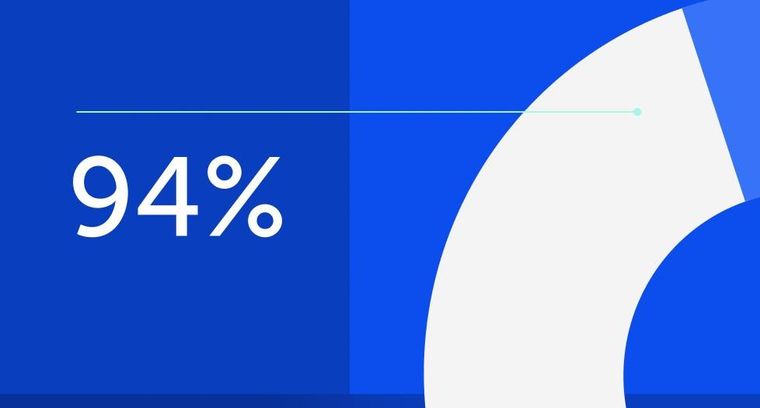
94% of researchers rate our articles as excellent or good
Learn more about the work of our research integrity team to safeguard the quality of each article we publish.
Find out more
REVIEW article
Front. Med., 14 March 2023
Sec. Pulmonary Medicine
Volume 10 - 2023 | https://doi.org/10.3389/fmed.2023.1118583
This article is part of the Research TopicRecent Advances on the Physiology and Pathophysiology of the Lymphatic SystemView all 6 articles
The lymphatic vasculature maintains tissue homeostasis via fluid drainage in the form of lymph and immune surveillance due to migration of leukocytes through the lymphatics to the draining lymph nodes. Lymphatic endothelial cells (LECs) form the lymphatic vessels and lymph node sinuses and are key players in shaping immune responses and tolerance. In the healthy lung, the vast majority of lymphatic vessels are found along the bronchovascular structures, in the interlobular septa, and in the subpleural space. Previous studies in both mice and humans have shown that the lymphatics are necessary for lung function from the neonatal period through adulthood. Furthermore, changes in the lymphatic vasculature are observed in nearly all respiratory diseases in which they have been analyzed. Recent work has pointed to a causative role for lymphatic dysfunction in the initiation and progression of lung disease, indicating that these vessels may be active players in pathologic processes in the lung. However, the mechanisms by which defects in lung lymphatic function are pathogenic are understudied, leaving many unanswered questions. A more comprehensive understanding of the mechanistic role of morphological, functional, and molecular changes in the lung lymphatic endothelium in respiratory diseases is a promising area of research that is likely to lead to novel therapeutic targets. In this review, we will discuss our current knowledge of the structure and function of the lung lymphatics and the role of these vessels in lung homeostasis and respiratory disease.
Historically, the lymphatic vascular system has been understudied compared to the blood vascular system, in part because of difficulty identifying these vessels and unique anatomical differences that make their characterization challenging. However, there have been significant advances in lymphatic research over the past two decades, and the importance of lymphatic function has been increasingly uncovered (1–3). In the lung, lymphatic vessels play critical roles spanning from the first breath after birth to roles in diseases such as asthma, tuberculosis, and COPD (4–9). While the importance of the lung lymphatics is starting to be understood, the precise mechanisms by which these vessels affect lung homeostasis and disease pathogenesis are not entirely clear. However, given that the lung is both constantly exposed to outside pathogens and toxins and also exquisitely sensitive to fluid accumulation, lymphatic function is predicted to play a key mechanistic role in lung function.
The lymphatic system has been described since the 16th century, and landmark studies by Dr. Florence Sabin in the 1900s provided critical information about the origins and anatomy of the lymphatic vasculature during development. However, it has only been in the past 20 years that the molecular basis for lymphatic development has been uncovered. In mammals, the lymphatic system develops embryonically and is driven by specification of endothelial cells to a lymphatic identity via the transcription factor PROX1, which is required to bias and maintain a population of progenitor cells to the lymphatic program (10–14). Embryonic lymphangiogenesis is driven by vascular endothelial growth factor C (VEGFC) signaling through its receptor VEGFR3 on LECs (15). Lymphatic vessels form an extensive network throughout the skin and in most internal organs where they function to promote interstitial fluid drainage, trafficking of antigen presenting cells into regional lymph nodes, and absorption of lipids and small molecules (16, 17). In the lung and elsewhere, the lymphatic vascular network consists of small, thin-walled, and blind-ended initial lymphatics that drain into larger collecting lymphatic vessels (18, 19). Discontinuous button-like junctions between LECs in the initial lymphatics facilitate uptake of interstitial fluid and macromolecules (18). The collecting lymphatics have tighter and continuous zipper-like junctions and in most organs are covered with specialized muscle cells that promote lymph flow by providing contractile activity, though notably, the lymphatics in the lung lack this smooth muscle cell coverage (18, 19). Initial lymphatics in the lung parenchyma drain into collecting lymphatic vessels that are present in the bronchovascular bundles and interlobular septa (Figure 1). These collecting lymphatics drain to thoracic lymph nodes, and eventually into the thoracic duct, where lymph is returned to the blood circulation.
Figure 1. Anatomical distribution of lymphatics in healthy lung. (A) Distribution of lymphatics in the lung (green, left) with arrows indicating the direction of lymph flow. Cross section of the lung (right) and the distribution of lymphatics in relation to other lung structures. Images created using MediBang Paint Pro. (B,C) Visualization of lung lymphatics using whole mount immunohistochemistry of lung tissue from a Prox1-EGFP lymphatic reporter mouse, in which all LECs are labeled by GFP expression (green). Initial lymphatics are shown in B, with blunt ends of these vessels indicated by arrowheads. A larger collecting lymphatic is shown in C. Values indicated with asterisks. Scalebars = 50 um.
Much of our recent understanding of the development and origins of the lymphatics are the result of studies using mouse and zebrafish embryos. In addition, the identification of mutations in several genes that are essential for lymphatic development in patients with lymphatic disorders has also aided in our knowledge of the molecular basis for lymphatic development (3). The lymphatic vasculature, including the lung lymphatics, arise from specification of LEC progenitors expressing the master transcriptional factor PROX1 in the cardinal veins to form lymph sacs (14, 20–22). Lymphatic identity in these LECs is maintained by a feedback loop involving both PROX1 and VEGFR3 (23). Migration of LECs from the lymph sacs into the developing lobes of the lungs begins at embryonic day 11.5 in mice, with more extensive patterning and lymphatic vessels with lumens detected in proximal and distal bronchovascular bundles at E14.5 (24). By E18.5, the lung lymphatic vasculature is a well-defined and continuous network that is associated with both bronchovascular bundles and veins (24).
Study of the lymphatic system in general, and the lung lymphatics in particular, has historically been quite challenging due to the lack of reliable imaging techniques and molecular markers as well as their thin walls, small size, variable anatomy, and complex interconnections (25). Even directly after surgical exposure, lymphatic vessels are not easily visible, as lymph is normally transparent. Studies using casting techniques in animal models provided critical early insights into the anatomy of the lung lymphatic vasculature. Using corrosion casting (26–28), lymphatics within the lung were visualized and found to consist of blind-ended initial lymphatics that empty into tubular conduit lymphatics around blood vessels and airways (29, 30). A series of ‘pre-lymphatics’ were also seen and consisted simply of tissue planes that connected with lymphatic channels on the pleural surface.
Since these studies, visualization of the lung lymphatics in animal models is now greatly aided by identification of lymphatic-specific markers and genetically modified mice that allow for microscopic analysis of these vessels. For example, lymphatic reporter mice in which LECs are labeled by expression of fluorescent proteins driven by the lymphatic-specific promoter gene PROX1 provide a key tool for identification of lung LECs (31, 32). Immunohistochemical techniques can also be used for identification of lung lymphatic vessels in human and mouse tissue using antibodies targeting lymphatic markers such as VEGFR3, PROX1, and Podoplanin. However, care must be taken in the use of these markers given organ-specific differences in their specificity for the lymphatic endothelium (33–35). For example, while relatively specific for the lymphatics in the lung, VEGFR3 is not a lymphatic-specific marker in other organs, particularly in the liver, where VEGFR3 is highly expressed in sinusoidal endothelial cells (36). Similarly, while Podoplanin can be used to detect lymphatics in the skin in mice, it lacks specificity for lymphatics in the lung, where it also expressed at high levels by type I alveolar epithelial cells (37). Expression of lymphatic markers may also change in settings of lung injury, further complicating the identification of lung lymphatic vessels (38, 39). Despite this, reliable markers for the lung lymphatics in both mice and humans have been identified, particularly when used in combination with each other (Table 1). Notably, novel radiographic techniques using inhaled lipid nanoparticles to map the draining patterns of the lung lymphatics have been developed in animal models (44, 45) and may be adapted for use in patients in the future.
Clinically, lymphangiography has significantly advanced our ability to image the lymphatics at high resolution due to direct administration of contrast agent into cannulated lymph vessels for computed tomography (CT) of lymphatic architecture. Contrast enhanced magnetic resonance lymphangiography has also provided a great deal of new information about the anatomy of the pulmonary lymphatics, particularly in the setting of pathological lymphatic flow into the lung parenchyma (46). Newer experimental techniques combine immunohistochemistry and high-resolution micro-CT (μCT) to obtain 3D imaging and microfluidic modeling of human pulmonary lymphatics at high resolution (47).
The lungs are susceptible to inflammation and injury, as they are constantly exposed to pathogens, environmental toxins, and inflammatory stimuli. Therefore, a major role of the lung lymphatics is clearing toxic substances and regulating the immune response (22, 48–50). In addition, though most fluid drainage in the lung can be accommodated via Starling forces to the pulmonary capillaries, lymphatic vessels clearly play a role in drainage of excess fluid and preventing edema that would compromise gas exchange (51, 52). Animal models have demonstrated that the lymphatic vessels begin draining interstitial fluid at the late gestational period, which increases compliance and changes lung mechanics to prepare for inflation at birth (9, 53).
The lung lymphatics also play a central role in coordinating the adaptive immune response by serving as a conduit for immune cell trafficking from lungs to draining lymph nodes where the response to infection and inflammation is coordinated (54, 55). Immature dendritic cells (DCs) reside in the periphery of the lung where they sample antigens found in inhaled air. These DCs extend their cellular processes between airway epithelial cells and into the airway lumen to provide continuous immune surveillance of the airway luminal surface (56–58). In addition, passive leakage of smaller antigens into the afferent lymph vessels also occurs through the tight junction barrier of LECs which acts as a molecular sieve. Lung DCs first migrate to bronchopulmonary lymph nodes and then drain to the mediastinal trunk (30). This egression of DCs from the lungs to the draining lymph nodes is tightly regulated (59), and sampling of antigens by pulmonary DCs that traffic to the lung draining lymph nodes is a key mechanism for orchestrating an appropriate immune response.
Studies using molecular tracing of DCs have proven useful for studying the kinetics of DC migration in the lung lymphatics to the draining lymph nodes (60–62). In normal conditions, a limited number of DCs reside in the lung and patrol the environment, and these cells maintain a steady state of migration via lymphatics to mediastinal lymph nodes every 1 to 2 days (63). Circulating pre-DCs migrate into lungs at a constant rate to replenish the resident DC pool, which turns over every 10 to 14 days (64–68). However, the turnover rate of DCs depends on anatomical location, and it has been found that the kinetics of migration of antigen presenting cells in the upper airways is much faster than in the lung parenchyma (63, 68). In one study, roughly 80% of the DCs in the upper airways were replaced by new cells within 18 h whereas only 12% of the parenchymal cells were replaced within 9 days (68). Thus, there is a balance between migration of immune cells from the lungs via the lymphatic vessels and replenishment of these cells from the circulation that affects lung homeostasis. Changes in the rate of lung lymphatic trafficking or in the rate of cellular influx can have profound implications on the lung immune milieu. For example, lung lymphatic dysfunction alone, in the absence of inflammatory stimuli, is sufficient to generate profound lung inflammation characterized by accumulation of lung immune cells and the formation of tertiary lymphoid organs (TLOs) (7). Conversely, in the settings of infection, lung lymphatic migration of DCs to draining lymph nodes is increased (68–73). In this way, changes in lung lymphatic trafficking coupled with the rate of immune cell recruitment to the lung can govern the inflammatory state of the lung and its response to injury.
Interactions between LECs and trafficking leukocytes also affect immune cell migration and the immune response. Chemokines provide critical cues for the directional guidance of leukocyte transmigration in lymphatics, with chemokines and adhesion molecules synthesized and secreted by LECs tightly regulating leukocyte migration along chemotactic gradients under both steady state and inflammatory conditions (59). CCL21 expression on the lymphatic endothelium and expression of its receptor CCR7 on DCs and T cells is essential for uptake and migration of immune cells via pulmonary lymphatic vessels (74). Immobilized CCL21 on the lymphatic endothelium also plays an essential role in directing leukocyte movement within the lymphatic vessels (75–77). Antigen presentation by DCs to the lymph nodes is therefore dependent on gradients of ligands for CCR7 on the lymphatic endothelium (62, 78–80). During inflammatory conditions, other factors such as sphingosine-1-phosphate (SIP-1), ICAM-1 and VCAM-1, and prostaglandin E2 expressed by the lymphatic endothelium are also important for leukocyte trafficking in lymphatics (38, 81–84).
Lymphatics are now considered to actively participate in physiological and pathophysiological processes in the lung, in part because of the role of these vessels in modulating the lung immune milieu. Morphological or functional defects in the lymphatic vasculature have been uncovered in many diverse pathological lung conditions (Figure 2). However, the mechanisms by which lymphatic dysfunction contributes to disease pathogenesis are not entirely clear. In some settings, lymphatic dysfunction may be secondary to lung injury and remodeling, but it is clear that in other settings, lymphatic dysfunction itself drives disease progression.
Figure 2. The lymphatic vasculature in lung disease. LECs, lymphatic endothelial cells; ILD, interstitial lung disease; LAM, Lymphangioleiomyomatosis; GLA, generalized lymphatic anomaly; KLA, Kaposiform lymphangiomatosis; VEGF, vascular endothelial growth factor.
Asthma is an inflammatory disease characterized by mostly reversible airflow obstruction showing features such as subepithelial fibrosis, changes in the extracellular matrix, mucosal edema, and angiogenesis (85, 86). Remodeling of airway lymphatic vessels is seen in asthma (86) and in animal models has been shown to be mediated by VEGFC, VEGFD and TNF-alpha (87–89). Blockade of VEGFR3 signaling prevents tracheal lymphangiogenesis in a model of chronic airway inflammation (90). Furthermore, VEGFR3 signaling may play a role in airway inflammation by regulating the adaptive immune response (91). Interestingly, despite elevated levels of VEGFC and VEGFD, decreased lymphatic vessel density is associated with airway edema and fibrotic changes, and has been reported in fatal asthma cases (86), suggesting that anti-lymphangiogenic factors may be more prominent in severe disease. However, the exact roles that pro-lymphangiogenic and anti-lymphangiogenic factors play in acute or chronic airway inflammation are yet to be completely characterized. Further studies are required to explore the contribution of lymphatics to disease pathogenesis in asthma and how this may be targeted for therapies.
Sarcoidosis is a systemic granulomatous disease that is characterized by non-caseating granulomas that are found mainly in the lungs and lymph nodes (92–94). Changes in the lymphatic vasculature are associated with these pulmonary granulomas, which are distributed along the lymphatic drainage pathways in the lung. Lymphangiogenesis has been implicated in the pathogenesis of pulmonary sarcoidosis given the close association of the pulmonary sarcoid granulomas with lymphatic vessels (95–98) and the elevated levels of VEGFC and VEGFA that are seen in the serum and bronchoalveolar lavage fluid of patients with this disease. In addition, immunohistochemical staining has demonstrated that the sarcoid granulomas are a source of these lymphangiogenic factors (97, 99). Though staining for Podoplanin has revealed atypical tubular vessels around the granulomas that resemble lymphatics, they lack staining for other lymphatic markers such as VEGFR3, making their identity as lymphatic vessels a bit unclear (97).
In case of the untreatable end stage pulmonary diseases such as COPD, pulmonary fibrosis and cystic fibrosis, lung transplantation is the only viable option available. However, long-term outcomes after lung transplantation remain poor, and acute rejection is seen in nearly 30% of recipients, which carries the additional risk of development of chronic lung allograft dysfunction (100–103). Lymphatic vessels in the lung are severed during transplant surgery and are not surgically reconnected. Despite this, reestablishment of lung lymphatic drainage has been demonstrated in both humans and animal models (104–106). Furthermore, careful analysis of the lung lymphatics post-transplant in an animal model demonstrated that functional lymphatic drainage after lung transplant is achieved via sprouting of donor lung lymphatics and anastomosis of these vessels with those of the host (107).
The important role of lymphatics in lung transplant can be attributed to both the function of these vessels in fluid drainage as well as immune tolerance. On the one hand, lymphatic vessels transport antigen presenting cells loaded with allogeneic antigens to the draining lymph nodes which could lead to development of immune responses leading to graft rejection. On the other hand, lymphatic vessels help clear inflammatory molecules including hyaluronan (HA), which has been shown to be associated with rejection (108, 109). Important studies using an orthotopic mouse lung transplantation model showed that simulation of lymphangiogenesis with recombinant VEGFC suppresses lung rejection and promotes clearance of HA from rejected lung grafts, improving graft survival (110). In addition, genetic deletion of the lung lymphatics in a transplant model resulted in lung inflammation and the formation of bronchus-associated lymphoid tissue (BALT) in the lungs of mice (7). Interestingly, animal models suggest that severe rejection can be detected prior to the reestablishment of lymphatic drainage (111), and furthermore, that acute lung rejection can still occur in recipients that are completely devoid of secondary lymphoid organs (112). Therefore, several lines of data offer evidence for a therapeutic role for lymphatic function in lung transplant.
The lung lymphatics can themselves be a site of latent infection in tuberculosis (TB). Mycobacterium tuberculosis (Mtb) infection can cause lung lymphatic vasculitis that plays a role in Mtb dissemination by providing a direct route for spread from the initial site of infection in the lungs to the draining nodes (113–116). In addition, lymph nodes serve as a niche for Mtb growth and persistence (117). There is also evidence of interactions between the granulomas that form in Mtb infection and the lymphatic vasculature, as the granulomas may induce lymphangiogenesis (98), perhaps due to upregulation of a VEGFC (118, 119). In addition, extrapulmonary TB is caused by spread of the bacterium via the lymphatic system outside of the lung (120–122), providing a potential therapeutic target for this disease.
Chronic obstructive pulmonary disease (COPD) is most commonly caused by cigarette smoking and is characterized by progressive respiratory symptoms and airflow limitations that are not fully reversible (123, 124). Histologic analysis of human tissue has found increased lymphatic vessel density associated with the alveolar spaces (125) as well as an increased number of lymphoid follicles in patients with advanced COPD (126). In one study, upregulated expression of CCL21 and lymphatic chemokine scavenger receptor D6 was also observed in the lung lymphatics of patients with COPD (8). Recent studies using animal models have uncovered that lymphatic dysfunction may play a role in the pathogenesis of this disease. Mice with impaired lymphatic function develop many of the histologic and pathologic hallmarks of human COPD, including hypoxia, formation of lung lymphoid follicles, and airspace enlargement due to breakdown of elastin (7). Furthermore, cigarette smoke exposure causes lymphatic dysfunction with impaired drainage, decreased leukocyte trafficking, and prothrombotic lymph resulting in lymphatic thrombosis (6). Lung lymphatic dysfunction appears prior to the development of airspace enlargement in this model, suggesting that damage to the lymphatics is an early pathogenic event in this disease. Interestingly, lung lymphatic thrombosis is also seen in human COPD, and is correlated with disease severity (6). Further studies are needed to determine the mechanisms by which cigarette smoke causes lymphatic dysfunction and how lymphatic impairment drives lung injury.
Interstitial lung disease (ILD) is a debilitating disease characterized by chronic and progressive fibrosis and parenchymal remodeling. Changes in the lung lymphatics have been observed histologically in ILD, where increased alveolar lymphangiogenesis is correlated with collagen deposition and disease severity (127). In addition, though lymphatics are rarely found near alveoli in normal lungs, lymphatic vessels in the alveolar space are seen in the lungs of patients with interstitial pulmonary fibrosis, the most common form of ILD (128, 129). Severe damage of the subpleural and intralobular lymphatics has also been reported, with fragmented and disconnected vessels due to the massive fibrosis seen in this disease (130). Therefore, it is unclear whether the lymphangiogenesis seen in ILD results in functional vessels, and it is more likely that remodeling of the lung renders the lymphatic vessels unable for drain properly. Improving lymphatic function may be a novel therapeutic target in ILD, and preclinical models have indicated that stimulation of lymphatic proliferation may prevent fibrosis, suggesting a protective role for lymphatics in this disease (131).
Lymphangioleiomyomatosis (LAM) is a rare multisystem disease primarily affecting premenopausal women (132) that results from mutations in the tuberous sclerosis complex (TSC) genes TSC1, or TSC2 (4) in aberrant smooth muscle-like cells (LAM cells) that infiltrate the lungs, airways, and lymph nodes via the lymphatics (133). LAM is characterized by lymphangioleiomyomas that are comprised of the LAM cells and abnormal lymphatic channels lined by LECs (134, 135). Chylous effusions are often seen due to lymphatic dilation and dysfunction in the lungs (136). Around 70% of the patients have elevated levels of the lymphangiogenic factor VEGFD, which is a biomarker for the diagnosis of LAM (137), and is produced by the LAM cells (105). In addition, recent analysis using single cell RNA sequencing has identified transcriptional changes in LECs that suggest that crosstalk between the lymphatic endothelium and LAM cells may play an important role in the pathogenesis of this disease (138), representing a novel therapeutic target.
Complex lymphatic anomalies (CLAs) are rare disorders of embryonic lymphatic development and overlapping clinical symptoms caused by defects in the central collecting lymphatics (139–142). Generalized lymphatic anomaly (GLA) has multiorgan manifestations including in the lung, and is characterized by diffuse or multicentric proliferation of dilated lymphatic vessels (141, 143). The thoracic involvement in GLA can cause respiratory failure and is associated with poor prognosis (144). Kaposiform lymphangiomatosis (KLA) is another disease of abnormal lymphatic development and is characterized by foci of abnormal “kaposiform” spindle LECs (145, 146). Dilated and malformed lymphatic channels lined by a single layer of endothelial cells, pleural and pericardial effusions, and multiorgan involvement are common to both GLA and KLA, however patients with KLA exhibit more severe features and have coagulation disorders, hemorrhagic pericardial and pleural effusions, and some degree of fibrosis (139, 146). CLAs are generally caused by somatic mutations in genes that encode components of oncogenic growth factor signal transduction pathways, including PIK3/AKT/mTOR and RAS/MAPK (145, 147, 148). Accordingly, sirolimus, an inhibitor of mammalian target of rapamycin (mTOR), a kinase in the PI3K/AKT/mTOR pathway, appears effective at stabilizing signs/symptoms of disease in patients with GLA with mutations in this pathway (149, 150).
Tertiary lymphoid organs (TLOs), which in the lung are also known as inducible bronchus-associated lymphoid tissue (iBALT), are accumulations of lymphoid cells and resemble LNs in their cellular content, organization, high endothelial venules, and the presence of lymphatic vessels (151–153). Lymphatic vessels and lymphangiogenesis are key features of TLOs (152, 154) and presence of iBALT are a hallmark of chronic inflammatory lung diseases (155). iBALT are not present at the time of birth, however they can develop at any time postnatally after a pulmonary insult (156). iBALT formation is seen in association with diverse lung diseases including COPD, infection, rheumatoid arthritis-associated lung disease, and lung transplantation (157–162). They can be protective for lung injury in the case of infection, where iBALT result in improved viral and bacterial clearance in animal models (163–165). Conversely, iBALT can be a source of autoreactive antibodies and autoimmune lung injury in other settings, including COPD and rheumatoid arthritis (161, 166–169). In models of lung transplantation, iBALT are associated with development of antibody-mediated rejection (170, 171), however other models indicate that iBALT may be a source of regulatory T cells that promote graft tolerance (172–174). The ways in which the pathogenicity of iBALT is regulated is of great importance for studies of the role of these structures in lung disease. Interestingly, lymphatic dysfunction alone in mice is sufficient to cause iBALT formation, in the absence of any other inflammatory insult (7). Lymphatic function is also implicated in the formation of TLOs in the gut, where TLOs restrict lymphatic drainage and immune cell migration in a model of inflammatory bowel disease (175). Crosstalk between the lymphatics and immune cells may play an important role both in the formation of these structures and their function, and is subject of ongoing investigations.
Rather than passive conduits, the lymphatic vasculature is increasingly recognized for its role in modulating organ function and disease pathogenesis. Indeed, changes in lymphatic morphology or function have been observed in nearly every lung disease in which they have been studied. The explosion of research indicating the critical role of these vessels in other organs is a model on which to build more thorough and mechanistic studies of the lung lymphatics. Novel tools for imaging these vessels, identification and isolation of lymphatic endothelial cells, and animal models for manipulating lung lymphatic function will greatly aid in our ability to investigate lymphatic function in lung homeostasis and disease. Unpacking the molecular mechanisms that govern lymphatic function and how these changes in setting of lung disease will undoubtedly lead to new therapeutic targets.
AT drafted manuscript and generated figures and tables. HR edited manuscript and provided guidance on content. All authors contributed to the article and approved the submitted version.
Supported by the NHLBI K01HL145365 (H.O.R.) and the Robert Wood Johnson Foundation (H.O.R.).
The authors declare that the research was conducted in the absence of any commercial or financial relationships that could be construed as a potential conflict of interest.
All claims expressed in this article are solely those of the authors and do not necessarily represent those of their affiliated organizations, or those of the publisher, the editors and the reviewers. Any product that may be evaluated in this article, or claim that may be made by its manufacturer, is not guaranteed or endorsed by the publisher.
1. Hong, YK, Shin, JW, and Detmar, M. Development of the lymphatic vascular system: a mystery unravels. Dev Dyn. (2004) 231:462–73. doi: 10.1002/dvdy.20179
2. Xu, W, Harris, NR, and Caron, KM. Lymphatic vasculature: an emerging therapeutic target and drug delivery route. Annu Rev Med. (2021) 27:167–82. doi: 10.1146/annurev-med-051419-114417
3. Oliver, G, Kipnis, J, Randolph, GJ, and Harvey, NL. The lymphatic vasculature in the 21(st) century: novel functional roles in homeostasis and disease. Cell. (2020) 182:270–96. doi: 10.1016/j.cell.2020.06.039
4. Stump, B, Cui, Y, Kidambi, P, Lamattina, AM, and El-Chemaly, S. Lymphatic changes in respiratory diseases: more than just remodeling of the lung? Am J Respir Cell Mol Biol. (2017) 57:272–9. doi: 10.1165/rcmb.2016-0290TR
5. El-Chemaly, S, Levine, SJ, and Moss, J. Lymphatics in lung disease. Ann N Y Acad Sci. (2008) 1131:195–202. doi: 10.1196/annals.1413.017
6. Summers, BD, Kim, K, Clement, CC, Khan, Z, Thangaswamy, S, McCright, J, et al. Lung lymphatic thrombosis and dysfunction caused by cigarette smoke exposure precedes emphysema in mice. Sci Rep. (2022) 12:5012. doi: 10.1038/s41598-022-08617-y
7. Reed, HO, Wang, L, Sonett, J, Chen, M, Yang, J, Li, L, et al. Lymphatic impairment leads to pulmonary tertiary lymphoid organ formation and alveolar damage. J Clin Invest. (2019) 129:2514–26. doi: 10.1172/JCI125044
8. Mori, M, Andersson, CK, Graham, GJ, Löfdahl, CG, and Erjefält, JS. Increased number and altered phenotype of lymphatic vessels in peripheral lung compartments of patients with COPD. Respir Res. (2013) 14:65. doi: 10.1186/1465-9921-14-65
9. Jakus, Z, Gleghorn, JP, Enis, DR, Sen, A, Chia, S, Liu, X, et al. Lymphatic function is required prenatally for lung inflation at birth. J Exp Med. (2014) 211:815–26. doi: 10.1084/jem.20132308
10. Jafree, DJ, Long, DA, Scambler, PJ, and Ruhrberg, C. Mechanisms and cell lineages in lymphatic vascular development. Angiogenesis. (2021) 24:271–88. doi: 10.1007/s10456-021-09784-8
11. Semo, J, Nicenboim, J, and Yaniv, K. Development of the lymphatic system: new questions and paradigms. Development. (2016) 143:924–35. doi: 10.1242/dev.132431
12. Johnson, NC, Dillard, ME, Baluk, P, McDonald, DM, Harvey, NL, Frase, SL, et al. Lymphatic endothelial cell identity is reversible and its maintenance requires Prox1 activity. Genes Dev. (2008) 22:3282–91. doi: 10.1101/gad.1727208
13. Wigle, JT, Harvey, N, Detmar, M, Lagutina, I, Grosveld, G, Gunn, MD, et al. An essential role for Prox1 in the induction of the lymphatic endothelial cell phenotype. EMBO J. (2002) 21:1505–13. doi: 10.1093/emboj/21.7.1505
14. Wigle, JT, and Oliver, G. Prox1 function is required for the development of the murine lymphatic system. Cell. (1999) 98:769–78. doi: 10.1016/S0092-8674(00)81511-1
15. Karkkainen, MJ, Haiko, P, Sainio, K, Partanen, J, Taipale, J, Petrova, TV, et al. Vascular endothelial growth factor C is required for sprouting of the first lymphatic vessels from embryonic veins. Nat Immunol. (2004) 5:74–80. doi: 10.1038/ni1013
16. Kim, KW, and Song, JH. Emerging roles of lymphatic vasculature in immunity. Immune Netw. (2017) 17:68–76. doi: 10.4110/in.2017.17.1.68
17. Breslin, JW, Yang, Y, Scallan, JP, Sweat, RS, Adderley, SP, and Murfee, WL. Lymphatic vessel network structure and physiology. Compr Physiol. (2018) 9:207–99. doi: 10.1002/cphy.c180015
18. Baluk, P, Fuxe, J, Hashizume, H, Romano, T, Lashnits, E, Butz, S, et al. Functionally specialized junctions between endothelial cells of lymphatic vessels. J Exp Med. (2007) 204:2349–62. doi: 10.1084/jem.20062596
19. Muthuchamy, M, and Zawieja, D. Molecular regulation of lymphatic contractility. Ann N Y Acad Sci. (2008) 1131:89–99. doi: 10.1196/annals.1413.008
20. Srinivasan, RS, Dillard, ME, Lagutin, OV, Lin, FJ, Tsai, S, Tsai, MJ, et al. Lineage tracing demonstrates the venous origin of the mammalian lymphatic vasculature. Genes Dev. (2007) 21:2422–32. doi: 10.1101/gad.1588407
21. Sabin, FR . On the origin of the lymphatic system from the veins and the development of the lymph hearts and thoracic duct in the pig. Am J Anat. (1902) 1:367–89. doi: 10.1002/aja.1000010310
22. Oliver, G . Lymphatic vasculature development. Nat Rev Immunol. (2004) 4:35–45. doi: 10.1038/nri1258
23. Srinivasan, RS, Escobedo, N, Yang, Y, Interiano, A, Dillard, ME, Finkelstein, D, et al. The Prox1-Vegfr3 feedback loop maintains the identity and the number of lymphatic endothelial cell progenitors. Genes Dev. (2014) 28:2175–87. doi: 10.1101/gad.216226.113
24. Kulkarni, RM, Herman, A, Ikegami, M, Greenberg, JM, and Akeson, AL. Lymphatic ontogeny and effect of hypoplasia in developing lung. Mech Dev. (2011) 128:29–40. doi: 10.1016/j.mod.2010.09.003
25. Maldonado-Zimbron, VE, Hong, J, Russell, P, Trevaskis, NL, Windsor, JA, and Phillips, ARJ. Methods for studying pulmonary lymphatics. Eur Respir J. (2021) 57:2004106. doi: 10.1183/13993003.04106-2020
26. Leak, LV, and Jamuar, MP. Ultrastructure of pulmonary lymphatic vessels. Am Rev Respir Dis. (1983) 128:S59–65. doi: 10.1164/arrd.1983.128.2P2.S59
27. Dickie, R, Cormack, M, Semmler-Behnke, M, Kreyling, WG, and Tsuda, A. Deep pulmonary lymphatics in immature lungs. J Appl Physiol. (1985) 107:859–63. doi: 10.1152/japplphysiol.90665.2008
28. Schraufnagel, DE . Forms of lung lymphatics: a scanning electron microscopic study of casts. Anat Rec. (1992) 233:547–54. doi: 10.1002/ar.1092330409
29. Peão, MN, Aguas, AP, de Sá, CM, Pereira, AS, and Grande, NR. Scanning electron microscopy of the deep lymphatic network of the murine lung as viewed in corrosion casts. Lymphology. (1993) 26:42–8.
30. Schraufnagel, DE . Lung lymphatic anatomy and correlates. Pathophysiology. (2010) 17:337–43. doi: 10.1016/j.pathophys.2009.10.008
31. Choi, I, Chung, HK, Ramu, S, Lee, HN, Kim, KE, Lee, S, et al. Visualization of lymphatic vessels by Prox1-promoter directed GFP reporter in a bacterial artificial chromosome-based transgenic mouse. Blood. (2011) 117:362–5. doi: 10.1182/blood-2010-07-298562
32. Truman, LA, Bentley, KL, Smith, EC, Massaro, SA, Gonzalez, DG, Haberman, AM, et al. ProxTom lymphatic vessel reporter mice reveal Prox1 expression in the adrenal medulla, megakaryocytes, and platelets. Am J Pathol. (2012) 180:1715–25. doi: 10.1016/j.ajpath.2011.12.026
33. Kahn, HJ, Bailey, D, and Marks, A. Monoclonal antibody D2-40, a new marker of lymphatic endothelium, reacts with Kaposi's sarcoma and a subset of angiosarcomas. Mod Pathol. (2002) 15:434–40. doi: 10.1038/modpathol.3880543
34. Scavelli, C, Weber, E, Aglianò, M, Cirulli, T, Nico, B, Vacca, A, et al. Lymphatics at the crossroads of angiogenesis and lymphangiogenesis. J Anat. (2004) 204:433–49. doi: 10.1111/j.0021-8782.2004.00293.x
35. Baluk, P, and McDonald, DM. Imaging lymphatics in mouse lungs. Methods Mol Biol. (2018) 1846:161–80. doi: 10.1007/978-1-4939-8712-2_11
36. Ding, BS, Nolan, DJ, Butler, JM, James, D, Babazadeh, AO, Rosenwaks, Z, et al. Inductive angiocrine signals from sinusoidal endothelium are required for liver regeneration. Nature. (2010) 468:310–5. doi: 10.1038/nature09493
37. Rishi, AK, Joyce-Brady, M, Fisher, J, Dobbs, LG, Floros, J, VanderSpek, J, et al. Cloning, characterization, and development expression of a rat lung alveolar type I cell gene in embryonic endodermal and neural derivatives. Dev Biol. (1995) 167:294–306. doi: 10.1006/dbio.1995.1024
38. Vigl, B, Aebischer, D, Nitschké, M, Iolyeva, M, Röthlin, T, Antsiferova, O, et al. Tissue inflammation modulates gene expression of lymphatic endothelial cells and dendritic cell migration in a stimulus-dependent manner. Blood. (2011) 118:205–15. doi: 10.1182/blood-2010-12-326447
39. Huggenberger, R, Siddiqui, SS, Brander, D, Ullmann, S, Zimmermann, K, Antsiferova, M, et al. An important role of lymphatic vessel activation in limiting acute inflammation. Blood. (2011) 117:4667–78. doi: 10.1182/blood-2010-10-316356
40. Takizawa, H, Kondo, K, Fujino, H, Kenzaki, K, Miyoshi, T, Sakiyama, S, et al. The balance of VEGF-C and VEGFR-3 mRNA is a predictor of lymph node metastasis in non-small cell lung cancer. Br J Cancer. (2006) 95:75–9. doi: 10.1038/sj.bjc.6603209. Pubmed; PMID 16755294
41. Vanderbilt, JN, and Dobbs, LG. Characterization of the gene and promoter for RTI40, a differentiation marker of type I alveolar epithelial cells. Am J Respir Cell Mol Biol. (1998) 19:662–71. doi: 10.1165/ajrcmb.19.4.3121
42. Gunn, MD, Tangemann, K, Tam, C, Cyster, JG, Rosen, SD, and Williams, LT. A chemokine expressed in lymphoid high endothelial venules promotes the adhesion and chemotaxis of naive T lymphocytes. Proc Natl Acad Sci U S A. (1998) 95:258–63. doi: 10.1073/pnas.95.1.258
43. Manzo, A, Bugatti, S, Caporali, R, Prevo, R, Jackson, DG, Uguccioni, M, et al. CCL21 expression pattern of human secondary lymphoid organ stroma is conserved in inflammatory lesions with lymphoid neogenesis. Am J Pathol. (2007) 171:1549–62. doi: 10.2353/ajpath.2007.061275
44. Soltesz, EG, Kim, S, Laurence, RG, DeGrand, AM, Parungo, CP, Dor, DM, et al. Intraoperative sentinel lymph node mapping of the lung using near-infrared fluorescent quantum dots. Ann Thorac Surg. (2005) 79:269–77. doi: 10.1016/j.athoracsur.2004.06.055
45. Videira, MA, Botelho, MF, Santos, AC, Gouveia, LF, de Lima, JJ, and Almeida, AJ. Lymphatic uptake of pulmonary delivered radiolabelled solid lipid nanoparticles. J Drug Target. (2002) 10:607–13. doi: 10.1080/1061186021000054933
46. Itkin, M, and Nadolski, GJ. Modern techniques of lymphangiography and interventions: current status and future development. Cardiovasc Intervent Radiol. (2018) 41:366–76. doi: 10.1007/s00270-017-1863-2
47. Robinson, SK, Ramsden, JJ, Warner, J, Lackie, PM, and Roose, T. Correlative 3D imaging and microfluidic modelling of human pulmonary lymphatics using immunohistochemistry and high-resolution μCT. Sci Rep. (2019) 9:6415. doi: 10.1038/s41598-019-42794-7
48. Tammela, T, and Alitalo, K. Lymphangiogenesis: molecular mechanisms and future promise. Cells. (2010) 140:460–76. doi: 10.1016/j.cell.2010.01.045
49. Petrova, TV, and Koh, GY. Organ-specific lymphatic vasculature: from development to pathophysiology. J Exp Med. (2018) 215:35–49. doi: 10.1084/jem.20171868
50. Alitalo, K, Tammela, T, and Petrova, TV. Lymphangiogenesis in development and human disease. Nature. (2005) 438:946–53. doi: 10.1038/nature04480
51. Pearse, DB, Searcy, RM, Mitzner, W, Permutt, S, and Sylvester, JT. Effects of tidal volume and respiratory frequency on lung lymph flow. J Appl Physiol. (1985) 99:556–63. doi: 10.1152/japplphysiol.00254.2005
52. Grimbert, FA, Martin, D, Parker, JC, and Taylor, AE. Lymph flow during increases in pulmonary blood flow and microvascular pressure in dogs. Am J Phys. (1988) 255:H1149–55. doi: 10.1152/ajpheart.1988.255.5.H1149
53. Stritt, S, Koltowska, K, and Mäkinen, T. Homeostatic maintenance of the lymphatic vasculature. Trends Mol Med. (2021) 27:955–70. doi: 10.1016/j.molmed.2021.07.003
54. Bakocević, N, Worbs, T, Davalos-Misslitz, A, and Förster, R. T cell-dendritic cell interaction dynamics during the induction of respiratory tolerance and immunity. J Immunol. (2010) 184:1317–27. doi: 10.4049/jimmunol.0902277
55. Cook, DN, and Bottomly, K. Innate immune control of pulmonary dendritic cell trafficking. Proc Am Thorac Soc. (2007) 4:234–9. doi: 10.1513/pats.200701-026AW
56. Jahnsen, FL, Strickland, DH, Thomas, JA, Tobagus, IT, Napoli, S, Zosky, GR, et al. Accelerated antigen sampling and transport by airway mucosal dendritic cells following inhalation of a bacterial stimulus. J Immunol. (2006) 177:5861–7. doi: 10.4049/jimmunol.177.9.5861
57. Asokananthan, N, Graham, PT, Stewart, DJ, Bakker, AJ, Eidne, KA, Thompson, PJ, et al. House dust mite allergens induce proinflammatory cytokines from respiratory epithelial cells: the cysteine protease allergen, Der p 1, activates protease-activated receptor (PAR)-2 and inactivates PAR-1. J Immunol. (2002) 169:4572–8. doi: 10.4049/jimmunol.169.8.4572
58. Chieppa, M, Rescigno, M, Huang, AY, and Germain, RN. Dynamic imaging of dendritic cell extension into the small bowel lumen in response to epithelial cell TLR engagement. J Exp Med. (2006) 203:2841–52. doi: 10.1084/jem.20061884
59. Hammad, H, and Lambrecht, BN. Lung dendritic cell migration. Adv Immunol. (2007) 93:265–78. doi: 10.1016/s0065-2776(06)93007-7
60. Jakubzick, C, Helft, J, Kaplan, TJ, and Randolph, GJ. Optimization of methods to study pulmonary dendritic cell migration reveals distinct capacities of DC subsets to acquire soluble versus particulate antigen. J Immunol Methods. (2008) 337:121–31. doi: 10.1016/j.jim.2008.07.005
61. Vermaelen, KY, Carro-Muino, I, Lambrecht, BN, and Pauwels, RA. Specific migratory dendritic cells rapidly transport antigen from the airways to the thoracic lymph nodes. J Exp Med. (2001) 193:51–60. doi: 10.1084/jem.193.1.51
62. Wikstrom, ME, and Stumbles, PA. Mouse respiratory tract dendritic cell subsets and the immunological fate of inhaled antigens. Immunol Cell Biol. (2007) 85:182–8. doi: 10.1038/sj.icb.7100039
63. Holt, PG, and Stumbles, PA. Characterization of dendritic cell populations in the respiratory tract. J Aerosol Med. (2000) 13:361–7. doi: 10.1089/jam.2000.13.361
64. Holt, PG, Haining, S, Nelson, DJ, and Sedgwick, JD. Origin and steady-state turnover of class II MHC-bearing dendritic cells in the epithelium of the conducting airways. J Immunol. (1994) 153:256–61. doi: 10.4049/jimmunol.153.1.256
65. Liu, K, Victora, GD, Schwickert, TA, Guermonprez, P, Meredith, MM, Yao, K, et al. In vivo analysis of dendritic cell development and homeostasis. Science. (2009) 324:392–7. doi: 10.1126/science.1170540
66. Liu, H, Jakubzick, C, Osterburg, AR, Nelson, RL, Gupta, N, McCormack, FX, et al. Dendritic cell trafficking and function in rare lung diseases. Am J Respir Cell Mol Biol. (2017) 57:393–402. doi: 10.1165/rcmb.2017-0051PS
67. Liu, K, Waskow, C, Liu, X, Yao, K, Hoh, J, and Nussenzweig, M. Origin of dendritic cells in peripheral lymphoid organs of mice. Nat Immunol. (2007) 8:578–83. doi: 10.1038/ni1462
68. Legge, KL, and Braciale, TJ. Accelerated migration of respiratory dendritic cells to the regional lymph nodes is limited to the early phase of pulmonary infection. Immunity. (2003) 18:265–77. doi: 10.1016/S1074-7613(03)00023-2
69. Vermaelen, K, and Pauwels, R. Accelerated airway dendritic cell maturation, trafficking, and elimination in a mouse model of asthma. Am J Respir Cell Mol Biol. (2003) 29:405–9. doi: 10.1165/rcmb.2003-0008OC
70. Grayson, MH, Ramos, MS, Rohlfing, MM, Kitchens, R, Wang, HD, Gould, A, et al. Controls for lung dendritic cell maturation and migration during respiratory viral infection. J Immunol. (2007) 179:1438–48. doi: 10.4049/jimmunol.179.3.1438
71. GeurtsvanKessel, CH, and Lambrecht, BN. Division of labor between dendritic cell subsets of the lung. Mucosal Immunol. (2008) 1:442–50. doi: 10.1038/mi.2008.39
72. Jakubzick, C, Tacke, F, Llodra, J, van Rooijen, N, and Randolph, GJ. Modulation of dendritic cell trafficking to and from the airways. J Immunol. (2006) 176:3578–84. doi: 10.4049/jimmunol.176.6.3578
73. Ballesteros-Tato, A, León, B, Lund, FE, and Randall, TD. Temporal changes in dendritic cell subsets, cross-priming and costimulation via CD70 control CD8(+) T cell responses to influenza. Nat Immunol. (2010) 11:216–24. doi: 10.1038/ni.1838
74. Johnson, LA, and Jackson, DG. Control of dendritic cell trafficking in lymphatics by chemokines. Angiogenesis. (2014) 17:335–45. doi: 10.1007/s10456-013-9407-0
75. Tal, O, Lim, HY, Gurevich, I, Milo, I, Shipony, Z, Ng, LG, et al. DC mobilization from the skin requires docking to immobilized CCL21 on lymphatic endothelium and intralymphatic crawling. J Exp Med. (2011) 208:2141–53. doi: 10.1084/jem.20102392
76. Weber, M, Hauschild, R, Schwarz, J, Moussion, C, de Vries, I, Legler, DF, et al. Interstitial dendritic cell guidance by haptotactic chemokine gradients. Science. (2013) 339:328–32. doi: 10.1126/science.1228456
77. Russo, E, Teijeira, A, Vaahtomeri, K, Willrodt, AH, Bloch, JS, Nitschke, M, et al. Intralymphatic CCL21 promotes tissue egress of dendritic cells through afferent lymphatic vessels. Cell Rep. (2016) 14:1723–34. doi: 10.1016/j.celrep.2016.01.048
78. Hintzen, G, Ohl, L, del Rio, ML, Rodriguez-Barbosa, JI, Pabst, O, Kocks, JR, et al. Induction of tolerance to innocuous inhaled antigen relies on a CCR7-dependent dendritic cell-mediated antigen transport to the bronchial lymph node. J Immunol. (2006) 177:7346–54. doi: 10.4049/jimmunol.177.10.7346
79. Dieu, MC, Vanbervliet, B, Vicari, A, Bridon, JM, Oldham, E, Aït-Yahia, S, et al. Selective recruitment of immature and mature dendritic cells by distinct chemokines expressed in different anatomic sites. J Exp Med. (1998) 188:373–86. doi: 10.1084/jem.188.2.373
80. Ricart, BG, John, B, Lee, D, Hunter, CA, and Hammer, DA. Dendritic cells distinguish individual chemokine signals through CCR7 and CXCR4. J Immunol. (2011) 186:53–61. doi: 10.4049/jimmunol.1002358
81. Johnson, LA, Clasper, S, Holt, AP, Lalor, PF, Baban, D, and Jackson, DG. An inflammation-induced mechanism for leukocyte transmigration across lymphatic vessel endothelium. J Exp Med. (2006) 203:2763–77. doi: 10.1084/jem.20051759
82. Pham, TH, Okada, T, Matloubian, M, Lo, CG, and Cyster, JG. S1P1 receptor signaling overrides retention mediated by G alpha i-coupled receptors to promote T cell egress. Immunity. (2008) 28:122–33. doi: 10.1016/j.immuni.2007.11.017
83. Mandala, S, Hajdu, R, Bergstrom, J, Quackenbush, E, Xie, J, Milligan, J, et al. Alteration of lymphocyte trafficking by sphingosine-1-phosphate receptor agonists. Science. (2002) 296:346–9. doi: 10.1126/science.1070238
84. Yen, JH, Khayrullina, T, and Ganea, D. PGE2-induced metalloproteinase-9 is essential for dendritic cell migration. Blood. (2008) 111:260–70. doi: 10.1182/blood-2007-05-090613
85. Hough, KP, Curtiss, ML, Blain, TJ, Liu, RM, Trevor, J, Deshane, JS, et al. Airway remodeling in asthma. Front Med. (2020) 7:191. doi: 10.3389/fmed.2020.00191
86. Ebina, M . Remodeling of airway walls in fatal asthmatics decreases lymphatic distribution; beyond thickening of airway smooth muscle layers. Allergol Int. (2008) 57:165–74. doi: 10.2332/allergolint.O-07-497
87. Baluk, P, Yao, LC, Feng, J, Romano, T, Jung, SS, Schreiter, JL, et al. TNF-alpha drives remodeling of blood vessels and lymphatics in sustained airway inflammation in mice. J Clin Invest. (2009) 119:2954–64. doi: 10.1172/JCI37626
88. Yao, LC, Baluk, P, Feng, J, and McDonald, DM. Steroid-resistant lymphatic remodeling in chronically inflamed mouse airways. Am J Pathol. (2010) 176:1525–41. doi: 10.2353/ajpath.2010.090909
89. Moldobaeva, A, Jenkins, J, Zhong, Q, and Wagner, EM. Lymphangiogenesis in rat asthma model. Angiogenesis. (2017) 20:73–84. doi: 10.1007/s10456-016-9529-2
90. Baluk, P, Tammela, T, Ator, E, Lyubynska, N, Achen, MG, Hicklin, DJ, et al. Pathogenesis of persistent lymphatic vessel hyperplasia in chronic airway inflammation. J Clin Invest. (2005) 115:247–57. doi: 10.1172/JCI200522037
91. Maisel, K, Hrusch, CL, Medellin, JEG, Potin, L, Chapel, DB, Nurmi, H, et al. Pro-lymphangiogenic VEGFR-3 signaling modulates memory T cell responses in allergic airway inflammation. Mucosal Immunol. (2021) 14:144–51. doi: 10.1038/s41385-020-0308-4
92. Valeyre, D, Nunes, H, and Bernaudin, JF. Advanced pulmonary sarcoidosis. Curr Opin Pulm Med. (2014) 20:488–95. doi: 10.1097/MCP.0000000000000075
93. El Jammal, T, Pavic, M, Gerfaud-Valentin, M, Jamilloux, Y, and Sève, P. Sarcoidosis and cancer: a complex relationship. Front Med. (2020) 7:594118. doi: 10.3389/fmed.2020.594118
94. Rao, DA, and Dellaripa, PF. Extrapulmonary manifestations of sarcoidosis. Rheum Dis Clin N Am. (2013) 39:277–97. doi: 10.1016/j.rdc.2013.02.007
95. Rosen, Y, Vuletin, JC, Pertschuk, LP, and Silverstein, E. Sarcoidosis: from the pathologist's vantage point. Pathol Annu. (1979) 14:405–39.
96. Kambouchner, M, Pirici, D, Uhl, JF, Mogoanta, L, Valeyre, D, and Bernaudin, JF. Lymphatic and blood microvasculature organisation in pulmonary sarcoid granulomas. Eur Respir J. (2011) 37:835–40. doi: 10.1183/09031936.00086410
97. Yamashita, M, Mouri, T, Niisato, M, Kowada, K, Kobayashi, H, Chiba, R, et al. Heterogeneous characteristics of lymphatic microvasculatures associated with pulmonary sarcoid granulomas. Ann Am Thorac Soc. (2013) 10:90–7. doi: 10.1513/AnnalsATS.201209-078OC
98. Patterson, KC, Queval, CJ, and Gutierrez, MG. Granulomatous inflammation in tuberculosis and sarcoidosis: does the lymphatic system contribute to disease? BioEssays. (2019) 41:e1900086. doi: 10.1002/bies.201900086
99. Biener, L, Kruse, J, Tuleta, I, Pizarro, C, Kreuter, M, Birring, SS, et al. Association of proangiogenic and profibrotic serum markers with lung function and quality of life in sarcoidosis. PLoS One. (2021) 16:e0247197. doi: 10.1371/journal.pone.0247197
100. Liu, Y, Liu, Y, Su, L, and Jiang, SJ. Recipient-related clinical risk factors for primary graft dysfunction after lung transplantation: a systematic review and meta-analysis. PLoS One. (2014) 9:e92773. doi: 10.1371/journal.pone.0092773
101. Chambers, DC, Yusen, RD, Cherikh, WS, Goldfarb, SB, Kucheryavaya, AY, Khusch, K, et al. The registry of the International Society for Heart and Lung Transplantation: thirty-fourth adult lung and heart-lung transplantation Report-2017; focus theme: allograft ischemic time. J Heart Lung Transplant. (2017) 36:1047–59. doi: 10.1016/j.healun.2017.07.016
102. Boehler, A . Update on cystic fibrosis: selected aspects related to lung transplantation. Swiss Med Wkly. (2003) 133:111–7. doi: 10.4414/smw.2003.10019
103. Trulock, EP, Christie, JD, Edwards, LB, Boucek, MM, Aurora, P, Taylor, DO, et al. Registry of the International Society for Heart and Lung Transplantation: twenty-fourth official adult lung and heart-lung transplantation report-2007. J Heart Lung Transplant. (2007) 26:782–95. doi: 10.1016/j.healun.2007.06.003
104. Ruggiero, R, Fietsam, R Jr, Thomas, GA, Muz, J, Farris, RH, Kowal, TA, et al. Detection of canine allograft lung rejection by pulmonary lymphoscintigraphy. J Thorac Cardiovasc Surg. (1994) 108:253–8.
105. Cui, Y, Liu, K, Lamattina, AM, Visner, G, and El-Chemaly, S. Lymphatic vessels: the next frontier in lung transplant. Ann Am Thorac Soc. (2017) 14:S226–32. doi: 10.1513/AnnalsATS.201606-465MG
106. Dashkevich, A, Heilmann, C, Kayser, G, Germann, M, Beyersdorf, F, Passlick, B, et al. Lymph angiogenesis after lung transplantation and relation to acute organ rejection in humans. Ann Thorac Surg. (2010) 90:406–11. doi: 10.1016/j.athoracsur.2010.03.013
107. Outtz Reed, H, Wang, L, Kahn, ML, and Hancock, WW. Donor-host lymphatic anastomosis after murine lung transplantation. Transplantation. (2020) 104:511–5. doi: 10.1097/TP.0000000000003041
108. Tesar, BM, Jiang, D, Liang, J, Palmer, SM, Noble, PW, and Goldstein, DR. The role of hyaluronan degradation products as innate alloimmune agonists. Am J Transplant. (2006) 6:2622–35. doi: 10.1111/j.1600-6143.2006.01537.x
109. Todd, JL, Wang, X, Sugimoto, S, Kennedy, VE, Zhang, HL, Pavlisko, EN, et al. Hyaluronan contributes to bronchiolitis obliterans syndrome and stimulates lung allograft rejection through activation of innate immunity. Am J Respir Crit Care Med. (2014) 189:556–66. doi: 10.1164/rccm.201308-1481OC
110. Cui, Y, Liu, K, Monzon-Medina, ME, Padera, RF, Wang, H, George, G, et al. Therapeutic lymphangiogenesis ameliorates established acute lung allograft rejection. J Clin Invest. (2015) 125:4255–68. doi: 10.1172/JCI79693
111. Krupnick, AS, Lin, X, Li, W, Higashikubo, R, Zinselmeyer, BH, Hartzler, H, et al. Central memory CD8+ T lymphocytes mediate lung allograft acceptance. J Clin Invest. (2014) 124:1130–43. doi: 10.1172/JCI71359
112. Gelman, AE, Li, W, Richardson, SB, Zinselmeyer, BH, Lai, J, Okazaki, M, et al. Cutting edge: acute lung allograft rejection is independent of secondary lymphoid organs. J Immunol. (2009) 182:3969–73. doi: 10.4049/jimmunol.0803514
113. Basaraba, RJ, Smith, EE, Shanley, CA, and Orme, IM. Pulmonary lymphatics are primary sites of mycobacterium tuberculosis infection in Guinea pigs infected by aerosol. Infect Immun. (2006) 74:5397–401. doi: 10.1128/IAI.00332-06
114. Kraft, SL, Dailey, D, Kovach, M, Stasiak, KL, Bennett, J, McFarland, CT, et al. Magnetic resonance imaging of pulmonary lesions in Guinea pigs infected with mycobacterium tuberculosis. Infect Immun. (2004) 72:5963–71. doi: 10.1128/IAI.72.10.5963-5971.2004
115. Chackerian, AA, Alt, JM, Perera, TV, Dascher, CC, and Behar, SM. Dissemination of mycobacterium tuberculosis is influenced by host factors and precedes the initiation of T-cell immunity. Infect Immun. (2002) 70:4501–9. doi: 10.1128/IAI.70.8.4501-4509.2002
116. Tian, T, Woodworth, J, Sköld, M, and Behar, SM. In vivo depletion of CD11c+ cells delays the CD4+ T cell response to mycobacterium tuberculosis and exacerbates the outcome of infection. J Immunol. (2005) 175:3268–72. doi: 10.4049/jimmunol.175.5.3268
117. Ganchua, SKC, White, AG, Klein, EC, and Flynn, JL. Lymph nodes-the neglected battlefield in tuberculosis. PLoS Pathog. (2020) 16:e1008632. doi: 10.1371/journal.ppat.1008632
118. Harding, J, Ritter, A, Rayasam, A, Fabry, Z, and Sandor, M. Lymphangiogenesis is induced by mycobacterial granulomas via vascular endothelial growth factor receptor-3 and supports systemic T-cell responses against mycobacterial antigen. Am J Pathol. (2015) 185:432–45. doi: 10.1016/j.ajpath.2014.09.020
119. Kumar, NP, Banurekha, VV, Nair, D, and Babu, S. Circulating Angiogenic factors as biomarkers of disease severity and bacterial burden in pulmonary tuberculosis. PLoS One. (2016) 11:e0146318. doi: 10.1371/journal.pone.0146318
120. Baykan, AH, Sayiner, HS, Aydin, E, Koc, M, Inan, I, and Erturk, SM. Extrapulmonary tuberculosıs: an old but resurgent problem. Insights Imaging. (2022) 13:39. doi: 10.1186/s13244-022-01172-0
121. Rodriguez-Takeuchi, SY, Renjifo, ME, and Medina, FJ. Extrapulmonary tuberculosis: pathophysiology and imaging findings. Radiographics. (2019) 39:2023–37. doi: 10.1148/rg.2019190109
122. Lerner, TR, de Souza, C-WC, Repnik, U, Russell, MR, Borel, S, Diedrich, CR, et al. Lymphatic endothelial cells are a replicative niche for mycobacterium tuberculosis. J Clin Invest. (2016) 126:1093–108. doi: 10.1172/JCI83379
123. Vestbo, J, Hurd, SS, Agustí, AG, Jones, PW, Vogelmeier, C, Anzueto, A, et al. Global strategy for the diagnosis, management, and prevention of chronic obstructive pulmonary disease: GOLD executive summary. Am J Respir Crit Care Med. (2013) 187:347–65. doi: 10.1164/rccm.201204-0596PP
124. Hogg, JC . Why does airway inflammation persist after the smoking stops? Thorax. (2006) 61:96–7. doi: 10.1136/thx.2005.049502
125. Hardavella, G, Tzortzaki, EG, Siozopoulou, V, Galanis, P, Vlachaki, E, Avgousti, M, et al. Lymphangiogenesis in COPD: another link in the pathogenesis of the disease. Respir Med. (2012) 106:687–93. doi: 10.1016/j.rmed.2011.11.011
126. Hogg, JC, Paré, PD, and Hackett, TL. The contribution of small airway obstruction to the pathogenesis of chronic obstructive pulmonary disease. Physiol Rev. (2017) 97:529–52. doi: 10.1152/physrev.00025.2015
127. Lara, AR, Cosgrove, GP, Janssen, WJ, Huie, TJ, Burnham, EL, Heinz, DE, et al. Increased lymphatic vessel length is associated with the fibroblast reticulum and disease severity in usual interstitial pneumonia and nonspecific interstitial pneumonia. Chest. (2012) 142:1569–76. doi: 10.1378/chest.12-0029
128. El-Chemaly, S, Malide, D, Zudaire, E, Ikeda, Y, Weinberg, BA, Pacheco-Rodriguez, G, et al. Abnormal lymphangiogenesis in idiopathic pulmonary fibrosis with insights into cellular and molecular mechanisms. Proc Natl Acad Sci U S A. (2009) 106:3958–63. doi: 10.1073/pnas.0813368106
129. Yamashita, M, Iwama, N, Date, F, Chiba, R, Ebina, M, Miki, H, et al. Characterization of lymphangiogenesis in various stages of idiopathic diffuse alveolar damage. Hum Pathol. (2009) 40:542–51. doi: 10.1016/j.humpath.2008.06.031
130. Ebina, M, Shibata, N, Ohta, H, Hisata, S, Tamada, T, Ono, M, et al. The disappearance of subpleural and interlobular lymphatics in idiopathic pulmonary fibrosis. Lymphat Res Biol. (2010) 8:199–207. doi: 10.1089/lrb.2010.0008
131. Baluk, P, Naikawadi, RP, Kim, S, Rodriguez, F, Choi, D, Hong, YK, et al. Lymphatic proliferation ameliorates pulmonary fibrosis after lung injury. Am J Pathol. (2020) 190:2355–75. doi: 10.1016/j.ajpath.2020.08.018
132. Glasgow, CG, Taveira-Dasilva, AM, Darling, TN, and Moss, J. Lymphatic involvement in lymphangioleiomyomatosis. Ann N Y Acad Sci. (2008) 1131:206–14. doi: 10.1196/annals.1413.018
133. Cong, CV, Anh, TT, Ly, TT, and Duc, NM. Pulmonary lymphangioleiomyomatosis (LAM): a literature overview and case report. Radiol Case Rep. (2022) 17:1646–55. doi: 10.1016/j.radcr.2022.02.075
134. Steagall, WK, Pacheco-Rodriguez, G, Darling, TN, Torre, O, Harari, S, and Moss, J. The Lymphangioleiomyomatosis lung cell and its human cell models. Am J Respir Cell Mol Biol. (2018) 58:678–83. doi: 10.1165/rcmb.2017-0403TR
135. Pacheco-Rodriguez, G, Glasgow, CG, Ikeda, Y, Steagall, WK, Yu, ZX, Tsukada, K, et al. A mixed blood-lymphatic endothelial cell phenotype in Lymphangioleiomyomatosis and idiopathic pulmonary fibrosis but not in Kaposi's sarcoma or tuberous sclerosis complex. Am J Respir Cell Mol Biol. (2022) 66:337–40. doi: 10.1165/rcmb.2021-0293LE
136. Glasgow, CG, El-Chemaly, S, and Moss, J. Lymphatics in lymphangioleiomyomatosis and idiopathic pulmonary fibrosis. Eur Respir Rev. (2012) 21:196–206. doi: 10.1183/09059180.00009311
137. Young, L, Lee, HS, Inoue, Y, Moss, J, Singer, LG, Strange, C, et al. Serum VEGF-D a concentration as a biomarker of lymphangioleiomyomatosis severity and treatment response: a prospective analysis of the multicenter international Lymphangioleiomyomatosis efficacy of Sirolimus (MILES) trial. Lancet Respir Med. (2013) 1:445–52. doi: 10.1016/S2213-2600(13)70090-0
138. Guo, M, Yu, JJ, Perl, AK, Wikenheiser-Brokamp, KA, Riccetti, M, Zhang, EY, et al. Single-cell Transcriptomic analysis identifies a unique pulmonary Lymphangioleiomyomatosis cell. Am J Respir Crit Care Med. (2020) 202:1373–87. doi: 10.1164/rccm.201912-2445OC
139. Mäkinen, T, Boon, LM, Vikkula, M, and Alitalo, K. Lymphatic malformations: genetics, mechanisms and therapeutic strategies. Circ Res. (2021) 129:136–54. doi: 10.1161/CIRCRESAHA.121.318142
140. Trenor, CC 3rd, and Chaudry, G. Complex lymphatic anomalies. Semin Pediatr Surg. (2014) 23:186–90. doi: 10.1053/j.sempedsurg.2014.07.006
141. Ozeki, M, Fujino, A, Matsuoka, K, Nosaka, S, Kuroda, T, and Fukao, T. Clinical features and prognosis of generalized lymphatic anomaly, Kaposiform Lymphangiomatosis, and Gorham-stout disease. Pediatr Blood Cancer. (2016) 63:832–8. doi: 10.1002/pbc.25914
142. Iacobas, I, Adams, DM, Pimpalwar, S, Phung, T, Blei, F, Burrows, P, et al. Multidisciplinary guidelines for initial evaluation of complicated lymphatic anomalies-expert opinion consensus. Pediatr Blood Cancer. (2020) 67:e28036. doi: 10.1002/pbc.28036
143. Adams, DM, and Ricci, KW. Vascular anomalies: diagnosis of complicated anomalies and new medical treatment options. Hematol Oncol Clin North Am. (2019) 33:455–70. doi: 10.1016/j.hoc.2019.01.011
144. Luisi, F, Torre, O, and Harari, S. Thoracic involvement in generalised lymphatic anomaly (or lymphangiomatosis). Eur Respir Rev. (2016) 25:170–7. doi: 10.1183/16000617.0018-2016
145. Ozeki, M, Aoki, Y, Nozawa, A, Yasue, S, Endo, S, Hori, Y, et al. Detection of NRAS mutation in cell-free DNA biological fluids from patients with kaposiform lymphangiomatosis. Orphanet J Rare Dis. (2019) 14:215. doi: 10.1186/s13023-019-1191-5
146. Croteau, SE, Kozakewich, HP, Perez-Atayde, AR, Fishman, SJ, Alomari, AI, Chaudry, G, et al. Kaposiform lymphangiomatosis: a distinct aggressive lymphatic anomaly. J Pediatr. (2014) 164:383–8. doi: 10.1016/j.jpeds.2013.10.013
147. Rodriguez-Laguna, L, Agra, N, Ibañez, K, Oliva-Molina, G, Gordo, G, Khurana, N, et al. Somatic activating mutations in PIK3CA cause generalized lymphatic anomaly. J Exp Med. (2019) 216:407–18. doi: 10.1084/jem.20181353
148. Barclay, SF, Inman, KW, Luks, VL, McIntyre, JB, Al-Ibraheemi, A, Church, AJ, et al. A somatic activating NRAS variant associated with kaposiform lymphangiomatosis. Genet Med. (2019) 21:1517–24. doi: 10.1038/s41436-018-0390-0
149. Adams, DM, Trenor, CC 3rd, Hammill, AM, Vinks, AA, Patel, MN, Chaudry, G, et al. Efficacy and safety of Sirolimus in the treatment of complicated vascular anomalies. Pediatrics. (2016) 137:e20153257. doi: 10.1542/peds.2015-3257
150. Ricci, KW, Hammill, AM, Mobberley-Schuman, P, Nelson, SC, Blatt, J, Bender, JLG, et al. Efficacy of systemic sirolimus in the treatment of generalized lymphatic anomaly and Gorham-stout disease. Pediatr Blood Cancer. (2019) 66:e27614. doi: 10.1002/pbc.27614
151. Ruddle, NH . Lymphatic vessels and tertiary lymphoid organs. J Clin Invest. (2014) 124:953–9. doi: 10.1172/JCI71611
152. Stranford, S, and Ruddle, NH. Follicular dendritic cells, conduits, lymphatic vessels, and high endothelial venules in tertiary lymphoid organs: parallels with lymph node stroma. Front Immunol. (2012) 3:350. doi: 10.3389/fimmu.2012.00350
153. Jones, GW, Hill, DG, and Jones, SA. Understanding immune cells in tertiary lymphoid organ development: it is all starting to come together. Front Immunol. (2016) 7:401. doi: 10.3389/fimmu.2016.00401
154. Baluk, P, Adams, A, Phillips, K, Feng, J, Hong, YK, Brown, MB, et al. Preferential lymphatic growth in bronchus-associated lymphoid tissue in sustained lung inflammation [research support, N.I.H., extramural research support, non-U.S. Gov't]. Am J Pathol. (2014) 184:1577–92. doi: 10.1016/j.ajpath.2014.01.021
155. Aloisi, F, and Pujol-Borrell, R. Lymphoid neogenesis in chronic inflammatory diseases. Nat Rev Immunol. (2006) 6:205–17. doi: 10.1038/nri1786
156. Hwang, JY, Silva-Sanchez, A, Carragher, DM, Garcia-Hernandez, ML, Rangel-Moreno, J, and Randall, TD. Inducible bronchus-associated lymphoid tissue (iBALT) attenuates pulmonary pathology in a mouse model of allergic airway disease. Front Immunol. (2020) 11:570661. doi: 10.3389/fimmu.2020.570661
157. Elliot, JG, Jensen, CM, Mutavdzic, S, Lamb, JP, Carroll, NG, and James, AL. Aggregations of lymphoid cells in the airways of nonsmokers, smokers, and subjects with asthma. Am J Respir Crit Care Med. (2004) 169:712–8. doi: 10.1164/rccm.200308-1167OC
158. Lee, JJ, McGarry, MP, Farmer, SC, Denzler, KL, Larson, KA, Carrigan, PE, et al. Interleukin-5 expression in the lung epithelium of transgenic mice leads to pulmonary changes pathognomonic of asthma. J Exp Med. (1997) 185:2143–56. doi: 10.1084/jem.185.12.2143
159. Gosman, MM, Willemse, BW, Jansen, DF, Lapperre, TS, van Schadewijk, A, Hiemstra, PS, et al. Increased number of B-cells in bronchial biopsies in COPD. Eur Respir J. (2006) 27:60–4. doi: 10.1183/09031936.06.00007005
160. Hogg, JC, Chu, F, Utokaparch, S, Woods, R, Elliott, WM, Buzatu, L, et al. The nature of small-airway obstruction in chronic obstructive pulmonary disease. N Engl J Med. (2004) 350:2645–53. doi: 10.1056/NEJMoa032158
161. Rangel-Moreno, J, Hartson, L, Navarro, C, Gaxiola, M, Selman, M, and Randall, TD. Inducible bronchus-associated lymphoid tissue (iBALT) in patients with pulmonary complications of rheumatoid arthritis. J Clin Invest. (2006) 116:3183–94. doi: 10.1172/JCI28756
162. Hasegawa, T, Iacono, A, and Yousem, SA. The significance of bronchus-associated lymphoid tissue in human lung transplantation: is there an association with acute and chronic rejection? Transplantation. (1999) 67:381–5. doi: 10.1097/00007890-199902150-00007
163. Foo, SY, and Phipps, S. Regulation of inducible BALT formation and contribution to immunity and pathology. Mucosal Immunol. (2010) 3:537–44. doi: 10.1038/mi.2010.52
164. Wiley, JA, Richert, LE, Swain, SD, Harmsen, A, Barnard, DL, Randall, TD, et al. Inducible bronchus-associated lymphoid tissue elicited by a protein cage nanoparticle enhances protection in mice against diverse respiratory viruses. PLoS One. (2009) 4:e7142. doi: 10.1371/journal.pone.0007142
165. Ramakrishnan, L . Revisiting the role of the granuloma in tuberculosis. Nat Rev Immunol. (2012) 12:352–66. doi: 10.1038/nri3211
166. Feghali-Bostwick, CA, Gadgil, AS, Otterbein, LE, Pilewski, JM, Stoner, MW, Csizmadia, E, et al. Autoantibodies in patients with chronic obstructive pulmonary disease. Am J Respir Crit Care Med. (2008) 177:156–63. doi: 10.1164/rccm.200701-014OC
167. Demoor, T, Bracke, KR, Maes, T, Vandooren, B, Elewaut, D, Pilette, C, et al. Role of lymphotoxin-alpha in cigarette smoke-induced inflammation and lymphoid neogenesis. Eur Respir J. (2009) 34:405–16. doi: 10.1183/09031936.00101408
168. Caramori, G, Ruggeri, P, Di Stefano, A, Mumby, S, Girbino, G, Adcock, IM, et al. Autoimmunity and COPD: clinical implications. Chest. (2018) 153:1424–31. doi: 10.1016/j.chest.2017.10.033
169. Silva-Sanchez, A, and Randall, TD. Role of iBALT in respiratory immunity. Curr Top Microbiol Immunol. (2020) 426:21–43. doi: 10.1007/82_2019_191
170. Prop, J, Wildevuur, CR, and Nieuwenhuis, P. Lung allograft rejection in the rat. II. Specific immunological properties of lung grafts. Transplantation. (1985) 40:126–31. doi: 10.1097/00007890-198508000-00003
171. Bery, AI, and Hachem, RR. Antibody-mediated rejection after lung transplantation. Ann Transl Med. (2020) 8:411. doi: 10.21037/atm.2019.11.86
172. Li, W, Gauthier, JM, Higashikubo, R, Hsiao, HM, Tanaka, S, Vuong, L, et al. Bronchus-associated lymphoid tissue-resident Foxp3+ T lymphocytes prevent antibody-mediated lung rejection. J Clin Invest. (2019) 129:556–68. doi: 10.1172/JCI122083
173. Li, W, Bribriesco, AC, Nava, RG, Brescia, AA, Ibricevic, A, Spahn, JH, et al. Lung transplant acceptance is facilitated by early events in the graft and is associated with lymphoid neogenesis. Mucosal Immunol. (2012) 5:544–54. doi: 10.1038/mi.2012.30
174. Tanaka, S, Gauthier, JM, Fuchs, A, Li, W, Tong, AY, Harrison, MS, et al. IL-22 is required for the induction of bronchus-associated lymphoid tissue in tolerant lung allografts. Am J Transplant. (2020) 20:1251–61. doi: 10.1111/ajt.15701
175. Czepielewski, RS, Erlich, EC, Onufer, EJ, Young, S, Saunders, BT, Han, YH, et al. Ileitis-associated tertiary lymphoid organs arise at lymphatic valves and impede mesenteric lymph flow in response to tumor necrosis factor. Immunity. (2021) 54:2795–2811.e9. Declaration of interests The authors declare no competing interests. doi: 10.1016/j.immuni.2021.10.003
Keywords: lung lymphatics, LECs, lymphatic dysfunction, inflammation, lung disease
Citation: Trivedi A and Reed HO (2023) The lymphatic vasculature in lung function and respiratory disease. Front. Med. 10:1118583. doi: 10.3389/fmed.2023.1118583
Received: 08 December 2022; Accepted: 23 February 2023;
Published: 14 March 2023.
Edited by:
Dawei Yang, Fudan University, ChinaReviewed by:
Sathish Srinivasan, Oklahoma Medical Research Foundation, United StatesCopyright © 2023 Trivedi and Reed. This is an open-access article distributed under the terms of the Creative Commons Attribution License (CC BY). The use, distribution or reproduction in other forums is permitted, provided the original author(s) and the copyright owner(s) are credited and that the original publication in this journal is cited, in accordance with accepted academic practice. No use, distribution or reproduction is permitted which does not comply with these terms.
*Correspondence: Hasina Outtz Reed, aGhvMjAwMUBtZWQuY29ybmVsbC5lZHU=
Disclaimer: All claims expressed in this article are solely those of the authors and do not necessarily represent those of their affiliated organizations, or those of the publisher, the editors and the reviewers. Any product that may be evaluated in this article or claim that may be made by its manufacturer is not guaranteed or endorsed by the publisher.
Research integrity at Frontiers
Learn more about the work of our research integrity team to safeguard the quality of each article we publish.