- 1CHRU Nancy, Service de Médecine Intensive et Réanimation, Hôpital Brabois, Vandœuvre-lès-Nancy, France
- 2INSERM U 1116, Groupe Choc, Équipe 2, Faculté de Médecine, Vandœuvre-lès-Nancy, France
- 3Université de Lorraine, Faculté de Médecine, Nancy, France
- 4Service de Médecine Intensive - Réanimation, Hôpital de la Croix-Rousse, Hospices Civils de Lyon, Lyon, France
- 5Université de Lyon, Université Claude Bernard Lyon 1, Lyon, France
- 6CHRU de Nancy, Département D'Anatomie Pathologique, Laboratoires de Biologie Médicale et de Biopathologie, Hôpital Brabois, Vandœuvre-lès-Nancy, France
- 7Ecole de Chirurgie, Faculté de Médecine, Université de Lorraine, Nancy, France
- 8Université Paris XI, Institut de Cardiologie, Groupe Hospitalier Pitié-Salpêtrière, Paris, France
Background: The current standard of care during severe acute respiratory distress syndrome (ARDS) is based on low tidal volume (VT) ventilation, at 6 mL/kg of predicted body weight. The time-controlled adaptive ventilation (TCAV) is an alternative strategy, based on specific settings of the airway pressure release ventilation (APRV) mode. Briefly, TCAV reduces lung injury, including: (1) an improvement in alveolar recruitment and homogeneity; (2) reduction in alveolar and alveolar duct micro-strain and stress-risers. TCAV can result in higher intra-thoracic pressures and thus impair hemodynamics resulting from heart-lung interactions. The objective of our study was to compare hemodynamics between TCAV and conventional protective ventilation in a porcine ARDS model.
Methods: In 10 pigs (63–73 kg), lung injury was induced by repeated bronchial saline lavages followed by 2 h of injurious ventilation. The animals were then randomized into two groups: (1) Conventional protective ventilation with a VT of 6 mL/kg and PEEP adjusted to a plateau pressure set between 28 and 30 cmH2O; (2) TCAV group with P-high set between 27 and 29 cmH2O, P-low at 0 cmH2O, T-low adjusted to terminate at 75% of the expiratory flow peak, and T-high at 3–4 s, with I:E > 6:1.
Results: Both lung elastance and PaO2:FiO2 were consistent with severe ARDS after 2 h of injurious mechanical ventilation. There was no significant difference in systemic arterial blood pressure, pulmonary blood pressure or cardiac output between Conventional protective ventilation and TCAV. Levels of total PEEP were significantly higher in the TCAV group (p < 0.05). Driving pressure and lung elastance were significantly lower in the TCAV group (p < 0.05).
Conclusion: No hemodynamic adverse events were observed in the TCAV group compared as to the standard protective ventilation group in this swine ARDS model, and TCAV appeared to be beneficial to the respiratory system.
Introduction
Acute Respiratory Distress Syndrome (ARDS) is a life-threatening condition due to a lung injury that can result from numerous causes (e.g., infectious, toxic, or inflammatory). Its mortality raises up to 50% in the most severe cases (1).
ARDS treatment is based on protective mechanical ventilation, prone positioning, neuromuscular blockade or VV-ECMO (2). The current standard of care is based on the limitation of ventilator-induced lung injury (VILI) by reducing the insufflated tidal volume (VT) to 6 mL/kg of predicted body weight (PBW) and by maintaining driving pressure (ΔP) below 15 cmH2O (3, 4). As positive end expiratory pressure (PEEP) can provide both lung recruitment and overdistension, it can lead to an increase in pulmonary blood pressure (PBP) (5, 6). An alternative strategy is the time-controlled adaptive ventilation (TCAV), a specific combination of settings applied to set the airway pressure release ventilation (APRV) mode. Initially reported by Habashi et al., TCAV reduces lung injury in both experimental and clinical studies (7–9). TCAV is based on delivering a continuous inspiratory positive airway pressure (CPAP) phase (Phigh), followed by a brief expiratory release phase (Tlow) (10).
A significant concern is the hemodynamic effect of an increase in intrathoracic pressure leading to a decrease in cardiac output (6, 11). Our hypothesis is that TCAV, that results in higher intra-thoracic pressures due to the prolonged inspiratory phase, can lead to harmful heart-lung interactions. The main objective of our study was to compare hemodynamics during the first hour of TCAV or conventional protective ventilation in a porcine ARDS model.
Methods
The present study was conducted in accordance with the ARRIVE consensus guideline for reporting animal experimental studies (12).
Ethics
All experiments were reviewed and approved by the Nancy University Ethics Committee for Animal Experimentation (APAFIS Number 2020082407561244). The procedure for the care and sacrifice of the study animals was in accordance with the European Community Standards on the Care and Use of Laboratory Animals.
Animal Preparation
Animals were fasted overnight with free access to water. All the pigs were of male sex with a median weight of 67 kilograms. Intramuscular premedication was performed with ketamine (1.5 mg/kg, Warner Lambert, Nordic, AB Solna, Sweden) before transportation to the experiment facility. Sedation was deepened with propofol (2.5 mg/kg, B. Braun, Melsungen, Germany) via an ear vein cannula. After being placed in a supine position, animals were intubated with a 7.5-mm internal diameter endotracheal tube (ETT). Anesthesia was maintained with a continuous infusion of midazolam 5 mg/h and sufentanyl 20 μg/h. Depth of anesthesia was assessed regularly by checking on movements and hemodynamic response to a painful stimulus. Muscle paralysis was then maintained with a continuous infusion of cisatracurium (0.5 mg/kg/h) (GlaxoSmithKline, Marly-le-Roi, France) throughout the experiment. Pigs were connected to the ventilator (Dräger Evita Infinity V500, Lübeck, Germany), with the baseline settings adjusted to the following levels: VT,7 mL/kg; respiratory rate (RR), 22 breaths/min; PEEP, 5 cmH2O; fraction of inspired oxygen (FiO2),100%. Automatic tube compensation (ATC) was adjusted to 100%. The ventilator settings were then adjusted to pH > 7.35 and PaCO2 between 40 and 45 mmHg.
Hemodynamic Monitoring
Measurements were performed at the following successive periods: after intubation and catheters placement at basal state (TB), after ARDS induction with saline lavages and injurious mechanical ventilation (T0), and at 15 min (T15) and 60 min (T60) following randomization to either conventional protective ventilation or TCAV (Supplementary Figure 1). A pulmonary artery catheter (Swan-Ganz, Edwards Lifesciences, Irvine, USA) was inserted via the left internal jugular vein for measuring PBP, pulmonary artery wedge pressure (PAWP), right atrial pressure (RAP) and mixed venous oxygen saturation (SVO2). The pressure transducer was positioned at the level of the right atrium. A conductance catheter (Transonic Systems Inc., Ithaca, USA) was inserted into the left ventricle via the left carotid artery for simultaneous registration of both instantaneous high-fidelity left ventricular pressure (PLV) and instantaneous left ventricular volume. Central aortic pressure (ABP) was assessed by a high-fidelity pressure catheter (HIFI) (Transonic Systems Inc., Ithaca, USA) percutaneously inserted via the femoral artery into the descending thoracic aorta. The catheters were inserted under fluoroscopy. The right carotid artery was dissected, and a Transit Time Flow probe (Transonic Systems Inc., Ithaca, USA) was secured around it. Data were computed using a designated analysis program (IOX 2.4.2.6®, EMKA Technologies, France). The signals were recorded continuously at a sampling rate of 2,000 Hz. A period of 2 h was required for the calibration and the correct positioning of the probes, assessed by fluoroscopy and chest X Ray. The core body temperature was measured via a rectal probe and maintained between 37 and 38° by a warming blanket system.
Respiratory Monitoring
Airway pressure (Paw) was continuously registered by a probe set on the ventilator Y-piece. The esophageal pressure (Pes) was assessed by an esophageal balloon (BA-A-008 probe, MBMed, Argentina) positioned with fluoroscopy and inflated up to 4 mL. The correct positioning of the devices was checked by using the Baydur manoeuver (13). Transpulmonary pressure (PL) was calculated in absolute value, as follows: PL = Paw – Pes. ΔPL is defined as the difference between PLend−insp and PLend−exp. The absolute value of PL reflects the local pressure in the dependent lung regions, adjacent to the esophageal balloon, independently of the mediastinal structures (14). Elastance of the respiratory system (ElRS) was assessed by: ElRS = ΔPaw/VT. The elastance ratio (ER) was calculated as follows: ER = ElL/ElRS, i.e., the lung elastance (ElL) to total respiratory system elastance ratio (15). Inspiratory transpulmonary pressure based on elastance ratio (PLEr) reflects the local pressure in the non-dependent lung regions (16). It was calculated as follows: PLEr = Paw x ER. End inspiratory and end expiratory PL were measured after a 5-s airway occlusion of the ventilator circuitry. Data were computed using a designated analysis program with sampling rate of 2,000 Hz (IOX 2.4.2.6®, EMKA Technologies, France). In TCAV, total PEEP was measured during a 5-s occlusion period at the end of expiration.
End-tidal carbon dioxide (EtCO2) was monitored for assessing the PaCO2-EtCO2 gradient and estimate the physiologic dead space as described by Enghoff's modification of the Bohr equation: = where VD is the dead space volume (mL), VT is tidal volume (mL), EtCO2 is the end tidal expiratory CO2 (mmHg), and PaCO2 (mmHg) is the systemic arterial CO2 pressure (17).
Electrical Impedance Tomography
An electrical impedance tomography (EIT) electrode belt, which carries 16 electrodes with an inter-electrode distance of 40 mm, was placed around the thorax in the fifth intercostal space, and one reference electrode was placed on the animal's abdomen (PulmoVista 500, Dräger Medical, Lübeck, Germany). The measures of EIT were averaged over five respiratory cycles and the images were divided into four regions of interest (ROI): ROI 1 being the most ventral, to ROI 4, being the most dorsal. Results are expressed as the percentage of total tidal volume ventilation in the four ROIs (18, 19). The regional compliance was calculated in the four ROIs as follows: expressed in mL/cmH2O.
ARDS Induction
Induction of a double hit lung injury was performed by 4 repeated lung lavages for a total of 30 mL/kg warm 0.9% saline solution intratracheally at 38.5°C. The lung was filled up to the endotracheal tube and fluid was drawn from the airways after 2 min via a tracheal aspiration. During the bronchoalveolar lavage, all the animals developed a profound desaturation with SpO2 < 60% without any bradycardia or life-threatening hemodynamic alteration. This was followed by 2 h of injurious ventilation with PEEP 0 cmH2O and inspiratory pressure of 40 cmH2O, RR 10/min, inspiratory to expiratory time ratio (I:E) of 1:1 (20). The FiO2 was set at 1.0, providing an additional mechanism of lung injury (21). Of note, mechanical power of mechanical ventilation transferred to the respiratory system was estimated at 41 J/min, by applying the equation proposed by Louis et al. (22). The animals received a continuous intravenous infusion of normal saline at 10 mL/Kg/h during lung injury induction, and 2 mL/Kg/h during the study period.
Interventions and Study Groups
After the induction of ARDS, animals were randomly allocated to one of the following two groups:
• Conventional protective group (n = 5): with VT 6 mL/kg, PEEP adjusted to reach a plateau pressure of 28 to 30 cmH2O, RR 25 bpm, I:E 1:2.
• TCAV group (n = 5): Phigh set between 27 and 29 cmH2O, Plow at 0 cmH2O, Tlow set to terminate at 75% of the expiratory flow peak, Thigh at 3–4 s, and I:E > 6:1.
Statistical Analyses
Given the small sample size, all results are expressed as median and interquartile range (IQR). Baseline and T0 measurements were compared by using the non-parametric Friedman test for analysis of variance by ranks. Respiratory and hemodynamics values between the two groups at T0, T15, and T60 were compared by using mixed effects regression models for evaluating the association of variables of interest (fixed effects) with the dependent variable, using the animal number as random effect to account for the repetition of regional measurements in each animal, and the lung level as a random slope. Multicollinearity and interactions were systematically evaluated in multivariate models; in the case of a significant interaction, a post-hoc analysis using pairwise comparison adjusted for the repetition of statistical tests was performed using the Tukey method. In the case of post-hoc multiple comparisons to a single reference level, we used the Dunnett adjustment method. All statistical analyses were with a significance level of 0.05 and performed using R version 4.0.1 for MacOS® (https://www.r-project.org/, accessed March 2020).
Results
Effect of Experimental ARDS on Respiratory Mechanics and Hemodynamics
Thirteen pigs were involved in the experiment. Ten pigs were included into the final analyses. Two pigs developed an early hemorrhagic shock, and one pig developed a refractory ventricular fibrillation at the time of the left ventricular catheter insertion before randomization.
Respiratory and hemodynamic parameters at baseline and after ARDS induction are summarized in Supplementary Table 1. At T0 (after ARDS induction) both ElL [32 cmH2O/L (29–33)] and PaO2/FiO2 ratio [99 (88–115)] were consistent with a severe ARDS.
Effect of Ventilation Strategies on Hemodynamics
All the results related to hemodynamics are presented in Table 1 and Supplementary Table 2. There were no significant between group differences at T0 for the main hemodynamic parameters: heart rate (HR), cardiac output (CO), ABP, PBP, RAP, and pulmonary vascular resistance (PVR). The only significant difference was observed for the left ventricle (LV) isovolumic relaxation time constant (Tau) and LV maximal rate of pressure rise (LV + dP/dtmax) values, which reached higher levels in the conventional protective ventilation population at T0 but also at T15 and T60 (p < 0.05, no interaction was detected in multivariate analysis). There was no between-group difference at T60 for HR, CO, ABP, PBP, RAP and PVR. There was no between group difference in lactate values at T60 between the TCAV group [1.1 mmol/L (1.0–2.1)] vs. 1.5 (1.5–1.7) in the conventional protective group (p = 0.06).
Polygraphic recordings between two groups at T60 of the main hemodynamic and respiratory outcomes are presented in Figure 1.
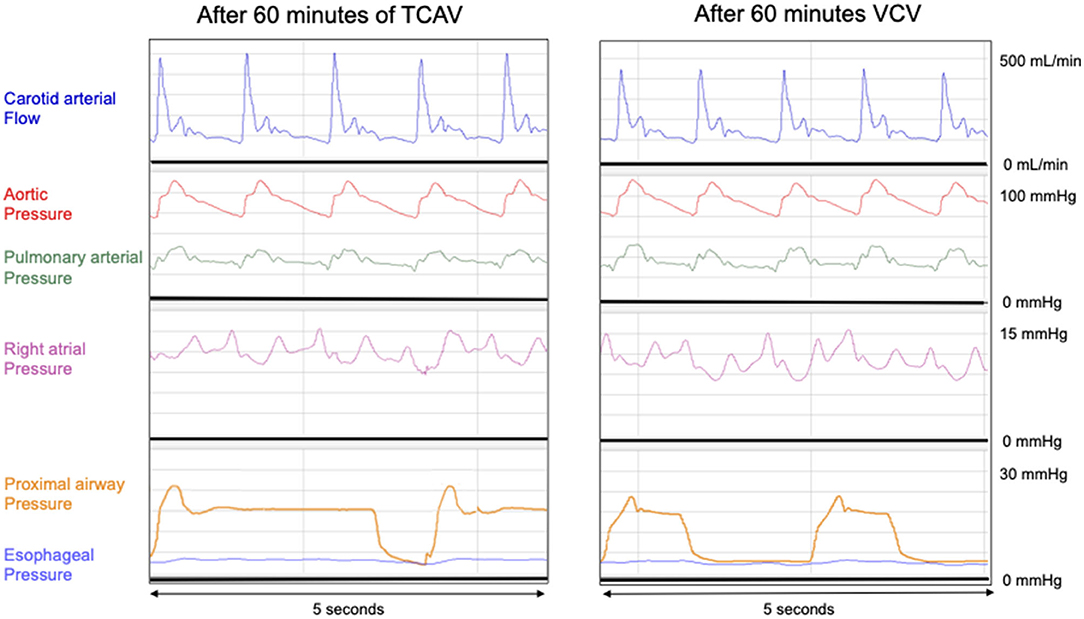
Figure 1. Polygraphic recordings between two groups at T60 of the main hemodynamic and respiratory outcomes. VCV: Conventional protective group with VT 6 ml.kg−1, PEEP 10 cmH2O, RR 25 bpm, I:E 1:2. TCAV: Phigh 27 cmH2O, Plow at 0 cmH2O, Tlow 0.4 s, Thigh 4.
Effects of the Ventilation Strategies on Respiratory Mechanics
All the results related to respiratory parameters are presented in Table 2 and Supplementary Table 3. There were no significant differences in the respiratory parameters between the TCAV and conventional protective groups at T0 except for pH (p < 0.05).
Respiratory rate was significantly lower at T60 in the TCAV group compared to the conventional protective group (p < 0.05). Levels of total PEEP were significantly higher in the TCAV group at T60 (p < 0.05). Mean airway pressure was significantly higher in the TCAV group at T15 and T60 (p < 0.05). The ΔPaw was significantly lower in the TCAV group at T15 and T60 (p < 0.05). VT in the TCAV group significantly differed from conventional protective group at T60: 7.4 mL/kg (6.4–7.8) in the TCAV group vs. 6.1 mL/kg (5.8–6.2) in the conventional protective group (p < 0.05). Elastance of the lung at T15 and T60 was significantly lower in the TCAV group (p < 0.05). PaO2/FiO2 increased in both groups at T60 without significant differences between the two groups. During the study period PaCO2 did not differ significantly. The regional compliance in the mid-ventral and mid-dorsal regions (RCROI 2 and RCROI 3) was significantly higher at T60 in the TCAV group (p < 0.05) (Supplementary Table 4).
Fluid Loading and Vasopressors
The total fluid loading was of 1,675 mL (1,650–1,825) in the TCAV group and of 1,660 ml (1,570–1,830) in the VCV group (p = 0.3) and no norepinephrine was infused during the study period (Table 2).
Discussion
The main result of the present study is that TCAV did not significantly impact hemodynamics, despite the increase in intrathoracic pressures. Additionally, TCAV improved the lung elastance after only 1 h of ventilation.
ARDS Model
Saline lavages followed by 2 h of injurious mechanical ventilation is a well-established model for inducing ARDS. It provides a highly reproducible and significant homogenous alteration of the PaO2/FiO2, ElL, and the dead space volume. ER was 0.8 after ARDS induction, indicating specific lung involvement for ElRS alteration without chest wall participation (16). This method provided a triple-hit lung injury: saline lavages leads to surfactant depletion, 100% oxygen delivery can lead to denitrogenation and injurious ventilation provides both barotrauma and volotrauma (23).
Hemodynamic Assessment of TCAV
In our work, TCAV was not associated with a hemodynamic impairment compared to standard ventilation. Regarding the right ventricular function, there were no elements suggestive of right ventricle failure, as right atrial pressure values remained low in both groups and the cardiac output was stable during the study period. Even if higher intrathoracic pressures can impair hemodynamics, changes in lung physiology can have beneficial consequences on the right ventricle and thus on hemodynamics. As pulmonary vascular resistance relates to lung volume, higher intrathoracic pressures could be in fact associated with an increase in FRC and thus a reduction in PVR (24). Sharpey-Shafer et al. reported in 1965 that a “square wave” response of the arterial pressure to the Valsalva maneuver was observed in the case of inferior vena cava (IVC) maximal repletion (25). Conversely, under hypovolemic conditions, increased mean thoracic pressure could induce the compressive occlusion of the IVC at its distal portion, at the junction with the right atrium, and lead to an acute cardiovascular collapse (26). Sympatho-vagal tone drives tolerance for acute intra thoracic pressure variation as it provides immediate inotropic, lusitropic and chronotropic adaptation (27).
Regarding the LV function, LV + dP/dtmax and shortened LV relaxation duration were observed in the conventional protective group, which can be explained by both higher ΔPL in relation to probable overdistention and more marked sympathetic stress in this group. In line with the above-mentioned literature, our results suggest that TCAV might be safe assuming the IVC repletion. Further studies are needed to assess hemodynamic safety underlying increased mean thoracic pressures during prolonged periods of ventilation.
These results are in line with data from an existing animal sepsis model, with a less robust cardiac assessment, in which TCAV was safe compared with low tidal volume ventilation, in terms of CO and MAP. Further studies are needed to evaluate TCAV in other injury models (28).
Respiratory Assessment of TCAV
The higher mean airway pressure and the lower respiratory rate observed in the TCAV group compared to the conventional protective group are explained by a longer I/E ratio, which is one of the fundamental characteristics of TCAV. Total PEEP was also higher, in relation with the decrease in ΔPaw and improvement in ElL. Tidal volume delivered in the TCAV group was closely monitored and averaged 7 mL/kg as Tlow was adjusted to terminate at 75% of PEFR, in order to prevent alveolar collapse (7). PLEr provides indirect information about overdistension in the non-dependent lung areas and was lower at T60 in the TCAV group. TCAV significantly improved ΔPaw and EIT regional compliance at T60.This can be explained by a gain in aerated lung tissue volume. There were no differences between the two groups regarding both PaCO2 and pH values. Our results are in line with the literature, suggesting benefits of TCAV in terms of lung protective ventilation (12, 29).
Study Limitations
One of the limitations of our study lies in the small sample size of each study group. The study might have been underpowered in its attempt to assess a clinically relevant effect of TCAV on hemodynamics. It is worth mentioning that dorsal decubitus is poorly tolerated in pigs and involves important modifications in both “West physiology” and hemodynamics that could mitigate external validation of the present results. Improvement in pulmonary elastance in the TCAV group can be in relation with higher levels of total PEEP and mean airway pressure. Furthermore, it could be suggestive of alveolar recruitment, but we did not perform any CT scan in order to verify this hypothesis, especially with the use of an recruitable ARDS (29, 30). In our work, the right ventricular function was assessed only with measures obtained with a pulmonary arterial catheter, as placement of the conductance catheter in the right ventricle and transthoracic echocardiography in pigs was not feasible in our study setting. The addition of paralysis may not fully encompass the hemodynamics associated with either ventilator mode as it does not incorporate the hemodynamic and respiratory effects of spontaneous breathing (28). To finish, this study was designed with only a 1-h ventilation period to observe the safety of initiation of TCAV on heart-lung interactions, limiting the evaluation of a longer period of TCAV on lung mechanics (31).
Conclusion
In conclusion, no hemodynamic adverse events were observed with TCAV compared to standard protective ventilation in this swine ARDS model, as TCAV appeared to be beneficial for the respiratory system.
Data Availability Statement
The original contributions presented in the study are included in the article/Supplementary Material, further inquiries can be directed to the corresponding author.
Ethics Statement
The animal study protocol was approved by Nancy University Ethics Committee for Animal Experimentation (APAFIS Number 2020082407561244).
Author Contributions
ML, BP, BL, MK, HP, and N'GT contributed to conception and design of the study. N'GT organized the study. BP and ML wrote the first draft of the manuscript. LB, J-LH, J-CR and MK wrote sections of the manuscript. All authors contributed to manuscript revision, read, and approved the submitted version.
Funding
This research was funded by INSERM 1116, Région Grand Est, and FEDER.
Conflict of Interest
The authors declare that the research was conducted in the absence of any commercial or financial relationships that could be construed as a potential conflict of interest.
Publisher's Note
All claims expressed in this article are solely those of the authors and do not necessarily represent those of their affiliated organizations, or those of the publisher, the editors and the reviewers. Any product that may be evaluated in this article, or claim that may be made by its manufacturer, is not guaranteed or endorsed by the publisher.
Acknowledgments
Pulmovista were furnished by Draguer. Draguer was not involved in the study design, collection, analysis, interpretation of data, the writing of this article or the decision to submit it for publication.
Supplementary Material
The Supplementary Material for this article can be found online at: https://www.frontiersin.org/articles/10.3389/fmed.2022.883950/full#supplementary-material
Abbreviations
APRV, Airway pressure release ventilation; ARDS, Acute respiratory distress syndrome; ATC, Automatic tube compensation; CO, Cardiac output; CPAP, Continuous positive airway pressure; CRS, Compliance of respiratory system; EL, Elastance of the lung; ER, Elastance ratio; ERS, Elastance of respiratory system; EtCO2, End-tidal carbon dioxide; EIT, Electrical Impedance Tomography; FIO2, Fraction of oxygen inspired; HiFi, High-fidelity pressure catheter; I:E, inspiratory to expiratory time ratio; IVC, Inferior vena cava; LVV, Left ventricular volume; PAWP, Pulmonary artery wedge pressure; Paw, Airway pressure; Pes, Esophageal pressure; PBW, Predicted body weight; PEEP, Positive end-expiratory pressure; PEFR, Peak expiration flow rate; Phigh, High pressure; PL, Transpulmonary pressure; PLER, Transpulmonary pressure according to ratio of elastance method; Plow, Low pressure; PLV, Left ventricular pressure; Pes, Pleural pressure or esophageal pressure; PVR, Pulmonary vascular resistance; RAP, Right atrial pressure; RR, Respiratory rate; S/D/M ABP, Systolic, diastolic, mean aortic blood pressure; S/D/M CBF, Systolic, diastolic, mean carotid blood flow; S/D/M PBP, Systolic, diastolic, mean pulmonary blood pressure; SVO2, Mixed venous oxygen saturation; TCAV, Time-controlled adaptive ventilation; Thigh, Time high; Tlow, Time low; VCV, Volume-controlled ventilation; VD, Dead volume; VILI, Ventilator-induced lung injury; LVV, Left ventricular volume; VT, Tidal volume; VV-ECMO, Veno-venous extracorporeal membrane oxygenation; ΔPaw, Driving pressure; ΔPL, Inspiratory transpulmonary pressure—expiratory transpulmonary pressure; ROI, Region of interest; RCROI, Regional compliance.
References
1. Fuchs L, Feng M, Novack V, Lee J, Taylor J, Scott D, et al. The effect of ARDS on survival: do patients die from ARDS or with ARDS? J Intensive Care Med. (2019) 34:374–82. doi: 10.1177/0885066617717659
2. Papazian L, Aubron C, Brochard L, Chiche JD, Combes A, Dreyfuss D, et al. Formal guidelines: management of acute respiratory distress syndrome. Ann Intens Care. (2019) 9:69. doi: 10.1186/s13613-019-0540-9
3. Fan E, Del Sorbo L, Goligher EC, Hodgson CL, Munshi L, Walkey AJ, et al. An Official American Thoracic Society/European Society of Intensive Care Medicine/Society of Critical Care Medicine Clinical Practice Guideline: mechanical ventilation in adult patients with acute respiratory distress syndrome. Am J Respir Crit Care Med. (2017) 195:1253–63. doi: 10.1164/rccm.19511erratum
4. Amato MBP, Meade MO, Slutsky AS, Brochard L, Costa ELV, Schoenfeld DA, et al. Driving pressure and survival in the acute respiratory distress syndrome. N Engl J Med. (2015) 372:747–55. doi: 10.1056/NEJMsa1410639
5. Luciano G, Pietro C, Massimo C, Davide C, Marco RV, Michael Q, et al. Lung recruitment in patients with the acute respiratory distress syndrome. J Med. (2006) 354:1775–86. doi: 10.1056/NEJMoa052052
6. Schmitt J-M, Vieillard-Baron A, Augarde R, Prin S, Page B, Jardin F. Positive end-expiratory pressure titration in acute respiratory distress syndrome patients: Impact on right ventricular outflow impedance evaluated by pulmonary artery Doppler flow velocity measurements. Crit Care Med. (2001) 29:1154–8. doi: 10.1097/00003246-200106000-00012
7. Nieman GF, Gatto LA, Andrews P, Satalin J, Camporota L, Daxon B, et al. Prevention and treatment of acute lung injury with time-controlled adaptive ventilation: physiologically informed modification of airway pressure release ventilation. Ann Intensive Care. (2020) 10:3. doi: 10.1186/s13613-019-0619-3
8. Nieman GF, Andrews P, Satalin J, Wilcox K, Kollisch-Singule M, Madden M, et al. Acute lung injury: how to stabilize a broken lung. Crit Care. (2018) 22:136. doi: 10.1186/s13054-018-2051-8
9. Zhou Y, Jin X, Lv Y, Wang P, Yang Y, Liang G, et al. Early application of airway pressure release ventilation may reduce the duration of mechanical ventilation in acute respiratory distress syndrome. Intensive Care Med. (2017) 43:1648–59. doi: 10.1007/s00134-017-4912-z
10. Habashi NM. Other approaches to open-lung ventilation: airway pressure release ventilation. Crit Care Med. (2005) 33(Suppl. 3):S228–240. doi: 10.1097/01.CCM.0000155920.11893.37
11. Mekontso Dessap A, Boissier F, Charron C, Bégot E, Repessé X, Legras A, et al. Acute cor pulmonale during protective ventilation for acute respiratory distress syndrome: prevalence, predictors, and clinical impact. Intensive Care Med. (2016) 42:862–70. doi: 10.1007/s00134-015-4141-2
12. Sert NP, du Hurst V, Ahluwalia A, Alam S, Avey MT, Baker M, et al. The ARRIVE guidelines 20: updated guidelines for reporting animal research. PLoS Biol. (2020) 18:e3000410. doi: 10.1371/journal.pbio.3000410
13. Baydur A, Behrakis PK, Zin WA, Jaeger M, Milic-Emili J. A simple method for assessing the validity of the esophageal balloon technique. Am Rev Respir Dis. (1982) 126:788–91.
14. Yoshida T, Brochard L. Esophageal pressure monitoring: why, when and how? Curr Opin Crit Care. (2018) 24:216–22. doi: 10.1097/MCC.0000000000000494
15. Gattinoni L, Chiumello D, Carlesso E, Valenza F. Bench-to-bedside review: chest wall elastance in acute lung injury/acute respiratory distress syndrome patient. Crit Care. (2004) 8:350. doi: 10.1186/cc2854
16. Yoshida T, Amato MBP, Grieco DL, Chen L, Lima CAS, Roldan R, et al. Esophageal manometry and regional transpulmonary pressure in lung injury. Am J Respir Crit Care Med. (2018) 197:1018–26. doi: 10.1164/rccm.201709-1806OC
17. Siobal MS, Ong H, Valdes J, Tang J. Calculation of physiologic dead space: comparison of ventilator volumetric capnography to measurements by metabolic analyzer and volumetric CO2 monitor. Respir Care. (2013) 58:1143–51. doi: 10.4187/respcare.02116
18. Scaramuzzo G, Spadaro S, Spinelli E, Waldmann AD, Bohm SH, Ottaviani I, et al. Calculation of transpulmonary pressure from regional ventilation displayed by electrical impedance tomography in acute respiratory distress syndrome. Front Physiol. (2021) 12:693736. doi: 10.3389/fphys.2021.693736
19. Frerichs I, Amato MBP, van Kaam AH, Tingay DG, Zhao Z, Grychtol B, et al. Chest electrical impedance tomography examination, data analysis, terminology, clinical use and recommendations: consensus statement of the TRanslational EIT developmeNt stuDy group. Thorax. (2017) 72:83–93. doi: 10.1136/thoraxjnl-2016-208357
20. Araos J, Alegría L, García P, Damiani F, Tapia P, Soto D, et al. Extracorporeal membrane oxygenation improves survival in a novel 24-hour pig model of severe acute respiratory distress syndrome. Am J Transl Res. (2016) 8:2826–37.
21. Déry R, Pelletier J, Jacques A, Clavet M, Houde J. Alveolar collapse induced by denitrogenation. Can Anaesth Soc J. (1965) 12:531–57. doi: 10.1007/BF03004416
22. Louis B, Guérin C. Comparison of geometric and algebraic methods to determine mechanical power in patients with acute respiratory distress syndrome. Intensive Care Med. (2019) 45:738–40. doi: 10.1007/s00134-019-05521-4
23. Gattinoni L, Tonetti T, Cressoni M, Cadringher P, Herrmann P, Moerer O, et al. Ventilator-related causes of lung injury: the mechanical power. Intensive Care Med. (2016) 42:1567–75. doi: 10.1007/s00134-016-4505-2
24. Simmons DH, Linde LM, Miller JH, O'reilly RJ. Relation between lung volume and pulmonary vascular resistance. Circ Res. (1961) 9:465–71. doi: 10.1161/01.RES.9.2.465
25. Lee GDJ, Matthews MB, Sharpey-Schafer EP. The effect of the valsalva manæuvre on the systemic and pulmonary arterial pressure in man. Heart. (1954) 16:311–6.
26. On behalf of the LUNG SAFE Investigators and the ESICM Trials Group, Bellani G, Laffey JG, Pham T, Fan E. The LUNG SAFE study: a presentation of the prevalence of ARDS according to the Berlin Definition! Crit Care. (2016) 20:268. doi: 10.1186/s13054-016-1443-x
27. Hébert J-L, Coirault C, Zamani K, Fontaine G, Lecarpentier Y, Chemla D. Pulse pressure response to the strain of the Valsalva maneuver in humans with preserved systolic function. J Appl Physiol. (1998) 85:817–23. doi: 10.1152/jappl.1998.85.3.817
28. Kollisch-Singule M, Emr B, Jain SV, Andrews P, Satalin J, Liu J, et al. The effects of airway pressure release ventilation on respiratory mechanics in extrapulmonary lung injury. ICMx. (2015) 3:35. doi: 10.1186/s40635-015-0071-0
29. Chiumello D, Marino A, Brioni M, Cigada I, Menga F, Colombo A, et al. Lung recruitment assessed by respiratory mechanics and computed tomography in patients with acute respiratory distress syndrome. What is the relationship? Am J Respir Crit Care Med. (2016) 193:1254–63. doi: 10.1164/rccm.201507-1413OC
30. Xia F, Pan C, Wang L, Liu L, Liu S, Guo F, et al. Physiological effects of different recruitment maneuvers in a pig model of ARDS. BMC Anesthesiol. (2020) 20:266. doi: 10.1186/s12871-020-01164-x
Keywords: mechanical ventilation, ARDS, TCAV, APRV, hemodynamic, heart-lung interactions
Citation: Lescroart M, Pequignot B, Bitker L, Pina H, Tran N, Hébert J-L, Richard J-C, Lévy B and Koszutski M (2022) Time-Controlled Adaptive Ventilation Does Not Induce Hemodynamic Impairment in a Swine ARDS Model. Front. Med. 9:883950. doi: 10.3389/fmed.2022.883950
Received: 25 February 2022; Accepted: 19 April 2022;
Published: 17 May 2022.
Edited by:
Savino Spadaro, University of Ferrara, ItalyReviewed by:
Tommaso Mauri, University of Milan, ItalyMichaela Kollisch-Singule, Upstate Medical University, United States
Copyright © 2022 Lescroart, Pequignot, Bitker, Pina, Tran, Hébert, Richard, Lévy and Koszutski. This is an open-access article distributed under the terms of the Creative Commons Attribution License (CC BY). The use, distribution or reproduction in other forums is permitted, provided the original author(s) and the copyright owner(s) are credited and that the original publication in this journal is cited, in accordance with accepted academic practice. No use, distribution or reproduction is permitted which does not comply with these terms.
*Correspondence: Benjamin Pequignot, YnBlcXVpZ25vdCYjeDAwMDQwO2hvdG1haWwuZnI=
†These authors have contributed equally to this work