- 1International Society of Microbiota, Tokyo, Japan
- 2INSERM, CNRS, Institut Cochin, Université de Paris, Paris, France
- 3Laboratory of Excellence GR-Ex, Paris, France
- 4Department of Life Sciences, University of Bath, Bath, United Kingdom
- 5Medicines Development, Centre for Therapeutic Innovation, University of Bath, Bath, United Kingdom
Iron is a double-edged sword. It is vital for all that’s living, yet its deficiency or overload can be fatal. In humans, iron homeostasis is tightly regulated at both cellular and systemic levels. Extracellular vesicles (EVs), now known as major players in cellular communication, potentially play an important role in regulating iron metabolism. The gut microbiota was also recently reported to impact the iron metabolism process and indirectly participate in regulating iron homeostasis, yet there is no proof of whether or not microbiota-derived EVs interfere in this relationship. In this review, we discuss the implication of EVs on iron metabolism and homeostasis. We elaborate on the blooming role of gut microbiota in iron homeostasis while focusing on the possible EVs contribution. We conclude that EVs are extensively involved in the complex iron metabolism process; they carry ferritin and express transferrin receptors. Bone marrow-derived EVs even induce hepcidin expression in β-thalassemia. The gut microbiota, in turn, affects iron homeostasis on the level of iron absorption and possibly macrophage iron recycling, with still no proof of the interference of EVs. This review is the first step toward understanding the multiplex iron metabolism process. Targeting extracellular vesicles and gut microbiota-derived extracellular vesicles will be a huge challenge to treat many diseases related to iron metabolism alteration.
Introduction
Iron is a vital trace element, essential for several fundamental processes: globin synthesis and erythropoiesis (1), energy production (2), DNA synthesis and repair (3), and immune function (4). Its mode of action lies in its ability to reversibly gain or lose a single electron to participate in oxidation-reduction reactions, which also catalyze the generation of reactive oxygen species (ROS) (5). Hence, in spite of its vital role iron overload is toxic due to its ability to generate ROS and trigger cell death (6). Excess of free reactive iron leads to several types of cell death, including ferroptosis, the oxidative cell death prompted by the accumulation of iron-mediated lipid peroxidation (6).
No wonder, iron metabolism is a tightly regulated process. The regulation of iron metabolism occurs at a cellular level via transcriptional and post-transcriptional regulation of iron genes. At the systemic level, iron metabolism is regulated via the hepatic hormone hepcidin, which regulates iron absorption, plasma concentrations, and tissue distribution (7). The human microbiome, in turn, was recently identified as an effector in the iron metabolism regulation process, considering the fact that the microbiota also requires iron to maintain symbiosis. The composition of the gut microbiota is affected by iron availability, and microbiota-derived metabolites were reported to impact both iron absorption (enterocyte) and iron recycling (macrophage).
The iron metabolism process and its regulation are highly complex; up till now, there remain a lot of missing links. Ferritin, a main cargo of blood iron, and transferrin receptors, involved in the cellular membrane transportation of iron, have been identified in vesicular locations (8–10). Also, bone marrow-derived extracellular vesicles (EVs) in β thalassemia patients were reported to affect hepcidin production (11). However, no paper has yet discussed the possible role of EVs in iron homeostasis. Considering the blooming significance of extracellular EVs in cell–cell communication, delivering cargo, and modulating the physiological condition (12); we suggest that EVs might play a role in transporting iron regulators thus maintaining iron homeostasis.
Here we will discuss the critical process of iron metabolism and the systems of its regulation, while focusing on the potential interference of extracellular vesicles. We will also discuss the role of gut microbiota and its metabolites in iron homeostasis, and consider the potential involvement of microbiota-derived extracellular vesicles in this relationship.
Iron metabolism
Iron metabolism consists of iron absorption, use, storage, and transfer. Absorbable dietary iron can be in the form of heme and non-heme iron (13). Heme iron contributes to 10–15% of the absorbed dietary iron. It is more absorbable by the body (15–35%) as compared to non-heme iron (2–20%) (14). Even though heme iron absorption surpasses that of non-heme iron, its absorption mechanisms remain ambiguous. Non-heme iron is absorbed at the level of the duodenum and proximal jejunum, by enterocytes divalent metal-ion transporter 1 (DMT1), after being reduced to ferrous iron (Fe2+) via duodenal cytochrome b (Dcytb) (15, 16). If not needed inside the cell, ferrous iron, is either stored in the form of ferritin or transferred to circulating transferrin (TF) via the iron exporter ferroportin (FPN), after being oxidized to ferric iron (Fe3+) by hephaestin (17). Transferrin bound iron is delivered to sites of utilization, where it binds to cell surface transferrin receptor 1 (TFR1) and endocytose into the cell (18); it then enters the cytoplasm via DMT1 in the endosomal membrane, after being reduced to (Fe2+) under the action of six-transmembrane epithelial antigen of prostate 3 (STEAP3) (19). This iron can be used for metabolic functions, or stored within cytosolic ferritin- iron in the ferric form associated with hydroxide and phosphate anion (20).
Merely 1–2 mg of dietary iron are absorbed in the gut daily; most iron is recycled upon phagocytosis of erythrocytes by macrophages (21). Senescent/damaged erythrocytes are phagocytosed by macrophages. Macrophages recover iron from heme via heme oxygenase 1 (HMOX1) for utilization, conservation or recycling depending on the body needs (22).
Iron homeostasis
The iron metabolism process is highly critical, considering the danger of excess free reactive iron. It is supposed to be tightly regulated. Iron homeostasis is essential to maintain normal physiology. To achieve homeostasis, iron regulation takes place at both cellular and systemic levels. At the cellular level, iron homeostasis involves mechanisms that balance iron uptake with intracellular iron storage and utilization (23). Systemic iron homeostasis embodies the mechanisms that synchronize dietary iron absorption and iron concentration in plasma and the extracellular milieu (24).
Iron homeostasis at the cellular level
At the cellular level, iron metabolism regulation is based on transcriptional and post-transcriptional regulation of iron genes. Post transcriptional regulation is facilitated by the binding of iron regulatory proteins (IRPs) to iron-responsive elements (IREs) of their mRNA untranslated regions (25). In case of iron-deficiency, IRP1 and IRP2 bind to the IREs in TFR1 mRNA and stabilize it, thus increasing iron uptake. They also bind to the 5′-UTR of the mRNAs that encode ferroportin and ferritin to suppress their translation, thus blocking iron export and storage (26). Conversely in iron-replete cells, iron can bind IRPs and induce their conformational change. Notably, IRP1 assembles an aconitase-type 4Fe-4s and this assembly alters its conformation (27, 28). The latter conformational changes weaken the IRPs IRE-binding ability, thus leading to (a) destabilization and degradation of TFR1 mRNA and (b) facilitated translation of the target 5′-UTR mRNA encoding ferroportin and ferritin (29). Micro RNAs (miRNAs) also count as significant posttranscriptional regulators of gene expression. They can regulate cellular iron homeostasis by influencing iron absorption, transport, storage, and utilization. For example, miR-Let-7d was reported to target the DMT1-non-IRE isoform (30) and miR-320 was found to post-transcriptionally control TfR1 expression (31). The mRNA encoding FPN in turn was shown to be targeted by miR-485-3p (32). Furthermore, the storage of iron as ferritin is downregulated by miR-200b (33).
Transcriptional regulation is dependent on hypoxia inducible factors (HIFs). Hypoxia inducible factor -2α (HIF-2α) plays an important physiological role in transcriptional regulation of iron homeostasis. HIF-2α regulates iron absorption notably by activating the expression of DcytB and DMT1 proteins during iron deficiency or ineffective erythropoiesis to increase iron uptake (34, 35). In fact, HIF-2α mRNA 5′-UTR contains an IRE that binds both IRP1 and IRP2 (36, 37) for iron-dependent regulation of the transcript. By regulating HIF-2α mRNA, IRP1 amends the erythropoietic response to hypoxia. This IRP1-HIF-2α axis synchronizes both iron and oxygen sensing with erythropoiesis and iron absorption (38). Furthermore, HIF regulates hepcidin, the orchestrator of systemic iron homeostasis, via erythropoietin-induced erythropoiesis. HIF can suppress the hepcidin gene Hamp1 indirectly through erythropoietin-induced erythropoiesis (39, 40).
Iron homeostasis at the systemic level
Systemic iron homeostasis is regulated via hepcidin, a hormone that is primarily secreted by hepatocytes. Iron and inflammatory cytokines induce hepcidin expression, while iron deficiency, erythropoiesis, and anemia/hypoxia downregulate it (17). High circulating iron levels upregulate hepcidin expression by hepatocytes, through the BMP/SMAD pathway (41). The binding of transferrin to transferrin receptor 2 (TFR2), to which it has less affinity than TFR 1, in case of high plasma iron concentration, has been anticipated to affect hepcidin expression. The binding of diferric transferrin to TFR2 induces overexpression of hepcidin in hepatocytes and reduced erythropoietin responsiveness in erythroid cells (42), where TFR2 binds erythropoietin receptors (43). Inflammatory cytokines upregulate hepcidin gene expression through the Janus kinase/signal transducers and activators of transcription (JAK/STAT); for example, IL-6, increases hepcidin expression via activating the IL-6R-JAK2-STAT3 pathway (44). When activated, hepcidin combines FPN1, internalizes it, and degrades it in the lysosome (45), thereby reducing iron absorption by duodenal cells and iron recycling by macrophages.
Extracellular vesicles: Key players in iron homeostasis?
Extracellular vesicles are membrane-bound vesicles secreted by cells into the extracellular space. They constitute microvesicles, exosomes, and apoptotic bodies- released by dying cells; they vary in size with microvesicles being the smallest (less than 100 nm–1 μm), followed by exosomes and apoptotic bodies, respectively (46). They function by facilitating the intercellular exchange of proteins, lipids, and genetic material, thus facilitating intercellular signaling/communication (47). While it is known that iron metabolism requires a lot of cell communication, the exact role of EVs in either iron metabolism or the maintenance of iron homeostasis remains to be elucidated. The putative role of EVs in iron homeostasis is summarized in Figure 1.
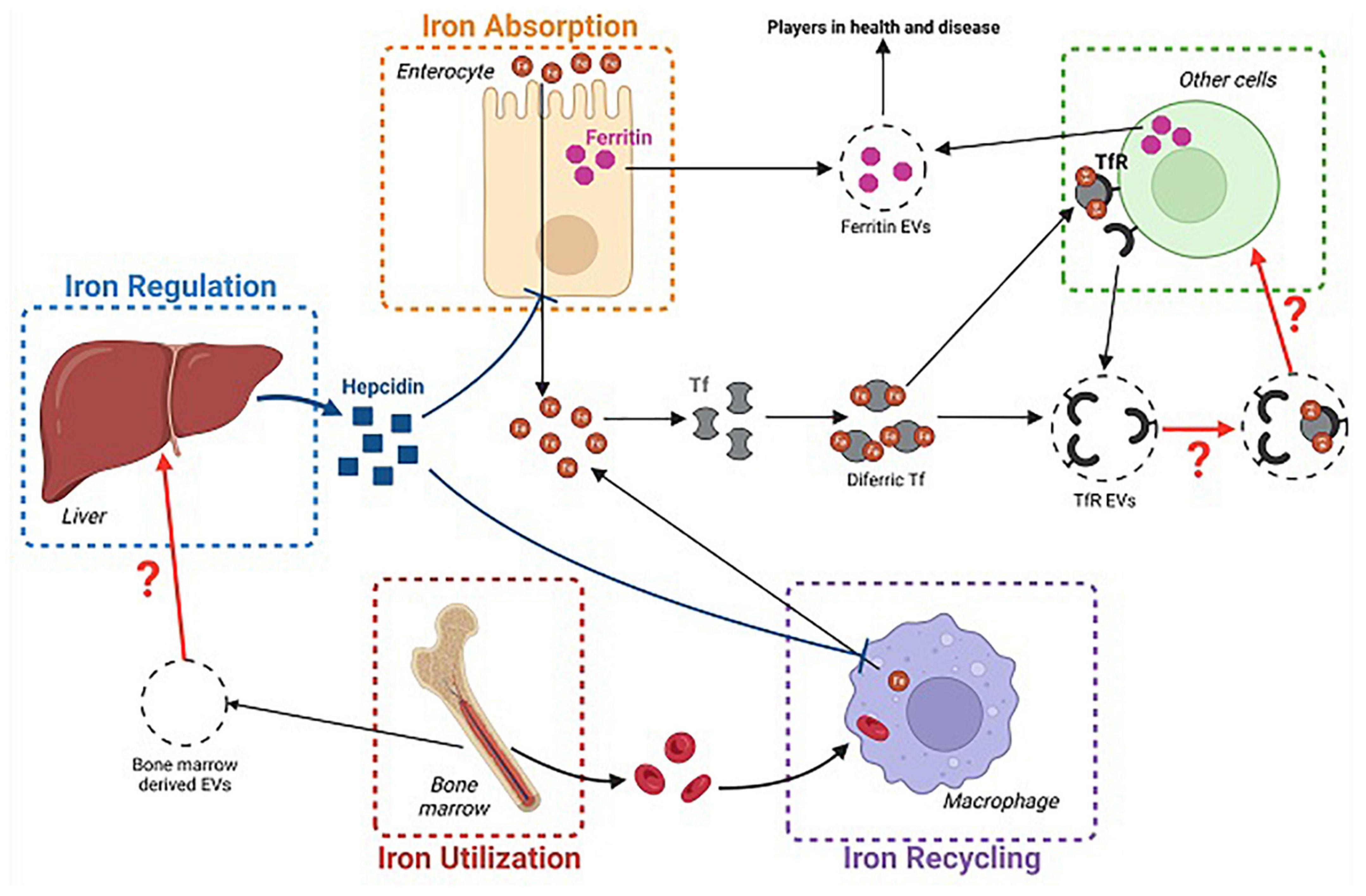
Figure 1. Schematic representation of the potential implication of extracellular vesicles (EVs) in iron homeostasis. This figure illustrates the possible involvement of (1) Transferrin carrying EVs (TFR EVs) in iron delivery to host cells, (2) Ferritin EVs in mediating disease symptoms, and (3) Bone marrow-derived EVs in affecting hepcidin release. ©Created with BioRender.com.
Ferritin extracellular vesicles and their fate
Iron is stored in the cells in the form of ferritin, as mentioned before. The synthesis and degradation of ferritin are both orchestrated by cellular iron status. Under low iron levels, ferritin synthesis decreases via translational repression (48), and ferritin lysosomal degradation, mediated by the selective autophagy receptor NCOA4, increases. The degradation of the ferritin to free the iron stored is a selective macro-autophagy mechanism called ferritinophagy and is mediated by NCOA4 (49). On the other hand, high iron levels decrease the entry of ferritin into the lysosome (50). Ferritin can be found in the cytoplasm (51), nucleus (52), and mitochondria (53). However, it has also been observed in vesicular locations.
Some researches succeeded to report the presence of ferritin in human urinary exosomes, via a large-scale proteomic analysis (9). However, there is a gap in our knowledge underlying ferritin intracellular trafficking and secretion. It was thought to be secreted through the Endoplasmic Reticulum–Golgi route (54), but Cohen et al. showed that ferritin is secreted primarily by macrophages through lysosomal pathway, by secretory lysosomes (55). Truman-Rosentsvit et al. provided evidence on the secretion of ferritin via the multivesicular body–exosome pathway (56). Yanatori et al. studied the mechanism of secretion of ferritin in extracellular vesicles (57). They discovered that CD63, which plays a role in EVs secretion (58), is post-transcriptionally regulated by iron via the IRE-IRP system, the system that induces ferritin under iron upsurge (59). Thus, increased levels of iron induce CD63 expression. Upon loading iron, intracellular ferritin is transported via nuclear receptor coactivator 4 (NCOA4)/ferritin vesicles to CD63+ EVs that are secreted (57).
Exosomes play a role in iron homeostasis after all, but the destination of ferritin carried by the exosomes remains to be explored. Is it transferred to hepatocytes, the major sites for iron storage? Can those exosomes fuse with the free mitochondria and deliver ferritin to them? Can the ferritin stored in mitochondria be released via mitochondria-derived vesicles (MDVs) too? Vasam et al. had reported that MDVs revealed high levels of mitochondrial iron–sulfur clusters biogenesis proteins, that are responsible for the biogenesis of iron-containing cofactors, and iron-binding capable proteins. They suggested that MDVs can serve as a potential source of biomarkers for mitochondrial stress (60).
The serum ferritin level mirrors the body’s iron stock; it is considered a hematologic index for iron-associated diseases (61). Whether this serum ferritin is contained in EVs or possibly released from ferritin carrying EVs is ambiguous. Excessively elevated levels of both ferritin (62, 63) and EVs (64) have long been noted in the circulation of iron overload β-thalassemia patients. Recently, Atipimonpat et al. reported the presence of high levels of ferritin-bearing exosomes in the plasma of β-thalassemia patients (65). They explained that those high levels of circulating red blood cells and activated platelets derived EVs, especially ferritin-carrying exosomes, can speed up the proliferation of H9C2 cardiac cells leading to cell hyperplasia, progression of cardiac hypertrophy, and eventually heart failure. What remains to be clarified is whether iron overload can induce or increase the ferritin-loaded exosomes and if ferritin-loaded exosomes can contribute to disease symptoms.
Strzyz in turn reported ferritin exosomes to induce ferroptosis resistance (66). In this regard, exosomes provide a route for ejecting iron out of ferroptotic cells, thus protecting them from ferroptosis. Accordingly, Mukherjee et al. reported that disrupting the EV release or ferritin heavy chain expression in oligodendrocytes resulted in neuronal loss and oxidative damage in mice (67). In case of cancer, carcinoma cells may use this iron export pathway involving multivesicular body/exosome trafficking of iron out of the cell to avoid ferroptotic death (68). Interestingly, a study conducted by Ito et al. showed that macrophages that engulf asbestos produce ferroptosis-dependent extracellular vesicles that contain ferritin and transport it to mesothelial cells, thereby contributing to mesothelial carcinogenesis by loading ferritin (69). Alterations in EV levels during therapy have been reported in Glioblastoma patients; interestingly the EV protein signature showed common iron metabolism proteins and disappeared post-surgical resection (70). We can speculate that ferritin extracellular vesicles can halt ferroptosis in health and in disease. They show potential in becoming biomarkers for disease diagnosis, notably cancer.
Transferrin receptor carrying extracellular vesicles
Transferrin receptor expression was also detected in exosomes. The presence of Glyceraldehyde-3-phosphate dehydrogenase (GAPDH), which has been characterized as a transferrin receptor (71, 72), in exosomes from different cell lines was confirmed by Malhotra et al. along with its ability to bind transferrin (10). They also reported that iron preloaded exosomes delivered more iron into various cells, thus raising an interesting chance of exosomes playing a role in the delivery of iron and iron homeostasis. Interestingly a recent study conducted by Dar et al. demonstrated that GAPDH also induces clustering of EVs in vitro and in vivo (73). Further, Mattera et al. identified the expression of TFR1 in extracellular vesicles derived from human and mouse plasma, rat oligodendroglioma cells, mouse neuroblastoma cells, and rat astrocytes (8). However, no research has been conducted on the presence of TFR2, that induces hepcidin expression upon binding diferric transferrin, in hepatocytes and erythroblasts derived EVs.
Extracellular vesicle-mediated hepcidin modulation
Hepcidin, the key iron regulator, is produced primarily by hepatocytes neighboring the portal veins and Kupffer cells (7), macrophages (74), adipocytes (75), and dendritic cells (76). Also, several studies have reported local synthesis of hepcidin by multiple other tissues, notably in disease, like the and the lungs (77), kidney (78), stomach (79), adipose tissue (75), brain (80), heart (81), and even the skin (82). The organ that produces the most hepcidin after the liver is the heart (83). As mentioned before, under active erythropoiesis, hepcidin production is somewhat inhibited via erythroferrone, an erythroid factor produced by the erythroblasts, that suppresses the BMP/SMAD pathway in the liver (84). β-Thalassemia patients are known to have ineffective erythropoiesis and iron overload. This ineffective erythropoiesis suppresses hepcidin leading to iron overload (85). Ruiz Martinez et al. hypothesized that bone-marrow derived exosomes modulate hepcidin expression and regulate iron metabolism (11); they investigated the link between exosomes and hepcidin regulation in β-thalassemia. They were able to demonstrate that those exosomes boost hepcidin expression by increasing SMAD1/5/8 signaling. Increased hepcidin, in response to exosomes, will possibly influence several signaling pathways by an autocrine mechanism. Exosomes compensated for suppressed hepcidin in the exosome-depleted serum of β-thalassemic samples. Proteomic analysis of β-thalassemic patients’ bone marrow derived exosomes can help us better understand their role in hepcidin regulation. On the other hand, EVs derived from the plasma of β-thalassemic patients showed dysregulation of certain miRNAs involved in oxidative stress, erythropoiesis, and apoptosis- in particular overexpressed miR-144-3p. They were reported to induce apoptosis in endothelial, pancreatic, and hepatic cells, possibly contributing to the organ damage in β-thalassemia (86). Thus, studying EVs derived from β-thalassemic patients not only can help to understand the implication of EVs in iron metabolism regulation, but they can also help to comprehend the health complication associated with β-thalassemia. They can also serve as biomarkers for β-thalassemia severity.
Gut microbiota: Modulation of iron homeostasis
Almost all living organisms require iron in order to survive. A deficiency or excess in iron is dangerous hence iron homeostasis is firmly regulated. Iron acquisition takes place at the level of the small intestine (87). The small intestine is colonized by symbiotic microorganisms, called the “gut microbiota,” that share a mutually beneficial relationship with the host (88, 89). The gut microbiota relies on the host for nutrients and survival, while it plays an indirect role in regulating complex endocrine networks. Gut microbiota compete with the host to acquire iron, for survival. Studies have reported how iron deficiency/repletion in rats (90) or genetic modification of iron metabolism in mice (91) affect the gut microbiota composition and metabolic activity. The fecal microbiota has even been proposed as a non-invasive biomarker for tissue iron accumulation prediction in intestine epithelial cells and liver (92).
The gut microbiota must in turn also impact the iron absorption process and play an indirect role in regulating iron homeostasis. Most studies, however, focus on the strategies used by microbiota to acquire iron, studies on the possible role of microbiota in regulating iron homeostasis are scarce. Deschemin et al. were the first to investigate the impact of microbiota on host iron sensing (93). They demonstrated that gut microbes induce a specific iron-related protein signature and revealed a new feature of the microbiota – intestinal epithelium crosstalk. Further, Das et al., in their turn, then studied the impact of gut microbiota on iron intestinal absorption, and discussed its unforeseen role in regulating host iron homeostasis (94). They identified that the host iron-sensing mechanism is connected to the gut microbiome and regulated by it. They reported that, intestinal iron deficiency leads to the positive selection of Lactobacillus species that produce reuterin and 1,3 diaminopropane (DAP). Those microbiota metabolites (a) suppress iron absorption, by inhibiting the transcription factor HIF-2α that targets the expression of key iron transporters (34, 35, 95), and (b) induce ferritin expression to eventually lead to its degradation by the host in due to the iron overload caused - in order to maintain homeostasis (34); thereby indirectly preventing tissue iron accumulation (94).
From another perspective, Zhang et al. also reported that microbiota derived short chain fatty acids (SCFAs), specifically butyrate, can lead to iron distribution, for fueling hematopoietic regeneration, by promoting emergency erythro-phagocytosis by bone marrow macrophages (96). However, it is important to note that microbiota depletion resulted in only reduced local iron levels without affecting systemic iron homeostasis (96). Yet, future studies should further explore a possible involvement of a microbiota-macrophage-iron axis in iron homeostasis. All the current knowledge on the relationship between gut microbiota and host iron levels is summarized in Figure 2.
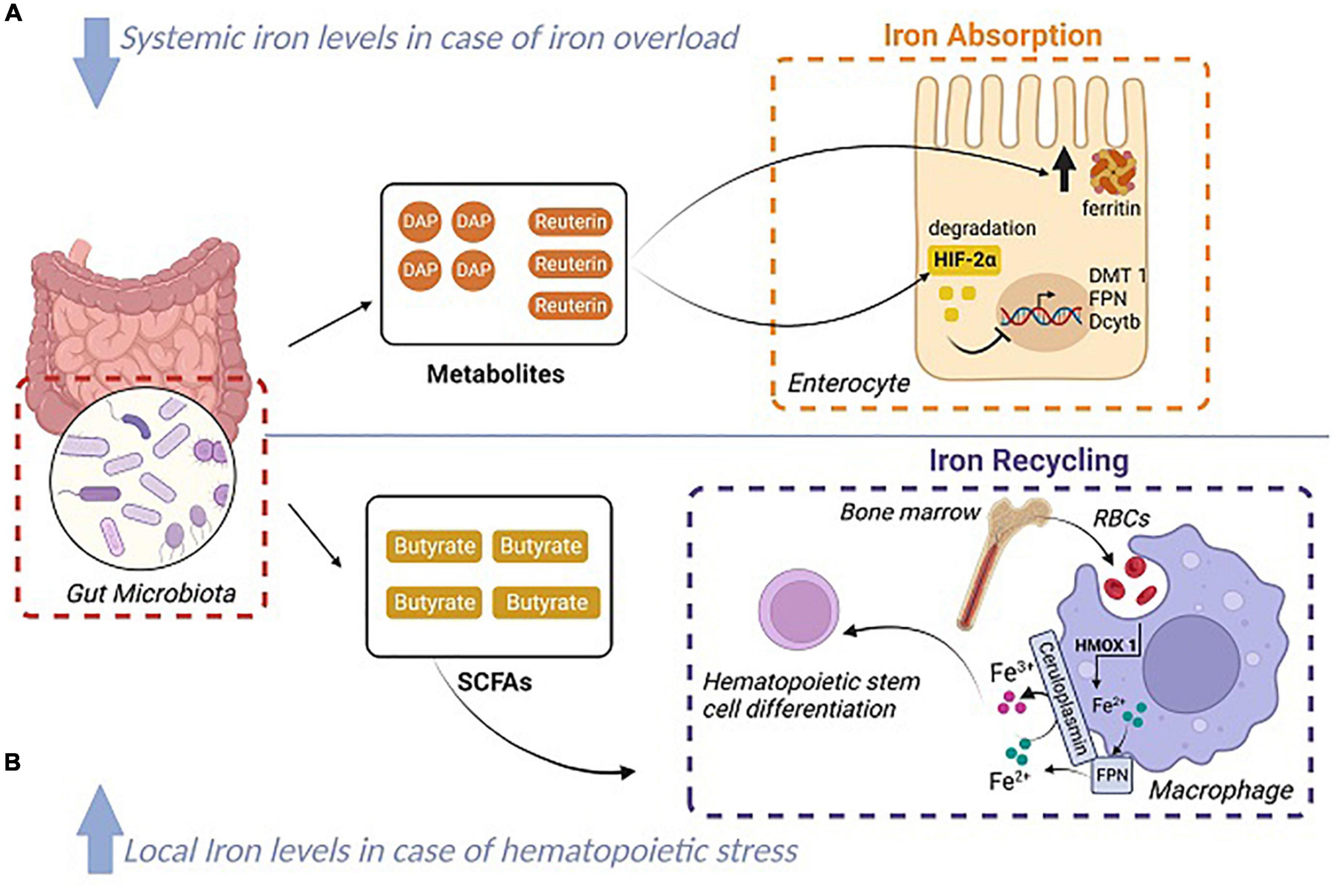
Figure 2. Summary of the achieved relationship between the gut microbiota and systemic/local iron levels. (A) The gut microbiota releases 1,3 diaminopropane (DAP) and reuterin in case of iron overload and decreases systemic iron levels. DAP and reuterin induce ferritin expression, and cause the degradation of HIF-2α consequently blocking the expression of the iron transporters (Dcytb, DMT1, and FPN). (B) Hematopoietic stress leads to the release of butyrate by the gut microbiota. Butyrate decreases local iron levels by promoting iron recycling (erythro-phagocytosis) in order to allow hematopoietic stem cells differentiation. ©Created with BioRender.com.
In reality, we are still in the infancy of understanding the metabolic crosstalk between gut microbiota and the host intestinal epithelium- in terms of iron homeostasis. A lot of studies are required to identify the role of microbiota-derived metabolites in context of iron homeostasis, and to determine whether microbiota commensals can secrete hormones that directly regulate or impact iron metabolism. Additionally, it is important to study the potential of targeting gut microbiota therapeutically via prebiotics, probiotics, or fecal microbiota transplant for iron-related diseases.
Gut microbiota and hepcidin production: An intriguing question
As explained before, hepcidin is the central iron regulator, it is overexpressed in response to iron overload and inflammation (97); it blocks iron export (98) and degrades the iron exporter ferroportin (45), thereby reducing iron absorption by duodenal cells and iron recycling macrophages. The microbiota produces a wide range of metabolite-derived humoral agents including SCFA, secondary bile acids, and neurotransmitters all of which play important body functions (99). As mentioned above, Lactobacillus derived metabolites were lately reported to play an indirect role in regulating iron homeostasis.
The secretion of hepcidin by myeloid cells occurs via the toll-like receptor 4-hepcidin pathway as a host response to bacterial pathogens (100). Layoun and Santos showed that bacterial cell wall lipopolysaccharide induced hepcidin expression in macrophages (101). Microbiota dysbiosis in the inflamed intestine of humans was found to induce the release of hepcidin by conventional dendritic cells, for tissue repair (76). However, one intriguing question that remains to be answered is whether the microbiota can modulate iron homeostasis, directly, by producing hepcidin.
Gut microbiota derived extracellular vesicles: Players in iron homeostasis?
Iron is essential for the majority of microorganisms. Some bacteria have evolved efficient strategies to acquire iron from the host. The microbiota acquires iron from the host by using host iron compounds (e.g., heme, transferrin, lactoferrin ferritin), producing high-affinity iron chelators called siderophores, and/or uptaking ferrous iron (102). Gram-negative bacteria derived outer membrane vesicles (103) and gram-positive bacteria derived extracellular vesicles (104) are well reported to play significant roles in bacterial survival, material exchange, cell-to-cell communication, and pathophysiology. EVs of some pathogenic bacteria are known to help them acquire iron from the host (105). Additionally, bacteria-derived EVs (Diaetzia sp.) were found to allow homologous bacterial species to share iron (106).
Microbiota-derived EVs are involved in inter-kingdom communication with host cells in the gut (107, 108). They were reported to deliver to host cells effector molecules that modulate host signaling pathways and cell processes (108). The probable effects of gut microbiota–derived EVs on metabolic diseases such as obesity and diabetes have been reviewed (109). Knowing that the gut microbiota plays an indirect role in regulating the host’s iron homeostasis, no research has yet addressed the potential role of microbiota-derived EVs in the regulation of iron homeostasis.
Bacteria can store iron in the form of bacterial ferritin, heme-containing bacterioferritin, and DNA binding dodecameric ferritin (110). The existence of bacterial ferritin, the prototype of ferritin that possesses a classical ferritin H-chain with ferroxidase activity (111), in microbiota-derived EVs might suggest their interference in host iron homeostasis. In fact, Zakharzhevskaya et al. were able to identify non-heme ferritin with special oxidoreductase activity in toxigenic Bacteroides fragilis (112). Further research is required to test for the presence of bacterial ferritin in microbiota-derived EVs and unravel the possibility of the association of bacterial ferritin-carrying vesicles with host iron homeostasis.
The presence of iron modulating microbial metabolites in microbiota-derived EVs should also be addressed. The potential implication of metabolite-carrying microbiota-derived EVs in the microbiota host iron regulatory mechanisms is depicted in Figure 3. As reported above, the gut microbiota plays a role in regulating the host’s iron homeostasis via Lactobacillus derived metabolites reuterin and DAP (94). Moreover, proteomic analysis of L. reuteri derived EVs revealed the presence of different functional and structural proteins signifying the possible involvement of L. reuteri EVs in metabolism, transport, and signaling (113). Further studies ought to specifically test for the presence of the bacteriocins reuterin and DAP in L. reuteri-derived EVs, taking into consideration that species like Lactobacillus acidophilus can carry bacteriocin peptides via EVs in order to deliver them to opportunistic pathogens (114).
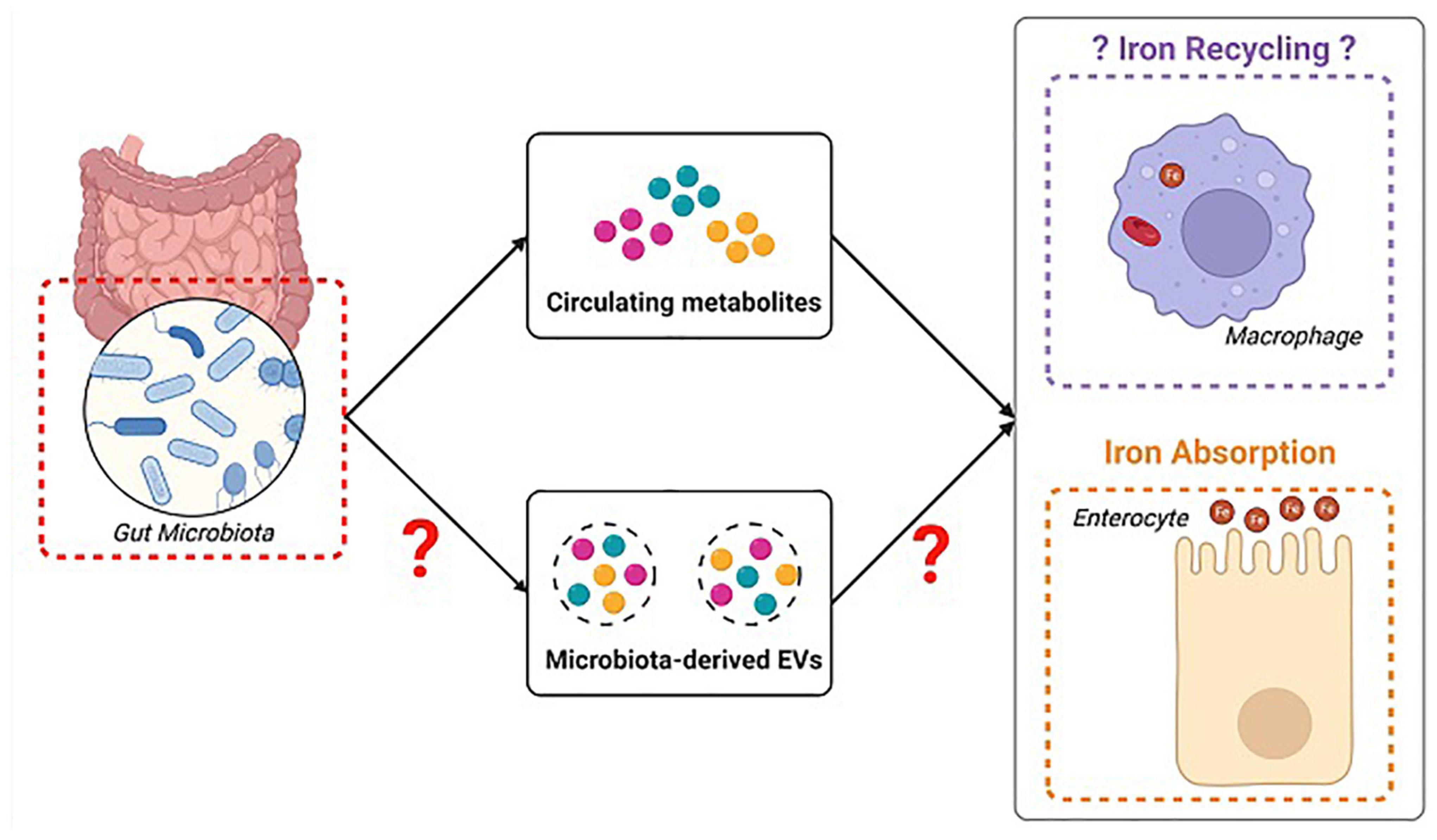
Figure 3. Schematic representation of the putative role of metabolite carrying microbiota-derived extracellular vesicles (EVs) in modulating iron homeostasis. Gut microbiota metabolites mainly interfere in iron homeostasis by acting on iron absorption at the level of the gut. They may possibly interfere with heme recycling at the level of the macrophages. We propose that those metabolites can be released in EVs. ©Created with BioRender.com.
The presence of SCFAs specifically butyrate in EVs should also be tested to assess the prospective involvement of bacteria-derived EVs in modulating the iron recycling process. Streptococcus pneumoniae derived EVs for example were enriched in short chain saturated fatty acids (115).
Thus, the characterization of bioactive molecules and different types of cargo of gut microbiota– and probiotic-derived EVs is required. Once this is achieved, we can begin to explore the potential role of microbiota- derived EVs in regulating the host iron homeostasis. However, it is important to note that there are current limitations to characterize those EVs and their cargo due to the similarity between bacterial and mammalian EVs (107).
Conclusion
Maintaining iron homeostasis is a challenge reflected by the complexity of iron metabolism. EVs seem to be indispensable in mediating this process. They appear to be key players in iron homeostasis, ferritin is released via exosomes under high iron levels, transferrin receptors are also expressed in exosomes. The question of the destination of ferritin carrying EVs has yet to be answered. Is ferritin transferred via EVs to be stored in hepatocytes? Do they serve in delivering ferritin to free mitochondria? Do mitochondria export iron via MDVs? On another note, EVs should be tested for any ability to deliver iron to distal sites especially to the brain knowing that they can cross the blood brain barrier (116).
Extracellular vesicles are involved in iron regulation in health and disease. Bone marrow-derived EVs can regulate iron homeostasis by compensating for low hepcidin in β-thalassemic patients. However, plasma-derived EVs were also reported to contribute to organ dysfunction and complications of β-thalassemia. A better understanding of EVs composition and cargo might help identify new mechanisms underlying iron-overload diseases such as β-thalassemia or even treat them. Ferritin-containing EVs were reported to suppress ferroptosis in health, but also promote cell survival and proliferation in cancer. This opens doors for better diagnostics and therapeutic options in the future; what if cancer can be diagnosed by identifying ferritin-containing EVs? What if it can be treated by targeting those EVs and modulating iron levels?
Achieving iron homeostasis is also linked to the gut microbiota, the endocrine organ that keeps surprising us with its importance day by day. Gut microbiota metabolites can participate in achieving iron homeostasis by acting on intestinal iron absorption, and can impact local iron levels by affecting bone marrow macrophage erythro-phagocytosis. Knowing that the gut microbiota secretes a wide range of metabolite-derived humoral agents, can it regulate iron metabolism by directly releasing hepcidin? We are still in the infancy of understanding the many roles of gut microbiota in achieving/maintaining iron homeostasis. Later studies should address the effect of gut dysbiosis on iron homeostasis. They should also investigate where microbiota-derived EVs stand in this gut microbiota - iron homeostasis relationship. The future of medicine lies in the microbiota (117). Microbiota derived EVs might be key in future therapies for iron related diseases. Targeting host and gut microbiota-derived EVs will be a huge challenge to prevent and treat many diseases related to the alteration of iron homeostasis and metabolism.
Author contributions
ME: conception. ME, MF, CPo, CPe, and YD: writing, interpretation, and critical revision of the manuscript. All authors have read and agreed to the published version of the manuscript.
Acknowledgments
We thank the International Society of Microbiota (ISM) for their constant support.
Conflict of interest
The authors declare that the research was conducted in the absence of any commercial or financial relationships that could be construed as a potential conflict of interest.
Publisher’s note
All claims expressed in this article are solely those of the authors and do not necessarily represent those of their affiliated organizations, or those of the publisher, the editors and the reviewers. Any product that may be evaluated in this article, or claim that may be made by its manufacturer, is not guaranteed or endorsed by the publisher.
Abbreviations
EVs, extracellular vesicles; ROS, reactive oxygen species; DMT1, divalent metal-ion transporter 1; Dcytb, duodenal cytochrome b; TF, transferrin; FPN, ferroportin; STEAP3, six-transmembrane epithelial antigen of prostate 3; HMOX1, heme oxygenase 1; IRPs, iron regulatory proteins; IREs, iron-responsive elements; HIFs, hypoxia inducible factors; TFR 1, transferrin receptor 1; TFR 2, transferrin receptor 2; JAK/STAT, Janus kinase/signal transducers and activators of transcription; MDVs, mitochondria derived vesicles; GAPDH, glyceraldehyde-3-phosphate dehydrogenase; DAP, 1,3 diaminopropane; SCFA, short chain fatty acids.
References
1. Singh AK. Chapter 12 – Erythropoiesis: the roles of erythropoietin and iron. 2nd ed. In: Singh AK, Williams GH, editors. Textbook of Nephro-Endocrinology. Cambridge, MA: Academic Press (2018). p. 207–15.
2. Ward DM, Cloonan SM. Mitochondrial iron in human health and disease. Annu Rev Physiol. (2019) 81:453–82. doi: 10.1146/annurev-physiol-020518-114742
3. Puig S, Ramos-Alonso L, Romero AM, Martínez-Pastor MT. The elemental role of iron in DNA synthesis and repair. Metallomics. (2017) 9:1483–500. doi: 10.1039/c7mt00116a
4. Ward RJ, Crichton RR, Taylor DL, Corte LD, Srai SK, Dexter DT. Iron and the immune system. J Neural Transmiss. (2011) 118:315–28. doi: 10.1007/s00702-010-0479-3
5. Torti SV, Torti FM. Iron and cancer: more ore to be mined. Nat Rev Cancer. (2013) 13:342–55. doi: 10.1038/nrc3495
6. Chen X, Yu C, Kang R, Tang D. Iron metabolism in ferroptosis. Front Cell Dev Biol. (2020) 8:590226. doi: 10.3389/fcell.2020.590226
7. Ganz T, Nemeth E. Hepcidin and iron homeostasis. Biochim Biophys Acta. (2012) 1823:1434–43. doi: 10.1016/j.bbamcr.2012.01.014
8. Mattera VS, Pereyra Gerber P, Glisoni R, Ostrowski M, Verstraeten SV, Pasquini JM, et al. Extracellular vesicles containing the transferrin receptor as nanocarriers of apotransferrin. J Neurochem. (2020) 155:327–38. doi: 10.1111/jnc.15019
9. Principe S, Jones EE, Kim Y, Sinha A, Nyalwidhe JO, Brooks J, et al. In-depth proteomic analyses of exosomes isolated from expressed prostatic secretions in urine. Proteomics. (2013) 13:1667–71. doi: 10.1002/pmic.201200561
10. Malhotra H, Sheokand N, Kumar S, Chauhan AS, Kumar M, Jakhar P, et al. Exosomes: tunable nano vehicles for macromolecular delivery of transferrin and lactoferrin to specific intracellular compartment. J Biomed Nanotechnol. (2016) 12:1101–14. doi: 10.1166/jbn.2016.2229
11. Ruiz Martinez M, Castro-Mollo M, Dogra N, An W, Borroni E, Follenzi A, et al. Role of exosomes in hepcidin regulation in β-thalassemia. Blood. (2019) 134(Suppl. 1):954. doi: 10.1182/blood-2019-131094
12. Yoon YJ, Kim OY, Gho YS. Extracellular vesicles as emerging intercellular communicasomes. BMB Rep. (2014) 47:531–9. doi: 10.5483/bmbrep.2014.47.10.164
13. Ems T, St Lucia K, Huecker MR. Biochemistry, iron absorption. In: StatPearls [Internet]. Treasure Island, FL: StatPearls Publishing (2022).
14. Beck KL. Anemia: prevention and dietary strategies. In: Caballero B, Finglas PM, Toldrá F, editors. Encyclopedia of Food and Health. Oxford: Academic Press (2016). p. 164–8.
15. Gunshin H, Fujiwara Y, Custodio AO, Direnzo C, Robine S, Andrews NC. Slc11a2 is required for intestinal iron absorption and erythropoiesis but dispensable in placenta and liver. J Clin Invest. (2005) 115:1258–66. doi: 10.1172/jci24356
16. Choi J, Masaratana P, Latunde-Dada GO, Arno M, Simpson RJ, McKie AT. Duodenal reductase activity and spleen iron stores are reduced and erythropoiesis is abnormal in DCYTB knockout mice exposed to hypoxic conditions. J Nutr. (2012) 142:1929–34. doi: 10.3945/jn.112.160358
17. Hentze MW, Muckenthaler MU, Galy B, Camaschella C. Two to tango: regulation of mammalian iron metabolism. Cell. (2010) 142:24–38. doi: 10.1016/j.cell.2010.06.028
18. Kawabata H, Germain RS, Vuong PT, Nakamaki T, Said JW, Koeffler HP. Transferrin receptor 2-alpha supports cell growth both in iron-chelated cultured cells and in vivo. J Biol Chem. (2000) 275:16618–25. doi: 10.1074/jbc.M908846199
19. Ohgami RS, Campagna DR, Greer EL, Antiochos B, McDonald A, Chen J, et al. Identification of a ferrireductase required for efficient transferrin-dependent iron uptake in erythroid cells. Nat Genet. (2005) 37:1264–9. doi: 10.1038/ng1658
20. Torti FM, Torti SV. Regulation of ferritin genes and protein. Blood. (2002) 99:3505–16. doi: 10.1182/blood.v99.10.3505
21. Clara C, Antonella N, Laura S. Iron metabolism and iron disorders revisited in the hepcidin era. Haematologica. (2020) 105:260–72. doi: 10.3324/haematol.2019.232124
22. Korolnek T, Hamza I. Macrophages and iron trafficking at the birth and death of red cells. Blood. (2015) 125:2893–7. doi: 10.1182/blood-2014-12-567776
23. Ward RJ, Crichton RR. Chapter 15 – Iron homeostasis and aluminium toxicity. In: Exley C, editor. Aluminium and Alzheimer’s Disease. Amsterdam: Elsevier (2001). p. 293–310.
24. Ganz T. Systemic iron homeostasis. Physiol Rev. (2013) 93:1721–41. doi: 10.1152/physrev.00008.2013
25. Anderson CP, Shen M, Eisenstein RS, Leibold EA. Mammalian iron metabolism and its control by iron regulatory proteins. Biochim Biophys Acta. (2012) 1823:1468–83. doi: 10.1016/j.bbamcr.2012.05.010
26. Wilkinson N, Pantopoulos K. The IRP/IRE system in vivo: insights from mouse models. Front Pharmacol. (2014) 5:176. doi: 10.3389/fphar.2014.00176
27. Dupuy J, Volbeda A, Carpentier P, Darnault C, Moulis J-M, Fontecilla-Camps JC. Crystal structure of human iron regulatory protein 1 as cytosolic aconitase. Structure. (2006) 14:129–39. doi: 10.1016/j.str.2005.09.009
28. Walden WE, Selezneva AI, Dupuy J, Volbeda A, Fontecilla-Camps JC, Theil EC, et al. Structure of dual function iron regulatory protein 1 complexed with ferritin IRE-RNA. Science. (2006) 314:1903–8. doi: 10.1126/science.1133116
29. Pantopoulos K. Iron metabolism and the IRE/IRP regulatory system: an update. Ann N Y Acad Sci. (2004) 1012:1–13. doi: 10.1196/annals.1306.001
30. Andolfo I, De Falco L, Asci R, Russo R, Colucci S, Gorrese M, et al. Regulation of divalent metal transporter 1 (DMT1) non-IRE isoform by the microRNA let-7d in erythroid cells. Haematologica. (2010) 95:1244–52. doi: 10.3324/haematol.2009.020685
31. Schaar DG, Medina DJ, Moore DF, Strair RK, Ting Y. MIR-320 targets transferrin receptor 1 (Cd71) and inhibits cell proliferation. Exp Hematol. (2009) 37:245–55. doi: 10.1016/j.exphem.2008.10.002
32. Sangokoya C, Doss JF, Chi JT. Iron-responsive MIR-485-3p regulates cellular iron homeostasis by targeting ferroportin. PLoS Genet. (2013) 9:e1003408. doi: 10.1371/journal.pgen.1003408
33. Shpyleva SI, Tryndyak VP, Kovalchuk O, Starlard-Davenport A, Chekhun VF, Beland FA, et al. Role of ferritin alterations in human breast cancer cells. Breast Cancer Res Treat. (2011) 126:63–71. doi: 10.1007/s10549-010-0849-4
34. Shah YM, Matsubara T, Ito S, Yim S-H, Gonzalez FJ. Intestinal hypoxia-inducible transcription factors are essential for iron absorption following iron deficiency. Cell Metab. (2009) 9:152–64. doi: 10.1016/j.cmet.2008.12.012
35. Mastrogiannaki M, Matak P, Keith B, Simon MC, Vaulont S, Peyssonnaux C. HIF-2α, but not HIF-1α, promotes iron absorption in mice. J Clin Invest. (2009) 119:1159–66. doi: 10.1172/JCI38499
36. Sanchez M, Galy B, Muckenthaler MU, Hentze MW. Iron-regulatory proteins limit hypoxia-inducible factor-2alpha expression in iron deficiency. Nat Struct Mol Biol. (2007) 14:420–6. doi: 10.1038/nsmb1222
37. Zimmer M, Ebert BL, Neil C, Brenner K, Papaioannou I, Melas A, et al. Small-molecule inhibitors of HIF-2a translation link Its 5’UTR iron-responsive element to oxygen sensing. Mol Cell. (2008) 32:838–48. doi: 10.1016/j.molcel.2008.12.004
38. Anderson Sheila A, Nizzi Christopher P, Chang Y-I, Deck Kathryn M, Schmidt Paul J, Galy B, et al. The IRP1-HIF-2α axis coordinates iron and oxygen sensing with erythropoiesis and iron absorption. Cell Metab. (2013) 17:282–90. doi: 10.1016/j.cmet.2013.01.007
39. Maria M, Pavle M, Jacques RRM, Stéphanie D, Patrick M, Sophie V, et al. Hepatic hypoxia-inducible factor-2 down-regulates hepcidin expression in mice through an erythropoietin-mediated increase in erythropoiesis. Haematologica. (2012) 97:827–34. doi: 10.3324/haematol.2011.056119
40. Liu Q, Davidoff O, Niss K, Haase VH. Hypoxia-inducible factor regulates hepcidin via erythropoietin-induced erythropoiesis. J Clin Invest. (2012) 122:4635–44. doi: 10.1172/JCI63924
41. Parrow NL, Fleming RE. Bone morphogenetic proteins as regulators of iron metabolism. Annu Rev Nutr. (2014) 34:77–94. doi: 10.1146/annurev-nutr-071813-105646
42. Camaschella C, Pagani A, Nai A, Silvestri L. The mutual control of iron and erythropoiesis. Int J Lab Hematol. (2016) 38:20–6.
43. Forejtnikova H, Vieillevoye M, Zermati Y, Lambert M, Pellegrino RM, Guihard S, et al. Transferrin receptor 2 is a component of the erythropoietin receptor complex and is required for efficient erythropoiesis. Blood. (2010) 116:5357–67. doi: 10.1182/blood-2010-04-281360
44. Wrighting DM, Andrews NC. Interleukin-6 induces hepcidin expression through STAT3. Blood. (2006) 108:3204–9. doi: 10.1182/blood-2006-06-027631
45. Nemeth E, Tuttle MS, Powelson J, Vaughn MB, Donovan A, Ward DM, et al. Hepcidin regulates cellular iron efflux by binding to ferroportin and inducing its internalization. Science. (2004) 306:2090–3. doi: 10.1126/science.1104742
46. Doyle LM, Wang MZ. Overview of extracellular vesicles, their origin, composition, purpose, and methods for exosome isolation and analysis. Cells. (2019) 8:727. doi: 10.3390/cells8070727
47. Bang C, Thum T. Exosomes: new players in cell-cell communication. Int J Biochem Cell Biol. (2012) 44:2060–4. doi: 10.1016/j.biocel.2012.08.007
48. Rouault TA, Tang CK, Kaptain S, Burgess WH, Haile DJ, Samaniego F, et al. Cloning of the CDNA encoding an RNA regulatory protein–the human iron-responsive element-binding protein. Proc Natl Acad Sci U.S.A. (1990) 87:7958–62. doi: 10.1073/pnas.87.20.7958
49. Mancias JD, Wang X, Gygi SP, Harper JW, Kimmelman AC. Quantitative proteomics identifies NCOA4 as the cargo receptor mediating ferritinophagy. Nature. (2014) 509:105–9. doi: 10.1038/nature13148
50. Mancias JD, Pontano Vaites L, Nissim S, Biancur DE, Kim AJ, Wang X, et al. Ferritinophagy via NCOA4 is required for erythropoiesis and is regulated by iron dependent HERC2-mediated proteolysis. Elife. (2015) 4:e10308. doi: 10.7554/eLife.10308
51. Harrison PM, Arosio P. The ferritins: molecular properties, iron storage function and cellular regulation. Biochim Biophys Acta. (1996) 1275:161–203. doi: 10.1016/0005-272800022-9
52. Cai CX, Birk DE, Linsenmayer TF. Nuclear ferritin protects DNA from UV damage in corneal epithelial cells. Mol Biol Cell. (1998) 9:1037–51. doi: 10.1091/mbc.9.5.1037
53. Levi S, Corsi B, Bosisio M, Invernizzi R, Volz A, Sanford D, et al. A human mitochondrial ferritin encoded by an intronless gene. J Biol Chem. (2001) 276:24437–40.
54. Ghosh S, Hevi S, Chuck SL. Regulated secretion of glycosylated human ferritin from hepatocytes. Blood. (2004) 103:2369–76. doi: 10.1182/blood-2003-09-3050
55. Cohen LA, Gutierrez L, Weiss A, Leichtmann-Bardoogo Y, Zhang DL, Crooks DR, et al. Serum ferritin is derived primarily from macrophages through a nonclassical secretory pathway. Blood. (2010) 116:1574–84. doi: 10.1182/blood-2009-11-253815
56. Truman-Rosentsvit M, Berenbaum D, Spektor L, Cohen LA, Belizowsky-Moshe S, Lifshitz L, et al. Ferritin is secreted via 2 distinct nonclassical vesicular pathways. Blood. (2018) 131:342–52. doi: 10.1182/blood-2017-02-768580
57. Yanatori I, Richardson DR, Dhekne HS, Toyokuni S, Kishi F. Cd63 is regulated by iron via the IRE-IRP system and is important for ferritin secretion by extracellular vesicles. Blood. (2021) 138:1490–503. doi: 10.1182/blood.2021010995
58. Hurwitz SN, Conlon MM, Rider MA, Brownstein NC, Meckes DG Jr. Nanoparticle analysis sheds budding insights into genetic drivers of extracellular vesicle biogenesis. J Extracell Vesicles. (2016) 5:31295. doi: 10.3402/jev.v5.31295
59. Eisenstein RS, Blemings KP. Iron regulatory proteins, iron responsive elements and iron homeostasis. J Nutr. (1998) 128:2295–8. doi: 10.1093/jn/128.12.2295
60. Vasam G, Nadeau R, Cadete VJJ, Lavallée-Adam M, Menzies KJ, Burelle Y. Proteomics characterization of mitochondrial-derived vesicles under oxidative stress. FASEB J. (2021) 35:e21278. doi: 10.1096/fj.202002151R
61. Daru J, Colman K, Stanworth SJ, De La Salle B, Wood EM, Pasricha S-R. Serum ferritin as an indicator of iron status: what do we need to know? Am J Clin Nutr. (2017) 106(Suppl. 6):1634S–9S. doi: 10.3945/ajcn.117.155960
62. Thiengtavor C, Siriworadetkun S, Paiboonsukwong K, Fucharoen S, Pattanapanyasat K, Vadolas J, et al. Increased ferritin levels in non-transfusion-dependent β°-thalassaemia/HBE are associated with reduced CXCR2 expression and neutrophil migration. Br J Haematol. (2020) 189:187–98. doi: 10.1111/bjh.16295
63. Pootrakul P, Vongsmasa V, La-ongpanich P, Wasi P. Serum ferritin levels in thalassemias and the effect of splenectomy. Acta Haematol. (1981) 66:244–50. doi: 10.1159/000207129
64. Kittivorapart J, Crew VK, Wilson MC, Heesom KJ, Siritanaratkul N, Toye AM. Quantitative proteomics of plasma vesicles identify novel biomarkers for hemoglobin E/β-thalassemic patients. Blood Adv. (2018) 2:95–104. doi: 10.1182/bloodadvances.2017011726
65. Atipimonpat A, Siwaponanan P, Khuhapinant A, Svasti S, Sukapirom K, Khowawisetsut L, et al. Extracellular vesicles from thalassemia patients carry iron-containing ferritin and hemichrome that promote cardiac cell proliferation. Ann Hematol. (2021) 100:1929–46. doi: 10.1007/s00277-021-04567-z
66. Strzyz P. Iron expulsion by exosomes drives ferroptosis resistance. Nat Rev Mol Cell Biol. (2020) 21:4–5. doi: 10.1038/s41580-019-0195-2
67. Mukherjee C, Kling T, Russo B, Miebach K, Kess E, Schifferer M, et al. Oligodendrocytes provide antioxidant defense function for neurons by secreting ferritin heavy chain. Cell Metab. (2020) 32:259–72.e10. doi: 10.1016/j.cmet.2020.05.019
68. Brown CW, Mercurio AM. Ferroptosis resistance mediated by exosomal release of iron. Mol Cell Oncol. (2020) 7:1730144. doi: 10.1080/23723556.2020.1730144
69. Ito F, Kato K, Yanatori I, Murohara T, Toyokuni S. Ferroptosis-dependent extracellular vesicles from macrophage contribute to asbestos-induced mesothelial carcinogenesis through loading ferritin. Redox Biol. (2021) 47:102174. doi: 10.1016/j.redox.2021.102174
70. Osti D, Del Bene M, Rappa G, Santos M, Matafora V, Richichi C, et al. Clinical significance of extracellular vesicles in plasma from glioblastoma patients. Clin Cancer Res. (2019) 25:266–76. doi: 10.1158/1078-0432.ccr-18-1941
71. Kumar S, Sheokand N, Mhadeshwar MA, Raje CI, Raje M. Characterization of glyceraldehyde-3-phosphate dehydrogenase as a novel transferrin receptor. Int J Biochem Cell Biol. (2012) 44:189–99. doi: 10.1016/j.biocel.2011.10.016
72. Sheokand N, Kumar S, Malhotra H, Tillu V, Raje CI, Raje M. Secreted glyceraldehye-3-phosphate dehydrogenase is a multifunctional autocrine transferrin receptor for cellular iron acquisition. Biochim Biophys Acta. (2013) 1830:3816–27. doi: 10.1016/j.bbagen.2013.03.019
73. Dar GH, Mendes CC, Kuan W-L, Speciale AA, Conceição M, Görgens A, et al. GAPDH controls extracellular vesicle biogenesis and enhances the therapeutic potential of EV mediated siRNA delivery to the brain. Nat Commun. (2021) 12:6666. doi: 10.1038/s41467-021-27056-3
74. Liu X-B, Nguyen N-BH, Marquess KD, Yang F, Haile DJ. Regulation of hepcidin and ferroportin expression by lipopolysaccharide in splenic macrophages. Blood Cells Mol Dis. (2005) 35:47–56. doi: 10.1016/j.bcmd.2005.04.006
75. Bekri S, Gual P, Anty R, Luciani N, Dahman M, Ramesh B, et al. Increased adipose tissue expression of hepcidin in severe obesity is independent from diabetes and nash. Gastroenterology. (2006) 131:788–96. doi: 10.1053/j.gastro.2006.07.007
76. Bessman NJ, Mathieu JRR, Renassia C, Zhou L, Fung TC, Fernandez KC, et al. Dendritic cell-derived hepcidin sequesters iron from the microbiota to promote mucosal healing. Science. (2020) 368:186–9. doi: 10.1126/science.aau6481
77. Zhang V, Nemeth E, Kim A. Iron in lung pathology. Pharmaceuticals. (2019) 12:30. doi: 10.3390/ph12010030
78. Kulaksiz H, Theilig F, Bachmann S, Gehrke SG, Rost D, Janetzko A, et al. The iron-regulatory peptide hormone hepcidin: expression and cellular localization in the mammalian kidney. J Endocrinol. (2005) 184:361–70. doi: 10.1677/joe.1.05729
79. Schwarz P, Kübler JA, Strnad P, Müller K, Barth TF, Gerloff A, et al. Hepcidin is localised in gastric parietal cells, regulates acid secretion and is induced by Helicobacter pylori infection. Gut. (2012) 61:193–201. doi: 10.1136/gut.2011.241208
80. Zechel S, Huber-Wittmer K, von Bohlen und Halbach O. Distribution of the iron-regulating protein hepcidin in the murine central nervous system. J Neurosci Res. (2006) 84:790–800. doi: 10.1002/jnr.20991
81. Merle U, Fein E, Gehrke SG, Stremmel W, Kulaksiz H. The iron regulatory peptide hepcidin is expressed in the heart and regulated by hypoxia and inflammation. Endocrinology. (2007) 148:2663–8. doi: 10.1210/en.2006-1331
82. Malerba M, Louis S, Cuvellier S, Shambat SM, Hua C, Gomart C, et al. Epidermal hepcidin is required for neutrophil response to bacterial infection. J Clin Invest. (2020) 130:329–34. doi: 10.1172/JCI126645
83. Lakhal-Littleton S, Wolna M, Chung YJ, Christian HC, Heather LC, Brescia M, et al. An essential cell-autonomous role for hepcidin in cardiac iron homeostasis. Elife. (2016) 5:e19804. doi: 10.7554/eLife.19804
84. Arezes J, Foy N, McHugh K, Sawant A, Quinkert D, Terraube V, et al. Erythroferrone inhibits the induction of hepcidin by BMP6. Blood. (2018) 132:1473–7. doi: 10.1182/blood-2018-06-857995
85. Gardenghi S, Grady RW, Rivella S. Anemia, ineffective erythropoiesis, and hepcidin: interacting factors in abnormal iron metabolism leading to iron overload in β-thalassemia. Hematol Oncol Clin North Am. (2010) 24:1089–107. doi: 10.1016/j.hoc.2010.08.003
86. Levin C, Koren A, Rebibo-Sabbah A, Levin M, Koifman N, Brenner B, et al. Extracellular vesicle microrna that are involved in β-thalassemia complications. Int J Mol Sci. (2021) 22:9760. doi: 10.3390/ijms22189760
87. Sharp P, Srai SK. Molecular mechanisms involved in intestinal iron absorption. World J Gastroenterol. (2007) 13:4716–24. doi: 10.3748/wjg.v13.i35.4716
88. Donaldson GP, Lee SM, Mazmanian SK. Gut biogeography of the bacterial microbiota. Nat Rev Microbiol. (2016) 14:20–32. doi: 10.1038/nrmicro3552
89. Bäckhed F, Ley RE, Sonnenburg JL, Peterson DA, Gordon JI. Host-bacterial mutualism in the human intestine. Science. (2005) 307:1915–20. doi: 10.1126/science.1104816
90. Dostal A, Chassard C, Hilty FM, Zimmermann MB, Jaeggi T, Rossi S, et al. Iron depletion and repletion with ferrous sulfate or electrolytic iron modifies the composition and metabolic activity of the gut microbiota in rats. J Nutr. (2011) 142:271–7. doi: 10.3945/jn.111.148643
91. Buhnik-Rosenblau K, Moshe-Belizowski S, Danin-Poleg Y, Meyron-Holtz EG. Genetic modification of iron metabolism in mice affects the gut microbiota. Biometals. (2012) 25:883–92. doi: 10.1007/s10534-012-9555-5
92. Liu B, Pan X, Liu Z, Han M, Xu G, Dai X, et al. Fecal microbiota as a noninvasive biomarker to predict the tissue iron accumulation in intestine epithelial cells and liver. FASEB J. (2020) 34:3006–20. doi: 10.1096/fj.201901635RR
93. Deschemin J-C, Noordine M-L, Remot A, Willemetz A, Afif C, Canonne-Hergaux F, et al. The microbiota shifts the iron sensing of intestinal cells. FASEB J. (2015) 30:252–61. doi: 10.1096/fj.15-276840
94. Das NK, Schwartz AJ, Barthel G, Inohara N, Liu Q, Sankar A, et al. Microbial metabolite signaling is required for systemic iron homeostasis. Cell Metab. (2020) 31:115–30.e6. doi: 10.1016/j.cmet.2019.10.005
95. Taylor M, Qu A, Anderson ER, Matsubara T, Martin A, Gonzalez FJ, et al. Hypoxia-inducible factor-2α mediates the adaptive increase of intestinal ferroportin during iron deficiency in mice. Gastroenterology. (2011) 140:2044–55. doi: 10.1053/j.gastro.2011.03.007
96. Zhang D, Gao X, Li H, Borger DK, Wei Q, Yang E, et al. The microbiota regulates hematopoietic stem cell fate decisions by controlling iron availability in bone marrow. Cell Stem Cell. (2022) 29:232–47.e7. doi: 10.1016/j.stem.2021.12.009
97. Nicolas G, Chauvet C, Viatte L, Danan JL, Bigard X, Devaux I, et al. The gene encoding the iron regulatory peptide hepcidin is regulated by anemia, hypoxia, and inflammation. J Clin Invest. (2002) 110:1037–44. doi: 10.1172/JCI15686
98. Aschemeyer S, Qiao B, Stefanova D, Valore EV, Sek AC, Ruwe TA, et al. Structure-function analysis of ferroportin defines the binding site and an alternative mechanism of action of hepcidin. Blood. (2018) 131:899–910. doi: 10.1182/blood-2017-05-786590
99. Clarke G, Stilling RM, Kennedy PJ, Stanton C, Cryan JF, Dinan TG. Minireview: gut microbiota: the neglected endocrine organ. Mol Endocrinol. (2014) 28:1221–38. doi: 10.1210/me.2014-1108
100. Peyssonnaux C, Zinkernagel AS, Datta V, Lauth X, Johnson RS, Nizet V. TLR4-dependent hepcidin expression by myeloid cells in response to bacterial pathogens. Blood. (2006) 107:3727–32. doi: 10.1182/blood-2005-06-2259
101. Layoun A, Santos MM. Bacterial cell wall constituents induce hepcidin expression in macrophages through MYD88 signaling. Inflammation. (2012) 35:1500–6. doi: 10.1007/s10753-012-9463-4
102. Seyoum Y, Baye K, Humblot C. Iron homeostasis in host and gut bacteria – A complex interrelationship. Gut Microbes. (2021) 13:1874855. doi: 10.1080/19490976.2021.1874855
103. Jan AT. Outer membrane vesicles (OMVS) of gram-negative bacteria: a perspective update. Front Microbiol. (2017) 8:1053. doi: 10.3389/fmicb.2017.01053
104. Bose S, Aggarwal S, Singh DV, Acharya N. Extracellular vesicles: an emerging platform in gram-positive bacteria. Microbial Cell. (2020) 7:312–22. doi: 10.15698/mic2020.12.737
105. Prados-Rosales R, Weinrick BC, Piqué DG, Jacobs WR, Casadevall A, Rodriguez GM. Role for Mycobacterium tuberculosis membrane vesicles in iron acquisition. J Bacteriol. (2014) 196:1250–6. doi: 10.1128/JB.01090-13
106. Wang M, Nie Y, Wu X-L. Membrane vesicles from a Dietzia bacterium containing multiple cargoes and their roles in iron delivery. Environ Microbiol. (2021) 23:1009–19. doi: 10.1111/1462-2920.15278
107. Ñahui Palomino RA, Vanpouille C, Costantini PE, Margolis L. Microbiota–host communications: bacterial extracellular vesicles as a common language. PLoS Pathog. (2021) 17:e1009508. doi: 10.1371/journal.ppat.1009508
108. Díaz-Garrido N, Badia J, Baldomà L. Microbiota-derived extracellular vesicles in interkingdom communication in the gut. J Extracell Vesicles. (2021) 10:e12161. doi: 10.1002/jev2.12161
109. Díez-Sainz E, Milagro FI, Riezu-Boj JI, Lorente-Cebrián S. Effects of gut microbiota–derived extracellular vesicles on obesity and diabetes and their potential modulation through diet. J Physiol Biochem. (2022) 78:485–99. doi: 10.1007/s13105-021-00837-6
110. Smith JL. The physiological role of ferritin-like compounds in bacteria. Crit Rev Microbiol. (2004) 30:173–85. doi: 10.1080/10408410490435151
111. Arosio P, Elia L, Poli M. Ferritin, cellular iron storage and regulation. IUBMB Life. (2017) 69:414–22. doi: 10.1002/iub.1621
112. Zakharzhevskaya NB, Vanyushkina AA, Altukhov IA, Shavarda AL, Butenko IO, Rakitina DV, et al. Outer membrane vesicles secreted by pathogenic and nonpathogenic Bacteroides fragilis represent different metabolic activities. Sci Rep. (2017) 7:5008. doi: 10.1038/s41598-017-05264-6
113. Hu R, Lin H, Wang M, Zhao Y, Liu H, Min Y, et al. Lactobacillus reuteri-derived extracellular vesicles maintain intestinal immune homeostasis against lipopolysaccharide-induced inflammatory responses in broilers. J Anim Sci Biotechnol. (2021) 12:25. doi: 10.1186/s40104-020-00532-4
114. Dean SN, Rimmer MA, Turner KB, Phillips DA, Caruana JC, Hervey WJT, et al. Lactobacillus acidophilus membrane vesicles as a vehicle of bacteriocin delivery. Front Microbiol. (2020) 11:710. doi: 10.3389/fmicb.2020.00710
115. Olaya-Abril A, Prados-Rosales R, McConnell MJ, Martín-Peña R, González-Reyes JA, Jiménez-Munguía I, et al. Characterization of protective extracellular membrane-derived vesicles produced by Streptococcus pneumoniae. J Proteomics. (2014) 106:46–60. doi: 10.1016/j.jprot.2014.04.023
116. Matsumoto J, Stewart T, Banks WA, Zhang J. The transport mechanism of extracellular vesicles at the blood-brain barrier. Curr Pharmaceut Design. (2017) 23:6206–14. doi: 10.2174/1381612823666170913164738
Keywords: iron metabolism, extracellular vesicles, gut microbiota, ferritin, transferrin receptors, hepcidin
Citation: Daou Y, Falabrègue M, Pourzand C, Peyssonnaux C and Edeas M (2022) Host and microbiota derived extracellular vesicles: Crucial players in iron homeostasis. Front. Med. 9:985141. doi: 10.3389/fmed.2022.985141
Received: 03 July 2022; Accepted: 26 September 2022;
Published: 13 October 2022.
Edited by:
Puja Khare, Council of Scientific and Industrial Research (CSIR), IndiaReviewed by:
Suresh V. Chinni, MAHSA University, MalaysiaRajnikant Dixit, National Institute of Malaria Research (ICMR), India
Copyright © 2022 Daou, Falabrègue, Pourzand, Peyssonnaux and Edeas. This is an open-access article distributed under the terms of the Creative Commons Attribution License (CC BY). The use, distribution or reproduction in other forums is permitted, provided the original author(s) and the copyright owner(s) are credited and that the original publication in this journal is cited, in accordance with accepted academic practice. No use, distribution or reproduction is permitted which does not comply with these terms.
*Correspondence: Marvin Edeas, bWFydmluLmVkZWFzQGluc2VybS5mcg==