- 1Department of Mechanical Engineering, Khalifa University of Science and Engineering, Abu Dhabi, United Arab Emirates
- 2Istituto Italiano di Tecnologia, Genoa, Italy
Diabetes mellitus is a chronic disease requiring a careful management to prevent its collateral complications, such as cardiovascular and Alzheimer's diseases, retinopathy, nephropathy, foot and hearing impairment, and neuropathy. Self-monitoring of blood glucose at point-of-care settings is an established practice for diabetic patients. However, current technologies for glucose monitoring are invasive, costly, and only provide single snapshots for a widely varying parameter. On the other hand, tears are a source of physiological information that mirror the health state of an individual by expressing different concentrations of metabolites, enzymes, vitamins, salts, and proteins. Therefore, the eyes may be exploited as a sensing site with substantial diagnostic potential. Contact lens sensors represent a viable route for targeting minimally-invasive monitoring of disease onset and progression. Particularly, glucose concentration in tears may be used as a surrogate to estimate blood glucose levels. Extensive research efforts recently have been devoted to develop smart contact lenses for continual glucose detection. The latest advances in the field are reviewed herein. Sensing technologies are described, compared, and the associated challenges are critically discussed.
Introduction
Recent years have seen an increasing interest in improving the quality of human life, with considerable attention given to the development of reliable physiological monitoring systems. Wearable biosensors represent an important slice of the subject, due to their non-invasiveness and the potential to achieve continuous monitoring of electrical parameters and body fluid composition. Physical vital signs include body temperature, heart rate, heart pulse; body fluids analysis gives information on metabolites concentrations such as glucose, lactate, and alcohol. The most common collection sites are epidermis, mouth, and eyes (1–3). Among the various body parts, the eyes have a significant potential as sensing sites for monitoring biological signals. Glucose monitoring is relevant for a broad range of analyses, from nutritional monitoring to diabetes care.
Diabetes mellitus is a metabolic disease characterized by rising blood glucose levels. Long-term consequences of high glucose levels may cause complications such as microvascular disease, increasing the risk of stroke or ischemia, heart disease, peripheral vascular disease, retinopathy, nephropathy, and neuropathy. According to the World Health Organization (WHO), more than 220 million people worldwide suffer from diabetes. In 2012, diabetes was the tacit cause of death for approximately 1.5 million individuals, and this figure is estimated to be doubled by 2030 (4). Early diagnosis and tight control of blood glucose levels are crucial to avoid the short and long-term complications of diabetes (5). Continuous monitoring of glucose is essential in diabetic patients to take action in restoring glucose levels to healthy values in real-time. Diabetic bodies cannot automatically offset the rise and fall of glucose concentrations, correlated to nutrition, insulin production, and fitness. For instance, eating sessions and hormonal oscillations influence glucose mobilization and metabolism. Metabolism capacity of organs, stress events, and the administration of drugs may directly or indirectly affect glucose availability and consumption. Hypoglycemia (low glucose levels) might acutely endanger neuronal cell viability which is a life-threatening condition, while hyperglycemia (high glucose levels) may cause diabetic ketoacidosis and hyperosmolar in the short-term, and in the long-run, permanent vascular and neurotoxic damages. Continuous glucose monitoring, along with the quick normalize for any glucose level deviations might significantly enhances diabetic health through minimizing the hypo- and hyperglycemic episodes that compromise homeostasis.
The literature reviewed in this article was searched on PubMed, Scopus, ScienceDirect, and Google Scholar, for the period between 2003 and 2022. The used keywords were, ‘smart,' ‘contact lenses', and ‘glucose monitoring'. Here, we present an overview on the progress in smart contact lenses developed for continuous glucose monitoring. First, the history, materials, and design of contact lenses are summarized. The composition and function of the tear fluid are further discussed, with particular attention given to tear biomarkers and their correlation with blood counterparts. The different classes of glucose sensors integrated into contact lenses are described in details including the sensor's working principle and properties. The latest advances in smart contact lenses for glucose detection are highlighted.
Contact Lenses
A contact lens is a thin curved layer of a soft or a rigid transparent material and it is worn in direct contact with the cornea. Contact lenses are obtained from transparent materials, and are sometimes slightly tinted to make them easier to handle. Although contact lenses may seem a modern invention in eye-care, they have a long development history starting from the sixteenth century (6). In 1508, Leonardo da Vinci produced sketches proposing optical solutions for vision correction. He demonstrated that looking through the bottom of a glass bowel filled with water could help rectifying the vision. However, his suggestion was impractical (Figure 1A) (7). Later, in the seventeenth century, René Déscartes introduced the concept of using a test tube filled with water to achieve similar results (Figure 1B). The suggestion of using a tube instead of an entire bowel of water was simpler, but still impractical. The first contact lenses were made of blown glass in 1887 and they were designed such to cover the whole eye surface (7). Although these lenses were beneficial for vision correction, they brought to light several other points with a space for improvement. Firstly, glass lenses were heavy on the eye for long-term wear. In addition, thick glass is not oxygen-permeable, making these lenses harmful for the eye cornea when worn for few hours. In 1940s, hybrid contact lenses made of glass and poly (methyl methacrylate) (PMMA), were introduced. The glass portion covered the cornea, and the sclera was covered by the polymer portion. The lenses were lighter and allowed more oxygen to permeate. The lens design was further brought to the next stage, where the lens covered only the eye cornea and the sclera was left free to breathe naturally (Figure 1C). Non-porous, thin PMMA allowed oxygen to reach the cornea only during blink where the lens moves, and subsequently the lens could be worn for longer periods without causing irritation. A massive breakthrough in contact lenses manufacturing was made by the Czech chemist Otto Wichterle who made the first soft hydrogel contact lens. The lens was made of poly (2-hydroxethyl methacrylate) which is transparent, capable of absorbing water up to 40% of its weight, and could be molded into a more anatomical shape (Figure 1C) (8, 9). In early 1970s, the first commercial soft contact lenses were released after being approved by the Food and Drug Administration (FDA). Since then, soft contact lenses were subjected to developments to increase their oxygen permeability and water absorption properties. In the late of 90s and early 2000s, silicon hydrogel became the material of choice for most contact lens manufacturers (10). Silicon hydrogel lenses allowed up to five times higher oxygen permeability, which helped to keep the eye hydrated, healthy, and comfortable for longer times than before.
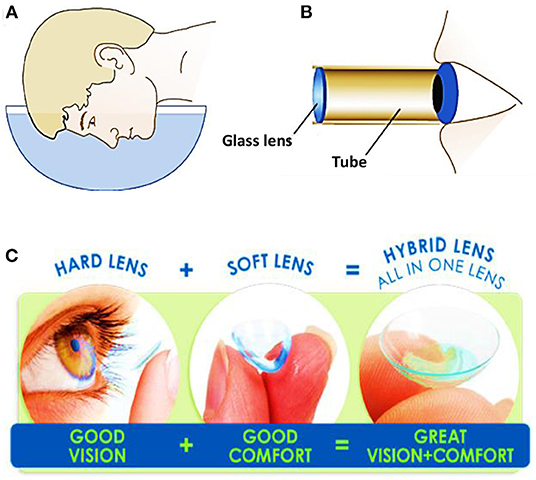
Figure 1. Schematics showing the proposed methods for vision correction: (A) Leonardo da Vinci concept: a man looking through a bowel filled of water, and (B) René Descartes concept: a man looking through a tube filled with fluid. (C) Categories of contact lenses based on the constituents materials.
Commercially available contact lenses can be classified into three categories according to their constituent materials: (i) soft contact lenses such as poly-HEMA, polyacrylamide (PA), polyethylene terephthalate (PET), poly(vinyl) alcohol (PVA), and polydimethylsiloxane (PDMS), (ii) rigid contact lenses made of hard polymers such as PMMA, and (iii) hybrid contact lenses, which combine soft and rigid materials, i.e., a center rigid gas permeable portion with an outer skirt made of a soft contact lens material. Among these contact lenses, soft lenses are the most commonly used in the integrated diagnostic technologies, because of their high oxygen permeability along with the user comfortability and the usage prevalence (11). Characteristics of the three categories of the contact lenses are summarized in Table 1, along with some selected commercial models. Advantages, disadvantages, polymer materials, equilibrium water content EWC%, supplier, and the commercial name (C.N) of the contact lenses are provided.
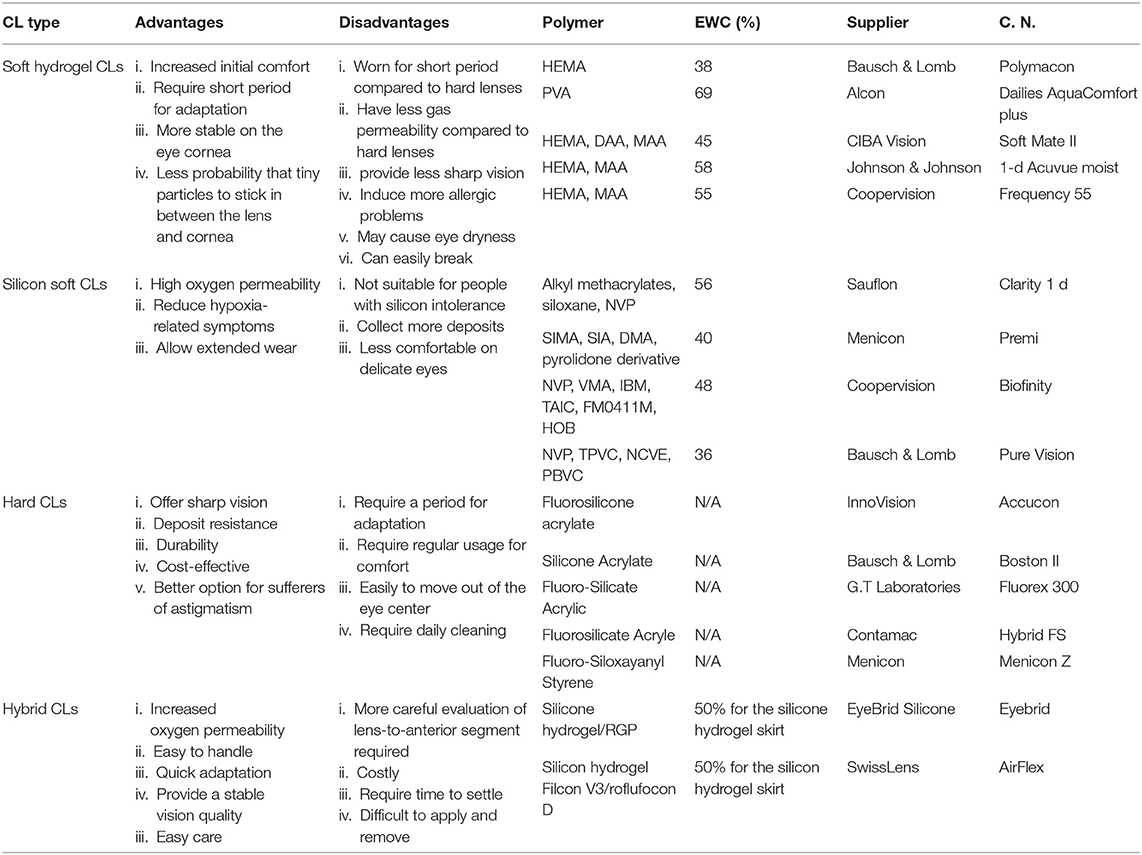
Table 1. Comparison among the different categories of contact lenses and some selected commercial contact lenses (9).
Contact Lens Design
In designing contact lenses, different parameters must be considered. Contact lenses have to be fabricated with base curve radius (BCR) in the range of 8–10 mm to fit comfortably in the eye cornea, and to facilitate tear exchange and oxygen permeability (12, 13). Contact lenses are produced with a diameter range of 14–15 mm to fit different eye sizes easily. The central thickness (CT) of the contact lens is a significant parameter, as it influences the amount of oxygen permeating the lens and further reaching the cornea. Most commercial contact lenses are produced with CT of about 0.1 mm (14). The optical power of the contact lens is the main responsible factor for vision correction, and it measures the degree to which the lens converges the light. The lens optical power is given by the reciprocal of the focal length and its SI unit is inverse meter (m−1), which is called diopter (15). Worn contact lenses are subjected to stresses resulting from eye movements and repeated usage, which might cause irreversible deformation or fracture, leading to deterioration of optical performances and comfort. Hence, the mechanical properties of the contact lens, such as Young's modulus and tensile strength, have to be optimized (16). The transparency of the contact lens material that allows the incident light to go through the lens to be focused in the eyes is a significant property, besides the relatively high refractive index. Chemical properties of the contact lens material such as water content, free-to-bound water ratio, and biological inertness, also affect contact lens performance (17, 18).
Tear Fluid and Biomarkers
Intense investigations have been carried out for decades to find an alternative non-invasive body fluid that could replace blood sampling in clinical settings. Examples include interstitial fluid, tears, salvia, and urine. However, alternative fluids could not provide a reliable alternatives in many cases. However, a successful example of blood replacement was found in the context of bladder cancer screening (19), where urine is the ideal sample for diagnosing due to its direct contact with the tissue (20). Eye basal tear fluid is a multilayered structure containing enzymes, proteins, lipids, and electrolytes (6). Basal tears count three layers which serve to protect, clean, and lubricate the eye. In addition, the eye produces psychic and reflex tears resulting from laughing/crying and irritating conditions, respectively. Tears and blood are separated by a barrier, which makes a compositional difference between blood and tear fluid (21). However, the blood supplies the brain passes through this barrier, causing the leak of metabolites from blood to the tears. Consequently, blood-tear correlations were established as the basis to develop tear proxies that could mirror the blood levels of some metabolites (Table 2) (36–38). It was demonstrated that concentration of glucose, lactate, Na+, K+, Mg2+, Ca2+, Cl−, and urea are correlated with their counterparts in blood (38–40). Additionally, the number of proteins detected in eye tears was in the range of 54–1,543, but the protein figure was found to be strongly dependent upon the sampling method (41). Researchers are investigating the tear fluid for healthy people and cancer patients to establish a relationship among the biomarkers and the different types of cancers. For instance, high levels of lacryglobin were reported for breast cancer patients. Also, lacryglobin was detected in tears for patients suffer lung, colon, prostate, ovarian, and breast cancer (42–44). Several breast cancer biomarkers were detected in eye tears such as protein S 100A8 and triosephsphate isomerase as the concentration of these biomarkers were different in cancer patients compared to healthy controls. L-lactate concentration in tear fluid was found to be correlated with different types of cancer (23). In fact, many types of cancers were found to share the same biomarkers, pinpointing that diagnosing a specific type of cancer based on tear analysis only is a challenge. However, it is noteworthy to measure the susceptibility of cancer at early stages.
Contact lenses which are in direct contact with eye tears can be functionalized or integrated with tiny sensors to continuously detect different metabolites for diagnostic applications. For instance, smart contact lenses for continuous glucose detection is under development by Inwith Corporation, and another contact lens for glaucoma monitoring has been recently approved by the FDA.
Advantages of Using Contact Lenses as Wearable Medical Diagnostic Devices
Conventional diagnostic devices require blood or serum sampling which is considered invasive, painful, inconvenient, and accompanied by the risk of infection. Additionally, most of these devices are complicated to handle, costly, and bench-top, hence they cannot be used at point-of-care settings. Moreover, they are not designed for continuous sensing over 24-h, due to the inaccessibility of the sample. However, multiple diseases would greatly benefit from continuous monitoring technologies, such as diabetes and glaucoma. In contrast, contact lenses combine many features that make them ideal as medical devices for biosensing applications (45–51). In the United States, 45 million people were relying daily on contact lenses in 2016, and this figure has been increasing over time, reflecting the popularity of contact lenses (52). Additionally, contact lenses provide physical contact with eye tears and human tissues for long periods. Contact lenses are small in size, light in weight, cost-effective, portable, and are considered minimally-invasive devices. with the capacity of incorporating an assortment of sensors. The intimate relationship among the eye parts and contact lenses gave possibility to develop lenses to function as continuous monitoring platforms.
Smart Contact Lenses for Continual Glucose Detection
As mentioned earlier, the tear fluid composition has a close relationship with the one in blood, due to plasma leakage from blood into tears via the blood-tear barrier (35, 37, 38). Recent studies show that glucose levels in tears correlated with blood glucose; however, the glucose levels in tears were found to delay for 10–20 min (53). Smart contact lenses developed for glucose detection are classified into three categories based on the type of the sensor integrated with the contact lens. This section discusses each category, including their working principles, advantages, drawbacks, limitations, and recent advances.
Fluorescence-Based Contact Lenses for Glucose Detection
Fluorescence occurs when an incident light of a wavelength range 200–800 nm is absorbed by specific molecules, which transit certain electrons to higher energy levels (54). The excited electrons return to their ground states emitting the absorbed light in the form of fluorescence. However, some of the absorbed light is lost in form of heat or vibration. Consequently, the emitted light has lower energy than that of the absorbed. The electronic transition is an instantaneous process lasting 10−15 s, and the lifetime of the excited state is around 10−8 s. Therefore, the whole process of fluorescence emission lasts about 10−8 s (55). The fluorescent molecules (fluorophores) can lose the absorbed light not only by re-emission heat or vibration, but also by transferring the energy into another fluorescent molecule or fluorophore nearby. When part of the excitation energy is transferred from the excited/donor fluorophore to a ground state of an acceptor fluorophore, the process called Förster resonance energy transfer (FRET) (56). The amount of the transferred energy depends on the spectral overlapping between the fluorophores (donor and acceptor fluorophores) and the interspace between them (57). Förster resonance energy transfer is considered as a fluorescence suppression process for the energy absorbed by the donor fluorophore.
Fluorescence-based sensors are synthesized from a certain analyte-receptor/molecular recognition agent, a donor fluorophore, and an acceptor fluorophore, which all lie in vicinity of each other. In the FRET-based sensors, when the analyte binds with the receptor, the receptor undergoes a chemical structural change moving the fluorophores farther apart, which decreases number of electrons transferred to the acceptor fluorophore. The FRET decreases, resulting in an increase in the fluorescent emitted light, which can be correlated to the analyte concentration. An additional mechanism can be used for developing fluorescent sensors: a receptor/molecular recognition agent which competitively binds the biomarker/analyte (58). In this mechanism, the fluorophore binds the receptor, leading to an electron transfer from the fluorophore to the receptor, which causes the fluorophore to emit fluorescent light. However, in presence of the biomarker/analyte, the receptor binds the biomarker separating the fluorophore from the receptor, and because the conduction energy levels of the fluorophore are full, no fluorescence occurs. This means that in this mechanism the higher the biomarker/analyte concentration, the lower the fluorescent emission (59).
Fluorescent sensors have been utilized in various applications due to their versatility, sensitivity, and selectivity. Fluorescent glucose sensors were integrated into contact lenses for continuous glucose detection in tear fluid. The first contact lens integrated with fluorescent sensor for glucose tear monitoring was developed by Badugu et al., in 2003 (60). Daily disposal contact lenses were embedded with boronic acid containing fluorophores. The contact lens showed to be suitable for detecting tear glucose levels in the range of 0.05–1.0 mM. The contact lenses showed a reversible response, and functioned in the physiological pH and ionic strength. The same work team developed colorless contact lens for glucose sensor based on a novel boronic acid containing fluorophores, which were compatible with low pH and methanol-like polarity (61). The excitation and emission light for the embedded sensor were in the UV range and the sensor responded to tear glucose concentration in the range of 0.05–0.5 mM. The sensitivity of the sensor was 20% for glucose change from 0.05 to 0.5 mM, and the response time was 10 min. Recently, the same research team reported a methodology for tear glucose monitoring based on silicon hydrogel contact lenses (SiHG) (62). The glucose recognition agent, fluorophore Quin-C18, strongly bounded to the lens without showing any significant leaching after multiple rinses. The contact lens was tested in-vitro and showed a robust performance as similar response to glucose after 3 months of storage, was observed. March et al. developed fluorescent glucose sensors based on a fluorophore and a glucose recognition agent trapped in hydrogel spheres that were inserted into a soft contact lens made of poly (vinyl alcohol) (63). When the glucose molecules reacted with the glucose receptor, the fluorophores shifted away from the receptor, decreasing FRET and increasing the fluorescent light upon increasing glucose concentrations. However, a 15 min delay in the glucose concentration readouts was observed. The sensor was compatible with a hand-held fluorometer, used to collect the output signal. Moreover, pHEMA and PDMS contact lenses have been integrated with fluorescent glucose sensors. The glucose-responsive molecules and the organic fluorescent dye were encapsulated in silica nanoparticles, and were embedded in the contact lenses. The silica retained the capsule shell integrity and prohibited the leakage. The contact lenses were able to detect glucose concentration in the range of 0.5–5.0 mM (64). Also, daily disposal commercial contact lenses were integrated with fluorescent glucose sensors based on boronic acid-containing fluorophores. Glucose concentrations in the range 0.05–0.50 mM were detected with an equilibrium time of 10 min. The pH, chloride, and polarity were found to be interfering with the sensor readings (65, 66). Recently, a highly sensitive fluorescent glucose sensor together with a reference probe were implemented into pHEMA contact lens to achieve ratiometric analysis with obvious fluorescence color changes, for the colorimetric detection of glucose concentrations in eye tears (Figures 2A–F) (67). The glucose levels in tears were monitored via a smartphone as a reader, which worked by capturing and analyzing the fluorescent images of the contact lenses. An increase in glucose concentrations led to a shift in the fluorescent color of the contact lens from pink to blue. The contact lens detected glucose concentrations in the range of 0.23 μM-1.0 mM, in artificial eye tears. The biocompatibility of the contact lenses were demonstrated in the tests carried out on animals. More recently, Deng et al. reported soft contact lenses which could detect glucose in tears with high sensitivity (67). A glucose fluorescent recognition molecule probe and a reference fluorescent dye formed the glucose sensor that was immobilized into the hydrogel matrix of the contact lens. With increasing glucose concentration, the color of the contact lens changed from pink to blue due to shifting the fluorescent color. The detection system of the sensor's signal was replying on a smartphone camera that capture photographs for the contact lens and a software transformed the images into RGB signals to quantify the glucose levels. The sensor showed a working range of 0.023–1.0 mM, and biosafety when it was tested on animal models (67).
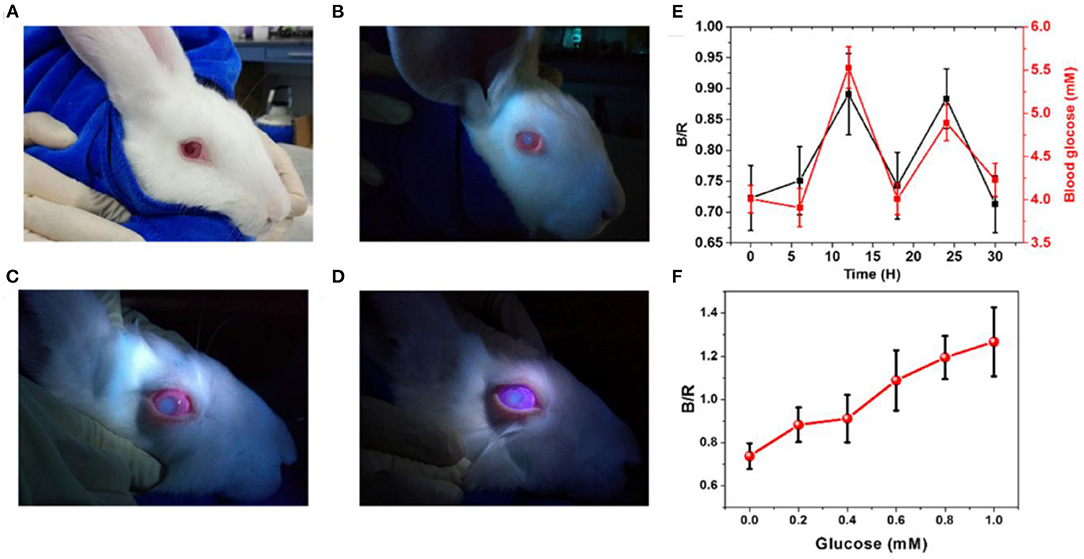
Figure 2. Florescence-based smart contact lens for tear glucose sensing. (A) Photographs of the rabbit wearing the smart contact lens under ambient light illumination. (B) The rabbit eye wearing contact lens under illumination by UV LED light. (C) The worn lens in artificial tears containing glucose of 1 mM concentration. (D) The worn lens in artificial tears containing glucose of 5 mM concentration. (E) Blood glucose levels measured by a commercial glucose meter over time and their corresponding tear glucose levels measured by analyzing the fluorescent signals emitted from the contact lens worn on the rabbit eye. (F) The ratio of the blue and red channel (B/R) for the images taken for the contact lens worn in the rabbit eye and immersed in artificial tears with different glucose concentrations (67).
A variety of fluorescent sensors were developed and integrated with contact lenses to monitor many other biomarkers in tears. For example, Lakowicz and his group developed ion sensors (Cl−, K+, Na+, Ca2+, and Mg+2), and integrated them into silicon commercial lenses for long-term detection of dry eye syndrome (68). These proof-of-concept studies paved the way for more fluorescent probes to be coupled with contact lenses and utilized for monitoring biomarkers, such as lactate, potassium, magnesium, sodium, and urea.
Fluorescent sensors have been utilized in various applications due to their versatility, sensitivity, and selectivity. However, they suffer from high intensity background fluorescence which commonly exists in biological media. The fluorescence-based molecular recognition agents are influenced by the ambient oxygen concentration and temperature. The fluorophores have low chemical stability due to photodegradation of the optical species (69). Furthermore, some fluorescence receptors require the presence of solvents to function (70), which may be challenging to be integrated in a wearable lens.
Electrochemical Contact Lens Sensors for Glucose Detection
Electrochemical sensors have been developed for medical diagnosis over the last few decades. They can be classified into two categories based on their response to glucose: (i) selective, and (ii) non-selective. Only the glucose selective electrochemical sensors lies in the scope of this review. Semiconductor fabrication techniques were employed for producing the electrochemical sensors (71). Generally, electrochemical sensors are constructed from three electrodes: reference, working, and counter electrodes. Selective electrochemical sensors are manufactured for detecting various biomarkers such as glucose, lactate, uric acid, cholesterol, dopamine, and drugs (72–75). For selective glucose detection, enzymes offer some superior advantages in terms of selectivity, sensitivity, and rapid response. For example, glucose oxidase is used in glucose detection where the enzymatic reaction converts glucose into gluoconolactone and hydrogen peroxide. Hydrogen ions, oxygen, and electrons come out of the dissociation process for the produced hydrogen peroxide. The generated electrons are utilized by the three-electrode system to measure the glucose concentration.
Recent advances in transparent electrodes, wireless communication technology, and biosensors have accelerated the progress of smart contact lenses. Extensive research efforts were carried out by Google and Novartis to develop contact lens sensors that can be powered wirelessly and provide glucose concentrations in the tear continuously. Soft contact lenses for glucose measurements in tear fluid have been developed by integrating electrochemical sensors with contact lenses (76). For instance, Yao et al. developed PET contact lenses for continuous glucose detection based on a three-electrode sensor made of titanium (Ti, 10 nm), palladium (Pd, 10 nm), and platinum (Pt, 100 nm). Thin films were sequentially deposited by thermal evaporation onto the wafer, to form the electrodes. Immobilization of glucose oxidase was achieved through a deposited film of titanium oxide which also acted as a passivation layer for the contact lens surface. Potential interferences of urea, ascorbic acid, and lactate were minimized by using Nafion film. In order to test the sensor performance, electrical connections were defined by three large pads of 2 mm2 each, and the current flow through the working electrode was recorded. The sensor exhibited a fast response time of 20 s, and a limit of detection lower than 0.01 mM. A sensitivity of 240 μA cm−2 mM−1 was recorded in the glucose concentration range of 0.1–0.6 mM. The same group reported PDMS contact lenses integrated with another type of electrochemical sensor for glucose detection in tears (77). The sensor combines three electrodes made of platinum, silver, and silver chloride. The fabrication of the sensor was carried on a PDMS layer of thickness 70 nm that later was bended to conform to a contact lens shape. Sputtering techniques were used to deposit the electrodes: 200 nm of Pt layer, and 300 nm of silver layer. GOD was immobilized on the electrodes through trapping it in polypropylene membranes. For in vitro glucose testing, the contact lens-integrated sensor measured glucose concentrations in the range from 0.03 to 5.0 mM. The in situ testing was carried out by placing the contact lens onto the eyeball of a rabbit, and the results were correlated with the outputs given by conventional blood glucose readers. Glucose concentrations in both blood and tear were peaked following the oral intake of glucose. The tear glucose showed a delay of 15–20 min compared to the glucose levels in blood, and both reached the maximum after 55 min of glucose intake. The contact lens showed a linear relationship between glucose concentrations and the recorded current in the range of 0.025–1.475 mM, with a correlation coefficient of 0.99. The highest sensitivity was achieved at a pH of 7.0, and it was temperature-dependent. The decrease in current density beyond pH 7.0 and 45°C was attributed to the degradation of GOD in the alkaline medium, and to its thermal deactivation, respectively. Also, M. Chu et al. developed PDMS contact lenses for in situ glucose monitoring based on GOD enzyme deposited on flexible electrodes (78). The sensor showed a linear relationship with a correlation coefficient 0.99, between glucose levels and the output current in the glucose concentration range of 0.03–5.0 mM The contact lens sensor was tested on a rabbit model and detected its tear glucose levels. Recently, Kim et al. developed a graphene field effect transistor combined with an antenna to function as a glucose sensor. The sensor was integrated into a soft contact lens and operated wirelessly (79). The contact lens was tested for glucose quantification in vitro and in vivo. Glucose oxidase was chemically attached to the pyrene linker, and was further immobilized on the graphene channel. The signal receiver was placed at 10 mm from the contact lens to collect the antenna signals. More recently, a wireless electrochemical glucose sensor with display pixels was incorporated into a soft contact lens (Elastofilcon A) for monitoring glucose concentration in tears (Figure 3) (80). For the first time, a display was introduced in smart contact lenses to eliminate the necessity of bulky equipment used in signal detection. The performance of the lens was tested on a rabbit eye for glucose concentration in the range of 0.1–0.9 mM. Upon increasing the glucose concentration above the normal levels, the display turned off. Jeon et al. (81) reported polyethylene terephthalate contact lenses-integrated with 3-electrode electrochemical glucose sensor. The electrodes were composed of gold, titanium and silver chloride. The designed IC was implemented in a 0.18 CMOS processor. The RF transmitter and the thin film rechargeable battery were integrated with the contact lens. The system was tested in vitro for glucose detection in the glucose range of 3–25 mg/dL, and showed a linear response. K. Hayashi et al. developed the first self-powered contact lenses for continuous glucose monitoring by combining the transmitter with a glucose fuel cell that functioned as both the power source and a sensing transducer (82). The self-power operation of the contact lens was verified in a glucose concentration of 30 mM. Recently, the first solar cell powered contact lenses for glucose monitoring, was reported (83). A LED was implemented in the contact lens to eliminate the necessity for wireless communication and the power supply on-lens solar cell eliminated the necessity for wireless power delivery, enabling a stand-alone operation under room-ambient light. Besides glucose sensing alone, smart contact lenses were also developed as drug delivery systems to allow insulin release (84). For instance, Keum et al. (84) reported soft contact lenses for simultaneous glucose detection and insulin delivery. The contact lens was built on a biocompatible polymer containing ultrathin flexible electrical circuit, microcontroller chip, drug delivery system, wireless power management, and data communication apparatus. The contact lens was tested on rabbit models and was validated by conventional blood glucose tests. The drugs were electronically triggered to be released from the reservoirs for bringing glucose levels back to normal levels.
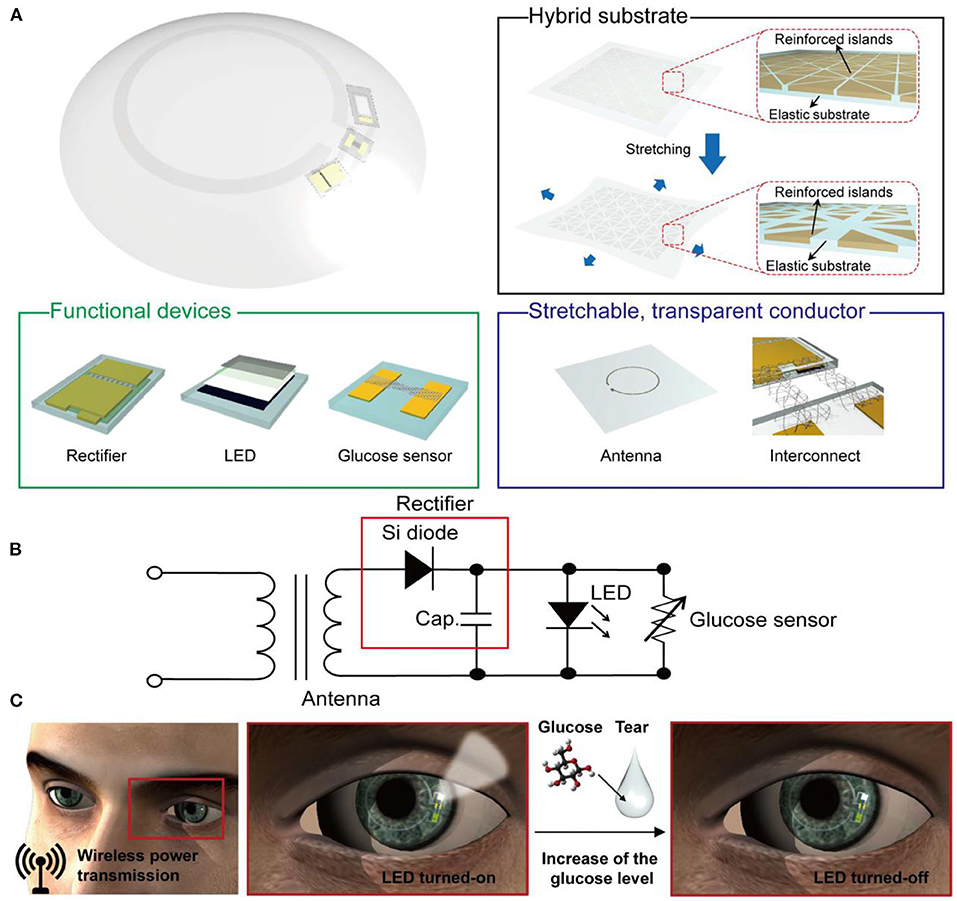
Figure 3. A stretchable transparent smart contact lens system. (A) Schematic diagram of the soft contact lens; the lens is composed of a hybrid substrate, functional devise (rectifier, LED, and glucose sensor), and a transparent stretchable conductor (for antenna and interconnects). (B) A diagram of the circuit used in the smart contact lens system. (C) Operation of the smart contact lens; the electric power is wirelessly transmitted to the lens through the antenna, which in turn activates the LED pixel and the glucose sensor. The pixel turns off once the detected glucose level exceeds the normal limit (80).
Multifunctional contact lens sensors that can monitor glucose along with other physiological parameters were introduced. For instance, smart sensors were integrated with soft contact lenses for wireless detection of glucose and intraocular pressure, simultaneously (Figure 4) (79). Highly transparent and stretchable sensors that can monitor glucose and intraocular pressure were incorporated into soft contact lenses. The breakthrough in this study was the introduction of the hybrid structure of 1D and 2D nanomaterials which added reliability, robustness, flexibility and transparency. Among the incorporated electronic RLC, R responded for the glucose concentration whereas L and C varied with the intraocular pressure. The change in the reflection coefficient due to R changes, and the shift in the resonant frequency resulting from L and C changes, were independent of each other. The contact lenses were tested in vitro and in vivo for detecting glucose in the range 0–10 mM. Another multiplexed contact lens sensor was developed for simultaneously monitoring glucose and corneal temperature (85). The smart contact lenses relied on ultrathin MoS2 transistors and gold nanowires. The sensors were incorporated directly on the surface of PDMS contact lenses to leave the sensors in direct contact with the tear fluid and the ocular surface. In vitro studies showed that the sensor system was biocompatible. Continuous glucose monitoring investigations were carried out in vitro, in the glucose concentration range of 0.0–0.6 mM, and the measured electric current vs. glucose concentrations showed a linear relationship. The recoded sensitivity in the whole tested glucose range was 45%. The potential interference from the tear ingredients were negligible as the sensor showed the same response in the buffer and artificial tears. The sensitivity for temperature was recorded in the temperature range of 30–50°C and the resistance of the gold nanowires showed a linear trend with the temperature in the tested range. This system provided a novel insight into designing multifunctional bioelectronics sensors. Further, the fabrication strategy allows for embedding other functioning components, such as electrode arrays for electro-retinograms, antennas for wireless communications, thin film batteries/super capacitors, and wireless radiofrequency transmitters. Such multiplexed contact lenses holds great promise in next-generation ocular diagnostics.
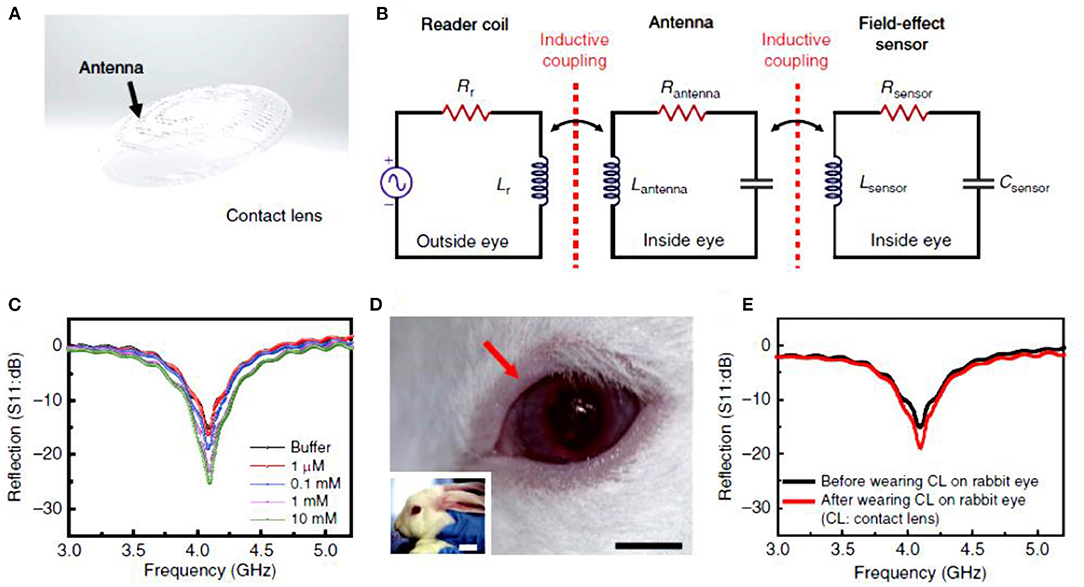
Figure 4. Smart contact lenses for wireless glucose detection. (A–E) Electrochemical sensor integrated with a contact lens: (A) schematic illustration of the transparent glucose sensor integrated in the contact lens, (B) schematic illustration of the reading circuit in the wireless sensing mode, (C) wireless glucose measurements in the glucose range of 1–10 mM, (D) photographs of the smart contact lens placed on rabbit eyes, and (E) wireless glucose detection by the smart contact lens in vitro and in vivo (79).
However, many challenges still have to be overcome to achieve usability in clinical settings (86). Electrochemical sensors are based on enzymes, which are unstable by nature and suffer degradation in a short lifespan, which limit the employability of the sensor for long periods. Furthermore, the operation conditions such as ambient oxygen, pH, temperature, and humidity influence the sensor performance. Additionally, sterilizing the smart contact lens according to health regulations may lead to enzymes denaturation. The interaction of the hydrogen peroxide with tear ingredients like ascorbic acid interferes with the sensor response (87). The microfabrication of the electrochemical sensor on polymer substrates is also a challenge due to the limitations of the thermal and mechanical properties of the polymer material (88). Electrochemical sensors require an applied power to drive the chemical reaction, which makes the fabrication more challenging. Mostly, electrochemical sensors are made of opaque electronic materials, metal antennas, interconnects, and integrated circuit chips which may block the user vision, and decrease the oxygen permeability (77, 89).
Light Diffractive Contact Lenses for Glucose Detection
Photonic crystals (PCs) are made of periodically ordered materials of different refractive indexes, and they are classified according to number of periodicity directions into 1D, 2D, and 3D (86). When a photonic crystal is illuminated by a polychromatic light beam, the diffracted light follows Bragg's law:
where m is the diffraction order, λd represents the diffracted wavelength, neff is the effective refractive index of the PC, θd is the diffraction angle, and Λ is the periodic constant of the PC. Accordingly, the diffracted wavelength changes due to any modification in the periodic constant or in the effective refractive index. Based on this principle, 1D, 2D, and 3D PCs have been developed for sensing applications. When the periodicity Λ of the PC is comparable to the wavelengths of the incident visible light, the PC diffracts a visible color that can be seen by naked eyes. Hence, PC-based sensors exhibit a visible color change. Therefore, PC sensors are strong candidates to be employed in point-of-care settings.
Unlike the selective electrochemical glucose sensors, most of the photonic glucoses sensors are based on phenylboronic acid (PBA) derivatives which are used as glucose recognition agents instead of enzymes used in electrochemical sensors. PBA derivatives form reversible covalent bonds with cis-diol molecules and α-hydroxyl acids, such as glucose and lactate, respectively (90). Studies showed that 1D PC (1D-hologram gratings) can be fabricated in many natural and synthetic hydrogel matrices, and can be functionalized with the appropriate receptors to detect a variety of analytes (91). For instance, 1D-PC glucose sensors were developed with polyacrylamide functionalized with 4-vinylphenylboronic acid (4-VBPA). The sensors were prepared by the diffusion method along with the laser interference technique. Complexation of glucose and boronic acid moieties immobilized in the hydrogel network caused volumetric shifts of the hydrogel matrix, inducing a change in the periodic constant Λ, and subsequently the diffracted wavelength/color (91). The main concern was that the sensor could function at a high pH only, above physiological values (92). To develop 1D-PC glucose sensor operates in the physiological pH range, Kabilan et al. used an alternative phenylboronic acid derivative called 3-(acrylamido)phenylboronic acid (3-PBA) (91). The ability of the sensor to bind with glucose under physiological conditions was attributed to the lower pKa (dissociation constant) of the 3-PBA, which resulted from the meta-position of the acrylamide group on the phenyl ring. The sensor exhibited a visible and reversible response to glucose concentrations under physiological conditions. The sensor appeared green in the glucose-free buffer (PBS) and when exposed to the glucose solution, the diffracted light shifted toward longer wavelengths. The glucose molecules diffused into the hydrogel sensor to bind with the pendant boronic acid groups, resulting in a decrease in the pKa, and allowed to reconfigure the boronic acid groups to form the charged tetrahedral phenyl boronate anion (93). These charged anions generated Donnan's potential that induced an osmotic pressure, resulting in swelling the hydrogel matrix (94). The swelling was reflected on the diffracted wavelength which red-shifted due to increasing the periodic constant of the sensor. Upon removing the glucose solution and washing the sensor in PBS buffer, the sensor returned to its original volume, hence the diffracted spectrum returned to its original basal diffracted wavelength. Since cis-diols binding with boronic acid is reversible, washing out the glucose decreased the concentration of the boronate anions, leading to shrinkage of the sensor due to the elastic restoring force of the hydrogel network that expelled the absorbed water and counter-ions (95). The 1D-PC glucose sensor showed a sensitivity of 18 nm mM−1 in the glucose range of 0–11 mM, and it was more selective for glucose over lactate. Later, this sensor was attached to a PVA contact lens for clinical testing (96). Toxicity and performance of the contact lens as a non-invasive glucose sensor were examined after sterilization. The sensitivity of the sensor showed a decline, which means that the sterilization procedures negatively impacted on the sensor performance. However, the sensor responded to glucose in a reversible manner, the sensitivity dropped to 4.5 nm mM−1, compared to its value of 18 nm mM−1 prior to sterilization. The readout was performed using a white light source connected to an optical fiber, and a spectrometer connected to a computer to record the diffracted wavelengths. An alternative practical detection system would be needed, and the sensitivity of the contact lens sensor should be improved for applicability. Another concern about the described 1D-PC sensor is the usage of phenylboronic acid derivatives that binds with other biomarkers such as lactate, galactose, fructose, and mannose. In this regards, another study on the same sensor considered the response and the equilibrium times, showing that the response time for glucose concentrations below 1 mM was around 7 min. However, faster responses were received for higher glucose concentrations. The equilibrium time for the sensor was about 50 and 70 min for high and low glucose concentrations, respectively. The 1D-PC sensors were fabricated by the diffusion method and the laser interference technique, requiring a multistage process of 10 stages (64). Additionally, there may be concerns about the potential interference of the tears ingredients and the long response time.
On the other hand, 3D-PC glucose sensors consist of highly charged nanoparticles (polystyrene or silica) periodically arranged in three dimensions, in a polymer matrix. Asher's group developed 3D-PC glucose sensors based on polystyrene nanoparticles embedded in co-polymerized hydrogel (polyacrylamide-polyethylene glycol) (97). Two phenylboronic acid derivatives were attached separately to the hydrogel post-photopolymerization. The sensors were tested in synthetic tear fluids at physiological pH and ionic strength. Binding the glucose with the boronate anions occurred through the formation of a bis-bidentate cross-link, a 2:1 PBA-glucose complex, which led to a shrink in the hydrogel, resulting in a blue shift in the diffracted wavelengths due to shortening the periodicity constant. The sensor hue changed and was observable by the naked eye, and shifted from red to blue over the physiological glucose concentration range. The selectivity of the sensor for glucose compared to galactose, mannose, and fructose was demonstrated. The sensitivity was approximately 14 nm mM−1 and the limit of detection was as low as 1 μM in synthetic tear fluid. The same group promoted the response kinetics of the 3D-PC glucose sensor by copolymerizing n-hexylacrylate with a acrylamide, and functionalized the sensor with 5-amino-2-fluorophenylboronic acid (5A-2F-PBA) (98). The sensor showed a response time of 300 s in glucose concentration of 1 mM at pH 7.4 and a temperature of 22°C. However, the response time was shorter at higher temperatures and at higher glucose concentrations. In the physiological temperature (37°C), the response time went down to 90 s for a glucose concentration of 5 mM. The authors recommended attaching the sensor to a contact lens or inserting the sensor under the lower eyelid for achieving the continuous glucose detection. Diabetic patients could determine their glucose concentrations by observing the color of the sensor using a compact device combining a white light source, a mirror, and a color chart. Hu et al. developed near-infrared 3D-photonic crystal sensor for ratiometric sensing of glucose in tears (99). The novel design of the reported sensor was to overcome the slow response and low sensitivity of the 3D-photonic crystal sensors. In the sensor, the crystalline colloidal array (CCA) itself was glucose-responsive and made of poly (strene-co-acylamide-3-acrylamidophenylboronic acid). The CCA was arranged in a positively hydrogel matrix of poly (acrylamide-co-2-(dimethylamino)ethyl acrylate). Unlike the previous sensors 'designs, the arranged particles in the hydrogel matrix were responding to glucose. The glucose sensor diffracted the wavelength of 1,722 nm, whose intensity decreased with increasing glucose concentration over the physiological relevant range in tears. The sensor showed to be able to detect glucose concentration as low as 6.1 μg/dL. The response time was 22 min for glucose concentration 7.5 mg/dL and decreased with increasing glucose concentration. Braun's group introduced a 3D-PC glucose sensor that linearly and rapidly responded to glucose by introducing a volume resetting agent, polyvinyl alcohol (PVA) (100). The acrylamide pre-gel was polymerized with highly charged polystyrene nanoparticles, followed by attaching the PBA derivative to the hydrogel –and the PVA was diffused into the hydrogel to bind with the boronate anions forming crosslinks, which led to shrinking the hydrogel matrix. When the sensor was exposed to glucose, the PBA-PVA crosslinks were superseded with 1:1 PBA-glucose complexes, resulting in swelling of the hydrogel, and subsequently a red-shift of the diffracted light. The sensor covered the clinical relevant glucose range of 2.2–38.9 mM, presenting a maximum sensitivity of 12 nm mM−1 in glucose concentration range of 0–10 mM when 5-amino-2-fluorophenylboronic acid (5A2FPBA) was used as the glucose recognition agent (101, 102). However, the best kinetic response was recorded for the sensor which was functionalized by the derivative 3-PBA, as the time required for the sensor to reach 90% of equilibrium binding was 7 and 12 min for glucose concentration ranges of 0–10 mM and 30–40 mM, respectively. Leakage of PVA from the sensor is expected, which significantly would affect the sensor response/sensitivity and reusability. The highly charged monodispersed polystyrene spheres used in the sensor were synthesized by the emulsion polymerization process, which takes 3 weeks (55, 60). In order to fulfill a rapid fabrication process for the photonic-based glucose sensors, 2D-PC glucose sensor was introduced where a fast fabrication process of 2D-PC at the air/water interface has been reported (103). At the air/water interface, it was possible to produce a hexagonal arrangement of nanoparticles of area 280 cm2 in 2 min. The studies showed that these arranged nanoparticles in a monolayer form can be incorporated into stimuli-responsive hydrogels. The forward diffracted monochromatic light passing through the 2D-PC hydrogel showed a Debye diffraction ring of a diameter depends upon the particles interspace, and the Debye ring was exploited to monitor the hydrogel volumetric change. The Debye diffraction follows the formula:
where α is the forward diffraction angle of the Debye diffraction, d is the interspace among the particles, and λ is the incident wavelength. The forward diffraction angle is given by the relationship;
where D denotes to the diameter of Debye ring and h represents the distance between the sensor and the screen. For instance, Xue et al. developed a 2D-PC glucose sensor based on the air/water interface approach and the sensor was investigated in artificial tears (103). The sensor was selective for glucose over galactose and fructose at the physiological ionic strength (150 mM), and presented short response time (50). However, the sensor was functioning only at high pH values above the physiological pH. Another study reported a 2D-PC glucose sensor which can operate in the physiological pH (104). The sensor showed high sensitivity and a short response time of 180 s. Although detecting the sensor response was simple, the readout methodology is not practical, especially when the sensor is attached to contact lenses. Because the contact lens constrains the sensor expansion/extraction in both x and y directions, leaving the sensor to be free in z-direction only. However, the expansion/extraction in z-direction has no effect on the particles interspace which induces the shifts in the Debye ring's diameter. In addition, measuring the Debye ring diameter in the transmission configuration is not practical in wearable devices. The response of the 2D-PC could not be distinguished by the naked eye, which may limit its applications.
Recently, Elsherif et al. introduced a smart contact lens for continuous glucose detection under physiological conditions (Figures 5A–C). The hydrogel glucose sensor was made of 3-(acrylamido)phenyl boronic acid immobilized in polyacrylamide hydrogel matrix. A light diffraction element (1D grating) was replicated on the glucose-responsive hydrogel by replica molding. The replicated 1D grating functioned as a transducer to convert the volumetric shift of the hydrogel into an optical signal. The 1D-grating enhanced the surface-to-volume ratio of the sensor and the hydrophobicity, which aided in shortening the sensor response time and saturation time to 3 s and 4 min, respectively, in continuous sensing mode. The glucose sensor was attached to a commercial contact lens for glucose detection in eye tears and a smartphone was used as a reader by exploiting its integrated ‘ambient light sensor'. However, the limit of detection of the smart contact lens was not low enough to work in glucose concentration range of eye tears. Another study by the same group reported smart contact lenses for glucose detection based on light diffusing microstructures (Figures 5D–F). In this study, 3-PBA was used as the glucose recognition agent and the polyacrylamide was the hosting matrix. The light diffusing microstructures were replicated on the glucose-responsive hydrogel to convert the volumetric change of the sensor into a change in the light intensity and the spatial profile of the light beam. A smartphone captured the reflected light signals from the lens and correlated them with glucose concentrations. This study showed that the contact lens sensor can be readout by a white light beam which is more safe than the laser which was used in their previous developed contact lens. At the same time, the light diffusing microstructures imprinted on the sensor kept the advantages of high surface-to-volume ratio and the short response time of the glucose sensor. Recently, bifocal contact lenses were developed for continuous glucose detection and presbyopia (107). The commercial contact lens used for myopia treatment was integrated with a hydrogel glucose sensor. The hydrogel glucose sensor offered a light conversion properties as its surface was imprinted with Fresnel structure. Upon integrating the sensor with the contact lenses, the contact lens offered two focal lengths; one came from the lens curvature which aid in far seeing, and another focal length resulted from the Fresnel structure which assist for near vision and glucose detection (Figures 5G,H). A smartphone was used as the contact lens sensor reader and it was capable of detecting the optical signals of the contact lens sensor, and correlate them with the glucose concentrations. The smart contact lenses operated in the artificial eye tears and showed a linear response trend in the glucose concentration range of 0–10 mM with a sensitivity of 1.5% mM−1.
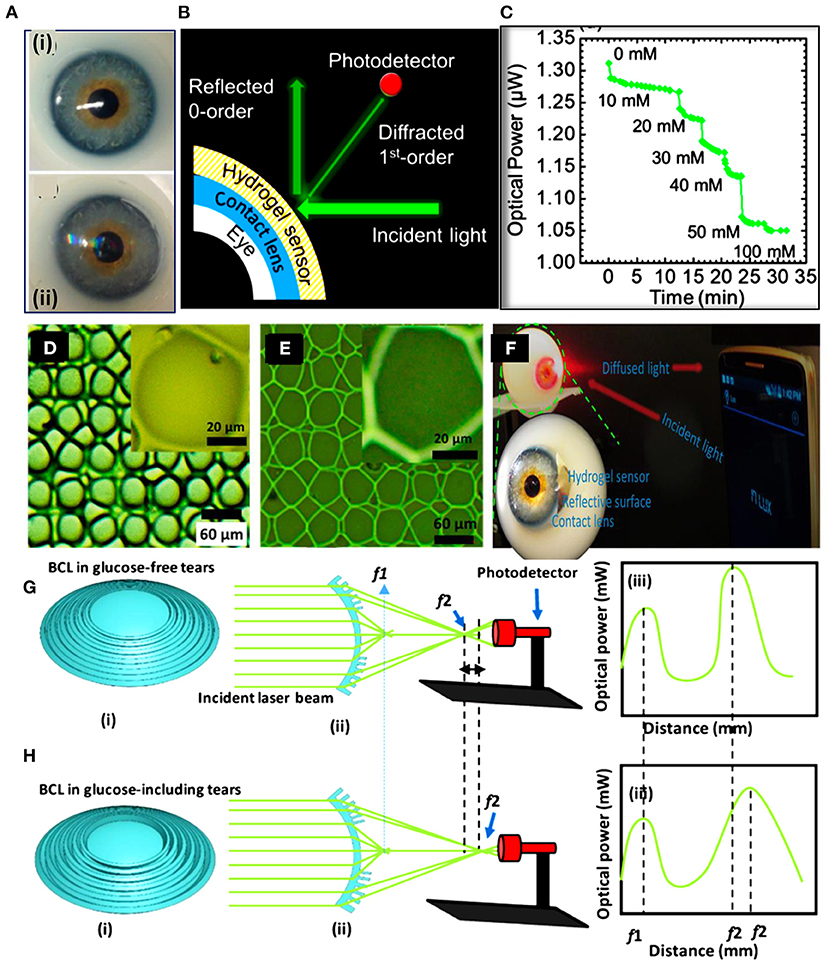
Figure 5. Smart contact lenses for glucose monitoring developed based on light diffractive sensors. (A–C) A light diffraction-based glucose sensor integrated with a soft contact lens: (A) a soft commercial contact lens placed on an eye ball (i), and the commercial contact lens integrated with a 1D grating glucose sensor (ii), (B) schematic illustration of the readout methodology for the contact lens integrated with the 1D grating glucose sensor, and (C) continuous glucose detection measurements shows the reflected optical power from the contact lens vs. the glucose concentration (105). (D–F) Light diffuser-based glucose sensor: (D) optical microscope image for the master holographic light diffuser, (E) the surface of the glucose sensor imprinted with the light diffuser microstructures, and (F) photographs of the smart contact lens integrated with the light diffuser-glucose sensor and placed on an eye ball to be investigated by a smartphone as a reader (106). (G,H) Bifocal contact lenses for glucose detection: (G) schematic illustration of the bifocal contact lens in glucose-free buffer, and (H) schematic of the bifocal contact lens in glucose-complexation conditions (107).
Generally, photonic band gap-based sensors offer inherent merits over their electrochemical counterparts, such as the lack of electrical connections, immunity to electromagnetic interference, relatively easier fabrication process, possibility of remote sensing, and cost-effectiveness (91). Compared to fluorescent glucose sensors, the photonic sensors do not require dyes or fluorophores to operate, eliminating the problem of photobleaching (86).
Conclusion and Future Prospects
Continuous and real-time monitoring of glucose levels allows for tight control of diabetes early stages and progression. Non-invasive glucose detection is a promising method for improving the life quality and expectancy for diabetics. Optical glucose sensors are under progress, including light diffractive and fluorescent sensors. On the other hand, electrochemical sensors are few steps ahead. Minimizing the electronics and employing transparent conductive polymers in addition to the 3D printing technology may revolutionize electrochemical glucose sensors. As the contact lenses come in direct contact with the eye cornea, significant challenged need to be faced. For example, electric current flow through the electrochemical sensors results in Joule heating, which may affect the temperature of the eye. Hydrogen peroxide is a byproduct of the chemical reaction taking place in the enzymatic glucose sensors, and may cause eye irritation.
Contact lenses initially emerged for vision correction but, recently, novel applications have been unlocked. It might become feasible in the near future to use contact lens sensors to monitor a range of ocular and systemic conditions.
Author Contributions
ME wrote the original manuscript. RM edited the manuscript. HB led the project and acquired the financial support. FA, AS, and IA assisted in preparing the manuscript and proofread the manuscript. All authors contributed to the article and approved the submitted version.
Funding
The authors acknowledge Khalifa University of Science and Technology (KUST) and KU-KAIST Joint Research Center for research funding in support on this research (Project Code: 8474000220-KKJRC-2019-Health1). HB acknowledges Sandooq Al Watan LLC and Aldar Properties for the research funding (SWARD Program—AWARD Ref. SWARD-F19-008).
Conflict of Interest
This study received funding from Sandooq Al Watan LLC jointly with Aldar Properties (SWARD Program—AWARD Ref. SWARD-F19-008. The funder was not involved in the study design, collection, analysis, interpretation of data, the writing of this article or the decision to submit it for publication.
Publisher's Note
All claims expressed in this article are solely those of the authors and do not necessarily represent those of their affiliated organizations, or those of the publisher, the editors and the reviewers. Any product that may be evaluated in this article, or claim that may be made by its manufacturer, is not guaranteed or endorsed by the publisher.
References
1. Xu S, Zhang Y, Jia L, Mathewson KE, Jang KI, Kim J, et al. Soft microfluidic assemblies of sensors, circuits, and radios for the skin. Science. (2014) 344:70–4. doi: 10.1126/science.1250169
2. Gao W, Emaminejad S, Nyein HYY, Challa S, Chen K, Peck A, et al. Fully integrated wearable sensor arrays for multiplexed in situ perspiration analysis. Nature. (2016) 529:509–14. doi: 10.1038/nature16521
3. Kim SY, Kim J, Cheong WH, Lee IJ, Lee H, Im HG, et al. Alcohol gas sensors capable of wireless detection using In2O3/Pt nanoparticles and Ag nanowires. Sens Actuators B Chem. (2018) 259:825–32. doi: 10.1016/j.snb.2017.12.139
4. Guariguata L, Whiting DR, Hambleton I, Beagley J, Linnenkamp U, Shaw JE. Global estimates of diabetes prevalence for 2013 and projections for 2035. Diabetes Res Clin Prac. (2014) 103:137–49. doi: 10.1016/j.diabres.2013.11.002
5. Long AN, Dagogo-Jack S. Comorbidities of diabetes and hypertension: mechanisms and approach to target organ protection. J Clin Hyperten. (2011) 13:244–51. doi: 10.1111/j.1751-7176.2011.00434.x
6. Farandos NM, Yetisen AK, Monteiro MJ, Lowe CR, Yun SH. Contact lens sensors in ocular diagnostics. Advanced Healthcare Mat. (2015) 4:792–810. doi: 10.1002/adhm.201400504
7. Goodlaw E. A personal perspective on the history of contact lenses. Int Cont Lens Clin. (2000) 4:139–45. doi: 10.1016/S0892-8967(02)00085-8
8. Otto W, Drahoslav L. Process for Producing Shaped Articles from Three Dimensional Hydrophilic High Polymers. U.S. Patent No. 2, 976, 576. Washington, DC: U.S. Patent Trademark Office (1961). Available online at: https://patents.google.com/patent/US2976576A/e
9. Moreddu R, Vigolo D, Yetisen AK. Contact lens technology: from fundamentals to applications. Adv Healthc Mater. (2019) 8:e1900368. doi: 10.1002/adhm.201900368
10. Musgrave CSA, Fang F. Contact lens materials: a materials science perspective. Materials. (2019) 12:261. doi: 10.3390/ma12020261
11. March WF, Mueller A, Herbrechtsmeier P. Clinical trial of a noninvasive contact lens glucose sensor. Diabetes Technol Therap. (2004) 6:782–9. doi: 10.1089/dia.2004.6.782
12. Bennett ES, Weissman BA. Clinical Contact Lens Practice Lippincott. Philadelphia, PA: Williams & Wilkins (2005).
13. Chen GZ, Chan IS, Lam DC. Capacitive contact lens sensor for continuous non-invasive intraocular pressure monitoring. Sens Actuat A: Physical. (2013) 203:112–8. doi: 10.1016/j.sna.2013.08.029
14. Fink BA, Mitchell GL, Hill RM. Rigid gas-permeable contact lens base curve radius and transmissibility effects on corneal oxygen uptake. Optometry Vision Sci. (2006) 83:740–4. doi: 10.1097/01.opx.0000232806.06677.97
15. Douthwaite WA. Contact Lens Optics and Lens Design. Elsevier Health Science (2006). p. 420. doi: 10.1016/B978-0-7506-8879-6.50009-9
16. Tranoudis I, Efron N. Tensile properties of soft contact lens materials. Contact Lens Anterior Eye. (2004) 27:177–91. doi: 10.1016/j.clae.2004.08.002
17. Tranoudis I, Efron N. Water properties of soft contact lens materials. Contact Lens Anterior Eye. (2004) 27:193–208. doi: 10.1016/j.clae.2004.08.003
18. Tranoudis I, Efron N. In-eye performance of soft contact lenses made from different materials. Contact Lens Anterior Eye. (2004) 27:133–48. doi: 10.1016/j.clae.2004.02.004
19. Lin C, Pratt B, Honikel M, Jenish A, Ramesh B, Alkhan A, La Belle JT. Toward the development of a glucose dehydrogenase-based saliva glucose sensor without the need for sample preparation. J Diab Sci Technol. (2018) 12:83–9. doi: 10.1177/1932296817712526
20. Lim SH, Martino R, Anikst V, Xu Z, Mix S, Benjamin R, Banaei N, et al. Rapid diagnosis of tuberculosis from analysis of urine volatile organic compounds. ACS Sens. (2016) 1:852–6. doi: 10.1021/acssensors.6b00309
21. Van Delft J, Meijer F, Van Best J, Van Haeringen N. Permeability of blood-tear barrier to fluorescein and albumin after application of platelet-activating factor to the eye of the guinea pig. Mediat Inflamm. (1997) 6:381–3. doi: 10.1080/09629359791532
22. Taormina CR, Baca JT, Asher SA, Grabowski JJ, Finegold DN. Analysis of tear glucose concentration with electrospray ionization mass spectrometry. J Am Soc Mass Spectrometry. (2007) 18:332–6. doi: 10.1016/j.jasms.2006.10.002
23. Thomas N, Lähdesmäki I, Parviz BA. A contact lens with an integrated lactate sensor. Sensors and Actuators B: Chemical. (2012) 162:128–34. doi: 10.1016/j.snb.2011.12.049
24. Farkas Á, Vámos R, Bajor T, Müllner N, Lázár Á, Hrabá A. Utilization of lacrimal urea assay in the monitoring of hemodialysis: conditions, limitations and lacrimal arginase characterization. Experimental Eye Res. (2003) 76:183–92. doi: 10.1016/S0014-4835(02)00276-2
25. Andoralov V, Shleev S, Arnebrant T, Ruzgas T. Flexible micro (bio) sensors for quantitative analysis of bioanalytes in a nanovolume of human lachrymal liquid. Ana Bioanalytical Chem. (2013) 405:3871–79. doi: 10.1007/s00216-013-6756-x
26. Ohashi Y, Dogru M, Tsubota K. Laboratory findings in tear fluid analysis. Clinica Chimica Acta. (2006) 369:17–28. doi: 10.1016/j.cca.2005.12.035
27. Zhang WM, Natowicz MR. Cerebrospinal fluid lactate and pyruvate concentrations and their ratio. Clinical Biochem. (2013) 46:694–7. doi: 10.1016/j.clinbiochem.2012.11.008
28. Stankova L, Riddle M, Larned J, Burry K, Menashe D, Hart J, Bigley R. Plasma ascorbate concentrations and blood cell dehydroascorbate transport in patients with diabetes mellitus. Metabolism. (1984) 33:347–53. doi: 10.1016/0026-0495(84)90197-5
29. Walker HK, Hall WD, Hurst JW. Peripheral Blood Smear–Clinical Methods: The History, Physical, and Laboratory Examinations. Boston, MA: Butterworths (1990).
30. Banbury LK. Stress Biomarkers in the Tear Film. Southern Cross Uni. PhD thesis, Southern Cross University, Lismore, NSW. (2009). Available online at: https://researchportal.scu.edu.au/esploro/outputs/doctoral/Stress-biomarkers-in-the-tear-film/991012821564402368
31. DiBartola SP. Fluid, Electrolyte, and Acid-Base Disorders in Small Animal Practice. Elsevier Health Science. (2011). doi: 10.1016/B978-1-4377-0654-3.00017-2
32. Walker HK, Hall WD, Hurst JW. Clinical Methods: The History, Physical, and Laboratory Examinations. Boston, MA: Butterworths (1990).
33. Mochizuki M. Analysis of bicarbonate concentration in human blood plasma at steady state in vivo. Yamagata Med J. (2004) 22:9–24. Available online at: http://www2.lib.yamagata-u.ac.jp/kiyou/kiyoum/kiyoum-22-1/image/kiyoum-22-1-009to024.pdf
34. Weglicki WB. Hypomagnesemia and inflammation: clinical and basic aspects. Ann Rev Nutr. (2012) 32:55–71. doi: 10.1146/annurev-nutr-071811-150656
35. Harvey D, Hayes NW, Tighe B. Fibre optics sensors in tear electrolyte analysis: Towards a novel point of care potassium sensor. Contact Lens Anterior Eye. (2012) 35:137–44. doi: 10.1016/j.clae.2012.02.004
36. Panaser A, Tighe BJ. Function of lipids–their fate in contact lens wear: an interpretive review. Contact Lens Anterior Eye. (2012) 35:100–11. doi: 10.1016/j.clae.2012.01.003
37. Thaysen JH, Thorn NA. Excretion of urea, sodium, potassium and chloride in human tears. Am J Physiol-Legacy Content. (1954) 178:160–4. doi: 10.1152/ajplegacy.1954.178.1.160
38. Baca JT, Finegold DN, Asher SA. Tear glucose analysis for the noninvasive detection and monitoring of diabetes mellitus. Ocular Surf . (2007) 5:280–93. doi: 10.1016/S1542-0124(12)70094-0
39. Khuri RN. Device for Determination of Tear Constituents. U.S. Patent No. 5, 352, 411. Washington, DC: U.S. Patent Trademark Office (1994). Available online at: https://patents.google.com/patent/US5352411
40. Domschke A, March WF, Kabilan S, Lowe C. Initial clinical testing of a holographic non-invasive contact lens glucose sensor. Diabetes Technol Therap. (2006) 8:89–93. doi: 10.1089/dia.2006.8.89
41. Zhou L, Beuerman RW. Tear analysis in ocular surface diseases. Progress Retinal Eye Res. (2012) 31:527–50. doi: 10.1016/j.preteyeres.2012.06.002
42. Lebrecht A, Boehm D, Schmit M, Koelbl H, Schwirz RL, Grus FH. Diagnosis of breast cancer by tear proteomic pattern. Cancer Genomics-Proteomics. (2009) 6:177–82. Available online at: https://citeseerx.ist.psu.edu/viewdoc/download?doi=10.1.1.881.4595&rep=rep1&type=pdf
43. Evans V, Vockler C, Friedlander M, Walsh B, Willcox MD. Lacryglobin in human tears, a potential marker for cancer. Clin Exp Ophthalmol. (2001) 29:161–63. doi: 10.1046/j.1442-9071.2001.00408.x
44. Lebrecht A, Boehm D, Schmidt M, Koelbl H, Grus FH. Surface-enhanced laser desorption/ionisation time-of-flight mass spectrometry to detect breast cancer markers in tears and serum. Cancer Genomics-Proteomics. (2009) 6:75–83. Available online at: https://cgp.iiarjournals.org/content/cgp/6/2/75.full.pdf
45. Chen Y, Zhang S, Cui Q, Ni J, Wang X, Cheng X, et al. Microengineered poly(HEMA) hydrogels for wearable contact lens biosensing. Lab Chip. (2020) 20:4205-4214. doi: 10.1039/D0LC00446D
46. Moreddu R, Elsherif M, Adams H, Moschou D, Cordeiro MF, Wolffsohn JS, et al. Integration of paper microfluidic sensors into contact lenses for tear fluid analysis. Lab Chip. (2020) 20:3970–79. doi: 10.1039/D0LC00438C
47. Moreddu R, Elsherif M, Butt H, Vigolo D, Yetisen AK. Contact lenses for continuous corneal temperature monitoring. RSC Advances. (2019) 9:11433–42. doi: 10.1039/C9RA00601J
48. Moreddu R, Nasrollahi V, Kassanos P, Dimov S, Vigolo D, Yetisen AK. Lab-on-a-contact lens platforms fabricated by multi-axis femtosecond laser ablation. Small. (2021) 17:e2102008. doi: 10.1002/smll.202102008
49. Moreddu R, Wolffsohn JS, Vigolo D, Yetisen AK. Laser-inscribed contact lens sensors for the detection of analytes in the tear fluid. Sensors Act B: Chem. (2020) 317. doi: 10.1016/j.snb.2020.128183
50. Riaz RS, Elsherif M, Moreddu R, Rashid I, Hassan MU, Yetisen AK, et al. Anthocyanin-functionalized contact lens sensors for ocular pH monitoring. ACS Omega. (2019) 4:21792–8. doi: 10.1021/acsomega.9b02638
51. Moreddu R, Mahmoodi N, Kassanos P, Vigolo D, Mendes PM, Yetisen AK. Stretchable nanostructures as optomechanical strain sensors for ophthalmic applications. ACS Applied Polymer Materials. (2021) 3:5416–24. doi: 10.1021/acsapm.1c00703
52. Cope JR, Collier SA, Nethercut H, Jones JM, Yates K, Yoder JS. Risk behaviors for contact lens–related eye infections among adults and adolescents—United States, 2016. MMWR. Morbidity Mortal Weekly Report. (2017) 66:841. doi: 10.15585/mmwr.mm6632a2
53. Ma X, Ahadian S, Liu S, Zhang J, Liu S, Cao T, et al. Smart contact lenses for biosensing applications. Adv Intell Sys. (2021) 3:2000263. doi: 10.1002/aisy.202000263
54. Klonoff DC. Overview of fluorescence glucose sensing: A technology with a bright future. J Diabetes Sci Technol. (2012) 6:1242–50. doi: 10.1177/193229681200600602
55. Williams R, Bridges J. Fluorescence of solutions: a review. J Clin Pathol. (1964) 17:371. doi: 10.1136/jcp.17.4.371
56. Kaláb P, Soderholm J. The design of Förster (fluorescence) resonance energy transfer (FRET)-based molecular sensors for Ran GTPase. Methods. (2010) 51:220–32. doi: 10.1016/j.ymeth.2010.01.022
57. Clapp AR, Medintz IL, Mattoussi H. Förster resonance energy transfer investigations using quantum-dot fluorophores. ChemPhysChem. (2006) 7:47–57. doi: 10.1002/cphc.200500217
58. Springsteen G, Wang B. Alizarin Red S. as a general optical reporter for studying the binding of boronic acids with carbohydrates. Chemical Comm. (2001) 17:1608–9. doi: 10.1039/b104895n
59. Cao H, Diaz DI, DiCesare N, Lakowicz JR, Heagy MD. Monoboronic acid sensor that displays anomalous fluorescence sensitivity to glucose. Organic Letters. (2002) 4:1503–5. doi: 10.1021/ol025723x
60. Badugu R, Lakowicz JR, Geddes CD. A glucose sensing contact lens: A non-invasive technique for continuous physiological glucose monitoring. J Fluorescence. (2003) 13:371–4. doi: 10.1023/A:1026103804104
61. Badugu R, Lakowicz JR, Geddes CD. Ophthalmic glucose sensing: a novel monosaccharide sensing disposable and colorless contact lens. Analyst. (2004) 129:516–21. doi: 10.1039/b314463c
62. Badugu R, Reece EA, Lakowicz JR. Glucose-sensitive silicone hydrogel contact lens toward tear glucose monitoring. J Biomed Opt. (2018) 23:057005. doi: 10.1117/1.JBO.23.5.057005
63. March W, Lazzaro D, Rastogi S. Fluorescent measurement in the non-invasive contact lens glucose sensor. Diabetes Technol Therap. (2006) 8:312–7. doi: 10.1089/dia.2006.8.312
64. Zhang J, Hodge WG. Contact Lens Integrated With a Biosensor for the Detection of Glucose Other Components in Tears. U. S. Patent No. 8, 385, 998. Washington, DC: U.S. Patent Trademark Office (2013). Available online at: https://patents.google.com/patent/US8385998B2/en
65. Badugu R, Lakowicz JR, Geddes CD. Noninvasive continuous monitoring of physiological glucose using a monosaccharide-sensing contact lens. Analytical Chem. (2004) 76:610–8. doi: 10.1021/ac0303721
66. Badugu R, Lakowicz JR, Geddes CD. A glucose-sensing contact lens: from bench top to patient. Current Opinion Biotechnol. (2005) 16:100–07. doi: 10.1016/j.copbio.2004.12.007
67. Deng M, Song G, Zhong K, Wang Z, Xia X, Tian Y. Wearable fluorescent contact lenses for monitoring glucose via a smartphone. Sens. Actuators B Chem. (2022) 352:131067. doi: 10.1016/j.snb.2021.131067
68. Badugu R, Jeng BH, Reece EA, Lakowicz JR. Contact lens to measure individual ion concentrations in tears and applications to dry eye disease. Analytical Biochem. (2018) 542:84–94. doi: 10.1016/j.ab.2017.11.014
69. Yang W, Yan J, Springsteen G, Deeter S, Wang B. A novel type of fluorescent boronic acid that shows large fluorescence intensity changes upon binding with a carbohydrate in aqueous solution at physiological pH. Bioorg Med Chem Lett. (2003) 13:1019–22. doi: 10.1016/S0960-894X(03)00086-6
70. James TD, Sandanayake KS, Shinkai S. A glucose-selective molecular fluorescence sensor. Angewandte Chemie Int Ed Eng. (1994) 33:2207–9. doi: 10.1002/anie.199422071
71. Lee H, Hong YJ, Baik S, Hyeon T, Kim DH. Enzyme-based glucose sensor: from invasive to wearable device. Adv Healthcare Mat. (2018) 7:1701150. doi: 10.1002/adhm.201701150
72. Dungchai W, Chailapakul O, Henry CS. Electrochemical detection for paper-based microfluidics. Ana Chem. (2009) 81:5821–6. doi: 10.1021/ac9007573
73. Nie Z, Deiss F, Liu X, Akbulut O, Whitesides GM. Integration of paper-based microfluidic devices with commercial electrochemical readers. Lab Chip. (2010) 10:3163–9. doi: 10.1039/c0lc00237b
74. Rattanarat P, Dungchai W, Siangproh W, Chailapakul O, Henry CS. Sodium dodecyl sulfate-modified electrochemical paper-based analytical device for determination of dopamine levels in biological samples. Analytica Chimica Acta. (2012) 744:1–7. doi: 10.1016/j.aca.2012.07.003
75. Shiroma LY, Santhiago M, Gobbi AL, Kubota LT. Separation and electrochemical detection of paracetamol and 4-aminophenol in a paper-based microfluidic device. Analytica Chimica Acta. (2012) 725:44–50. doi: 10.1016/j.aca.2012.03.011
76. Yao H, Shum AJ, Cowan M, Lähdesmäki I, Parviz BA. A contact lens with embedded sensor for monitoring tear glucose level. Biosens Bioelect. (2011) 26:3290–6. doi: 10.1016/j.bios.2010.12.042
77. Chu MX, Miyajima K, Takahashi D, Arakawa T, Sano K, Sawada, et al. Soft contact lens biosensor for in situ monitoring of tear glucose as non-invasive blood sugar assessment. Talanta. (2011) 83:960–5. doi: 10.1016/j.talanta.2010.10.055
78. Chu M, Shirai T, Takahashi D, Arakawa T, Kudo H, Sano K, et al. Biomedical soft contact-lens sensor for in situ ocular biomonitoring of tear contents. Biomed Microdev. (2011) 13:603–11. doi: 10.1007/s10544-011-9530-x
79. Kim J, Kim M, Lee MS, Kim K, Ji S, Kim YT, et al. Wearable smart sensor systems integrated on soft contact lenses for wireless ocular diagnostics. Nature Comm. (2017) 8:14997. doi: 10.1038/ncomms14997
80. Park J, Kim J, Kim SY, Cheong WH, Jang J, Park YG, et al. Soft, smart contact lenses with integrations of wireless circuits, glucose sensors, and displays. Sci Adv. (2018) 4:eaap9841. doi: 10.1126/sciadv.aap9841
81. Jeon C, Koo J, Lee K, Kim SK, Hahn SK, Kim B, et al. in A 143nW Glucose-Monitoring Smart Contact Lens Ic With a Dual-Mode Transmitter for Wireless-Powered Backscattering and RF-Radiated Transmission Using a Single Loop Antenna, 2019 Symposium on VLSI Circuits, IEEE. (2019) pp C294–5. doi: 10.23919/VLSIC.2019.8777984
82. Hayashi K, Arata S, Xu G, Murakami S, Bui CD, Kobayashi A, Niitsu KA. 385× 385μm2 0.165 V 0.27 nW fully-integrated supply-modulated ook transmitter in 65nm cmos for glasses-free, self-powered, and fuel-cell-embedded continuous glucose monitoring contact lens. IEICE Transactions Electr. (2019) 102:590–4. doi: 10.1587/transele.2018CTS0005
83. Chen G, Yu X, Wang Y, Quan TM, Matsuyama N, Tsujimura T, et al. In A 0.5 mm 2 Ambient Light-Driven Solar Cell-Powered Biofuel Cell-Input Biosensing System with LED Driving for Stand-Alone RF-Less Continuous Glucose Monitoring Contact Lens, 2022 27th Asia and South Pacific Design Automation Conference (ASP-DAC). Taipei: IEEE (2022). 1–2. doi: 10.1109/ASP-DAC52403.2022.9712523
84. Keum DH, Kim SK, Koo J, Lee GH, Jeon C, Mok JW, et al. Wireless smart contact lens for diabetic diagnosis and therapy. Sci Adv. (2020) 6:eaba3252. doi: 10.1126/sciadv.aba3252
85. Guo S, Wu K, Li C, Wang H, Sun Z, Xi D, et al. Integrated contact lens sensor system based on multifunctional ultrathin MoS2 transistors. Matter. (2021) 4:969–85. doi: 10.1016/j.matt.2020.12.002
86. Tseng R, Chen CC, Hsu SM, Chuang HS. Contact-lens biosensors. Sensors. (2018) 18:2651. doi: 10.3390/s18082651
87. Park S, Boo H, Chung TD. Electrochemical non-enzymatic glucose sensors. Analytica Chimica Acta. (2006) 556:46–57. doi: 10.1016/j.aca.2005.05.080
88. Saeedi E, Kim S, Parviz BA. Self-assembled crystalline semiconductor optoelectronics on glass and plastic. J Micromech Microenginee. (2008) 18:075019. doi: 10.1088/0960-1317/18/7/075019
89. Leonardi M, Leuenberger P, Bertrand D, Bertsch A, Renaud P. First steps toward noninvasive intraocular pressure monitoring with a sensing contact lens. Investigative Ophthalmol Visual Sci. (2004) 45:3113–7. doi: 10.1167/iovs.04-0015
90. Lorand JP. Edwards JO. Polyol complexes and structure of the benzeneboronate ion. J Org Chem. (1959) 24:769–74. doi: 10.1021/jo01088a011
91. Kabilan S, Marshall AJ, Sartain FK, Lee MC, Hussain A, Yang X, et al. Holographic glucose sensors. Biosensors Bioelect. (2005) 20:1602–10. doi: 10.1016/j.bios.2004.07.005
92. Kabilan S, Blyth J, Lee M, Marshall A, Hussain A, Yang XP, Lowe C. Glucose-sensitive holographic sensors. J Mol Recog. (2004) 17:162–6. doi: 10.1002/jmr.663
93. Ward CJ, Patel P, James TD. Molecular color sensors for monosaccharides. Org Letters. (2002) 4:477–9. doi: 10.1021/ol016923w
94. Asher SA, Alexeev VL, Goponenko AV, Sharma AC, Lednev IK, Wilcox CS, et al. Photonic crystal carbohydrate sensors: low ionic strength sugar sensing. J Am Chem Soc. (2003) 125:3322–9. doi: 10.1021/ja021037h
95. Ravve A. Principles of Polymer Chemistry: New York, NY: Springer Science & Business Media. (2013). doi: 10.1007/978-1-4614-2212-9
96. Domschke A, Kabilan S, Anand R, Caines M, Fetter D, Griffith P, et al. in Holographic Sensors in Contact Lenses for Minimally-Invasive Glucose Measurements, SENSORS. Vienna: IEEE (2004). p. 1320–1323.
97. Alexeev VL, Das S, Finegold DN, Asher SA. Photonic crystal glucose-sensing material for noninvasive monitoring of glucose in tear fluid. Clin Chem. (2004) 50:2353–60. doi: 10.1373/clinchem.2004.039701
98. Ben-Moshe M, Alexeev VL, Asher SA. Fast responsive crystalline colloidal array photonic crystal glucose sensors. Analytical Chem. (2006) 78:5149–57. doi: 10.1021/ac060643i
99. Hu Y, Jiang X, Zhang L, Fan J, Wu W. Construction of near-infrared photonic crystal glucose-sensing materials for ratiometric sensing of glucose in tears. Biosensors Bioelectr. (2013) 48:94–9. doi: 10.1016/j.bios.2013.03.082
100. Zhang C, Cano GG, Braun PV. Linear and fast hydrogel glucose sensor materials enabled by volume resetting agents. Adv Mat. (2014) 26:5678–83. doi: 10.1002/adma.201401710
101. Updike SJ, Shults MC, Gilligan BJ, Rhodes RK. A subcutaneous glucose sensor with improved longevity, dynamic range, and stability of calibration. Diab Care. (2000) 23:208–14. doi: 10.2337/diacare.23.2.208
102. Koschinsky T, Heinemann L. Sensors for glucose monitoring: technical and clinical aspects. Diab/Metabol Rese Rev. (2001) 17:113–23. doi: 10.1002/dmrr.188
103. Xue F, Meng Z, Wang F, Wang Q, Xue M, Xu Z. A 2-D photonic crystal hydrogel for selective sensing of glucose. J Materials Chem A. (2014) 2:9559–65. doi: 10.1039/C4TA01031K
104. Chen C, Dong ZQ, Shen JH, Chen HW, Zhu YH, Zhu ZG. 2D photonic crystal hydrogel sensor for tear glucose monitoring. ACS Omega. (2018) 3:3211–7. doi: 10.1021/acsomega.7b02046
105. Elsherif M, Hassan MU, Yetisen AK, Butt H. Wearable contact lens biosensors for continuous glucose monitoring using smartphones. ACS Nano. (2018) 12:5452–62. doi: 10.1021/acsnano.8b00829
106. Elsherif M, Hassan MU, Yetisen AK, Butt H. Glucose sensing with phenylboronic acid functionalized hydrogel-based optical diffusers. ACS Nano. (2018) 12:2283–91. doi: 10.1021/acsnano.7b07082
Keywords: contact lenses, glucose sensors, enzymes, phenylboronic acid, fluorescence, light diffraction
Citation: Elsherif M, Moreddu R, Alam F, Salih AE, Ahmed I and Butt H (2022) Wearable Smart Contact Lenses for Continual Glucose Monitoring: A Review. Front. Med. 9:858784. doi: 10.3389/fmed.2022.858784
Received: 20 January 2022; Accepted: 10 March 2022;
Published: 04 April 2022.
Edited by:
Michele Lanza, University of Campania Luigi Vanvitelli, ItalyReviewed by:
Tao Wu, Shanghai University of Traditional Chinese Medicine, ChinaMainul Haque, National Defence University of Malaysia, Malaysia
Viney Lather, Amity University, India
Copyright © 2022 Elsherif, Moreddu, Alam, Salih, Ahmed and Butt. This is an open-access article distributed under the terms of the Creative Commons Attribution License (CC BY). The use, distribution or reproduction in other forums is permitted, provided the original author(s) and the copyright owner(s) are credited and that the original publication in this journal is cited, in accordance with accepted academic practice. No use, distribution or reproduction is permitted which does not comply with these terms.
*Correspondence: Mohamed Elsherif, ZWxzaGVyaWZtb2hhbWVkMTA5QGdtYWlsLmNvbQ==; Haider Butt, aGFpZGVyLmJ1dHRAa3UuYWMuYWU=