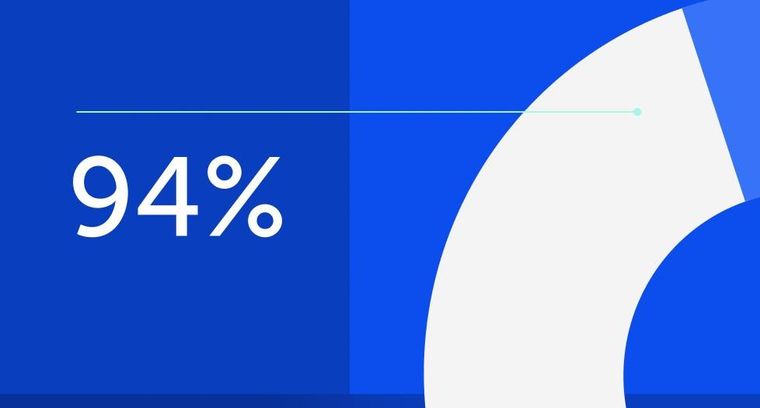
94% of researchers rate our articles as excellent or good
Learn more about the work of our research integrity team to safeguard the quality of each article we publish.
Find out more
MINI REVIEW article
Front. Med., 18 April 2022
Sec. Precision Medicine
Volume 9 - 2022 | https://doi.org/10.3389/fmed.2022.829304
The vitamin K antagonist is a commonly prescribed effective oral anticoagulant with a narrow therapeutic range, and the dose requirements for different patients varied greatly. In recent years, studies on human intestinal microbiome have provided many valuable insights into disease development and drug reactions. A lot of studies indicated the potential relationship between microbiome and the vitamin K antagonist. Vitamin K is absorbed by the gut, and the intestinal bacteria are a major source of vitamin K in human body. A combined use of the vitamin K antagonist and antibiotics may result in an increase in INR, thus elevating the risk of bleeding, while vitamin K supplementation can improve stability of anticoagulation for oral vitamin K antagonist treatment. Recently, how intestinal bacteria affect the response of the vitamin K antagonist remains unclear. In this review, we reviewed the research, focusing on the physiology of vitamin K in the anticoagulation treatment, and investigated the potential pathways of intestinal bacteria affecting the reaction of the vitamin K antagonist.
Vitamin K antagonist (VKA) has been found as one of the most extensively used first-line oral anticoagulants (1). Since VKA has a narrow therapeutic range, the international normalized ratio (INR) should be monitored when VKA is used, and the dose should be regulated in time to avoid adverse reactions (e.g., bleeding and embolism). However, VKA still has a high risk of adverse reactions. Statistics suggest that warfarin (the most commonly used VKA) has led to the largest number of emergency department visits for adverse drug events in the United States (2).
The individual dose difference of VKA has been considered the major contributors to the adverse reactions. Existing studies have found that VKA dose requirements are dependent on genetics and clinical factors (3–6). Based on the above factors, researchers have established the pharmacogenomics-guided dosing algorithms for VKA, whereas the clinical value of the above algorithms has been controversial (7–9). Retrospective studies have found that the pharmacogenomics-guided dosing algorithm has a higher VKA stable dose predictive power than the fixed-dose approach and the clinical algorithm (4), while the prospective study results have not yet been satisfying (10–14). The dissatisfactory clinical effect of VKA dosing algorithm primarily arises from the insufficient contribution of the factors included in the algorithm, thus leading to the low prediction accuracy of the algorithm. Therefore, the potential mechanism of VKA dose change should be studied in depth.
Over the past two decades, researchers have primarily identified the possible factors of VKA response at the genetic level, while the effect arising from other levels is ignored. However, except for polymorphisms in CYP2C9, VKORC1, CYP4F2, and GGCX, few genetic factors can be included in the dosing algorithm (15). To find more factors and further optimize the VKA dosing algorithm, more attention should be paid to the non-genetic level. As reported by numerous studies, VKA reactions are significantly correlated with gut microbiota. The major effect of VKA is to antagonize vitamin K (VK), which is absorbed by the intestine. Moreover, menaquinone, a vital type of VK for the human body, is mainly synthesized by intestinal bacteria. A combined use of VKA and antibiotics may result in an increase in INR, thus elevating the risk of bleeding (16–20). Accordingly, there may be a potential correlation between intestinal microbiome and VKA response. Through the research on pharmacomicrobiomics of VKA, a new thought may be provided to clarify the mechanism of individual variability of VKA response. In this review, the research focusing on the physiology of VK in the anticoagulation treatment was reviewed, and the potential pathways of intestinal bacteria affecting the reaction of VKA were investigated. Thus, this review is expected to lay a new scientific basis for improving the individualized medication of VKA.
In this narrative review, the focus was placed on the available in vivo and in vitro studies, as well as reviews. Medical subject headings (MeSH), text word, and Boolean calculation search were conducted on the Pubmed database. The retrieval type applied in the database consisted of [(“vitamin K” OR “phylloquinone” OR “menaquinone”) AND (“VKA” OR “warfarin” OR “acenocoumarol” OR “phenprocoumon” OR “INR”)] OR [(“vitamin K” OR “phylloquinone” OR “menaquinone”) AND (“intestin*” OR “gut”) AND (“bacteria” OR “flora” OR “microbiome” OR “microbiota”)] OR [(“VKA” OR “warfarin” OR “acenocoumarol” OR “phenprocoumon” OR “INR”) AND (“intestin*” OR “gut”) AND (“bacteria” OR “flora” OR “microbiome” OR “microbiota”)]. Furthermore, related publications in the reference of the eligible publications and the mechanism research of intestinal bacteria were included as well.
Vitamin K refers to a group of liposoluble vitamin with a wide variety of biology activities, which include playing a certain role in several coagulation factor syntheses, participation in osteocalcin carboxylation, promoting the transition of osteoblasts to osteocytes, limiting osteoclastogenesis and preventing vascular calcifications (21, 22). Naturally occurring VK primarily has two forms, including phylloquinone and menaquinone. All types of VK have a 2-methyl-1,4-naphthoquinone structure, which is termed menadione. Phylloquinone contains a phytyl side chain, consisting of 4 prenyl units, while menaquinone covers an isoprenoid structure side chain with different lengths and degrees of saturation at the 3-position (23). Phylloquinone of humans is largely derived from the diet (24). In accordance with the number of isoprenoid units, menaquinone is termed menaquinone-n, and 12 types of menaquinone-n (MK-4 to MK-15) have been generally reported (25). MK-4 has been found as the most common menaquinone in humans, as well as the only menaquinone converted by phylloquinone (26). The other menaquinone is synthesized by some obligate and facultative anaerobic bacteria (27). Except for MK-4, the other menaquinone in humans is mostly synthesized by intestinal bacteria.
The concentration of VK in plasma and feces has been generally evaluated to indicate the status of vitamin K in humans. In plasma, the concentrations of phylloquinone, MK-4, and MK-7 have been frequently quantified, while the other menaquinone isoforms have been rarely investigated due to the low plasma concentration (28). In feces, almost all types of VK can be quantified, and the concentration level is significantly higher than that in plasma. In humans, there are significant population and individual differences of VK concentration levels. The mean plasma concentrations of phylloquinone have been reported to range from.22 to 8.88 nmol/L. In most studies, the concentrations were lower than 2 nmol/L (29). Diet and medication have a significant effect on the in vivo levels of VK.
Vitamin K is of critical significance to VK-dependent clotting factors, which include prohemorrhagic factors (II, VII, IX, and X) and antithrombotic factors (protein C and protein S) that work together to clot the blood (30). VKA is capable of inhibiting the activity of vitamin K epoxide reductase (VKOR) and hindering the production of VKH2 (an active form of VK) (31, 32). Under a normal condition of the VK cycle, the gamma-glutamyl carboxylase converts glutamate residues into γ-carboxyglutamate residues through the cofactor VKH2, while facilitating the activation of VK-dependent proteins (26). During the carboxylation reaction, VKH2 is oxidized into vitamin K 2,3-epoxide (an inactive form of VK). Subsequently, the inactive VK is reduced to VKH2 by VKOR to complete the cycle (Figure 1). Impacted by the lower half-life of proteins C and S compared with factors II, VII, IX, and X, the patient is placed in a pro-thrombotic state and then converted into an anticoagulant state when receiving VKA therapy (30).
Figure 1. The source of vitamin K and the pharmacodynamic pathway of vitamin K antagonist. MKn, menaquinone, n is the number of isoprenoid units; GGCX, the gamma-glutamyl carboxylase enzyme; VKOR, vitamin K epoxide reductase. The figure was drawing by Microsoft PowerPoint 2016, and the materials were provided by Figdraw (www.figdraw.com).
The change of VK levels has a significant effect on the anticoagulant effect of VKA. A clinical study in Japanese population reported that the plasma MK-4 concentration showed a negative correlation with the warfarin sensitivity index (the ration of INR and the plasma-unbound concentration of each warfarin enantiomer) (33). Another study in European population found that the plasma phylloquinone concentration in the group with a high dose of warfarin was significantly higher than that in the group with a low dose of warfarin (34). In addition, daily VK intake has an effect on the VKA response. Low daily VK intake increases the risk of unstable anticoagulation control in patients treated with VKA, while daily VK supplementation improves anticoagulation control (35–42). Sconce et al. found that, compared with patients with a stable control of anticoagulation, patients with an unstable control had a poor dietary intake of VK (43), and patients receiving 150-μg phylloquinone one time daily had a better anticoagulation control than those patients treated with placebo (38). Menaquinone supplementation has also been considered a way to improve the anticoagulant management for VKA. MK-7 has been the most widely studied menaquinone isoform in clinical practice (39, 44). MK-7 has much more stable serum levels than phylloquinone because of its long elimination half-life (44). Low-dose supplements of MK-7 could also improve the anticoagulant management in patients (39). However, not all patients benefit from VK supplementation, and the dose supplementation of VK improves the stability of VKA therapy in patients with unstable INR only (45).
Besides the dose of VK intake, the stability of VK intake is another vital factor in anticoagulation stability (46). Sudden changes of VK intake may elevate the risk of potential bleeding and thromboembolic complications during VKA therapy (46). Two case reports described that changes in the dietary intake of VK would lead to diffuse bruising or thrombosis, and even result in myocardial infarction (47, 48). Thus, clinicians have generally advised patients who need long-term anticoagulation therapy to control the intake of food rich in VK to ensure the anticoagulant stability of VKA.
There are two ways for intestinal bacteria to synthesize VK, including the Men pathway and the Mqn pathway. In the Men pathway, 2-demethylmenaquinone serves as a precursor for menaquinone synthesis, while the Mqn pathway is completed through futalosine (49–51). Nine types of enzymes (MenA-MenI) are involved in the Men pathway, while the Mqn pathway has five (MqnA-MqnE). In the Human Microbiome Project, 254 genomes of intestinal bacteria have been sequenced. About 24 genomes of Firmicutes and Proteobacteria have a complete Men pathway (with all MenA-MenI encoded genes), and 10 genomes of Firmicutes, Proteobacteria, and Bacteroidetes have a complete Mqn pathway (52).
In mammals, different intestinal bacteria have genes encoding different enzymes, thus producing different menaquinone isoforms. For instance, Eubacterium lentum synthesizes MK-6, Veillonella primarily produces MK-7, the Escherichia coli mainly produces MK-8, while MK-10 and MK-11 are the major isoforms in Bacteroides (53–56). As a result, different compositions of intestinal microbiome produce menaquinone isoforms at different concentrations. According to a recent shotgun metagenomic sequencing study, McCann et al. found that the elderly individuals could be assigned into 4 clusters in accordance with a similar presence and prevalence of menaquinone biosynthesis genes (57). Significant differences in menaquinone isoforms concentration in fecal were found among 4 clusters, except for MK-4, MK-6, and MK-7 (57). It is noteworthy that different menaquinone isoforms may exhibit different biological functions. The total concentrations of menaquinone do not covary with cognition in elderly individuals, whereas certain menaquinone isoforms (e.g., MK-12 and MK-13) are positively correlated with cognition (57).
A considerable number of bacteria live in human intestines, especially in the colon (58, 59). Gut microbiome participates in various biological processes in the human body, which cover the development of immune system, the regulation of nervous system, and the biosynthesis of vitamins (vitamins K and B) (60–62). Moreover, the gut microbiome has been confirmed to interact with liver via the intestine-liver axis, and it is reported to be correlated with cirrhosis, liver’s antitumor immune function, etc. (63–65). The above results indicated a potential correlation between microbiome and liver function. Since VKA is mainly metabolized and has the VK antagonistic effect in liver, the intestinal bacteria may also have an effect on the anticoagulant response of VKA.
Some studies have reported the indirect correlation between intestinal bacteria and the anticoagulant response of VKA. Numerous antibiotics (e.g., ciprofloxacin, levofloxacin, metronidazole, and fluconazole) have been found to significantly affect the anticoagulant effect of VKA (16, 19, 66, 67). As compared with patients that do not use antibiotics, the patients using antibiotics during warfarin therapy have a significantly higher risk of hemorrhage (16–19). Furthermore, small intestine bacterial overgrowth (SIBO) affects the anticoagulant therapy of warfarin (68). Giuliano et al. reported that patients with SIBO would require a higher warfarin dose compared with patients without SIBO, whereas Emidio et al. achieved the opposite results (34, 69). In human gut, some bacteria are correlated with the synthesis of menaquinone, some bacteria would cause chronic damage of the small bowel mucosa, and some bacteria would impair the absorption of bile acids and VK (69). Accordingly, different research results may be dependent on the types of bacteria abnormal overgrowth in small intestines.
At present, only two studies reported the direct correlation between intestinal bacteria and the anticoagulant response of VKA. In an in vitro study, the researchers tested the relationship between 76 intestinal bacteria and 271 drugs, and they found that nearly 2/3 of the 271 drugs could be metabolized by one or more bacteria (70). According to the results, warfarin was found to be metabolized by Bacteroides vulgatus, Collinsella aerofaciens, Anaerotruncus colihominis, Edwardsiell atarda, and Bacteroides fragilis in vitro. In another study, an association analysis was conducted between intestinal bacteria and warfarin response in patients with heart valve replacement. They found that, compared with the group with a low dose of warfarin, the relative abundance of genus Escherichia-Shigella was significantly higher in the group with a high dose of warfarin, while the genus Enterococcus was enriched in the low dose group (71). Further analysis indicated that the amount of menaquinone synthesized by intestinal bacteria in the group with a high dose of warfarin was significantly higher than that in the low dose group.
Besides directly affecting the anticoagulant effect of VKA by participating in the coagulation pathway, menaquinone can indirectly affect the efficacy of VKA through pregnane X receptor (PXR). PXR is a nuclear receptor highly expressed in liver and intestines. in vitro studies have confirmed that menaquinone could activate PXR (72–75). PXR activation would upregulate the expression of cholesterol transporter Niemann-Pick C1-Like 1 (NPC1L1) in intestinal (76). CD36 refers to a known PXR target gene. Deficiency of PXR decreases CD36 expression in mice, while PXR activation upregulates CD36 expression (77–79). Another in vitro study reported that PXR activation could downregulate the expression of SR-BI (80). All of the above three proteins play vital roles in VK absorption in intestines (81, 82). However, since PXR activation has different effects on the above three proteins, how menaquinone affects the absorption of VK in vivo should be further investigated. Besides, activation of PXR could upregulate the expression of CYP2C9 in liver (83, 84). CYP2C9 refers to the main metabolic enzyme of VKA, so the concentration of menaquinone in the liver may affect the metabolism of VKA, thus having an influence on the anticoagulant effect.
In general, intestinal bacteria may affect the VKA response by metabolizing the drug in intestinal or regulating the synthesis of menaquinone. Nevertheless, due to the high bioavailability of VKA (nearly 100%), the metabolism of VKA in intestines is negligible (85, 86).
Another possible approach to intestinal bacteria affecting the VKA response may be through short-chain fatty acids (SCFAs). SCFAs have been reported as the major type of intestinal bacteria metabolites and derived primarily from intestinal bacteria fermentation of dietary fibers. SCFAs play pivotal roles in many biological processes, consisting of host energy metabolism, cell proliferation, and immune system regulation (87, 88). The main three types of SCFAs produced by intestinal bacteria include butyrate, propionic, and acetic acids, and butyrate has been the most extensively studied SCFAs type.
Intestinal disorders might affect the absorption of VKA and VK and then influence the VKA response. Butyrate serves as a preferred fuel for colonocytes (89). The deficiency of butyrate will lead to nutritional deficiency of colonic epithelial cells and cause colitis (90). Moreover, butyrate protects intestinal mucosa by upregulating the secretion of intestinal mucins and trefoil factors (91). As a result, butyrate plays a vital role in intestinal homeostasis maintaining, which is correlated with the VKA response. Moreover, existing studies found that butyrate could reduce the absorption of dietary cholesterol by downregulating the expression of NPC1L1 in the intestines (92, 93). NPC1L1, a key protein affecting cholesterol uptake, refers to a therapeutic target of dyslipidemia, while ezetimibe serves as a selective inhibitor of NPC1L1. Researchers have found that a combined use of ezetimibe could increase the anticoagulant activity of warfarin (94). Further studies showed that NPC1L1 is a regulatory factor of intestinal phylloquinone absorption (82, 95, 96). In vitro experiments found that overexpression of NPC1L1 significantly increased the absorption of phylloquinone in the intestinal cell line (82). In NPC1L1 gene knockout mice, the concentration of VK is significantly decreased in liver and plasma (82). Moreover, butyrate refers to a well-known Peroxisome Proliferator-Activated Receptor (PPAR) agonist, and activation of PPARα and PPARβ will decrease the expression of NPC1L1 in intestines (92, 97–99). The above results suggest that butyrate may affect the VKA response by regulating VK absorption.
Another metabolite that may affect VKA response is lithocholic acid, which is a secondary bile acid produced by intestinal bacteria (100, 101). Same as menaquinone, lithocholic acid could activate PXR (72). Moreover, lithocholic acid is also vitamin D receptor (VDR) agonists (102, 103). Consistent with PXR, VDR is a drug-activated nuclear receptor, and has been shown to mediate the transcriptional upregulation of CYP2C genes (104). Thus, lithocholic acid can regulate CYP2C9 in liver, and then affect the anticoagulant effect of VKA. Figure 2 shows the possible mechanism of how intestinal microbiome affects the anticoagulant response of VKA.
Figure 2. A hypothesis diagram of intestinal microbiome affects the anticoagulant response of vitamin K antagonist VKA, vitamin K inhibitors; PPARα, the peroxisome proliferator-activated receptor α; PPARβ, the peroxisome proliferator-activated receptor β; PXR, pregnane X receptor; VDR, vitamin D receptor; NPC1L1: NPC1like intracellular cholesterol transporter 1; SR-BI: scavenger receptor Class B Member 1; CYP2C9, cytochrome P450 Family 2 Subfamily C Member 9. The figure was drawing by Microsoft PowerPoint 2016, and the materials were provided by Figdraw (www.figdraw.com).
In this review, the correlations between VKA, VK and intestinal bacteria were investigated. VK has been found as a vital cofactor in the activation of clotting factors. Phylloquinone and menaquinone are found as two major types of VK. The intestinal bacteria may have an effect on the VKA response by producing menaquinone, affecting VK absorption, regulating liver function, etc. Based on the above results, we speculated that there might be significant correlations between VK, intestinal bacteria, and the VKA response. However, the underlying mechanisms still need to be clarified. Whether intestinal bacteria have an effect on the pharmacokinetics and pharmacodynamics of VKA in vivo remains unclear, so more pieces of pharmacomicrobiomics research should be conducted.
The current research results revealed that intestinal bacteria could potentially become a warfarin response prediction biomarker. Integration of intestinal bacteria and genetic and clinical factors may improve the accuracy of warfarin dose prediction algorithm and help predict the INR stability of patients after taking warfarin. Intervention of intestinal microbiome may improve the stability of INR.
In this review, the only focus was placed on the correlation between intestinal bacteria and VKA. Impacted by the extensive role of intestinal microbiome, intestinal bacteria may affect the efficacy of other anticoagulants by directly metabolizing drugs, bioaccumulation drugs, or other ways (70, 105). However, the current pharmacomicrobiomics studies of anticoagulants have been rare. To investigate the effect arising from intestinal bacteria on anticoagulant drug response, more research should be conducted in this field. Moreover, although VK status in human body is significantly correlated with VKA response, we can only detect VK concentration in blood and feces for most patients with anticoagulant at present; it is hard to evaluate the systemic VK status and the pleiotropic effects of VK in the body. In the future, novel evaluation methods for global VK status and effects should be developed.
XL and HY designed the outline of this manuscript and reviewed and edited the manuscript. YC and HY were the major contributors to the writing of the initial manuscript. HZ, W-HH, and X-HC designed the figures. DL, Y-JL, and Si-Zhao prepared the figures. H-HZ and F-YL selected and organized the references. WZ reviewed and amended the references. All authors contributed to the article and approved the submitted version.
This review was supported by the Chinese National Science Foundation (No. 81803583), the Hunan Provincial Natural Science Foundation of China (2019JJ50854 and 2021JJ31074), the Open Fund Project of Hunan Universities Innovation Platform (18K006), the Project Program of National Clinical Research Center for Geriatric Disorders (Xiangya Hospital, Grant No. 2020LNJJ06), and the National Key Research and Development Program of China (No. 2021YFA1301203).
The authors declare that the research was conducted in the absence of any commercial or financial relationships that could be construed as a potential conflict of interest.
All claims expressed in this article are solely those of the authors and do not necessarily represent those of their affiliated organizations, or those of the publisher, the editors and the reviewers. Any product that may be evaluated in this article, or claim that may be made by its manufacturer, is not guaranteed or endorsed by the publisher.
We thank Figdraw (www.figdraw.com) for providing the material of the figures.
1. Ho KH, van Hove M, Leng G. Trends in anticoagulant prescribing: a review of local policies in English primary care. BMC Health Serv Res. (2020) 20:279. doi: 10.1186/s12913-020-5058-1
2. Shehab N, Lovegrove MC, Geller AI, Rose KO, Weidle NJ, Budnitz DS, et al. Visits for outpatient adverse drug events, 2013-2014. JAMA. (2016) 316:2115–25. doi: 10.1001/jama.2016.16201
3. Zhang JE, Klein K, Jorgensen AL, Francis B, Alfirevic A, Bourgeois S, et al. Effect of genetic variability in the CYP4F2, CYP4F11, and CYP4F12 genes on liver mRNA levels and warfarin response. Front Pharmacol. (2017) 8:323. doi: 10.3389/fphar.2017.00323
4. International Warfarin Pharmacogenetics Consortium, Klein TE, Altman RB, Eriksson N, Gage BF, Kimmel SE, et al. Estimation of the warfarin dose with clinical and pharmacogenetic data. N Engl J Med. (2009) 360:753–64. doi: 10.1056/nejmoa0809329
5. Liu J, Jiang HH, Wu DK, Zhou YX, Ye HM, Li X, et al. Effect of gene polymorphims on the warfarin treatment at initial stage. Pharmacogenomics J. (2017) 17:47–52. doi: 10.1038/tpj.2015.81
6. Li X, Liu R, Yan H, Tang J, Yin JY, Mao XY, et al. Effect of CYP2C9-VKORC1 interaction on warfarin stable dosage and its predictive algorithm. J Clin Pharmacol. (2015) 55:251–7. doi: 10.1002/jcph.392
7. Li X, Li D, Wu JC, Liu ZQ, Zhou HH, Yin JY. Precision dosing of warfarin: open questions and strategies. Pharmacogenomics J. (2019) 19:219–29. doi: 10.1038/s41397-019-0083-3
8. Emery JD. Pharmacogenomic testing and warfarin: what evidence has the GIFT trial provided? JAMA. (2017) 318:1110–2. doi: 10.1001/jama.2017.11465
9. Kimmel SE. Warfarin pharmacogenomics: current best evidence. J Thromb Haemost. (2015) 13(Suppl. 1):S266–71. doi: 10.1111/jth.12978
10. Kimmel SE, French B, Kasner SE, Johnson JA, Anderson JL, Gage BF, et al. A pharmacogenetic versus a clinical algorithm for warfarin dosing. N Engl J Med. (2013) 369:2283–93. doi: 10.1056/NEJMoa1310669
11. Gage BF, Bass AR, Lin H, Woller SC, Stevens SM, Al-Hammadi N, et al. Effect of genotype-guided warfarin dosing on clinical events and anticoagulation control among patients undergoing hip or knee arthroplasty: the GIFT randomized clinical trial. JAMA. (2017) 318:1115–24. doi: 10.1001/jama.2017.11469
12. Pirmohamed M, Burnside G, Eriksson N, Jorgensen AL, Toh CH, Nicholson T, et al. A randomized trial of genotype-guided dosing of warfarin. N Engl J Med. (2013) 369:2294–303.
13. Verhoef TI, Ragia G, de Boer A, Barallon R, Kolovou G, Kolovou V, et al. A randomized trial of genotype-guided dosing of acenocoumarol and phenprocoumon. N Engl J Med. (2013) 369:2304–12. doi: 10.1056/NEJMoa1311388
14. Yan H, Yin JY, Zhang W, Li X. Possible strategies to make warfarin dosing algorithm prediction more accurately in patients with extreme dose. Clin Pharmacol Ther. (2018) 103:184. doi: 10.1002/cpt.800
15. Cosgun E, Limdi NA, Duarte CW. High-dimensional pharmacogenetic prediction of a continuous trait using machine learning techniques with application to warfarin dose prediction in African Americans. Bioinformatics. (2011) 27:1384–9. doi: 10.1093/bioinformatics/btr159
16. Baillargeon J, Holmes HM, Lin YL, Raji MA, Sharma G, Kuo YF. Concurrent use of warfarin and antibiotics and the risk of bleeding in older adults. Am J Med. (2012) 125:183–9. doi: 10.1016/j.amjmed.2011.08.014
17. Fischer HD, Juurlink DN, Mamdani MM, Kopp A, Laupacis A. Hemorrhage during warfarin therapy associated with cotrimoxazole and other urinary tract anti-infective agents: a population-based study. Arch Intern Med. (2010) 170:617–21. doi: 10.1001/archinternmed.2010.37
18. Schelleman H, Bilker WB, Brensinger CM, Han X, Kimmel SE, Hennessy S. Warfarin with fluoroquinolones, sulfonamides, or azole antifungals: interactions and the risk of hospitalization for gastrointestinal bleeding. Clin Pharmacol Ther. (2008) 84:581–8. doi: 10.1038/clpt.2008.150
19. Chaudhuri A, Wade SL. Flucloxacillin-warfarin interaction: an under-appreciated phenomenon. Intern Med J. (2018) 48:860–3. doi: 10.1111/imj.13944
20. Ghaswalla PK, Harpe SE, Tassone D, Slattum PW. Warfarin-antibiotic interactions in older adults of an outpatient anticoagulation clinic. Am J Geriatr Pharmacother. (2012) 10:352–60.
21. Palermo A, Tuccinardi D, D’Onofrio L, Watanabe M, Maggi D, Maurizi AR, et al. Vitamin K and osteoporosis: myth or reality? Metabolism. (2017) 70:57–71.
23. Newman P, Shearer MJ. Metabolism and cell biology of vitamin K. Thrombosis Haemostasis. (2017) 100:530–47.
24. Karl JP, Fu X, Wang X, Zhao Y, Shen J, Zhang C, et al. Fecal menaquinone profiles of overweight adults are associated with gut microbiota composition during a gut microbiota-targeted dietary intervention. Am J Clin Nutr. (2015) 102:84–93. doi: 10.3945/ajcn.115.109496
25. Sakano T, Nagaoka T, Morimoto A, Hirauchi K. Measurement of K vitamins in human and animal feces by high-performance liquid chromatography with fluorometric detection. Chem Pharm Bull (Tokyo). (1986) 34:4322–6. doi: 10.1248/cpb.34.4322
26. Shearer MJ, Okano T. Key pathways and regulators of vitamin K function and intermediary metabolism. Annu Rev Nutr. (2018) 38:127–51. doi: 10.1146/annurev-nutr-082117-051741
27. Shearer MJ, Newman P. Recent trends in the metabolism and cell biology of vitamin K with special reference to vitamin K cycling and MK-4 biosynthesis. J Lipid Res. (2014) 55:345–62. doi: 10.1194/jlr.R045559
28. Fusaro M, Gallieni M, Rizzo MA, Stucchi A, Delanaye P, Cavalier E, et al. Vitamin K plasma levels determination in human health. Clin Chem Lab Med. (2017) 55:789–99. doi: 10.1515/cclm-2016-0783
29. Shea MK, Booth SL. Concepts and controversies in evaluating vitamin K status in population-based studies. Nutrients. (2016) 8:8. doi: 10.3390/nu8010008
30. Girolami A, Ferrari S, Cosi E, Santarossa C, Randi ML. Vitamin K-dependent coagulation factors that may be responsible for both bleeding and thrombosis (FII, FVII, and FIX). Clin Appl Thromb Hemost. (2018) 24(9 Suppl.):42s–7s. doi: 10.1177/1076029618811109
31. Tie JK, Stafford DW. Functional study of the vitamin K cycle enzymes in live cells. Methods Enzymol. (2017) 584:349–94. doi: 10.1016/bs.mie.2016.10.015
32. Chen X, Jin DY, Stafford DW, Tie JK. Evaluation of oral anticoagulants with vitamin K epoxide reductase in its native milieu. Blood. (2018) 132:1974–84. doi: 10.1182/blood-2018-05-846592
33. Hirai K, Hayashi H, Ono Y, Izumiya K, Tanaka M, Suzuki T, et al. Influence of CYP4F2 polymorphisms and plasma vitamin K levels on warfarin sensitivity in Japanese pediatric patients. Drug Metab Pharmacokinet. (2013) 28:132–7. doi: 10.2133/dmpk.dmpk-12-rg-078
34. Giuliano V, Bassotti G, Mourvaki E, Castellani D, Filippucci E, Sabatino G, et al. Small intestinal bacterial overgrowth and warfarin dose requirement variability. Thromb Res. (2010) 126:12–7. doi: 10.1016/j.thromres.2009.11.032
35. Schurgers LJ, Shearer MJ, Hamulyak K, Stocklin E, Vermeer C. Effect of vitamin K intake on the stability of oral anticoagulant treatment: dose-response relationships in healthy subjects. Blood. (2004) 104:2682–9. doi: 10.1182/blood-2004-04-1525
36. Ford SK, Misita CP, Shilliday BB, Malone RM, Moore CG, Moll S. Prospective study of supplemental vitamin K therapy in patients on oral anticoagulants with unstable international normalized ratios. J Thromb Thrombolysis. (2007) 24:23–7. doi: 10.1007/s11239-007-0014-z
37. Rombouts EK, Rosendaal FR, Van Der Meer FJ. Daily vitamin K supplementation improves anticoagulant stability. J Thromb Haemost. (2007) 5:2043–8. doi: 10.1111/j.1538-7836.2007.02715.x
38. Sconce E, Avery P, Wynne H, Kamali F. Vitamin K supplementation can improve stability of anticoagulation for patients with unexplained variability in response to warfarin. Blood. (2007) 109:2419–23. doi: 10.1182/blood-2006-09-049262
39. Theuwissen E, Teunissen KJ, Spronk HM, Hamulyak K, Ten Cate H, Shearer MJ, et al. Effect of low-dose supplements of menaquinone-7 (vitamin K2) on the stability of oral anticoagulant treatment: dose-response relationship in healthy volunteers. J Thromb Haemost. (2013) 11:1085–92. doi: 10.1111/jth.12203
40. Crowther MA, Ageno W, Garcia D, Wang L, Witt DM, Clark NP, et al. Oral vitamin K versus placebo to correct excessive anticoagulation in patients receiving warfarin: a randomized trial. Ann Intern Med. (2009) 150:293–300. doi: 10.7326/0003-4819-150-5-200903030-00005
41. Gebuis EP, Rosendaal FR, van Meegen E, van der Meer FJ. Vitamin K1 supplementation to improve the stability of anticoagulation therapy with vitamin K antagonists: a dose-finding study. Haematologica. (2011) 96:583–9. doi: 10.3324/haematol.2010.035162
42. Dentali F, Crowther M, Galli M, Pomero F, Garcia D, Clark N, et al. Effect of vitamin K intake on the stability of treatment with vitamin K antagonists: a systematic review of the literature. Semin Thromb Hemost. (2016) 42:671–81. doi: 10.1055/s-0036-1581105
43. Sconce E, Khan T, Mason J, Noble F, Wynne H, Kamali F. Patients with unstable control have a poorer dietary intake of vitamin K compared to patients with stable control of anticoagulation. Thromb Haemost. (2005) 93:872–5. doi: 10.1160/TH04-12-0773
44. Schurgers LJ, Teunissen KJ, Hamulyak K, Knapen MH, Vik H, Vermeer C. Vitamin K-containing dietary supplements: comparison of synthetic vitamin K1 and natto-derived menaquinone-7. Blood. (2007) 109:3279–83. doi: 10.1182/blood-2006-08-040709
45. Kramps M, Flanagan A, Smaldone A. The use of vitamin K supplementation to achieve INR stability: a systematic review and meta-analysis. J Am Assoc Nurse Pract. (2013) 25:535–44. doi: 10.1111/1745-7599.12022
46. Kampouraki E, Kamali F. Dietary implications for patients receiving long-term oral anticoagulation therapy for treatment and prevention of thromboembolic disease. Expert Rev Clin Pharmacol. (2017) 10:789–97. doi: 10.1080/17512433.2017.1345622
47. Chow WH, Chow TC, Tse TM, Tai YT, Lee WT. Anticoagulation instability with life-threatening complication after dietary modification. Postgrad Med J. (1990) 66:855–7. doi: 10.1136/pgmj.66.780.855
48. Walker FBT. Myocardial infarction after diet-induced warfarin resistance. Arch Intern Med. (1984) 144:2089–90. doi: 10.1001/archinte.144.10.2089
49. Hiratsuka T, Furihata K, Ishikawa J, Yamashita H, Itoh N, Seto H, et al. An alternative menaquinone biosynthetic pathway operating in microorganisms. Science. (2008) 321:1670–3. doi: 10.1126/science.1160446
50. Bentley R, Meganathan R. Biosynthesis of vitamin K (menaquinone) in bacteria. Microbiol Rev. (1982) 46:241–80. doi: 10.1128/mr.46.3.241-280.1982
51. Meganathan R. Biosynthesis of menaquinone (vitamin K2) and ubiquinone (coenzyme Q): a perspective on enzymatic mechanisms. Vitam Horm. (2001) 61:173–218. doi: 10.1016/s0083-6729(01)61006-9
52. Ravcheev DA, Thiele I. Genomic analysis of the human gut microbiome suggests novel enzymes involved in quinone biosynthesis. Front Microbiol. (2016) 7:128. doi: 10.3389/fmicb.2016.00128
53. Kurosu M, Begari E. Vitamin K2 in electron transport system: are enzymes involved in vitamin K2 biosynthesis promising drug targets? Molecules. (2010) 15:1531–53. doi: 10.3390/molecules15031531
54. Mathers JC, Fernandez F, Hill MJ, McCarthy PT, Shearer MJ, Oxley A. Dietary modification of potential vitamin K supply from enteric bacterial menaquinones in rats. Br J Nutr. (1990) 63:639–52. doi: 10.1079/bjn19900150
55. Iyobe S, Tsunoda M, Mitsuhashi S. Cloning and expression in Enterobacteriaceae of the extended-spectrum beta-lactamase gene from a Pseudomonas aeruginosa plasmid. FEMS Microbiol Lett. (1994) 121:175–80. doi: 10.1016/0378-1097(94)90124-4
56. Conly JM, Stein K. Quantitative and qualitative measurements of K vitamins in human intestinal contents. Am J Gastroenterol. (1992) 87:311–6.
57. McCann A, Jeffery IB, Ouliass B, Ferland G, Fu X, Booth SL, et al. Exploratory analysis of covariation of microbiota-derived vitamin K and cognition in older adults. Am J Clin Nutr. (2019) 110:1404–15. doi: 10.1093/ajcn/nqz220
58. Human Microbiome Project Consortium. Structure, function and diversity of the healthy human microbiome. Nature. (2012) 486:207–14. doi: 10.1038/nature11234
59. Sender R, Fuchs S, Milo R. Are we really vastly outnumbered? Revisiting the ratio of bacterial to host cells in humans. Cell. (2016) 164:337–40. doi: 10.1016/j.cell.2016.01.013
60. Wang L, Ravichandran V, Yin Y, Yin J, Zhang Y. Natural products from mammalian gut microbiota. Trends Biotechnol. (2019) 37:492–504. doi: 10.1016/j.tibtech.2018.10.003
61. Dodd D, Spitzer MH, Van Treuren W, Merrill BD, Hryckowian AJ, Higginbottom SK, et al. A gut bacterial pathway metabolizes aromatic amino acids into nine circulating metabolites. Nature. (2017) 551:648–52. doi: 10.1038/nature24661
62. Sharon G, Sampson TR, Geschwind DH, Mazmanian SK. The central nervous system and the gut microbiome. Cell. (2016) 167:915–32. doi: 10.1016/j.cell.2016.10.027
63. Tripathi A, Debelius J, Brenner DA, Karin M, Loomba R, Schnabl B, et al. The gut-liver axis and the intersection with the microbiome. Nat Rev Gastroenterol Hepatol. (2018) 15:397–411. doi: 10.1038/s41575-018-0011-z
64. Lee JY, Arai H, Nakamura Y, Fukiya S, Wada M, Yokota A. Contribution of the 7beta-hydroxysteroid dehydrogenase from Ruminococcus gnavus N53 to ursodeoxycholic acid formation in the human colon. J Lipid Res. (2013) 54:3062–9. doi: 10.1194/jlr.M039834
65. Yoshimoto S, Loo TM, Atarashi K, Kanda H, Sato S, Oyadomari S, et al. Obesity-induced gut microbial metabolite promotes liver cancer through senescence secretome. Nature. (2013) 499:97–101. doi: 10.1038/nature12347
66. Powers A, Loesch EB, Weiland A, Fioravanti N, Lucius D. Preemptive warfarin dose reduction after initiation of sulfamethoxazole-trimethoprim or metronidazole. J Thromb Thrombolysis. (2017) 44:88–93. doi: 10.1007/s11239-017-1497-x
67. Saum LM, Balmat RP. Ceftriaxone potentiates warfarin activity greater than other antibiotics in the treatment of urinary tract infections. J Pharm Pract. (2016) 29:121–4. doi: 10.1177/0897190014544798
68. Ponziani FR, Pompili M, Di Stasio E, Zocco MA, Gasbarrini A, Flore R. Subclinical atherosclerosis is linked to small intestinal bacterial overgrowth via vitamin K2-dependent mechanisms. World J Gastroenterol. (2017) 23:1241–9. doi: 10.3748/wjg.v23.i7.1241
69. Scarpellini E, Gabrielli M, Za T, Lauritano EC, Santoliquido A, Rossi E, et al. The interaction between small intestinal bacterial overgrowth and warfarin treatment. Am J Gastroenterol. (2009) 104:2364–5. doi: 10.1038/ajg.2009.288
70. Zimmermann M, Zimmermann-Kogadeeva M, Wegmann R, Goodman AL. Mapping human microbiome drug metabolism by gut bacteria and their genes. Nature. (2019) 570:462–7. doi: 10.1038/s41586-019-1291-3
71. Wang L, Liu L, Liu X, Xiang M, Zhou L, Huang C, et al. The gut microbes, Enterococcus and Escherichia-Shigella,affect the responses of heart valve replacement patients to the anticoagulant warfarin. Pharmacol Res. (2020) 159:104979. doi: 10.1016/j.phrs.2020.104979
72. Avior Y, Levy G, Zimerman M, Kitsberg D, Schwartz R, Sadeh R, et al. Microbial-derived lithocholic acid and vitamin K2 drive the metabolic maturation of pluripotent stem cells-derived and fetal hepatocytes. Hepatology. (2015) 62:265–78. doi: 10.1002/hep.27803
73. Sultana H, Watanabe K, Rana MM, Takashima R, Ohashi A, Komai M, et al. Effects of vitamin K2 on the expression of genes involved in bile acid synthesis and glucose homeostasis in mice with humanized PXR. Nutrients. (2018) 10:982. doi: 10.3390/nu10080982
74. Yang W, Yu Z, Chiyoya M, Liu X, Daitoku K, Motomura S, et al. Menaquinone-4 accelerates calcification of human aortic valve interstitial cells in high-phosphate medium through PXR. J Pharmacol Exp Ther. (2020) 372:277–84. doi: 10.1124/jpet.119.263160
75. Sultana H, Kato A, Ohashi A, Takashima R, Katsurai T, Sato S, et al. Effect of vitamin K-mediated PXR activation on drug-metabolizing gene expression in human intestinal carcinoma LS180 cell line. Nutrients. (2021) 13:1709. doi: 10.3390/nu13051709
76. Meng Z, Gwag T, Sui Y, Park SH, Zhou X, Zhou C. The atypical antipsychotic quetiapine induces hyperlipidemia by activating intestinal PXR signaling. JCI Insight. (2019) 4:e125657. doi: 10.1172/jci.insight.125657
77. Gwag T, Meng Z, Sui Y, Helsley RN, Park SH, Wang S, et al. Non-nucleoside reverse transcriptase inhibitor efavirenz activates PXR to induce hypercholesterolemia and hepatic steatosis. J Hepatol. (2019) 70:930–40. doi: 10.1016/j.jhep.2018.12.038
78. Sui Y, Xu J, Rios-Pilier J, Zhou C. Deficiency of PXR decreases atherosclerosis in apoE-deficient mice. J Lipid Res. (2011) 52:1652–9. doi: 10.1194/jlr.M017376
79. Zhou C, King N, Chen KY, Breslow JL. Activation of PXR induces hypercholesterolemia in wild-type and accelerates atherosclerosis in apoE deficient mice. J Lipid Res. (2009) 50:2004–13. doi: 10.1194/jlr.M800608-JLR200
80. Sporstøl M, Tapia G, Malerød L, Mousavi SA, Berg T. Pregnane X receptor-agonists down-regulate hepatic ATP-binding cassette transporter A1 and scavenger receptor class B type I. Biochem Biophys Res Commun. (2005) 331:1533–41. doi: 10.1016/j.bbrc.2005.04.071
81. Goncalves A, Margier M, Roi S, Collet X, Niot I, Goupy P, et al. Intestinal scavenger receptors are involved in vitamin K1 absorption. J Biol Chem. (2014) 289:30743–52. doi: 10.1074/jbc.M114.587659
82. Takada T, Yamanashi Y, Konishi K, Yamamoto T, Toyoda Y, Masuo Y, et al. NPC1L1 is a key regulator of intestinal vitamin K absorption and a modulator of warfarin therapy. Sci Transl Med. (2015) 7:275ra23. doi: 10.1126/scitranslmed.3010329
83. Sahi J, Shord SS, Lindley C, Ferguson S, LeCluyse EL. Regulation of cytochrome P450 2C9 expression in primary cultures of human hepatocytes. J Biochem Mol Toxicol. (2009) 23:43–58. doi: 10.1002/jbt.20264
84. Al-Dosari MS, Knapp JE, Liu D. Activation of human CYP2C9 promoter and regulation by CAR and PXR in mouse liver. Mol Pharm. (2006) 3:322–8. doi: 10.1021/mp0500824
85. Lara LF, Delgado LL, Frazee LA, Haupt KM, Rutecki GW. A subtherapeutic international normalized ratio despite increasing doses of warfarin: could this be malabsorption? Am J Med Sci. (2000) 320:214–8. doi: 10.1097/00000441-200009000-00015
86. Esmerian MO, Mitri Z, Habbal MZ, Geryess E, Zaatari G, Alam S, et al. Influence of CYP2C9 and VKORC1 polymorphisms on warfarin and acenocoumarol in a sample of Lebanese people. J Clin Pharmacol. (2011) 51:1418–28. doi: 10.1177/0091270010382910
87. Tan J, McKenzie C, Potamitis M, Thorburn AN, Mackay CR, Macia L. The role of short-chain fatty acids in health and disease. Adv Immunol. (2014) 121:91–119. doi: 10.1016/B978-0-12-800100-4.00003-9
88. Koh A, De Vadder F, Kovatcheva-Datchary P, Backhed F. From dietary fiber to host physiology: short-chain fatty acids as key bacterial metabolites. Cell. (2016) 165:1332–45. doi: 10.1016/j.cell.2016.05.041
89. Xu S, Liu CX, Xu W, Huang L, Zhao JY, Zhao SM. Butyrate induces apoptosis by activating PDC and inhibiting complex I through SIRT3 inactivation. Signal Transduct Target Ther. (2017) 2:16035. doi: 10.1038/sigtrans.2016.35
90. Furusawa Y, Obata Y, Fukuda S, Endo TA, Nakato G, Takahashi D, et al. Commensal microbe-derived butyrate induces the differentiation of colonic regulatory T cells. Nature. (2013) 504:446–50. doi: 10.1038/nature12721
91. Silva JPB, Navegantes-Lima KC, Oliveira ALB, Rodrigues DVS, Gaspar SLF, Monteiro VVS, et al. Protective mechanisms of butyrate on inflammatory bowel disease. Curr Pharm Des. (2018) 24:4154–66. doi: 10.2174/1381612824666181001153605
92. Bridgeman SC, Northrop W, Melton PE, Ellison GC, Newsholme P, Mamotte CDS. Butyrate generated by gut microbiota and its therapeutic role in metabolic syndrome. Pharmacol Res. (2020) 160:105174. doi: 10.1016/j.phrs.2020.105174
93. Chen Y, Xu C, Huang R, Song J, Li D, Xia M. Butyrate from pectin fermentation inhibits intestinal cholesterol absorption and attenuates atherosclerosis in apolipoprotein E-deficient mice. J Nutr Biochem. (2018) 56:175–82. doi: 10.1016/j.jnutbio.2018.02.011
94. Ritchie SR, Orr DW, Black PN. Severe jaundice following treatment with ezetimibe. Eur J Gastroenterol Hepatol. (2008) 20:572–3. doi: 10.1097/MEG.0b013e3282f1752d
95. Narushima K, Takada T, Yamanashi Y, Suzuki H. Niemann-pick C1-like 1 mediates alpha-tocopherol transport. Mol Pharmacol. (2008) 74:42–9. doi: 10.1124/mol.107.043034
96. Yamanashi Y, Takada T, Kurauchi R, Tanaka Y, Komine T, Suzuki H. Transporters for the intestinal absorption of cholesterol, vitamin E, and vitamin K. J Atheroscler Thromb. (2017) 24:347–59. doi: 10.5551/jat.RV16007
97. Oliver WR Jr., Shenk JL, Snaith MR, Russell CS, Plunket KD, Bodkin NL, et al. A selective peroxisome proliferator-activated receptor delta agonist promotes reverse cholesterol transport. Proc Natl Acad Sci U.S.A. (2001) 98:5306–11. doi: 10.1073/pnas.091021198
98. van der Veen JN, Kruit JK, Havinga R, Baller JF, Chimini G, Lestavel S, et al. Reduced cholesterol absorption upon PPARdelta activation coincides with decreased intestinal expression of NPC1L1. J Lipid Res. (2005) 46:526–34. doi: 10.1194/jlr.M400400-JLR200
99. Russo R, De Caro C, Avagliano C, Cristiano C, La Rana G, Mattace Raso G, et al. Sodium butyrate and its synthetic amide derivative modulate nociceptive behaviors in mice. Pharmacol Res. (2016) 103:279–91. doi: 10.1016/j.phrs.2015.11.026
100. Hashimoto N, Matsui I, Ishizuka S, Inoue K, Matsumoto A, Shimada K, et al. Lithocholic acid increases intestinal phosphate and calcium absorption in a vitamin D receptor dependent but transcellular pathway independent manner. Kidney Int. (2020) 97:1164–80. doi: 10.1016/j.kint.2020.01.032
101. Sato Y, Atarashi K, Plichta DR, Arai Y, Sasajima S, Kearney SM, et al. Novel bile acid biosynthetic pathways are enriched in the microbiome of centenarians. Nature. (2021) 599:458–64. doi: 10.1038/s41586-021-03832-5
102. Sasaki H, Masuno H, Kawasaki H, Yoshihara A, Numoto N, Ito N, et al. Lithocholic acid derivatives as potent vitamin D receptor agonists. J Med Chem. (2021) 64:516–26. doi: 10.1021/acs.jmedchem.0c01420
103. Masuno H, Kazui Y, Tanatani A, Fujii S, Kawachi E, Ikura T, et al. Development of novel lithocholic acid derivatives as vitamin D receptor agonists. Bioorg Med Chem. (2019) 27:3674–81. doi: 10.1016/j.bmc.2019.07.003
104. Chen Y, Goldstein JA. The transcriptional regulation of the human CYP2C genes. Curr Drug Metab. (2009) 10:567–78. doi: 10.2174/138920009789375397
Keywords: vitamin K, intestinal bacteria, butyrate, vitamin K antagonist, lithocholic acid
Citation: Yan H, Chen Y, Zhu H, Huang W-H, Cai X-H, Li D, Lv Y-J, Si-Zhao, Zhou H-H, Luo F-Y, Zhang W and Li X (2022) The Relationship Among Intestinal Bacteria, Vitamin K and Response of Vitamin K Antagonist: A Review of Evidence and Potential Mechanism. Front. Med. 9:829304. doi: 10.3389/fmed.2022.829304
Received: 05 December 2021; Accepted: 03 March 2022;
Published: 18 April 2022.
Edited by:
Lalitha Nayak, Case Western Reserve University, United StatesReviewed by:
Jonathan Douxfils, University of Namur, BelgiumCopyright © 2022 Yan, Chen, Zhu, Huang, Cai, Li, Lv, Si-Zhao, Zhou, Luo, Zhang and Li. This is an open-access article distributed under the terms of the Creative Commons Attribution License (CC BY). The use, distribution or reproduction in other forums is permitted, provided the original author(s) and the copyright owner(s) are credited and that the original publication in this journal is cited, in accordance with accepted academic practice. No use, distribution or reproduction is permitted which does not comply with these terms.
*Correspondence: Xi Li, YmF5ZXJuQGNzdS5lZHUuY24=; Yi Chen, Y3kxOTk0MDljeUAxNjMuY29t
†These authors have contributed equally to this work
Disclaimer: All claims expressed in this article are solely those of the authors and do not necessarily represent those of their affiliated organizations, or those of the publisher, the editors and the reviewers. Any product that may be evaluated in this article or claim that may be made by its manufacturer is not guaranteed or endorsed by the publisher.
Research integrity at Frontiers
Learn more about the work of our research integrity team to safeguard the quality of each article we publish.