- 1Occupational Medicine Section, Department of Biomedical and Dental Sciences and Morphofunctional Imaging, University of Messina, Messina, Italy
- 2Clinical and Experimental Medicine Department, University of Messina, Messina, Italy
There is a growing interest in the characterization of the involvement of toxicant and pollutant exposures in the development and the progression of several diseases such as obesity, diabetes, cancer, as well as in the disruption of the immune and reproductive homeostasis. The gut microbiota is considered a pivotal player against the toxic properties of chemicals with the establishment of a dynamic bidirectional relationship, underlining the toxicological significance of this mutual interplay. In fact, several environmental chemicals have been demonstrated to affect the composition, the biodiversity of the intestinal microbiota together with the underlining modulated metabolic pathways, which may play an important role in tailoring the microbiotype of an individual. In this review, we aimed to discuss the latest updates concerning the environmental chemicals–microbiota dual interaction, toward the identification of a distinctiveness of the gut microbial community, which, in turn, may allow to adopt personalized preventive strategies to improve risk assessment for more susceptible workers.
Introduction
During the entire lifespan, since the conception and whole fetal development, we are constantly exposed to the so-called exposome, defined as the totality of the environmental exposures, which are dynamic in their quality and quantity over time (1). Such environmental factors may include air pollutants, radiations, chemicals present in soil, food, and water, but also individual factors associated with the personal lifestyle, such as tobacco smoking, food consumption, specific use of drugs, and xenobiotics, altogether representing the external exposome (2).
Another constant source of exposure is considered our internal exposome, mainly accounting for the effects mediated by the microbiota, a heterogeneous consortium of microorganisms that populates all the exposed surfaces of the body, and having with the host a relationship of mutual advantage (3). For many chemicals, the health impact associated with exposure (and the corresponding exposure pathways) remains yet poorly understood (4). To find novel biomarkers of exposure, as well as to characterize the real associations existing between exposures and the development of a certain disease, both represent goals of pivotal importance, especially for the most exposed occupational categories (including agriculture, construction plant manufacturing, and mining) (5). Given its role as a key link between the external exposome and the human health, the internal exposome, and in particular our microbiota represents a promising source of novel functional correlations and biomarkers of exposure (6).
Among the different microbiota, the most characterized is the gastro-intestinal one, which plays a main role as a dynamical interface between the host and the external exposome (7). Intestinal dysbiosis has been associated with marked structural changes in the mucosa, including permeability and inflammation. The dysbiosis may, in turn, favor either the insurgence or the worsening of several chronic, non-communicable diseases, including cardiovascular diseases, diabetes, neurological diseases, and cancer (8, 9). Furthermore, dysbiosis has been associated with multiple extraintestinal diseases such as neurological or behavioral outcomes (10–14), respiratory dysfunctions (15, 16), metabolic/endocrine impairment (17–19), and inflammatory/autoimmune diseases (20–26).
Additionally, it is now commonly recognized that stress actively modulates both structure and activity of the Gut Microbiota (GM) community and it may be a critical factor in causing dysbiosis (27–30). There is a growing evidence that a healthy and resilient GM can contribute to optimize the health and performance of the general host. However, developing responses for this goal requires elucidating the impact of stressors on the GM. Environmental pollutants such as microplastics or synthetic compounds interact with GM through several toxicokinetic and toxicodynamic pathways and, in turn, GM may alter the bioavailability and toxicity of xenobiotic metabolites. This bidirectional interaction can modulate the physiological homeostasis, finally leading to health disorders (31–33).
This review explores all the up-to-date studies regarding the role played by the GM in xenobiotics metabolism and protection mechanisms, especially for what concerns the main groups of environmental chemicals (i.e., pesticides, metals, and microplastics) to tailor personalized preventive strategies, as well as to improve the risk assessment for more susceptible workers.
Features of Gut Microbiota
GM includes bacteria, archaea, viruses, yeasts, and other fungi whose population density progressively increases from 103 to 104 cells/ml within the gastric acidic environment to about 1011 cells/ml within the colon (34). The dominant phyla are Firmicutes, Bacteroidetes, Actinobacteria, Proteobacteria, and Fusobacteria. Overall, a healthy human adult hosts over 100 different bacterial species in the gastrointestinal tract, with a marked interindividual variation in genus and species compositions (35).
The so-called gut microbiome includes the whole genome of the GM, encoding for over 100-fold more genes than the human genome (36). The recent advent of metagenomics, which combines the next-generation sequencing (NGS) technology with the computational analysis of the 16S ribosomal RNA (rRNA) amplicons, helps in the characterization of both diversity and abundance of the gut microbiome (37). Metagenomics, together with metatranscriptomics, metaproteomics, and metabolomics, is currently allowing us to understand the impact of each individual bacterial species on the health of the host (38).
Despite the existence of several definitions of healthy GM, a number of endogenous and exogenous factors may cause the microbiota to shift from eubiotic to dysbiotic; in general, a more diverse GM, both in terms of diversity and abundance of taxa, is considered a healthier GM (39). Additionally, a healthy GM can resist or overcome perturbations by returning to a state of balance or eubiosis.
Currently, species belonging to the Eubacterium, Roseburia, and Faecalibacterium genera are included among the beneficial taxa, given their ability to secrete butyrate, a short-chain fatty acid (SCFA) with several health effects, such as to improve the integrity of the intestinal barrier, as well as to reduce the gut oxidative stress or inflammatory status (40).
Potentially harmful bacteria are considered those belonging to the Enterobacteriaceae family that includes the intestinal commensals Escherichia, Shigella, Proteus, and Klebsiella with pronounced pro-inflammatory effects (41). Several bacterial species are able to actively secrete toxins, including CagA from Helicobacter pylori, colibactin and cytolethal distending toxin (CDT) from Escherichia coli, inositol phosphate phosphatase D (IpgD), and cysteine protease-like virulence gene A (VirA) from Shigella flexneri, that in turn damage the intestinal epithelial integrity (9). These toxins may induce direct damage to the epithelial cellular DNA, trigger proproliferative pathways (including Akt serine/threonine kinase family and Wnt/β-Catenin signaling), or stimulate the local secretion of reactive oxygen species (ROS). Overall, these toxins may promote a proinflammatory milieu and even trigger the local cellular neoplastic transformation (9).
The proinflammatory environment may also have a counterintuitive health effect on the human host. For example, the lipopolysaccharide (LPS, also known as endotoxin), the main component of the outer membrane in gram-negative bacteria, may activate the pattern recognition receptors (PRRs) of host, including the toll-like receptor 4 (TLR4), thereby activating the immune T cell-mediated response, which activates a solid proinflammatory reaction. Although the inflammation may often promote colitis or mucositis, a proinflammatory status may also be protective in certain conditions, such as during tumor development (42).
Specific intestinal taxa are able to secrete essential micronutrients, such as vitamins (i.e., vitamin K and vitamin B) or the linoleic acid, which is an antidiabetic compound. Also, specific bacteria can catabolize secondary bile acids and phenolic compounds (43). Finally, certain gut-resident taxa, upon fermentation of dietary fibers in the large intestine, may produce hormone-like metabolites, such as the SCFAs.
Additionally, the gut microbial functions are tightly interconnected and directly affect the host immune response, both locally and systemically (44). This topic is deeply analyzed elsewhere, and, although out from the scope of this review, it is important here to underline that the microbial dysbiosis deriving by both environmental exposure and genetic susceptibility may be associated with aberrant mucosal immune responses, such as the upregulation of the Th17, Th1, and Th2 immune phenotypes, the downregulation of the T regulatory cells, and dysregulated humoral immunity. Overall, this immune shift may result in chronic intestinal inflammation and a generally altered immune response to pathogens and insults (45).
Given the role as a barrier, metabolic, and immune interface, the GM is extremely important as the joining link between the external exposome and the human host (46). In details, the GM plays a pivotal role in xenobiotics metabolism, including toxicants and chemical pollutants (47) (Figure 1).
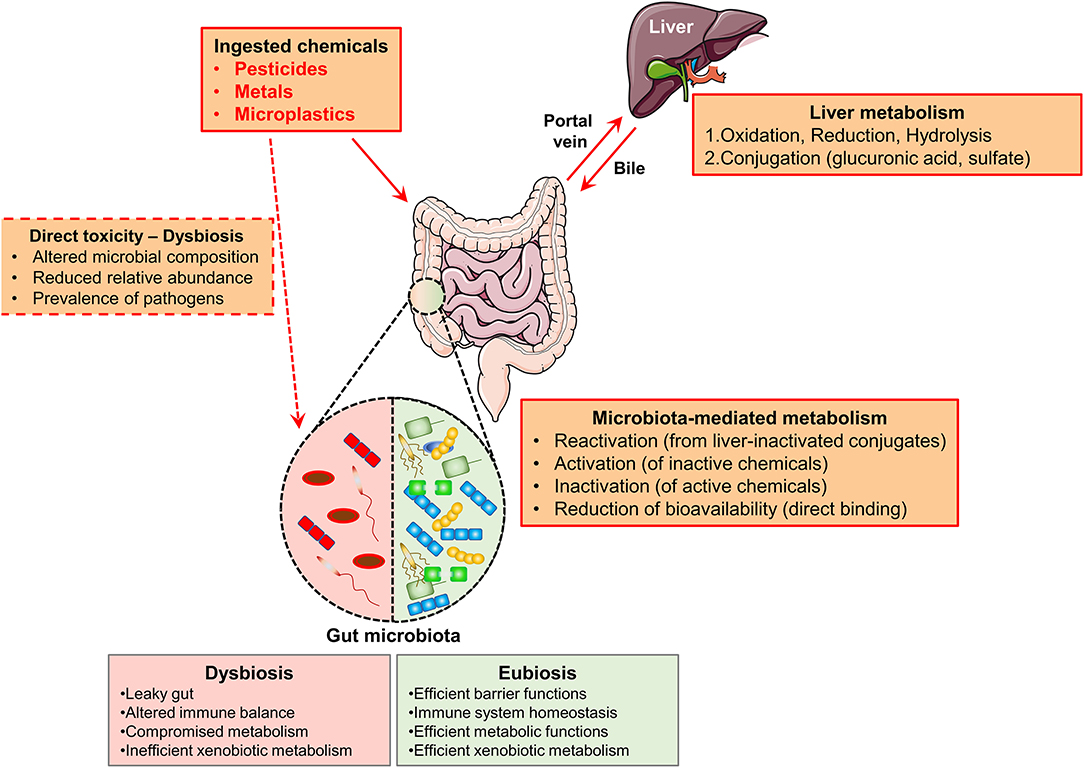
Figure 1. Schematic representation of the biotransformation routes of ingested chemicals. Ingested chemicals (pesticides, metals, microplastics), arrive in the intestine through the oral route. Well-adsorbed compounds are transported to the liver through the portal vein. In the liver, such compounds may be metabolized (through the action of liver enzymes, the compounds are oxidized, reduced, or hydrolysed and finally they are conjugated) and hence released in the intestine within the bile. The gut microbes can: reactivate conjugated chemicals, directly metabolize non-adsorbed chemicals (activation or inactivation), or direct bind such compounds (reducing their bioavailability). Importantly, several chemicals might directly induce microbial dysbiosis (red dotted box). The features of a dysbiotic or eubiotic intestinal microbiota are summarized, respectively, in the red and green boxes.
Once ingested, the toxicants, which are efficiently adsorbed in the intestine, through the bloodstream, arrive at the liver where they are oxidized, forming conjugates with glucuronic acid, sulfate, or glutathione that can be excreted in the bile and enter the intestine again, where the GM may interfere with their excretion (47). The GM can additionally directly metabolize the chemicals which are poorly adsorbed and hence transported to the large intestine through the action of several bacterial enzymes (such as beta-glucosidases, beta-glucuronidases, sulfatases, azoreductases, nitroreductases, and transferases) (48). Overall, the GM-mediated metabolism can lead to: (1) inactivation, (2) activation, or (3) reactivation (upon liver inactivation) of the specific compound (49). In the case of toxicants such as chemical pollutants, the GM-mediated inactivation can neutralize the hazard of the exposure. On the contrary, the activator outcome may be detrimental and increase the risk of developing associated pathologies, including cancer (50). In parallel, the GM composition may be actively shaped by the chemicals, as it was demonstrated in a number of preclinical studies (51). Many examples will be further discussed in the following section.
Consequently, the maintenance of a healthy GM may be protective against the toxicity of such chemicals and the occurrence of associated chronic diseases (52). This could be very relevant in specific occupational settings with a high rate of specific exposures (53). Overall, the modulation of the intestinal gut microbes, through active strategies such as the assumption of specific nutrients or also specific beneficial probiotics, with the aim to repristinate the eubiosis, may be protective against the development of the linked diseases and it can be suggested as a preventive intervention method.
Bidirectional Interaction Between Environmental Chemicals and Gut Microbiota
The concern is about the adverse health effects deriving from occupational exposure to toxic substances and environmental pollutants. Several xenobiotic chemicals have been described to interact with the biological activity of GM affecting the microbial composition and global homeostasis, with dangerous alterations to the host (48, 49). Noteworthy are occupational exposures to pesticides, used for the control of pests, which could affect human GM (54). Heavy metals (HMs) including cadmium, lead, arsenic, and other metals can contaminate soils and reach the human GM through the food chain (55). Exposures to environmental toxicants have been studied primarily for long-term systemic health effects on respiratory disease and cognition, among others, but there is growing evidence that these components also affect the GM. The molecular mechanisms leading to these interactions are not well-known. GM composition depends on several factors and significant changes in this composition, even if minor, are very expected to happen in studies involving animals and chemicals, but it is not clear if these alterations lead to biologically relevant outcomes in the host (56). Establishing such causal relationships should be a priority to elucidate this topic. Now we discuss recent literature findings of interaction between main environmental chemicals (pesticides, metals, and microplastics) and GM. The main findings are shown in Table 1.
Pesticides
Many studies have focused on the mechanisms underlying the relationship between pesticides and GM (47, 77). The GM can metabolize pesticides after absorption and reciprocally, active metabolites can affect GM homeostasis with adverse effects for the host. Glyphosate (Gly) is the most widely used herbicide worldwide and its use has been related to several adverse outcomes in humans (48, 49). Gly-induced GM alteration has been hypothesized to be related to neurological impairment such as autism spectrum disorders (78, 79). Several studies showed that Gly can alter the abundances of gut microbial species both in vivo (57, 58, 80–82), in vitro (59), and also through bioinformatics tools (83). It has been estimated that more than half of species living in the central human GM are sensitive to Gly (84). In addition to altering the microbial composition, a possible mechanism of action could be the modification of microRNAs (miRNAs) expression and immunomodulation as suggested by several studies (85–87). The mRNA expression levels of several inflammatory mediators [Nuclear factor kappa B (NF-κB), Tumour necrosis factor α (TNF-α), Caspase-3, MAPK3, IL-1β, and IL-6] resulted in increase after exposure to Gly, highlighting a notable decrease in the abundance of Firmicutes and enhancement of pathogenic bacteria (60, 61). Nielsen et al. showed that the presence of aromatic amino acids could relieve the antimicrobial effect of Gly (62). Another commonly used pesticide is Chlorpyrifos (Cpf), an organophosphate insecticide effective against fruit and vegetable pests. In vivo, it was observed that a chronic exposure to Cpf could cause an increment in Proteobacteria phylum and a decrease in Bacteroidetes phylum (63). The effect of chlorpyrifos has been explored using an in vitro simulator mimicking the human intestinal environment. It was observed a reduction of the Lactobacillus and the Bifidobacterium counts and alteration of the epithelial barrier integrity (64, 88) although other authors described an increment in the cultured Enterococcus spp. and Bacteroides spp. counts (65). Honeybees seem to be severely affected by neonicotinoid insecticides such as Imidacloprid and many studies suggested that a chronic exposure can alter normal GM composition with a decrement in the global bacterial count, mainly due to Firmicutes reduction (66, 89). Both Gly and Cpf can modulate mucosal-associated invariant T-cells activity in humans, leading to a pro-inflammatory immune response (90).
Metals
Heavy metals include naturally occurring chemicals with high atomic weight and density. Typically, these chemicals can be conveyed with particulate matter especially in urban areas and then reach the water and the soil (91). Several studies on humans or in vitro models suggest that HMs exposure can alter the composition and integrity of the GM (92–95). The changes of diversity and composition profile of GM composition resulted altered after a chronic exposure to several metals, such as arsenic (As), cadmium (Cd), cuprum (Cu), lead (Pb), and zinc (Zn). The authors observed an increase in the counts of some families (Porphyromonadaceae, Erysipelotrichaceae, Lachnospiraceae, and Acidaminococcaceae) vs. a reduction of the Prevotellaceae family. Moreover, it was found a gender difference because microbiota alterations of men were associated with work activity (mining and smelting) in polluted areas (96). As is common chemicals in nature, defined as a human carcinogen since 2012 (97). It can be found in water and soil, as both the organic and inorganic structures. It has been demonstrated to shape the GM depleting gut commensals and enriching pathogenic bacteria (98). In children exposed to As the GM alteration resulted in an abundance of Proteobacteria, highlighting changes in genes involved in multidrug resistance (67). As exposure not only affects GM composition but can also alter immune response increasing inflammatory cytokines such as IL-17, TNF-α, and interferon-γ (IFN-γ) (99). As can induce shifts in the earthworm GM, increasing the counts of Proteobacteria; these effects are amplified in a synergistic manner in a mixture exposure of As and microplastics (MPs) (68). Other authors described this combined effect of MPs and HMs including Cd, Pb, and Zn underlining GM perturbation and gonadal development in aquatic organisms (100). Pb exposure has been associated with alterations in the composition of the adult GM in humans. Increased urinary Pb level was related to GM richness and α and β-diversity, remarking an increment of Proteobacteria (69). Cd determines a reduction in GM richness and SCFAs production in mice; additionally, it can change the expression of genes involved in several metabolic pathways (101). In amphibians Cd has been demonstrated to reduce GM biodiversity and arrangement, disclosing significant gut histological alteration at Cd exposure (100 and 200 μg/l) (102). Mercury (Hg) toxicity on GM is confirmed by several in-vivo studies. The main described effects are intestinal injury, dysbiosis, enhanced expression of apoptosis genes, and neurotoxic effects (103, 104). Similarly, Pb exposure may act as a disruptor in GM homeostasis, inducing structural intestinal injuries and perturbing GM diversity both in vivo and in vitro (70). A disproportionate diet introit of Zn in mice, has been shown to cause oxidative stress in the intestinal trait, accompanied by significant shifts in GM, such as enrichment in pathogenic taxa (71).
Microplastics
Since plastics appeared, the MPs represent a novel occupational and environmental hazard (105). Although there is no scientifically agreed definition of MPs, they are usually defined as plastic particles <5 mm in diameter. Its toxicity has been broadly debated (106, 107), even though knowledge about MPs effects on gut microbiota still lacks. The accumulation of different forms of microplastics can cause several effects in the intestinal tract, damaging mucosa, and increasing permeability. Some authors have hypothesized that MPs could carrier and release phthalate esters into intestinal traits with consequential toxic effects (108). Also, MPs also can induce GM dysbiosis and specific bacteria alterations (109, 110). In the aquatic organism, MPs can affect the GM causing several harmful effects. Authors showed that low chronic MP exposure in mussel GM can lead to an increased abundance of human pathogens (111). In the zebrafish gut, it was observed a significant alteration in the microbial community after exposure to MPs. These alterations were likely mediated by inflammation and oxidative stress (112–114). At the phylum level, the increased count of Proteobacteria was accompanied by a significant reduction in the count of Fusobacteria, Firmicutes, and Verrucomicrobiota after 21-day exposure to 1 mg/L of MPs (72). MPs exposure seems to enhance the expression of immune cytokines (TNF-α, IFN-γ, TLR4, and IL-6) as well as inducing microbiota dysbiosis (115). Besides aquatic animals, also terrestrial ecosystems seem to be affected by MPs. In soil animals exposed to MPs, it has been observed a remarkable reduced bacterial diversity; particularly a reduction of the abundance of Bacteroides and an increment of the abundance of Firmicutes (73). Several studies have shown that polystyrene MP can gut dysbiosis in GM and intestinal barrier dysfunction (promoting inflammation) besides metabolic disorders, including hepatic lipid metabolism, in the mice model (116, 117). In the same in-vivo model, polyethylene MPs were suggested to cause intestinal dysbiosis and inflammation. Particularly it was observed a significant increment in Staphylococcus abundance and a significant reduction in Parabacteroides abundance. Furthermore, serum levels of interleukin-1α were found significantly raised (74). Maternal MPs exposure resulted associated with GM dysbiosis and gut barrier impairment in mice, with long-term metabolic penalties in offspring (118). It was observed that polystyrene microplastics could decrease α-diversity of GM of bees and alter the expression of antioxidative, detoxification, and immune system-related genes (75). In soil containing MPs, the gut of earthworms can be damaged. Polypropylene MPs exposure can reduce diversity and alter microbial community in earthworms GM. Specifically, an increment in Aeromonadaceae and Pseudomonadaceae was observed with a decline of Nitrososphaeraceae and Proteobacteria (76). MPs can reach the intestine trait and accumulate interacting with GM and altering its composition. This toxicology assessment may represent a new target to evaluate the health hazards for humans in the future.
Conclusion and Perspectives
The GM plays a multifaceted role in the exposure to toxic compounds. It represents the first interface between an exogenous chemical. Hence, the GM could represent a key factor in the toxicity of environmental pollutants and this may become really relevant for the identification of both novel biomarkers of exposure and molecular pathways underneath, therefore representing an indication of the true association of the exposure to the health effect observed. In this review, it has been discussed the emerging dual role played by the GM in the metabolisms of toxicants. The active modulation of the GM (e.g., with the administration of specific probiotics) to preserve the beneficial species able to neutralize the toxicity of such chemicals, may be explored in the future as a preventive therapeutic integrated approach to actively counteract exposure damages and detrimental health consequences.
In occupational medicine, a toxicological approach should be considered to elucidate GM alterations relating to environmental exposure to pollutants (119). GM composition together with the determination of well-known biomarkers could be helpful tools to assess susceptibility for disease. The risk assessment should consider that common human exposures to toxic compounds occur at low doses for a long time, while in most experimental studies, the exposure occurs for a short time at acute or sub-acute doses. It could be a new useful approach to analyze microbiome composition from a fecal sample as a screening tool, to assess the individual microbiome signature in order to address the specific intervention for preventive measures amelioration toward specific risk factors. Therefore, from a translational point-of-view, the GM may represent an important indicator for toxicological assessment, and future studies in clinics, especially in exposed cohorts of individuals, could identify the human GM as a helpful tool for the early surveillance of host health.
Author Contributions
CF, FG, and MT contributed to the conceptualization. CC and CF contributed to the methodology and supervision. FG and MT contributed to the data curation and writing—original draft preparation. CC contributed to writing—review and editing. All authors have read and agreed to the published version of the manuscript.
Conflict of Interest
The authors declare that the research was conducted in the absence of any commercial or financial relationships that could be construed as a potential conflict of interest.
Publisher's Note
All claims expressed in this article are solely those of the authors and do not necessarily represent those of their affiliated organizations, or those of the publisher, the editors and the reviewers. Any product that may be evaluated in this article, or claim that may be made by its manufacturer, is not guaranteed or endorsed by the publisher.
Acknowledgments
The authors thank Dr. Silvia Vivarelli for her helpful comments on this manuscript.
References
1. Wild CP. Complementing the genome with an “exposome”: the outstanding challenge of environmental exposure measurement in molecular epidemiology. Cancer Epidemiol Biomarkers Prev. (2005) 14:1847–50. doi: 10.1158/1055-9965.EPI-05-0456
2. Vrijheid M. The exposome: a new paradigm to study the impact of environment on health. Thorax. (2014) 69:876–8. doi: 10.1136/thoraxjnl-2013-204949
3. Dai D, Prussin AJ, Marr LC, Vikesland PJ, Edwards MA, Pruden A. Factors shaping the human exposome in the built environment: opportunities for engineering control. Environ Sci Technol. (2017) 51:7759–74. doi: 10.1021/acs.est.7b01097
4. Bopp SK, Barouki R, Brack W, Dalla Costa S, Dorne JLCM, Drakvik PE, et al. Current EU research activities on combined exposure to multiple chemicals. Environ Int. (2018) 120:544–62. doi: 10.1016/j.envint.2018.07.037
5. Ohlander J, Kromhout H, van Tongeren M. Interventions to reduce exposures in the workplace: a systematic review of intervention studies over six decades, 1960–2019. Front Public Heal. (2020) 8:67. doi: 10.3389/fpubh.2020.00067
6. Rinninella E, Raoul P, Cintoni M, Franceschi F, Miggiano GAD, Gasbarrini A, et al. What is the healthy gut microbiota composition? A changing ecosystem across age, environment, diet, and diseases. Microorganisms. (2019) 7:14. doi: 10.3390/microorganisms7010014
7. Defois C, Ratel J, Garrait G, Denis S, Le Goff O, Talvas J, et al. Food chemicals disrupt human gut microbiota activity and impact intestinal homeostasis as revealed by in vitro systems. Sci Rep. (2018) 8:11006. doi: 10.1038/s41598-018-29376-9
8. Koliarakis I, Messaritakis I, Nikolouzakis TK, Hamilos G, Souglakos J, Tsiaoussis J. Oral bacteria and intestinal dysbiosis in colorectal cancer. Int J Mol Sci. (2019) 20:4146. doi: 10.3390/ijms20174146
9. Vivarelli S, Salemi R, Candido S, Falzone L, Santagati M, Stefani S, et al. Gut microbiota and cancer: from pathogenesis to therapy. Cancers. (2019) 11:38. doi: 10.3390/cancers11010038
10. Fattorusso A, Di Genova L, Dell'isola GB, Mencaroni E, Esposito S. Autism spectrum disorders and the gut microbiota. Nutrients. (2019) 11:521. doi: 10.3390/nu11030521
11. Hughes HK, Rose D, Ashwood P. The gut microbiota and dysbiosis in autism spectrum disorders. Curr Neurol Neurosci Rep. (2018) 18:81. doi: 10.1007/s11910-018-0887-6
12. Liu S, Gao J, Zhu M, Liu K, Zhang HL. Gut microbiota and dysbiosis in Alzheimer's disease: implications for pathogenesis and treatment. Mol Neurobiol. (2020) 57:5026–43. doi: 10.1007/s12035-020-02073-3
13. Li T, Tian D, Zhu Z, Jin W, Wu S, Li H. The gut microbiota: a new perspective on the toxicity of malachite green (MG). Appl Microbiol Biotechnol. (2019) 103:9723–37. doi: 10.1007/s00253-019-10214-5
14. Capuco A, Urits I, Hasoon J, Chun R, Gerald B, Wang JK, et al. Current perspectives on gut microbiome dysbiosis and depression. Adv Ther. (2020) 37:1328–46. doi: 10.1007/s12325-020-01272-7
15. Hufnagl K, Pali-Schöll I, Roth-Walter F, Jensen-Jarolim E. Dysbiosis of the gut and lung microbiome has a role in asthma. Semin Immunopathol. (2020) 42:75–93. doi: 10.1007/s00281-019-00775-y
16. Chunxi L, Haiyue L, Yanxia L, Jianbing P, Jin S. The gut microbiota and respiratory diseases: new evidence. J Immunol Res. (2020) 2020:2340670. doi: 10.1155/2020/2340670
17. Knezevic J, Starchl C, Berisha AT, Amrein K. Thyroid-gut-axis: how does the microbiota influence thyroid function? Nutrients. (2020) 12:1–16. doi: 10.3390/nu12061769
18. Gomes AC, Hoffmann C, Mota JF. The human gut microbiota: metabolism and perspective in obesity. Gut Microbes. (2018) 9:308–25. doi: 10.1080/19490976.2018.1465157
19. Fenneman AC, Rampanelli E, Yin YS, Ames J, Blaser MJ, Fliers E, et al. Gut microbiota and metabolites in the pathogenesis of endocrine disease. Biochem Soc Trans. (2020) 48:915–31. doi: 10.1042/BST20190686
20. Guo XY, Liu XJ, Hao JY. Gut microbiota in ulcerative colitis: insights on pathogenesis and treatment. J Dig Dis. (2020) 21:147–59. doi: 10.1111/1751-2980.12849
21. Little R, Wine E, Kamath BM, Griffiths AM, Ricciuto A. Gut microbiome in primary sclerosing cholangitis: a review. World J Gastroenterol. (2020) 26:2768–80. doi: 10.3748/WJG.V26.I21.2768
22. Amoroso C, Perillo F, Strati F, Fantini MC, Caprioli F, Facciotti F. The role of gut microbiota biomodulators on mucosal immunity and intestinal inflammation. Cells. (2020) 9:1234. doi: 10.3390/cells9051234
23. Lavoie S, Conway KL, Lassen KG, Jijon HB, Pan H, Chun E, et al. The Crohn's disease polymorphism, ATG16L1 T300A, alters the gut microbiota and enhances the local Th1/Th17 response. Elife. (2019) 8:e39982. doi: 10.7554/ELIFE.39982
24. Xu H, Zhao H, Fan D, Liu M, Cao J, Xia Y, et al. Interactions between gut microbiota and immunomodulatory cells in rheumatoid arthritis. Mediators Inflamm. (2020) 2020:1430605. doi: 10.1155/2020/1430605
25. Siljander H, Honkanen J, Knip M. Microbiome and type 1 diabetes. EBioMedicine. (2019) 46:512–21. doi: 10.1016/j.ebiom.2019.06.031
26. Konig MF. The microbiome in autoimmune rheumatic disease. Best Pract Res Clin Rheumatol. (2020) 34:101473. doi: 10.1016/j.berh.2019.101473
27. Holtmann G, Shah A, Morrison M. Pathophysiology of functional gastrointestinal disorders: a holistic overview. Dig Dis. (2018) 35:5–13. doi: 10.1159/000485409
28. Molina-Torres G, Rodriguez-Arrastia M, Roman P, Sanchez-Labraca N, Cardona D. Stress and the gut microbiota-brain axis. Behav Pharmacol. (2019) 30:187–200. doi: 10.1097/FBP.0000000000000478
29. Simpson CA, Diaz-Arteche C, Eliby D, Schwartz OS, Simmons JG, Cowan CSM. The gut microbiota in anxiety and depression – a systematic review. Clin Psychol Rev. (2021) 83:101943. doi: 10.1016/j.cpr.2020.101943
30. Illiano P, Brambilla R, Parolini C. The mutual interplay of gut microbiota, diet and human disease. FEBS J. (2020) 287:833–55. doi: 10.1111/febs.15217
31. Bertotto LB, Catron TR, Tal T. Exploring interactions between xenobiotics, microbiota, and neurotoxicity in zebrafish. Neurotoxicology. (2020) 76:235–44. doi: 10.1016/j.neuro.2019.11.008
32. Nakov R, Velikova T. Chemical metabolism of xenobiotics by gut microbiota. Curr Drug Metab. (2020) 21:260–9. doi: 10.2174/1389200221666200303113830
33. Mammo FK, Amoah ID, Gani KM, Pillay L, Ratha SK, Bux F, et al. Microplastics in the environment: Interactions with microbes and chemical contaminants. Sci Total Environ. (2020) 743:140518. doi: 10.1016/j.scitotenv.2020.140518
34. Shreiner AB, Kao JY, Young VB. The gut microbiome in health and in disease. Curr Opin Gastroenterol. (2015) 31:69–75. doi: 10.1097/MOG.0000000000000139
35. Huttenhower C, Gevers D, Knight R, Abubucker S, Badger JH, Chinwalla AT, et al. Structure, function and diversity of the healthy human microbiome. Nature. (2012) 486:207–14. doi: 10.1038/nature11234
36. Grice EA, Segre JA. The human microbiome: Our second genome. Annu Rev Genomics Hum Genet. (2012) 13:151–70. doi: 10.1146/annurev-genom-090711-163814
37. Tudela H, Claus SP, Saleh M. Next generation microbiome research: identification of keystone species in the metabolic regulation of host-gut microbiota interplay. Front Cell Dev Biol. (2021) 9:719072. doi: 10.3389/fcell.2021.719072
38. Haber AL, Biton M, Rogel N, Herbst RH, Shekhar K, Smillie C, et al. A single-cell survey of the small intestinal epithelium. Nature. (2017) 551:333–9. doi: 10.1038/nature24489
39. Lloyd-Price J, Abu-Ali G, Huttenhower C. The healthy human microbiome. Genome Med. (2016) 8:51. doi: 10.1186/s13073-016-0307-y
40. Geirnaert A, Calatayud M, Grootaert C, Laukens D, Devriese S, Smagghe G, et al. Butyrate-producing bacteria supplemented in vitro to Crohn's disease patient microbiota increased butyrate production and enhanced intestinal epithelial barrier integrity. Sci Rep. (2017) 7:11450. doi: 10.1038/s41598-017-11734-8
41. Zeng MY, Inohara N, Nuñez G. Mechanisms of inflammation-driven bacterial dysbiosis in the gut. Mucosal Immunol. (2017) 10:18–26. doi: 10.1038/mi.2016.75
42. Paulos CM, Wrzesinski C, Kaiser A, Hinrichs CS, Chieppa M, Cassard L, et al. Microbial translocation augments the function of adoptively transferred self/tumor-specific CD8+ T cells via TLR4 signaling. J Clin Invest. (2007) 117:2197–204. doi: 10.1172/JCI32205
43. Rowland I, Gibson G, Heinken A, Scott K, Swann J, Thiele I, et al. Gut microbiota functions: metabolism of nutrients and other food components. Eur J Nutr. (2018) 57:1–24. doi: 10.1007/s00394-017-1445-8
44. Yoo JY, Groer M, Dutra SVO, Sarkar A, McSkimming DI. Gut microbiota and immune system interactions. Microorganisms. (2020) 8:1–22. doi: 10.3390/microorganisms8101587
45. Zheng D, Liwinski T, Elinav E. Interaction between microbiota and immunity in health and disease. Cell Res. (2020) 30:492–506. doi: 10.1038/s41422-020-0332-7
46. Rothschild D, Weissbrod O, Barkan E, Kurilshikov A, Korem T, Zeevi D, et al. Environment dominates over host genetics in shaping human gut microbiota. Nature. (2018) 555:210–5. doi: 10.1038/nature25973
47. Claus SP, Guillou H, Ellero-Simatos S. The gut microbiota: a major player in the toxicity of environmental pollutants? NPJ Biofilms Microbiomes. (2016) 2:1–11. doi: 10.1038/npjbiofilms.2016.3
48. Koppel N, Rekdal VM, Balskus EP. Chemical transformation of xenobiotics by the human gut microbiota. Science. (2017) 356:1246–57. doi: 10.1126/science.aag2770
49. Collins SL, Patterson AD. The gut microbiome: an orchestrator of xenobiotic metabolism. Acta Pharm Sin B. (2020) 10:19–32. doi: 10.1016/j.apsb.2019.12.001
50. Abdelsalam NA, Ramadan AT, ElRakaiby MT, Aziz RK. Toxicomicrobiomics: the human microbiome vs. pharmaceutical, dietary, and environmental xenobiotics. Front Pharmacol. (2020) 11:390. doi: 10.3389/fphar.2020.00390
51. Rosenfeld CS. Gut dysbiosis in animals due to environmental chemical exposures. Front Cell Infect Microbiol. (2017) 7:396. doi: 10.3389/fcimb.2017.00396
52. Durack J, Lynch S V. The gut microbiome: relationships with disease and opportunities for therapy. J Exp Med. (2019) 216:20–40. doi: 10.1084/jem.20180448
53. Lai PS, Christiani DC. Impact of occupational exposure on human microbiota. Curr Opin Allergy Clin Immunol. (2019) 19:86–91. doi: 10.1097/ACI.0000000000000502
54. Giambò F, Teodoro M, Costa C, Fenga C. Toxicology and microbiota: how do pesticides influence gut microbiota? a review. Int J Environ Res Public Health. (2021) 18:5510. doi: 10.3390/ijerph18115510
55. Giambò F, Italia S, Teodoro M, Briguglio G, Furnari N, Catanoso R, et al. Influence of toxic metal exposure on the gut microbiota (Review). World Acad Sci J. (2021) 3:90. doi: 10.3892/wasj.2021.90
56. Utembe W. Kamng'ona AW. Gut microbiota-mediated pesticide toxicity in humans: Methodological issues and challenges in risk assessment of pesticides. Chemosphere. (2021) 271:129817. doi: 10.1016/j.chemosphere.2021.129817
57. Mao Q, Manservisi F, Panzacchi S, Mandrioli D, Menghetti I, Vornoli A, et al. The Ramazzini Institute 13-week pilot study on glyphosate and Roundup administered at human-equivalent dose to Sprague Dawley rats: effects on the microbiome. Environ Heal A Glob Access Sci Source. (2018) 17:50. doi: 10.1186/s12940-018-0394-x
58. Ruuskanen S, Rainio MJ, Gómez-Gallego C, Selenius O, Salminen S, Collado MC, et al. Glyphosate-based herbicides influence antioxidants, reproductive hormones and gut microbiome but not reproduction: a long-term experiment in an avian model. Environ Pollut. (2020) 266:115108. doi: 10.1016/j.envpol.2020.115108
59. Krause JL, Haange SB, Schäpe SS, Engelmann B, Rolle-Kampczyk U, Fritz-Wallace K, et al. The glyphosate formulation Roundup® LB plus influences the global metabolome of pig gut microbiota in vitro. Sci Total Environ. (2020) 745:140932. doi: 10.1016/j.scitotenv.2020.140932
60. Ding W, Shangguan Y, Zhu Y, Sultan Y, Feng Y, Zhang B, Liu Y, Ma J, Li X. Negative impacts of microcystin-LR and glyphosate on zebrafish intestine: linked with gut microbiota and microRNAs? Environ Pollut. (2021) 286:117685. doi: 10.1016/j.envpol.2021.117685
61. Tang Q, Tang J, Ren X, Li C. Glyphosate exposure induces inflammatory responses in the small intestine and alters gut microbial composition in rats. Environ Pollut. (2020) 261:114129. doi: 10.1016/j.envpol.2020.114129
62. Nielsen LN, Roager HM, Casas ME, Frandsen HL, Gosewinkel U, Bester K, et al. Glyphosate has limited short-term effects on commensal bacterial community composition in the gut environment due to sufficient aromatic amino acid levels. Environ Pollut. (2018) 233:364–76. doi: 10.1016/j.envpol.2017.10.016
63. Liang Y, Zhan J, Liu D, Luo M, Han J, Liu X, et al. Organophosphorus pesticide chlorpyrifos intake promotes obesity and insulin resistance through impacting gut and gut microbiota. Microbiome. (2019) 7:19. doi: 10.1186/s40168-019-0635-4
64. Joly Condette C, Bach V, Mayeur C, Gay-Quéheillard J, Khorsi-Cauet H. Chlorpyrifos exposure during perinatal period affects intestinal microbiota associated with delay of maturation of digestive tract in rats. J Pediatr Gastroenterol Nutr. (2015) 61:30–40. doi: 10.1097/MPG.0000000000000734
65. Reygner J, Condette CJ, Bruneau A, Delanaud S, Rhazi L, Depeint F, et al. Changes in composition and function of human intestinal microbiota exposed to chlorpyrifos in oil as assessed by the SHIME® model. Int J Environ Res Public Health. (2016) 13:1088. doi: 10.3390/ijerph13111088
66. Alberoni D, Favaro R, Baffoni L, Angeli S, Di Gioia D. Neonicotinoids in the agroecosystem: in-field long-term assessment on honeybee colony strength and microbiome. Sci Total Environ. (2021) 762:144116. doi: 10.1016/j.scitotenv.2020.144116
67. Dong X, Shulzhenko N, Lemaitre J, Greer RL, Peremyslova K, Quamruzzaman Q, et al. Arsenic exposure and intestinal microbiota in children from Sirajdikhan, Bangladesh. PLoS ONE. (2017) 12:e0188487. doi: 10.1371/journal.pone.0188487
68. Wang H-T, Ma L, Zhu D, Ding J, Li G, Jin B-J, et al. Responses of earthworm Metaphire vulgaris gut microbiota to arsenic and nanoplastics contamination. Sci Total Environ. (2022) 806:150279. doi: 10.1016/j.scitotenv.2021.150279
69. Eggers S, Safdar N, Sethi AK, Suen G, Peppard PE, Kates AE, et al. Urinary lead concentration and composition of the adult gut microbiota in a cross-sectional population-based sample. Environ Int. (2019) 133:105122. doi: 10.1016/j.envint.2019.105122
70. Yu L, Yu Y, Yin R, Duan H, Qu D, Tian F, et al. Dose-dependent effects of lead induced gut injuries: An in vitro and in vivo study. Chemosphere. (2021) 266:129130. doi: 10.1016/j.chemosphere.2020.129130
71. Podany A, Rauchut J, Wu T, Kawasawa YI, Wright J, Lamendella R, et al. Excess dietary zinc intake in neonatal mice causes oxidative stress and alters intestinal host–microbe interactions. Mol Nutr Food Res. (2019) 63:947. doi: 10.1002/mnfr.201800947
72. Xie S, Zhou A, Wei T, Li S, Yang B, Xu G, et al. Nanoplastics induce more serious microbiota dysbiosis and inflammation in the gut of adult zebrafish than microplastics. Bull Environ Contam Toxicol. (2021) 107:640–50. doi: 10.1007/s00128-021-03348-8
73. Zhu D, Chen QL, An XL, Yang XR, Christie P, Ke X, etn al. Exposure of soil collembolans to microplastics perturbs their gut microbiota and alters their isotopic composition. Soil Biol Biochem. (2018) 116:302–10. doi: 10.1016/j.soilbio.2017.10.027
74. Li B, Ding Y, Cheng X, Sheng D, Xu Z, Rong Q, et al. Polyethylene microplastics affect the distribution of gut microbiota and inflammation development in mice. Chemosphere. (2020) 244:125492. doi: 10.1016/j.chemosphere.2019.125492
75. Wang K, Li J, Zhao L, Mu X, Wang C, Wang M, et al. Gut microbiota protects honey bees (Apis mellifera L.) against polystyrene microplastics exposure risks. J Hazard Mater. (2021) 402:123828. doi: 10.1016/j.jhazmat.2020.123828
76. Cheng Y, Song W, Tian H, Zhang K, Li B, Du Z, et al. The effects of high-density polyethylene and polypropylene microplastics on the soil and earthworm Metaphire guillelmi gut microbiota. Chemosphere. (2021) 267:129219. doi: 10.1016/j.chemosphere.2020.129219
77. Syromyatnikov MY, Isuwa MM, Savinkova O V., Derevshchikova MI, Popov VN. The effect of pesticides on the microbiome of animals. Agriculture. (2020) 10:79. doi: 10.3390/agriculture10030079
78. Rueda-Ruzafa L, Cruz F, Roman P, Cardona D. Gut microbiota and neurological effects of glyphosate. Neurotoxicology. (2019) 75:1–8. doi: 10.1016/j.neuro.2019.08.006
79. He X, Tu Y, Song Y, Yang G, You M. The relationship between pesticide exposure during critical neurodevelopment and autism spectrum disorder: a narrative review. Environ Res. (2022) 203:111902. doi: 10.1016/j.envres.2021.111902
80. Suppa A, Kvist J, Li X, Dhandapani V, Almulla H, Tian AY, et al. Roundup causes embryonic development failure and alters metabolic pathways and gut microbiota functionality in non-target species. Microbiome. (2020) 8:170. doi: 10.1186/s40168-020-00943-5
81. Mesnage R, Teixeira M, Mandrioli D, Falcioni L, Ducarmon QR, Zwittink RD, et al. Use of shotgun metagenomics and metabolomics to evaluate the impact of glyphosate or roundup mon 52276 on the gut microbiota and serum metabolome of sprague-dawley rats. Environ Health Perspect. (2021) 129:1–15. doi: 10.1289/EHP6990
82. Hu J, Lesseur C, Miao Y, Manservisi F, Panzacchi S, Mandrioli D, et al. Low-dose exposure of glyphosate-based herbicides disrupt the urine metabolome and its interaction with gut microbiota. Sci Rep. (2021) 11:1–10. doi: 10.1038/s41598-021-82552-2
83. Mesnage R, Antoniou MN. Computational modelling provides insight into the effects of glyphosate on the shikimate pathway in the human gut microbiome. Curr Res Toxicol. (2020) 1:25–33. doi: 10.1016/j.crtox.2020.04.001
84. Leino L, Tall T, Helander M, Saloniemi I, Saikkonen K, Ruuskanen S, Puigbò P. Classification of the glyphosate target enzyme (5-enolpyruvylshikimate-3-phosphate synthase) for assessing sensitivity of organisms to the herbicide. J Hazard Mater. (2021) 408:124556. doi: 10.1016/j.jhazmat.2020.124556
85. Giambò F, Leone GM, Gattuso G, Rizzo R, Cosentino A, Cinà D, et al. Genetic and epigenetic alterations induced by pesticide exposure: integrated analysis of gene expression, microrna expression and dna methylation datasets. Int J Environ Res Public Health. (2021) 18:8697. doi: 10.3390/ijerph18168697
86. Costa C, Briguglio G, Giambò F, Catanoso R, Teodoro M, Caccamo D, et al. Association between oxidative stress biomarkers and PON and GST polymorphisms as a predictor for susceptibility to the effects of pesticides. Int J Mol Med. (2020) 45:1951–9. doi: 10.3892/ijmm.2020.4541
87. Costa C, Briguglio G, Catanoso R, Giambò F, Polito I, Teodoro M, et al. New perspectives on cytokine pathways modulation by pesticide exposure. Curr Opin Toxicol. (2020) 19:99–104. doi: 10.1016/j.cotox.2020.01.002
88. Réquilé M, Gonzàlez Alvarez DO, Delanaud S, Rhazi L, Bach V, Depeint F, et al. Use of a combination of in vitro models to investigate the impact of chlorpyrifos and inulin on the intestinal microbiota and the permeability of the intestinal mucosa. Environ Sci Pollut Res. (2018) 25:22529–40. doi: 10.1007/s11356-018-2332-4
89. Rothman JA, Russell KA, Leger L, McFrederick QS, Graystock P. The direct and indirect effects of environmental toxicants on the health of bumblebees and their microbiomes. Proc R Soc B Biol Sci. (2020) 287:20200980. doi: 10.1098/rspb.2020.0980
90. Mendler A, Geier F, Haange SB, Pierzchalski A, Krause JL, Nijenhuis I, et al. Mucosal-associated invariant T-Cell (MAIT) activation is altered by chlorpyrifos- and glyphosate-treated commensal gut bacteria. J Immunotoxicol. (2020) 17:10–20. doi: 10.1080/1547691X.2019.1706672
91. Kholodov AS, Tarasenko IA, Zinkova EA, Teodoro M, Docea AO, Calina D, et al. The study of airborne particulate matter in dalnegorsk town. Int J Environ Res Public Health. (2021) 18:9234. doi: 10.3390/ijerph18179234
92. Wu N, Wang X, Xu X, Cai R, Xie S. Effects of heavy metals on the bioaccumulation, excretion and gut microbiome of black soldier fly larvae (Hermetia illucens). Ecotoxicol Environ Saf . (2020) 192:110323. doi: 10.1016/j.ecoenv.2020.110323
93. Li X, Brejnrod AD, Ernst M, Rykær M, Herschend J, Olsen NMC, et al. Heavy metal exposure causes changes in the metabolic health-associated gut microbiome and metabolites. Environ Int. (2019) 126:454–67. doi: 10.1016/j.envint.2019.02.048
94. Duan H, Yu L, Tian F, Zhai Q, Fan L, Chen W. Gut microbiota: a target for heavy metal toxicity and a probiotic protective strategy. Sci Total Environ. (2020) 742:140429. doi: 10.1016/j.scitotenv.2020.140429
95. Yin N, Zhao Y, Wang P, Du H, Yang M, Han Z, et al. Effect of gut microbiota on in vitro bioaccessibility of heavy metals and human health risk assessment from ingestion of contaminated soils. Environ Pollut. (2021) 279:116943. doi: 10.1016/j.envpol.2021.116943
96. Shao M, Zhu Y. Long-term metal exposure changes gut microbiota of residents surrounding a mining and smelting area. Sci Rep. (2020) 10:4453. doi: 10.1038/s41598-020-61143-7
97. IARC Working Group on the Evaluation of Carcinogenic Risks to Humans. Arsenic, metals, fibres, and dusts. In: IARC Monographs on the Evaluation of Carcinogenic Risks to Humans. Lyon: International Agency for Research on Cancer (2012) 100:11–465.
98. Brabec JL, Wright J, Ly T, Wong HT, McClimans CJ, Tokarev V, et al. Arsenic disturbs the gut microbiome of individuals in a disadvantaged community in Nepal. Heliyon. (2020) 6:e03313. doi: 10.1016/j.heliyon.2020.e03313
99. Tikka C, Manthari RK, Ommati MM, Niu R, Sun Z, Zhang J, Wang J. Immune disruption occurs through altered gut microbiome and NOD2 in arsenic induced mice: Correlation with colon cancer markers. Chemosphere. (2020) 246:e125971. doi: 10.1016/j.chemosphere.2019.125791
100. Yan W, Hamid N, Deng S, Jia PP, Pei DS. Individual and combined toxicogenetic effects of microplastics and heavy metals (Cd, Pb, and Zn) perturb gut microbiota homeostasis and gonadal development in marine medaka (Oryzias melastigma). J Hazard Mater. (2020) 397:122795. doi: 10.1016/j.jhazmat.2020.122795
101. He X, Qi Z, Hou H, Qian L, Gao J, Zhang XX. Structural and functional alterations of gut microbiome in mice induced by chronic cadmium exposure. Chemosphere. (2020) 246:125747. doi: 10.1016/j.chemosphere.2019.125747
102. Ya J, Li X, Wang L, Kou H, Wang H, Zhao H. The effects of chronic cadmium exposure on the gut of Bufo gargarizans larvae at metamorphic climax: histopathological impairments, microbiota changes and intestinal remodeling disruption. Ecotoxicol Environ Saf . (2020) 195:110523. doi: 10.1016/j.ecoenv.2020.110523
103. Zhao Y, Zhou C, Wu C, Guo X, Hu G, Wu Q, et al. Subchronic oral mercury caused intestinal injury and changed gut microbiota in mice. Sci Total Environ. (2020) 721:137639. doi: 10.1016/j.scitotenv.2020.137639
104. Zhou C, Xu P, Huang C, Liu G, Chen S, Hu G, et al. Effects of subchronic exposure of mercuric chloride on intestinal histology and microbiota in the cecum of chicken. Ecotoxicol Environ Saf . (2020) 188:109920. doi: 10.1016/j.ecoenv.2019.109920
105. Murashov V, Geraci CL, Schulte PA, Howard J. Nano- and microplastics in the workplace. J Occup Environ Hyg. (2021) 18:1–6. doi: 10.1080/15459624.2021.1976413
106. Jiang B, Kauffman AE, Li L, McFee W, Cai B, Weinstein J, et al. Health impacts of environmental contamination of micro- And nanoplastics: a review. Environ Health Prev Med. (2020) 25:29. doi: 10.1186/s12199-020-00870-9
107. Yong CQY, Valiyaveetill S, Tang BL. Toxicity of microplastics and nanoplastics in Mammalian systems. Int J Environ Res Public Health. (2020) 17:1509. doi: 10.3390/ijerph17051509
108. Deng Y, Yan Z, Shen R, Wang M, Huang Y, Ren H, et al. Microplastics release phthalate esters and cause aggravated adverse effects in the mouse gut. Environ Int. (2020) 143:105916. doi: 10.1016/j.envint.2020.105916
109. Qiao R, Deng Y, Zhang S, Wolosker MB, Zhu Q, Ren H, et al. Accumulation of different shapes of microplastics initiates intestinal injury and gut microbiota dysbiosis in the gut of zebrafish. Chemosphere. (2019) 236:124334. doi: 10.1016/j.chemosphere.2019.07.065
110. Qiao J, Chen R, Wang M, Bai R, Cui X, Liu Y, et al. Perturbation of gut microbiota plays an important role in micro/nanoplastics-induced gut barrier dysfunction. Nanoscale. (2021) 13:8806–16. doi: 10.1039/d1nr00038a
111. Li LL, Amara R, Souissi S, Dehaut A, Duflos G, Monchy S. Impacts of microplastics exposure on mussel (Mytilus edulis) gut microbiota. Sci Total Environ. (2020) 745:141018. doi: 10.1016/j.scitotenv.2020.141018
112. Qiao R, Sheng C, Lu Y, Zhang Y, Ren H, Lemos B. Microplastics induce intestinal inflammation, oxidative stress, and disorders of metabolome and microbiome in zebrafish. Sci Total Environ. (2019) 662:246–53. doi: 10.1016/j.scitotenv.2019.01.245
113. Jin Y, Xia J, Pan Z, Yang J, Wang W, Fu Z. Polystyrene microplastics induce microbiota dysbiosis and inflammation in the gut of adult zebrafish. Environ Pollut. (2018) 235:322–9. doi: 10.1016/j.envpol.2017.12.088
114. Kang HM, Byeon E, Jeong H, Kim MS, Chen Q, Lee JS. Different effects of nano- and microplastics on oxidative status and gut microbiota in the marine medaka Oryzias melastigma. J Hazard Mater. (2021) 405:124207. doi: 10.1016/j.jhazmat.2020.124207
115. Huang JN, Wen B, Zhu JG, Zhang YS, Gao JZ, Chen ZZ. Exposure to microplastics impairs digestive performance, stimulates immune response and induces microbiota dysbiosis in the gut of juvenile guppy (Poecilia reticulata). Sci Total Environ. (2020) 733:138929. doi: 10.1016/j.scitotenv.2020.138929
116. Jin Y, Lu L, Tu W, Luo T, Fu Z. Impacts of polystyrene microplastic on the gut barrier, microbiota and metabolism of mice. Sci Total Environ. (2019) 649:308–17. doi: 10.1016/j.scitotenv.2018.08.353
117. Lu L, Wan Z, Luo T, Fu Z, Jin Y. Polystyrene microplastics induce gut microbiota dysbiosis and hepatic lipid metabolism disorder in mice. Sci Total Environ. (2018) 631–2:449–58. doi: 10.1016/j.scitotenv.2018.03.051
118. Luo T, Wang C, Pan Z, Jin C, Fu Z, Jin Y. Maternal polystyrene microplastic exposure during gestation and lactation altered metabolic homeostasis in the dams and their F1 and F2 offspring. Environ Sci Technol. (2019) 53:10978–92. doi: 10.1021/acs.est.9b03191
Keywords: microbiota, environmental pollutants, occupational medicine, chronic diseases, occupational toxicology
Citation: Giambò F, Costa C, Teodoro M and Fenga C (2022) Role-Playing Between Environmental Pollutants and Human Gut Microbiota: A Complex Bidirectional Interaction. Front. Med. 9:810397. doi: 10.3389/fmed.2022.810397
Received: 06 November 2021; Accepted: 18 January 2022;
Published: 16 February 2022.
Edited by:
Anca Oana Docea, University of Medicine and Pharmacy of Craiova, RomaniaReviewed by:
Robin Mesnage, King's College London, United KingdomTaxiarchis Konstantinos Nikolouzakis, University of Crete, Greece
Copyright © 2022 Giambò, Costa, Teodoro and Fenga. This is an open-access article distributed under the terms of the Creative Commons Attribution License (CC BY). The use, distribution or reproduction in other forums is permitted, provided the original author(s) and the copyright owner(s) are credited and that the original publication in this journal is cited, in accordance with accepted academic practice. No use, distribution or reproduction is permitted which does not comply with these terms.
*Correspondence: Michele Teodoro, bWljaGVsZS50ZW9kb3JvQHVuaW1lLml0