- 1Instituto Gonçalo Moniz, Fundação Oswaldo Cruz, Salvador, Brazil
- 2Faculdade de Medicina, Universidade Federal da Bahia, Salvador, BA, Brazil
- 3Multinational Organization Network Sponsoring Translational and Epidemiological Research Initiative, Salvador, Brazil
- 4Curso de Medicina, Universidade Salvador, Salvador, Brazil
- 5Programa de Pós-Graduação em Clínica Médica, Universidade Federal do Rio de Janeiro, Rio de Janeiro, Brazil
- 6Bahiana School of Medicine and Public Health, Bahia Foundation for the Development of Sciences, Salvador, Brazil
- 7Immunobiology Section, Laboratory of Parasitic Diseases, National Institute of Allergy and Infectious Diseases, National Institutes of Health, Bethesda, MD, United States
Tuberculosis (TB) is a lethal disease and remains one of the top ten causes of mortality by an infectious disease worldwide. It can also result in significant morbidity related to persistent inflammation and tissue damage. Pulmonary TB treatment depends on the prolonged use of multiple drugs ranging from 6 months for drug-susceptible TB to 6–20 months in cases of multi-drug resistant disease, with limited patient tolerance resulting from side effects. Treatment success rates remain low and thus represent a barrier to TB control. Adjunct host-directed therapy (HDT) is an emerging strategy in TB treatment that aims to target the host immune response to Mycobacterium tuberculosis in addition to antimycobacterial drugs. Combined multi-drug treatment with HDT could potentially result in more effective therapies by shortening treatment duration, improving cure success rates and reducing residual tissue damage. This review explores the rationale and challenges to the development and implementation of HDTs through a succinct report of the medications that have completed or are currently being evaluated in ongoing clinical trials.
Introduction
Tuberculosis (TB) is caused by infection with Mycobacterium tuberculosis (Mtb), and represents one of the most important infectious diseases worldwide (1). Until the COVID-19 pandemic, TB was the leading cause of death from a single infectious agent (2).
Throughout the past century, TB morbidity and mortality have declined significantly as a result of a number of factors including improved socioeconomic conditions, introduction of intradermal Bacilli Calmette-Guerin vaccine (BCG), particularly in children younger than 5 years old (3, 4) and most importantly with the introduction of antimycobacterial treatment (5). The use of highly effective therapy against HIV, a co-infection primarily responsible for increased TB incidence and death over the past decades, has also positively impacted TB control (4, 6). Notably, widespread access to anti-TB medications resulted in the closure of inpatient hospitals with a shift to outpatient-based treatment (5).
While worldwide efforts to curb TB incidence and mortality have been effective, the COVID-19 pandemic and subsequent limited access to health services has reversed years of progress in providing essential TB services and reducing TB disease burden (1). In addition, new challenges to control TB include continued insufficient treatment success rates, low treatment adherence and the emergence of drug resistant TB infections (7). In 2020, 132,222 individuals were diagnosed with multidrug resistant or rifampicin resistant TB (MDR/RR-TB) along with 25,681 subjects classified as extensively drug resistant TB (XDR-TB) patient, a decrease in 22% compared with 2019 (201,997) that reflects underdiagnosis of this condition (1).
Current TB treatment relies on a combination of multiple antimicrobial drugs with treatment duration ranging from 6 months for drug-susceptible TB to 6–20 months for MDR/RR-TB and even longer in cases of XDR-TB or poor clinical response (8). Globally, the TB treatment success rate is 85% for drug-susceptible TB and 57% for MDR/RR-TB (1). Outcomes are affected by several factors ranging from social determinants to the long duration and complexity of medication regimens, which directly impact patient adherence to the therapeutic protocol as well as drug toxicity (9). While changing social factors to improve treatment success is a complex, lengthy, and gradual process, development of more effective, affordable, and well-tolerated medications may shorten treatment duration and reduce collateral effects thereby improving treatment outcomes.
Two different strategies have been adopted to develop novel therapeutics: (1) traditional search for new antimycobacterial drugs, (2) host directed therapy (HDT) capable of modulating the immune response to TB as adjuvant therapy to current anti-TB treatment. The development of new anti-TB drugs is a lengthy and costly process (10), thus the study of HDT may offer an effective alternative that is readily available.
Host-directed therapy (HDT) has emerged as an attractive adjuvant treatment option using repurposed approved immune modulation therapy. It has become apparent that the determinants of TB immunopathogenesis and the mechanisms underlying successful infection control involve the following domains: inflammation (Figures 1A–C) (11), cellular metabolism (Figure 1D) (12), and the mechanisms used by Mtb to evade the immune system (13; Figure 1E). HDTs aim to modulate host factors to enhance favorable responses and dampen host detrimental responses, which contribute to tissue damage and perpetuation of mycobacterial multiplication (14). If proven to be beneficial, HDT may aid in resolving unmet needs in TB treatment, thereby resulting in improved adherence, reduction of resistant strains, shortened treatment duration and Mtb transmission in the community with increased cure rates and fewer chronic sequelae caused by excessive inflammatory response to TB (15).
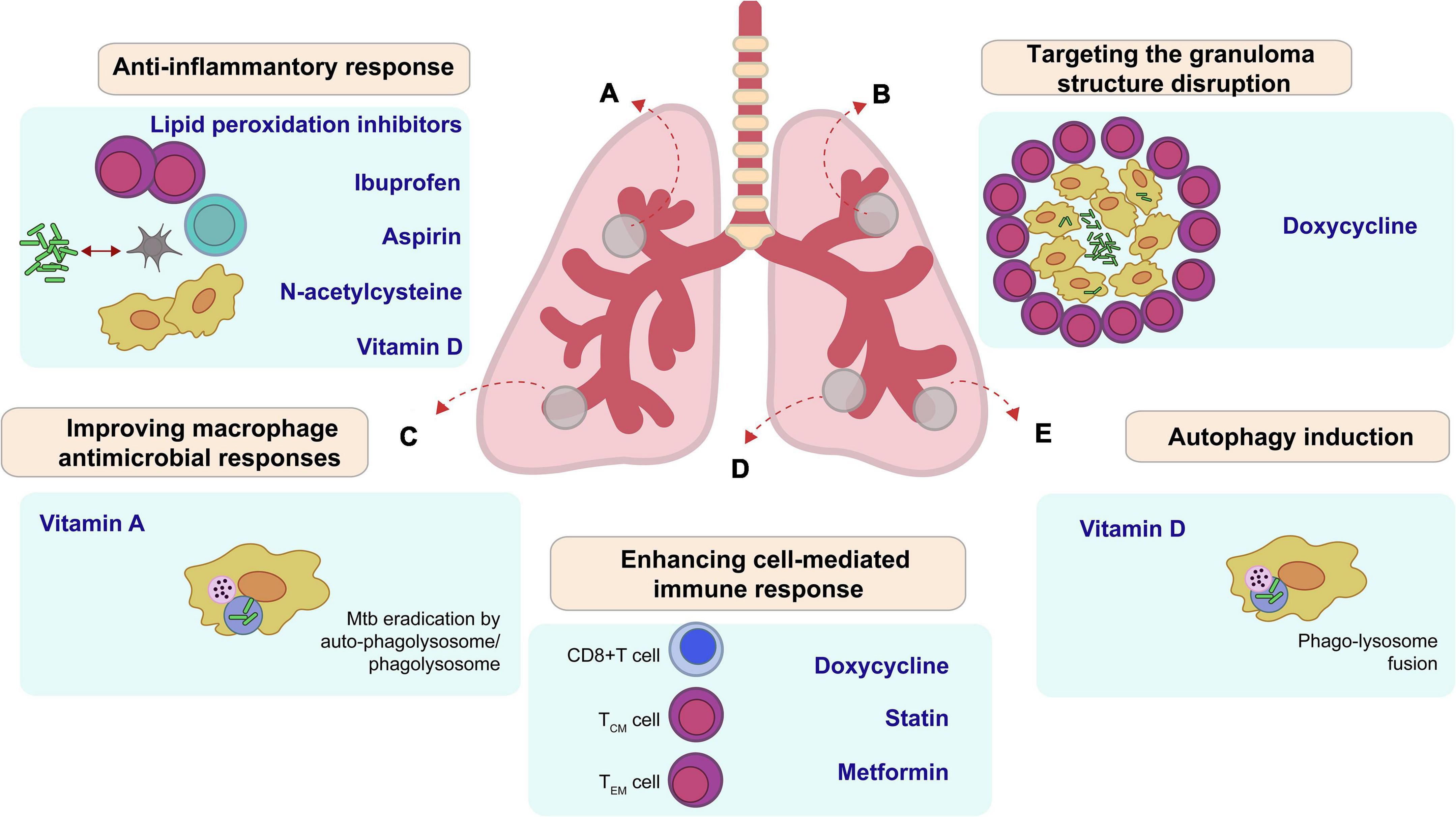
Figure 1. Main potential host therapeutic targets (HDT) improve outcome in Mycobacterium tuberculosis. (A) Lipid peroxidation inhibitors, Ibuprofen, Aspirin, N-acetylcysteine, and Vitamin D suppress proinflammatory responses, which decrease inflammation and tissue damage during active stage of the disease. (B) Doxycycline changes the integrity of granuloma and enhances drug accessibility. (C) Vitamin A reduces bacilli growth by apoptosis or auto-phagolysosome. (D) Doxycycline, Statin, and Metformin regulate cell-mediated immune responses, including antigen-specific T cell responses. (E) Some HDT like vitamin D induce autophagy in infected cells.
Currently, there are many potential therapies with ongoing research at different stages in the pipeline for HDT in TB. Most are repurposed drugs in pre-clinical or clinical studies to be used as adjuvants with anti-TB therapy. This review aims to describe the rationale used in the development of HDTs, their potential and main challenges as adjuvant therapy, as well as to provide a succinct report of the medications that have completed or are evaluated in ongoing clinical trials (CT), registered in the ClinicalTrials.gov database.
Tuberculosis and the immune system
After infection with Mtb, the development of active disease results from both pathogen and host factors (16). Immune response against Mtb is a very complex and dynamic process, involving different cell types, cytokines and chemokines (17). Multiple inflammatory cells such as macrophages, monocytes, dendritic cells, neutrophils, epithelioid cells, and multinucleated giant cells, enclosed by B and T lymphocytes, accumulate at the tissue level to form a granuloma (18). Myeloid cells produce many cytokines and chemokines that are critical to recruit additional leukocytes from capillary vessels (19, 20). The interaction between differentially localized populations of intracellular Mtb and the cellular organelles will dictate whether Mtb replicates or restrict its growth through control of intracellular bacilli (21). The majority of Mtb exposed individuals contain primary infection with the formation of granulomas. Nevertheless, it is possible that a small proportion of bacilli survive, driving infection into a latent stage. The other potential outcome is increased hypoxic necrotic centers, rich in lipids and foamy macrophages that fail to control bacterial replication, ultimately leading to granuloma caseation (20). This process is responsible for the latter formation of cavities and destruction of alveolar cells, vessels, and bronchi, with consequent bacilli spread (19). The fate of granulomas is determined by a variety of host factors that involve a network of inflammatory cytokines, eicosanoids, prostaglandins, and other mediators contributing to disease exacerbation as well as tissue necrosis (19, 20). Therefore, modulating host immune response could potentially optimize TB treatment, although the ideal target for HDTs remains unclear (22).
Currently, multiple therapies that act on different host immune targets are under investigation including the following: modulation of vascular endothelial growth factor (VEGF) potentially reduces central necrosis and improves drug delivery (23); reduction of neutrophil-mediated inflammation (i.e., aspirin) to limit severe tissue damage (24); and modulation of tumor necrosis factor alpha (TNFα), transforming growth factor beta (TGF-β), and Interleukin-1 beta (IL-1β) may reduce lung damage (25).
Potential advantages of host-directed therapy in tuberculosis
Some medications studied as HDT in TB are used for other conditions and offer a wealth of clinical experience and research, such as acetylsalicylic acid or statins, to bypass the need to explore the safety and toxicity properties in prolonged use. Furthermore, most of the studied drugs are already available worldwide with accessible costs, facilitating, and accelerating their incorporation into routine practice if benefit in TB treatment is proven in robust CTs (26). The use of HDTs may avoid the undesirable adverse effects with prolonged use of repurposed antimicrobials such as oxazolidonones, carbapenems, and fluorquinolones in TB treatment (27). Among other adverse effects, long-term therapies employing broad-spectrum antibiotics will contribute to the emergence of antibiotic-resistant strains of Mtb as well as other opportunistic pathogens (28). Lastly, anti-inflammatory effects offered by some HDT agents may lead to potential benefits in the host by reducing tissue damage and improving long-term quality of life (14).
The challenge of finding effective host-directed therapy
In vitro studies play an essential role in the screening of potential drugs (29), while animal studies allow the understanding of immunopathology, as well the confirmation of mycobacterial infection control, as measured by the drug impact in mycobacterial load, time to sterilization of lesions, tissue damage size and overall survival (30). With the knowledge obtained through both experimental models, clinical studies to validate these findings will be critical to address questions beyond drug efficacy (31; Figure 2).
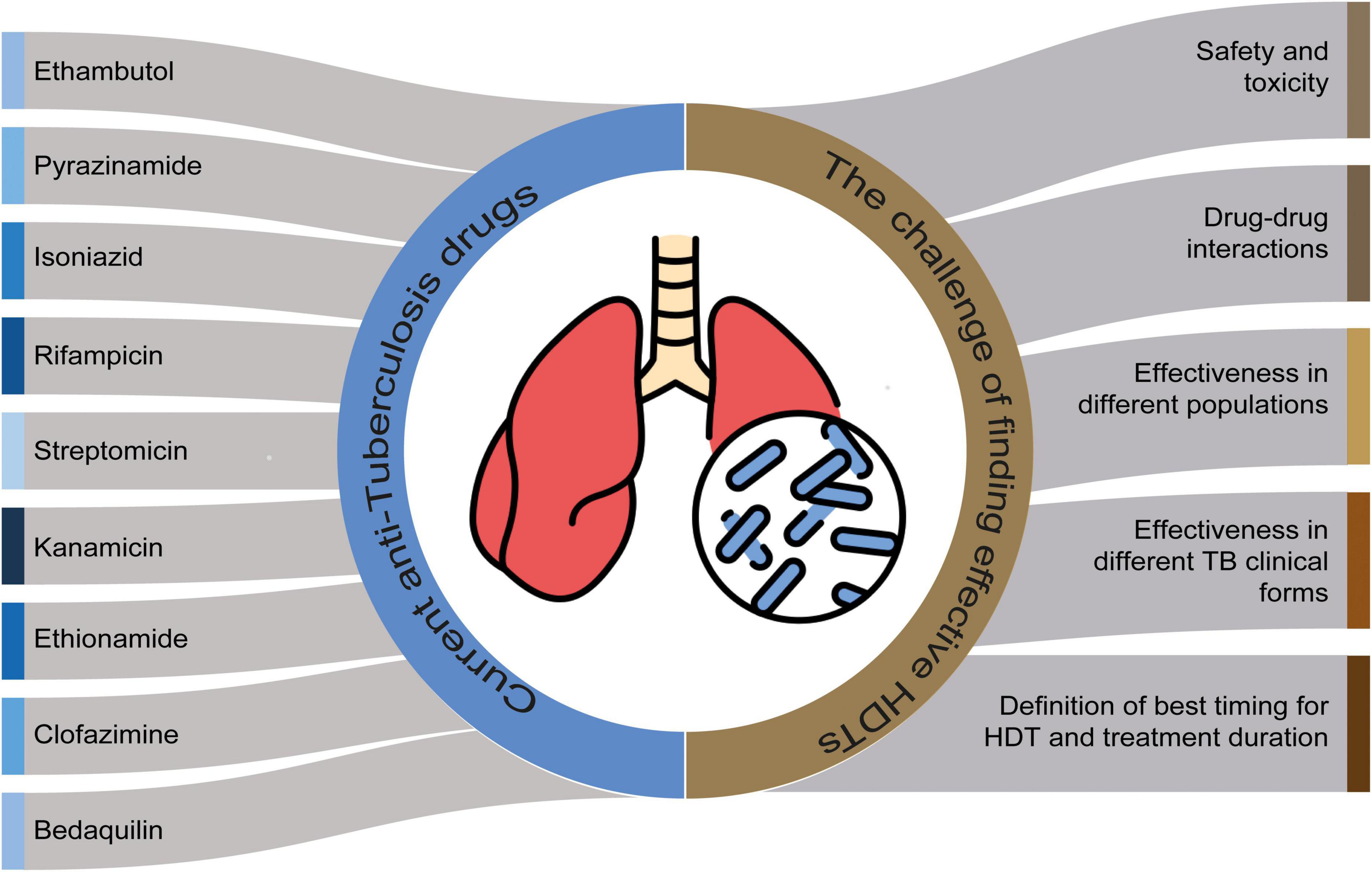
Figure 2. The challenge of finding effective host-directed therapy (HDTs) and the current anti-tuberculosis therapy. Perspectives for clinical studies for HDT efficacy.
Safety and toxicity
Though many drugs re-purposed as HDTs have known safety profiles, they must be evaluated in the context of individuals with TB, given that factors such as malnutrition, co-infection with HIV and inflammation tend to alter drug metabolism (32, 33).
Drug-drug interactions
The concomitant use of HDT with anti-TB therapy needs to be evaluated. For instance, mild risk of hepatotoxicity of some drugs could be potentiated when used along with anti-mycobacterial drugs. Additionally, it is important to evaluate drug-drug interactions with antiretroviral therapy, as co-infection with TB-HIV is common and of particular concern (26).
Effectiveness in different populations
People living with HIV (PWH) and those with other types of immunosuppression, children, and individuals with resistant TB may have different immune responses to TB and thus could respond differently to HDTs (34). Similarly, ethnic differences in TB immune response may also be reflected in different responses to HDTs (35). It is critical that clinical studies include ethnically diverse and clinically relevant populations.
Effectiveness in different clinical forms of tuberculosis
While most studies focus on pulmonary TB, extra-pulmonary TB has a high prevalence in some areas, particularly in countries with a high burden of TB (36) with worse outcomes when compared with pulmonary TB (37–39). For instance, having tuberculous meningitis or disseminated TB is associated with lower cure rates and higher mortality rates (37–39).
Determine the most effective protocol for host-directed therapy
There may be an ideal time for use during disease course depending on how the host immune response is modulated, and administration in the wrong time frame may be deleterious (31, 40).
Evaluation of the possibility of reducing total treatment time
Earlier sterilization and control of inflammation may result in shorter antimycobacterial treatment durations leading to improved patient adherence and increased likelihood of cure (41).
Drugs targeting the anti-inflammatory response
Aspirin
Aspirin is a drug based on acetylsalicylic acid that performs antiplatelet (42), anti-inflammatory (43), and analgesic (44) functions and is a potential adjuvant in the treatment of TB (45, 46).
The anti-inflammatory role of aspirin has been increasingly studied for modulating neutrophil-mediated inflammatory responses. The effect of low-dose aspirin seems to enhance control of bacillary load and improve survival in the late stages of TB in C3HeB/FeJ murine model (45). A study in C3HeB/FeJ mice found that low-dose aspirin had an anti-inflammatory effect in the later stage of active TB by reducing excess, non-productive inflammation, while enhancing Th1-cell responses for the elimination of bacilli (47). In BALB/c mice, aspirin administration enhanced the effect of pyrazinamide and resulted in additional clearance of viable mycobacteria in the lungs and spleen during the initial phase of TB treatment (48). However, the combination of aspirin and Isoniazid-like treatment of murine pulmonary TB was associated with increased mycobacterial load in the spleen and lungs (48). Together, these findings highlight the urgent need for additional clinical studies to assess the impact of timing in disease course and the efficacy of concomitant aspirin use with different anti-TB drug combinations.
In addition to the anti-inflammatory effects, the antiplatelet role of aspirin may also be beneficial in TB treatment given that TB promotes a basal state of hypercoagulability that favors thromboembolic events (49) and platelets have been directly associated with pro-inflammatory status (50). A cohort study of pulmonary TB patients from Taiwan found that low doses of aspirin were associated with decreased morbidity and increased survival of patients on anti-TB regimen, without increasing the risk of bleeding (46). Similarly, a phase two CT in HIV-unexposed adults with tuberculous meningitis found that 1,000 mg of aspirin reduced 3-month mortality rates for this group of patients (51). A hypercoagulation state is present in tuberculous meningitis, leading to vascular complications (49). Using aspirin in this scenario has previously been shown to reduce the incidence of strokes and mortality at 3 months (52).
The results of these preliminary studies are underpowered and require additional robust CTs to prompt a change in current treatment guidelines. Nevertheless, they suggest that aspirin may aid TB treatment in all cases or in subgroups, in those with pulmonary TB or tuberculous meningitis. Importantly, the use of aspirin would be easily implemented given the low cost, high availability and limited side effect profile.
The ClinicalTrials.gov database lists one ongoing multi-center, phase IIB, placebo controlled, randomized CT that aims to evaluate the efficacy and safety of aspirin and ibuprofen as adjunct drugs in TB treatment, as detailed in Table 1.
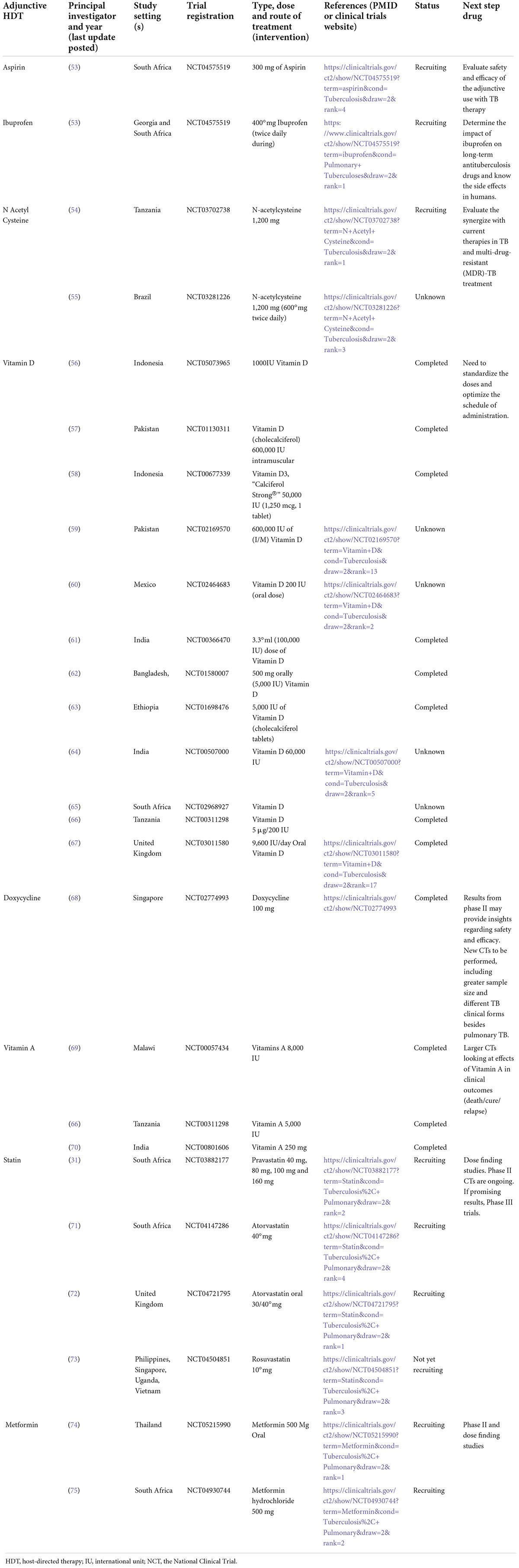
Table 1. Clinical trials investigating drugs for host directed therapy in pulmonary tuberculosis (TB).
Ibuprofen
Ibuprofen is a non-steroidal anti-inflammatory drug that inhibits both COX1 and COX2 cyclooxygenases. It is widely used and has an excellent safety profile, even in children (76). In a mouse model mimicking active TB in humans, the use of ibuprofen reduced bacillary load and affected lung area, leading to increased survival (77). These effects are mainly attributed to inhibition of the synthesis of PGE2, which inhibits phagocytosis, bacterial killing, production of nitrite (76) and T-helper 1 cytokines, and production of tumor necrosis factor α (TNF-α) (78). Another study in a murine model noted that ibuprofen enhanced the bactericidal effect of pyrazinamide during TB treatment (48). As noted above, this approach is currently under investigation in a phase II multi-center placebo-controlled trial (Table 1).
Antioxidants: N-acetylcysteine and lipid peroxidation inhibitors
N-acetylcysteine (NAC) is a potent antioxidant widely used as a mucolytic agent in chronic obstructive pulmonary disease (COPD) and cystic fibrosis (79). The role of NAC in TB therapy remains under study. To date, prior studies have indicated that NAC might reduce host oxidative responses (80), reduce pro-inflammatory cytokines such as IL-1, IL-6, and TNF-α (81) and have direct antimycobacterial properties (81, 82).
A study conducted in Brazil compared oxidative stress status in plasma of patients with pulmonary TB, latent TB infection, and healthy uninfected individuals (82). Pulmonary TB patients exhibited higher levels of oxidation products and a reduction of antioxidants compared with latent TB cases or uninfected controls. Cultures were exposed to different doses of NAC and the authors found decreased oxidative stress in treated macrophages and reduced mycobacterial growth when exposed to a high concentration of NAC (82). The capacity of NAC to control Mtb infection was further tested in vivo in a mouse (C57BL/6) model, resulting in a significant reduction of mycobacterial loads in the lungs (82).
In a phase II randomized CT that evaluated the adjuvant use of NAC in hospitalized individuals with TB-HIV (RIPENACTB study) (80), NAC-treated patients exhibited a significant increase in glutathione levels and total antioxidant status along with lowered levels of lipid peroxidation, a toxic process of oxidative stress response. In this study, the adjuvant use of NAC was not unsafe (80). Similarly, another randomized CT in those with newly diagnosed pulmonary TB in India reported a significant increase in glutathione peroxidase levels in patients receiving NAC and conventional anti-TB therapy compared to placebo group (patients under TB therapy only). Patients receiving NAC-based adjunctive therapy exhibited significant reduction of radiological lung infiltration, faster sputum conversion and more regulated immunological response, when compared to the group without NAC. A substantial body weight gain and improved antioxidant status was noted in the intervention group suggesting a potential promising role for NAC as adjuvant anti-TB therapy (83). Moreover, NAC may play a role in preventing hepatotoxicity of anti-TB usual therapy. An Iranian randomized CT (84) evaluated the effect of adjuvant NAC in those undergoing four drug anti-TB therapy compared with those without NAC therapy. Liver enzyme levels including aspartate aminotransferase, alanine aminotransferase and bilirubin were significantly lower following 1 and 2 weeks of NAC treatment. In this study NAC co-administration appeared to reduce the risk of hepatotoxicity commonly associated with anti-TB therapy.
N-acetylcysteine is a low-cost drug that seems to be safe for use in pulmonary TB treatment. Nevertheless, its effectiveness is still to be proven. One CT is currently in progress to clarify this question (Table 1).
Autophagy induction
Vitamin D
Vitamin D (VITD) participates in the reabsorption of calcium from the bone and intestine and has a fundamental role in bone constitution and remodeling (85). It also acts as an immunomodulatory hormone and influences other processes including central nervous system function and cardiovascular health (86, 87).
In the presence of Mtb, VITD plays an essential role in activated macrophages and monocytes in response to antigen exposure by enhancing levels of 1,25(OH) 2D in monocyte/macrophages from normal human hosts (88). The increased levels of 1,25(OH) 2D induces the expression of cathelicidin, an antimicrobial protein responsible for killing infectious agents like Mtb (89). Some studies have reported that VITD can down-regulate the expression of mTOR protein, thus inducing autophagy (90). Importantly, VITD deficiency has been associated with susceptibility to TB infection in a comparative cross-sectional study that identified a high prevalence of VITD deficiency among newly diagnosed TB patients and in their household contacts (91). To date, 10 completed studies and four ongoing related to VITD and pulmonary TB were identified in the ClinicalTrials.gov records (Table 1).
The use of VITD as adjunctive therapy has had conflicting results regarding improvement of sputum conversion. Faster sputum conversion rates were found in TB patients receiving adjunctive VITD supplementation in a randomized placebo-controlled CT in Indonesia (92) and China (93). Another CT from Bangladesh demonstrated enhanced intracellular killing of Mtb in macrophages ex vivo in combination with increased sputum culture conversion at week 4 and at week 8 compared to the placebo group (94). This effect, however, was unable to be replicated in a number of CTs of VITD supplementation in TB disease (93, 95–97). Two CTs with MDR/RR-TB patients in Georgia (95) and Mongolia (96) identified higher sputum culture conversion with high dose of VITD use, suggesting a possible role in this subset of patients with MDR/RR TB. Another randomized controlled trial found that daily VITD administration in TB infected patients led to enhanced clinical recovery, particularly those with lower levels of VITD and an elevated TB score at enrollment (97).
Although lower levels of VITD are commonly observed in individuals with pulmonary TB, clinical and bacteriological results from randomized controlled trials of adjunctive VITD supplementation have demonstrated limited clinical benefits. A meta-analysis found that there is no evidence to support the adjuvant use of VITD. Most studies are limited by small sample sizes and include only HIV-uninfected adults with pulmonary TB (98). Additional larger studies are needed to investigate the effects of VITD and other micronutrients in specific TB treatment subgroups that have worse prognosis, immunosupression, MDR/RR-TB and individuals with diabetes.
Targeting the granuloma structure disruption
Doxycycline
Matrix metalloproteinases (MMPs) are proteolytic enzymes capable of degrading collagen and other structural proteins (99). When highly expressed, as in inflammatory conditions, they contribute to tissue damage (100). TB leads to upregulation of MMPs and an imbalance between MMPs and tissue inhibitors of metalloproteinases (TIMPs) (101, 102). This imbalance is associated with TB severity and extent of TB lesions, as well as formation of lung cavitation (103), which in turn is associated with high bacillary burden, delayed sputum culture conversion, emergence of drug resistance, and higher transmission of Mtb (104). MMPs also play a role in the formation of granulomas (105) and are responsible for the breakdown of the blood brain barrier, leading to poor outcomes in cases of central nervous system TB (106–108). In this context, inhibitors of MMPs may be a particularly effective HDT for TB. Doxycycline is the only FDA−approved MMP inhibitor (102). It is originally used as a bacteriostatic antibiotic of the tetracycline class. It is also an inhibitor of MMP1 and MMP9 (102) which may be responsible for reducing pulmonary cavity volume and loss of granuloma size (109). Doxycycline has been shown to inhibit mycobacterial growth in animal and in vitro models (110, 111). A pilot phase 2 CT (Doxy-TB) (109), comparing doxycycline plus standard TB therapy to placebo plus standard TB therapy has been concluded with results pending (Table 1). Doxycycline is a licensed, safe and affordable drug, with significant potential to improve TB outcomes (112). Future CTs are needed with larger sample sizes and different TB clinical forms besides pulmonary TB, such as central nervous system TB.
Improving macrophage antimicrobial responses
Vitamin A
Vitamin A deficiency is a serious and widespread public health problem (113). It is more common during infection and can increase the severity of infectious diseases and the risk of death (113).
A recently systematic review/meta-analysis found that supplementation with vitamin A associated with earlier sputum conversion, decreased abnormalities in chest radiography, and improved lung function in patients undergoing TB treatment (114). A randomized controlled trial with a 2 × 2 factorial design in Qingdao, China determined that adjunctive supplementation with vitamin A did not improve time to smear conversion in pulmonary TB patients (93). Conversely, a double-blind, placebo-controlled study in patients with newly diagnosed TB found that vitamin A supplementation was associated with earlier sputum smear conversion (115). Furthermore, a randomized placebo-controlled, double-blind, two-by-two factorial trial evaluated the use of multivitamin/mineral supplementation with vitamin A and found a significant decrease in mortality during treatment of sputum-positive TB patients co-infected with HIV (116).
Three CTs registered with ClinicalTrials.gov with the status of completed were identified for Vitamin A supplementation in TB treatment (Table 1). The CT NCT00057434 conducted in Malawi did not identify a survival benefit with micronutrient supplementation with vitamin A in adults with HIV and pulmonary TB (69). Another CT (NCT00311298) found that multi-vitamin/mineral supplementation (Vitamin A) and zinc decrease mortality during treatment in patients with HIV and pulmonary TB (116). The remaining CT (NCT00801606) observed that micronutrient supplementation (Vitamin A) during treatment is associated with weight gain in children with TB though did not impact the clearance of lesions by chest X-ray (117).
These studies highlight conflicting results, though significantly lack evaluation of impact on clinical relevant outcomes (cure/death/recurrence). Larger trials with clinical important outcomes are needed to better evaluate the adjuvant use of vitamin A in TB treatment.
Enhancing cell-mediated immune response
Statins
Mycobacterium tuberculosis has been shown to thrive in cholesterol rich environments, as cholesterol can improve both survival and growth of the pathogen in a host cell. Mtb uses cholesterol in the macrophage membrane to bind and enter the cell (118). Subsequently, after the infection, macrophage accumulates lipid bodies forming foamy cells that utilize cholesterol as the main source of nutrition for bacteria. The lipid bodies have also been associated with Mtb growth restriction, drug resistance and delayed phagosome maturation due to enhanced IL-10 induction (119).
Statins are the most used cholesterol reducing drugs. They are inhibitors of 3-hydroxy-3-methylglutaryl coenzyme reductase (HMG-CoA), with both cholesterol lowering and anti-inflammatory and immunomodulatory functions and offer the most promising candidates for adjuvant HDT against TB (15, 120). The mechanism of action of statins against TB continue to be studied though research to date has identified the following: restrict the generation of foamy cells that otherwise support Mtb persistence by decreasing cholesterol biosynthesis (121); promote phagosome maturation and autophagy (122); increase percentages of Natural killer T (NKT) cells within cultures and expression of co-stimulatory molecules on monocytes, with higher secretion of IL-1b and IL-12p70 (123, 124); inhibit TGF-β (125, 126). Animal studies have found that simvastatin therapy in Mtb-infected mice reduces dissemination of Mtb from the lungs (122), promotes killing of intracellular Mtb by macrophages and enhances the bactericidal activity of isoniazid (119) and rifampin (127). A study in mice evaluated the treatment-shortening potential of therapy with statins plus anti-TB drugs and found a reduced time required to eradicate TB infection with no increased risk of relapse (128).
Some retrospective studies in Taiwan and South Korea have evaluated the role of statin use in preventing TB finding that chronic statin users had a lower risk of developing TB, compared to non-statin users (120, 129). Though intriguing, these study findings unfortunately have not been reproduced in different populations distinct from those with COPD and diabetes and lack adjustment for important confounding factors (130–134). A meta-analysis of data from nine of these cohort studies concluded that statin use was associated with reduced incidence of active TB (135). To our knowledge no retrospective studies have evaluated the impact of statins during TB treatment on clinical outcomes.
Four ongoing CTs of statins in TB may offer further clarity (Table 1): StAT-TB, a phase 2B dose finding study using pravastatin (NCT03882177); ATORTUB, using atorvastatin (NCT04721795); ROSETTA, using rosuvastatin (NCT04504851), and StatinTB (NCT04147286), a proof-of-concept phase II study testing the use of atorvastatin in reducing lung inflammation after TB treatment.
Given the pre-clinical studies results and the number of ongoing CTs, statins may represent one of the most promising drugs in the pipeline for HDT. If proven beneficial in clinical studies, its use in clinical practice can be easily implemented, considering its low cost and wide availability though likely will need additional safety testing given the possibility of hepatoxicity.
Metformin
Metformin is the first-line therapy in those with type 2 diabetes (136) and is a safe and widely used medication. Results from multiple studies suggest that concurrent use of metformin for TB may be beneficial even in non-diabetic individuals (137).
How metformin acts against TB remains unclear. Pre-clinical studies have found that it facilitates phagosome-lysosome fusion and promotes expression of 5′ adenosine monophosphate-activated protein kinase (AMPK) (14), an enzyme usually activated during metabolic stress that controls energy homeostasis (138). As such, metformin may promote increased production of reactive oxygen species (ROS) and subsequent killing of intracellular Mtb (14).
A study performed by Singhal et al. in TB infected mice revealed that metformin reduced intracellular growth of Mtb, enhanced the efficacy of anti-TB drugs, and reduced lung damage and inflammation (139). This study served as a proof-of-concept demonstrating that metformin may be an option as adjunctive therapy of TB. Another study evaluating the sterilizing role of metformin found no differences in Mtb burden in the metformin adjuvant group versus TB treatment alone (140). Some possible reasons for the divergent results are the different model mouses and the different TB drugs used in both studies. Singhal et al. used a single anti-TB drug (isoniazid or ethambutol), whereas Duta NK et al. employed four drugs, which may have masked a possible role of metformin in sterilization. The inclusion of rifampin may also have altered the pharmacokinetics of metformin (140). Alternatively, it may be that metformin acts in an immunomodulatory role that is more clinically relevant than sterilization. Another study with mice found that metformin enhanced the effectiveness of Mtb-specific CD8 + T cell responses in local and systemic sites during infection, with increased decreased mortality and anti-mycobacterial properties and decreased inflammatory cytokine production such as TNF (141). Furthermore, Bohme J. et al. found that metformin enhances the immunogenicity and protective efficacy of BCG in mice (142).
Prior retrospective studies in patients with type 2 diabetes and TB suggest that metformin use was beneficial during TB treatment with reduced risk of cavitary TB (139), more rapid sputum conversion (143), lower mortality despite significantly higher glycated hemoglobin values (139, 144) and lower risk of recurrence (145) when compared to the use of other diabetes treatment regimens during TB treatment.
Considering the evidence above, the drug safety profile, wide availability, and low cost, there remains an important role for CTs to evaluate the effectiveness of metformin as TB adjunctive therapy. Currently, ClinicalTrials.gov reports two CTs investigating Metformin use in individuals with pulmonary TB that are currently recruiting participants in Thailand and South Africa (Table 1).
Conclusion
Novel TB interventions beyond antimicrobials are urgently needed to improve treatment effectiveness with shorter duration and without increased adverse effects. HDTs offer a promising strategy through repurposing of pre-existing drugs, that often are widely available with low cost, and therefore easily implemented if efficacy and safety are proven in robust CTs. Currently, HDT drugs are in different stages of research with primarily pre-clinical studies with conflicting conclusions. New CTs, ideally multicenter, are underway to answer questions regarding HDT drugs in TB treatment, including all populations of clinical relevance. The existent TB networks or consortia that include standardized cohorts of patients with confirmed TB represent an opportunity for harmonized CTs with heterogenous populations and homogeneous protocols. We suggest the use of these networks or consortia to expedite quality research in this field.
Author contributions
JC-A, BN, and BB-D contributed to conception and design of the study. JC-A, BN, CF, CV, KV-S, VN, JM-P, MA-P, MA, and EA also collected the information and organized the different subsections. JC-A, BN, and BA wrote the first draft of the manuscript. BA supervised the project execution. All authors contributed to manuscript revision, read, and approved the submitted version.
Funding
This study was supported by the Intramural Research Program of the Fundação Oswaldo Cruz, Brazil. The work of BA was supported by grants from NIH (U01AI115940 and U01AI069923). BA was a senior scientist from the Conselho Nacional de Desenvolvimento Científico e Tecnológico (CNPq). JC-A was supported by the Organization of American States-Partnerships Program for Education and Training (OAS-PAEC) and his study was financed in part by the Coordenação de Aperfeiçoamento de Pessoal de Nível Superior-Brazil (CAPES)-Finance Code 001. BB-D and M-AP also received fellowship from CAPES. JM-P reveived fellowship from CNPq. MA, KV-S, and VN received research fellowships from the Fundação de Amparo à Pesquisa da Bahia (FAPESB).
Acknowledgments
We thank the study participants and Dr. Kevan Akrami for assistance with language and proofreading the manuscript.
Conflict of interest
The authors declare that the research was conducted in the absence of any commercial or financial relationships that could be construed as a potential conflict of interest.
Publisher’s note
All claims expressed in this article are solely those of the authors and do not necessarily represent those of their affiliated organizations, or those of the publisher, the editors and the reviewers. Any product that may be evaluated in this article, or claim that may be made by its manufacturer, is not guaranteed or endorsed by the publisher.
References
2. Shariq M, Sheikh JA, Quadir N, Sharma N, Hasnain SE, Ehtesham NZ. COVID-19 and tuberculosis: the double whammy of respiratory pathogens. Eur Respir Rev. (2022) 31:210264. doi: 10.1183/16000617.0264-2021
3. Antunes JL, Waldman EA. Tuberculosis in the twentieth century: time-series mortality in Sao Paulo, Brazil, 1900-97. Cad Saude Publica. (1999) 15:463–76. doi: 10.1590/s0102-311x1999000300003
4. Jung RS, Bennion JR, Sorvillo F, Bellomy A. Trends in tuberculosis mortality in the United States, 1990-2006: a population-based case-control study. Public Health Rep. (2010) 125:389–97. doi: 10.1177/003335491012500307
5. Murray JF. A century of tuberculosis. Am J Respir Crit Care Med. (2004) 169:1181–6. doi: 10.1164/rccm.200402-140OE
6. Albalak R, O’brien RJ, Kammerer JS, O’brien SM, Marks SM, Castro KG, et al. Trends in tuberculosis/human immunodeficiency virus comorbidity, United States, 1993-2004. Arch Intern Med. (2007) 167:2443–52. doi: 10.1001/archinte.167.22.2443
7. Sakamoto H, Lee S, Ishizuka A, Hinoshita E, Hori H, Ishibashi N, et al. Challenges and opportunities for eliminating tuberculosis – leveraging political momentum of the UN high-level meeting on tuberculosis. BMC Public Health. (2019) 19:76. doi: 10.1186/s12889-019-6399-8
8. Tiberi S, Munoz-Torrico M, Duarte R, Dalcolmo M, D’ambrosio L, Migliori GB. New drugs and perspectives for new anti-tuberculosis regimens. Pulmonology. (2018) 24:86–98. doi: 10.1016/j.rppnen.2017.10.009
9. De Maio F, Bianco DM, Delogu G. The dark side of the COVID-19 treatments on Mycobacterium tuberculosis infection. Mediterr J Hematol Infect Dis. (2022) 14:e2022021. doi: 10.4084/MJHID.2022.021
10. van den Boogaard J, Kibiki GS, Kisanga ER, Boeree MJ, Aarnoutse RE. New drugs against tuberculosis: problems, progress, and evaluation of agents in clinical development. Antimicrob Agents Chemother. (2009) 53:849–62. doi: 10.1128/AAC.00749-08
11. Zumla A, Rao M, Parida SK, Keshavjee S, Cassell G, Wallis R, et al. Inflammation and tuberculosis: host-directed therapies. J Intern Med. (2015) 277:373–87. doi: 10.1111/joim.12256
12. Kim JS, Kim YR, Yang CS. Host-directed therapy in tuberculosis: targeting host metabolism. Front Immunol. (2020) 11:1790. doi: 10.3389/fimmu.2020.01790
13. Ahmed S, Raqib R, Guethmundsson GH, Bergman P, Agerberth B, Rekha RS. Host-directed therapy as a novel treatment strategy to overcome tuberculosis: targeting immune modulation. Antibiotics (Basel). (2020) 9:21. doi: 10.3390/antibiotics9010021
14. Kaufmann SHE, Dorhoi A, Hotchkiss RS, Bartenschlager R. Host-directed therapies for bacterial and viral infections. Nat Rev Drug Discov. (2018) 17:35–56. doi: 10.1038/nrd.2017.162
15. Wallis RS, Hafner R. Advancing host-directed therapy for tuberculosis. Nat Rev Immunol. (2015) 15:255–63. doi: 10.1038/nri3813
16. Pai M, Behr MA, Dowdy D, Dheda K, Divangahi M, Boehme CC, et al. Tuberculosis. Nat Rev Dis Primers. (2016) 2:16076. doi: 10.1038/nrdp.2016.76
17. Jee B. Understanding the early host immune response against Mycobacterium tuberculosis. Cent Eur J Immunol. (2020) 45:99–103. doi: 10.5114/ceji.2020.94711
18. de Martino M, Lodi L, Galli L, Chiappini E. Immune response to Mycobacterium tuberculosis: a narrative review. Front Pediatr. (2019) 7:350. doi: 10.3389/fped.2019.00350
19. Abreu R, Giri P, Quinn F. Host-pathogen interaction as a novel target for host-directed therapies in tuberculosis. Front Immunol. (2020) 11:1553. doi: 10.3389/fimmu.2020.01553
20. Tsenova L, Singhal A. Effects of host-directed therapies on the pathology of tuberculosis. J Pathol. (2020) 250:636–46. doi: 10.1002/path.5407
21. Silva Miranda M, Breiman A, Allain S, Deknuydt F, Altare F. The tuberculous granuloma: an unsuccessful host defence mechanism providing a safety shelter for the bacteria? Clin Dev Immunol. (2012) 2012:139127. doi: 10.1155/2012/139127
22. Kilinc G, Saris A, Ottenhoff THM, Haks MC. Host-directed therapy to combat mycobacterial infections. Immunol Rev. (2021) 301:62–83. doi: 10.1111/imr.12951
23. Rohrig F, Vorlova S, Hoffmann H, Wartenberg M, Escorcia FE, Keller S, et al. VEGF-ablation therapy reduces drug delivery and therapeutic response in ECM-dense tumors. Oncogene. (2017) 36:1–12. doi: 10.1038/onc.2016.182
24. Schaible UE, Linnemann L, Redinger N, Patin EC, Dallenga T. Strategies to improve vaccine efficacy against tuberculosis by targeting innate immunity. Front Immunol. (2017) 8:1755. doi: 10.3389/fimmu.2017.01755
25. Torrado E, Cooper AM. IL-17 and Th17 cells in tuberculosis. Cytokine Growth Factor Rev. (2010) 21:455–62. doi: 10.1016/j.cytogfr.2010.10.004
26. Kolloli A, Subbian S. Host-directed therapeutic strategies for tuberculosis. Front Med (Lausanne). (2017) 4:171. doi: 10.3389/fmed.2017.00171
27. Young C, Walzl G, Du Plessis N. Therapeutic host-directed strategies to improve outcome in tuberculosis. Mucosal Immunol. (2020) 13:190–204. doi: 10.1038/s41385-019-0226-5
28. Melander RJ, Zurawski DV, Melander C. Narrow-spectrum antibacterial agents. Medchemcomm. (2018) 9:12–21. doi: 10.1039/C7MD00528H
29. Yang HJ, Wang D, Wen X, Weiner DM, Via LE. One size fits all? Not in in vivo modeling of tuberculosis chemotherapeutics. Front Cell Infect Microbiol. (2021) 11:613149. doi: 10.3389/fcimb.2021.613149
30. Rojas-Caraballo J, Lopez-Aban J, Perez Del Villar L, Vizcaino C, Vicente B, Fernandez-Soto P, et al. In vitro and in vivo studies for assessing the immune response and protection-inducing ability conferred by Fasciola hepatica-derived synthetic peptides containing B- and T-cell epitopes. PLoS One. (2014) 9:e105323. doi: 10.1371/journal.pone.0105323
31. Karakousis PC, Hafner R, Gennaro ML. Advances in Host-Directed Therapies Against Tuberculosis. Berlin: Springer (2021). doi: 10.1007/978-3-030-56905-1
32. Mehta S. Malnutrition and drugs: clinical implications. Dev Pharmacol Ther. (1990) 15:159–65. doi: 10.1159/000457640
33. Morgan ET. Impact of infectious and inflammatory disease on cytochrome P450-mediated drug metabolism and pharmacokinetics. Clin Pharmacol Ther. (2009) 85:434–8. doi: 10.1038/clpt.2008.302
34. Frank DJ, Horne DJ, Dutta NK, Shaku MT, Madensein R, Hawn TR, et al. Remembering the host in tuberculosis drug development. J Infect Dis. (2019) 219:1518–24. doi: 10.1093/infdis/jiy712
35. Coussens LM, Zitvogel L, Palucka AK. Neutralizing tumor-promoting chronic inflammation: a magic bullet? Science. (2013) 39(6127):1522. doi: 10.1126/science.1232227
36. Kulchavenya E. Extrapulmonary tuberculosis: are statistical reports accurate? Ther Adv Infect Dis. (2014) 2:61–70. doi: 10.1177/2049936114528173
37. Khan AH, Sulaiman SAS, Laghari M, Hassali MA, Muttalif AR, Bhatti Z, et al. Treatment outcomes and risk factors of extra-pulmonary tuberculosis in patients with co-morbidities. BMC Infect Dis. (2019) 19:691. doi: 10.1186/s12879-019-4312-9
38. Zurcher K, Ballif M, Kiertiburanakul S, Chenal H, Yotebieng M, Grinsztejn B, et al. Diagnosis and clinical outcomes of extrapulmonary tuberculosis in antiretroviral therapy programmes in low- and middle-income countries: a multicohort study. J Int AIDS Soc. (2019) 22:e25392. doi: 10.1002/jia2.25392
39. Atif M, Fatima R, Ahmad N, Babar ZU. Treatment outcomes of extrapulmonary tuberculosis in Bahawalpur, Pakistan; a record review. J Pharm Policy Pract. (2020) 13:35. doi: 10.1186/s40545-020-00227-1
40. Mayer-Barber KD, Andrade BB, Oland SD, Amaral EP, Barber DL, Gonzales J, et al. Host-directed therapy of tuberculosis based on interleukin-1 and type I interferon crosstalk. Nature. (2014) 511:99–103. doi: 10.1038/nature13489
41. Palucci I, Delogu G. Host directed therapies for tuberculosis: futures strategies for an ancient disease. Chemotherapy. (2018) 63:172–80. doi: 10.1159/000490478
42. Schror K. Aspirin and platelets: the antiplatelet action of aspirin and its role in thrombosis treatment and prophylaxis. Semin Thromb Hemost. (1997) 23:349–56. doi: 10.1055/s-2007-996108
43. Koester MC. An overview of the physiology and pharmacology of aspirin and nonsteroidal anti-inflammatory drugs. J Athl Train. (1993) 28:252–9.
44. Ornelas A, Zacharias-Millward N, Menter DG, Davis JS, Lichtenberger L, Hawke D, et al. Beyond COX-1: the effects of aspirin on platelet biology and potential mechanisms of chemoprevention. Cancer Metastasis Rev. (2017) 36:289–303. doi: 10.1007/s10555-017-9675-z
45. Marzo E, Vilaplana C, Tapia G, Diaz J, Garcia V, Cardona PJ. Damaging role of neutrophilic infiltration in a mouse model of progressive tuberculosis. Tuberculosis (Edinb). (2014) 94:55–64. doi: 10.1016/j.tube.2013.09.004
46. Lee MR, Lee MC, Chang CH, Liu CJ, Chang LY, Zhang JF, et al. Use of antiplatelet agents and survival of tuberculosis patients: a population-based cohort study. J Clin Med. (2019) 8:923. doi: 10.3390/jcm8070923
47. Kroesen VM, Rodriguez-Martinez P, Garcia E, Rosales Y, Diaz J, Martin-Cespedes M, et al. A beneficial effect of low-dose aspirin in a murine model of active tuberculosis. Front Immunol. (2018) 9:798. doi: 10.3389/fimmu.2018.00798
48. Byrne ST, Denkin SM, Zhang Y. Aspirin and ibuprofen enhance pyrazinamide treatment of murine tuberculosis. J Antimicrob Chemother. (2007) 59:313–6. doi: 10.1093/jac/dkl486
49. Schoeman J, Mansvelt E, Springer P, Van Rensburg AJ, Carlini S, Fourie E. Coagulant and fibrinolytic status in tuberculous meningitis. Pediatr Infect Dis J. (2007) 26:428–31. doi: 10.1097/01.inf.0000261126.60283.cf
50. Fox KA, Kirwan DE, Whittington AM, Krishnan N, Robertson BD, Gilman RH, et al. Platelets regulate pulmonary inflammation and tissue destruction in tuberculosis. Am J Respir Crit Care Med. (2018) 198:245–55. doi: 10.1164/rccm.201710-2102OC
51. Mai NT, Dobbs N, Phu NH, Colas RA, Thao LT, Thuong NT, et al. A randomised double blind placebo controlled phase 2 trial of adjunctive aspirin for tuberculous meningitis in HIV-uninfected adults. Elife. (2018) 7:e33478. doi: 10.7554/eLife.33478
52. Misra UK, Kalita J, Nair PP. Role of aspirin in tuberculous meningitis: a randomized open label placebo controlled trial. J Neurol Sci. (2010) 293:12–7. doi: 10.1016/j.jns.2010.03.025
53. Fundació Institut Germans Trias I Pujol. Phase 2b Randomized Double-blind, Placebo-controlled Trial to Estimate the Potential Efficacy and Safety of Two Repurposed Drugs, Acetylsalicylic Acid and Ibuprofen, for Use as Adjunct Therapy Added to, and Compared With, the Standard WHO-recommended TB Regimen (SMA-TB). [Clinical trial registration]. Clinicaltrials.Gov. (2021). Available online at: https://clinicaltrials.gov/ct2/show/NCT04575519 (accessed August 28, 2022).
54. The Aurum Institute NPC. A Prospective Randomized Controlled Trial of Adjunctive N-acetylcysteine (NAC) in Adult Patients With Pulmonary Tuberculosis: A Sub-study of TB Sequel. [Clinical trial registration]. Clinicaltrials.Gov. (2021). Available online at: https://clinicaltrials.gov/ct2/show/NCT03702738 (accessed August 28, 2022).
55. Fundação de Medicina Tropical Dr. Heitor Vieira Dourado. An Open Label Randomized Phase 2 Clinical Trial to Assess Safety and Tolerability of RIPE vs RIPE Plus N-acetylcysteine in Patients With HIV/Aids and Pulmonary Tuberculosis. [Clinical trial registration]. Clinicaltrials.Gov. (2019). Available online at: https://clinicaltrials.gov/ct2/show/NCT03281226 (accessed August 28, 2022).
56. Tamara L, Kartasasmita CB, Alam A, Gurnida DA. Effects of Vitamin D supplementation on resolution of fever and cough in children with pulmonary tuberculosis: A randomized double-blind controlled trial in Indonesia. J Glob Health. (2022) 12:04015. doi: 10.7189/jogh.12.04015
57. Hasan Z, Salahuddin N, Rao N, Aqeel M, Mahmood F, Ali F, et al. Change in serum CXCL10 levels during anti-tuberculosis treatment depends on vitamin D status [Short communication]. Int J Tuberc Lung Dis. (2014) 18:466–9. doi: 10.5588/ijtld.13.0460
58. Ralph AP, Waramori G, Pontororing GJ, Kenangalem E, Wiguna A, Tjitra E, et al. L-arginine and vitamin D adjunctive therapies in pulmonary tuberculosis: a randomised, double-blind, placebo-controlled trial. PLoS One. (2013) 8:e70032. doi: 10.1371/journal.pone.0070032
59. Shafique K. Effect of Supplementary Vitamin D in Patients With Diabetes Mellitus and Pulmonary Tuberculosis (EVIDENT Study): a Randomized, Double Blind, Controlled Trial. [Clinical trial registration]. Clinicaltrials.Gov. (2014). Available online at: https://clinicaltrials.gov/ct2/show/NCT02169570 (accessed August 28, 2022).
60. Rojas MT. Effect of Vitamin D as Adjunctive Therapy in Patients With Pulmonary Evolution Tuberculosis in the National Institute of Respiratory Diseases. [Clinical trial registration]. Clinicaltrials.Gov. (2015). Available online at: https://clinicaltrials.gov/ct2/show/NCT02464683 (accessed August 28, 2022).
61. Daley P, Jagannathan V, John KR, Sarojini J, Latha A, Vieth R, et al. Adjunctive vitamin D for treatment of active tuberculosis in India: a randomised, double-blind, placebo-controlled trial. Lancet Infect Dis. (2015) 15:528–34. doi: 10.1016/S1473-3099(15)70053-8
62. Rekha RS, Mily A, Sultana T, Haq A, Ahmed S, Mostafa Kamal SM, et al. Immune responses in the treatment of drug-sensitive pulmonary tuberculosis with phenylbutyrate and vitamin D3 as host directed therapy. BMC Infect Dis. (2018) 18:303. doi: 10.1186/s12879-018-3203-9
63. Bekele A, Gebreselassie N, Ashenafi S, Kassa E, Aseffa G, Amogne W, et al. Daily adjunctive therapy with vitamin D3 and phenylbutyrate supports clinical recovery from pulmonary tuberculosis: a randomized controlled trial in Ethiopia. J Intern Med. (2018) 284:292–306. doi: 10.1111/joim.12767
64. Indian Council of Medical Research. Role of Oral Vitamin D as an Adjunct Therapy in Category I Pulmonary Tuberculosis Along With Assessment of Immunological Parameters. (Double-blind, Randomized, Placebo-Controlled, Clinical Trial). [Clinical trial registration]. Clinicaltrials.Gov. (2011). Available online at: https://clinicaltrials.gov/ct2/show/NCT00507000 (accessed August 28, 2022).
65. Wallis RS, Ginindza S, Beattie T, Arjun N, Likoti M, Edward VA, et al. Adjunctive host-directed therapies for pulmonary tuberculosis: a prospective, open-label, phase 2, randomised controlled trial. Lancet Respir Med. (2021) 9:897–908. doi: 10.1016/S2213-2600(20)30448-3
66. Range N, Changalucha J, Krarup H, Magnussen P, Andersen AB, Friis H. The effect of multi-vitamin/mineral supplementation on mortality during treatment of pulmonary tuberculosis: a randomised two-by-two factorial trial in Mwanza. Tanzania. Br J Nutr. (2006) 95(4):762–70.
67. Martineau AR, Jolliffe DA, Greenberg L, Aloia JF, Bergman P, Dubnov-Raz G, et al. Vitamin D supplementation to prevent acute respiratory infections: individual participant data meta-analysis. Health Technol Assess. (2019) 23:1–44. doi: 10.3310/hta23020
68. Miow QH, Vallejo AF, Wang Y, Hong JM, Bai C, Teo FS, et al. Doxycycline host-directed therapy in human pulmonary tuberculosis. J Clin Invest. (2021) 131:e141895. doi: 10.1172/JCI141895
69. Semba RD, Kumwenda J, Zijlstra E, Ricks MO, Van Lettow M, Whalen C, et al. Micronutrient supplements and mortality of HIV-infected adults with pulmonary TB: a controlled clinical trial. Int J Tuberc Lung Dis. (2007) 11:854–9.
70. Lodha R, Mukherjee A, Singh V, Singh S, Friis H, Faurholt-Jepsen D, et al. Effect of micronutrient supplementation on treatment outcomes in children with intrathoracic tuberculosis: a randomized controlled trial. Am J Clin Nutr. (2014) 100:1287–97. doi: 10.3945/ajcn.113.082255
71. Thienemann DF. Double-blind, Randomized, Placebo-controlled Trial to Evaluate the Safety and Efficacy of Atorvastatin to Reduce Inflammation After Tuberculosis Treatment Completion in HIV-infected and HIV-uninfected Adults Measured by FDG-PET/CT. [Clinical trial registration]. Clinicaltrials.Gov. (2020). Available online at: https://clinicaltrials.gov/ct2/show/NCT04147286 (accessed August 28, 2022).
72. Olufemi A. Repurposing a Lipid Lowering Drug to Treat Tuberculosis: Effectiveness of Statins as Adjuvant to Treatment of Pulmonary Tuberculosis in Nigeria. [Clinical trial registration]. Clinicaltrials.Gov. (2021). Available online at: https://clinicaltrials.gov/ct2/show/NCT04721795 (accessed August 28, 2022).
73. National University Hospital, Singapore. Rosuvastatin Evaluation as a Tuberculosis Treatment Adjunct. [Clinical trial registration]. Clinicaltrials.Gov. (2020). Available online at: https://clinicaltrials.gov/ct2/show/NCT04504851 (accessed August 28, 2022).
74. Phuphuakrat A. Efficacy of Metformin for Sputum Conversion in Patients With Active Pulmonary Tuberculosis: A Randomized Controlled Trial. [Clinical trial registration]. Clinicaltrials.Gov. (2022). Available online at: https://clinicaltrials.gov/ct2/show/NCT05215990 (accessed August 28, 2022).
75. MD HK. A Prospective, Randomized Open-Label Phase II Study of the Safety and Tolerability of Metformin in Combination With Standard Antimicrobial Treatment of Pulmonary Tuberculosis in People With TB and Co-infected With HIV. [Clinical trial registration]. Clinicaltrials.Gov. (2022). Available online at: https://clinicaltrials.gov/ct2/show/NCT04930744 (accessed August 28, 2022).
76. Ivanyi J, Zumla A. Nonsteroidal antiinflammatory drugs for adjunctive tuberculosis treatment. J Infect Dis. (2013) 208:185–8. doi: 10.1093/infdis/jit153
77. Vilaplana C, Marzo E, Tapia G, Diaz J, Garcia V, Cardona PJ. Ibuprofen therapy resulted in significantly decreased tissue bacillary loads and increased survival in a new murine experimental model of active tuberculosis. J Infect Dis. (2013) 208:199–202. doi: 10.1093/infdis/jit152
78. Eisen DP, Mcbryde ES, Walduck A. Low-dose aspirin and ibuprofen’s sterilizing effects on Mycobacterium tuberculosis suggest safe new adjuvant therapies for tuberculosis. J Infect Dis. (2013) 208:1925–7. doi: 10.1093/infdis/jit476
79. Sadowska AM. N-acetylcysteine mucolysis in the management of chronic obstructive pulmonary disease. Ther Adv Respir Dis. (2012) 6:127–35. doi: 10.1177/1753465812437563
80. Safe IP, Amaral EP, Araujo-Pereira M, Lacerda MVG, Printes VS, Souza AB, et al. Adjunct N-acetylcysteine treatment in hospitalized patients with HIV-associated tuberculosis dampens the oxidative stress in peripheral blood: results from the RIPENACTB study trial. Front Immunol. (2020) 11:602589. doi: 10.3389/fimmu.2020.602589
81. Ejigu DA, Abay SM. N-acetyl cysteine as an adjunct in the treatment of tuberculosis. Tuberc Res Treat. (2020) 2020:5907839. doi: 10.1155/2020/5907839
82. Amaral EP, Conceicao EL, Costa DL, Rocha MS, Marinho JM, Cordeiro-Santos M, et al. N-acetyl-cysteine exhibits potent anti-mycobacterial activity in addition to its known anti-oxidative functions. BMC Microbiol. (2016) 16:251. doi: 10.1186/s12866-016-0872-7
83. Mahakalkar SM, Nagrale D, Gaur S, Urade C, Murhar B, Turankar A. N-acetylcysteine as an add-on to directly observed therapy short-I therapy in fresh pulmonary tuberculosis patients: a randomized, placebo-controlled, double-blinded study. Perspect Clin Res. (2017) 8:132–6. doi: 10.4103/2229-3485.210450
84. Baniasadi S, Eftekhari P, Tabarsi P, Fahimi F, Raoufy MR, Masjedi MR, et al. Protective effect of N-acetylcysteine on antituberculosis drug-induced hepatotoxicity. Eur J Gastroenterol Hepatol. (2010) 22:1235–8. doi: 10.1097/MEG.0b013e32833aa11b
85. Wei R, Christakos S. Mechanisms underlying the regulation of innate and adaptive immunity by vitamin D. Nutrients. (2015) 7:8251–60. doi: 10.3390/nu7105392
86. Selvaraj P, Harishankar M, Afsal K. Vitamin D: immuno-modulation and tuberculosis treatment. Can J Physiol Pharmacol. (2015) 93:377–84. doi: 10.1139/cjpp-2014-0386
87. Gil A, Plaza-Diaz J, Mesa MD. Vitamin D: classic and novel actions. Ann Nutr Metab. (2018) 72:87–95. doi: 10.1159/000486536
88. Adams JS, Ren S, Liu PT, Chun RF, Lagishetty V, Gombart AF, et al. Vitamin D-directed rheostatic regulation of monocyte antibacterial responses. J Immunol. (2009) 182:4289–95. doi: 10.4049/jimmunol.0803736
89. Charoenngam N, Holick MF. Immunologic effects of vitamin D on human health and disease. Nutrients. (2020) 9:12. doi: 10.3390/nu12072097
90. Wu S, Sun J. Vitamin D, vitamin D receptor, and macroautophagy in inflammation and infection. Discov Med. (2011) 11:325–35.
91. Workineh M, Mathewos B, Moges B, Gize A, Getie S, Stendahl O, et al. Vitamin D deficiency among newly diagnosed tuberculosis patients and their household contacts: a comparative cross-sectional study. Arch Public Health. (2017) 75:25. doi: 10.1186/s13690-017-0195-7
92. Nursyam EW, Amin Z, Rumende CM. The effect of vitamin D as supplementary treatment in patients with moderately advanced pulmonary tuberculous lesion. Acta Med Indones. (2006) 38:3–5.
93. Wang J, Xiong K, Wang Q, Zhao S, Liu Y, Ma A. Adjunctive vitamin A and D during pulmonary tuberculosis treatment: a randomized controlled trial with a 2 x 2 factorial design. Food Funct. (2020) 11:4672–81. doi: 10.1039/c9fo02751c
94. Mily A, Rekha RS, Kamal SM, Arifuzzaman AS, Rahim Z, Khan L, et al. Significant effects of oral phenylbutyrate and vitamin D3 adjunctive therapy in pulmonary tuberculosis: a randomized controlled trial. PLoS One. (2015) 10:e0138340. doi: 10.1371/journal.pone.0138340
95. Tukvadze N, Sanikidze E, Kipiani M, Hebbar G, Easley KA, Shenvi N, et al. High-dose vitamin D3 in adults with pulmonary tuberculosis: a double-blind randomized controlled trial. Am J Clin Nutr. (2015) 102:1059–69. doi: 10.3945/ajcn.115.113886
96. Ganmaa D, Munkhzul B, Fawzi W, Spiegelman D, Willett WC, Bayasgalan P, et al. High-dose vitamin D3 during tuberculosis treatment in Mongolia. A randomized controlled trial. Am J Respir Crit Care Med. (2017) 196:628–37. doi: 10.1164/rccm.201705-0936OC
97. Bekele A, Gebreselassie N, Ashenafi S, Kassa E, Aseffa G, Amogne W, et al. Daily adjunctive therapy with vitamin D3 and phenylbutyrate supports clinical recovery from pulmonary tuberculosis: a randomized controlled trial in Ethiopia. J Intern Med. (2018) 284:292–306. doi: 10.1111/joim.12767
98. Grobler L, Nagpal S, Sudarsanam TD, Sinclair D. Nutritional supplements for people being treated for active tuberculosis. Cochrane Database Syst Rev. (2016) 2016:CD006086. doi: 10.1002/14651858.CD006086.pub4
99. Parks WC, Shapiro SD. Matrix metalloproteinases in lung biology. Respir Res. (2001) 2:10–9. doi: 10.1186/rr33
100. Parks WC, Wilson CL, Lopez-Boado YS. Matrix metalloproteinases as modulators of inflammation and innate immunity. Nat Rev Immunol. (2004) 4:617–29. doi: 10.1038/nri1418
101. Rohlwink UK, Walker NF, Ordonez AA, Li YJ, Tucker EW, Elkington PT, et al. Matrix metalloproteinases in pulmonary and central nervous system tuberculosis-a review. Int J Mol Sci. (2019) 20:1350. doi: 10.3390/ijms20061350
102. Sabir N, Hussain T, Mangi MH, Zhao D, Zhou X. Matrix metalloproteinases: expression, regulation and role in the immunopathology of tuberculosis. Cell Prolif. (2019) 52:e12649. doi: 10.1111/cpr.12649
103. Kubler A, Luna B, Larsson C, Ammerman NC, Andrade BB, Orandle M, et al. Mycobacterium tuberculosis dysregulates MMP/TIMP balance to drive rapid cavitation and unrestrained bacterial proliferation. J Pathol. (2015) 235:431–44. doi: 10.1002/path.4432
104. Ong CW, Elkington PT, Friedland JS. Tuberculosis, pulmonary cavitation, and matrix metalloproteinases. Am J Respir Crit Care Med. (2014) 190:9–18. doi: 10.1164/rccm.201311-2106PP
105. Salgame P. MMPs in tuberculosis: granuloma creators and tissue destroyers. J Clin Invest. (2011) 121:1686–8. doi: 10.1172/JCI57423
106. Matsuura E, Umehara F, Hashiguchi T, Fujimoto N, Okada Y, Osame M. Marked increase of matrix metalloproteinase 9 in cerebrospinal fluid of patients with fungal or tuberculous meningoencephalitis. J Neurol Sci. (2000) 173:45–52. doi: 10.1016/S0022-510X(99)00303-2
107. Price NM, Farrar J, Tran TT, Nguyen TH, Tran TH, Friedland JS. Identification of a matrix-degrading phenotype in human tuberculosis in vitro and in vivo. J Immunol. (2001) 166:4223–30. doi: 10.4049/jimmunol.166.6.4223
108. Lee KY, Kim EH, Yang WS, Ryu H, Cho SN, Lee BI, et al. Persistent increase of matrix metalloproteinases in cerebrospinal fluid of tuberculous meningitis. J Neurol Sci. (2004) 220:73–8. doi: 10.1016/j.jns.2004.02.008
109. Miow QH, Vallejo AF, Wang Y, Hong JM, Bai C, Teo FS, et al. Doxycycline host-directed therapy in human pulmonary tuberculosis. J Clin Invest. (2021) 131:e141895. doi: 10.1172/JCI141895
110. Walker NF, Clark SO, Oni T, Andreu N, Tezera L, Singh S, et al. Doxycycline and HIV infection suppress tuberculosis-induced matrix metalloproteinases. Am J Respir Crit Care Med. (2012) 185:989–97. doi: 10.1164/rccm.201110-1769OC
111. Ugarte-Gil CA, Elkington P, Gilman RH, Coronel J, Tezera LB, Bernabe-Ortiz A, et al. Induced sputum MMP-1, –3 & –8 concentrations during treatment of tuberculosis. PLoS One. (2013) 8:e61333. doi: 10.1371/journal.pone.0061333
112. Alsaad N, Wilffert B, Van Altena R, De Lange WC, Van Der Werf TS, Kosterink JG, et al. Potential antimicrobial agents for the treatment of multidrug-resistant tuberculosis. Eur Respir J. (2014) 43:884–97. doi: 10.1183/09031936.00113713
113. Wiseman EM, Bar-El Dadon S, Reifen R. The vicious cycle of vitamin a deficiency: a review. Crit Rev Food Sci Nutr. (2017) 57:3703–14. doi: 10.1080/10408398.2016.1160362
114. Cabrera Andrade BK, Garcia-Perdomo HA. Effectiveness of micronutrients supplement in patients with active tuberculosis on treatment: systematic review/meta-analysis. Complement Ther Med. (2020) 48:102268. doi: 10.1016/j.ctim.2019.102268
115. Karyadi E, West CE, Schultink W, Nelwan RH, Gross R, Amin Z, et al. A double-blind, placebo-controlled study of vitamin A and zinc supplementation in persons with tuberculosis in Indonesia: effects on clinical response and nutritional status. Am J Clin Nutr. (2002) 75:720–7. doi: 10.1093/ajcn/75.4.720
116. Range N, Changalucha J, Krarup H, Magnussen P, Andersen AB, Friis H. The effect of multi-vitamin/mineral supplementation on mortality during treatment of pulmonary tuberculosis: a randomised two-by-two factorial trial in Mwanza, Tanzania. Br J Nutr. (2006) 95:762–70. doi: 10.1079/bjn20051684
117. Lodha R, Mukherjee A, Singh V, Singh S, Friis H, Faurholt-Jepsen D, et al. Effect of micronutrient supplementation on treatment outcomes in children with intrathoracic tuberculosis: a randomized controlled trial. Am J Clin Nutr. (2014) 100:1287–97. doi: 10.3945/ajcn.113.082255
118. Brzostek A, Pawelczyk J, Rumijowska-Galewicz A, Dziadek B, Dziadek J. Mycobacterium tuberculosis is able to accumulate and utilize cholesterol. J Bacteriol. (2009) 191:6584–91. doi: 10.1128/JB.00488-09
119. Skerry C, Pinn ML, Bruiners N, Pine R, Gennaro ML, Karakousis PC. Simvastatin increases the in vivo activity of the first-line tuberculosis regimen. J Antimicrob Chemother. (2014) 69:2453–7. doi: 10.1093/jac/dku166
120. Tahir F, Bin Arif T, Ahmed J, Shah SR, Khalid M. Anti-tuberculous effects of statin therapy: a review of literature. Cureus. (2020) 12:e7404. doi: 10.7759/cureus.7404
121. Shim D, Kim H, Shin SJ. Mycobacterium tuberculosis infection-driven foamy macrophages and their implications in tuberculosis control as targets for host-directed therapy. Front Immunol. (2020) 11:910. doi: 10.3389/fimmu.2020.00910
122. Parihar SP, Guler R, Khutlang R, Lang DM, Hurdayal R, Mhlanga MM, et al. Statin therapy reduces the Mycobacterium tuberculosis burden in human macrophages and in mice by enhancing autophagy and phagosome maturation. J Infect Dis. (2014) 209:754–63. doi: 10.1093/infdis/jit550
123. Pandey AK, Sassetti CM. Mycobacterial persistence requires the utilization of host cholesterol. Proc Natl Acad Sci U.S.A. (2008) 105:4376–80. doi: 10.1073/pnas.0711159105
124. Guerra-De-Blas PDC, Bobadilla-Del-Valle M, Sada-Ovalle I, Estrada-Garcia I, Torres-Gonzalez P, Lopez-Saavedra A, et al. Simvastatin enhances the immune response against Mycobacterium tuberculosis. Front Microbiol. (2019) 10:2097. doi: 10.3389/fmicb.2019.02097
125. Rodrigues Diez R, Rodrigues-Diez R, Lavoz C, Rayego-Mateos S, Civantos E, Rodriguez-Vita J, et al. Statins inhibit angiotensin II/Smad pathway and related vascular fibrosis, by a TGF-beta-independent process. PLoS One. (2010) 5:e14145. doi: 10.1371/journal.pone.0014145
126. Ma YX, Li WH, Xie Q. Rosuvastatin inhibits TGF-beta1 expression and alleviates myocardial fibrosis in diabetic rats. Pharmazie. (2013) 68:355–8.
127. Lobato LS, Rosa PS, Ferreira Jda S, Neumann Ada S, Da Silva MG, Do Nascimento DC, et al. Statins increase rifampin mycobactericidal effect. Antimicrob Agents Chemother. (2014) 58:5766–74. doi: 10.1128/AAC.01826-13
128. Dutta NK, Bruiners N, Pinn ML, Zimmerman MD, Prideaux B, Dartois V, et al. Statin adjunctive therapy shortens the duration of TB treatment in mice. J Antimicrob Chemother. (2016) 71:1570–7. doi: 10.1093/jac/dkw014
129. Su VY, Su WJ, Yen YF, Pan SW, Chuang PH, Feng JY, et al. Statin use is associated with a lower risk of TB. Chest. (2017) 152:598–606. doi: 10.1016/j.chest.2017.04.170
130. Lai CC, Lee MT, Lee SH, Hsu WT, Chang SS, Chen SC, et al. Statin treatment is associated with a decreased risk of active tuberculosis: an analysis of a nationally representative cohort. Thorax. (2016) 71:646–51. doi: 10.1136/thoraxjnl-2015-207052
131. Liao KF, Lin CL, Lai SW. Population-based case-control study assessing the association between statins use and pulmonary tuberculosis in Taiwan. Front Pharmacol. (2017) 8:597. doi: 10.3389/fphar.2017.00597
132. Lee MY, Lin KD, Hsu WH, Chang HL, Yang YH, Hsiao PJ, et al. Statin, calcium channel blocker and beta blocker therapy may decrease the incidence of tuberculosis infection in elderly Taiwanese patients with type 2 diabetes. Int J Mol Sci. (2015) 16:11369–84. doi: 10.3390/ijms160511369
133. Yeh JJ, Lin CL, Hsu CY, Shae Z, Kao CH. Statin for tuberculosis and pneumonia in patients with asthma(-)chronic pulmonary disease overlap syndrome: a time-dependent population-based cohort study. J Clin Med. (2018) 7:381. doi: 10.3390/jcm7110381
134. Pan SW, Yen YF, Feng JY, Chuang PH, Su VY, Kou YR, et al. Opposite effects of statins on the risk of tuberculosis and herpes zoster in patients with diabetes: a population-based cohort study. Br J Clin Pharmacol. (2020) 86:569–79. doi: 10.1111/bcp.14142
135. Li X, Sheng L, Lou L. Statin use may be associated with reduced active tuberculosis infection: a meta-analysis of observational studies. Front Med (Lausanne). (2020) 7:121. doi: 10.3389/fmed.2020.00121
136. Salber GJ, Wang YB, Lynch JT, Pasquale KM, Rajan TV, Stevens RG, et al. Metformin use in practice: compliance with guidelines for patients with diabetes and preserved renal function. Clin Diabetes. (2017) 35:154–61. doi: 10.2337/cd15-0045
137. Restrepo BI. Metformin: candidate host-directed therapy for tuberculosis in diabetes and non-diabetes patients. Tuberculosis (Edinb). (2016) 101S:S69–72. doi: 10.1016/j.tube.2016.09.008
138. Viollet B, Andreelli F. AMP-activated protein kinase and metabolic control. Handb Exp Pharmacol. (2011) 203:303–30. doi: 10.1007/978-3-642-17214-4_13
139. Singhal A, Jie L, Kumar P, Hong GS, Leow MK, Paleja B, et al. Metformin as adjunct antituberculosis therapy. Sci Transl Med. (2014) 6:263ra159. doi: 10.1126/scitranslmed.3009885
140. Dutta NK, Pinn ML, Karakousis PC. Metformin adjunctive therapy does not improve the sterilizing activity of the first-line antitubercular regimen in mice. Antimicrob Agents Chemother. (2017) 61:e00652–17. doi: 10.1128/AAC.00652-17
141. Russell SL, Lamprecht DA, Mandizvo T, Jones TT, Naidoo V, Addicott KW, et al. Compromised metabolic reprogramming is an early indicator of CD8(+) T cell dysfunction during chronic Mycobacterium tuberculosis infection. Cell Rep. (2019) 29:3564–79.e3565. doi: 10.1016/j.celrep.2019.11.034
142. Bohme J, Martinez N, Li S, Lee A, Marzuki M, Tizazu AM, et al. Metformin enhances anti-mycobacterial responses by educating CD8+ T-cell immunometabolic circuits. Nat Commun. (2020) 11:5225. doi: 10.1038/s41467-020-19095-z
143. Lee YJ, Han SK, Park JH, Lee JK, Kim DK, Chung HS, et al. The effect of metformin on culture conversion in tuberculosis patients with diabetes mellitus. Korean J Intern Med. (2018) 33:933–40. doi: 10.3904/kjim.2017.249
144. Degner NR, Wang JY, Golub JE, Karakousis PC. Metformin use reverses the increased mortality associated with diabetes mellitus during tuberculosis treatment. Clin Infect Dis. (2018) 66:198–205. doi: 10.1093/cid/cix819
Keywords: host-directed therapy, Mycobacterium, tuberculosis, adjunct therapy, immunotherapies
Citation: Cubillos-Angulo JM, Nogueira BMF, Arriaga MB, Barreto-Duarte B, Araújo-Pereira M, Fernandes CD, Vinhaes CL, Villalva-Serra K, Nunes VM, Miguez-Pinto JP, Amaral EP and Andrade BB (2022) Host-directed therapies in pulmonary tuberculosis: Updates on anti-inflammatory drugs. Front. Med. 9:970408. doi: 10.3389/fmed.2022.970408
Received: 15 June 2022; Accepted: 22 August 2022;
Published: 23 September 2022.
Edited by:
Mohammad Asif Sherwani, University of Alabama at Birmingham, United StatesReviewed by:
Shashank Gupta, Division of Intramural Research, National Heart, Lung, and Blood Institute (NIH), United StatesFaraz Ahmad, University of Missouri, United States
Copyright © 2022 Cubillos-Angulo, Nogueira, Arriaga, Barreto-Duarte, Araújo-Pereira, Fernandes, Vinhaes, Villalva-Serra, Nunes, Miguez-Pinto, Amaral and Andrade. This is an open-access article distributed under the terms of the Creative Commons Attribution License (CC BY). The use, distribution or reproduction in other forums is permitted, provided the original author(s) and the copyright owner(s) are credited and that the original publication in this journal is cited, in accordance with accepted academic practice. No use, distribution or reproduction is permitted which does not comply with these terms.
*Correspondence: Bruno B. Andrade, YnJ1bm8uYW5kcmFkZUBmaW9jcnV6LmJy
†These authors have contributed equally to this work