- 1Department of Radiology, University of Texas Southwestern Medical Center, Dallas, TX, United States
- 2Advanced Imaging Research Center, University of Texas Southwestern Medical Center, Dallas, TX, United States
- 3Section of Hematologic Malignancies/Transplant and Cell Therapy, Division of Hematology-Oncology, University of Texas Southwestern Medical Center, Dallas, TX, United States
Chimeric antigen receptor (CAR) T-cell therapies have evolved as breakthrough treatment options for the management of hematological malignancies and are also being developed as therapeutics for solid tumors. However, despite the impressive patient responses from CD19-directed CAR T-cell therapies, ~ 40%−60% of these patients' cancers eventually relapse, with variable prognosis. Such relapses may occur due to a combination of molecular resistance mechanisms, including antigen loss or mutations, T-cell exhaustion, and progression of the immunosuppressive tumor microenvironment. This class of therapeutics is also associated with certain unique toxicities, such as cytokine release syndrome, immune effector cell-associated neurotoxicity syndrome, and other “on-target, off-tumor” toxicities, as well as anaphylactic effects. Furthermore, manufacturing limitations and challenges associated with solid tumor infiltration have delayed extensive applications. The molecular imaging modalities of immunological positron emission tomography and single-photon emission computed tomography (immuno-PET/-SPECT) offer a target-specific and highly sensitive, quantitative, non-invasive platform for longitudinal detection of dynamic variations in target antigen expression in the body. Leveraging these imaging strategies as guidance tools for use with CAR T-cell therapies may enable the timely identification of resistance mechanisms and/or toxic events when they occur, permitting effective therapeutic interventions. In addition, the utilization of these approaches in tracking the CAR T-cell pharmacokinetics during product development and optimization may help to assess their efficacy and accordingly to predict treatment outcomes. In this review, we focus on current challenges and potential opportunities in the application of immuno-PET/-SPECT imaging strategies to address the challenges encountered with CAR T-cell therapies.
Introduction
In recent years, strategies leveraging the human immuno-surveillance system to achieve complete remission of cancer have revolutionized the landscape of cancer immunotherapy. For instance, cytotoxic lymphocytes and natural killer cells, key players in the armor of the human immune system, have been employed to mediate immune surveillance. This approach takes advantage of their antitumor effector functions via distinct mechanisms, including (a) granule exocytosis resulting in the release of perforin and granule-associated enzymes (granzymes) and (b) release of exosomes containing Fas ligand (FasL) and tumor necrosis factor (TNF)-related apoptosis-inducing ligand (TRAIL), leading to the predominantly programmed apoptotic tumor cell death process (1). Currently, a solid foundation has been laid by the successes of T-cell-based cancer therapies in patients with metastatic cancers (2–4) as well as in patients with malignancies that have relapsed after, or were refractory to, the initial conventional treatments (5, 6). The redesign of a patient's own tumor-infiltrating lymphocytes (TILs) as cancer therapeutics, termed “adoptive cell therapy,” has garnered increasing interest in the field of immunotherapy since the 1960s (7). Efforts over the past three decades in adoptive T-cell therapy have resulted in the establishment of three types of cell therapies, namely, TIL therapies, engineered T-cell receptor (TCR) therapies, and chimeric antigen receptor (CAR) T-cell therapies (8, 9). In general, TIL cell therapies have achieved success in the effective management of melanomas (10), although the time required for donor cell expansion is a limiting factor. Moreover, factors such as T-cell exhaustion and T-cell dysfunction in the donor samples/starting material significantly impact the quality of the final products. More recently, the field has evolved with the sophisticated genetic engineering of peripheral T lymphocytes. This approach enables the design of superior antigen-targeted CAR T-cell therapies capable of effective tumor antigen binding for T-cell activation and proliferation independent of the major histocompatibility complex (MHC). Notably, the introduction of co-stimulatory domains in second-generation CAR T-cell therapy has enabled improved T-cell proliferation and persistence. Due to its high specificity toward a broader spectrum of membrane-expressed targets, CAR T-cell therapies have undergone significant translational development for treating cancers beyond B-cell malignancies. This has also prompted pharmaceutical companies to proceed toward commercialization of these therapies (11, 12).
In a Phase II single-cohort, multi-center global trial, the investigational Cluster of Differentiation 19 (CD19)-directed CAR T-cell therapeutic CTL019 (Tisagenlecleucel, Kymriah®, Novartis) demonstrated high response rates with an overall remission rate of 81% within 3 months and overall survival of 76% at 12 months in pediatric and young adult patients with relapsed/refractory (R/R) B-cell acute lymphoblastic leukemia (B-ALL) (13). This treatment received “breakthrough therapy status” and approval from the United States Food and Drug Administration (US-FDA) in 2017 for treating adult and pediatric R/R B-ALL (14). In yet another pivotal global Phase II trial with CTL019, a best overall response rate of 52% was observed in adult patients with R/R diffuse large B-cell lymphoma (LBCL), with an estimated 65% rate of relapse-free survival 12 months post initial response (15). Another successful trial was with axicabtagene ciloleucel (axi-cel, Yescarta®, Gilead), an autologous anti-CD19 CAR T-cell therapy. This received US-FDA approval in 2017 (16) for the treatment of adults with R/R LBCL after two or more lines of systemic therapies [ZUMA-1 trial (17)]. Recently, it has been extended for use in R/R follicular lymphoma (FL) and in earlier lines of therapy in LBCL with manageable adverse events (ZUMA-5, ZUMA-7, and ZUMA-12 trials) (17–19). Other approved CD19-directed CAR T-cell therapies include lisocabtagene maraleucel [liso-cel, Breyanzi®, Bristol Myers Squibb (BMS)] (20, 21) for R/R LBCL in the second/third-line setting and brexucabtagene autoleucel (brexu-cel, Tecartus®, Gilead) for R/R mantle cell lymphoma (MCL) (22) and adult B-ALL (23). Two CAR T-cell therapies targeting the B-cell maturation antigen (BCMA), idecabtagene vicleucel (idecel, Abecma®, BMS) (24) and ciltacabtagene autoleucel (ciltacel, Carvykti®, Janssen) (25), have been approved in R/R multiple myeloma. Additionally, other investigational CAR T-cell therapies targeting a variety of antigens are showing promising results in Phase I/II trials across the spectrum of hematologic malignancies (26–30). In solid malignancies, however, CAR T-cell therapies have encountered several hurdles resulting from tumor target heterogeneity, tumor penetration issues, and the immunosuppressive tumor microenvironment (TME). However, there are several ongoing early-phase clinical trials working on advancing these therapies to solid tumors such as brain, pulmonary, gastrointestinal, renal, hepatic, thoracic, ovarian, and prostate cancers (31, 32).
While overall response rates to CAR T-cell therapies have been impressive, challenges remain including manufacturing difficulties as a result of dysfunctional T-cells and expansion times. Toxicities regarded as a “class-effect” with these therapies are also of major concern. These include life-threatening forms of toxicity such as cytokine release syndrome (CRS), immune effector cell-associated neurotoxicity syndrome (ICANS), and others associated with “on-target, off-tumor” recognition and anaphylaxis (33–35). While the exact mechanisms underlying these adverse events (AEs) remain to be elucidated, the likely cause is thought to be a cytokine surge, which occurs with the immunological-activation cascade triggered by effector mechanisms of CAR T-cells. Another significant challenge is the recurrence of cancer in a significant proportion of patients after CAR T-cell therapy. Cancer relapses may occur due to varied combinations of intrinsic and/or extrinsic factors in the TME during and post CAR T-cell therapy. These include target antigen loss/mutations, peripheral and tumor-infiltrating T-cell exhaustion or senescence, immunogenicity-reducing alterations in the tumor mutational burden, and tumor progression due to an immunosuppressive TME (5, 34–37). Thus, it is imperative to identify such events when they occur to enable timely changes in treatment. Currently, efforts have been made to identify physiological biomarkers with prognostic implications, which may enable better management of these cell therapies (38).
Conventional pathology assays used in clinical practice for cancer management [e.g., immunohistochemistry, flow cytometry, enzyme- or polymerase chain reaction (PCR)-based assays] are limited by the availability of biopsy tissues. Additionally, the results obtained from these biopsied tumor samples suffer from spatial limitations. Given that the TME is physiologically and genetically heterogeneous, a tumor sample biopsied at any given location may not be representative of the characteristics of the entire primary tumor or distant metastases (39). Moreover, such tissue-based invasive techniques lack the “real-time” detection capabilities to capture dynamic variations in target expression during therapy or when AEs occur.
Together with their inherent capabilities for deep tissue penetration, “real-time” whole-body imaging, and high sensitivity for quantification, the non-invasive radionuclide imaging modalities of positron emission tomography (PET) and single-photon emission computed tomography (SPECT) are now enabling the development of technologies to address these challenges when equipped with radiolabeled monoclonal antibodies (mAbs) or engineered mAb fragments (40–45). Especially in the case of solid tumors, where CAR T-cell therapies suffer from challenges associated with infiltration into an immunosuppressive TME, these molecular imaging modalities may find application for the detection of CAR T-cell distribution, expansion, and clearance throughout the therapeutic regimen (46).
In this review, we discuss opportunities for immuno-PET/-SPECT imaging strategies to address the challenges encountered with these CAR T-cell therapies, and thereby to act as an important guidance tool for optimal therapeutic management.
Current advances in immuno-PET/-SPECT imaging methods and their potential to address the challenges with CAR T-cell therapy
The most commonly used PET radiotracer is 2-deoxy-2-[18F]fluoroglucose ([18F]FDG). A glucose analog, [18F]FDG is taken up by tumor cells via membrane-bound glucose transporters, where it is phosphorylated into [18F]FDG-6-phosphate and trapped in cells. This trapped metabolite uptake can be quantified by PET using a standardized uptake value (SUV) and correlates with disease severity. PET with [18F]FDG is used in clinical practice across a wide range of cancers for initial tumor diagnosis and staging and for the longitudinal assessment of therapy response (47–49). However, since [18F]FDG is primarily a metabolic radiotracer that measures elevated glycolysis, it cannot differentiate malignancies from co-existing non-malignant inflammatory conditions caused by rheumatological diseases, infections, or AEs encountered with cell-based immunotherapies (50). Owing to the increasing applications of inherently immunogenic CAR T-cell therapies for cancers, the development of immuno-PET/-SPECT strategies is warranted to delineate the interactions between malignancies and these supraphysiological immunological processes.
Early identification of the dominant resistance mechanisms within the heterogeneous and often immunosuppressive TME or the occurrence of toxic events associated with CAR T-cell therapies is essential to ensure successful therapeutic interventions. Additionally, as novel CAR T-cell therapies are developed, an assessment of their in vivo pharmacokinetics (biodistribution and “homing” to tumors, expansion, and clearance or potential destruction) is critical for reliable determination of their efficacy and prediction of the therapeutic outcome (46, 51). Combining the intrinsic sensitivity of PET/SPECT with the superior targeting specificity offered by mAbs (41, 42, 52, 53), immunological PET/-SPECT (immuno-PET/-SPECT) can be leveraged or tailored to address the following challenges encountered with CAR T-cell therapies (Figure 1).
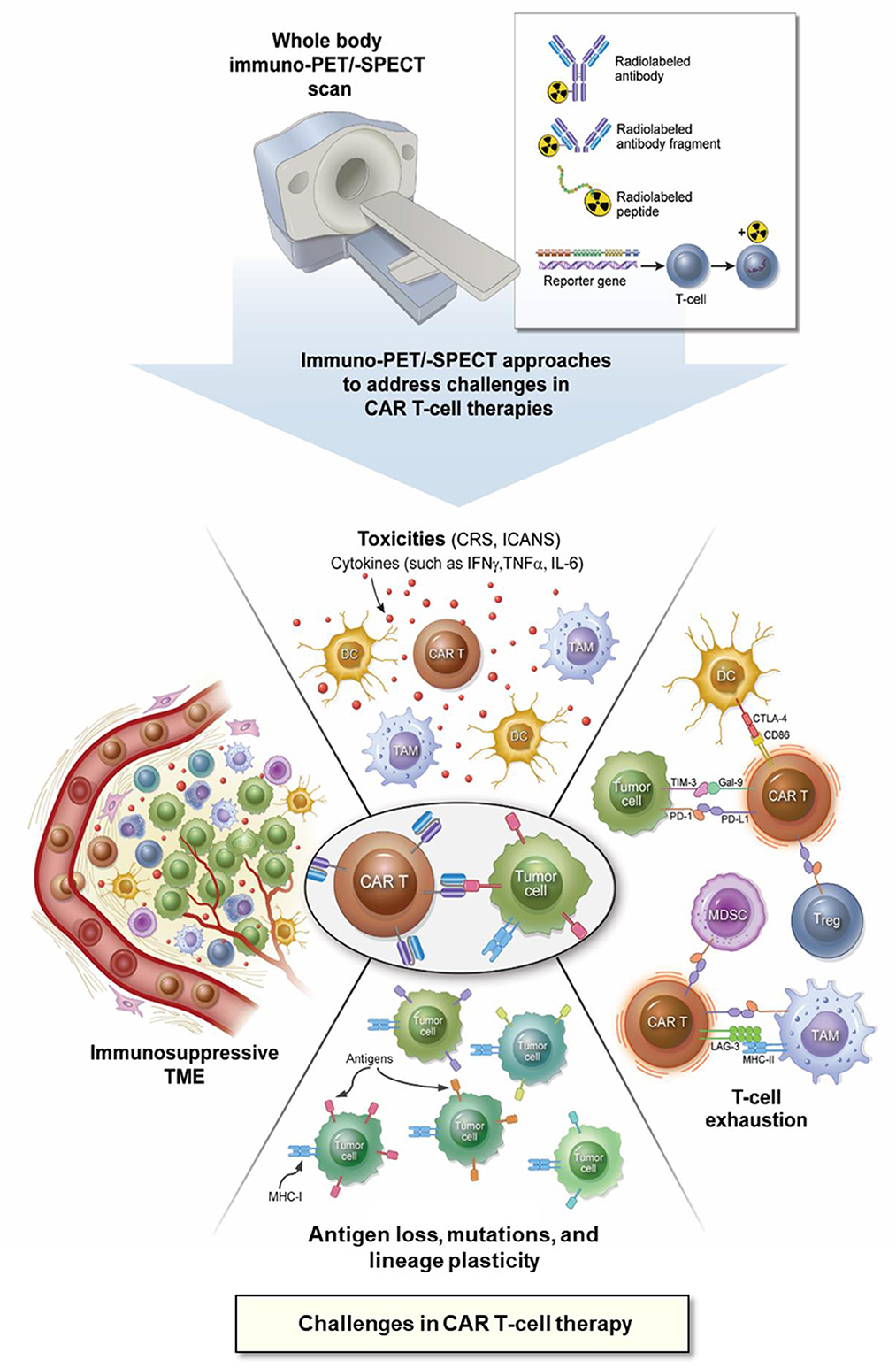
Figure 1. Application of immuno-PET/-SPECT imaging approaches to address the challenges with CAR T-cell therapies. CAR, chimeric antigen receptor; CD, cluster of differentiation; CRS, cytokine release syndrome; CTLA-4, cytotoxic T lymphocyte antigen-4; DC, dendritic cell; Gal-9, galectin 9; ICANS, immune effector cell-associated neurotoxicity syndrome; IFNγ, interferon gamma; IL-6, interleukin 6; immuno-PET/-SPECT, immunological positron emission tomography or single-photon emission computed tomography; LAG-3, lymphocyte activation gene 3 protein; MDSC, myeloid-derived suppressor cell; MHC, major histocompatibility complex; PD-1, programmed cell death protein 1; PD-L1, programmed cell death protein ligand 1; TAM, tumor-associated macrophage; TIM-3, T-cell immunoglobulin and mucin domain protein 3; TME, tumor microenvironment; TNFα, tumor necrosis factor alpha, Treg, regulatory T-cell.
Antigen loss
Antigen escape is one of the most commonly encountered mechanisms of cancer resistance to CAR T-cell therapy. It usually occurs in cases of cancer relapse after complete remission in patients, resulting in a phenotypically similar disease, but with either complete loss or downregulation of the target antigen expression. Consequently, the relapsed disease becomes non-responsive to the CAR T-cell treatment. For example, while remarkable response rates (70%−90%) have been observed for B-ALL in patients treated with CD19-directed CAR T-cell therapies such as CTL019 (54) in early-stage trials, follow-up studies have reported leukemia recurrence in ~50% of patients, typically 1 year post-therapy (54–56). Such cancer relapses associated with the loss of CD19 antigen have been reported in both children (~18%−25%) and in adult populations (~7%−9%) in Phase I studies (6, 54, 57–59). Given the multitude of clinical studies being conducted using CD19-directed CARs and bispecific T-cell engagers (BiTEs) (60), decreased/loss of antigen expression by R/R tumors may be attributed to various mechanisms, depending on the subject pool of a given study. Persistent immune pressure from CAR T-cell therapies may result in selective progression of tumor cells with genetic alterations in the CD19 protein, enabling antigen escape from CD19-directed CAR T-cell therapy (61–63). For example, in one reported study, whole-exome DNA- and RNA-sequencing analysis of baseline vs. post-relapse CD19-negative patient samples of R/R B-ALL demonstrated acquired frameshift mutations in CD19 exons 2–5. This likely resulted in a truncated protein sequence lacking transmembrane anchorage, leading to antigen escape (62). Furthermore, this study found that the allelic frequencies of the mutations correlated with the CD19-negative cells by flow cytometry and concluded that homozygous bi-allelic mutations (loss of heterozygosity) in CD19 are the primary resistance mechanism for CD19-negative relapse. Similar mechanisms for such inherited molecular resistance as a result of target antigen modulation have been observed for other targets of hematological malignancies, including CD22 in LBCLs (26), BCMA in myelomas (64), and even in solid tumors such as glioblastomas [epidermal growth factor receptor (EGFR) (65) and interleukin 13 receptor alpha 2 (IL13Rα2) (66)]. “Lineage switch” is another poorly understood mechanism of resistance to CAR T-cell therapy. In lineage switch, hematological cancer cells can undergo intrinsic changes to relapse as a clonally similar but phenotypically different cancer sub-type (67, 68). Such lineage plasticity is often encountered in pediatric and infant patients with refractory B-ALLs expressing mixed-lineage leukemia rearrangements (MLL-r). In such cases, the leukemia cells “switch” lymphoid physiological markers to become cells of a myeloid phenotype (68, 69). Relapses associated with MLL-r to acute myeloid leukemia (AML) have been seen with CD19-directed CAR T-cell therapy as well as BiTEs; however, other cases of phenotypic variation have also been observed (70–74). Other reported mechanisms for antigen reduction and escape include a phenomenon known as “trogocytosis,” whereby the CAR T-cells can strip the neighboring lymphoma cells of their target protein and incorporate it into the plasma membrane of the CAR T-cells, resulting in reduced surface target density. Trogocytosis may also result in “fratricide” by causing CD19+ T-cell death and promoting T-cell exhaustion (75–77). Such conditions could negatively impact the efficacy of CAR T-cell therapies (75, 76, 78, 79).
For the assessment of transient modulation, loss in antigen expression, or loss of function due to antigen mutation, immuno-PET/-SPECT imaging approaches using radiotracers derived from specific mAbs against cancer-overexpressing targets would be extremely valuable (Supplementary Table S1). Indeed, several surface target antigens in hematological malignancies have been considered for such imaging evaluations, including CD19, CD22, CD20, BCMA, and CD38 (53, 80). For example, immuno-PET imaging with a zirconium-89 ([89Zr])-labeled anti-CD20 mAb, [89Zr]Zr-DFO-rituximab, was reported in five patients with DLBCL (81). A correlation was found in this study between the imaging signal and CD20 expression measured by immunohistochemical (IHC) staining (Figure 2). More recently, a case report on immuno-PET with the same mAb but labeled with copper-64 (64Cu), [64Cu]Cu-DOTA-rituximab, demonstrated higher sensitivity than [18F]FDG-PET for imaging lymphoma tumors in two patients (82). Interestingly, although CD19 is an ideal target for CD19-directed CAR T-cell therapy in B-cell malignancies, to the best of our knowledge, there are no reports yet on CD19-targeted immuno-PET/-SPECT imaging to address antigen loss. However, a CD19-targeted immuno-PET method with [64Cu]Cu-CD19-mAb, a murine anti-CD19, has been reported to produce a PET signal correlating with B-cell distribution in the central nervous system (CNS) in an experimental autoimmune encephalomyelitis mouse model (83). Recently, with increasing efforts directed toward the application of CAR T-cell therapies for treating solid tumors, the target spectrum for CAR T-cell engineering has broadened considerably. Apparently, reported immuno-PET strategies using specific mAbs targeting solid tumor surface antigens, including EGFR [[89Zr]Zr-cetuximab (84), [89Zr]Zr-panitumumab (85, 86)], human epidermal growth factor receptor 2 (HER2) [[89Zr]Zr-trastuzumab (87–89), [111In]In-pertuzumab (90)], prostate-specific membrane antigen (PSMA) [[89Zr]Zr-J591 (91)], vascular endothelial growth factor (VEGF) [[89Zr]Zr-bevacizumab (92)], can be leveraged to non-invasively monitor antigen expression throughout the duration of CAR T-cell therapy (93). Furthermore, immuno-PET imaging methods may play an instrumental role in the detection of transient shifts in antigen expression, especially in ambiguous-lineage hematological cancers. For instance, longitudinal tracking of lymphoid lineage-specific surface antigens, such as CD19, CD20, CD3, and CD4, by specific mAbs or their fragments may enable the detection of resistance induced by “lineage switching” in rare high-risk ambiguous-lineage leukemias [such as in the case of mixed-phenotype acute leukemia (MPAL) switching to B- or T-cell ALL or vice versa (74, 94), or in MLL switching to AML (67)]. Of course, such rare malignancies often involve variations in multiple lymphoid or myeloid lineage surface markers, necessitating additional investigations on a case-by-case basis regarding the practicality of using immuno-PET/-SPECT imaging as a guidance tool for their management. It is noteworthy that immuno-SPECT is capable of simultaneous imaging of multiple surface markers if the corresponding surface antigens are targeted with mAbs labeled with radionuclides emitting differentiable gamma energies. Indeed, SPECT imaging with dual radiotracers has been reported in clinical applications (95–99) and preclinical studies (100–102). To date, non-invasive assessment of multiple biomarkers/molecular processes via a single immuno-SPECT scan has been made possible by the use of solid-state cadmium zinc telluride (CZT) gamma detectors, which offer higher energy resolution and detection sensitivity than the conventional sodium iodide (NaI) detectors, as well as the current implementation of novel image processing algorithms (96, 103).
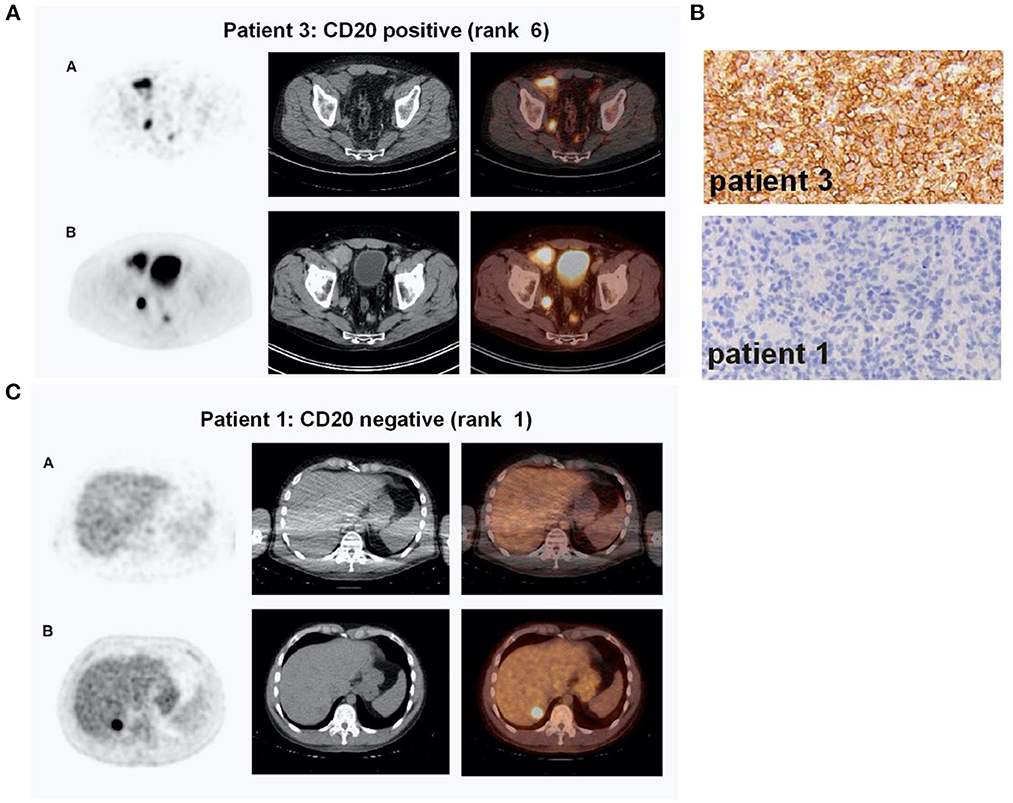
Figure 2. Immuno-PET imaging with [89Zr]Zr-Rituximab in patients with DLBCL treated with a therapeutic dose of rituximab. (A) Immuno-PET imaging shows intense tumor uptake (top panel) concordant with CD20-positive IHC staining of the inguinal lymph node biopsy sample (B, top panel). Corresponding [18F]FDG-PET images are shown in the bottom panel (A). (C) CD20-negative tumor shows no appreciable uptake of [89Zr]Zr-Rituximab (top panel) concordant with IHC staining of the biopsy (B, bottom panel). In contrast, the tumor exhibits a focal spot on [18F]FDG-PET images (C, bottom panel). Shown in (A) and (C) are attenuation-corrected PET, low-dose CT, and fused PET/CT images from left to right. Reproduced with slight format modifications from the open-access article in ref. (81) under the Creative Commons license (http://creativecommons.org/licenses/by/4.0/).
Given the long circulation half-lives of antibodies in the blood, immuno-PET/-SPECT imaging requires a half-life-matched radionuclide to label a mAb or an engineered fragment. Recently, 89Zr with a half-life (t1/2) of 3.27 days and 64Cu (t1/2 = 12.7 h) have gained popularity for labeling of antibodies and fragments because they can be produced in-house by a biomedical cyclotron equipped with solid-target capability. In terms of imaging sensitivity, immuno-PET is preferred over immuno-SPECT (53), while the latter can be readily performed when a radioimmunotheranostic agent is used. The decay of therapeutic radionuclides, such as lutetium-177 (177Lu, t1/2 = 6.65 days) and copper-67 (67Cu, t1/2 = 2.57 days), usually involves gamma rays that can be imaged with a SPECT scanner (42, 46). As shown in Figure 3, we reported on a study with [67Cu]Cu-pertuzumab in murine HER-2-positive xenografts demonstrating the specificity of immuno-SPECT imaging as well as the improved therapeutic efficiency resulting from the increase in molar activity of the radiotracer (42). Of note, if imaging sensitivity is desired, the therapeutic radionuclides can be replaced or paired with proper positron-emitting radionuclides [e.g., iodine-131 (131I, t1/2 = 8 days) with iodine-124 (124I, t1/2 = 4.2 days), 67Cu with 64Cu, yttrium-90 (90Y, t1/2 = 2.7 days) with yttrium-86 (86Y, t1/2 = 14.7 hours), and 177Lu (177Lu, t1/2 = 6.6 days) with gallium-68 (68Ga, t1/2 = 68 min)] for immuno-PET without drastically altering the targeting properties and in vivo kinetics of the radioimmunotheranostic agents. Such theranostic agents may find a greater role in the non-invasive monitoring of dynamic changes in antigen levels for precision CAR T-cell therapies.
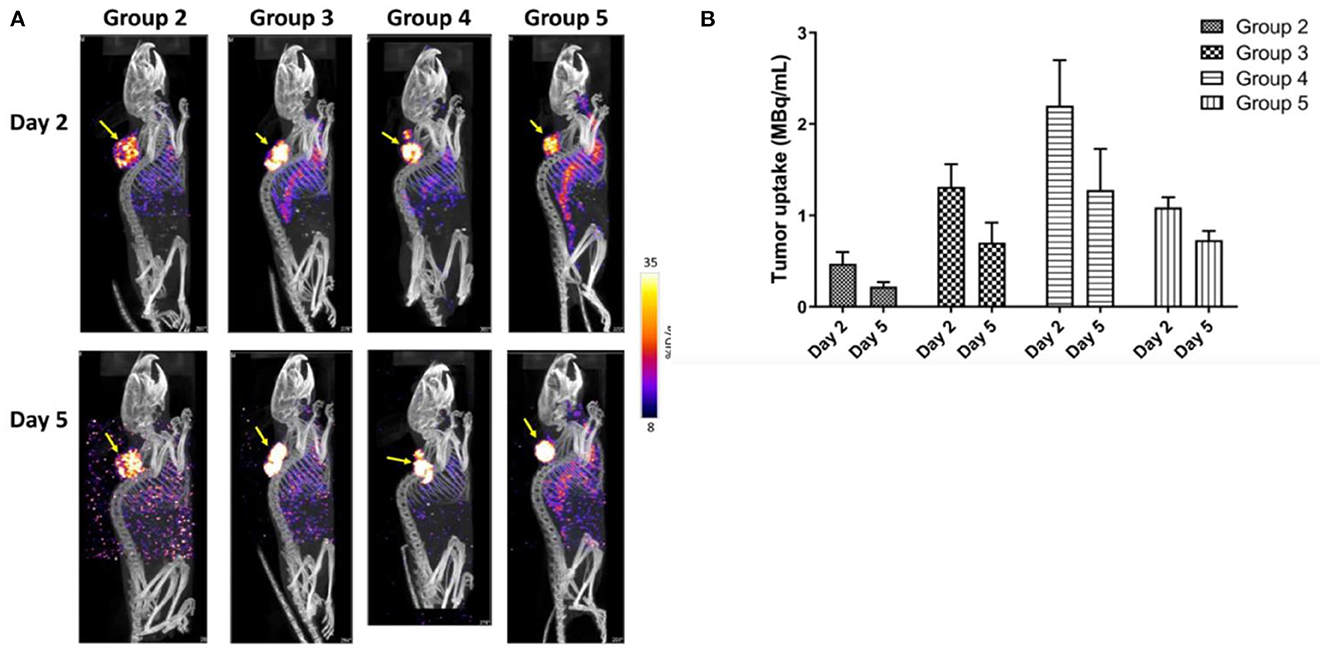
Figure 3. Immuno-SPECT/CT imaging of HCC1954 HER2+ tumor-bearing mice injected with [67Cu]Cu-NOTA-Pertuzumab. (A) Representative maximum intensity projection (MIP) of SPECT/CT images in mouse groups as indicated at days 2 and 5 post-treatment (yellow arrows indicate the tumors). (B) Actual radioactivity concentration in tumors (MBq/ml) on days 2 and 5 (without decay correction). Reproduced from the open access article in ref. (42) under the Creative Commons license (http://creativecommons.org/licenses/by/4.0/).
T-cell exhaustion and senescence
T-cell exhaustion and senescence are two dysfunctional states that heavily influence cancer management and patient outcomes with CAR T-cell therapies (104–106). While T-cell senescence is often associated with aging, it also occurs in chronic infections and in certain cancers (107). T-cell senescence is characterized by events including telomere shortening (during cell division), phenotypic changes (loss of CD28 domain), and cell cycle arrest, where the T-cells are live and metabolically active, but incapable of further proliferation or differentiation. This results in the loss of naïve and effector T-cells and dysregulation of the immune system (108, 109). T-cell exhaustion is a “hypo-responsive” state attained by T-cells after losing their effector functions as a result of persistent activation by antigens in response to chronic infections or tumor progression. In acute infections, the effector T-cell (Teff) population is gradually deactivated after the antigen is cleared or destructed, with the retention of a functional memory phenotype (Tmem). However, under conditions of persistent activation by CAR T-cell therapy, or during pathological chronic antigen stimulation, the CD8+ Teff cell population eventually differentiates into an exhausted phenotype (Tex) characterized by a lack of further differentiation and loss of effector function (107, 110, 111). Notably, the immunosuppressive TME plays an important role in driving the Teff population toward exhaustion and senescence (37, 105, 112). While senescent T-cells share overlapping phenotypes with exhausted T-cells, each has distinct mechanisms. Current studies indicate that while mitogen-activated protein kinase regulates T-cell senescence, T-cell exhaustion is mediated by inhibitory checkpoint proteins in the immunosuppressive TME and characterized by decreased cytokine secretion (106).
Some classic immune checkpoint receptors, which are T-cell exhaustion markers, include programmed cell death protein 1 (PD-1), lymphocyte activation gene 3 protein (LAG-3), T-cell immunoglobulin and mucin domain protein 3 (TIM-3), cytotoxic T lymphocyte antigen-4 (CTLA-4), B and T lymphocyte attenuator (BTLA), V-domain immunoglobulin-containing suppressor of T-cell activation (VISTA), and T-cell immunoglobulin and immunoreceptor tyrosine-based inhibitory motif domain (TIGIT) (69, 106, 107, 111, 113–115). Among the comprehensively studied proteins, binding of PD-1 to its ligand PD-L1 is reported to regulate T-cell immunosuppression by initiating the inhibitory downstream signaling of zeta-chain-associated protein kinase 70-extracellular signal-regulated kinase (ZAP70-ERK) and phosphatidylinositol-3 kinase-protein kinase B (PI3K-AKT) via the recruitment of Src homology 2 domain-containing tyrosine phosphatases 1 and 2 (SHP1 and SHP2), and can arrest T-cell proliferation via inhibition of cyclin-dependent kinases (106, 116). CAR T-cell therapies with CD28 stimulation domain (rather than 4-1BB) have shown more susceptibility to inhibition via the PD-1/PD-L1 checkpoint axis by direct inactivation of the CD28 signaling domain (106, 117–119). Interestingly, clinical trials combining immune checkpoint inhibitor (ICI) antibodies with CD19-directed CAR T-cell therapies (120–124) and novel CAR T-cell design strategies blocking the PD-1/PD-L1 interactions (119, 125–127) have demonstrated prolonged T-cell persistence and promising treatment outcomes.
Thus far, immuno-PET has advanced to a point enabling imaging evaluation of T-cell exhaustion pathways and immunosuppressive biomarkers in the TME (Supplementary Table S2). Promising clinical data using radiolabeled intact mAbs targeting the inhibitory checkpoint proteins, such as PD-1 (128, 129), PD-L1 (41, 130–132), and CTLA-4 (133, 134), have fundamentally validated the immuno-PET approach. For instance, clinical studies with [89Zr]-labeled atezolizumab have demonstrated that ICI treatment outcomes can be better predicted with immuno-PET performed before ICI than with other tissue-based methods (e.g., ribonucleic acid (RNA)-sequencing or IHC) in three solid tumor types (triple-negative breast cancer, bladder cancer, and non-small cell lung cancer) (130). As shown in Figure 4, immuno-PET with this radiotracer has also shown potential for stratification of patients with renal cell carcinomas based on imaging-assessed PD-L1 expression in patient-derived tumor grafts and in a clinical report (41, 131). In addition to intact mAbs of PD-L1, immuno-PET with radiotracers derived from small adnectin proteins have also produced encouraging results in multiple clinical studies (135–137). More recently, alternative inhibitory immune checkpoint receptor/ligand pathways (e.g., TIGIT, LAG-3, and TIM-3) have been employed to design immunotherapies that can avert the toxicities associated with anti–PD-1/PD-L1 and anti-CTLA-4 ICIs and improve treatment efficacy as combination therapies. These immunotherapies can be readily adapted for immuno-PET imaging. Indeed, such imaging methods have demonstrated the capability to capture variation in these checkpoint proteins (138–141). For instance, with variable but high expression on TILs in solid tumors, TIGIT is also present on activated CD8+ T-cells, activated CD4+ regulatory T-cells, and natural killer (NK) cells (138, 142). This protein mediates inhibition of innate and adaptive immunity through inhibition of T-cell and NK cell immune responses (143, 144). Immuno-PET with TIGIT-specific [64Cu]Cu-TIGIT-mAb and [89Zr]Zr-TIGIT-mAb has demonstrated high specificity for TIGIT expression in xenograft (HeLa-TIGIT in nu/nu mice) and allograft (B16 melanoma in B6 mice) models (138). These results suggest the feasibility of utilizing immuno-PET for non-invasive patient stratification based on TIGIT expression for anti-TIGIT therapy. Such imaging methods hold great potential to guide combination ICI treatment (145) in order to overcome T-cell exhaustion during CAR T-cell therapies.
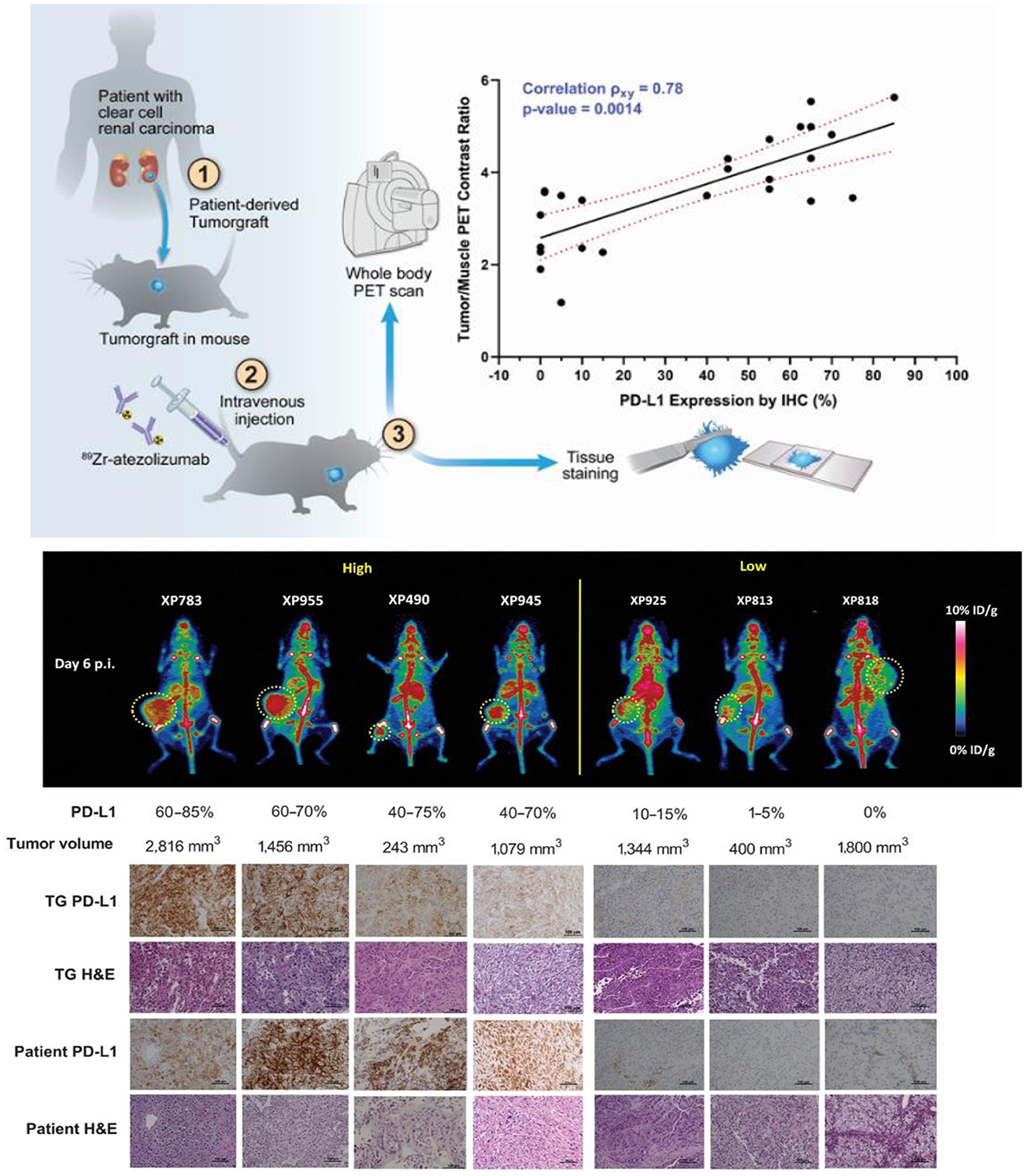
Figure 4. Integrated immuno-PET, IHC, and hematoxylin and eosin (H&E) images of mouse tumorgraft lines from corresponding patient tumors with high and low PD- L1 expression groups. (Top) Schematic representative of the workflow. (Middle) Representative whole-body MIP immuno-PET images (posterior view) of mice, one from each group. The corresponding PD-L1 expression ranges measured by IHC and the volume of the tumor in the mouse as indicated are shown below (n = 3–4 for each line; a single remaining XP258 mouse is not included). Tumors are indicated with a yellow lasso. (Bottom) PD-L1 IHC and H&E staining of the corresponding tumor tissues explanted from the TG models. Patient tumor samples shown as a reference. A part of this figure has been reproduced from the referenced article (41) under reuse permission from standard copyright in AACR journals for authors.
In contrast to the well-documented roles of T-cell exhaustion in cancer relapse/resistance against CAR T-cell therapies, the mechanisms of T-cell senescence mediated by the TME remain largely unknown (146, 147). The senescent T-cell phenotype is associated with substantial downregulation of CD28 and CD27 stimulatory markers and an increase in beta-galactosidase (SA-β-gal) activity (147, 148). Other T-cell senescence-associated markers include TIM-3, CD57, and killer cell lectin-like receptor subfamily G member 1 (KLRG-1) (149–152). Moreover, a unique senescence-associated secretory phenotype (SASP) has been reported with senescent T-cells; this phenotype generates large amounts of pro-inflammatory cytokines, such as interleukin 2 (IL-2), IL-6, IL-8, TNF-α, and interferon gamma (IFN-γ), in addition to the suppressive cytokines IL-10 and transforming growth factor β (TGF-β) (146, 153, 154). As such, PET has been employed to detect T-cell senescence via imaging of surrogate markers, such as TIM-3 (141), overexpression of SA-β-gal enzyme (155–157), IL-2 (158), TNF-α (159), and IFN-γ (160) (Supplementary Tables S2–S4).
There are several important factors to consider in the development of radiolabeled antibodies for immuno-PET/-SPECT imaging: for instance, the Fc-receptor interactions. Although these interactions may be advantageous for some therapeutic mAbs due to the resulting prolongation of their systemic half-lives and accentuation of their effector functions (161), they may expose patients to higher doses of radiation when radiolabeled for radiotherapy or imaging. In addition, such Fc interactions may compromise the desired antigen-targeted immuno-PET signal due to a high non-target uptake (162). To overcome this issue, it is plausible to silence the Fc domain (163) [e.g., through selection of mAbs such as atezolizumab (164)] or to use engineered mAb fragments (e.g., minibodies, diabodies, and BiTEs) (165). Other issues with this approach include the solubility of target antigens in plasma (166–168).
CAR T-cell distribution
For manufacturing of CAR T-cell products, the recipient patient's own (autologous) T-cells are the preferred source in order to avoid the possibility of graft-vs.-host reactions due to the use of donor T-cells. However, adherence to this personalized adoptive cell therapy can jeopardize the extension of treatment benefits to a larger cohort of patients (126). In general, CAR T-cell therapies inherently suffer from manufacturing limitations associated with long production times due to the required T-cell selection and expansion processes to ensure a high-quality product (34). Moreover, most patients receiving these CAR T-cell therapies suffer from advanced cancers and have previously undergone conventional chemotherapies or other immunotherapies. Consequently, these patients may be lymphopenic with a high possibility of dysfunctional or exhausted T-cells (169). This can be a severe limiting factor for product development, significantly impacting the treatment outcome (170–172). To address these challenges, the development of allogeneic CAR T-cells from donors has gained impetus as an alternative strategy enabling a large scale universal production for “off-the-shelf” doses of the CAR T-cell product. Novel research strategies may also involve designing combination CAR T-cells and CAR T-cells with BiTE formulations (60, 126, 173). Moreover, “universal CARs” can be designed with dual targeting capabilities to overcome resistance to CAR T-cell therapy owing to the loss of a single antigen (174, 175). Nevertheless, the risks associated with potential toxicities, such as graft-vs.-host and autoimmunity reactions, require careful consideration in the context of such strategies (176). To avoid toxicities, standard-of-care CAR T-cell therapy protocols recommend the injection of only a limited number of CAR T-cells (~105 to 106 cells per kg of body weight) (177). With the capability for direct tracking of the in vivo dynamic distribution of CAR T-cells and TILs, immuno-PET/-SPECT may provide pivotal information for the optimization therapeutic outcomes and enable evaluation of therapy-induced alterations and detection of resistance mechanisms (43, 178). Because others have reviewed these approaches in detail (46, 51, 179), we provide only a few highlights below and in Supplementary Table S3.
In the TME and in the systemic circulation, TILs, cytotoxic CD8+ and helper CD4+ T-cells, play a key role in driving the antitumor immunological responses in immunotherapies. With radiolabeled CD8- and CD4-specific minibodies (72, 180, 181) and cys-diabodies (43, 182), immuno-PET imaging has been proven with the capability to reveal T-cell-enriched tissues, such as the lymph nodes, spleen, and thymus in mouse models. For instance, immuno-PET with 89Zr-labeled anti-CD8 cys-diabody has been found to be able to detect the mobilization of CD8-expressing T lymphocytes from the systemic circulation to tumors in syngeneic mouse models when subjected to immunotherapies with an agonistic mAb (anti-CD137/4-1BB), checkpoint blockade mAb (anti–PD-L1), and ACT (182). Recently, a 89Zr-labeled anti-CD8 minibody (89Zr-Df-IAB22M2C) has advanced to early-phase clinical trials in subjects with primary (183) (Figure 5) and metastatic solid tumors (melanoma, non-small cell lung cancer, and hepatocellular carcinomas) (184). Furthermore, another report using 64Cu labeled IAB22M2C has described similar applications in brain tumors (66, 180). Notably, bispecific antibody constructs consisting of two ScFv arms have been seen in this endeavor, one targeting the tumor antigen and the other often targeting CD3 markers on T-cells. However, in such bispecific constructs, the target with the stronger affinity to the radiotracer may likely predominate in the radiotracer's biodistribution. For example, in a first-in-human imaging study with a carcinoembryonic antigen (CEA)/CD3-targeting radiotracer, 89Zr-AMG 211, intra- and inter-subject heterogeneous tumor uptake was observed, which was largely dominated by the CD3 arm. As such, the imaging results likely depicted T-cell distribution (166, 185). While the faster clearance and earlier imaging time points seen with mAb fragments are clinically advantageous, their imaging sensitivity and specificity remain to be improved, as their specific binding affinities are inevitably compromised as compared to their mAb counterparts (186).
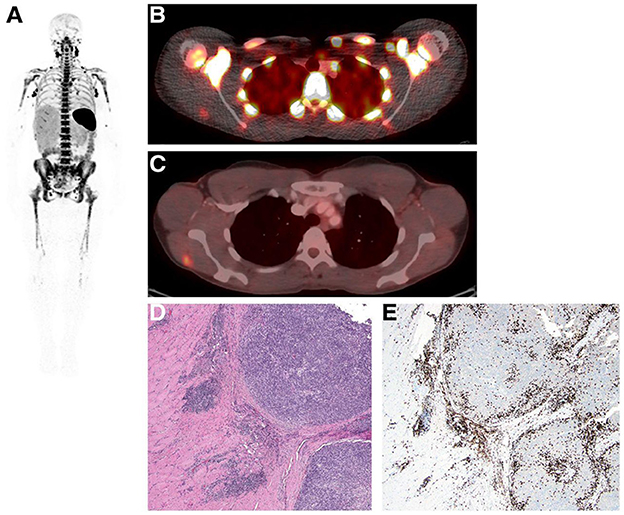
Figure 5. Whole-body immuno-PET imaging with [89Zr]Zr-IAB22M2C in a patient at 24 h post-injection. (A) Intense uptake is noted in lymph nodes. (B, C) Fusion image at 24 h shows [89Zr]Zr-IAB22M2C uptake in lesion and deltoid (B), which were also [18F]FDG positive (C). (D) H&E stained section shows melanoma tumor nodules on the right within skeletal muscle. (E) IHC highlights the presence of CD8+ T-cells at the periphery and infiltrating tumors [reproduced with permission from original publication Pandit-Taskar et al. (183)].
In vivo tracking of CAR T-cells can be realized by direct labeling with 111In, 89Zr, 99mTc, or 68Ga (187–191). However, these techniques face major challenges for longitudinal in vivo tracking due to the loss of radiolabels during subsequent passages of CAR T-cells and decay of the radionuclide (192). Moreover, this type of radiolabeling technique cannot distinguish between live and dead CAR T-cells, although the latter are likely to be digested or sequestered in the liver or spleen. Therefore, transduction of CAR T-cells with a protein reporter has been employed for pairing with a well-established PET imaging method. To date, many such reporter/radiotracer pairs have been developed to capture the spatiotemporal expansion of CAR T-cells in preclinical mouse models (51, 178, 193): for instance, PSMA can be paired with [18F]F-DCFPyL, a PSMA-specific PET agent (194). PSMA was chosen because of its well-accepted role in theranostic treatment of cancers (195, 196). Another protein of interest is the somatostatin receptor 2 (SSTR2), a G-protein-coupled membrane receptor with basal expression in normal tissues and overexpression in many neuroendocrine tumors (NETs) (197–199). Notably, a recent study has gone one step further to investigate the potential of using the SSTR2 reporter as a suicide switch to destroy the CAR T-cells when they generate toxic AEs. In this approach, a maytansine–octreotate conjugate, PEN-221 (Tarveda), was used for imaging and elimination of CAR T-cells when they became toxic (200). Another interesting study used an engineered antibody against DOTA (1,4,7,10-tetraazacyclododecane-1,4,7,10-tetraacetic acid; DAbR1) for both cell tracking and a potential antibody–drug conjugate (201). DAbR1 contains a single-chain fragment of the anti–lanthanoid-DOTA antibody 2D12.5/G54C fused to the human CD4-transmembrane domain and binds irreversibly to lanthanoid (S)-2-(4-acrylamidobenzyl)-DOTA (AABD) (202) for imaging of DAbR1-positive T-cells when labeled with 86Y (201).
While most of these reporter/radiotracer studies are still at the preclinical stages, a successful first-in-human trial tracking CAR T-cells has been reported in the case of a 57-year-old man with grade IV glioblastoma whose autologous CD8+ T-cells were genetically engineered to express the herpes simplex virus type 1 thymidine kinase (HSV1-tk) suicide gene for PET imaging with 9-[4-[18F]fluoro-3-(hydroxymethyl)butyl]guanine ([18F]F-FHBG) (203). This approach was further validated in a subsequent clinical trial with a cohort of six patients with glioblastoma (204). It is noteworthy that, while these studies have set the stage for clinical CAR T-cell imaging, the challenges are also evident in terms of their limited clinical practicality (e.g., extrinsic viral proteins are required and signal-to-noise ratios are suboptimal) (205).
T-cell activation
While imaging of T-cell lineage markers (e.g., CD3, CD4, and CD8) can provide information regarding mobilization and tumor retention of the T-cells (both CAR T-cell therapies and other T-cells), it is essential to know whether these T-cells are activated. Immuno-PET imaging of proteins specifically upregulated during T-cell activation can function as biomarkers to address this issue (Supplementary Table S4). For instance, inducible T-cell costimulator (ICOS) is a T-cell co-stimulatory molecule upregulated during T-cell activation. Using a 89Zr-labeled anti-ICOS mAb, the activation, expansion, and tumor retention of CD19-directed CAR T-cells have been investigated in a mouse model of B-cell lymphoma (44, 206). Absent in resting naïve T-cells, CD134 or OX40 could also be used as T-cell activation markers (207). Furthermore, cytokines generated in response to T-cell activation, such as IFN-γ (160) and IL-2 (Figure 6) (158, 208), have also been reported on for use in immuno-PET imaging of T-cell activation.
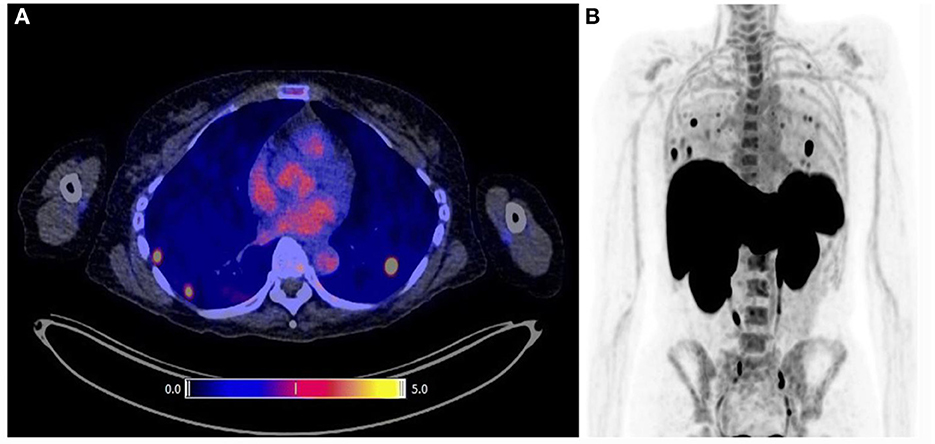
Figure 6. Representative [18F]FB-IL2 PET images of human melanoma. (A) Transversal PET/CT image of three regions showing high [18F]FB-IL2 uptake. (B) MIP image of the same patient showing multiple areas of high radiotracer accumulation in the lungs. Reproduced from the open access article in ref. (158) under the Creative Commons license (http://creativecommons.org/licenses/by/4.0/).
Molecular imaging of T-cell distribution and activation is capable of providing an immune signature of ongoing cytotoxic responses or possibly of resistance to immunotherapies (209, 210). Recent reports on a PET agent that targets human granzyme B, 68Ga-NOTA-GZP, are noteworthy (209, 211, 212). Granzyme B is a pro-apoptotic serine protease, secreted and activated via granular exocytosis along with perforin by activated cytotoxic T-cells and NK cells. It initiates the target cell death cascade by caspase activation (213, 214). Interestingly, 68Ga-NOTA-GZP only targets the active secreted form of the enzyme (biological t1/2 = 14 days), making it an ideal candidate for detection of the extent of cytotoxic response, or lack thereof, in the TME (212). Interestingly, PET with 68Ga-NOTA-GZP has been found to be able to reveal distinct immune signatures associated with immunoactivation in tumors and tumor-draining lymph nodes.
Adverse toxic events
CAR T-cell therapies as a class have been found to be associated with certain unique toxicities due to the immunological surge of cytokines that follows the CAR T-cell-based T-cell activation cascade. These AEs are termed CRS and ICANS, which encompass the most notable CAR T-cell toxicities. Additionally, other toxicities such as “on-target, off-tumor” and anaphylactic effects have been reported. Unlike side effects observed with other chemotherapeutics, which are often non-specific, the toxicities observed in CAR T-cell therapy are on-target and reversible in most cases. Minimization of these toxic events is highly desirable in clinical management with CAR T-cell therapies (33).
To date, several pathophysiological mechanisms behind the occurrence of CRS and ICANS have been elucidated in the literature (215–217). Usually, CRS is triggered within days after CAR T-cell activation post-infusion, although delayed AEs may occur up to 3 weeks post-infusion due to prolonged systemic circulation of the CAR T-cells (218). The symptoms of CRS are mainly perpetuated by elevated circulating levels of pro-inflammatory cytokines, including IFN-γ, TNF-α granulocyte-macrophage colony-stimulating factor (GM-CSF), IL-10, IL-1, and IL-6, as well as other inflammatory mediators such as nitric oxide (216, 219). These AEs range from mild (grade 1) flu-like symptoms to more severe (grade 3–4) manifestations, including hypotension, tachycardia, difficulty breathing, hypoxia and capillary leak, hypoalbuminemia, coagulopathy, shock, and, in some rare cases, multiple organ injury/failure, which need immediate medical attention (220–222). CRS-related AEs are in fact quite commonly encountered in patients treated with CAR T-cell therapeutics, with ~53%−93% experiencing different grades of CRS-related AEs, ~13%−14% undergoing severe (≥ grade 3) reactions, and ~20%−50% needing to be transferred to intensive care units (30, 216, 223, 224). These AEs are not limited by disease phenotype (they are seen in lymphomas, leukemias, and even myelomas) or by the type of antigen being targeted, although the CRS AEs and neurotoxicity are more commonly seen with CD19-directed CAR T-cell therapies than others (30, 219, 220, 225). Notably, CRS is not limited to CAR T-cell therapies and may be seen with other agents (215, 226) and immunopathologic conditions that may affect B- and/or T-cell function (216). ICANS is the second major form of toxicity seen with CAR T-cell therapies as well as BiTEs, with symptoms including encephalopathy, aphasia, delirium, tremor, and seizures (225). The pathophysiology of ICANS is still largely unknown as compared to CRS. Under the mechanisms that have been proposed, initial pro-inflammatory cytokine activation mediated by CAR T-cells may result in endothelial activation and increased microvascular permeability, which may lead to disruption of the blood–brain barrier and subsequent passive diffusion of CAR T-cells and cytokines in the central nervous system (CNS). These events may further trigger a positive immunoactivation feedback loop for manifestation of ICANS (216, 217). While they are more commonly seen with CD19-directed CAR T-cells, neurotoxic AEs also occur with other non-CD19-targeting CAR T-cell therapies (225). Although less common than CRS, ICANS is known to occur in ~21%−66% of all patients treated with CAR T-cell therapies, with severe ICANS AEs (≥ grade 3) occurring in ~12%−45% (225, 227). Elevated cytokine levels in CRS may often precede neurotoxic events. However, ICANS may occur concurrently, after CRS has subsided, or even independently of CRS. The symptoms of ICANS may be relatively mild without CRS interplay (216, 217). As CAR T-cell therapies progress toward applications in solid tumors, unique “on-target, off-tumor” forms of toxicity have been encountered. Such toxicities may occur in non-diseased tissues, wherein CAR T-cells primed to attack the tumors overexpressing the target antigen may also affect normal tissues with basal antigen expression (228). In these situations, the expression level of the target antigen on the non-diseased tissues becomes a determinant of the severity of the AE (33, 229). Other rare instances of toxicity include symptoms such as anaphylaxis following an immune response to the CAR (230); in most instances, these occur as a result of the mAb-derived antigen-recognition domains in the CAR structure (231).
Currently, there are no approved preventive measures for these toxicities (232). However, whole-body PET or SPECT imaging has the capability to locate these AEs as they occur anywhere in the body, which can be leveraged to guide therapeutic interventions (233). In clinical practice, [18F]FDG-PET plays a role in the management of CAR T-cell therapies, but it cannot differentiate neoplastic disease or other inflammatory events from a hyper-inflammatory episode such as CRS (233). To the best of our knowledge, no immuno-PET approaches have been reported for imaging of these toxicities. As a wide array of cytokines are upregulated at different points during the CRS cascade (216), imaging specificity is difficult to achieve. Another major hurdle is the lack of a suitable mouse model for investigation of CRS events, although two humanized mouse models have been reported for CRS and ICANS that may be useful for proof-of-concept studies (234, 235). To date, sufficient evidence has shown IL-6 serum cytokine levels to be the most significantly elevated during CRS (215, 236, 237). Clinically, tocilizumab, a mAb inhibitor of IL-6 receptor (IL-6R), has therapeutic applications as a first-line agent with corticosteroids to treat grade 2 CRS AEs in patients receiving CAR T-cell therapies (237, 238). Therefore, immuno-SPECT with 99mTc-labeled tocilizumab and optical imaging with Cy7-tagged tocilizumab have been reported for preclinical imaging of myelomas (239–241). Siltuximab is another IL-6 inhibitor mAb that is used as an alternative third-line treatment in patients with CRS and ICANS who are unresponsive to tocilizumab and corticosteroids (220, 242). Both mAbs can be considered for immuno-PET imaging in CRS. IL-1 is another key player generated early in CRS initiation, and Anakinra®, a recombinant human IL-1 antagonist, has demonstrated favorable efficacy against CRS and ICANS based on a study in humanized models and early clinical trials (234, 235). While 18F and 99mTc radiolabeling methods have been reported for peptides inhibiting IL-1, in vivo imaging remains to be evaluated for these probes (243–246). Moreover, immuno-PET imaging with [89Zr]Zr-α-IL-1β has been found to be able to detect colonic inflammation in murine dextran sodium sulfate-treated colitic models, which correlates with the severity of the disease (247). Rather than directly targeting individual cytokines mediating CRS or ICANS, targeting of their common upregulated downstream immune checkpoints (indirect targeting) may hold promise for imaging of these toxicities. For instance, PD-L1 is known to be upregulated by multiple cytokines as an inhibitory ligand in the PD-1/PD-L1 checkpoint axis; this synergistic upregulation may provide a strong imaging signal enhancement for sensitive detection (41, 114, 248, 249). As such, the validated methods for immuno-PET imaging of PD-L1 remain to be tested for imaging of these AEs.
Notably, crosstalk between cancer cells and the TME plays an important role in targeted therapies for cancer. Advanced solid malignancies often feature a hypoxic and immunosuppressive TME, which acts as a barrier impairing the effectiveness of therapies. In fact, despite impressive clinical outcomes in patients with advanced R/R B-cell hematological malignancies and multiple myelomas, CAR T-cell therapy faces hurdles in treating solid tumors with an immunosuppressive TME (31, 250). Moreover, the neovasculature encompassing solid tumors often restricts infiltration of the TME by CAR T-cells. TME is a specialized environment consisting of dynamic interplays between varieties of cells in the milieu of aberrant metabolites and cell signals (251, 252). Cancer cells can gain survival advantages by manipulating innate cellular mechanisms, for instance, by hijacking immunomodulation pathways to evade immune surveillance or to escape killing by immunotherapies. Due to the dynamic nature of the TME, it can be morphologically, phenotypically, and functionally heterogeneous across time, subjects, and tumor sites (primary vs. metastases), and even across regions within the same tumor (32, 253). Immuno-PET/-SPECT imaging can be leveraged to non-invasively track dynamic changes in the TME in real-time, thus providing invaluable information for precision treatment strategies.
The “hyper-metabolic” state of aggressive malignancies often produces conditions of nutritional deficit, hypoxia, pH reduction (acidosis due to lactic acid generation post-glycolysis) of the TME, and oxidative stress (37). It is well-known that hypoxic conditions stabilize hypoxia-inducible factors that promote angiogenesis. Recently, it has been found that hypoxia induces immune evasion by upregulating the immune checkpoint proteins [e.g., PD-L1, PD-L2, human leukocyte antigen-G (HLA-G), and soluble CD137] (254, 255), impairs the expansion of CAR T-cells, and reduces immune activation (256). Therefore, recent CAR T-cell therapy strategies have been expanded to include transduction of the CAR design with hypoxia-sensing domains (257) and targeting of antigens upregulated in hypoxia [e.g., carbonic anhydrase IX (CAIX) (258)] in order to improve the therapeutic efficacy (32, 259). Of note, immuno-PET with 124I or 89Zr-labeled girentuximab, an anti-CAIX mAb, has advanced to clinical trials in patients with renal cell carcinoma (260–262) and urothelial cancers (263), while the 89Zr-labeled mAb has demonstrated improved detection sensitivity due to the residualizing properties of the radionuclide (264). Immuno-PET/-SPECT with radiolabeled pH-selective mAbs, which has not yet been reported, might find application in non-invasive assessment of TME acidosis (265).
Conclusion
Adoptive cell therapy has brought about a paradigm shift in cancer treatment using innovative immunotherapy approaches. Evidently, these novel drugs come with their own unknowns, challenges, and certain unique toxicities. Molecular resistance mechanisms, such as antigen loss and T-cell exhaustion, particularly in the immunosuppressive TME, are still the most significant challenges faced by CAR T-cell therapy. Consequently, there is an urgent unmet clinical need for early identification and tracking of these mechanisms in order to implement timely treatment interventions. In recent years, synergizing of the highly sensitive PET and SPECT functional imaging modalities with anatomical/physiological computed tomography or magnetic resonance imaging has generated a multifaceted, highly sensitive, non-invasive platform for real-time detection of dynamic events in live subjects. To date, this platform has been validated for use in clinical diagnosis and disease management in various diseases and conditions, including cancer. Moreover, recent technological advancements and sophisticated algorithms for SPECT have advanced its capability for simultaneous imaging of two radionuclides with differentiable emission energies, thus enabling non-invasive assessment of the simultaneous occurrences of two biological events (266–268). In addition, recent solid-state detectors and advanced reconstruction algorithms have further improved the sensitivity and spatial resolution of SPECT. As such, we expect to see accelerated progresses in immuno-PET/-SPECT imaging and their applications in immunotherapies. In conjunction with the explosive development of innovative strategies in the realm of spatial-omics (transcriptomics, proteomics, and metabolomics), novel targets will certainly emerge for the future development of more practical immuno-PET/-SPECT imaging methodologies to address the challenges of CAR T-cell therapy (269). To add to this arsenal, deep learning-based radiomic analysis of image features extracted from the vast datasets of available images could further move the field forward.
Author contributions
OKÖ, PR, and XS: conceptualization. AM, DU, and YY: writing—preparation of original draft. AM, DU, SH, OKÖ, PR, and XS: writing—review and editing. AM and DU: visualization. XS: supervision. All authors have read and agreed to the published version of the manuscript.
Acknowledgments
The authors acknowledge the partial support of their immuno-PET/-SPECT-relevant projects by the Cancer Prevention and Research Institute of Texas (RP170638), the Prostate Cancer Research Program of the Department of Defense (W81XWH-19-1-0711), the Dr. Jack Krohmer Professorship Funds, and a pilot grant from the Circle of Friends of the University of Texas Southwestern Medical Center (Dallas, Texas). The authors also would like to thank Ms. Erin Moore, a Senior Graphics Designer in the Department of Radiology for her assistance with the illustrative figures.
Conflict of interest
The authors declare that the research was conducted in the absence of any commercial or financial relationships that could be construed as a potential conflict of interest.
Publisher's note
All claims expressed in this article are solely those of the authors and do not necessarily represent those of their affiliated organizations, or those of the publisher, the editors and the reviewers. Any product that may be evaluated in this article, or claim that may be made by its manufacturer, is not guaranteed or endorsed by the publisher.
Supplementary material
The Supplementary Material for this article can be found online at: https://www.frontiersin.org/articles/10.3389/fmed.2023.1199146/full#supplementary-material
References
1. Martínez-Lostao L, Anel A, Pardo J. How do cytotoxic lymphocytes kill cancer cells? Clin Cancer Res. (2015) 21:5047–56. doi: 10.1158/1078-0432.CCR-15-0685
2. Zhou J, Shen X, Huang J, Hodes RJ, Rosenberg SA, Robbins PF. Telomere length of transferred lymphocytes correlates with in vivo persistence and tumor regression in melanoma patients receiving cell transfer therapy. J Immunol. (2005) 175:7046–52. doi: 10.4049/jimmunol.175.10.7046
3. Robbins PF, Lu YC, El-Gamil M, Li YF, Gross C, Gartner J, et al. Mining exomic sequencing data to identify mutated antigens recognized by adoptively transferred tumor-reactive T cells. Nat Med. (2013) 19:747–52. doi: 10.1038/nm.3161
4. Rosenberg SA. IL-2: the first effective immunotherapy for human cancer. J Immunol. (2014) 192:5451–8. doi: 10.4049/jimmunol.1490019
5. Rosenberg SA, Yang JC, Sherry RM, Kammula US, Hughes MS, Phan GQ, et al. Durable complete responses in heavily pretreated patients with metastatic melanoma using T-cell transfer immunotherapy. Clin Cancer Res. (2011) 17:4550–7. doi: 10.1158/1078-0432.CCR-11-0116
6. Maude SL, Frey N, Shaw PA, Aplenc R, Barrett DM, Bunin NJ, et al. Chimeric antigen receptor T cells for sustained remissions in leukemia. N Engl J Med. (2014) 371:1507–17. doi: 10.1056/NEJMoa1407222
7. Bourbon E, Ghesquières H, Bachy E. CAR-T cells, from principle to clinical applications. Bull Cancer. (2021) 108(10, Supplement):S4–17. doi: 10.1016/j.bulcan.2021.02.017
8. He Q, Jiang X, Zhou X, Weng J. Targeting cancers through TCR-peptide/MHC interactions. J Hematol Oncol. (2019) 12:139. doi: 10.1186/s13045-019-0812-8
9. De Bousser E, Callewaert N, Festjens N. T Cell engaging immunotherapies, highlighting chimeric antigen receptor (CAR) T cell therapy. Cancers. (2021) 13:6067. doi: 10.3390/cancers13236067
10. Lee S, Margolin K. Tumor-infiltrating lymphocytes in melanoma. Curr Oncol Rep. (2012) 14:468–74. doi: 10.1007/s11912-012-0257-5
11. June CH, O'Connor RS, Kawalekar OU, Ghassemi S, Milone MC. CAR T Cell immunotherapy for human cancer. Science. (2018) 359:1361–5. doi: 10.1126/science.aar6711
12. Tang J, Hubbard-Lucey VM, Pearce L, O'Donnell-Tormey J, Shalabi A. The global landscape of cancer cell therapy. Nat Rev Drug Discov. (2018) 17:465–6. doi: 10.1038/nrd.2018.74
13. Maude SL, Laetsch TW, Buechner J, Rives S, Boyer M, Bittencourt H, et al. Tisagenlecleucel in children and young adults with B-cell lymphoblastic leukemia. N Engl J Med. (2018) 378:439–48. doi: 10.1056/NEJMoa1709866
14. FDA Approves Tisagenlecleucel for B-cell ALL Tocilizumab for Cytokine Release Syndrome: United States FDA. (2017) [09/07/2017]. Available online at: https://www.fda.gov/drugs/resources-information-approved-drugs/fda-approves-tisagenlecleucel-b-cell-all-and-tocilizumab-cytokine-release-syndrome (accessed May 01, 2023).
15. Schuster SJ, Bishop MR, Tam CS, Waller EK, Borchmann P, McGuirk JP, et al. Tisagenlecleucel in adult relapsed or refractory diffuse large B-cell lymphoma. N Engl J Med. (2018) 380:45–56. doi: 10.1056/NEJMoa1804980
16. FDA approves Tisagenlecleucel for adults with relapsed or refractory large B-cell lymphoma United States Food and Drug Administration (2018) [updated 05/03/2018]. Available online at: https://www.fda.gov/drugs/resources-information-approved-drugs/fda-approves-tisagenlecleucel-adults-relapsed-or-refractory-large-b-cell-lymphoma (accessed May 01, 2023).
17. Neelapu SS, Locke FL, Bartlett NL, Lekakis LJ, Miklos DB, Jacobson CA, et al. Axicabtagene ciloleucel CAR T-cell therapy in refractory large B-cell lymphoma. N Engl J Med. (2017) 377:2531–44. doi: 10.1056/NEJMoa1707447
18. Locke FL, Miklos DB, Jacobson CA, Perales MA, Kersten MJ, Oluwole OO, et al. Axicabtagene ciloleucel as second-line therapy for large B-cell lymphoma. N Engl J Med. (2021) 386:640–54. doi: 10.1056/NEJMoa2116133
19. Neelapu SS, Dickinson M, Munoz J, Ulrickson ML, Thieblemont C, Oluwole OO, et al. Primary analysis of ZUMA-12: a phase 2 study of axicabtagene ciloleucel (Axi-Cel) as first-line therapy in patients with high-risk large B-cell lymphoma (LBCL). Blood. (2021) 138:739. doi: 10.1182/blood-2021-148009
20. Abramson JS, Palomba ML, Gordon LI, Lunning MA, Wang M, Arnason J, et al. Lisocabtagene maraleucel for patients with relapsed or refractory large B-cell lymphomas (TRANSCEND NHL 001): a multicentre seamless design study. Lancet. (2020) 396:839–52. doi: 10.1016/S0140-6736(20)31366-0
21. Kamdar M, Solomon SR, Arnason J, Johnston PB, Glass B, Bachanova V, et al. Lisocabtagene maraleucel versus standard of care with salvage chemotherapy followed by autologous stem cell transplantation as second-line treatment in patients with relapsed or refractory large B-cell lymphoma (TRANSFORM): results from an interim analysis of an open-label, randomised, phase 3 trial. Lancet. (2022) 399:2294–308. doi: 10.1016/S0140-6736(22)00662-6
22. Wang M, Munoz J, Goy A, Locke FL, Jacobson CA, Hill BT, et al. Three-year follow-up of KTE-X19 in patients with relapsed/refractory mantle cell lymphoma, including high-risk subgroups, in the ZUMA-2 study. J Clin Oncol. (2023) 41:555–67. doi: 10.1200/JCO.21.02370
23. Shah BD, Ghobadi A, Oluwole OO, Logan AC, Boissel N, Cassaday RD, et al. KTE-X19 for relapsed or refractory adult B-cell acute lymphoblastic leukaemia: phase 2 results of the single-arm, open-label, multicentre ZUMA-3 study. Lancet. (2021) 398:491–502. doi: 10.1016/S0140-6736(21)01222-8
24. Munshi NC, Anderson LD Jr, Shah N, Madduri D, Berdeja J, Lonial S, et al. Idecabtagene vicleucel in relapsed and refractory multiple myeloma. N Engl J Med. (2021) 384:705–16. doi: 10.1056/NEJMoa2024850
25. Berdeja JG, Madduri D, Usmani SZ, Jakubowiak A, Agha M, Cohen AD, et al. Ciltacabtagene autoleucel, a B-cell maturation antigen-directed chimeric antigen receptor T-cell therapy in patients with relapsed or refractory multiple myeloma (CARTITUDE-1): a phase 1b/2 open-label study. Lancet. (2021) 398:314–24. doi: 10.1016/S0140-6736(21)00933-8
26. Spiegel JY, Patel S, Muffly L, Hossain NM, Oak J, Baird JH, et al. CAR T cells with dual targeting of CD19 and CD22 in adult patients with recurrent or refractory B cell malignancies: a phase 1 trial. Nat Med. (2021) 27:1419–31. doi: 10.1038/s41591-021-01436-0
27. Julio C Chavez FY, Jose Sandoval-Sus, Mohamed A Kharfan-Dabaja. Anti-CD19 chimeric antigen receptor T-cell therapy in B-cell lymphomas: current status and future directions. Int J Hematol Oncol. (2021) 10:IJH33. doi: 10.2217/ijh-2020-0021
28. First-ever CAR T-cell therapy approved in U.S. Cancer Discov. (2017) 7:OF1. doi: 10.1158/2159-8290.CD-NB2017-126
29. Holstein SA, Lunning MA, CAR. T-cell therapy in hematologic malignancies: a voyage in progress. Clin Pharmacol Ther. (2020) 107:112–22. doi: 10.1002/cpt.1674
30. Teoh PJ, Chng WJ, CAR. T-cell therapy in multiple myeloma: more room for improvement. Blood Cancer J. (2021) 11:84. doi: 10.1038/s41408-021-00469-5
31. Patel U, Abernathy J, Savani BN, Oluwole O, Sengsayadeth S, Dholaria B, et al. cell therapy in solid tumors: a review of current clinical trials. eJHaem. (2022) 3:24–31. doi: 10.1002/jha2.356
32. Kankeu Fonkoua LA, Sirpilla O, Sakemura R, Siegler EL, Kenderian SS. CAR T cell therapy and the tumor microenvironment: current challenges and opportunities. Mol Ther Oncolytics. (2022) 25:69–77. doi: 10.1016/j.omto.2022.03.009
33. Bonifant CL, Jackson HJ, Brentjens RJ, Curran KJ. Toxicity and management in CAR T-cell therapy. Mol Ther Oncolytics. (2016) 3:16011. doi: 10.1038/mto.2016.11
34. Shah NN, Fry TJ. Mechanisms of resistance to CAR T cell therapy. Nat Rev Clin Oncol. (2019) 16:372–85. doi: 10.1038/s41571-019-0184-6
35. Sterner RC, Sterner RM. CAR-T cell therapy: current limitations and potential strategies. Blood Cancer J. (2021) 11:69. doi: 10.1038/s41408-021-00459-7
36. Bai R, Chen N, Li L, Du N, Bai L, Lv Z, et al. Mechanisms of cancer resistance to immunotherapy. Front Oncol. (2020) 10:1290. doi: 10.3389/fonc.2020.01290
37. Cheng J, Zhao L, Zhang Y, Qin Y, Guan Y, Zhang T, et al. Understanding the mechanisms of resistance to CAR T-cell therapy in malignancies. Front Oncol. (2019) 9:1237. doi: 10.3389/fonc.2019.01237
38. Hong R, Hu Y, Huang H. Biomarkers for chimeric antigen receptor T cell therapy in acute lymphoblastic leukemia: prospects for personalized management and prognostic prediction. Front Immunol. (2021) 12:627764. doi: 10.3389/fimmu.2021.627764
39. Gerlinger M, Rowan AJ, Horswell S, Math M, Larkin J, Endesfelder D, et al. Intratumor heterogeneity and branched evolution revealed by multiregion sequencing. N Engl J Med. (2012) 366:883–92. doi: 10.1056/NEJMoa1113205
40. Rahmim A, Zaidi H. PET versus SPECT: strengths, limitations and challenges. Nuc Med Comm. (2008) 29:193–207. doi: 10.1097/MNM.0b013e3282f3a515
41. Mulgaonkar A, Elias R, Woolford L, Guan B, Nham K, Kapur P, et al. ImmunoPET imaging with 89Zr-labeled atezolizumab enables in vivo evaluation of PD-L1 in tumorgraft models of renal cell carcinoma. Clin Cancer Res. (2022) 28:4907–16. doi: 10.1158/1078-0432.CCR-22-1547
42. Hao G, Mastren T, Silvers W, Hassan G, Öz OK, Sun X. Copper-67 radioimmunotheranostics for simultaneous immunotherapy and immuno-SPECT. Sci Rep. (2021) 11:3622. doi: 10.1038/s41598-021-82812-1
43. Seo JW, Tavaré R, Mahakian LM, Silvestrini MT, Tam S, Ingham ES, et al. CD8+ T-Cell censity imaging with 64Cu-labeled cys-diabody informs immunotherapy protocols. Clin Cancer Res. (2018) 24:4976–87. doi: 10.1158/1078-0432.CCR-18-0261
44. Simonetta F, Alam IS, Lohmeyer JK, Sahaf B, Good Z, Chen W, et al. Molecular imaging of chimeric antigen receptor T cells by ICOS-immunoPET. Clin Cancer Res. (2021) 27:1058–68. doi: 10.1158/1078-0432.CCR-20-2770
45. Wu AM, Pandit-Taskar N. ImmunoPET: harnessing antibodies for imaging immune cells. Mol Imaging Biol. (2022) 24:181–97. doi: 10.1007/s11307-021-01652-7
46. Hu Y, Huang J. The chimeric antigen receptor detection toolkit. Front Immunol. (2020) 11:1770. doi: 10.3389/fimmu.2020.01770
47. Lopci E, Hicks RJ, Dimitrakopoulou-Strauss A, Dercle L, Iravani A, Seban RD, et al. Joint EANM/SNMMI/ANZSNM practice guidelines/procedure standards on recommended use of [18F]FDG PET/CT imaging during immunomodulatory treatments in patients with solid tumors version 10. Eur J Nuc Med Mol Imag. (2022) 49:2323–41. doi: 10.1007/s00259-022-05780-2
48. Parihar AS, Dehdashti F, Wahl RL. FDG PET/CT-based response assessment in malignancies. Radiographics. (2023) 43:e220122. doi: 10.1148/rg.220122
49. Shah NN, Nagle SJ, Torigian DA, Farwell MD, Hwang WT, Frey N, et al. Early positron emission tomography/computed tomography as a predictor of response after CTL019 chimeric antigen receptor -T-cell therapy in B-cell non-Hodgkin lymphomas. Cytotherapy. (2018) 20:1415–8. doi: 10.1016/j.jcyt.2018.10.003
50. Wang J, Hu Y, Yang S, Wei G, Zhao X, Wu W, et al. Role of fluorodeoxyglucose positron emission tomography/computed tomography in predicting the adverse effects of chimeric antigen receptor T cell therapy in patients with non-Hodgkin lymphoma. Biol Blood Marrow Transplant. (2019) 25:1092–8. doi: 10.1016/j.bbmt.2019.02.008
51. Volpe A, Adusumilli PS, Schoder H, Ponomarev V. Imaging cellular immunotherapies and immune cell biomarkers: from preclinical studies to patients. J Immunother Cancer. (2022) 10:e004902. doi: 10.1136/jitc-2022-004902
52. Wei W, Rosenkrans ZT, Liu J, Huang G, Luo Q-Y, Cai W. ImmunoPET: concept, design, and applications. Chem Rev. (2020) 120:3787–851. doi: 10.1021/acs.chemrev.9b00738
53. Lugat A, Bailly C, Chérel M, Rousseau C, Kraeber-Bodéré F, Bodet-Milin C, et al. Immuno-PET: design options and clinical proof-of-concept. Front Med. (2022) 9:1026083. doi: 10.3389/fmed.2022.1026083
54. Maude SL, Teachey DT, Rheingold SR, Shaw PA, Aplenc R, Barrett DM, et al. Sustained remissions with CD19-specific chimeric antigen receptor (CAR)-modified T cells in children with relapsed/refractory ALL. Am Soc Clin Oncol. (2016) 34(15_suppl):3011. doi: 10.1200/JCO.2016.34.15_suppl.3011
55. Lee DW, Kochenderfer JN, Stetler-Stevenson M, Cui YK, Delbrook C, Feldman SA, et al. T cells expressing CD19 chimeric antigen receptors for acute lymphoblastic leukaemia in children and young adults: a phase 1 dose-escalation trial. Lancet. (2015) 385:517–28. doi: 10.1016/S0140-6736(14)61403-3
56. Gardner RA, Finney O, Annesley C, Brakke H, Summers C, Leger K, et al. Intent-to-treat leukemia remission by CD19 CAR T cells of defined formulation and dose in children and young adults. Blood. (2017) 129:3322–31. doi: 10.1182/blood-2017-02-769208
57. Majzner RG, Mackall CL. Tumor antigen escape from CAR T-cell therapy. Cancer Discov. (2018) 8:1219–26. doi: 10.1158/2159-8290.CD-18-0442
58. Turtle CJ, Hanafi L-A, Berger C, Gooley TA, Cherian S, Hudecek M, et al. CD19 CAR–T cells of defined CD4+: CD8+ composition in adult B cell ALL patients. J Clin Investig. (2016) 126:2123–38. doi: 10.1172/JCI85309
59. Park JH, Rivière I, Gonen M, Wang X, Sénéchal B, Curran KJ, et al. Long-term follow-up of CD19 CAR therapy in acute lymphoblastic leukemia. N Engl J Med. (2018) 378:449–59. doi: 10.1056/NEJMoa1709919
60. Subklewe M. BiTEs better than CAR T cells. Blood Adv. (2021) 5:607–12. doi: 10.1182/bloodadvances.2020001792
61. Asnani M, Hayer KE, Naqvi AS, Zheng S, Yang SY, Oldridge D, et al. Retention of CD19 intron 2 contributes to CART-19 resistance in leukemias with subclonal frameshift mutations in CD19. Leukemia. (2020) 34:1202–7. doi: 10.1038/s41375-019-0580-z
62. Orlando EJ, Han X, Tribouley C, Wood PA, Leary RJ, Riester M, et al. Genetic mechanisms of target antigen loss in CAR19 therapy of acute lymphoblastic leukemia. Nat Med. (2018) 24:1504–6. doi: 10.1038/s41591-018-0146-z
63. Sotillo E, Barrett DM, Black KL, Bagashev A, Oldridge D, Wu G, et al. Convergence of acquired mutations and alternative splicing of CD19 enables resistance to CART-19 immunotherapy. Cancer Discov. (2015) 5:1282–95. doi: 10.1158/2159-8290.CD-15-1020
64. van de Donk NWCJ, Themeli M, Usmani SZ. Determinants of response and mechanisms of resistance of CAR T-cell therapy in multiple myeloma. Blood Can Discov. (2021) 2:302–18. doi: 10.1158/2643-3230.BCD-20-0227
65. O'Rourke DM, Nasrallah MP, Desai A, Melenhorst JJ, Mansfield K, Morrissette JJD, et al. A single dose of peripherally infused EGFRvIII-directed CAR T cells mediates antigen loss and induces adaptive resistance in patients with recurrent glioblastoma. Sci Translat Med. (2017) 9:eaaa0984. doi: 10.1126/scitranslmed.aaa0984
66. Brown CE, Alizadeh D, Starr R, Weng L, Wagner JR, Naranjo A, et al. Regression of glioblastoma after chimeric antigen receptor T-cell therapy. N Engl J Med. (2016) 375:2561–9. doi: 10.1056/NEJMoa1610497
67. Liao W, Kohler ME, Fry T, Ernst P. Does lineage plasticity enable escape from CAR-T cell therapy? Lessons from MLL-r leukemia. Exp Hematol. (2021) 100:1–11. doi: 10.1016/j.exphem.2021.07.002
68. Gardner R, Wu D, Cherian S, Fang M, Hanafi L-A, Finney O, et al. Acquisition of a CD19-negative myeloid phenotype allows immune escape of MLL-rearranged B-ALL from CD19 CAR-T-cell therapy. Blood, J Am Soc Hematol. (2016) 127:2406–10. doi: 10.1182/blood-2015-08-665547
69. Selli ME, Dalal P, Neelapu SS, Singh N. Mechanisms of resistance and relapse after CAR-T cell therapy. In:Ghobadi A, DiPersio JF, , editors. Gene and Cellular Immunotherapy for Cancer. Cham: Springer International Publishing (2022), p. 207–19. doi: 10.1007/978-3-030-87849-8_12
70. Zoghbi A, Zur Stadt U, Winkler B, Müller I, Escherich G. Lineage switch under blinatumomab treatment of relapsed common acute lymphoblastic leukemia without MLL rearrangement. Ped Blood Cancer. (2017) 64:e26594. doi: 10.1002/pbc.26594
71. He RR, Nayer Z, Hogan M, Cuevo RS, Woodward K, Heyer D, et al. Immunotherapy-(blinatumomab-) related lineage switch of KMT2A/AFF1 rearranged B-lymphoblastic leukemia into acute myeloid leukemia/myeloid sarcoma and subsequently into B/myeloid mixed phenotype acute leukemia. Case Rep Hematol. (2019) 2019:7394619. doi: 10.1155/2019/7394619
72. Nagel I, Bartels M, Duell J, Oberg H-H, Ussat S, Bruckmueller H, et al. Hematopoietic stem cell involvement in BCR-ABL1–positive ALL as a potential mechanism of resistance to blinatumomab therapy. Blood. (2017) 130:2027–31. doi: 10.1182/blood-2017-05-782888
73. Du J, Chisholm KM, Tsuchiya K, Leger K, Lee BM, Rutledge JC, et al. Lineage switch in an infant B-lymphoblastic leukemia with t (1; 11)(p32; q23); KMT2A/EPS15, following blinatumomab therapy. Ped Dev Pathol. (2021) 24:378–82. doi: 10.1177/10935266211001308
74. Rath A, Panda T, Dhawan R, Dass J, Mahapatra M, Viswanathan GK. A paradigm shift: lineage switch from T-ALL to B/myeloid MPAL. Blood Res. (2021) 56:50–3. doi: 10.5045/br.2021.2020268
75. Hamieh M, Dobrin A, Cabriolu A, van der Stegen SJ, Giavridis T, Mansilla-Soto J, et al. CAR T cell trogocytosis and cooperative killing regulate tumour antigen escape. Nature. (2019) 568:112–6. doi: 10.1038/s41586-019-1054-1
76. Olson ML, Mause ERV, Radhakrishnan SV, Brody JD, Rapoport AP, Welm AL, et al. Low-affinity CAR T cells exhibit reduced trogocytosis, preventing rapid antigen loss, and increasing CAR T cell expansion. Leukemia. (2022) 36:1943–6. doi: 10.1038/s41375-022-01585-2
77. Dance A. Cells nibble one another via the under-appreciated process of trogocytosis. Proc Natl Acad Sci. (2019) 116:17608–10. doi: 10.1073/pnas.1912252116
78. Walker AJ, Majzner RG, Zhang L, Wanhainen K, Long AH, Nguyen SM, et al. Tumor antigen and receptor densities regulate efficacy of a chimeric antigen receptor targeting anaplastic lymphoma kinase. Mol Ther. (2017) 25:2189–201. doi: 10.1016/j.ymthe.2017.06.008
79. Watanabe K, Terakura S, Martens AC, Van Meerten T, Uchiyama S, Imai M, et al. Target antigen density governs the efficacy of anti–CD20-CD28-CD3 ζ chimeric antigen receptor–modified effector CD8+ T cells. J Immunol. (2015) 194:911–20. doi: 10.4049/jimmunol.1402346
80. Sun R, Medeiros LJ, Young KH. Diagnostic and predictive biomarkers for lymphoma diagnosis and treatment in the era of precision medicine. Modern Pathol. (2016) 29:1118–42. doi: 10.1038/modpathol.2016.92
81. Jauw YWS, Zijlstra JM, de Jong D, Vugts DJ, Zweegman S, Hoekstra OS, et al. Performance of 89Zr-labeled-rituximab-PET as an imaging biomarker to assess CD20 targeting: a pilot study in patients with relapsed/refractory diffuse large B cell lymphoma. PLoS ONE. (2017) 12:e0169828. doi: 10.1371/journal.pone.0169828
82. Lee I, Lim I, Lee KC, Kang HJ, Lim SM. 64Cu-DOTA-rituximab PET/CT of B-cell non-Hodgkin lymphoma for imaging the CD20 expression. Clin Nuc Med. (2023) 48:e82–3. doi: 10.1097/RLU.0000000000004378
83. Stevens MY, Cropper HC, Lucot KL, Chaney AM, Lechtenberg KJ, Jackson IM, et al. Development of a CD19 PET tracer for detecting B cells in a mouse model of multiple sclerosis. J Neuroinflam. (2020) 17:275. doi: 10.1186/s12974-020-01880-8
84. der Houven CWM-v, Gootjes EC, Huisman MC, Vugts DJ, Roth C, Luik AM, et al. 89Zr-cetuximab PET imaging in patients with advanced colorectal cancer. Oncotarget. (2015) 6:30384–93. doi: 10.18632/oncotarget.4672
85. Lindenberg L, Adler S, Turkbey IB, Mertan F, Ton A, Do K, et al. Dosimetry and first human experience with 89Zr-panitumumab. Am J Nucl Med Mol Imaging. (2017) 7:195–203.
86. Stone LD, Massicano AVF, Stevens TM, Warram JM, Morlandt AB, Lapi SE, et al. 89Zr-panitumumab PET imaging for preoperative assessment of ameloblastoma in a PDX model. Sci Rep. (2022) 12:19187. doi: 10.1038/s41598-022-23531-z
87. Gebhart G, Lamberts L, Wimana Z, Garcia C, Emonts P, Ameye L, et al. Molecular imaging as a tool to investigate heterogeneity of advanced HER2-positive breast cancer and to predict patient outcome under trastuzumab emtansine (T-DM1): the ZEPHIR trial. Ann Oncol. (2016) 27:619–24. doi: 10.1093/annonc/mdv577
88. Ulaner GA, Hyman DM, Lyashchenko SK, Lewis JS, Carrasquillo JA. 89Zr-trastuzumab PET/CT for detection of human epidermal growth factor receptor 2-positive metastases in patients with human epidermal growth factor receptor 2-negative primary breast cancer. Clin Nuc Med. (2017) 42:912–7. doi: 10.1097/RLU.0000000000001820
89. Lumish MA, Maron SB, Paroder V, Chou JF, Capanu M, Philemond S, et al. Noninvasive assessment of human epidermal growth factor receptor 2 (HER2) in esophagogastric cancer using 89Zr-trastuzumab PET: a pilot study. J Nuc Med. (2023) 64:724–30. doi: 10.2967/jnumed.122.264470
90. Lam K, Chan C, Done SJ, Levine MN, Reilly RM. Preclinical pharmacokinetics, biodistribution, radiation dosimetry and acute toxicity studies required for regulatory approval of a cinical trial application for a Phase I/II clinical trial of 111In-BzDTPA-pertuzumab. Nuc Med Biol. (2015) 42:78–84. doi: 10.1016/j.nucmedbio.2014.09.011
91. Pandit-Taskar N, O'Donoghue JA, Durack JC, Lyashchenko SK, Cheal SM, Beylergil V, et al. A phase I/II study for analytic validation of 89Zr-J591 immunoPET as a molecular imaging agent for metastatic prostate cancer. Clin Cancer Res. (2015) 21:5277–85. doi: 10.1158/1078-0432.CCR-15-0552
92. Oosting SF, Brouwers AH, van Es SC, Nagengast WB, Munnink THO, Lub-de Hooge MN, et al. 89Zr-bevacizumab PET visualizes heterogeneous tracer accumulation in tumor lesions of renal cell carcinoma patients and differential effects of antiangiogenic treatment. J Nuc Med. (2015) 56:63–9. doi: 10.2967/jnumed.114.144840
93. Yoon J-K, Park B-N, Ryu E-K, An Y-S, Lee S-J. Current perspectives on 89Zr-PET imaging. Int J Mol Sc. (2020) 21:4309. doi: 10.3390/ijms21124309
94. Skhoun H, Khattab M, Chebihi ZT, Belkhayat A, Dakka N, BaghdadI JE. B/T mixed phenotype acute leukemia with high hyperdiploidy and lineage switch to B-cell acute leukemia. Leuk Res Rep. (2022) 17:100289. doi: 10.1016/j.lrr.2022.100289
95. Berman DS, Kang X, Tamarappoo B, Wolak A, Hayes SW, Nakazato R, et al. Stress thallium-201/rest technetium-99m sequential dual isotope high-speed myocardial perfusion imaging. JACC: Cardiovasc Imaging. (2009) 2:273–82. doi: 10.1016/j.jcmg.2008.12.012
96. Caobelli F, Wollenweber T, Bavendiek U, Kühn C, Schütze C, Geworski L, et al. Simultaneous dual-isotope solid-state detector SPECT for improved tracking of white blood cells in suspected endocarditis. Eur Heart J. (2016) 38:436–43. doi: 10.1093/eurheartj/ehw231
97. Hsieh PC, Lee IH, Yeh TL, Chen KC, Huang HC, Chen PS, et al. Distribution volume ratio of serotonin and dopamine transporters in euthymic patients with a history of major depression—a dual-isotope SPECT study. Psychiatry Res Neuroimaging. (2010) 184:157–61. doi: 10.1016/j.pscychresns.2010.09.004
98. Bellevre D, Manrique A, Legallois D, Bross S, Baavour R, Roth N, et al. First determination of the heart-to-mediastinum ratio using cardiac dual isotope (123I-MIBG/99mTc-tetrofosmin) CZT imaging in patients with heart failure: the ADRECARD study. Eur J Nuc Med Mol Imag. (2015) 42:1912–9. doi: 10.1007/s00259-015-3141-3
99. Quintana JC, Blend MJ. The dual-isotope prostascint imaging procedure: clinical experience and staging results in 145 patients. Clin Nuc Med. (2000) 25:33. doi: 10.1097/00003072-200001000-00008
100. Hijnen NM, de Vries A, Nicolay K, Grüll H. Dual-isotope 111In/177Lu SPECT imaging as a tool in molecular imaging tracer design. Contrast Media Mol Imag. (2012) 7:214–22. doi: 10.1002/cmmi.485
101. Hendrikx G, Saint-Hubert D, Dijkgraaf I, Bauwens M, Douma K, Wierts R, et al. Molecular imaging of angiogenesis after myocardial infarction by 111In-DTPA-cNGR and 99mTc-sestamibi dual-isotope myocardial SPECT. EJNMMI Res. (2015) 5:1–10. doi: 10.1186/s13550-015-0081-7
102. Welling MM, Spa SJ, van Willigen DM, Rietbergen DD, Roestenberg M, Buckle T, et al. In vivo stability of supramolecular host–guest complexes monitored by dual-isotope multiplexing in a pre-targeting model of experimental liver radioembolization. J Contr Rel. (2019) 293:126–34. doi: 10.1016/j.jconrel.2018.11.020
103. Uenomachi M, Shimazoe K, Ogane K, Takahashi H. Simultaneous multi-nuclide imaging via double-photon coincidence method with parallel hole collimators. Sci Rep. (2021) 11:13330. doi: 10.1038/s41598-021-92583-4
104. Zhang Z, Liu S, Zhang B, Qiao L, Zhang Y, Zhang Y. T cell dysfunction and exhaustion in cancer. Front Cell Develop Biol. (2020) 8:17. doi: 10.3389/fcell.2020.00017
105. Gumber D, Wang LD. Improving CAR-T immunotherapy: overcoming the challenges of T cell exhaustion. eBioMedicine. (2022) 77:103941. doi: 10.1016/j.ebiom.2022.103941
106. Zhao Y, Shao Q, Peng G. Exhaustion and senescence: two crucial dysfunctional states of T cells in the tumor microenvironment. Cell Mol Immunol. (2020) 17:27–35. doi: 10.1038/s41423-019-0344-8
107. Kasakovski D, Xu L, Li Y. T cell senescence and CAR-T cell exhaustion in hematological malignancies. J Hematol Oncol. (2018) 11:91. doi: 10.1186/s13045-018-0629-x
108. Adibzadeh M, Pohla H, Rehbein A, Pawelec G. Long-term culture of monoclonal human T lymphocytes: models for immunosenescence? Mechan Ageing Dev. (1995) 83:171–83. doi: 10.1016/0047-6374(95)01625-A
109. Effros RB. Replicative senescence in the immune system: impact of the Hayflick limit on T-cell function in the elderly. Am J Hum Gen. (1998) 62:1003–7. doi: 10.1086/301845
110. Poorebrahim M, Melief J, Pico de Coana Y, S LW, Cid-Arregui A, Kiessling R. Counteracting CAR T cell dysfunction. Oncogene. (2021) 40:421–35. doi: 10.1038/s41388-020-01501-x
111. Jiang Y, Li Y, Zhu B. T-cell exhaustion in the tumor microenvironment. Cell Death Dis. (2015) 6:e1792. doi: 10.1038/cddis.2015.162
112. Yao D, Xu L, Tan J, Zhang Y, Lu S, Li M, et al. Re-balance of memory T cell subsets in peripheral blood from patients with CML after TKI treatment. Oncotarg. (2017) 8:81852–9. doi: 10.18632/oncotarget.20965
113. Tang L, Zhang Y, Hu Y, Mei H. T cell exhaustion and CAR-T immunotherapy in hematological malignancies. BioMed Res Int. (2021) 2021:6616391. doi: 10.1155/2021/6616391
114. Spranger S, Spaapen RM, Zha Y, Williams J, Meng Y, Ha TT, et al. Up-regulation of PD-L1, IDO, and T(regs) in the melanoma tumor microenvironment is driven by CD8(+) T cells. Sci Translat Med. (2013) 5:200ra116. doi: 10.1126/scitranslmed.3006504
115. Schlichtner S, Yasinska IM, Ruggiero S, Berger SM, Aliu N, Prunk M, et al. Expression of the immune checkpoint protein VISTA Is differentially regulated by the TGF-β1 – Smad3 signaling pathway in rapidly proliferating human cells and T lymphocytes. Front Med. (2022) 9:790995. doi: 10.3389/fmed.2022.790995
116. Zuazo M, Gato-Cañas M, Llorente N, Ibañez-Vea M, Arasanz H, Kochan G, et al. Molecular mechanisms of programmed cell death-1 dependent T cell suppression: relevance for immunotherapy. Ann Transl Med. (2017) 5:385. doi: 10.21037/atm.2017.06.11
117. Zolov SN, Rietberg SP. Bonifant CL. Programmed cell death protein 1 activation preferentially inhibits CD28CAR-T cells. Cytotherapy. (2018) 20:1259–66. doi: 10.1016/j.jcyt.2018.07.005
118. Hui E, Cheung J, Zhu J, Su X, Taylor MJ, Wallweber HA, et al. T cell costimulatory receptor CD28 is a primary target for PD-1-mediated inhibition. Science. (2017) 355:1428–33. doi: 10.1126/science.aaf1292
119. Cherkassky L, Morello A, Villena-Vargas J, Feng Y, Dimitrov DS, Jones DR, et al. Human CAR T cells with cell-intrinsic PD-1 checkpoint blockade resist tumor-mediated inhibition. J Clin Investig. (2016) 126:3130–44. doi: 10.1172/JCI83092
120. Chong EA, Svoboda J, Nasta SD, Landsburg DJ, Winchell N, Napier E, et al. Sequential anti-CD19 directed chimeric antigen receptor modified T-cell therapy (CART19) and PD-1 blockade with pembrolizumab in patients with relapsed or refractory B-cell non-Hodgkin lymphomas. Blood. (2018) 132:4198. doi: 10.1182/blood-2018-99-119502
121. Chong EA, Melenhorst JJ, Lacey SF, Ambrose DE, Gonzalez V, Levine BL, et al. PD-1 blockade modulates chimeric antigen receptor (CAR)–modified T cells: refueling the CAR. Blood. (2017) 129:1039–41. doi: 10.1182/blood-2016-09-738245
122. Maude SL, Hucks GE, Seif AE, Talekar MK, Teachey DT, Baniewicz D, et al. The effect of pembrolizumab in combination with CD19-targeted chimeric antigen receptor (CAR) T cells in relapsed acute lymphoblastic leukemia (ALL). Am Soc Clin Oncol. (2017) 35:103. doi: 10.1200/JCO.2017.35.15_suppl.103
123. Li AM, Hucks GE, Dinofia AM, Seif AE, Teachey DT, Baniewicz D, et al. Checkpoint inhibitors augment CD19-directed chimeric antigen receptor (CAR) T cell therapy in relapsed B-cell acute lymphoblastic leukemia. Blood. (2018) 132:556. doi: 10.1182/blood-2018-99-112572
124. Grosser R, Cherkassky L, Chintala N, Adusumilli PS. Combination immunotherapy with CAR T cells and checkpoint blockade for the treatment of solid tumors. Cancer Cell. (2019) 36:471–82. doi: 10.1016/j.ccell.2019.09.006
125. Rafiq S, Yeku OO, Jackson HJ, Purdon TJ, van Leeuwen DG, Drakes DJ, et al. Targeted delivery of a PD-1-blocking scFv by CAR-T cells enhances anti-tumor efficacy in vivo. Nat Biotech. (2018) 36:847–56. doi: 10.1038/nbt.4195
126. Rafiq S, Hackett CS, Brentjens RJ. Engineering strategies to overcome the current roadblocks in CAR T cell therapy. Nat Rev Clin Oncol. (2020) 17:147–67. doi: 10.1038/s41571-019-0297-y
127. Liu X, Zhang Y, Li K, Liu Y, Xu J, Ma J, et al. A novel dominant-negative PD-1 armored anti-CD19 CAR T cell is safe and effective against refractory/relapsed B cell lymphoma. Translat Oncol. (2021) 14:101085. doi: 10.1016/j.tranon.2021.101085
128. Niemeijer A-LN, Oprea-Lager DE, Huisman MC, Hoekstra OS, Boellaard R, de Wit-van der Veen BJ, et al. Study of 89Zr-Pembrolizumab PET/CT in patients with advanced-stage non–small cell lung cancer. J Nuc Med. (2022) 63:362–7. doi: 10.2967/jnumed.121.261926
129. Niemeijer AN, Leung D, Huisman MC, Bahce I, Hoekstra OS, van Dongen GAMS, et al. Whole body PD-1 and PD-L1 positron emission tomography in patients with non-small-cell lung cancer. Nat Comm. (2018) 9:4664. doi: 10.1038/s41467-018-07131-y
130. Bensch F, van der Veen EL, Lub-de Hooge MN, Jorritsma-Smit A, Boellaard R, Kok IC, et al. 89Zr-Atezolizumab imaging as a non-invasive approach to assess clinical response to PD-L1 blockade in cancer. Nat Med. (2018) 24:1852–8. doi: 10.1038/s41591-018-0255-8
131. Vento J, Mulgaonkar A, Woolford L, Nham K, Christie A, Bagrodia A, et al. PD-L1 detection using 89Zr-atezolizumab immuno-PET in renal cell carcinoma tumorgrafts from a patient with favorable nivolumab response. J ImmunoTher Cancer. (2019) 7:144. doi: 10.1186/s40425-019-0607-z
132. Hegi-Johnson F, Rudd SE, Wichmann C, Akhurst T, Roselt P, Trinh J, et al. ImmunoPET: imaging of cancer immunotherapy targets with positron emission tomography: a phase 0/1 study characterising PD-L1 with 89Zr-durvalumab (MEDI4736) PET/CT in stage III NSCLC patients receiving chemoradiation study protocol. BMJ Open. (2022) 12:e056708. doi: 10.1136/bmjopen-2021-056708
133. Ehlerding EB, England CG, Majewski RL, Valdovinos HF, Jiang D, Liu G, et al. ImmunoPET imaging of CTLA-4 expression in mouse models of non-small cell lung cancer. Mol Pharmaceut. (2017) 14:1782–9. doi: 10.1021/acs.molpharmaceut.7b00056
134. Higashikawa K, Yagi K, Watanabe K, Kamino S, Ueda M, Hiromura M, et al. 64Cu-DOTA-anti-CTLA-4 mAb enabled PET visualization of CTLA-4 on the T-cell infiltrating tumor tissues. PLoS ONE. (2014) 9:e109866. doi: 10.1371/journal.pone.0109866
135. Robu S, Richter A, Gosmann D, Seidl C, Leung D, Hayes W, et al. Synthesis and preclinical evaluation of a 68Ga-labeled adnectin, 68Ga-BMS-986192, as a PET agent for imaging PD-L1 expression. J Nuc Med. (2021) 62:1228–34. doi: 10.2967/jnumed.120.258384
136. Stutvoet TS, van der Veen EL, Kol A, Antunes IF, de Vries EFJ, Hospers GAP, et al. Molecular imaging of PD-L1 expression and dynamics with the adnectin-based PET tracer 18F-BMS-986192. J Nuc Med. (2020) 61:1839–44. doi: 10.2967/jnumed.119.241364
137. Donnelly DJ, Smith RA, Morin P, Lipovšek D, Gokemeijer J, Cohen D, et al. Synthesis and biologic evaluation of a novel 18F-labeled adnectin as a PET radioligand for imaging PD-L1 expression. J Nuc Med. (2018) 59:529–35. doi: 10.2967/jnumed.117.199596
138. Shaffer T, Natarajan A, Gambhir SS. PET imaging of TIGIT expression on tumor-infiltrating lymphocytes. Clin Cancer Res. (2021) 27:1932–40. doi: 10.1158/1078-0432.CCR-20-2725
139. Rotte A, Sahasranaman S, Budha N. Targeting TIGIT for immunotherapy of cancer: update on clinical development. Biomedicines. (2021) 9:1277. doi: 10.3390/biomedicines9091277
140. Miedema IHC, Huisman MC, Zwezerijnen GJC, Grempler R, Pitarch AP, Thiele A, et al. 89Zr-immuno-PET using the anti-LAG-3 tracer [89Zr]Zr-BI 754111: demonstrating target specific binding in NSCLC and HNSCC. Eur J Nuc Med Mol Imag. (2023) 50:2068–80. doi: 10.1007/s00259-023-06164-w
141. Wei W, Jiang D, Lee HJ, Engle JW, Akiba H, Liu J, et al. ImmunoPET imaging of TIM-3 in murine melanoma models. Adv Ther. (2020) 3:2000018. doi: 10.1002/adtp.202000018
142. Blessin NC, Simon R, Kluth M, Fischer K, Hube-Magg C, Li W, et al. Patterns of TIGIT expression in lymphatic tissue, inflammation, and cancer. Dis Markers. (2019) 2019:5160565. doi: 10.1155/2019/5160565
143. Chauvin J-M, Zarour HM. TIGIT in cancer immunotherapy. J ImmunoTher Cancer. (2020) 8:e000957. doi: 10.1136/jitc-2020-000957
144. Lee YH, Lee HJ, Kim HC, Lee Y, Nam SK, Hupperetz C, et al. PD-1 and TIGIT downregulation distinctly affect the effector and early memory phenotypes of CD19-targeting CAR T cells. Mol Ther. (2022) 30:579–92. doi: 10.1016/j.ymthe.2021.10.004
145. Tu L, Guan R, Yang H, Zhou Y, Hong W, Ma L, et al. Assessment of the expression of the immune checkpoint molecules PD-1, CTLA4, TIM-3 and LAG-3 across different cancers in relation to treatment response, tumor-infiltrating immune cells and survival. Int J Cancer. (2020) 147:423–39. doi: 10.1002/ijc.32785
146. Ye J, Peng G. Controlling T cell senescence in the tumor microenvironment for tumor immunotherapy. Oncoimmunol. (2015) 4:e994398. doi: 10.4161/2162402X.2014.994398
147. Liu X, Hoft DF, Peng G. Senescent T cells within suppressive tumor microenvironments: emerging target for tumor immunotherapy. J Clin Investig. (2020) 130:1073–83. doi: 10.1172/JCI133679
148. Vallejo AN. CD28 extinction in human T cells: altered functions and the program of T-cell senescence. Immunol Rev. (2005) 205:158–69. doi: 10.1111/j.0105-2896.2005.00256.x
149. Heffner M, Fearon DT. Loss of T cell receptor-induced Bmi-1 in the KLRG1+ senescent CD8+ T lymphocyte. Proc Natl Acad Sci. (2007) 104:13414–9. doi: 10.1073/pnas.0706040104
150. Huang X, Bai X, Cao Y, Wu J, Huang M, Tang D, et al. Lymphoma endothelium preferentially expresses Tim-3 and facilitates the progression of lymphoma by mediating immune evasion. J Exp Med. (2010) 207:505–20. doi: 10.1084/jem.20090397
151. Li H, Wu K, Tao K, Chen L, Zheng Q, Lu X, et al. Tim-3/galectin-9 signaling pathway mediates T-cell dysfunction and predicts poor prognosis in patients with hepatitis B virus-associated hepatocellular carcinoma. Hepatology. (2012) 56:1342–51. doi: 10.1002/hep.25777
152. Brenchley JM, Karandikar NJ, Betts MR, Ambrozak DR, Hill BJ, Crotty LE, et al. Expression of CD57 defines replicative senescence and antigen-induced apoptotic death of CD8+ T cells. Blood. (2003) 101:2711–20. doi: 10.1182/blood-2002-07-2103
153. Ye J, Huang X, Hsueh EC, Zhang Q, Ma C, Zhang Y, et al. Human regulatory T cells induce T-lymphocyte senescence. Blood. (2012) 120:2021–31. doi: 10.1182/blood-2012-03-416040
154. Ye J, Ma C, Hsueh EC, Eickhoff CS, Zhang Y, Varvares MA, et al. Tumor-derived γδ regulatory T cells suppress innate and adaptive immunity through the induction of immunosenescence. J Immunol. (2013) 190:2403–14. doi: 10.4049/jimmunol.1202369
155. Krueger MA, Cotton JM, Zhou B, Wolter K, Kuehn A, Schwenck J, et al. Abstract LB-369: in vivo imaging of tumor senescence with a novel beta-galactosidase specific PET tracer. Cancer Res. (2018) 78(13_Supplement):LB-369-LB. doi: 10.1158/1538-7445.AM2018-LB-369
156. Schwenck J, Cotton J, Zhou B, Wolter K, Kuehn A, Fuchs K, et al. In vivo imaging of tumor senescence with a novel beta-galactosidase specific PET tracer. Nuklearmedizin. (2019) 58:L5. doi: 10.1055/s-0039-1683474
157. Brickute D, Chen C, Braga M, Barnes C, Wang N, Allott L, et al. Design, synthesis, and evaluation of a novel PET imaging agent targeting lipofuscin in senescent cells. RSC Adv. (2022) 12:26372–81. doi: 10.1039/D2RA04535D
158. van de Donk PP, Wind TT, Hooiveld-Noeken JS, van der Veen EL, Glaudemans AWJM, Diepstra A, et al. Interleukin-2 PET imaging in patients with metastatic melanoma before and during immune checkpoint inhibitor therapy. Eur J Nuc Med Mol Imag. (2021) 48:4369–76. doi: 10.1007/s00259-021-05407-y
159. Yan G, Wang X, Fan Y, Lin J, Yan J, Wang L, et al. Immuno-PET Imaging of TNF-α in Colitis Using 89Zr-DFO-infliximab. Mol Pharmaceut. (2022) 19:3632–9. doi: 10.1021/acs.molpharmaceut.2c00411
160. Gibson HM, McKnight BN, Malysa A, Dyson G, Wiesend WN, McCarthy CE, et al. IFNγ PET imaging as a predictive tool for monitoring response to tumor immunotherapy IFNγ PET detects active response to adaptive immunotherapy. Cancer Res. (2018) 78:5706–17. doi: 10.1158/0008-5472.CAN-18-0253
161. Liu R, Oldham RJ, Teal E, Beers SA, Cragg MS. Fc-engineering for modulated effector functions-improving antibodies for cancer treatment. Antibodies. (2020) 9:64. doi: 10.3390/antib9040064
162. Liu L. Pharmacokinetics of monoclonal antibodies and Fc-fusion proteins. Protein Cell. (2018) 9:15–32. doi: 10.1007/s13238-017-0408-4
163. Shields RL, Namenuk AK, Hong K, Meng YG, Rae J, Briggs J, et al. High resolution mapping of the binding site on human IgG1 for FcγRI, FcγRII, FcγRIII, and FcRn and design of IgG1 variants with improved binding to the FcγR*. J Biol Chem. (2001) 276:6591–604. doi: 10.1074/jbc.M009483200
164. Deng R, Bumbaca D, Pastuskovas CV, Boswell CA, West D, Cowan KJ, et al. Preclinical pharmacokinetics, pharmacodynamics, tissue distribution, and tumor penetration of anti-PD-L1 monoclonal antibody, an immune checkpoint inhibitor. MAbs. (2016) 8:593–603. doi: 10.1080/19420862.2015.1136043
165. Wu AM. Engineered antibodies for molecular imaging of cancer. Methods. (2014) 65:139–47. doi: 10.1016/j.ymeth.2013.09.015
166. Moek KL, Waaijer SJH, Kok IC, Suurs FV, Brouwers AH, Menke-van der Houven van Oordt CW, et al. 89Zr-labeled bispecific T-cell engager AMG 211 PET shows AMG 211 accumulation in CD3-rich tissues and clear, heterogeneous tumor uptake. Clin Cancer Res. (2019) 25:3517–27. doi: 10.1158/1078-0432.CCR-18-2918
167. Yang Q, Chen M, Gu J, Niu K, Zhao X, Zheng L, et al. Novel biomarkers of dynamic blood PD-L1 expression for immune checkpoint inhibitors in advanced non-small-cell lung cancer patients. Front Immunol. (2021) 12:665133. doi: 10.3389/fimmu.2021.665133
168. Gu D, Ao X, Yang Y, Chen Z, Xu X. Soluble immune checkpoints in cancer: production, function and biological significance. J ImmunoTher Cancer. (2018) 6:132. doi: 10.1186/s40425-018-0449-0
169. Das RK, Vernau L, Grupp SA, Barrett DM. Naïve T-cell deficits at diagnosis and after chemotherapy impair cell therapy potential in pediatric cancers. Cancer Discov. (2019) 9:492–9. doi: 10.1158/2159-8290.CD-18-1314
170. Fraietta JA, Lacey SF, Orlando EJ, Pruteanu-Malinici I, Gohil M, Lundh S, et al. Determinants of response and resistance to CD19 chimeric antigen receptor (CAR) T cell therapy of chronic lymphocytic leukemia. Nat Med. (2018) 24:563–71. doi: 10.1038/s41591-018-0010-1
171. Schietinger A, Philip M, Krisnawan VE, Chiu EY, Delrow JJ, Basom RS, et al. Tumor-specific T cell dysfunction is a dynamic antigen-driven differentiation program initiated early during tumorigenesis. Immunity. (2016) 45:389–401. doi: 10.1016/j.immuni.2016.07.011
172. Elavia N, Panch SR, McManus A, Bikkani T, Szymanski J, Highfill SL, et al. Effects of starting cellular material composition on chimeric antigen receptor T-cell expansion and characteristics. Transfusion. (2019) 59:1755–64. doi: 10.1111/trf.15287
173. Choi BD, Yu X, Castano AP, Bouffard AA, Schmidts A, Larson RC, et al. CAR-T cells secreting BiTEs circumvent antigen escape without detectable toxicity. Nat Biotech. (2019) 37:1049–58. doi: 10.1038/s41587-019-0192-1
174. Tokarew N, Ogonek J, Endres S, von Bergwelt-Baildon M, Kobold S. Teaching an old dog new tricks: next-generation CAR T cells. Br J Cancer. (2019) 120:26–37. doi: 10.1038/s41416-018-0325-1
175. Martínez Bedoya D, Dutoit V, Migliorini D. Allogeneic CAR T cells: an alternative to overcome challenges of CAR T cell therapy in glioblastoma. Front Immunol. (2021) 12:640082. doi: 10.3389/fimmu.2021.640082
176. Sanber K, Savani B, Jain T. Graft-versus-host disease risk after chimeric antigen receptor T-cell therapy: the diametric opposition of T cells. Br J Haematol. (2021) 195:660–8. doi: 10.1111/bjh.17544
177. Dasyam N, George P, Weinkove R. Chimeric antigen receptor T-cell therapies: optimising the dose. Br J Clin Pharmacol. (2020) 86:1678–89. doi: 10.1111/bcp.14281
178. Vedvyas Y, Shevlin E, Zaman M, Min IM, Amor-Coarasa A, Park S, et al. Longitudinal PET imaging demonstrates biphasic CAR T cell responses in survivors. JCI Insight. (2016) 1:e90064. doi: 10.1172/jci.insight.90064
179. Shao F, Long Y, Ji H, Jiang D, Lei P, Lan X. Radionuclide-based molecular imaging allows CAR-T cellular visualization and therapeutic monitoring. Theranostics. (2021) 11:6800–17. doi: 10.7150/thno.56989
180. Nagle VL, Henry KE, Hertz CAJ, Graham MS, Campos C, Parada LF, et al. Imaging tumor-infiltrating lymphocytes in brain tumors with [64Cu]Cu-NOTA-anti-CD8 PET. Clin Cancer Res. (2021) 27:1958–66. doi: 10.1158/1078-0432.CCR-20-3243
181. Tavaré R, McCracken MN, Zettlitz KA, Knowles SM, Salazar FB, Olafsen T, et al. Engineered antibody fragments for immuno-PET imaging of endogenous CD8+ T cells in vivo. Proc Natl Acad Sci U S A. (2014) 111:1108–13. doi: 10.1073/pnas.1316922111
182. Tavaré R, Escuin-Ordinas H, Mok S, McCracken MN, Zettlitz KA, Salazar FB, et al. An effective immuno-PET imaging method to monitor CD8-dependent responses to immunotherapy. Cancer Res. (2016) 76:73–82. doi: 10.1158/0008-5472.CAN-15-1707
183. Pandit-Taskar N, Postow MA, Hellmann MD, Harding JJ, Barker CA, O'Donoghue JA, et al. First-in-Humans imaging with 89Zr-Df-IAB22M2C anti-CD8 minibody in patients with solid malignancies: preliminary pharmacokinetics, biodistribution, and lesion targeting. J Nuc Med. (2020) 61:512–9. doi: 10.2967/jnumed.119.229781
184. Farwell MD, Gamache RF, Babazada H, Hellmann MD, Harding JJ, Korn R, et al. CD8-targeted PET imaging of tumor infiltrating T cells in patients with cancer: a phase I first-in-human study of 89Zr-Df-IAB22M2C, a radiolabeled anti-CD8 Minibody. J Nuc Med. (2022) 63:720–6. doi: 10.2967/jnumed.121.262485
185. Waaijer SJ, Warnders FJ, Stienen S, Friedrich M, Sternjak A, Cheung HK, et al. Molecular imaging of radiolabeled bispecific T-cell engager 89Zr-AMG211 targeting CEA-positive tumors. Clin Cancer Res. (2018) 24:4988–96. doi: 10.1158/1078-0432.CCR-18-0786
186. Fu R, Carroll L, Yahioglu G, Aboagye EO, Miller PW. Antibody fragment and affibody immunoPET imaging agents: radiolabelling strategies and applications. ChemMedChem. (2018) 13:2466–78. doi: 10.1002/cmdc.201800624
187. Djekidel M, Brown RK, Piert M. Benefits of hybrid SPECT/CT for 111In-oxine-and Tc-99m-hexamethylpropylene amine oxime-labeled leukocyte imaging. Clin Nucl Med. (2011) 36:e50–6. doi: 10.1097/RLU.0b013e31821738a0
188. Weist MR, Starr R, Aguilar B, Chea J, Miles JK, Poku E, et al. PET of adoptively transferred chimeric antigen receptor T cells with 89Zr-oxine. J Nuc Med. (2018) 59:1531–7. doi: 10.2967/jnumed.117.206714
189. Wang X-Y, Wang Y, Wu Q, Liu J-J, Liu Y, Pan D-H, et al. Feasibility study of 68Ga-labeled CAR T cells for in vivo tracking using micro-positron emission tomography imaging. Acta Pharmacol Sin. (2021) 42:824–31. doi: 10.1038/s41401-020-00511-5
190. Charoenphun P, Meszaros LK, Chuamsaamarkkee K, Sharif-Paghaleh E, Ballinger JR, Ferris TJ, et al. [89Zr]Oxinate 4 for long-term in vivo cell tracking by positron emission tomography. Eur J Nuc Med Mol Imag. (2015) 42:278–87. doi: 10.1007/s00259-014-2945-x
191. Lee SH, Soh H, Chung JH, Cho EH, Lee SJ, Ju J-M, et al. Feasibility of real-time in vivo 89Zr-DFO-labeled CAR T-cell trafficking using PET imaging. PLoS ONE. (2020) 15:e0223814. doi: 10.1371/journal.pone.0223814
192. Liu Z, Li Z. Molecular imaging in tracking tumor-specific cytotoxic T lymphocytes (CTLs). Theranostics. (2014) 4:990. doi: 10.7150/thno.9268
193. Minn I, Rowe SP, Pomper MG. Enhancing CAR T-cell therapy through cellular imaging and radiotherapy. Lancet Oncol. (2019) 20:e443–51. doi: 10.1016/S1470-2045(19)30461-9
194. Minn I, Huss DJ, Ahn HH, Chinn TM, Park A, Jones J, et al. Imaging CAR T cell therapy with PSMA-targeted positron emission tomography. Sci Adv. (2019) 5:eaaw5096. doi: 10.1126/sciadv.aaw5096
196. Lauri C, Chiurchioni L, Russo VM, Zannini L, Signore A. PSMA expression in solid tumors beyond the prostate gland: ready for theranostic applications? J Clin Med. (2022) 11:6590. doi: 10.3390/jcm11216590
197. de Herder WW, Hofland LJ, van der Lely AJ, Lamberts SW. Somatostatin receptors in gastroentero-pancreatic neuroendocrine tumours. Endocr Relat Cancer. (2003) 10:451–8. doi: 10.1677/erc.0.0100451
198. Yamada Y, Post SR, Wang K, Tager HS, Bell GI, Seino S. Cloning and functional characterization of a family of human and mouse somatostatin receptors expressed in brain, gastrointestinal tract, and kidney. Proc Natl Acad Sci U S A. (1992) 89:251–5. doi: 10.1073/pnas.89.1.251
199. Pauwels E, Cleeren F, Tshibangu T, Koole M, Serdons K, Boeckxstaens L, et al. 18F-AlF-NOTA-octreotide outperforms 68Ga-DOTA-TATE/-NOC PET in neuroendocrine tumor patients: results from a prospective, multicenter study. J Nuc Med. (2022) 64:632–8. doi: 10.2967/jnumed.122.264563
200. Alcaina Y, Yang Y, Vedvyas Y, McCloskey JE, Jin MM. SSTR2 as an anatomical imaging marker and a safety switch to monitor and manage CAR T cell toxicity. Sci Rep. (2022) 12:20932. doi: 10.1038/s41598-022-25224-z
201. Krebs S, Ahad A, Carter LM, Eyquem J, Brand C, Bell M, et al. Antibody with infinite affinity for in vivo tracking of genetically engineered lymphocytes. J Nuc Med. (2018) 59:1894–900. doi: 10.2967/jnumed.118.208041
202. Wei LH, Olafsen T, Radu C, Hildebrandt IJ, McCoy MR, Phelps ME, et al. Engineered antibody fragments with infinite affinity as reporter genes for PET imaging. J Nuc Med. (2008) 49:1828–35. doi: 10.2967/jnumed.108.054452
203. Yaghoubi SS, Jensen MC, Satyamurthy N, Budhiraja S, Paik D, Czernin J, et al. Noninvasive detection of therapeutic cytolytic T cells with 18F-FHBG PET in a patient with glioma. Nat Rev Clin Oncol. (2009) 6:53–8. doi: 10.1038/ncponc1278
204. Keu KV, Witney TH, Yaghoubi S, Rosenberg J, Kurien A, Magnusson R, et al. Reporter gene imaging of targeted T cell immunotherapy in recurrent glioma. Sci Translat Med. (2017) 9:eaag2196. doi: 10.1126/scitranslmed.aag2196
205. Krebs S, Dacek MM, Carter LM, Scheinberg DA, Larson SM. CAR chase: where do engineered cells go in humans? Front Oncol. (2020) 10:577773. doi: 10.3389/fonc.2020.577773
206. Xiao Z, Mayer AT, Nobashi TW, Gambhir SS, ICOS. Is an indicator of T-cell-mediated response to cancer immunotherapy. Cancer Res. (2020) 80:3023–32. doi: 10.1158/0008-5472.CAN-19-3265
207. Alam IS, Mayer AT, Sagiv-Barfi I, Wang K, Vermesh O, Czerwinski DK, et al. Imaging activated T cells predicts response to cancer vaccines. J Clin Investig. (2018) 128:2569–80. doi: 10.1172/JCI98509
208. van der Veen EL, Suurs FV, Cleeren F, Bormans G, Elsinga PH, Hospers GAP, et al. Development and evaluation of interleukin-2–derived radiotracers for PET imaging of T cells in mice. J Nuc Med. (2020) 61:1355–60. doi: 10.2967/jnumed.119.238782
209. LaSalle T, Austin EE, Rigney G, Wehrenberg-Klee E, Nesti S, Larimer B, et al. Granzyme B PET imaging of immune-mediated tumor killing as a tool for understanding immunotherapy response. J ImmunoTher Cancer. (2020) 8:e000291. doi: 10.1136/jitc-2019-000291
210. Volpe A, Nagle VL, Lewis JS, Ponomarev V. Predicting CAR-T cell immunotherapy success through immunoPET. Clin Cancer Res. (2021) 27:911–2. doi: 10.1158/1078-0432.CCR-20-4297
211. Larimer BM, Wehrenberg-Klee E, Dubois F, Mehta A, Kalomeris T, Flaherty K, et al. Granzyme B PET imaging as a predictive biomarker of immunotherapy response. Cancer Res. (2017) 77:2318–27. doi: 10.1158/0008-5472.CAN-16-3346
212. Larimer BM, Bloch E, Nesti S, Austin EE, Wehrenberg-Klee E, Boland G, et al. The effectiveness of checkpoint inhibitor combinations and administration timing can be measured by granzyme B PET imaging. Clin Cancer Res. (2019) 25:1196–205. doi: 10.1158/1078-0432.CCR-18-2407
213. Podack ER. Execution and suicide: cytotoxic lymphocytes enforce Draconian laws through separate molecular pathways. Curr Opin Immunol. (1995) 7:11–6. doi: 10.1016/0952-7915(95)80023-9
214. Voskoboinik I, Whisstock JC, Trapani JA. Perforin and granzymes: function, dysfunction and human pathology. Nat Rev Immunol. (2015) 15:388–400. doi: 10.1038/nri3839
215. Varadarajan I, Kindwall-Keller TL, Lee DW. Management of cytokine release syndrome. In:Lee DW, Shah NN, , editors Chimeric Antigen Receptor T-cell Therapies for Cancer. Amsterdam: Elsevier (2020), p. 45–64. doi: 10.1016/B978-0-323-66181-2.00005-6
216. Morris EC, Neelapu SS, Giavridis T, Sadelain M. Cytokine release syndrome and associated neurotoxicity in cancer immunotherapy. Nat Rev Immunol. (2022) 22:85–96. doi: 10.1038/s41577-021-00547-6
217. Schubert ML, Schmitt M, Wang L, Ramos CA, Jordan K, Müller-Tidow C, et al. Side-effect management of chimeric antigen receptor (CAR) T-cell therapy. Ann Oncol. (2021) 32:34–48. doi: 10.1016/j.annonc.2020.10.478
218. Porter D, Frey N, Wood PA, Weng Y, Grupp SA. Grading of cytokine release syndrome associated with the CAR T cell therapy tisagenlecleucel. J Hematol Oncol. (2018) 11:1–12. doi: 10.1186/s13045-018-0571-y
219. Chou CK, Turtle CJ. Assessment and management of cytokine release syndrome and neurotoxicity following CD19 CAR-T cell therapy. Expert Opin Biol Ther. (2020) 20:653–64. doi: 10.1080/14712598.2020.1729735
220. Santomasso B, Bachier C, Westin J, Rezvani K, Shpall EJ. The other side of CAR T-cell therapy: cytokine release syndrome, neurologic toxicity, and financial burden. Am Soc Clin Oncol. (2019) 39:433–44. doi: 10.1200/EDBK_238691
221. Siegler EL, Kenderian SS. Neurotoxicity and cytokine release syndrome after chimeric antigen receptor T cell therapy: insights into mechanisms and novel therapies. Front Immunol. (2020) 11:1973. doi: 10.3389/fimmu.2020.01973
222. Wang T, Tang Y, Cai J, Wan X, Hu S, Lu X, et al. Coadministration of CD19- and CD22-directed chimeric antigen receptor T-cell therapy in childhood B-cell acute lymphoblastic leukemia: a single-arm, multicenter, phase II trial. J Clin Oncol. (2023) 41:1670–83. doi: 10.1200/JCO.22.01214
223. Hayden PJ, Roddie C, Bader P, Basak GW, Bonig H, Bonini C, et al. Management of adults and children receiving CAR T-cell therapy: 2021 best practice recommendations of the European Society for Blood and Marrow Transplantation (EBMT) and the Joint Accreditation Committee of ISCT and EBMT (JACIE) and the European Haematology Association (EHA). Ann Oncol. (2022) 33:259–75. doi: 10.1016/j.annonc.2021.12.003
224. Liu D, Zhao J. Cytokine release syndrome: grading, modeling, and new therapy. J Hematol Oncol. (2018) 11:1–7. doi: 10.1186/s13045-018-0653-x
225. Gust J, Taraseviciute A, Turtle CJ. Neurotoxicity associated with CD19-targeted CAR-T cell therapies. CNS Drugs. (2018) 32:1091–101. doi: 10.1007/s40263-018-0582-9
226. Teachey DT, Rheingold SR, Maude SL, Zugmaier G, Barrett DM, Seif AE, et al. Cytokine release syndrome after blinatumomab treatment related to abnormal macrophage activation and ameliorated with cytokine-directed therapy. Blood. (2013) 121:5154–7. doi: 10.1182/blood-2013-02-485623
227. Santomasso BD, Park JH, Salloum D, Riviere I, Flynn J, Mead E, et al. Clinical and biological correlates of neurotoxicity associated with CAR T-cell therapy in patients with B-cell acute lymphoblastic leukemia. Cancer Discov. (2018) 8:958–71. doi: 10.1158/2159-8290.CD-17-1319
228. Sun S, Hao H, Yang G, Zhang Y, Fu Y. Immunotherapy with CAR-modified T cells: toxicities and overcoming strategies. J Immunol Res. (2018) 2018:2386187. doi: 10.1155/2018/2386187
229. Morgan RA, Yang JC, Kitano M, Dudley ME, Laurencot CM, Rosenberg SA. Case report of a serious adverse event following the administration of T cells transduced with a chimeric antigen receptor recognizing ERBB2. Mol Ther. (2010) 18:843–51. doi: 10.1038/mt.2010.24
230. Miao L, Zhang Z, Ren Z, Li Y. Reactions related to CAR-T cell therapy. Front Immunol. (2021) 12:663201. doi: 10.3389/fimmu.2021.663201
231. Maus MV, Haas AR, Beatty GL, Albelda SM, Levine BL, Liu X, et al. T cells expressing chimeric antigen receptors can cause anaphylaxis in humans. Cancer Immunol Res. (2013) 1:26–31. doi: 10.1158/2326-6066.CIR-13-0006
232. Neelapu SS, Tummala S, Kebriaei P, Wierda W, Gutierrez C, Locke FL, et al. Chimeric antigen receptor T-cell therapy - assessment and management of toxicities. Nat Rev Clin Oncol. (2018) 15:47–62. doi: 10.1038/nrclinonc.2017.148
233. Yoon JG, Smith DA, Tirumani SH, Caimi PF, Ramaiya NH, CAR. T-cell therapy: an update for radiologists. AJR Am J Roentgenol. (2021) 217:1461–74. doi: 10.2214/AJR.21.26091
234. Norelli M, Camisa B, Barbiera G, Falcone L, Purevdorj A, Genua M, et al. Monocyte-derived IL-1 and IL-6 are differentially required for cytokine-release syndrome and neurotoxicity due to CAR T cells. Nat Med. (2018) 24:739–48. doi: 10.1038/s41591-018-0036-4
235. Giavridis T, van der Stegen SJ, Eyquem J, Hamieh M, Piersigilli A, Sadelain M. CAR T cell–induced cytokine release syndrome is mediated by macrophages and abated by IL-1 blockade. Nat Med. (2018) 24:731–8. doi: 10.1038/s41591-018-0041-7
236. Xue L, Yi Y, Xu Q, Wang L, Yang X, Zhang Y, et al. Chimeric antigen receptor T cells self-neutralizing IL6 storm in patients with hematologic malignancy. Cell Discov. (2021) 7:84. doi: 10.1038/s41421-021-00299-6
237. Teachey DT, Lacey SF, Shaw PA, Melenhorst JJ, Maude SL, Frey N, et al. Identification of predictive biomarkers for cytokine release syndrome after chimeric antigen receptor T-cell therapy for acute lymphoblastic leukemia. Cancer Discov. (2016) 6:664–79. doi: 10.1158/2159-8290.CD-16-0040
238. Si S, Teachey DT. Spotlight on tocilizumab in the treatment of CAR-T-cell-induced cytokine release syndrome: clinical evidence to date. Ther Clin Risk Manag. (2020) 16:705–14. doi: 10.2147/TCRM.S223468
239. Camacho X, Machado C, Garcia MF, Fernandez M, Alonso O, Cerecetto H, et al. 99m technetium-tocilizumab fragments as molecular imaging agent for multiple myeloma. Blood. (2015) 126:4214. doi: 10.1182/blood.V126.23.4214.4214
240. Camacho X, Machado CL, García MF, Fernández M, Oddone N, Benech J, et al. Tocilizumab labeling with 99mTechnetium via HYNIC as a molecular diagnostic agent for multiple myeloma. Anticancer Agents Med Chem. (2017) 17:1267–77. doi: 10.2174/1871520617666170213144917
241. Camacho X, Perroni C, de Souza Junqueira M, Fernandez M, Chammas R, Buchpiguel C, et al. Cy7-tocilizumab/Fab(Tocilizumab): near infrared fluorescence in vivo imaging of multiple myeloma. Blood. (2018) 132:5621. doi: 10.1182/blood-2018-99-114186
242. Patel S, Cenin D, Corrigan D, Hamilton BK, Kalaycio M, Sobecks RM, et al. Siltuximab for first-line treatment of cytokine release syndrome: a response to the national shortage of tocilizumab. Blood. (2022) 140(Supplement 1):5073–4. doi: 10.1182/blood-2022-169809
243. Cawthorne C, Prenant C, Smigova A, Julyan P, Maroy R, Herholz K, et al. Biodistribution, pharmacokinetics and metabolism of interleukin-1 receptor antagonist (IL-1RA) using [18F]-IL1RA and PET imaging in rats. Br J Pharmacol. (2011) 162:659–72. doi: 10.1111/j.1476-5381.2010.01068.x
244. Kulkarni V, Cai M, Barber C, Wan L, Woolfenden J, Liu Z. 99mTc-Labeled interleukin-1 antagonist peptide for inflammation imaging. J Nuc Med. (2013) 54:1091.
245. Li L, Fei Z, Ren J, Sun R, Liu Z, Sheng Z, et al. Functional imaging of interleukin 1 beta expression in inflammatory process using bioluminescence imaging in transgenic mice. BMC Immunol. (2008) 9:49. doi: 10.1186/1471-2172-9-49
246. Prenant C, Cawthorne C, Fairclough M, Rothwell N, Boutin H. Radiolabeling with fluorine-18 of a protein, interleukin-1 receptor antagonist. Appl Radiat Isot. (2010) 68:1721–7. doi: 10.1016/j.apradiso.2010.04.007
247. Dmochowska N, Tieu W, Keller MD, Wardill HR, Mavrangelos C, Campaniello MA, et al. Immuno-PET of innate immune markers CD11b and IL-1β detects inflammation in murine colitis. J Nuc Med. (2019) 60:858–63. doi: 10.2967/jnumed.118.219287
248. Ju X, Zhang H, Zhou Z, Chen M, Wang Q. Tumor-associated macrophages induce PD-L1 expression in gastric cancer cells through IL-6 and TNF-α signaling. Exp Cell Res. (2020) 396:112315. doi: 10.1016/j.yexcr.2020.112315
249. Numata Y, Akutsu N, Ishigami K, Koide H, Wagatsuma K, Motoya M, et al. Synergistic effect of IFN-γ and IL-1β on PD-L1 expression in hepatocellular carcinoma. Biochem Biophys Rep. (2022) 30:101270. doi: 10.1016/j.bbrep.2022.101270
250. Marofi F, Motavalli R, Safonov VA, Thangavelu L, Yumashev AV, Alexander M, et al. CAR T cells in solid tumors: challenges and opportunities. Stem Cell Res Ther. (2021) 12:81. doi: 10.1186/s13287-020-02128-1
251. Mancini SJC, Balabanian K, Corre I, Gavard J, Lazennec G, Le Bousse-Kerdilès M-C, et al. Deciphering tumor niches: lessons from solid and hematological malignancies. Front Immunol. (2021) 12:766275. doi: 10.3389/fimmu.2021.766275
252. Murgai M, Giles A, Kaplan R. Physiological, tumor, and metastatic niches: opportunities and challenges for targeting the tumor microenvironment. Crit Rev Oncog. (2015) 20:301–14. doi: 10.1615/CritRevOncog.2015013668
253. Li Y, Zhao L, Li XF. Hypoxia and the tumor microenvironment. Technol Cancer Res Treat. (2021) 20:15330338211036304. doi: 10.1177/15330338211036304
254. You L, Wu W, Wang X, Fang L, Adam V, Nepovimova E, et al. The role of hypoxia-inducible factor 1 in tumor immune evasion. Med Res Rev. (2021) 41:1622–43. doi: 10.1002/med.21771
255. Martinez M, Moon EK. CAR T cells for solid tumors: new strategies for finding, infiltrating, and surviving in the tumor microenvironment. Front Immunol. (2019) 10:128. doi: 10.3389/fimmu.2019.00128
256. Berahovich R, Liu X, Zhou H, Tsadik E, Xu S, Golubovskaya V, et al. Hypoxia selectively impairs CAR-T cells in vitro. Cancers. (2019) 11:602. doi: 10.3390/cancers11050602
257. Juillerat A, Marechal A, Filhol JM, Valogne Y, Valton J, Duclert A, et al. An oxygen sensitive self-decision making engineered CAR T-cell. Sci Rep. (2017) 7:39833. doi: 10.1038/srep39833
258. Cui J, Zhang Q, Song Q, Wang H, Dmitriev P, Sun MY, et al. Targeting hypoxia downstream signaling protein, CAIX, for CAR T-cell therapy against glioblastoma. Neuro Oncol. (2019) 21:1436–46. doi: 10.1093/neuonc/noz117
259. Rodriguez-Garcia A, Palazon A, Noguera-Ortega E, Powell DJ, Guedan S. CAR-T cells hit the tumor microenvironment: strategies to overcome tumor escape. Front Immunol. (2020) 11:1109. doi: 10.3389/fimmu.2020.01109
260. Verhoeff SR, van Es SC, Boon E, van Helden E, Angus L, Elias SG, et al. Lesion detection by [89Zr]Zr-DFO-girentuximab and [18F]FDG-PET/CT in patients with newly diagnosed metastatic renal cell carcinoma. Eur J Nuc Med Mol Imag. (2019) 46:1931–9. doi: 10.1007/s00259-019-04358-9
261. Merkx RIJ, Lobeek D, Konijnenberg M, Jiménez-Franco LD, Kluge A, Oosterwijk E, et al. Phase I study to assess safety, biodistribution and radiation dosimetry for 89Zr-girentuximab in patients with renal cell carcinoma. Eur J Nuc Med Mol Imag. (2021) 48:3277–85. doi: 10.1007/s00259-021-05271-w
262. Divgi CR, Pandit-Taskar N, Jungbluth AA, Reuter VE, Gönen M, Ruan S, et al. Preoperative characterisation of clear-cell renal carcinoma using iodine-124-labelled antibody chimeric G250 (124I-cG250) and PET in patients with renal masses: a phase I trial. Lancet Oncol. (2007) 8:304–10. doi: 10.1016/S1470-2045(07)70044-X
263. Al-Zubaidi M, Viswambaram P, McCombie S, Liow E, Lenzo N, Ferguson T, et al. 89Zirconium-labelled girentuximab (89Zr-TLX250) PET in Urothelial Cancer Patients (ZiPUP): protocol for a phase I trial of a novel staging modality for urothelial carcinoma. BMJ Open. (2022) 12:e060478. doi: 10.1136/bmjopen-2021-060478
264. Stillebroer AB, Franssen GM, Mulders PF, Oyen WJ, van Dongen GA, Laverman P, et al. ImmunoPET imaging of renal cell carcinoma with 124I- and 89Zr-labeled anti-CAIX monoclonal antibody cG250 in mice. Cancer Biother Radiopharm. (2013) 28:510–5. doi: 10.1089/cbr.2013.1487
265. Klaus T, Deshmukh S. pH-responsive antibodies for therapeutic applications. J Biomed Sc. (2021) 28:11. doi: 10.1186/s12929-021-00709-7
266. Uenomachi M, Takahashi M, Shimazoe K, Takahashi H, Kamada K, Orita T, et al. Simultaneous in vivo imaging with PET and SPECT tracers using a Compton-PET hybrid camera. Sci Rep. (2021) 11:17933. doi: 10.1038/s41598-021-97302-7
267. Paquette M, Phoenix S, Lawson C, Guérin B, Lecomte R, Tai L-H, et al. A preclinical PET dual-tracer imaging protocol for ER and HER2 phenotyping in breast cancer xenografts. EJNMMI Res. (2020) 10:69. doi: 10.1186/s13550-020-00656-8
268. Andreyev A, Celler A. Dual-isotope PET using positron-gamma emitters. Phys Med Biol. (2011) 56:4539–56. doi: 10.1088/0031-9155/56/14/020
Keywords: immuno-PET, immuno-SPECT, cell therapy, CAR T-cell therapy, tumor microenvironment
Citation: Mulgaonkar A, Udayakumar D, Yang Y, Harris S, Öz OK, Ramakrishnan Geethakumari P and Sun X (2023) Current and potential roles of immuno-PET/-SPECT in CAR T-cell therapy. Front. Med. 10:1199146. doi: 10.3389/fmed.2023.1199146
Received: 03 April 2023; Accepted: 25 May 2023;
Published: 27 June 2023.
Edited by:
Francisca Mulero, Spanish National Cancer Research Center, SpainReviewed by:
Calogero D'Alessandria, Technical University of Munich, GermanyYongkang Gai, Huazhong University of Science and Technology, China
Copyright © 2023 Mulgaonkar, Udayakumar, Yang, Harris, Öz, Ramakrishnan Geethakumari and Sun. This is an open-access article distributed under the terms of the Creative Commons Attribution License (CC BY). The use, distribution or reproduction in other forums is permitted, provided the original author(s) and the copyright owner(s) are credited and that the original publication in this journal is cited, in accordance with accepted academic practice. No use, distribution or reproduction is permitted which does not comply with these terms.
*Correspondence: Praveen Ramakrishnan Geethakumari, cHJhdmVlbi5yYW1ha3Jpc2huYW5AdXRzb3V0aHdlc3Rlcm4uZWR1; Xiankai Sun, eGlhbmthaS5zdW5AdXRzb3V0aHdlc3Rlcm4uZWR1