- 1State Key Laboratory of Respiratory Disease, Guangzhou Institutes of Biomedicine and Health, Chinese Academy of Sciences, Guangzhou, China
- 2Guangdong-Hong Kong-Macao Joint Laboratory of Respiratory Infectious Diseases, Guangzhou Institutes of Biomedicine and Health, Chinese Academy of Sciences, Guangzhou, China
- 3University of Chinese Academy of Sciences, Beijing, China
- 4China-New Zealand Joint Laboratory on Biomedicine and Health, Guangzhou Institutes of Biomedicine and Health, Chinese Academy of Sciences, Guangzhou, China
- 5Department of Microbiology, Shaheed Shamsuzzoha Institute of Biosciences, Affiliated with University of Rajshahi, Rajshahi, Bangladesh
- 6Guangzhou Medical University, Guangzhou, China
- 7Guangzhou National Laboratory, Guangzhou, China
- 8Department of Medicine, Center for Emerging Pathogens, Rutgers-New Jersey Medical School, Newark, NJ, United States
- 9Laboratory of Pathology, Department of Pathobiology, University of Poonch Rawalakot, Azad Kashmir, Pakistan
- 10Department of Biochemistry and Molecular Biology, University of Rajshahi, Rajshahi, Bangladesh
- 11State Key Laboratory of Respiratory Disease, Guangzhou Chest Hospital, Guangzhou, China
- 12State Key Laboratory of Respiratory Disease, National Clinical Research Center for Respiratory Disease, The National Center for Respiratory Medicine, The First Affiliated Hospital of Guangzhou Medical University, Guangzhou, China
Clofazimine (CFZ) and bedaquiline (BDQ) are currently used for the treatment of multidrug-resistant (MDR) Mycobacterium tuberculosis (Mtb) strains. In recent years, adding CFZ and BDQ to tuberculosis (TB) drug regimens against MDR Mtb strains has significantly improved treatment results, but these improvements are threatened by the emergence of MDR and extensively drug-resistant (XDR) Mtb strains. Recently, CFZ and BDQ have attracted much attention for their strong clinical efficacy, although very little is known about the mechanisms of action, drug susceptibility test (DST), resistance mechanisms, cross-resistance, and pharmacokinetics of these two drugs. In this current review, we provide recent updates on the mechanisms of action, DST, associated mutations with individual resistance and cross-resistance, clinical efficacy, and pharmacokinetics of CFZ and BDQ against Mtb strains. Presently, known mechanisms of resistance for CFZ and/or BDQ include mutations within the Rv0678, pepQ, Rv1979c, and atpE genes. The cross-resistance between CFZ and BDQ may reduce available MDR-/XDR-TB treatment options. The use of CFZ and BDQ for treatment in the setting of limited DST could allow further spread of drug resistance. The DST and resistance knowledge are urgently needed where CFZ and BDQ resistance do emerge. Therefore, an in-depth understanding of clinical efficacy, DST, cross-resistance, and pharmacokinetics for CFZ and BDQ against Mtb can provide new ideas for improving treatment outcomes, reducing mortality, preventing drug resistance, and TB transmission. Along with this, it will also help to develop rapid molecular diagnostic tools as well as novel therapeutic drugs for TB.
1 Introduction
Tuberculosis (TB) is one of the most frequent chronic infectious diseases that poses an important threat to public health and a big global social problem (1) with 1.6 million deaths owing to TB in 2021 (2). One of the key reasons for deaths due to TB is drug resistance. The COVID-19 pandemic is still having a damaging affect for both TB diagnosis and treatment cases. Of note, the success rate of cure is very high for drug-sensitive patient using the two most-effective drugs isoniazid (INH) and rifampicin (RIF) compared to drug-resistant (DR) patient. The rapid increase of multidrug-resistant TB (MDR-TB, defined as combined resistance to RIF and INH) is a major challenge for treating and controlling of TB transmission worldwide. According to latest TB reports, close to half a million (450000) people developed MDR/ RIF-resistant TB (RR-TB) of which 3.6% among new cases and 18% among previously treated (2). Notwithstanding, pre-extensively drug-resistant TB (pre-XDR-TB) and XDR-TB raise more concerns for treating and preventing TB (1). The new definitions of pre-XDR-TB [defined as TB caused by Mtb strains that fulfill the definition of MDR/ RR-TB and are additional resistant to any fluoroquinolone (FQ) drug] and XDR-TB [defined as TB caused by Mtb strains that fulfill the definition of MDR/RR-TB and are additional resistant to any FQ drug plus at least one more drug of Group A, bedaquiline (BDQ) or linezolid (LZD)] have been introduced into TB program by the World Health Organization (WHO) in October 2021 and applicable from January 2021 (3, 4). The overall treatment success rates of MDR/RR-TB and XDR-TB were only 60 and 39%, respectively (1, 5). The treatment regimens for DR-TB, particularly for MDR-TB and XDR-TB, are longer, more expensive, more toxic, and less efficacious compare to drug-susceptible TB (DS-TB). However, the treatment of MDR-TB and XDR-TB has often failed owing to drug resistance and a lack of effective anti-TB drugs. Therefore, there is an urgent demand to develop novel anti-TB drugs with more effective, less expensive, and a short treatment cycle for the treatment of DR-TB.
Clofazimine (CFZ), a riminophenazine antimicrobial agent, is primarily used for the treatment of Mycobacterium leprosy infections. This drug is also recommended by the World Health Organization (WHO) as a key drug in both shorter and longer DR-TB regimens (6, 7). However, CFZ has good activity against DR Mtb strains for both in vitro and in vivo studies (8). The mechanism of action and resistance to CFZ are not fully understood (7). Mutation in Rv0678 gene, encoding the MmpR5 repressor protein, is associated with resistance to CFZ in Mtb strains. In addition, mutations in the Rv2535c (pepQ) and Rv1979c genes have also been found in CFZ-resistant mutants (9).
Bedaquiline (BDQ), a diarylquinoline, was approved in 2012 by the Food and Drug Administration for the treatment of MDR-TB (10) and it inhibits the adenosine triphosphate (ATP) synthase encoded by atpE gene of Mtb. Cohort studies reported that success rates of MDR-TB treatment with BDQ-containing regimens was 70–80% (11–13). Similar results have been found for XDR-TB, where treatment results without BDQ are even worse (13, 14). The use of BDQ for the treatment of MDR-and XDR-TB reduces the mortality rate (15, 16). Therefore, the rapid appearance of BDQ resistance soon after introduction is alarming. Recently, BDQ resistance appeared in Mtb in both in vitro and clinical studies (17–19). Currently known resistance mechanisms to BDQ are associated with mutations within the atpE, Rv0678, and pepQ genes (20, 21). Significantly, mutations in the atpE which is the main pathway for BDQ cause higher-level of BDQ resistance and these mutations do not appear to impart cross-resistance to CFZ.
Cross-resistance is appeared between BDQ and CFZ due to confer mutations in the Rv0678, Rv1979c, and pepQ genes (22). The mutations in Rv0678 gene have been reported in both MDR-and drug-sensitive Mtb strains from TB patients without a history of treatment with CFZ or BDQ (17, 23). Interestingly, CFZ-resistant spontaneous mutants harbored Rv0678 mutations that confer cross-resistance to BDQ, indicating clinical use of CFZ could lead to BDQ resistance even without BDQ use (18). Unfortunately, there are limited clinical data, particularly in TB-epidemic regions, on the association for cross-resistance between genotypic and phenotypic BDQ and CFZ resistance in Mtb clinical isolates. The development of reliable drug susceptibility testing (DST) and genetic-based resistance screening for CFZ and BDQ are urgently needed. Therefore, this review is focused on providing recent updates on the mechanism of action, associated mutations with individual resistance and cross-resistance, DST, clinical uses, and pharmacokinetics to CFZ and BDQ against Mtb strains.
2 Clofazimine
CFZ, a riminophenazine antibiotic, was initially discovered in Dublin in the 1950s’ and used in the treatment of leprosy caused by M. leprae (24, 25). CFZ possesses both anti-inflammatory, pro-oxidative, and anti-mycobacterial properties (26, 27). Recently, this drug has gained attention once more as a substitute for treating Mtb infections (24, 28). Notably, CFZ has demonstrated potent activity against Mtb, including MDR-TB strains in both studies in vitro and in animal (8, 29–31). Additionally, the adverse effects of CFZ include cutaneous, gastrointestinal, and skin side effects, as well as a relatively low plasma drug concentration and a lengthy half-life (32). The demand of the CFZ-containing regimens for the treatment of MDR-TB increased after a study conducted in Bangladesh (33). Their observational study assessed the efficiency of standardized regimens with second-line drugs for untreated MDR-TB strains. The introduction of CFZ increased the success rate in MDR-TB treatment cohorts in clinical trials (8, 28, 34). Therefore, understanding the initial level of resistance is necessary to formulate a suitable strategy for the potential widespread use of CFZ against MDR-TB strains in developing nations.
3 Mechanism of action of CFZ
Currently, the mechanism of action of CFZ is still not well understood (7). It is reported that CFZ targets several sites in tubercle bacilli (35). However, it has been asserted that the cellular membrane of this antibiotic appears to be its primary site of action. The putative targets for the mechanism of action of CFZ include the mycobacterial respiratory chain and ion transporters (36, 37). In Mtb, CFZ seems to function like a prodrug that is reduced by NADH dehydrogenase (NDH-II) leading to reoxidation by oxygen (O2) to produce reactive oxygen species (ROS). Menaquinone (MK-4), a key component in the electron transfer chain (ETC) enzyme NDH-II of mycobacteria (36, 38), competes with CFZ for NDH-II reduction (Figure 1). Significantly, CFZ acts as a synthetic electron acceptor and thereby reduces flow of electrons through the mycobacterial ETC (7) and ultimately affects the generation of ATP. According to another theory, CFZ interacts with bacterial membrane phospholipids to produce antimicrobial toxic lysophospholipids. Then, lysophospholipids prevent potassium (K+) absorption and ultimately decreasing or inhibiting ATP production and thereby cause significant membrane instability (24). Other proposed that CFZ binds to the bacterial DNA for blocking the function of DNA and thereby inhibits bacterial proliferation (39).
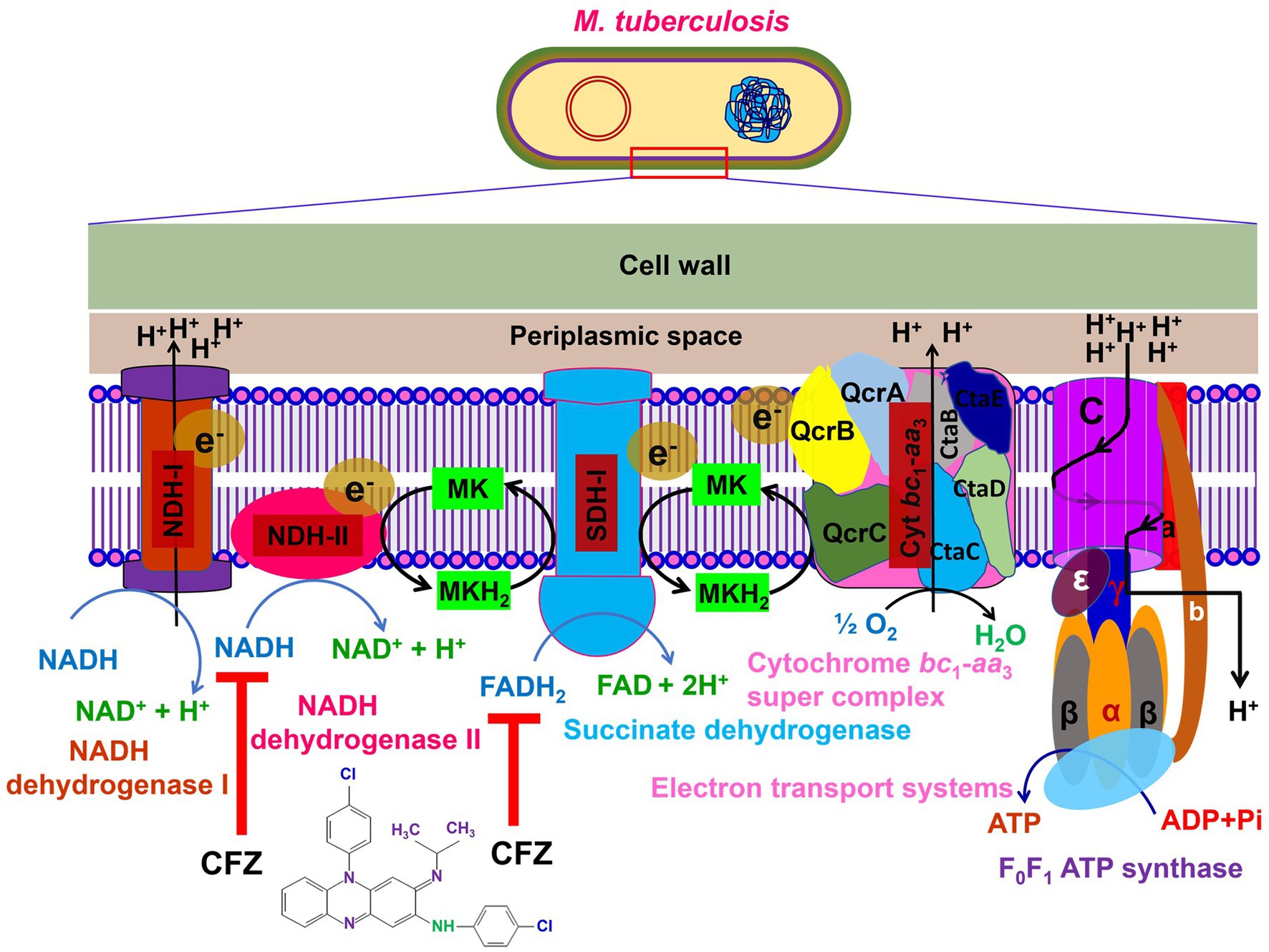
Figure 1. The mechanism of action of CFZ to the bacterial membrane. The electron transport chain (ETC) is a series of complexes for electron transfer from electron donors to electron acceptors across the bacterial membrane and shuttles electron from NADH and FADH2 to molecular O2. The protons are pumped out via electron movement from NADH and FADH2 then moves to ATP synthase for ATP production. So, CFZ accepts electron from the NADH dehydrogenase-II and thereby reduction flow of electrons through the mycobacterial ETC and reducing the synthesis of ATP production.
Recently, CFZ has garnered attention in order to its ability anti-inflammatory properties in both infectious diseases and immune diseases including Crohn disease, discoid lupus erythematosus, ulcerative colitis, chronic lymphocytic leukemia, etc. (26). Notably, the pandemic status of the coronavirus disease of 2019 (COVID-19) was announced in March 2020. Since then, a number of investigations have been carried out to determine the best treatment care for this unique infection. CFZ was among the drugs that demonstrated promising outcomes against COVID-19 (40–42). Because it prevents T-lymphocyte activation and proliferation, CFZ has anti-inflammatory activity. A number of mechanisms have been hypothesized, including the direct inhibition of T-cell Kv 1.3 potassium channels, and indirect action by encouraging the release of E-series prostaglandins and ROS from nearby monocytes and neutrophils. In conclusion, CFZ appears to have a variety of pathways for fighting bacteria, may be with varied significance placed on various processes depending on the physiological conditions (24).
4 Drug susceptibility test of CFZ
The Clinical & Laboratory Standards Institute (Wayne, PA, United States) and United States Food and Drug Administration (Silver Spring, MD, United States) have not established a breakpoint for CFZ susceptibility testing, despite the fact that the WHO has based its estimate of the critical concentration (CC) in mycobacteria growth indicator tube (MGIT) at 1 mg/L on tiny research and unpublished data (32, 43–45). Previous research has shown that CFZ has Mtb minimal inhibitory concentrations (MICs), including MDR-TB strains, with typical ranges between 0.125 and 2.0 mg/L. (32, 46, 47) According to several studies, CFZ breakpoints were ranges between 0.25 and 1 mg/L. (44, 45, 48–50) The CCs or cutoff of CFZ for DST are summarized in Table 1. In a different Chinese investigation, 90 XDR-TB strains were used to assess the MIC using the microplate alamar blue assay (MABA) method against several drugs includes CFZ, and BDQ (48). They found the breakpoints MIC of CFZ for drug susceptibility were defined as 1.0 μg/mL. Finally, it can be concluded that the supporting laboratory and clinical data for CFZ resistance are required regarding the DST and MICs. On the other hand, the range of BDQ susceptibility is 0.12 to 2.0 μg/mL in different methods (51–56).
5 Clinical treatment efficacy of CFZ
Several new and repurposed oral anti-TB drugs include BDQ, pretomanid (PMD), delamanid (DLM), CFZ, LZD, and carbapenems (CPM), appear to be safe and effective enough to treat in a majority of MDR-and XDR-TB patients. CFZ has been used for leprosy treatment since 1962. The use of CFZ has been interested to treat MDR-TB owing to increasing emergence of MDR-Mtb strains (57, 58). CFZ-containing regimens have shown improved results in the treatment of RR-TB and MDR-TB cases. Most of the CFZ-containing shorter treatment regimen (STR) studies conducted in different countries, i.e., Bangladesh, Cameroon, Niger, Guinea, Africa, Vietnam, Burundi, China, and Uzbekistan etc. have demonstrated great therapeutic success (the total of cure and treatment completed) rates between 66.3 and 92.9% for RR-/MDR-TB patients (Table 2) (33, 34, 59–71).
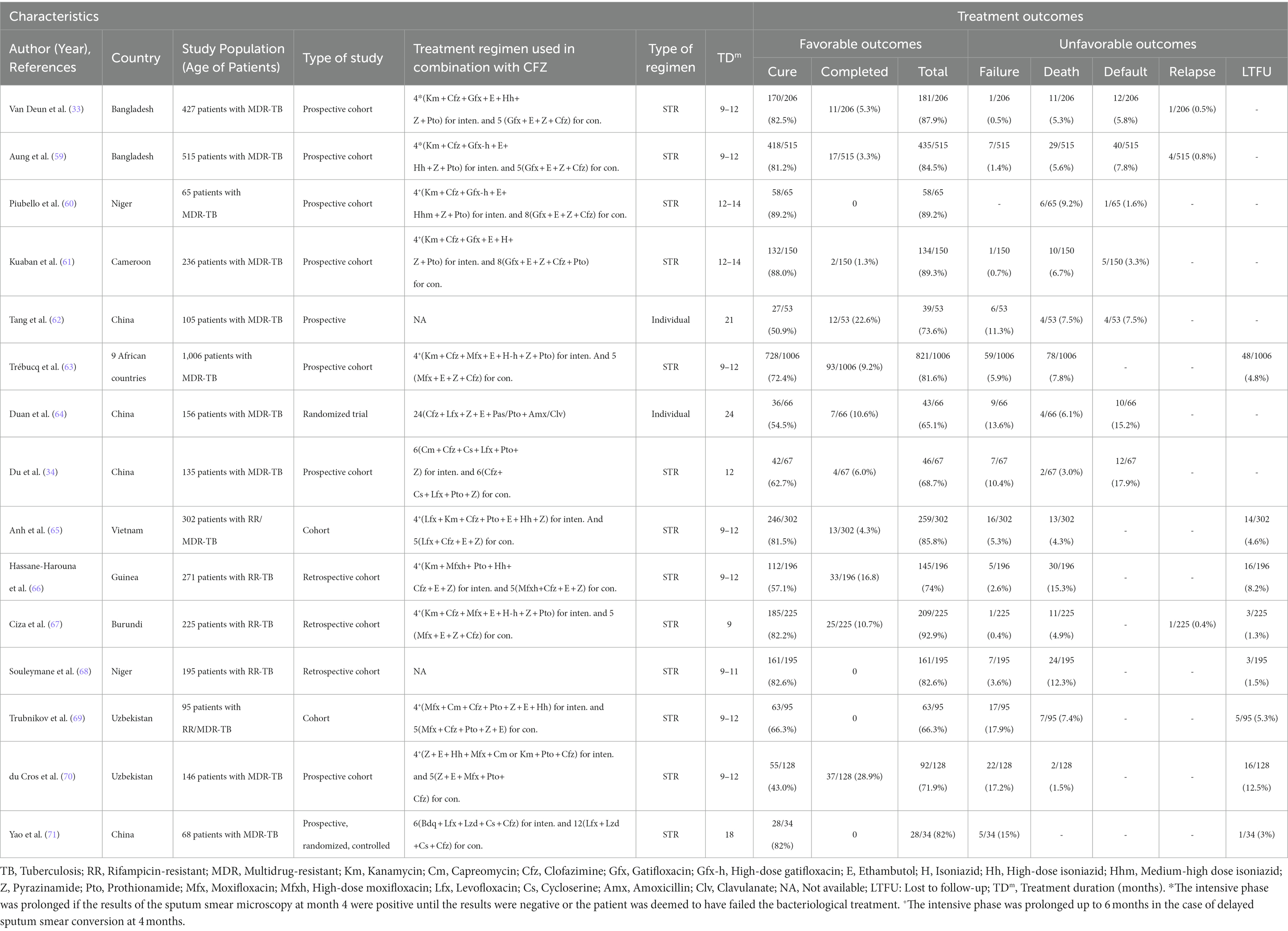
Table 2. Summary of observational research studies describing for RR-/MDR-TB patients treated with CFZ-containing individual or standardized shorter treatment regimen.
In Bangladesh, Van Deun and his team searched a shorter and more efficacies treatment regimen for MDR-TB to increase treatment success rate and to minimize treatment failure, death and lost to follow-up (33). They found a standardized 9-month Bangladesh regimen after evaluating six combinations of drugs and duration of treatment. The 9-month Bangladesh regimen includes gatifloxacin (GFX) in combination with CFZ, ethambutol (EMB), and pyrazinamide (PZA) throughout, supplemented by kanamycin (KAN), prothionamide (PTO), and high-dose isoniazid (INHh) during an intensive phase of 4 months (4–6 GFX-CFZ-EMB-PZA-KAN-PTO-INHh−/5 GFX-CFZ-EMB-PZA) and this phase was increased up to maximum 6 months, keeping the continuation phase duration at 5 months. Significantly, in 206 patients those received this STR treatment, the relapse-free cure rate was 87.9% (95% confidence interval, 82.7–91.6). In 2014, almost similar outcomes for 515 patients were reported by the same research team from Bangladesh (59). These findings were further supported those reported from China (82%), Niger (82.6%), Vietnam (85.8%), Cameroon (89.3%), Burundi (93%) and these results are highly effective and well tolerated against MDR-TB but not previously exposed or resistance to second-line drugs (61, 65, 67, 68, 71).
A recent interesting prospective, randomized, multicenter study conducted in China was carried to compare between 12-month treatment CFZ-containing shorter regimen and 18-month without CFZ-containing regimen for MDR-TB patients. Significantly, 68.7% MDR-TB patients receiving CFZ-containing shorter regimen had sputum culture conversion in comparison with 55.9% of those receiving regimen without CFZ, reflecting an early culture conversion (p = 0.04). This finding indicates that CFZ-containing shorter regimen had a comparable successful result when compared to without CFZ-containing regimen. The patients assigned to the CFZ-containing shorter regimen showed more rapid culture conversion compared to without CFZ-containing regimen, indicating impressive antimicrobial activity against MDR-TB (34). Another prospective, randomized, multicenter, controlled, and open study conducted in same country (China) was done 21-month of individual-based treatment regimens to compare between CFZ-containing group and control (without CFZ) group against MDR-TB. The treatment successful outcome rate of this study in the CFZ group was 73.6% which is significantly higher than that in control (without CFZ) group (53.8%; p = 0.035). The adverse effect in skin only found in the CFZ-containing group (62). A very recent study in West and Central Africa stated CFZ-containing a nine-month short regimen for the treatment of RR-TB cases. The overall treatment success rate of this short regimen (4–6 KAN + CFZ + MFX + EMB + INHh+PZA + PTO/5 MFX + EMB + PZA + CFZ) was close to 80% relapse-free cure rate among 1,006 patients, which indicates the good outcome in low-and middle-income settings against RR-TB cases (72). Similarly, a recent meta-analysis showed greater favorable treatment outcomes after the use of CFZ-containing regimen compared with those receive no CFZ against MDR-TB cases (73). In 2018, another previously published meta-analysis reported that CFZ-containing regimens were associated with significantly improved treatment outcomes for MDR-TB (74).
For the CFZ-containing STR against RR-/MDR-TB cases, the fluoroquinolones (FQs) including GFX, moxifloxacin (MFX), and levofloxacin (LFX) are the core drugs. The activity of high-dose GFX-based regimens is higher than that of high-dose LFX-based or normal-dose MFX-based regimens (75, 76). The GFX-based STR was highly effective (approximately >84% treatment success rate) among MDR-TB cases in Bangladesh, Niger and Cameroon (77). Significantly, low-level drug resistance can be overcome when high-dose GFX is used (75, 76). GFX is not currently included in most STR setting programs to treat MDR-TB. These findings suggest reintroducing GFX into STR against RR-/MDR-TB treatment programs (75, 77).
It is very important to note that CFZ-containing STR is used second-line injectable drug (SLID), i.e., KAN or capreomycin (CM), to prevent acquired FQs resistance during the 4–6 months intensive phase. However, drug adverse event ototoxicity induced by the SLID used in the intensive phase is the primary concern ranging between 3.2 and 32.6% (67, 69, 78, 79). In a very recent meta-analysis by Wrohan et al. (80) reported that the incidence of hearing loss was 28.3% of MDR-TB patients receiving SLID after analysis of 64 studies from different 25 countries including 12,793 patients. The drug adverse ototoxicity due to receiving SLID can be permanent and may continue even after stopping these medications. The WHO is not recommending to enabling access any SLID to STR for the treatment of patients with RR-/MDR-TB due to severe side effects. Therefore, in 2020, the WHO is urging all countries the use of fully-oral treatment regimens for RR-/MDR-TB patients, either short or long (6). Although the efficacy of fully-oral treatment regimens are still not well understood. Importantly, a retrospective cohort study has shown that BDQ can effectively replace the SLID (81). Notwithstanding, many studies are still needed to assess the safety and efficacy of fully-oral treatment regimens for patients with RR-, MDR-/XDR-TB.
For XDR-TB, a multicenter, randomized, and prospective study investigated 36-months of individual-based treatment regimen to find out the efficacy and safety after treatment with CFZ, compared with XDR-TB patients those received no CFZ. They found CFZ-containing individual-based regimen did not increase the favorable treatment results or shorten the culture conversion time, when compared with no CFZ-containing regimen against XDR-TB patients (82). On the other hand, the treatment of XDR-TB showed that CFZ-containing regimens were more effective with cure rates 40% compared with receiving a non-CFZ regimen cure rate 28.6%. Adverse effects due to CFZ were infrequent and rarely serious enough to be life-threatening (83). We advise empirical inclusion of CFZ in XDR-TB therapy regimens because to the current low rates of culture conversion.
6 Resistance mechanisms of CFZ
CFZ has recently been demonstrated a good therapeutic effect for the treatment of MDR-TB and to shorten TB treatment. Despite the significance of CFZ in different regimens, programmatic implementation attempts have been hampered by the length and safety of these regimens, both of which are factors that encourage the emergence of resistance. Of note, less than 40% of CFZ-resistant strains had changes in genes known to induce CFZ resistance, which is in line with this study and suggests that additional research should be done to understand how CFZ works (8). The resistance to CFZ was detected in 8.3% (23/277) of the MDR-TB isolates. It is significant to note that the rate of acquired resistance to CFZ (6.3%, 12/189) was noticeably higher than the rate of primary resistance (12.5%, 11/88, p = 0.028). According to these findings, MDR-TB patients in China showed a significant prevalence of CFZ resistance. Another similar reported a prevalence of 7.4% (29/391) from patients of MDR-TB in South Africa (21). CFZ was not frequently utilized for MDR-TB therapy in the Chinese population due to an increased prevalence of skin discoloration. As a result, the high level of CFZ resistance raises additional worries about the potential of high mutation rate following exposure to the treatment. Notably, a current study by Shang et al. (50) reported 12/13 (92.31%) CFZ-resistant isolates were resistant to BDQ when a MIC of CFZ was ≥4 mg/L. One of the main risk factors for associated BDQ resistance is pre-XDR, together with exposure to CFZ. The percentage of DR-Mtb isolates having CFZ resistance with MIC >1 mg/L was 4.1% in a recent study (32). Of note, this study also reported that XDR isolates have a higher rate of CFZ resistance than MDR isolates.
It is still not completely understood how CFZ resistance develops. Mutations in Rv0678, Rv1979c, and Rv1453 have been documented to be linked resistance to CFZ (9, 18, 32, 84, 85). A recently report demonstrated mutations in Rv1979c, Rv1453 and/or Rv0678 were present in only 40.2% (29/72) CFZ-resistant Mtb isolates (50). Interestingly, four of the seven CFZ-resistant Mtb isolates carried only the Rv1979c mutation, while the other three isolates carried Rv0678 or Rv1453 mutations. The four CFZ-resistant isolates, however, only had a mutation in Rv1979c, which had a comparatively low-level MIC to CFZ. Another similar study reported that mutations were harbored in Rv1979c and Rv0678 in CFZ-resistant Mtb isolates accounted for 15.4% (86). One the other hand, several studies have revealed no mutation in Rv1979c was found among the CFZ-resistant strains (32). Current and several prior findings indicate that while the mutation in Rv0678 did not the primary cause CFZ resistance in Mtb isolates, it did play a major role in generating the cross-resistance between BDQ and CFZ (8, 50, 87). The huge number of variant types as well as scattered, and lack of a clear hotspot characterization of Rv0678 mutations were shown in a recently published review and research on CFZ resistance (32, 88, 89). Conversely, Zhang et al. (9) depicted at positions 193 and 466 of nucleotides in Rv0678, two mutational hot sites accounted for 42/96 (43.8%) and 11/96 (11.5%) of the mutations. Of note, different types of mutations in the Rv0678 gene conferring resistance to CFZ derived clinical and in vitro isolates of Mtb are summarized in Figures 2, 3 (8, 18, 22, 50, 87, 88, 90–93). Finally, it can be concluded that phenotype and genotype tests are needed of CFZ in RR-/MDR-TB patients before considering as CFZ-containing regimen due to increasing resistance for inappropriate use.
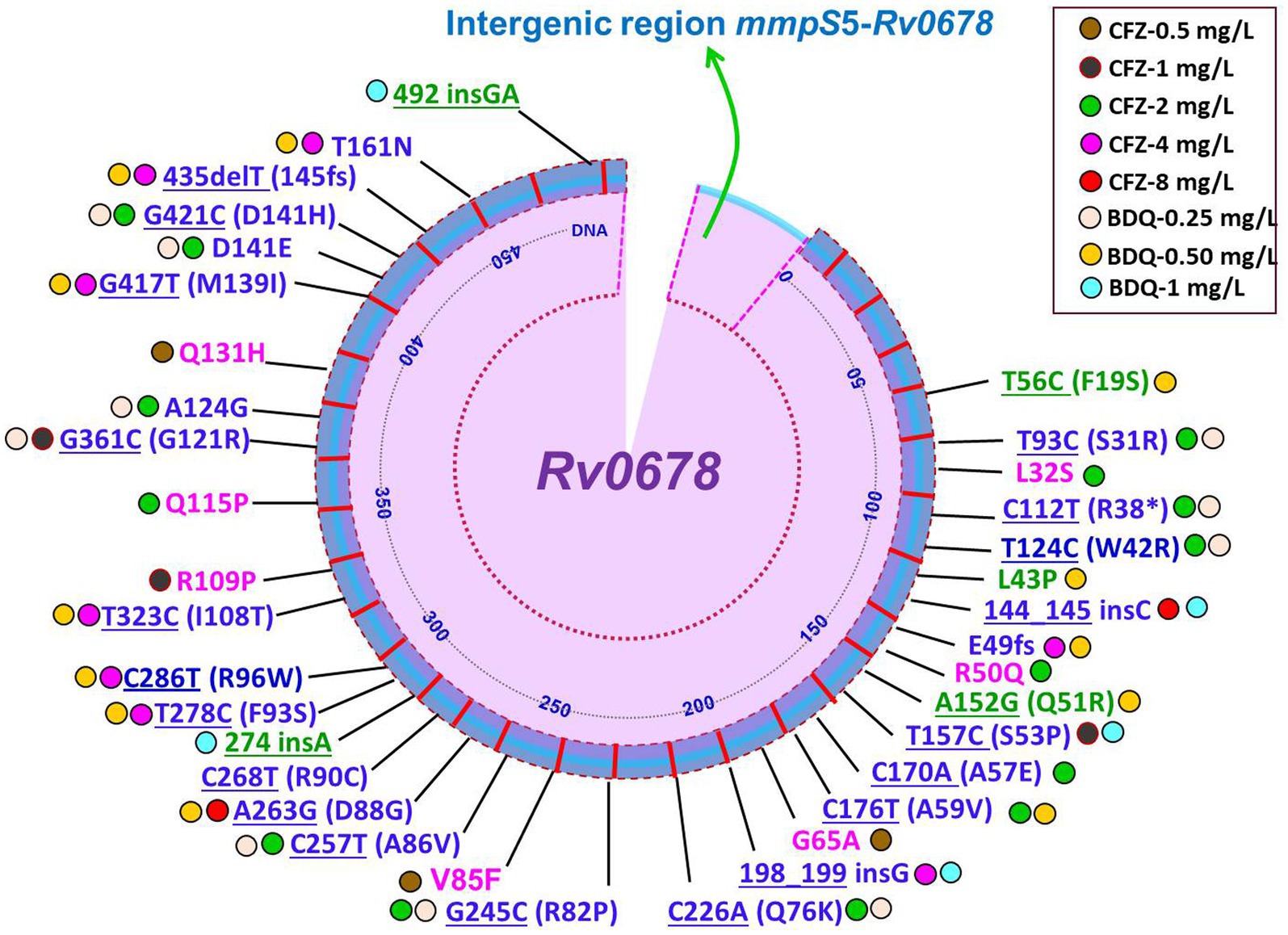
Figure 2. Schematic illustration of recently identified mutations in Rv0678 associated with individual resistance or cross-resistance to BDQ and CFZ in Mtb clinical isolates. Blue color mutations indicated cross-resistance; Magenta color mutations indicated resistance only for CFZ; Green color mutations indicated resistance only for BDQ; Underline mutations indicated DNA and without underline indicated amino acids; *indicated stop codon (8, 22, 50, 55, 56, 87, 88, 90, 91).
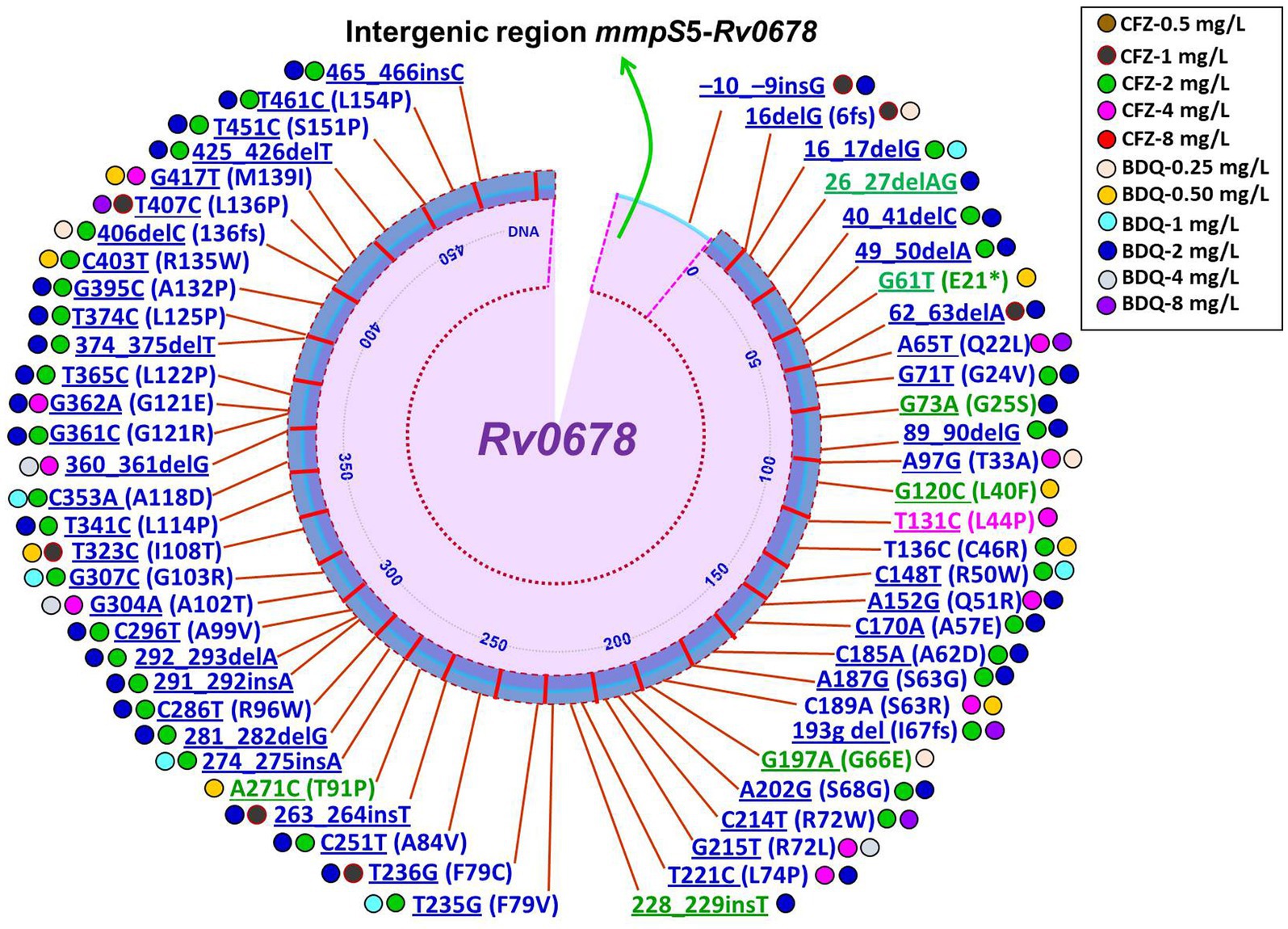
Figure 3. Schematic illustration of recently identified mutations in Rv0678 associated with individual resistance or cross-resistance to BDQ and CFZ in Mtb in vitro and in vivo isolates. Blue color mutations indicated cross-resistance; Magenta color mutations indicated resistance only for CFZ; Green color mutations indicated resistance only for BDQ; Underline mutations indicated DNA and without underline indicated amino acids; * indicated stop codon (16, 18, 91–93).
7 Pharmacokinetics of CFZ
There has not been enough research done on the pharmacokinetics of CFZ, and data from TB patients are particularly rare. CFZ is incredibly lipophilic and abundantly distributes throughout fatty tissues. Because of its high lipophilicity, CFZ accumulates heavily in fat tissues while having relatively low blood concentrations (0.7–1 mg/L) (24, 94). The macrophage-rich organs of the lungs, liver, brain, spleen, and bone marrow are included in the fat tissues (95, 96). Whether a drug is administered along with or without food can have a significant impact on how well it is absorbed in human. CFZ is varied in its absorption after oral administration, ranging from 45 to 62% based on whether or not the CFZ is taken with food (24). Ingestion of food simultaneously increases the rate of CFZ absorption. The unaltered plasma peak is attained 6 to 12 h after a single oral dose of CFZ in the form of a capsule. The peak plasma concentration was 0.41 mg/L and the time to Cmax was 8 h when a 200 mg CFZ tablet was ingested with food, on the other hand the peak plasma concentration was 30% lower and the time to Cmax was 12 h when a 200 mg CFZ tablet was administered without food (24). Swanson and colleagues (96) reported that no variations in bacterial killing were seen between any of the CFZ doses given to Mtb-infected mice and the lowest dose was equally effective as the maximum dose. They suggested that it is possible to treat TB with considerably lower doses because the anti-TB activity of CFZ was not affected by either the dose given or the drug concentrations in the tissues. On the other hand, recently a study from South Africa depicted the body fat percentage had a significant impact on CFZ disposition, which led to reduced plasma exposure in women (97). They reported CFZ may need dose individualization at variations of body composition to maximize utilization, although the therapeutic effects are unknown (97, 98). Of note, it is stated that CFZ is more effectively absorbed when consumed with a high-protein and fat diet. On the other hand, CFZ will decrease its bioavailability when consumed with orange juice and an antacid (99).
The body retains CFZ for a long time. Therefore, the toxicities such as skin discoloration, QT prolongation and elevated liver enzymes are associated with CFZ, while it is not clear how these side effects relate to dose or plasma concentrations of the drug (100). The fact that CFZ causes the QT prolongation raises concerns because numerous other drugs, including BDQ, FQs, and DLM, that are approved by the WHO for treating DR-TB, also cause the QT interval to lengthen (101). The QT prolongation of CFZ is associated with cardiac arrhythmias. Therefore, the combination of three drugs includes MXF, BDQ, and CFZ that significantly lengthen the QT interval should not be used together in a regimen for TB treatment.
8 Bedaquiline
BDQ, a novel diarylquinolone drug, demonstrated outstanding efficacy against both DR- and DS-TB (55, 90). BDQ is now an essential component of the shorter oral regimen for MDR-TB treatment (102, 103). Significantly, the United States FDA granted rapid approval the combined use of BDQ and DLM for the treatment of MDR-/XDR-TB (37). The WHO in 2018 has recommended for using BDQ as an important antibiotic to be used along with LZD and FQs to treat MDR-TB (43). Surprisingly, a three-drug regimen consisting of LZD, BDQ, and PMD was evaluated with MDR-/XDR-TB patients in a recent study called the NIX-TB trial and the treatment was effective for 90% of TB patients (15). The use of BDQ is now spreading quickly and more than 90 countries having started using BDQ. However, inadequate knowledge of resistance mechanisms is impeding quick molecular diagnosis.
9 Mechanism of action of BDQ
BDQ is closely linked to FQs and chloroquine, albeit it has a distinct side-chain moiety. The mechanism of action of BDQ is different from FQs, and it has no inhibitory effects on DNA gyrase. Energy metabolism enzymes in Mtb for the development of drugs/compounds, such as F0F1 (F0, a rotor and F1, a stator) and the respiratory chain complexes, have been proved as new promising targets. Among the class of bioenergetics inhibitors, BDQ was the first drug approved by the United States FDA and the European Medicines Agency (103, 104). According to reports, BDQ interacts with the F0 domain to specifically target F0F1 of Mtb (105). As a result, ATP generation is inhibited, and ATP levels drop significantly. The ATP synthase enzyme consists of two sectors: the membrane sector F0, which contains three subunits (a, b2 and c n ), and the cytoplasmic sector F1, which has five subunits (3α, 3β, γ, δ, and ε). The subunits c of F0 are organized in the shape of disks and serve as an ion-conducting route, while ADP and phosphate (Pi) are combined at three catalytic sites in F1 to generate ATP (104). It is widely known that BDQ has the ability to attach the subunit c in the F0 rotor ring of the ATP synthase and inhibit its function (Figure 4). In addition, BDQ has also been showed to prevent ATP synthesis via a second targeting binding site in the ε subunit on the mycobacterial F0F1-ATP synthase and prevent to generate ATP (106).
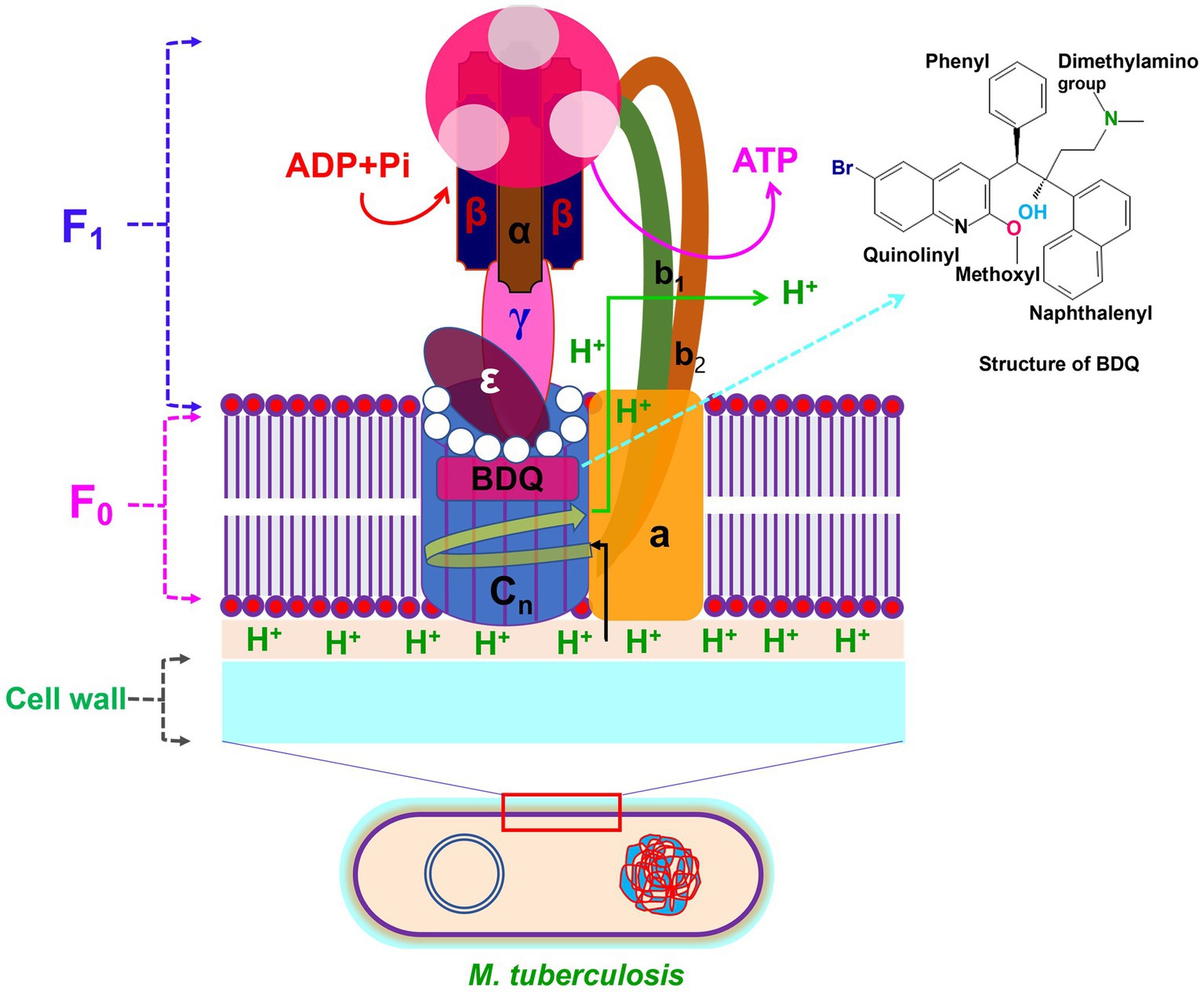
Figure 4. Structure of F0F1-ATP synthase with BDQ binding site. The F0 domain of ATP synthase is made of a, b2 and c n (n = 10–14) subunits. The F1 domain of ATP synthase is consist of 8 subunits including 3α, 3β, γ, δ and ε. BDQ binds to the subunit of c in the F0 rotor ring of the ATP synthase and inhibit the synthesis of ATP production.
10 Drug susceptibility test of BDQ
BDQ has been given accelerated/conditional authorization for usage based on phase-II trials conducted in the European Union (2014), the United States (2012), and seven other nations with prevalence of MDR-TB incidence. Nowadays, BDQ is an important drug for the treatment of MDR-TB because it is included in the revised definitive WHO criteria for XDR-TB in 2021 (107). Two phase-II clinical investigations for BDQ used the preliminary DST approach that had previously been evaluated. The CC/cutoff values reported for BDQ susceptibility are summarized in Table 1 (8, 18, 21, 45, 48–56). Quality control (QC) parameters have been established in a multilaboratory, multicountry study for the BDQ phenotype DST utilizing 7H10 and 7H11 agar proportion method (APM) and 7H9 broth microdilution (BMD) MIC techniques (108). BDQ DST techniques and QC of MIC ranges against the Mtb H37Rv reference strain have been developed 0.015 to 0.06 μg/mL for the 7H9 BMD and 0.015 to 0.12 μg/mL for the 7H11 and 7H10 APM. Importantly, Keller et al. (109) reported BDQ DST using the MGIT 960 method and epidemiological cutoff value of 1.6 μg/mL, on the other hand, another study by Torrea et al. (110) proposed 1.0 μg/mL. Of note, a very recent systemic study by Nieto Ramirez et al. (111) reported that the most popular DST technique for BDQ was MGIT960. The CC or cutoff for BDQ susceptibility confirmed by several studies is 0.25 μg/mL for the 7H11 and 7H10 APM, 0.25 μg/mL for the BMD and 1.0 μg/mL for the MGIT 960 (43, 111, 112). Two studies for the resazurin microtiter assay (REMA) technique indicated a CC for BDQ of 0.25 mg/L, which was similarly obtained when utilizing BMD and MABA methods (53). Notably, the MIC susceptible breakpoint concentration was defined as ≤0.12 μg/mL by another study for BDQ when using MABA method (56), while another very recent study from China reported the breakpoint concentrations of BDQ susceptibility were defined as 0.25 μg/mL (55). Finally, it can be said that it is crucial to assess the MIC of BDQ even in patients those have no prior exposure to the drug or CFZ exposure when selecting an effective therapeutic regimen.
11 Clinical treatment efficacy of BDQ
Novel therapeutic anti-TB drugs like BDQ, DLM, LZD, and PMD are promising for the treatment of MDR, pre- and XDR-TB patients. The BDQ, an anti-TB drug, has been approved for the treatment of MDR-TB, and recommended for 24 weeks duration (11). Most of the BDQ-containing treatment studies conducted in different countries, i.e., France, Armenia, Georgia, Congo, South Africa, China, Nigeria, India etc., have demonstrated great therapeutic success (the total of cure and treatment completed) rates between 58.5 and 93.5% (Table 3) (11, 12, 14, 15, 27, 113–122).
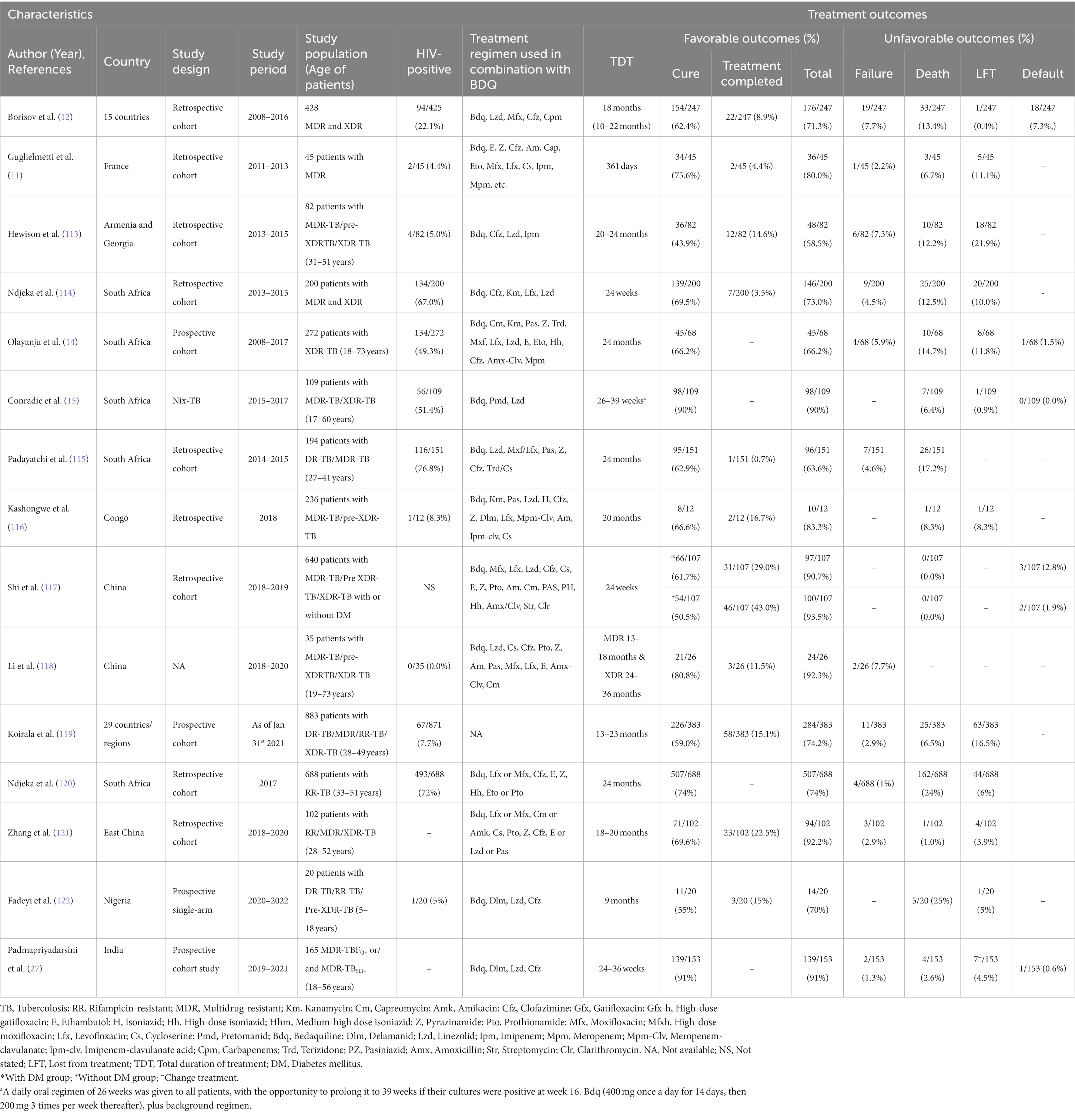
Table 3. Summary of observational research studies describing for RR-/MDR-TB patients treated with BDQ-containing individual or standardized shorter/longer treatment regimen with/without CFZ drug.
Indeed, numerous clinical studies confirm that the strong bactericidal and sterilizing activity of BDQ-containing regimen originally found in mouse models of TB (123, 124). BDQ has demonstrated potent clinical activity against MDR-and XDR-TB complex strains (27, 118, 121, 122). Importantly, BDQ-containing treatment regimens have showed improved outcomes rate over SLID-containing regimens for the treatment of patients with RR-/MDR-TB (120). Recently, the WHO suggested SLID be replaced in the standard STR with a BDQ-containing regimen. The recommended dose of BDQ is 400 mg orally once daily for 2 weeks followed by 200 mg orally three times weekly for 22 weeks and total duration of BDQ treatment was 24 weeks (117, 118, 120). Many studies have reported the use of BDQ favorable outcomes rates at week 24 of treatment exceeding 80% (15, 117). A very recent study in China has showed that 80.8% of MDR-/XDR-TB patients were cured after receiving BDQ-containing regimens (118). Another recent study from same country reported that the rate of success of culture conversion at 24 weeks is 85.3% of MDR-and XDR-TB patients after receiving BDQ-containing regimens (125). Of note, the findings of this study are more impressive for the treatment of 39 MDR-TB, 56 pre-XDR-TB, and 82 XDR-TB patients, even though for the treatment of pre-XDR and XDR-TB patients are more difficult than MDR-TB patients (125). They have given several feasible explanations for discrepancies in culture conversion rates across studies. Among these, the first one is background regimens which can influence results. For example, LZD can have a good impact on MDR-, pre-XDR- and XDR-TB patient in clinical outcomes. The second one is that BDQ accumulates to comparatively high concentrations in adipose tissue which is confirmed by previous pharmacokinetics research (126). It is interesting to note that MDR-/XDR-TB treatment containing BDQ with compassionate use of LZD showed relatively good success rates (118). Another similar retrospective cohort study reported that the combination of BDQ with LZD and/or imipenem (IPM) showed relatively good success results for treatment of MDR-TB with previously treated extensive and highly resistant TB patients (113). These findings indicate that the combination of BDQ and LZD has good activity in the treatment of MDR-/XDR-TB. Finally, it can be concluded that the administration of BDQ might be associated with additional useful for pre- and XDR-TB patients.
12 Resistance mechanisms of BDQ
BDQ is among the last anti-TB drugs approved for the use of MDR-and XDR-TB treatments, which are responsible for reducing mortality rates and improving outcomes (14, 127), and has been in use since 2012. Naturally, the risk of emerging resistance increases due to the widespread use of a new antibacterial drug. As of today, BDQ resistance raises concerns, soon after the introduction for the treatment of MDR-and XDR-TB patients. Indeed, the risk of increasing resistance to BDQ in Mtb can be occurred naturally or using for treatment in combination other antibiotics (56). Interestingly, the prevalence of BDQ resistance was 8.9% in isolates resistant to any first- and second-line drug, indicating that the rate of BDQ resistance also rose along with the diversity of drug resistance types and the complexity of resistant background (55). Additionally, retreated patients had a greater rate of BDQ resistance (66.7%) than newly diagnosed patients (33.3%), suggesting this attributed to the previous medical history requires for the use BDQ.
Importantly, the mechanism of BDQ resistance in Mtb mainly involves three genes, namely, the atpE (128), Rv0678 (52), and pepQ (54) genes. As of today, genetic mutations or resistance-associated variants (RAVs) in the atpE, Rv0678, Rv1979c, and pepQ genes have been associated with BDQ resistance (23, 129, 130). The atpE gene encodes the ATP synthase by targeting subunit C and its mutations are usually linked with high-level BDQ resistance in Mtb but the frequency of mutations is relatively low among TB patients (16). The binding of BDQ to subunit C of ATP synthase can be failure due to genetic mutations or RAVs in the atpE genes. Therefore, mutations in the atpE genes (A28P, A28V, G61A, A63P, and I66M) are associated with high-levels resistance (10 to 128-fold MIC) to BDQ. Furthermore, a report demonstrated that the frequency of atpE gene mutations in TB patients is extremely low, they are responsible for high-level of BDQ resistance (111).
Mutations in Rv0678, which codes for the repressor of the efflux pump MmpL5-MmpS5, are the primary cause of BDQ resistance and typically result in low-level resistance (131). Importantly, a very recent study reported from Chongqing, China that BDQ resistance was mostly caused by mutations in the Rv0678 gene, with the most frequent mutation type being A152G (55). Mutations in the Rv0678 gene encoding the efflux pumps MmpS5-MmpL5 as well as the intergenic region between Rv0678 and MmpS5 were also revealed to be the cause of BDQ resistance (132). Of note, different types of mutations in the Rv0678 gene conferring resistance to BDQ derived clinical and in vitro isolates of Mtb are summarized in Figures 2, 3 (8, 16, 22, 55, 56, 87, 90–93). In 2016, the gene pepQ (Rv2535c) was discovered as potential which may be associated to BDQ resistance (54). BDQ resistance has been associated to mutations in the genes pepQ and Rv1979c, which encodes a potential Xaa-Pro amino-peptidase and a putative permease, however the underlying mechanisms are yet unknown (111, 130). A study showed that mutations in pepQ gene have low-level resistance (up to 4 fold) to BDQ in mice (54). However, reduced antimycobacterial susceptibility to BDQ has been reported to be associated with mutations in Mtb strains in the Rv0678, pepQ, and atpE genes (90).
Nevertheless, a portion of BDQ resistant isolates identified no mutations in Rv0678, atpE, and pepQ in in vitro and clinical settings, although mutations in Rv0678, atpE, and pepQ confer major resistance to BDQ, suggesting that there are other unknown mechanisms of resistance to BDQ (133). Importantly, a new gene, glpK, (an insertion G572 mutation in glpK) is identified that resulted in a frame shift and loss of function, leading resistance to BDQ (93). The enzyme glycerol kinase (GlpK), encoded by Rv3696c, is a key enzyme for the glycerol uptake and metabolism. It catalyzes the phosphorylation glycerol to glycerol-3-phosphate for glycerophospholipids synthesis (134, 135) or the synthesis of glycolysis and gluconeogenesis (136). Further studies are required to explore the exact role of glpK gene. Finally, it can be concluded that resistance-conferring mutations in the atpE, Rv0678 and pepQ genes might be potential diagnosis determinants. In addition, careful evaluation is recommended for the prescription of BDQ in the regimen for the treatment of DR-, MDR-and XDR-TB patients. Although BDQ has been shown to be extremely effective in the treatment of MDR-TB until this point (55), but misdiagnosis, insufficient and/or incomplete use may lead to the emergency of resistance to Mtb strains (19, 92). For instance, patients infected with an MDR outbreak strain in Eswatini and South Africa those carried the RR variant I491F in the rpoB gene continued to receive RIF drug despite it being ineffective because this variant was not identifiable by conventional phenotypic or genotypic testing (137). Therefore, it is important to dynamically assess the BDQ resistance for optimizing BDQ administration regimen, furthermore to prevent the emergence of acquired resistance and maximize the efficacy of new drug.
13 Mechanisms of cross-resistance to CFZ and BDQ
Cross-resistance is a form of resistance to all drugs in the same class resulting from a single mechanism. Drugs that belong to the same class typically share a chemical structure, which means they act on the same cell target and can cause cross-resistance. Resistance to the CFZ and BDQ almost always arises from the build-up of mutations in the chromosomal genes that control permeability, active efflux, and drug targets. The presence of resistance-associated mutations linked to Rv0678 resulted in increased MICs for BDQ and CFZ in murine isolates (2 to 8 fold and 2 to 4 fold, respectively), and 2 to16 fold MICs for BDQ in clinical isolates. Clinicians are increasingly choosing BDQ and CFZ to treat DR-TB in recent years. Both drugs impair the energy metabolism in mycobacteria and several studies have reported cross-resistance between CFZ and BDQ (8, 50, 130, 137–140). Drug resistance for these two drugs should be closely monitored given their critical role in the treatment. The existence of cross-resistance between new and old drug used in MDR-TB strains undermines using new ones effectively. Therefore, cross-resistance to CFZ and BDQ thus emerges as a significant concern that possibly undermines the efficacy of BDQ treatment for DR-TB. Notably, 12/13 (92.31%) of the Mtb clinical isolates presented resistance to BDQ when the MIC of CFZ was ≥4 mg/L. (50) In addition, half of the CFZ-resistant isolates were classified as BDQ-resistant when the breakpoint for BDQ (0.12 mg/L) was used. This result was similar with other studies (8, 21), which revealed that at least half of the CFZ-resistant Mtb isolates were still sensitive to BDQ. However, every single isolate that was BDQ-resistant was also CFZ-resistant. Another important study reported that 12% of the MDR-TB patients had resistance to both BDQ and CFZ (45). According to clinical characterization, CFZ-resistant TB patients were more likely to develop BDQ resistance due to prior CFZ or BDQ exposure as well as pre-XDR-TB (50, 141). Conversely, it is speculated 4.4% MDR-TB isolates resistant to BDQ without record prior use of BDQ, suggesting this promising new drug could be rapidly lost due to the emergency of BDQ resistant isolates, although BDQ demonstrated remarkable effectiveness against MDR-TB strains (55).
Interestingly, MmpS5 and MmpL5, which are bacterial membrane proteins, are part of the efflux pump system shared by both BDQ and CFZ and exposure to CFZ may promote efflux-based resistance, leading to cross-resistance between these two drugs (52, 130). Many different studies have documented that mutation in Rv0678 can cause cross-resistance between BDQ and CFZ in Mtb strains (50, 142). Significantly, various mutations in the Rv0678 gene associated with cross-resistance to BDQ and/or CFZ derived clinical and in vitro isolates of Mtb are summarized in Figures 2, 3. Rv0678 RAVs enhanced the CFZ and BDQ MICs in murine isolates by 2- to 4-fold and 2- to 8-fold, respectively, and increased the BDQ MICs in clinical isolates by 2- to 16-fold (23, 131). Numerous studies have confirmed that CFZ exposure in the past promotes BDQ resistance linked to mutations in Rv0678 and pepQ genes (52, 54). On the other hand, a current study demonstrated that CFZ resistance developed following the sole administration of BDQ. Interestingly, while the other two cases lacked any known mutations linked to CFZ and BDQ resistance, one out of three cases harbored a genetic mutation at the Rv0678 locus (8). A recent study supported the potential of cross-resistance by demonstrating mutations L117R (T350G) and M146T (T437C) in Rv0678 and R409Q (G1226A) in Rv1979c in the strains Mtb resistant to both drugs BDQ and CFZ (86). The findings of the study suggest that more mechanism studies, i.e., whole genome sequencing, are needed to discover cross-resistance-related novel mechanisms in the BDQ and CFZ against Mtb strains (86). Significantly, clinical isolates rarely contain mutations in the BDQ target gene, atpE, and the majority of strains that exhibit phenotypic resistance to BDQ have mutations in the non-target Rv0678 gene. Reduced susceptibility to BDQ is linked to mutations in the Rv0678 and atpE genes, which have been found in both clinical isolates and strains that were chosen in vitro. There are few clinical studies reported to BDQ against Mtb strains that may be a possible reason to see mutations in laboratory BDQ-resistant Mtb strains in vitro that are very different from those isolated from mice or clinical isolates. Indeed, misdiagnosis can promote the spread of particular MDR Mtb strains through selection and subsequent transmission (92). Additionally, recent investigations showed that mutations in Rv0678 emerged that were linked to BDQ/CFZ resistance due to treatment failure and/or poor outcomes in MDR-TB patients.
14 Pharmacokinetics of BDQ
Compared to the data available for other anti-TB drugs, BDQ has fewer pharmacokinetics data published in the literature. Drug exposure in the case of BDQ is associated with body weight, age, race, albumin, and concurrent RIF use (143). The concentration of BDQ is significant for activity that has been shown to be concentration-based where the high BDQ concentrations were linked to a quicker reduction in mycobacterial load for patients within 24 weeks and culture conversion of sputum after 6-month treatment (87, 144). Therefore, it is crucial to know the optimal BDQ exposure for improvement of MDR-TB treatment. The recommended dosage of BDQ for adult patients is 400 mg daily for 2 weeks, then 200 mg three times a week for an additional 22 weeks (145). Surprisingly, drug susceptibility is a significant factor influencing the sputum culture conversion, as earlier stated (8). Of note, a current study by Shao et al. (146) demonstrated that the 24-h area under the curve (AUC0-24h/MIC) higher than 175.5 showed an increased probability of sputum culture conversion following a two-month therapy. In addition, after 6 months of treatment, those with AUC0-24h/MIC values higher than 118.2 demonstrated a higher likelihood of sputum culture conversion and AUC0-24 h/MIC higher than 74.6 showed a higher probability of a favorable outcome after treatment.
Importantly, the relative absorption of BDQ was found to increase around 2-fold when the drug is administered along with food compared to without food or fasting conditions, and consequently, it was advised to take it with food (147). The BDQ pharmacokinetic profile reveals that the maximal serum concentration (Cmax) is reached about 5 h after delivery, and the curative half-life is about 24 h following 2 weeks of 400 mg daily therapy. Therefore, it is advised to take BDQ with food. A very current study suggested that probability of target attainment (PTA) declines when patient body weight rises for both BDQ and PMD (148). An increase in BDQ dosage may not be practical for all individuals because the existing BDQ dose regimen is linked to safety hazards of the Fridericia-corrected QT (QTcF) prolongation and hepatic side effects. Further, bodyweight-based dose optimization for BDQ may be effective for assessing efficacy and safety.
15 Conclusion and perspectives
Our current review provides information on the efficacy of BDQ and CFZ alone and their combination use in the treatment of DR-/MDR-TB patients. The addition of BDQ and CFZ to the therapeutic TB regimens significantly improves results for MDR-TB patients. The use of BDQ and/or CFZ-containing regimens demonstrated low mortality with high culture conversion rates. Along with this, our review also supports and provides information regarding drug resistance as well as DST to BDQ and CFZ in in vitro and clinical Mtb strains. The second-line anti-TB drugs without DST lead to the potential for known and unknown drug resistance to the BDQ and CFZ. BDQ resistance is influenced by prior use of CFZ among CFZ-resistant patients. Drug resistance significantly reduces treatment efficiency, suggesting that phenotypic and genotypic tests are required before using BDQ and CFZ alone or their combination for the treatment of MDR-TB patients. The goal of TB drug development and discovery is to produce TB drugs and regimens that are better than those on the market today in terms of their accessibility, ease of use for all patient populations, efficacy, mechanisms of action and resistance. This review summarizes above all issues for BDQ and CFZ against Mtb strains, which may aid in the development and discovery of novel anti-TB drugs and even other drug combinations. TB control programs desperately need novel drugs that are as effective against MDR/XDR strains of Mtb while also having the advantage of being easier to administer and having shorter treatment duration. In the field of TB, the biochemical, target-driven approach to drug development has mainly been abandoned in favor of whole-cell or target based whole-cell screening methods. Additionally, this method produced a number of new, chemically verified targets that are currently useful for compound optimization based on targets. It is interesting to note that metabolism of energy and cell envelope biosynthesis seems to be heavily impacted by these novel anti-mycobacterial drugs, indicating that those metabolic regions are particularly susceptible or accessible. Therefore, an in-depth comprehension of the clinical efficacy, DST, mutations associated with individual resistance and cross-resistance, and pharmacokinetics of CFZ and BDQ against Mtb can offer fresh perspectives on how to enhance treatment outcomes, lower mortality, avoid drug resistance, and stop the spread of TB. Additionally, it will support the creation of quick molecular testing techniques and innovative TB drugs.
Author contributions
MI: Conceptualization, Investigation, Visualization, Writing – original draft. MA: Data curation, Writing – original draft. ZL: Data curation, Writing – original draft. MK: Data curation, Writing – original draft. BY: Data curation, Writing – original draft. HH: Investigation, Writing – original draft. XT: Investigation, Visualization, Writing – original draft. CC: Investigation, Visualization, Writing – original draft. RB: Writing – review & editing, Formal analysis, Resources. HA: Writing – review & editing, Formal analysis, Resources. XZ: Writing – review & editing, Formal analysis, Resources. SK: Writing – review & editing, Data curation, Investigation. CF: Writing – review & editing, Data curation, Investigation. CL: Writing – review & editing, Data curation, Investigation. SH: Writing – review & editing, Investigation. ST: Writing – review & editing, Investigation. NZ: Writing – review & editing, Investigation. JH: Writing – review & editing, Conceptualization, Funding acquisition, Project administration. TZ: Conceptualization, Funding acquisition, Project administration, Writing – review & editing.
Funding
The author(s) declare financial support was received for the research, authorship, and/or publication of this article. This work was funded by the National Key R&D Program of China (grant 2021YFA1300900), by the National Natural Science Foundation of China (21920102003), by the Guangzhou Science and Technology Planning Project (2023A03J0992), by the Key R&D Program of Sichuan Provenience (2023YFSY0047) and the State Key Lab of Respiratory Disease, Guangzhou Institute of Respiratory Diseases, First Affiliated Hospital of Guangzhou Medical University (grants SKLRD-OP-202113 and SKLRD-OP-202113).
Acknowledgments
We would like to thank Sayed Ala Moududee (School of Medicine, Tulane University, United States) for their kind advice and support on improving our manuscript.
Conflict of interest
The authors declare that the research was conducted in the absence of any commercial or financial relationships that could be construed as a potential conflict of interest.
The author(s) declared that they were an editorial board member of Frontiers, at the time of submission. This had no impact on the peer review process and the final decision.
Publisher’s note
All claims expressed in this article are solely those of the authors and do not necessarily represent those of their affiliated organizations, or those of the publisher, the editors and the reviewers. Any product that may be evaluated in this article, or claim that may be made by its manufacturer, is not guaranteed or endorsed by the publisher.
References
1. Bu, Q, Qiang, R, Fang, L, Peng, X, Zhang, H, and Cheng, H. Global trends in the incidence rates of MDR and XDR tuberculosis: findings from the global burden of disease study 2019. Front Pharmacol. (2023) 14:1156249. doi: 10.3389/fphar.2023.1156249
2. World Health Organization. Global tuberculosis report, 2022. Geneva, Switzerland: World Health Organization (2022). Available at: https://www.who.int/publications/i/item/9789240061729 (Accessed July 20, 2023).
3. World Health Organization. Global tuberculosis report, 2021. Geneva, Switzerland: World Health Organization (2021). https://www.who.int/publications/i/item/9789240037021 (Accessed July 21, 2023).
4. Migliori, GB, and Tiberi, SJ. WHO drug-resistant TB guidelines 2022: what is new. Int J Tuberc Lung Dis. (2022) 26:590–1. doi: 10.5588/ijtld.22.0263
5. Rich, ML, Khan, U, Zeng, C, LaHood, A, Franke, MF, Atwood, S, et al. Outcomes of WHO-conforming, longer, all-oral multidrug-resistant TB regimens and analysis implications. Int J Tuberc Lung Dis. (2023) 27:451–7. doi: 10.5588/ijtld.22.0613
6. World Health Organization. WHO consolidated guidelines on tuberculosis, module 4: Drug-resistant tuberculosis treatment. Geneva, Switzerland: World Health Organization (2020). Available at: https://www.who.int/publications/i/item/9789240007048 (Accessed July 25, 2023).
7. Stadler, JA, Maartens, G, Meintjes, G, and Wasserman, S. Clofazimine for the treatment of tuberculosis. Front Pharmacol. (2023) 14:1100488. doi: 10.3389/fphar.2023.1100488
8. Liu, Y, Gao, J, Du, J, Shu, W, Wang, L, Wang, Y, et al. Acquisition of clofazimine resistance following bedaquiline treatment for multidrug-resistant tuberculosis. Int J Infect Dis. (2021) 102:392–6. doi: 10.1016/j.ijid.2020.10.081
9. Zhang, S, Chen, J, Cui, P, Shi, W, Zhang, W, and Zhang, Y. Identification of novel mutations associated with clofazimine resistance in Mycobacterium tuberculosis. J Antimicrob Chemother. (2015) 70:2507–10. doi: 10.1093/jac/dkv150
10. Mase, S, Chorba, T, Parks, S, Belanger, A, Dworkin, F, Seaworth, B, et al. Bedaquiline for the treatment of multidrug-resistant tuberculosis in the United States. Clin Infect Dis. (2020) 71:1010–6. doi: 10.1093/cid/ciz914
11. Guglielmetti, L, Jaspard, M, Le Dû, D, Lachâtre, M, Marigot-Outtandy, D, Bernard, C, et al. Long-term outcome and safety of prolonged bedaquiline treatment for multidrug-resistant tuberculosis. Eur Respir J. (2017) 49:1601799. doi: 10.1183/13993003.01799-2016
12. Borisov, SE, Dheda, K, Enwerem, M, Leyet, RR, D'Ambrosio, L, Centis, R, et al. Effectiveness and safety of bedaquiline-containing regimens in the treatment of MDR-and XDR-TB: a multicentre study. Eur Respir J. (2017) 49:1700387. doi: 10.1183/13993003.00387-2017
13. van Dorp, L, Nimmo, C, Ortiz, AT, Pang, J, Acman, M, Tan, CC, et al. Detection of a bedaquiline/clofazimine resistance reservoir in Mycobacterium tuberculosis predating the antibiotic era. BioRxiv. (2020) 2020:799. doi: 10.1101/2020.10.06.328799
14. Olayanju, O, Limberis, J, Esmail, A, Oelofse, S, Gina, P, Pietersen, E, et al. Long-term bedaquiline-related treatment outcomes in patients with extensively drug-resistant tuberculosis from South Africa. Eur Respir J. (2018) 51:1800544. doi: 10.1183/13993003.00544-2018
15. Conradie, F, Diacon, AH, Ngubane, N, Howell, P, Everitt, D, Crook, AM, et al. Treatment of highly drug-resistant pulmonary tuberculosis. N Engl J Med. (2020) 382:893–902. doi: 10.1056/NEJMoa1901814
16. Degiacomi, G, Sammartino, JC, Sinigiani, V, Marra, P, Urbani, A, and Pasca, MR. In vitro study of bedaquiline resistance in Mycobacterium tuberculosis multi-drug resistant clinical isolates. Front Microbiol. (2020) 11:2290. doi: 10.3389/fmicb.2020.559469
17. Zimenkov, DV, Nosova, EY, Kulagina, EV, Antonova, OV, Arslanbaeva, LR, Isakova, AI, et al. Examination of bedaquiline- and linezolid-resistant Mycobacterium tuberculosis isolates from the Moscow region. J Antimicrob Chemother. (2017) 72:1901–6. doi: 10.1093/jac/dkx094
18. Ismail, N, Peters, RPH, Ismail, NA, and Omar, SV. Clofazimine exposure in vitro selects efflux pump mutants and bedaquiline resistance. Antimicrob Agents Chemother. (2019) 63:e02141–18. doi: 10.1128/aac.02141-18
19. Khoshnood, S, Goudarzi, M, Taki, E, Darbandi, A, Kouhsari, E, Heidary, M, et al. Bedaquiline: current status and future perspectives. J Glob Antimicrob Resist. (2021) 25:48–59. doi: 10.1016/j.jgar.2021.02.017
20. Nguyen, TVA, Anthony, RM, Banuls, AL, Nguyen, TVA, Vu, DH, and Alffenaar, JWC. Bedaquiline resistance: its emergence, mechanism, and prevention. Clin Infect Dis. (2018) 66:1625–30. doi: 10.1093/cid/cix992
21. Ismail, NA, Omar, SV, Joseph, L, Govender, N, Blows, L, Ismail, F, et al. Defining Bedaquiline susceptibility, resistance, cross-resistance and associated genetic determinants: a retrospective cohort study. EBioMedicine. (2018) 28:136–42. doi: 10.1016/j.ebiom.2018.01.005
22. Kabahita, JM, Kabugo, J, Kakooza, F, Adam, I, Guido, O, Byabajungu, H, et al. First report of whole-genome analysis of an extensively drug-resistant Mycobacterium tuberculosis clinical isolate with bedaquiline, linezolid and clofazimine resistance from Uganda. Antimicrob Resist Infect Control. (2022) 11:68. doi: 10.1186/s13756-022-01101-2
23. Villellas, C, Coeck, N, Meehan, CJ, Lounis, N, de Jong, B, Rigouts, L, et al. Unexpected high prevalence of resistance associated Rv0678 variants in MDR-TB patients without documented prior use of clofazimine or bedaquiline. J Antimicrob Chemother. (2017) 72:684–90. doi: 10.1093/jac/dkw502
24. Cholo, MC, Steel, HC, Fourie, PB, Germishuizen, WA, and Anderson, R. Clofazimine: current status and future prospects. J Antimicrob Chemother. (2012) 67:290–8. doi: 10.1093/jac/dkr444
25. Nugraha, RV, Yunivita, V, Santoso, P, Aarnoutse, RE, and Ruslami, R. Clofazimine as a treatment for multidrug-resistant tuberculosis: a review. Sci Pharma. (2021) 89:19. doi: 10.3390/scipharm89020019
26. Yang, B, Gao, Z, Li, QS, Zhang, XY, Song, L, Wang, YN, et al. Proteomic analysis and identification reveal the anti-inflammatory mechanism of clofazimine on lipopolysaccharide-induced acute lung injury in mice. Inflamm Res. (2022) 13:1–9. doi: 10.1007/s00011-022-01623-w
27. Padmapriyadarsini, C, Vohra, V, Bhatnagar, A, Solanki, R, Sridhar, R, Anande, L, et al. Bedaquiline, delamanid, linezolid, and clofazimine for treatment of pre-extensively drug-resistant tuberculosis. Clin Infect Dis. (2023) 76:e938–46. doi: 10.1093/cid/ciac528
28. Lange, C, Chesov, D, and Heyckendorf, J. Clofazimine for the treatment of multidrugresistant tuberculosis. Clin Microbiol Infect. (2019) 25:128–30. doi: 10.1016/j.cmi.2018.11.010
29. Xu, J, Lu, Y, Fu, L, Zhu, H, Wang, B, Mdluli, K, et al. In vitro and in vivo activity of clofazimine against Mycobacterium tuberculosis persisters. Int J Tuberc Lung Dis. (2012) 16:1119–25. doi: 10.5588/ijtld.11.0752
30. Yu, W, Chiwala, G, Gao, Y, Liu, Z, Sapkota, S, Lu, Z, et al. TB47 and clofazimine form a highly synergistic sterilizing block in a second-line regimen for tuberculosis in mice. Biomed Pharmacother. (2020) 131:110782. doi: 10.1016/j.biopha.2020.110782
31. Yu, W, Buhari, Y, Wang, S, Tian, X, Hameed, HMA, Lu, Z, et al. Sterilizing effects of novel regimens containing TB47, clofazimine and linezolid in a murine model of tuberculosis. Antimicrob Agents Chemother. (2021) 65:e00706–21. doi: 10.1128/aac.00706-21
32. Park, S, Jung, J, Kim, J, Han, SB, and Ryoo, S. Investigation of clofazimine resistance and genetic mutations in drug-resistant Mycobacterium tuberculosis isolates. J Clin Med. (2022) 11:1927. doi: 10.3390/jcm11071927
33. Van Deun, A, Maug, AK, Salim, MA, Das, PK, Sarker, MR, Daru, P, et al. Short, highly effective, and inexpensive standardized treatment of multidrug-resistant tuberculosis. Am J Respir Crit Care Med. (2010) 182:684–92. doi: 10.1164/rccm.201001-0077OC
34. Du, Y, Qiu, C, Chen, X, Wang, J, Jing, W, Pan, H, et al. Treatment outcome of a shorter regimen containing clofazimine for multidrug-resistant tuberculosis: a randomized control trial in China. Clin Infect Dis. (2020) 71:1047–54. doi: 10.1093/cid/ciz915
35. Mirnejad, R, Asadi, A, Khoshnood, S, Mirzaei, H, Heidary, M, Fattorini, L, et al. Clofazimine: A useful antibiotic for drug-resistant tuberculosis. Biomed Pharmacother. (2018) 105:1353–9. doi: 10.1016/j.biopha.2018.06.023
36. Lechartier, B, and Cole, ST. Mode of action of clofazimine and combination therapy with benzothiazinones against Mycobacterium tuberculosis. Antimicrob Agents Chemother. (2015) 59:4457–63. doi: 10.1128/AAC.00395-15
37. Cholo, MC, Mothiba, MT, Fourie, B, and Anderson, R. Mechanisms of action and therapeutic efficacies of the lipophilic antimycobacterial agents clofazimine and bedaquiline. J Antimicrob Chemother. (2017) 72:338–53. doi: 10.1093/jac/dkw426
38. Yano, T, Kassovska-Bratinova, S, Teh, JS, Winkler, J, Sullivan, K, Isaacs, A, et al. Reduction of clofazimine by mycobacterial type 2 NADH: quinone oxidoreductase: a pathway for the generation of bactericidal levels of reactive oxygen species. J Biol Chem. (2011) 286:10276–87. doi: 10.1074/jbc.M110.200501
39. Morrison, NE, and Marley, GM. The mode of action of clofazimine: DNA binding studies. Int J Lepr Other Mycobact Dis. (1976) 44:133–4.
40. Wan, W, Zhu, S, Li, S, Shang, W, Zhang, R, Li, H, et al. High-throughput screening of an FDA-approved drug library identifies inhibitors against arenaviruses and SARS-CoV-2. ACS Infect Dis. (2020) 7:1409–22. doi: 10.1021/acsinfecdis.0c00486
41. Yuan, S, Yin, X, Meng, X, Chan, J, Ye, ZW, Riva, L, et al. Clofazimine is a broad-spectrum coronavirus inhibitor that antagonizes SARS-CoV-2 replication in primary human cell culture and hamsters. Res Sq. (2020) 2020:169. doi: 10.21203/rs.3.rs-86169/v1
42. Ateya, AM. Clofazimine: a potential therapeutic option for severe COVID-19. Med Hypothese. (2021) 150:110535. doi: 10.1016/j.mehy.2021.110535
43. World Health Organization. Technical report on critical concentrations for drug susceptibility testing of medicines used in the treatment of drug-resistant tuberculosis. Geneva, Switzerland: World Health Organization (2018). Available at: http://apps.who.int/iris/handle/10665/260470 (Accessed July 26, 2023).
44. Singh, K, Sharma, S, Banerjee, T, Gupta, A, and Anupurba, S. Mutation detection and minimum inhibitory concentration determination against linezolid and clofazimine in confirmed XDR-TB clinical isolates. BMC Microbiol. (2022) 22:1–8. doi: 10.1186/s12866-022-02622-x
45. Monde, N, Munyeme, M, Chongwe, G, Wensman, JJ, Zulu, M, Siziya, S, et al. First and second-line anti-tuberculosis drug-resistance patterns in pulmonary tuberculosis patients in Zambia. Antibiotics. (2023) 12:166. doi: 10.3390/antibiotics12010166
46. Diacon, AH, Dawson, R, Von Groote-Bidlingmaier, F, Symons, G, Venter, A, Donald, PR, et al. Bactericidal activity of pyrazinamide and clofazimine alone and in combinations with pretomanid and bedaquiline. Am J Respir Crit Care Med. (2015) 191:943–53. doi: 10.1164/rccm.201410-1801OC
47. Cavanaugh, JS, Jou, R, Wu, MH, Dalton, T, Kurbatova, E, Ershova, J, et al. Investigators susceptibilities of MDR Mycobacterium tuberculosis isolates to unconventional drugs compared with their reported pharmacokinetic/pharmacodynamic parameters. J Antimicrob Chemother. (2017) 72:1678–87. doi: 10.1093/jac/dkx022
48. Pang, Y, Zong, Z, Huo, F, Jing, W, Ma, Y, Dong, L, et al. In vitro drug susceptibility of bedaquiline, delamanid, linezolid, clofazimine, moxifloxacin, and gatifloxacin against extensively drug-resistant tuberculosis in Beijing. China Antimicrob Agents Chemother. (2017) 61:e00900–17. doi: 10.1128/aac.00900-17
49. Puyén, ZM, Santos-Lázaro, D, Vigo, AN, Coronel, J, Alarcón, MJ, Cotrina, VV, et al. Evaluation of the broth microdilution plate methodology for susceptibility testing of Mycobacterium tuberculosis in Peru. BMC Infect Dis. (2022) 22:1. doi: 10.1186/s12879-022-07677-9
50. Shang, Y, Chen, S, Shi, W, Nie, W, Jing, W, Huo, F, et al. Bedaquiline resistance pattern in clofazimine-resistant clinical isolates of tuberculosis patients. J Glob Antimicrob Resist. (2023) 33:294–300. doi: 10.1016/j.jgar.2023.04.003
51. Bloemberg, GV, Keller, PM, and Stucki, D. Acquired resistance to bedaquiline and delamanid in therapy for tuberculosis. N Engl J Med. (2015) 373:1986–8. doi: 10.1056/NEJMc1505196
52. Hartkoorn, RC, Uplekar, S, and Cole, ST. Cross-resistance between clofazimine and bedaquiline through upregulation of MmpL5 in Mycobacterium tuberculosis. Antimicrob Agents Chemother. (2014) 58:2979–81. doi: 10.1128/AAC.00037-14
53. Martinez, E, Hennessy, D, Jelfs, P, Crighton, T, Chen, SCA, and Sintchenko, V. Mutations associated with in vitro resistance to bedaquiline in Mycobacterium tuberculosis isolates in Australia. Tuberculosis. (2018) 111:31–4. doi: 10.1016/j.tube.2018.04.007
54. Almeida, D, Ioerger, T, Tyagi, S, Li, SY, Mdluli, K, Andries, K, et al. Mutations in pepQ confer low-level resistance to bedaquiline and clofazimine in Mycobacterium tuberculosis. Antimicrob Agents Chemother. (2016) 60:4590–9. doi: 10.1128/AAC.00753-16
55. Hu, Y, Fan, J, Zhu, D, Liu, W, Li, F, Li, T, et al. Investigation of bedaquiline resistance and genetic mutations in multi-drug resistant Mycobacterium tuberculosis clinical isolates in Chongqing. China Ann Clin Microbiol Antimicrob. (2023) 22:19. doi: 10.1186/s12941-023-00568-0
56. Yang, J, Pang, Y, Zhang, T, Xian, X, Li, Y, Wang, R, et al. Molecular characteristics and in vitro susceptibility to bedaquiline of Mycobacterium tuberculosis isolates circulating in Shaanxi. China Int J Infect Dis. (2020) 99:163–70. doi: 10.1016/j.ijid.2020.07.044
57. Dey, T, Brigden, G, Cox, H, Shubber, Z, Cooke, G, and Ford, N. Outcomes of clofazimine for the treatment of drug-resistant tuberculosis: a systematic review and meta-analysis. J Antimicrob Chemother. (2013) 68:284–93. doi: 10.1093/jac/dks389
58. Gopal, M, Padayatchi, N, Metcalfe, JZ, and O'Donnell, MR. Systematic review of clofazimine for the treatment of drug-resistant tuberculosis. Int J Tuberc Lung Dis. (2013) 17:1001–7. doi: 10.5588/ijtld.12.0144
59. Aung, KJ, Van Deun, A, Declercq, E, Sarker, MR, Das, PK, Hossain, MA, et al. Successful ‘9-month Bangladesh regimen’for multidrug-resistant tuberculosis among over 500 consecutive patients. Int J Tuber Lung Dis. (2014) 18:1180–7. doi: 10.5588/ijtld.14.0100
60. Piubello, A, Harouna, SH, Souleymane, MB, Boukary, I, Morou, S, Daouda, M, et al. High cure rate with standardised short-course multidrug-resistant tuberculosis treatment in Niger: no relapses. Int J Tuberc Lung Dis. (2014) 18:1188–94. doi: 10.5588/ijtld.13.0075
61. Kuaban, C, Noeske, J, Rieder, HL, Aït-Khaled, N, Abena Foe, JL, and Trébucq, A. High effectiveness of a 12-month regimen for MDR-TB patients in Cameroon. Int J Tuberc Lung Dis. (2015) 19:517–24. doi: 10.5588/ijtld.14.0535
62. Tang, S, Yao, L, Hao, X, Liu, Y, Zeng, L, Liu, G, et al. Clofazimine for the treatment of multidrug-resistant tuberculosis: prospective, multicenter, randomized controlled study in China. Clin Infect Dis. (2015) 60:1361–7. doi: 10.1093/cid/civ027
63. Trébucq, A, Schwoebel, V, Kashongwe, Z, Bakayoko, A, Kuaban, C, Noeske, J, et al. Treatment outcome with a short MDR-TB regimen among patients with rifampicin-resistant TB in nine African countries. Int J Tuberc Lung Dis. (2018) 22:17–25. doi: 10.5588/ijtld.17.0498
64. Duan, H, Chen, X, Li, Z, Pang, Y, Jing, W, Liu, P, et al. Clofazimine improves clinical outcomes in multidrug-resistant tuberculosis: a randomized controlled trial. Clin Microbiol Infect. (2019) 25:190–5. doi: 10.1016/j.cmi.2018.07.012
65. Anh, LT, Kumar, MV, Ramaswamy, G, Htun, T, Thanh Hoang Thi, T, Hoai Nguyen, G, et al. High levels of treatment success and zero relapse in multidrug-resistant tuberculosis patients receiving a levofloxacin-based shorter treatment regimen in Vietnam. Trop Med Infect Dis. (2020) 5:43. doi: 10.3390/tropicalmed5010043
66. Hassane-Harouna, S, Cherif, GF, Ortuno-Gutierrez, N, Cisse, D, Camara, LM, Diallo, BD, et al. Better programmatic outcome with the shorter regimen for the treatment of multidrug-resistant tuberculosis (MDR-TB) in Guinea: a retrospective cohort study. PLoS One. (2020) 15:e0237355. doi: 10.1371/journal.pone.0237355
67. Ciza, F, Gils, T, Sawadogo, M, Decroo, T, Roggi, A, Piubello, A, et al. Course of adverse events during short treatment regimen in patients with rifampicin-resistant tuberculosis in Burundi. J Clin Med. (2020) 9:1873. doi: 10.3390/jcm9061873
68. Souleymane, MB, Piubello, A, Lawan, IM, Hassane-Harouna, S, Assao-Neino, MM, Soumana, A, et al. High rifampicin-resistant TB cure rates and prevention of severe ototoxicity after replacing the injectable by linezolid in early stage of hearing loss. Eur Respir J. (2021) 57:2002250. doi: 10.1183/13993003.02250-2020
69. Trubnikov, A, Hovhannesyan, A, Akopyan, K, Ciobanu, A, Sadirova, D, Kalandarova, L, et al. Effectiveness and safety of a shorter treatment regimen in a setting with a high burden of multidrug-resistant tuberculosis. Int J Environ Res Public Health. (2021) 18:4121. doi: 10.3390/ijerph18084121
70. du Cros, P, Khamraev, A, Tigay, Z, Abdrasuliev, T, Greig, J, Cooke, G, et al. Outcomes with a shorter multidrug-resistant tuberculosis regimen from Karakalpakstan. Uzbekistan ERJ Open Res. (2021) 7:00537–2020. doi: 10.1183/23120541.00537-2020
71. Yao, G, Zhu, M, Nie, Q, Chen, N, Tu, S, Zhou, Y, et al. Improved outcomes following addition of bedaquiline and clofazimine to a treatment regimen for multidrug-resistant tuberculosis. J Int Med Res. (2023) 51:8416. doi: 10.1177/03000605221148416
72. Schwœbel, V, Trébucq, A, Kashongwe, Z, Bakayoko, AS, Kuaban, C, Noeske, J, et al. Outcomes of a nine-month regimen for rifampicin-resistant tuberculosis up to 24 months after treatment completion in nine African countries. EClinicalMedicine. (2020) 20:100268. doi: 10.1016/j.eclinm.2020.100268
73. Wang, MG, Liu, XM, Wu, SQ, and He, JQ. Impacts of clofazimine on the treatment outcomes of drug-resistant tuberculosis. Microbes Infect. (2023) 25:105020. doi: 10.1016/j.micinf.2022.105020
74. Ahmad, N, Ahuja, SD, Akkerman, OW, Alffenaar, JW, Anderson, LF, Baghaei, P, et al. Treatment correlates of successful outcomes in pulmonary multidrug-resistant tuberculosis: an individual patient data meta-analysis. Lancet. (2018) 392:821–34. doi: 10.1016/S0140-6736(18)31644-1
75. Van Deun, A, Decroo, T, Kuaban, C, Noeske, J, Piubello, A, Aung, KJ, et al. Gatifloxacin is superior to levofloxacin and moxifloxacin in shorter treatment regimens for multidrug-resistant TB. Int J Tuberc Lung Dis. (2019) 23:965–71. doi: 10.5588/ijtld.19.0053
76. Trébucq, A, Decroo, T, Van Deun, A, Piubello, A, Chiang, CY, Koura, KG, et al. Short-course regimen for multidrug-resistant tuberculosis: a decade of evidence. J Clin Med. (2020) 9:55. doi: 10.3390/jcm9010055
77. Chiang, CY, Trebucq, A, Piubello, A, Rieder, HL, and Van Deun, A. Should gatifloxacin be included in the model list of essential medicines? (correspondence). Eur Respir J. (2018) 51:1702329. doi: 10.1183/13993003.02329-2017
78. Lee, EH, Yong, SH, Leem, AY, Lee, SH, Kim, SY, Chung, KS, et al. Improved fluoroquinolone-resistant and extensively drug-resistant tuberculosis treatment outcomes. Open forum. Infect Dis. (2019) 6:ofz 118. doi: 10.1093/ofid/ofz118
79. Piubello, A, Souleymane, MB, Hassane-Harouna, S, Yacouba, A, Lempens, P, Assao-Neino, MM, et al. Management of multidrug-resistant tuberculosis with shorter treatment regimen in Niger: nationwide programmatic achievements. Respir Med. (2020) 161:105844. doi: 10.1016/j.rmed.2019.105844
80. Wrohan, I, Redwood, L, Ho, J, Velen, K, and Fox, GJ. Ototoxicity among multidrug-resistant TB patients: a systematic review and meta-analysis. Int J Tuberc Lung Dis. (2021) 25:23–30. doi: 10.5588/ijtld.20.0217
81. Zhao, Y, Fox, T, Manning, K, Stewart, A, Tiffin, N, Khomo, N, et al. Improved treatment outcomes with bedaquiline when substituted for second-line injectable agents in multidrug-resistant tuberculosis: a retrospective cohort study. Clin Infect Dis. (2019) 68:1522–9. doi: 10.1093/cid/ciy727
82. Wang, Q, Pang, Y, Jing, W, Liu, Y, Wang, N, Yin, H, et al. Clofazimine for treatment of extensively drug-resistant pulmonary tuberculosis in China. Antimicrob Agents Chemother. (2018) 62:10–128. doi: 10.1128/aac.02149-17
83. Padayatchi, N, Gopal, M, Naidoo, R, Werner, L, Naidoo, K, Master, I, et al. Clofazimine in the treatment of extensively drug-resistant tuberculosis with HIV coinfection in South Africa: a retrospective cohort study. J Antimicrob Chemother. (2014) 69:3103–7. doi: 10.1093/jac/dku235
84. Islam, MM, Hameed, HA, Mugweru, J, Chhotaray, C, Wang, C, Tan, Y, et al. Drug resistance mechanisms and novel drug targets for tuberculosis therapy. J Genet Genomics. (2017) 44:21–37. doi: 10.1016/j.jgg.2016.10.002
85. Li, Y, Fu, L, Zhang, W, Chen, X, and Lu, Y. The transcription factor Rv1453 regulates the expression of qor and confers resistant to clofazimine in Mycobacterium tuberculosis. Infect Drug Resist. (2021) 14:3937–48. doi: 10.2147/IDR.S324043
86. Trisakul, K, Nonghanphithak, D, Chaiyachat, P, Kaewprasert, O, Sakmongkoljit, K, Reechaipichitkul, W, et al. High clustering rate and genotypic drug-susceptibility screening for the newly recommended anti-tuberculosis drugs among global extensively drug-resistant Mycobacterium tuberculosis isolates. Emerg Microbes Infect. (2022) 11:1857–66. doi: 10.1080/22221751.2022.2099304
87. Zheng, H, He, W, Jiao, W, Xia, H, Sun, L, Wang, S, et al. Molecular characterization of multidrug-resistant tuberculosis against levofloxacin, moxifloxacin, bedaquiline, linezolid, clofazimine, and delamanid in southwest of China. BMC Infect Dis. (2021) 21:330. doi: 10.1186/s12879-021-06024-8
88. Chen, X, He, G, Wang, S, Lin, S, Chen, J, and Zhang, W. Evaluation of whole-genome sequence method to diagnose resistance of 13 anti-tuberculosis drugs and characterize resistance genes in clinical multi-drug resistance Mycobacterium tuberculosis isolates from China. Front Microbiol. (2019) 10:1741. doi: 10.3389/fmicb.2019.01741
89. Ismail, N, Rivière, E, Limberis, J, Huo, S, Metcalfe, JZ, Warren, RM, et al. Genetic variants and their association with phenotypic resistance to bedaquiline in Mycobacterium tuberculosis: a systematic review and individual isolate data analysis. Lancet. Microbe. (2021) 2:e604–ee16. doi: 10.1016/S2666-5247(21)00175-0
90. Saeed, DK, Shakoor, S, Razzak, SA, Hasan, Z, Sabzwari, SF, Azizullah, Z, et al. Variants associated with Bedaquiline (BDQ) resistance identified in Rv0678 and efflux pump genes in Mycobacterium tuberculosis isolates from BDQ naïve TB patients in Pakistan. BMC Microbiol. (2022) 22:1–2. doi: 10.1186/s12866-022-02475-4
91. Snobre, J, Villellas, MC, Coeck, N, Mulders, W, Tzfadia, O, de Jong, BC, et al. Bedaquiline-and clofazimine-selected Mycobacterium tuberculosis mutants: further insights on resistance driven largely by Rv0678. Sci Rep. (2023) 13:10444. doi: 10.1038/s41598-023-36955-y
92. Sonnenkalb, L, Carter, JJ, Spitaleri, A, Iqbal, Z, Hunt, M, Malone, KM, et al. Bedaquiline and clofazimine resistance in Mycobacterium tuberculosis: an in-vitro and in-silico data analysis. Lancet Microbe. (2023) 4:e358–68. doi: 10.1016/S2666-5247(23)00002-2
93. Guo, Q, Bi, J, Lin, Q, Ye, T, Wang, Z, Wang, Z, et al. Whole genome sequencing identifies novel mutations associated with bedaquiline resistance in Mycobacterium tuberculosis. Front Cell Infect Microbiol. (2022) 12:807095. doi: 10.3389/fcimb.2022.807095
94. Prideaux, B, Via, LE, Zimmerman, MD, Eum, S, Sarathy, J, O’brien, P, et al. The association between sterilizing activity and drug distribution into tuberculosis lesions. Nat Med. (2015) 21:1223–7. doi: 10.1038/nm.3937
95. Baijnath, S, Naiker, S, Shobo, A, Moodley, C, Adamson, J, Ngcobo, B, et al. Evidence for the presence of clofazimine and its distribution in the healthy mouse brain. J Mol Histol. (2015) 46:439–42. doi: 10.1007/s10735-015-9634-3
96. Swanson, RV, Adamson, J, Moodley, C, Ngcobo, B, Ammerman, NC, Dorasamy, A, et al. Pharmacokinetics and pharmacodynamics of clofazimine in a mouse model of tuberculosis. Antimicrob Agents Chemother. (2015) 59:3042–51. doi: 10.1128/AAC.00260-15
97. Abdelwahab, MT, Wasserman, S, Brust, JC, Gandhi, NR, Meintjes, G, Everitt, D, et al. Clofazimine pharmacokinetics in patients with TB: dosing implications. J Antimicrob Chemother. (2020) 75:3269–77. doi: 10.1093/jac/dkaa310
98. Alghamdi, WA, Al-Shaer, MH, Kipiani, M, Barbakadze, K, Mikiashvili, L, Kempker, RR, et al. Pharmacokinetics of bedaquiline, delamanid and clofazimine in patients with multidrug-resistant tuberculosis. J Antimicrob Chemother. (2021) 76:1019–24. doi: 10.1093/jac/dkaa550
99. Nix, DE, Adam, RD, Auclair, B, Krueger, TS, Godo, PG, and Peloquin, CA. Pharmacokinetics and relative bioavailability of clofazimine in relation to food, orange juice and antacid. Tuberculosis. (2004) 84:365–73. doi: 10.1016/j.tube.2004.04.001
100. Wallis, RS. Cardiac safety of extensively drug-resistant tuberculosis regimens including bedaquiline, delamanid and clofazimine. Eur Respir J. (2016) 48:1526–7. doi: 10.1183/13993003.01207-2016
101. Meid, AD, Bighelli, I, Mächler, S, Mikus, G, Carrà, G, Castellazzi, M, et al. Combinations of QTc-prolonging drugs: towards disentangling pharmacokinetic and pharmacodynamic effects in their potentially additive nature. Ther Adv Psychopharmacol. (2017) 7:251–64. doi: 10.1177/2045125317721662
102. Esmail, A, Oelofse, S, Lombard, C, Perumal, R, Mbuthini, L, Goolam Mahomed, A, et al. An all-oral 6-month regimen for multidrug-resistant tuberculosis: a multicenter, randomized controlled trial (the NExT study). Am J Respir Crit Care Med. (2022) 205:1214–27. doi: 10.1164/rccm.202107-1779OC
103. Lyons, MA. Pharmacodynamics and bactericidal activity of bedaquiline in pulmonary tuberculosis. Antimicrob Agents Chemother. (2022) 66:e0163621. doi: 10.1128/AAC.01636-21
104. Zharova, TV, Grivennikova, VG, and Borisov, VB. F1·Fo ATP synthase/ATPase: contemporary view on unidirectional catalysis. Int J Mol Sci. (2023) 24:5417. doi: 10.3390/ijms24065417
105. Koul, A, Dendouga, N, Vergauwen, K, Molenberghs, B, Vranckx, L, Willebrords, R, et al. Diarylquinolines target subunit c of mycobacterial ATP synthase. Nat Chem Biol. (2007) 3:323–4. doi: 10.1038/nchembio884
106. Biukovic, G, Basak, S, Manimekalai, MS, Rishikesan, S, Roessle, M, Dick, T, et al. Variations of subunit of the Mycobacterium tuberculosis F1Fo ATP synthase and a novel model for mechanism of action of the tuberculosis drug TMC207. Antimicrob Agents Chemother. (2013) 57:168–76. doi: 10.1128/AAC.01039-12
107. Viney, K, Linh, NN, Gegia, M, Zignol, M, Glaziou, P, Ismail, N, et al. New definitions of pre-extensively and extensively drug-resistant tuberculosis: update from the World Health Organization. Eur Respir J. (2021) 57:2100361. doi: 10.1183/13993003.00361-2021
108. Kaniga, K, Cirillo, DM, Hoffner, S, Ismail, NA, Kaur, D, Lounis, N, et al. A multilaboratory, multicountry study to determine bedaquiline MIC quality control ranges for phenotypic drug susceptibility testing. J Clin Microbiol. (2016) 54:2956–62. doi: 10.1128/JCM.01123-16
109. Keller, PM, Homke, R, Ritter, C, Valsesia, G, Bloemberg, GV, and Bottger, EC. Determination of MIC distribution and epidemiological cutoff values for bedaquiline and delamanid in Mycobacterium tuberculosis using the MGIT 960 system equipped with TB eXiST. Antimicrob Agents Chemother. (2015) 59:4352–5. doi: 10.1128/AAC.00614-15
110. Torrea, G, Coeck, N, Desmaretz, C, Van De Parre, T, Van Poucke, T, et al. Bedaquiline susceptibility testing of Mycobacterium tuberculosis in an automated liquid culture system. J Antimicrob Chemother. (2015) 70:2300–5. doi: 10.1093/jac/dkv117
111. Nieto Ramirez, LM, Quintero Vargas, K, and Diaz, G. Whole genome sequencing for the analysis of drug resistant strains of Mycobacterium tuberculosis: a systematic review for bedaquiline and delamanid. Antibiotics. (2020) 9:133. doi: 10.3390/antibiotics9030133
112. Ismail, NA, Aono, A, Borroni, E, Cirillo, DM, Desmaretz, C, Hasan, R, et al. A multimethod, multicountry evaluation of breakpoints for bedaquiline resistance determination. Antimicrob Agents Chemother. (2020) 64:e00479–20. doi: 10.1128/aac.00479-20
113. Hewison, C, Bastard, M, Khachatryan, N, Kotrikadze, T, Hayrapetyan, A, Avaliani, Z, et al. Is 6 months of bedaquiline enough? Results from the compassionate use of bedaquiline in Armenia and Georgia. Int J Tuberc Lung Dis. (2018) 22:766–72. doi: 10.5588/ijtld.17.0840
114. Ndjeka, N, Schnippel, K, Master, I, Meintjes, G, Maartens, G, Romero, R, et al. High treatment success rate for multidrug-resistant and extensively drug-resistant tuberculosis using a bedaquiline-containing treatment regimen. Eur Respir J. (2018) 52:1801528. doi: 10.1183/13993003.01528-2018
115. Padayatchi, N, Bionghi, N, Osman, F, Naidu, N, Ndjeka, N, Master, I, et al. Treatment outcomes in patients with drugresistant TB-HIV co-infection treated with bedaquiline and linezolid. Int J Tuberc Lung Dis. (2020) 24:1024–31. doi: 10.5588/ijtld.20.0048
116. Kashongwe, IM, Mawete, F, Anshambi, N, Maingowa, N, Aloni, M, L'osenga, LL, et al. Challenge to treat pre-extensively drug-resistant tuberculosis in a low-income country: a report of 12 cases. J Clin Tuberc Other Mycobact Dis. (2020) 21:100192. doi: 10.1016/j.jctube.2020.100192
117. Shi, L, Gao, J, Gao, M, Deng, P, Chen, S, He, M, et al. Interim effectiveness and safety comparison of Bedaquiline-containing regimens for treatment of diabetic versus non-diabetic MDR/XDR-TB patients in China: a multicenter retrospective cohort study. Infect Dis Ther. (2021) 10:457–70. doi: 10.1007/s40121-021-00396-9
118. Li, J, Yang, G, Cai, Q, Wang, Y, Xu, Y, Zhang, R, et al. Safety, efficacy, and serum concentration monitoring of bedaquiline in Chinese patients with multidrug-resistant tuberculosis. Int J Infect Dis. (2021) 110:179–86. doi: 10.1016/j.ijid.2021.07.038
119. Koirala, S, Borisov, S, Danila, E, Mariandyshev, A, Shrestha, B, Lukhele, N, et al. Outcome of treatment of MDR-TB or drug-resistant patients treated with bedaquiline and delamanid: results from a large global cohort. Pulmonology. (2021) 27:403–12. doi: 10.1016/j.pulmoe.2021.02.006
120. Ndjeka, N, Campbell, JR, Meintjes, G, Maartens, G, Schaaf, HS, Hughes, J, et al. Treatment outcomes 24 months after initiating short, all-oral bedaquiline-containing or injectable-containing rifampicin-resistant tuberculosis treatment regimens in South Africa: a retrospective cohort study. Lancet Infect Dis. (2022) 22:1042–51. doi: 10.1016/S1473-3099(21)00811-2
121. Zhang, SJ, Yang, Y, Sun, WW, Zhang, ZS, Xiao, HP, Li, YP, et al. Effectiveness and safety of bedaquiline-containing regimens for treatment on patients with refractory RR/MDR/XDR-tuberculosis: a retrospective cohort study in East China. BMC Infect Dis. (2022) 22:1–2. doi: 10.1186/s12879-022-07693-9
122. Fadeyi, MO, Decroo, T, Ortuño-Gutiérrez, N, Ahmed, B, Jinadu, A, El-Tayeb, O, et al. A four-drug standardized short regimen for highly resistant TB in south-West Nigeria. Int. Health. (2023) 2023:23. doi: 10.1093/inthealth/ihad023
123. Li, SY, Tasneen, R, Tyagi, S, Soni, H, Converse, PJ, Mdluli, K, et al. Bactericidal and sterilizing activity of a novel regimen with bedaquiline, pretomanid, moxifloxacin, and pyrazinamide in a murine model of tuberculosis. Antimicrob Agents Chemother. (2017) 61:10–128. doi: 10.1128/aac.00913-17
124. Tasneen, R, Garcia, A, Converse, PJ, Zimmerman, MD, Dartois, V, Kurbatova, E, et al. Novel regimens of bedaquiline-pyrazinamide combined with moxifloxacin, rifabutin, delamanid and/or OPC-167832 in murine tuberculosis models. Antimicrob Agents Chemother. (2022) 66:e02398–21. doi: 10.1128/aac.02398-21
125. Gao, M, Gao, J, Xie, L, Wu, G, Chen, W, Chen, Y, et al. Early outcome and safety of bedaquiline-containing regimens for treatment of MDR-and XDR-TB in China: a multicentre study. Clin Microbiol Infect. (2021) 27:597–602. doi: 10.1016/j.cmi.2020.06.004
126. van Heeswijk, RP, Dannemann, B, and Hoetelmans, RM. Bedaquiline: a review of human pharmacokinetics and drug-drug interactions. J Antimicrob Chemother. (2014) 69:2310–8. doi: 10.1093/jac/dku171
127. Mbuagbaw, L, Guglielmetti, L, Hewison, C, Bakare, N, Bastard, M, Caumes, E, et al. Outcomes of bedaquiline treatment in patients with multidrugresistant tuberculosis. Emerg Infect Dis. (2019) 25:936–43. doi: 10.3201/eid2505.181823
128. Andries, K, Verhasselt, P, Guillemont, J, Gohlmann, HW, Neefs, JM, Winkler, H, et al. A diarylquinoline drug active on the ATP synthase of Mycobacterium tuberculosis. Science. (2005) 307:223–7. doi: 10.1126/science.1106753
129. Hameed, HA, Islam, MM, Chhotaray, C, Wang, C, Liu, Y, Tan, Y, et al. Molecular targets related drug resistance mechanisms in MDR-, XDR-, and TDR-Mycobacterium tuberculosis strains. Front Cell Infect Microbiol. (2018) 8:114. doi: 10.3389/fcimb.2018.00114
130. Kadura, S, King, N, Nakhoul, M, Zhu, H, Theron, G, Köser, CU, et al. Systematic review of mutations associated with resistance to the new and repurposed Mycobacterium tuberculosis drugs bedaquiline, clofazimine, linezolid, delamanid and pretomanid. J Antimicrob Chemother. (2020) 75:2031–43. doi: 10.1093/jac/dkaa136
131. Andries, K, Villellas, C, Coeck, N, Thys, K, Gevers, T, Vranckx, L, et al. Acquired resistance of Mycobacterium tuberculosis to bedaquiline. PLoS One. (2014) 9:e102135. doi: 10.1371/journal.pone.0102135
132. Ghajavand, H, Kargarpour Kamakoli, M, Khanipour, S, Pourazar Dizaji, S, Masoumi, M, Rahimi Jamnani, F, et al. High prevalence of bedaquiline resistance in treatment-naive tuberculosis patients and verapamil effectiveness. Antimicrob Agents Chemother. (2019) 63:e02530–18. doi: 10.1128/aac.02530-18
133. Andres, S, Merker, M, Heyckendorf, J, Kalsdorf, B, Rumetshofer, R, Indra, A, et al. Bedaquiline-resistant tuberculosis: dark clouds on the horizon. Am J Respir Crit Care Med. (2020) 201:1564–8. doi: 10.1164/rccm.201909-1819LE
134. Larrouy-Maumus, G, Biswas, T, Hunt, DM, Kelly, G, Tsodikov, OV, et al. Discovery of a glycerol 3-phosphate phosphatase reveals glycerophospholipid polar head recycling in Mycobacterium tuberculosis. Proc Natl Acad Sci U S A. (2013) 110:11320–5. doi: 10.1073/pnas.1221597110
135. Whitaker, M, Ruecker, N, Hartman, T, Klevorn, T, Andres, J, Kim, J, et al. Two interacting ATPases protect Mycobacterium tuberculosis from glycerol and nitric oxide toxicity. J Bacteriol. (2020) 202:10–128. doi: 10.1128/jb.00202-20
136. Ehrt, S, and Rhee, K. Mycobacterium tuberculosis metabolism and host interaction: mysteries and paradoxes In: J Pieters and J McKinney, editors. Pathogenesis of Mycobacterium tuberculosis and its interaction with the host organism. Berlin, Heidelberg: Springer (2012). 163–88.
137. Beckert, P, Sanchez-Padilla, E, Merker, M, Dreyer, V, Kohl, TA, Utpatel, C, et al. MDR M. tuberculosis outbreak clone in Eswatini missed by Xpert has elevated 611 bedaquiline resistance dated to the pre-treatment era. Genome Med. (2020) 12:1. doi: 10.1186/s13073-020-00793-8
138. Nimmo, C, Millard, J, Brien, K, Moodley, S, van Dorp, L, Lutchminarain, K, et al. Bedaquiline resistance in drug-resistant tuberculosis HIV co-infected patients. Eur Respir J. (2020) 55:1902383. doi: 10.1183/13993003.02383-2019
139. Sonnenkalb, L, Carter, J, Spitaleri, A, Iqbal, Z, Hunt, M, Malone, K, et al. Deciphering bedaquiline and clofazimine resistance in tuberculosis: an evolutionary medicine approach. Biorxiv. (2021) 2021:2021–03. doi: 10.1101/2021.03.19.436148
140. Ghodousi, A, Rizvi, AH, Khanzada, FM, Akhtar, N, Ghafoor, A, Trovato, A, et al. In vivo microevolution of Mycobacterium tuberculosis and transient emergence of atpE_Ala63Pro mutation during treatment in a pre-XDR TB patient. Eur Respir J. (2022) 59:2102102. doi: 10.1183/13993003.02102-2021
141. Ismail, NA, Omar, SV, Moultrie, H, Bhyat, Z, Conradie, F, Enwerem, M, et al. Assessment of epidemiological and genetic characteristics and clinical outcomes of resistance to bedaquiline in patients treated for rifampicin-resistant tuberculosis: a cross-sectional and longitudinal study. Lancet Infect Dis. (2022) 22:496–506. doi: 10.1016/S1473-3099(21)00470-9
142. Ghodousi, A, Rizvi, AH, Baloch, AQ, Ghafoor, A, Khanzada, FM, Qadir, M, et al. Acquisition of cross-resistance to bedaquiline and clofazimine following treatment for tuberculosis in Pakistan. Antimicrob Agents Chemother. (2019) 63:10–128. doi: 10.1128/aac.00915-19
143. Svensson, EM, Dosne, AG, and Karlsson, MO. Population pharmacokinetics of bedaquiline and metabolite M2 in patients with drug-resistant tuberculosis: the effect of time-varying weight and albumin. CPT Pharmacometrics Syst Pharmacol. (2016) 5:682–91. doi: 10.1002/psp4.12147
144. Tanneau, L, Karlsson, MO, and Svensson, EM. Understanding the drug exposure–response relationship of bedaquiline to predict efficacy for novel dosing regimens in the treatment of multidrug-resistant tuberculosis. Br J Clin Pharmacol. (2020) 86:913–22. doi: 10.1111/bcp.14199
145. Zou, J, Chen, S, Rao, W, Fu, L, Zhang, J, Liao, Y, et al. Population pharmacokinetic modeling of bedaquiline among multidrug-resistant pulmonary tuberculosis patients from China. Antimicrob Agents Chemother. (2022) 66:e00811–22. doi: 10.1128/aac.00811-22
146. Shao, G, Bao, Z, Davies Forsman, L, Paues, J, Werngren, J, Niward, K, et al. Population pharmacokinetics and model-based dosing evaluation of bedaquiline in multidrug-resistant tuberculosis patients. Front Pharmacol. (2023) 14:1022090. doi: 10.3389/fphar.2023.1022090
147. McLeay, SC, Vis, P, Van Heeswijk, RP, and Green, B. Population pharmacokinetics of bedaquiline (TMC207), a novel antituberculosis drug. Antimicrob Agents Chemother. (2014) 58:5315–24. doi: 10.1128/AAC.01418-13
148. Mehta, K, Guo, T, Van der Graaf, PH, and Van Hasselt, JC. Predictions of bedaquiline and pretomanid target attainment in lung lesions of tuberculosis patients using translational minimal physiologically based pharmacokinetic modeling. Clin Pharmacokinet. (2023) 62:519–32. doi: 10.1007/s40262-023-01217-7
Keywords: cross-resistance, drug resistance, mechanism of action, molecular diagnostic, regimens, treatment
Citation: Islam MM, Alam MS, Liu Z, Khatun MS, Yusuf B, Hameed HMA, Tian X, Chhotaray C, Basnet R, Abraha H, Zhang X, Khan SA, Fang C, Li C, Hasan S, Tan S, Zhong N, Hu J and Zhang T (2024) Molecular mechanisms of resistance and treatment efficacy of clofazimine and bedaquiline against Mycobacterium tuberculosis. Front. Med. 10:1304857. doi: 10.3389/fmed.2023.1304857
Edited by:
Lei Ji, Hangzhou Medical College, ChinaReviewed by:
Guoliang Zhang, Shenzhen Third People’s Hospital, ChinaYoufu Luo, Sichuan University, China
Copyright © 2024 Islam, Alam, Liu, Khatun, Yusuf, Hameed, Tian, Chhotaray, Basnet, Abraha, Zhang, Khan, Fang, Li, Hasan, Tan, Zhong, Hu and Zhang. This is an open-access article distributed under the terms of the Creative Commons Attribution License (CC BY). The use, distribution or reproduction in other forums is permitted, provided the original author(s) and the copyright owner(s) are credited and that the original publication in this journal is cited, in accordance with accepted academic practice. No use, distribution or reproduction is permitted which does not comply with these terms.
*Correspondence: Jinxing Hu, aHVqaW54aW5nMjAwMEAxNjMuY29t; Tianyu Zhang, emhhbmdfdGlhbnl1QGdpYmguYWMuY24=