen, J, Jacobs, J, et al. Dwarfism, impaired skin development, skeletal muscle atrophy, delayed bone development, and impeded adipogenesis in mice lacking Akt1 and Akt2. Genes Dev. (2003) 17:1352–65. doi: 10.1101/gad.1089403
58. Patel, KD, and Nguyen, DX. Condensing and constraining WNT by TGF-β. Nat Cell Biol. (2021) 23:213–4. doi: 10.1038/s41556-021-00649-2
59. Guerrero, F, Herencia, C, Almadén, Y, Martínez-Moreno, JM, Montes de Oca, A, Rodriguez-Ortiz, ME, et al. TGF-β prevents phosphate-induced osteogenesis through inhibition of BMP and Wnt/β-catenin pathways. PloS One. (2014) 9:e89179. doi: 10.1371/journal.pone.0089179
60. Fuentealba, LC, Eivers, E, Ikeda, A, Hurtado, C, Kuroda, H, Pera, EM, et al. Integrating patterning signals: Wnt/GSK3 regulates the duration of the BMP/Smad1 signal. Cell. (2007) 131:980–93. doi: 10.1016/j.cell.2007.09.027
61. Millet, C, Yamashita, M, Heller, M, Yu, LR, Veenstra, TD, and Zhang, YE. A negative feedback control of transforming growth factor-beta signaling by glycogen synthase kinase 3-mediated Smad3 linker phosphorylation at Ser-204. J Biol Chem. (2009) 284:19808–16. doi: 10.1074/jbc.M109.016667
62. Guo, X, Ramirez, A, Waddell, DS, Li, Z, Liu, X, and Wang, XF. Axin and GSK3- control Smad3 protein stability and modulate TGF- signaling. Genes Dev. (2008) 22:106–20. doi: 10.1101/gad.1590908
63. Blokzijl, A, Dahlqvist, C, Reissmann, E, Falk, A, Moliner, A, Lendahl, U, et al. Cross-talk between the notch and TGF-beta signaling pathways mediated by interaction of the notch intracellular domain with Smad3. J Cell Biol. (2003) 163:723–8. doi: 10.1083/jcb.200305112
64. Brown, C, McKee, C, Bakshi, S, Walker, K, Hakman, E, Halassy, S, et al. Mesenchymal stem cells: cell therapy and regeneration potential. J Tissue Eng Regen Med. (2019) 13:1738–55. doi: 10.1002/term.2914
65. Zayed, M, and Iohara, K. Immunomodulation and regeneration properties of dental pulp stem cells: a potential therapy to treat coronavirus disease 2019. Cell Transplant. (2020) 29:963689720952089. doi: 10.1177/0963689720952089
66. Zhang, Y, Xie, Y, Hao, Z, Zhou, P, Wang, P, Fang, S, et al. Umbilical mesenchymal stem cell-derived exosome-encapsulated hydrogels accelerate bone repair by enhancing angiogenesis. ACS Appl Mater Interfaces. (2021) 13:18472–87. doi: 10.1021/acsami.0c22671
67. Bar, JK, Lis-Nawara, A, and Grelewski, PG. Dental pulp stem cell-derived Secretome and its regenerative potential. Int J Mol Sci. (2021) 22:12018. doi: 10.3390/ijms222112018
68. Aithal, AP, Bairy, LK, and Seetharam, RN. Safety and therapeutic potential of human bone marrow-derived mesenchymal stromal cells in regenerative medicine. Stem cell investigation. (2021) 8:10. doi: 10.21037/sci-2020-036
69. Shi, X, Mao, J, and Liu, Y. Pulp stem cells derived from human permanent and deciduous teeth: biological characteristics and therapeutic applications. Stem Cells Transl Med. (2020) 9:445–64. doi: 10.1002/sctm.19-0398
70. Luo, L, He, Y, Wang, X, Key, B, Lee, BH, Li, H, et al. Potential roles of dental pulp stem cells in neural regeneration and repair. Stem Cells Int. (2018) 2018:1731289. doi: 10.1155/2018/1731289
71. Wu, T, Xu, W, Chen, H, Li, S, Dou, R, Shen, H, et al. Comparison of the differentiation of dental pulp stem cells and periodontal ligament stem cells into neuron-like cells and their effects on focal cerebral ischemia. Acta Biochim Biophys Sin. (2020) 52:1016–29. doi: 10.1093/abbs/gmaa082
72. Lyu, J, Hashimoto, Y, Honda, Y, and Matsumoto, N. Comparison of osteogenic potentials of dental pulp and bone marrow mesenchymal stem cells using the new cell transplantation platform, CellSaic, in a rat congenital cleft-jaw model. Int J Mol Sci. (2021) 22:9478. doi: 10.3390/ijms22179533
73. Zhou, Y, Xu, T, Wang, C, Han, P, and Ivanovski, S. Clinical usage of dental stem cells and their derived extracellular vesicles. Prog Mol Biol Transl Sci. (2023) 199:297–326. doi: 10.1016/bs.pmbts.2023.03.005
74. Meesuk, L, Suwanprateeb, J, Thammarakcharoen, F, Tantrawatpan, C, Kheolamai, P, Palang, I, et al. Osteogenic differentiation and proliferation potentials of human bone marrow and umbilical cord-derived mesenchymal stem cells on the 3D-printed hydroxyapatite scaffolds. Sci Rep. (2022) 12:19509. doi: 10.1038/s41598-022-24160-2
75. Vater, C, Männel, C, Bolte, J, Tian, X, Goodman, SB, and Zwingenberger, S. Effectiveness of dental pulp-derived stem cells and bone Marrowderived mesenchymal stromal cells implanted into a murine critical bone defect. Curr Stem Cell Res Ther. (2022) 17:480–91. doi: 10.2174/1574888X17666220215100732
76. Siegel, G, Kluba, T, Hermanutz-Klein, U, Bieback, K, Northoff, H, and Schäfer, R. Phenotype, donor age and gender affect function of human bone marrow-derived mesenchymal stromal cells. BMC Med. (2013) 11:146. doi: 10.1186/1741-7015-11-146
77. Riekstina, U, Cakstina, I, Parfejevs, V, Hoogduijn, M, Jankovskis, G, Muiznieks, I, et al. Embryonic stem cell marker expression pattern in human mesenchymal stem cells derived from bone marrow, adipose tissue, heart and dermis. Stem Cell Rev Rep. (2009) 5:378–86. doi: 10.1007/s12015-009-9094-9
78. Weiss, ML, Anderson, C, Medicetty, S, Seshareddy, KB, Weiss, RJ, VanderWerff, I, et al. Immune properties of human umbilical cord Wharton's jelly-derived cells. Stem cells (Dayton, Ohio). (2008) 26:2865–74. doi: 10.1634/stemcells.2007-1028
79. King, NM, and Perrin, J. Ethical issues in stem cell research and therapy. Stem Cell Res Ther. (2014) 5:85. doi: 10.1186/scrt474
80. Watanabe, Y, Tsuchiya, A, and Terai, S. The development of mesenchymal stem cell therapy in the present, and the perspective of cell-free therapy in the future. Clin Mol Hepatol. (2021) 27:70–80. doi: 10.3350/cmh.2020.0194
81. Műzes, G, and Sipos, F. Mesenchymal stem cell-derived Secretome: a potential therapeutic option for autoimmune and immune-mediated inflammatory diseases. Cell. (2022) 11:2300. doi: 10.3390/cells11152300
82. Najar, M, Raicevic, G, Fayyad-Kazan, H, Bron, D, Toungouz, M, and Lagneaux, L. Mesenchymal stromal cells and immunomodulation: a gathering of regulatory immune cells. Cytotherapy. (2016) 18:160–71. doi: 10.1016/j.jcyt.2015.10.011
83. Yamada, Y, Nakamura-Yamada, S, Kusano, K, and Baba, S. Clinical potential and current Progress of dental pulp stem cells for various systemic diseases in regenerative medicine: a concise review. Int J Mol Sci. (2019) 20:1132. doi: 10.3390/ijms20051132
84. Gaus, S, Li, H, Li, S, Wang, Q, Kottek, T, Hahnel, S, et al. Shared genetic and epigenetic mechanisms between the osteogenic differentiation of dental pulp stem cells and bone marrow stem cells. Biomed Res Int. (2021) 2021:6697810. doi: 10.1155/2021/6697810
85. Jin, Q, Yuan, K, Lin, W, Niu, C, Ma, R, and Huang, Z. Comparative characterization of mesenchymal stem cells from human dental pulp and adipose tissue for bone regeneration potential. Artificial cells, nanomedicine, and biotechnology. (2019) 47:1577–84. doi: 10.1080/21691401.2019.1594861
86. Pierdomenico, L, Bonsi, L, Calvitti, M, Rondelli, D, Arpinati, M, Chirumbolo, G, et al. Multipotent mesenchymal stem cells with immunosuppressive activity can be easily isolated from dental pulp. Transplantation. (2005) 80:836–42. doi: 10.1097/01.tp.0000173794.72151.88
87. Stanko, P, Kaiserova, K, Altanerova, V, and Altaner, C. Comparison of human mesenchymal stem cells derived from dental pulp, bone marrow, adipose tissue, and umbilical cord tissue by gene expression. Biomedical papers of the Medical Faculty of the University Palacky, Olomouc, Czechoslovakia. (2014) 158:373–7. doi: 10.5507/bp.2013.078
88. Davies, OG, Cooper, PR, Shelton, RM, Smith, AJ, and Scheven, BA. A comparison of the in vitro mineralisation and dentinogenic potential of mesenchymal stem cells derived from adipose tissue, bone marrow and dental pulp. J Bone Miner Metab. (2015) 33:371–82. doi: 10.1007/s00774-014-0601-y
89. Isobe, Y, Koyama, N, Nakao, K, Osawa, K, Ikeno, M, Yamanaka, S, et al. Comparison of human mesenchymal stem cells derived from bone marrow, synovial fluid, adult dental pulp, and exfoliated deciduous tooth pulp. Int J Oral Maxillofac Surg. (2016) 45:124–31. doi: 10.1016/j.ijom.2015.06.022
90. Aghajani, F, Hooshmand, T, Khanmohammadi, M, Khanjani, S, Edalatkhah, H, Zarnani, AH, et al. Comparative Immunophenotypic characteristics, proliferative features, and osteogenic differentiation of stem cells isolated from human permanent and deciduous teeth with bone marrow. Mol Biotechnol. (2016) 58:415–27. doi: 10.1007/s12033-016-9941-2
91. Zhang, Y, Xing, Y, Jia, L, Ji, Y, Zhao, B, Wen, Y, et al. An in vitro comparative study of multisource derived human mesenchymal stem cells for bone tissue engineering. Stem Cells Dev. (2018) 27:1634–45. doi: 10.1089/scd.2018.0119
92. Kunimatsu, R, Nakajima, K, Awada, T, Tsuka, Y, Abe, T, Ando, K, et al. Comparative characterization of stem cells from human exfoliated deciduous teeth, dental pulp, and bone marrow-derived mesenchymal stem cells. Biochem Biophys Res Commun. (2018) 501:193–8. doi: 10.1016/j.bbrc.2018.04.213
93. Kumar, A, Kumar, V, Rattan, V, Jha, V, and Bhattacharyya, S. Secretome proteins regulate comparative osteogenic and adipogenic potential in bone marrow and dental stem cells. Biochimie. (2018) 155:129–39. doi: 10.1016/j.biochi.2018.10.014
94. Lee, YC, Chan, YH, Hsieh, SC, Lew, WZ, and Feng, SW. Comparing the osteogenic potentials and bone regeneration capacities of bone marrow and dental pulp mesenchymal stem cells in a rabbit Calvarial bone defect model. Int J Mol Sci. (2019) 20:5015. doi: 10.3390/ijms20205015
95. Mohanram, Y, Zhang, J, Tsiridis, E, and Yang, XB. Comparing bone tissue engineering efficacy of HDPSCs, HBMSCs on 3D biomimetic ABM-P-15 scaffolds in vitro and in vivo. Cytotechnology. (2020) 72:715–30. doi: 10.1007/s10616-020-00414-7
96. Ishizaka, R, Hayashi, Y, Iohara, K, Sugiyama, M, Murakami, M, Yamamoto, T, et al. Stimulation of angiogenesis, neurogenesis and regeneration by side population cells from dental pulp. Biomaterials. (2013) 34:1888–97. doi: 10.1016/j.biomaterials.2012.10.045
97. Mead, B, Logan, A, Berry, M, Leadbeater, W, and Scheven, BA. Paracrine-mediated neuroprotection and neuritogenesis of axotomised retinal ganglion cells by human dental pulp stem cells: comparison with human bone marrow and adipose-derived mesenchymal stem cells. PloS One. (2014) 9:e109305. doi: 10.1371/journal.pone.0109305
98. Hayashi, Y, Murakami, M, Kawamura, R, Ishizaka, R, Fukuta, O, and Nakashima, M. CXCL14 and MCP1 are potent trophic factors associated with cell migration and angiogenesis leading to higher regenerative potential of dental pulp side population cells. Stem Cell Res Ther. (2015) 6:111. doi: 10.1186/s13287-015-0088-z
99. Ji, L, Bao, L, Gu, Z, Zhou, Q, Liang, Y, Zheng, Y, et al. Comparison of immunomodulatory properties of exosomes derived from bone marrow mesenchymal stem cells and dental pulp stem cells. Immunol Res. (2019) 67:432–42. doi: 10.1007/s12026-019-09088-6
100. Abbas, OL, Özatik, O, Gönen, ZB, Öğüt, S, Özatik, FY, Salkın, H, et al. Comparative analysis of mesenchymal stem cells from bone marrow, adipose tissue, and dental pulp as sources of cell therapy for zone of stasis burns. J Investigative Surg: Official J Academy of Surgl Res. (2019) 32:477–90. doi: 10.1080/08941939.2018.1433254
101. De la Rosa-Ruiz, MDP, Álvarez-Pérez, MA, Cortés-Morales, VA, Monroy-García, A, Mayani, H, Fragoso-González, G, et al. Mesenchymal stem/stromal cells derived from dental tissues: a comparative in vitro evaluation of their Immunoregulatory properties against T cells. Cell. (2019) 8:1491. doi: 10.3390/cells8121491
102. Anderson, S, Prateeksha, P, and Das, H. Dental pulp-derived stem cells reduce inflammation, accelerate wound healing and mediate M2 polarization of myeloid cells. Biomedicine. (2022) 10:999. doi: 10.3390/biomedicines10081999
103. Cui, SJ, Zhang, T, Fu, Y, Liu, Y, Gan, YH, Zhou, YH, et al. DPSCs attenuate experimental progressive TMJ arthritis by inhibiting the STAT1 pathway. J Dent Res. (2020) 99:446–55. doi: 10.1177/0022034520901710
104. Rolph, DN, Deb, M, Kanji, S, Greene, CJ, Das, M, Joseph, M, et al. Ferutinin directs dental pulp-derived stem cells towards the osteogenic lineage by epigenetically regulating canonical Wnt signaling. Biochim Biophys Acta Mol basis Dis. (2020) 1866:165314. doi: 10.1016/j.bbadis.2018.10.032
105. D'Alimonte, I, Nargi, E, Lannutti, A, Marchisio, M, Pierdomenico, L, Costanzo, G, et al. Adenosine A1 receptor stimulation enhances osteogenic differentiation of human dental pulp-derived mesenchymal stem cells via WNT signaling. Stem Cell Res. (2013) 11:611–24. doi: 10.1016/j.scr.2013.04.002
106. Xie, H, Lin, Y, and Fang, F. Glycogen synthase kinase-3β inhibitor promotes the migration and osteogenic differentiation of rat dental pulp stem cells via the β-catenin/PI3K/Akt signaling pathway. J Dental Sci. (2022) 17:802–10. doi: 10.1016/j.jds.2021.09.035
107. Kanji, S, Sarkar, R, Pramanik, A, Kshirsagar, S, Greene, CJ, and Das, H. Dental pulp-derived stem cells inhibit osteoclast differentiation by secreting osteoprotegerin and deactivating AKT signalling in myeloid cells. J Cell Mol Med. (2021) 25:2390–403. doi: 10.1111/jcmm.16071
108. Chan, Y-H, Ho, K-N, Lee, Y-C, Chou, M-J, Lew, W-Z, Huang, H-M, et al. Melatonin enhances osteogenic differentiation of dental pulp mesenchymal stem cells by regulating MAPK pathways and promotes the efficiency of bone regeneration in calvarial bone defects. Stem Cell Res Ther. (2022) 13:73. doi: 10.1186/s13287-022-02744-z
109. Stovall, KE, Tran, TDN, Suantawee, T, Yao, S, Gimble, JM, Adisakwattana, S, et al. Adenosine triphosphate enhances osteoblast differentiation of rat dental pulp stem cells via the PLC-IP(3) pathway and intracellular ca (2+) signaling. J Cell Physiol. (2020) 235:1723–32. doi: 10.1002/jcp.29091
110. Li, S, Hu, J, Zhang, G, Qi, W, Zhang, P, Li, P, et al. Extracellular Ca2+ promotes odontoblastic differentiation of dental pulp stem cells via BMP2-mediated Smad1/5/8 and Erk1/2 pathways. J Cell Physiol. (2015) 230:2164–73. doi: 10.1002/jcp.24945
111. Murakami, M, Hayashi, Y, Iohara, K, Osako, Y, Hirose, Y, and Nakashima, M. Trophic effects and regenerative potential of mobilized mesenchymal stem cells from bone marrow and adipose tissue as alternative cell sources for pulp/dentin regeneration. Cell Transplant. (2015) 24:1753–65. doi: 10.3727/096368914X683502
112. Dissanayaka, WL, Zhan, X, Zhang, C, Hargreaves, KM, Jin, L, and Tong, EH. Coculture of dental pulp stem cells with endothelial cells enhances osteo−/odontogenic and angiogenic potential in vitro. J Endod. (2012) 38:454–63. doi: 10.1016/j.joen.2011.12.024
113. Lee, RH, Pulin, AA, Seo, MJ, Kota, DJ, Ylostalo, J, Larson, BL, et al. Intravenous hMSCs improve myocardial infarction in mice because cells embolized in lung are activated to secrete the anti-inflammatory protein TSG-6. Cell Stem Cell. (2009) 5:54–63. doi: 10.1016/j.stem.2009.05.003
114. Miceli, V, Bulati, M, Iannolo, G, Zito, G, Gallo, A, and Conaldi, PG. Therapeutic properties of mesenchymal stromal/stem cells: the need of cell priming for cell-free therapies in regenerative medicine. Int J Mol Sci. (2021) 22:763. doi: 10.3390/ijms22020763
115. El Moshy, S, Radwan, IA, Rady, D, Abbass, MMS, El-Rashidy, AA, Sadek, KM, et al. Dental stem cell-derived Secretome/conditioned medium: the future for regenerative therapeutic applications. Stem Cells Int. (2020) 2020:7593402. doi: 10.1155/2020/7593402
116. Praveen Kumar, L, Kandoi, S, Misra, R, Vijayalakshmi, S, Rajagopal, K, and Verma, RS. The mesenchymal stem cell secretome: a new paradigm towards cell-free therapeutic mode in regenerative medicine. Cytokine Growth Factor Rev. (2019) 46:1–9. doi: 10.1016/j.cytogfr.2019.04.002
117. Abels, ER, and Breakefield, XO. Introduction to extracellular vesicles: biogenesis, RNA cargo selection, content, release, and uptake. Cell Mol Neurobiol. (2016) 36:301–12. doi: 10.1007/s10571-016-0366-z
118. Raposo, G, and Stoorvogel, W. Extracellular vesicles: exosomes, microvesicles, and friends. J Cell Biol. (2013) 200:373–83. doi: 10.1083/jcb.201211138
119. Keshtkar, S, Azarpira, N, and Ghahremani, MH. Mesenchymal stem cell-derived extracellular vesicles: novel frontiers in regenerative medicine. Stem Cell Res Ther. (2018) 9:63. doi: 10.1186/s13287-018-0791-7
120. Sultan, N, Amin, LE, Zaher, AR, Scheven, BA, and Grawish, ME. Dental pulp stem cells: novel cell-based and cell-free therapy for peripheral nerve repair. World J Stomatol. (2019) 7:1–19. doi: 10.5321/wjs.v7.i1.1
121. Pawitan, JA. Prospect of stem cell conditioned medium in regenerative medicine. Biomed Res Int. (2014) 2014:965849. doi: 10.1155/2014/965849
122. Bogatcheva, NV, and Coleman, ME. Conditioned medium of mesenchymal stromal cells: a new class of therapeutics. Biochemistry Biokhimiia. (2019) 84:1375–89. doi: 10.1134/S0006297919110129
123. Lin, H, Chen, H, Zhao, X, Chen, Z, Zhang, P, Tian, Y, et al. Advances in mesenchymal stem cell conditioned medium-mediated periodontal tissue regeneration. J Transl Med. (2021) 19:456. doi: 10.1186/s12967-021-03125-5
124. Huang, CY, Vesvoranan, O, Yin, X, Montoya, A, Londono, V, Sawatari, Y, et al. Anti-inflammatory effects of conditioned medium of periodontal ligament-derived stem cells on chondrocytes, Synoviocytes, and Meniscus cells. Stem Cells Dev. (2021) 30:537–47. doi: 10.1089/scd.2021.0010
125. Gharaei, MA, Xue, Y, Mustafa, K, Lie, SA, and Fristad, I. Human dental pulp stromal cell conditioned medium alters endothelial cell behavior. Stem Cell Res Ther. (2018) 9:69. doi: 10.1186/s13287-018-0815-3
126. Koh, B, Ab Rahman, FH, Matlan, NA, Rajan, M, Musta’ain, AY, Mohd Jeffry Lee, MR, et al. Potential role of dental pulp stem cells conditioned medium for odontoblastic differentiation. Biol Res. (2022) 55:11. doi: 10.1186/s40659-022-00380-8
127. Paschalidis, T, Bakopoulou, A, Papa, P, Leyhausen, G, Geurtsen, W, and Koidis, P. Dental pulp stem cells' secretome enhances pulp repair processes and compensates TEGDMA-induced cytotoxicity. Dent Mater. (2014) 30:e405–18. doi: 10.1016/j.dental.2014.08.377
128. Fujio, M, Xing, Z, Sharabi, N, Xue, Y, Yamamoto, A, Hibi, H, et al. Conditioned media from hypoxic-cultured human dental pulp cells promotes bone healing during distraction osteogenesis. J Tissue Eng Regen Med. (2017) 11:2116–26. doi: 10.1002/term.2109
129. Lee, JY, and Kim, HS. Extracellular vesicles in regenerative medicine: potentials and challenges. Tissue engineering and regenerative medicine. (2021) 18:479–84. doi: 10.1007/s13770-021-00365-w
130. Harrell, CR, Fellabaum, C, Jovicic, N, Djonov, V, Arsenijevic, N, and Volarevic, V. Molecular mechanisms responsible for therapeutic potential of mesenchymal stem cell-derived Secretome. Cell. (2019) 8:467. doi: 10.3390/cells8050467
131. Doyle, LM, and Wang, MZ. Overview of extracellular vesicles, their origin, composition, purpose, and methods for exosome isolation and analysis. Cell. (2019) 8:727. doi: 10.3390/cells8070727
132. Huang, LH, Rau, CS, Wu, SC, Wu, YC, Wu, CJ, Tsai, CW, et al. Identification and characterization of hADSC-derived exosome proteins from different isolation methods. J Cell Mol Med. (2021) 25:7436–50. doi: 10.1111/jcmm.16775
133. Tauro, BJ, Greening, DW, Mathias, RA, Ji, H, Mathivanan, S, Scott, AM, et al. Comparison of ultracentrifugation, density gradient separation, and immunoaffinity capture methods for isolating human colon cancer cell line LIM1863-derived exosomes. Methods (San Diego, Calif). (2012) 56:293–304. doi: 10.1016/j.ymeth.2012.01.002
134. Zhang, Z, Wang, C, Li, T, Liu, Z, and Li, L. Comparison of ultracentrifugation and density gradient separation methods for isolating Tca8113 human tongue cancer cell line-derived exosomes. Oncol Lett. (2014) 8:1701–6. doi: 10.3892/ol.2014.2373
135. Zeringer, E, Barta, T, Li, M, and Vlassov, AV. Strategies for isolation of exosomes. Cold Spring Harb Protoc. (2015) 2015:319–23. doi: 10.1101/pdb.top074476
136. Gao, Y, Yuan, Z, Yuan, X, Wan, Z, Yu, Y, Zhan, Q, et al. Bioinspired porous microspheres for sustained hypoxic exosomes release and vascularized bone regeneration. Bioactive materials. (2022) 14:377–88. doi: 10.1016/j.bioactmat.2022.01.041
137. Wei, F, Li, Z, Crawford, R, Xiao, Y, and Zhou, Y. Immunoregulatory role of exosomes derived from differentiating mesenchymal stromal cells on inflammation and osteogenesis. J Tissue Eng Regen Med. (2019) 13:1978–91. doi: 10.1002/term.2947
138. Zhou, Y, and Xiao, Y. The development of extracellular vesicle-integrated biomaterials for bone regeneration. Adv Exp Med Biol. (2020) 1250:97–108. doi: 10.1007/978-981-15-3262-7_7
139. Mi, B, Chen, L, Xiong, Y, Yang, Y, Panayi, AC, Xue, H, et al. Osteoblast/osteoclast and immune cocktail therapy of an exosome/drug delivery multifunctional hydrogel accelerates fracture repair. ACS Nano. (2022) 16:771–82. doi: 10.1021/acsnano.1c08284
140. Yahao, G, and Xinjia, W. The role and mechanism of exosomes from umbilical cord mesenchymal stem cells in inducing osteogenesis and preventing osteoporosis. Cell Transplant. (2021) 30:9636897211057465. doi: 10.1177/09636897211057465
141. Wei, F, Li, M, Crawford, R, Zhou, Y, and Xiao, Y. Exosome-integrated titanium oxide nanotubes for targeted bone regeneration. Acta Biomater. (2019) 86:480–92. doi: 10.1016/j.actbio.2019.01.006
142. Hu, X, Zhong, Y, Kong, Y, Chen, Y, Feng, J, and Zheng, J. Lineage-specific exosomes promote the odontogenic differentiation of human dental pulp stem cells (DPSCs) through TGFβ1/smads signaling pathway via transfer of microRNAs. Stem Cell Res Ther. (2019) 10:170. doi: 10.1186/s13287-019-1278-x
143. Shimizu, Y, Takeda-Kawaguchi, T, Kuroda, I, Hotta, Y, Kawasaki, H, Hariyama, T, et al. Exosomes from dental pulp cells attenuate bone loss in mouse experimental periodontitis. J Periodontal Res. (2022) 57:162–72. doi: 10.1111/jre.12949
144. Imanishi, Y, Hata, M, Matsukawa, R, Aoyagi, A, Omi, M, Mizutani, M, et al. Efficacy of extracellular vesicles from dental pulp stem cells for bone regeneration in rat calvarial bone defects. Inflammation and Regeneration. (2021) 41:12. doi: 10.1186/s41232-021-00163-w
145. Jiao, J., Milwid, J.M., Yarmush, M.L., and Parekkadan, B., A mesenchymal stem cell potency assay. Methods in molecular biology (Clifton, N.J.) 677 (2011) 221–231 BioMed Central.
146. Ward, CK, Gill, RG, Liddell, RS, and Davies, JE. Umbilical cord stem cell lysate: a new biologic Injectate for the putative treatment of acute temporomandibular joint inflammation. J Inflamm Res. (2023) 16:4287–300. doi: 10.2147/JIR.S420741
147. Wang, Z, Zhang, X, Qi, L, Feng, W, Gu, Y, and Ding, Y. Olfactory mucosa tissue-derived mesenchymal stem cells lysate ameliorates LPS-induced acute liver injury in mice. BMC Pulm Med. (2022) 22:414. doi: 10.1186/s12890-022-02204-7
148. Khubutiya, MS, Temnov, AA, Vagabov, VA, Sklifas, AN, Rogov, KA, and Zhgutov, YA. Effect of conditioned medium and bone marrow stem cell lysate on the course of acetaminophen-induced liver failure. Bull Exp Biol Med. (2015) 159:118–23. doi: 10.1007/s10517-015-2905-x
149. Nishikawa, T, Maeda, K, Nakamura, M, Yamamura, T, Sawada, T, Mizutani, Y, et al. Filtrated adipose tissue-derived mesenchymal stem cell lysate ameliorates experimental acute colitis in mice. Dig Dis Sci. (2021) 66:1034–44. doi: 10.1007/s10620-020-06359-3
150. Albersen, M, Fandel, TM, Lin, G, Wang, G, Banie, L, Lin, CS, et al. Injections of adipose tissue-derived stem cells and stem cell lysate improve recovery of erectile function in a rat model of cavernous nerve injury. J Sex Med. (2010) 7:3331–40. doi: 10.1111/j.1743-6109.2010.01875.x
151. Duan, X, Luo, Y, Zhang, R, Zhou, H, Xiong, W, Li, R, et al. ZIF-8 as a protein delivery system enhances the application of dental pulp stem cell lysate in anti-photoaging therapy. Materials Today Advances. (2023) 17:100336. doi: 10.1016/j.mtadv.2022.100336
152. Katagiri, W, Osugi, M, Kawai, T, and Hibi, H. First-in-human study and clinical case reports of the alveolar bone regeneration with the secretome from human mesenchymal stem cells. Head Face Med. (2016) 12:5. doi: 10.1186/s13005-016-0101-5
153. Vizoso, FJ, Eiro, N, Cid, S, Schneider, J, and Perez-Fernandez, R. Mesenchymal stem cell Secretome: toward cell-free therapeutic strategies in regenerative medicine. Int J Mol Sci. (2017) 18:1852. doi: 10.3390/ijms18091852
154. Hu, C, Zhao, L, Zhang, L, Bao, Q, and Li, L. Mesenchymal stem cell-based cell-free strategies: safe and effective treatments for liver injury. Stem Cell Res Ther. (2020) 11:377. doi: 10.1186/s13287-020-01895-1
155. Nowzari, F, Zare, M, Tanideh, N, Meimandi-Parizi, A, Kavousi, S, Saneian, SM, et al. Comparing the healing properties of intra-articular injection of human dental pulp stem cells and cell-free-secretome on induced knee osteoarthritis in male rats. Tissue Cell. (2023) 82:102055. doi: 10.1016/j.tice.2023.102055
Keywords: bone defect, cell therapy, cell-free therapy, dental pulp stem cells, bone regeneration
Citation: Liu Y, Xiong W, Li J, Feng H, Jing S, Liu Y, Zhou H, Li D, Fu D, Xu C, He Y and Ye Q (2024) Application of dental pulp stem cells for bone regeneration. Front. Med. 11:1339573. doi: 10.3389/fmed.2024.1339573
Edited by:
Chanyuan Jin, Peking University Hospital of Stomatology, ChinaReviewed by:
Xiaogang Xu, Zhejiang University, ChinaNa Li, Foshan University, China
Yinghong Zhou, The University of Queensland, Australia
Copyright © 2024 Xiong, Li, Feng, Jing, Liu, Zhou, Li, Fu, Xu, He and Ye. This is an open-access article distributed under the terms of the Creative Commons Attribution License (CC BY). The use, distribution or reproduction in other forums is permitted, provided the original author(s) and the copyright owner(s) are credited and that the original publication in this journal is cited, in accordance with accepted academic practice. No use, distribution or reproduction is permitted which does not comply with these terms.
*Correspondence: Qingsong Ye, cWluZ3Nvbmd5ZUB3aHUuZWR1LmNu; Yan He, aGVsZW4tMTEwMUBob3RtYWlsLmNvbQ==; Chun Xu, Y2h1bi54dUBzeWRuZXkuZWR1LmF1
†These authors have contributed equally to this work