- 1Division of Bacteriology, Indian Council of Medical Research (ICMR)-National Institute of Cholera and Enteric Diseases, Kolkata, India
- 2Division of Molecular Microbiology, Indian Council of Medical Research (ICMR)-National Institute of Cholera and Enteric Diseases, Kolkata, India
Acinetobacter baumannii (A. baumannii) is a leading cause of nosocomial infections as this pathogen has certain attributes that facilitate the subversion of natural defenses of the human body. A. baumannii acquires antibiotic resistance determinants easily and can thrive on both biotic and abiotic surfaces. Different resistance mechanisms or determinants, both transmissible and non-transmissible, have aided in this victory over antibiotics. In addition, the propensity to form biofilms (communities of organism attached to a surface) allows the organism to persist in hospitals on various medical surfaces (cardiac valves, artificial joints, catheters, endotracheal tubes, and ventilators) and also evade antibiotics simply by shielding the bacteria and increasing its ability to acquire foreign genetic material through lateral gene transfer. The biofilm formation rate in A. baumannii is higher than in other species. Recent research has shown how A. baumannii biofilm-forming capacity exerts its effect on resistance phenotypes, development of resistome, and dissemination of resistance genes within biofilms by conjugation or transformation, thereby making biofilm a hotspot for genetic exchange. Various genes control the formation of A. baumannii biofilms and a beneficial relationship between biofilm formation and “antimicrobial resistance” (AMR) exists in the organism. This review discusses these various attributes of the organism that act independently or synergistically to cause hospital infections. Evolution of AMR in A. baumannii, resistance mechanisms including both transmissible (hydrolyzing enzymes) and non-transmissible (efflux pumps and chromosomal mutations) are presented. Intrinsic factors [biofilm-associated protein, outer membrane protein A, chaperon-usher pilus, iron uptake mechanism, poly-β-(1, 6)-N-acetyl glucosamine, BfmS/BfmR two-component system, PER-1, quorum sensing] involved in biofilm production, extrinsic factors (surface property, growth temperature, growth medium) associated with the process, the impact of biofilms on high antimicrobial tolerance and regulation of the process, gene transfer within the biofilm, are elaborated. The infections associated with colonization of A. baumannii on medical devices are discussed. Each important device-related infection is dealt with and both adult and pediatric studies are separately mentioned. Furthermore, the strategies of preventing A. baumannii biofilms with antibiotic combinations, quorum sensing quenchers, natural products, efflux pump inhibitors, antimicrobial peptides, nanoparticles, and phage therapy are enumerated.
Introduction
Acinetobacter baumannii occupies the upper echelon of the WHO priority pathogen list- “critical,” a position indicative of how important this nosocomial pathogen is, particularly when they are resistant to the “last resort” antibiotic, carbapenem (1–3). Acinetobacter causes a wide range of infections mostly acquired in clinical settings and is frequently associated with high morbidity and mortality rates (26–60%) (4, 5). The rate of mortality due to multidrug-resistant (MDR) and extensively drug-resistant (XDR) strains of A. baumannii infections is high and several outbreaks have been reported worldwide (5, 6). A. baumannii infections frequently occur in patients in ICUs on life-support systems prolonging their stay in hospitals and treatment failures are frequently encountered (7). Outside the hospital environment, A. baumannii has been isolated from a wide range of environmental samples including soil, aquatic environment, animals, humans, food items including raw vegetables, gardens, inanimate objects and even from body lice samples of homeless people, which serve as the reservoirs for this bacterium (8, 9). This species has now gone beyond hospitals and is being reported to cause community-acquired infections (10). Infections in both paediatric and adult populations indicate that as with other pathogens, the vulnerable are targeted (11). All these factors clearly indicate why A. baumannii is considered a critical pathogen.
Pathogens can grow and replicate even under unfavorable conditions. The tools that they use are diverse and many, sometimes as simple as the ability to persist in an environment. This ability to persist, particularly in adverse conditions such as hospitals where the use of antibiotics and disinfectants are high, gives Acinetobacter a clear advantage. Acinetobacter can survive in hostile environments (desiccation, antimicrobial therapies, nutrient unavailability) and can colonize biotic and abiotic surfaces for prolonged periods of time due to their ability to form complex structures called biofilms (12, 13). Biofilm formation is an important virulence mechanism and a hallmark characteristic of A. baumannii. Numerous microbial features (e.g., adhesins, capsular polysaccharides, surface appendages, virulence genes, resistance determinants), physicochemical factors (temperature, growth media, surface hydrophobicity, pH, oxygen concentration), and various other factors [biofilm-associated protein (Bap), the outer membrane protein A (OmpA), chaperon-usher pilus (Csu), iron uptake mechanism, poly-β-(1, 6)-N-acetyl glucosamine (PNAG), two-component system (BfmS/BfmR), PER-1], facilitate the formation and maintenance of the A. baumannii biofilms (13).
Acinetobacter spp. can form biofilm at both air-liquid and solid-liquid interface. The biofilm formation rate in A. baumannii at the solid-liquid interface is 80–91%, which is 3 times higher than other Acinetobacter species (5–24%) (14–16). In addition, these isolates are able to form biofilm at the air-liquid interface, known as pellicle, which increases the surface-associated motility of the bacterium. However, pellicle formation is a rare trait in A. baumannii and a limited number of genes are essential for the expression of this phenotype, but within the ACB-complex, pellicle formation was almost four times higher for A. baumannii than other Acinetobacter genospecies (15, 17). Acinetobacter infections may be more difficult to treat when forming a biofilm and may be readily transmissible from patient to patient, making outbreaks that are difficult to control. Hospital surfaces and surfaces of medical devices such as cardiac valves, artificial joints, ventilators, urinary or intravascular catheters, endotracheal tubes made of polystyrene, polypropylene, polytetrafluoroethylene, and glass are excellent for biofilm formation (18). Indwelling devices provide pathogens a mode of entry into the body, therefore patients admitted to the hospitals are at high risk of Acinetobacter infection as Acinetobacter can colonize on abiotic surfaces efficiently. A recent study suggested that the clinical isolates of A. baumannii have better ability to form biofilm on abiotic surfaces than non-clinical isolates. Therefore, the high capability of A. baumannii to colonize and form biofilm on abiotic surfaces is considered an important factor contributing to chronic and persistent infections in hospital settings (19). This subsequently enhances the risk of infectious diseases such as cystic fibrosis, periodontitis, bloodstream infections, urinary & respiratory tract infections, burn-wound infections, chronic non-healing injury, endocarditis, necrotizing fasciitis, etc. (18, 20, 21). Moreover, A. baumannii is able to maintain its virulence even after long periods of survival in the hospital environment, which could facilitate infections (22).
In addition to the ability to form biofilms, the deft with which Acinetobacter acquires antibiotic resistance genes and also transmits them provides the species with an additional advantage in hospitals where the use of antibiotics is always higher than in other environments. Studies have been showing horizontal gene transfer (HGT) of antibiotic resistance genes (carbapenemases, oxacillinases, metallo-beta-lactamases, or metal resistance genes) via conjugation, transformation, bacteria phage-mediated, nanotube-mediated, or via outer membrane vesicles (23–29). The mechanism of resistance is similar to other Gram-negative bacteria (GNB) which employ hydrolyzing enzymes or modifying enzymes, pumps to efflux antibiotics, and decreased entry of antibiotics. Acinetobacter is reported to possess numerous pumps and several enzymes (30). The ability to transmit these determinants is in no way lesser than the ability to acquire; transformation, conjugation, and outer membrane vesicles contribute to the spread of resistance determinants.
Acinetobacter utilizes its abilities to form biofilm and acquire antibiotic resistance determinants to evade the immune system, which provides one-thousand times more tolerance to antimicrobials by shielding the bacteria from treatment with antibiotics (13, 18, 21). Numerous studies have reported a constructive relationship between biofilm formation and antibiotic resistance in A. baumannii isolates (13, 21, 31, 32). Cells in the biofilm not only tolerate antibiotic pressure but biofilm formation enhance their ability to acquire foreign genetic material through lateral gene transfer that promotes their survival in presence of antibiotics (13, 31).
With the increasing importance of A. baumannii as a nosocomial pathogen, the factors that give this organism an advantage have been reviewed. The review gives a holistic view of presently available information about (i) the aspect of antibiotic resistance and biofilm production by A. baumannii; (ii) its clinical significance; (iii) how biofilm production and antibiotic resistance add to the challenges of biofilm-mediated nosocomial infection; and (iv) recent developments in potential approaches to prevent A. baumannii biofilm formation by disrupting components of the biofilm matrix.
A. baumannii and the Tryst With Antibiotics
The genus Acinetobacter was discovered in 1911 by Beijerinck as Micrococcus calcoaceticus from the soil on a calcium acetate-mineral medium (33) and eventually, in the 1950s it became known as Acinetobacter (34). The genus Acinetobacter (the name came from the Greek word akinetos, i.e., non-motile), was originally suggested by Brisou and Prevot (35). Since then, more than 32 Acinetobacter spp. have been reported of which A. baumannii is most prevalent in clinical settings (4). Nosocomial infections, higher mortality among patients, and a higher degree of antimicrobial resistance are mostly encountered in A. baumannii compared to non-baumannii species (36).
Evolution of Antimicrobial Resistance: The Timeline
Up to the early 1970s, Acinetobacter strains showed susceptibility to most antibiotics including ampicillin, carbenicillin, nalidixic acid, and gentamicin (37). The resistance to sulfonamide, β-lactam, and aminoglycoside was noticed among Acinetobacter by the end of the 1970s as these drugs had already been used in clinical practice long before 1970 (38). Increased rate of resistance to many classes of antimicrobials was noticed among Acinetobacter in the 1980s including quinolones. Carbapenem resistance was first detected in 1985, the same year imipenem was discovered (39). Carbapenem-resistant A. baumannii is being frequently reported all over the world and the prevalence of carbapenem-resistant A. baumannii is high in developing countries including India, Pakistan, Chile, Korea, Portugal compared to the developed countries (40, 41). With the emergence of carbapenem resistance, as an alternative, colistin had been deployed to treat carbapenem-resistant bacteria, resulting in colistin resistance. The first report of colistin-resistant Acinetobacter came from the Czech Republic in 1999 (42). Since then, colistin resistance in A. baumannii has been reported worldwide including the USA, Europe, Spain, Korea, Iran, India (43). Apart from carbapenem and colistin resistance, resistance to tigecycline, the first member of the family of glycycline was reported in 2005 from Israel (44), the same year when it was approved by the US Food and Drug Administration (45). Tigecycline resistance has now been reported from all over the world (46). The timeline of the introduction of antimicrobials (approximate year) into the clinical practice and evolution of antimicrobial resistance in A. baumannii has been shown in Figure 1. For the creation of this figure, information was taken from different studies (47–49).
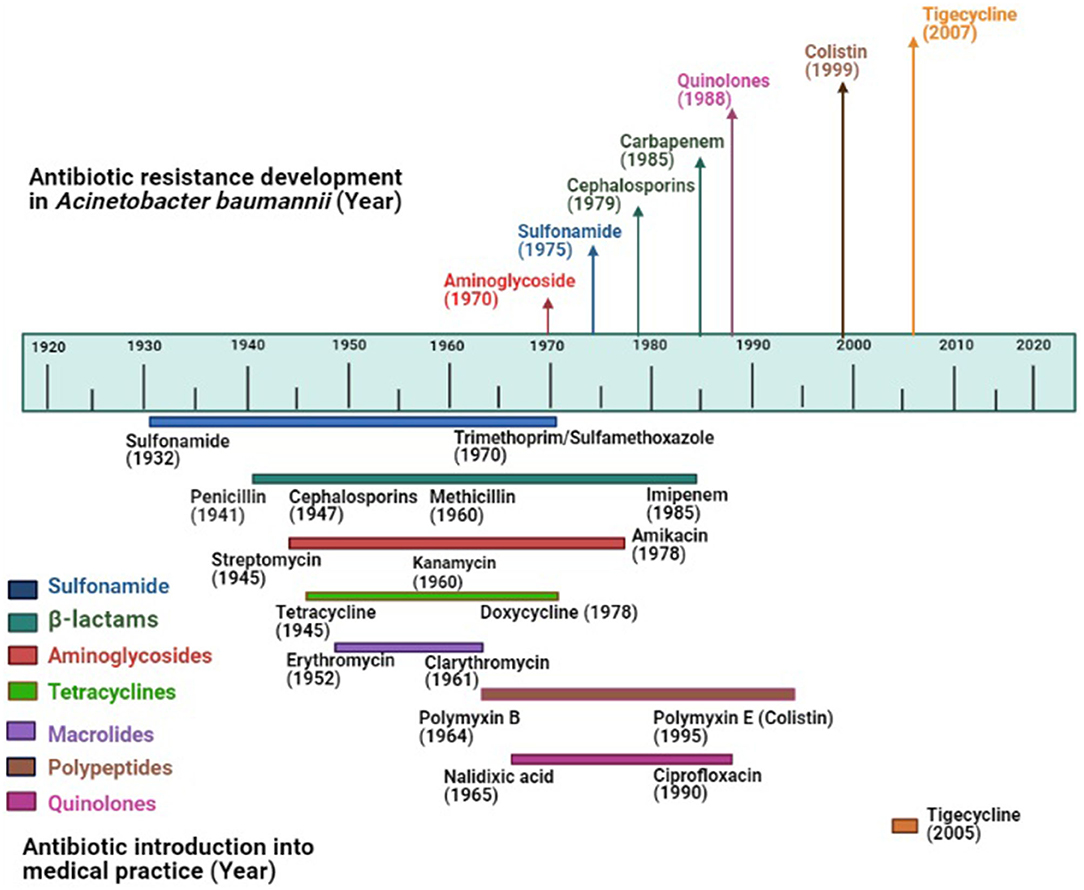
Figure 1. Evolution of antimicrobial resistance among Acinetobacter baumannii: Top portion of the diagram shows the year of the first report of antimicrobial resistance in A. baumannii; the lower portion shows the year of introduction of antimicrobials (approximate year) in the market where colored lines indicate different antimicrobial groups.
Enzymatic Mechanisms of Resistance: Degradation and Modification
The emergence of multidrug-resistant A. baumannii has been attributed to its ability to rapidly accumulate resistance determinants as well as being well-suited for genetic exchange. Recent studies have shown that A. baumannii has natural competence to incorporate exogenous DNA and its genome has foreign DNA at high frequencies, implying frequent HGT in this pathogen (50–52). Therefore, Acinetobacter belongs to a unique class of GNB that are characterized as “naturally transformable” and a large number of β-lactamases have been identified in this human pathogen (53, 54). These β-lactamases, depending on their amino acid sequences, can be grouped into four classes (Ambler group A, B, C, and D) among which classes A, C, and D contain serine at the active site and class B have Zn in the catalytic site (55). Class A β-lactamases are capable of hydrolyzing cephalosporins, penicillins and are inhibited by clavulanic acid (56). Many class A β-lactamases are reported in A. baumannii, such as TEM, SHV, CTX-M, GES, PER, VEB, SCO, or KPC. Of these, most are broad-spectrum β-lactamases (Extended Spectrum β-lactamases, ESBLs) (SHV-5, TEM-92, CTX-M-2, CTX-M-15, PER-1, PER-2, PER-7, VEB-1, and GES-14) while TEM-1 and SCO-1 are narrow-spectrum (30, 57, 58). In A. baumannii, another mechanism of resistance to third-generation cephalosporins is the overexpression of chromosomally-mediated AmpC which is a class C β-lactamase (10, 30, 59). In several clinical isolates of A. baumannii, ampC gene was found to be transcribed from a strong promoter contained within a putative insertion sequence element (ISAba1-like sequence), resulting in high resistance to ceftazidime (60). However, it was found that the exact contribution of ESBLs is complicated by the simultaneous presence of AmpC enzymes (53). Class D β-lactamases are also present in A. baumannii known as OXAs (oxacillinases) because they commonly hydrolyze isoxazolylpenicillin, oxacillin, much faster than benzylpenicillin (61). More than 400 OXA-type enzymes have been identified and many variants actually possess carbapenemase activity. OXA-23, OXA-24/40, OXA-58, OXA-143, and OXA-235 are examples of oxacillinases that are detected in A. baumannii and are able to hydrolyze carbapenems (10, 30, 62–64). Insertion of ISAba1 in the blaOXA−23 promoter sequence has been reported to be associated with overexpression of blaOXA−23, blaOXA−51, or blaOXA−58 in A. baumannii (65, 66). Class B β-lactamases which are very different from the other classes and known as MBLs (metallo-β-lactamases), can be inhibited by EDTA as they possess Zn at their active site (30). Several MBLs have been reported in A. baumannii such as IMP (imipenemases), VIM (Verona integron-encoded MBL), SPM (Sao Paolo MBL), SIM (Seoul imipenemase), GIM (imipenemase from Germany), and NDM (New Delhi MBL) (30, 67–71). These MBLs are the primary reason for carbapenem resistance in A. baumannii along with oxacillinases.
Apart from hydrolysis of antimicrobials by β-lactamases, enzymatic modification of the antibiotics is another mechanism of enzymatic resistance in A. baumannii. One of the best examples is the presence of three different aminoglycoside-modifying enzymes (acetyl transferases, nucleotidyl transferases, and phosphotransferases) which modify amino or hydroxyl- groups of the aminoglycosides (67).
Non-enzymatic Mechanisms of Resistance: The Active Pumps and More
Most GNB including A. baumannii also possess several non-enzymatic mechanisms of resistance which include efflux pumps, modifications of drug binding sites, and permeability defects.
To date, different categories of efflux pumps have been identified in A. baumannii: RND-family (resistance-nodulation-division), MFS-family (major facilitator superfamily), MATE-family (multidrug and toxic compound extrusion), and SMR-family (small multidrug resistance). The RND system more actively participates in antimicrobial resistance in A. baumannii and this family includes the AdeABC, AdeIJK, and AdeFGH efflux pumps (72). These efflux pumps are controlled by certain regulators such as AdeRS (two-component system), AdeL, and AdeN (72). Some other efflux pumps detected in A. baumannii include MATE-family (AbeM and CraA), MFS-family (AmvA, AbaF, and AbaQ), and SMR- family (AbeS) (72). tet(A), tet(B), and tet(G) are specific transposon-mediated efflux pumps also detected in A. baumannii (73).
Random point mutations, which are an important mechanism of bacterial resistance, alter the target site of antibiotic binding. The examples of such mechanisms among A. baumannii are (i) fluoroquinolone resistance due to spontaneous mutations in gyrase and topoisomerase IV encoding genes, i.e., gyrA, gyrB, and parC, parE; (ii) rifampin resistance due to point mutations in the RNA polymerase encoding gene rpoB; (iii) colistin resistance due to mutations in PmrAB two-component system and lpxA, lpxC, lpxD genes; and (iv) aminoglycoside resistance due to mutations in 16S ribosomal RNA gene armA (10, 30, 67, 74). Moreover, carbapenem resistance in A. baumannii is also associated with mutations in Penicillin Binding Protein PBP-2 (30, 67, 75).
Porins also play a significant role in antimicrobial resistance among A. baumannii. Decreased expression of several porins (Omp22–23, Omp33–36, Omp37, Omp43, Omp44, Omp47, OmpA, and CarO) has been noted in carbapenem-resistant A. baumannii (30, 76). A schematic diagram of the several antimicrobial resistance mechanisms has been depicted in Figure 2.
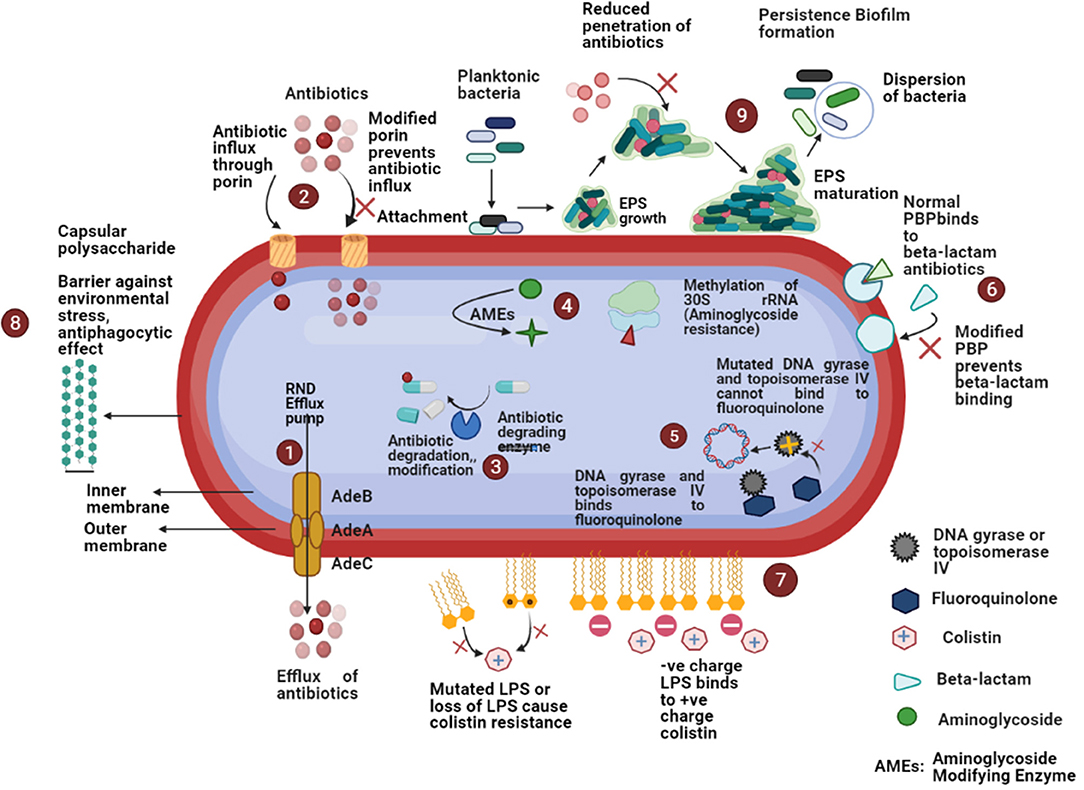
Figure 2. Schematic diagram of different antimicrobial resistance mechanisms in A. baumannii: (1) increased expression of efflux pumps that expel out antibiotics from the bacterial cell; (2) reduced expression of porin or porin loss results in the decreased antibiotic entry; (3) β-lactamases cause enzymatic inactivation of antibiotics; (4) aminoglycoside modifying enzymes decrease the affinity of aminoglycoside antibiotics for ribosomal subunit or methylation of 30S rRNA decrease the binding of aminoglycosides; (5) mutations in topoisomerase IV and DNA gyrase decrease the binding of fluoroquinolones; (6) modification of penicillin-binding-proteins (PBPs) prevent the bindings of β-lactams; (7) modification of lipopolysaccharides (LPS) cause decreased binding of colistin; (8) presence of capsular polysaccharide acts as a barrier against environmental stress, anti-phagocytic effect, etc.; (9) ability to form biofilm cause high antimicrobial resistance.
A. baumannii and Biofilm: Involvement of Intrinsic and Extrinsic Factors
Biofilm is a complex multicellular three-dimensional structure of the assembled population of bacterial cells which is enclosed within an exopolymer matrix called extracellular polymeric substance (EPS) (77). The EPS comprises nucleic acids, carbohydrates, proteins, and other macromolecules. In the EPS matrix, the most abundant carbohydrates are glucose, galactose, and mannose followed by fucose, arabinose, xylose, rhamnose, galacturonic acid, and N-acetyl-glucosamine. The complexity of the biofilm is enhanced by the presence of extracellular proteins which stabilize the biofilms and extracellular DNA (eDNA) secreted by the cells (77).
Formation of the biofilms, also known as the biofilm cycle, involves six major stages: (i) reversible attachment of planktonic bacteria with surfaces followed by settlement; (ii) proliferation and aggregation of the adherent bacteria (irreversible attachment); (iii) formation of microcolonies which either look like mushrooms or towered structures; (iv) initiation of quorum sensing (communication pathway between cells) at a critical cell density facilitating biofilm formation, positioning of cells in the microcolonies, formation of water channels within the biofilm and detachment of cells; (v) biofilm maturation (maximum antibiotic tolerance can be observed at this stage due to the presence of thick polysaccharide matrix surrounding them); and (vi) detachment and dispersion of cells to colonize in another location (78).
Intrinsic Factors Associated With A. baumannii Biofilm Formation: The Tools Within
Biofilm formation in A. baumannii on biotic and abiotic surfaces is regulated and influenced by several intrinsic factors such as virulence genes or proteins, cellular structures, and phenotypic or genotypic features. The factors associated with biofilm formation and regulation in A. baumannii are summarized in Table 1 and shown in Figure 3.
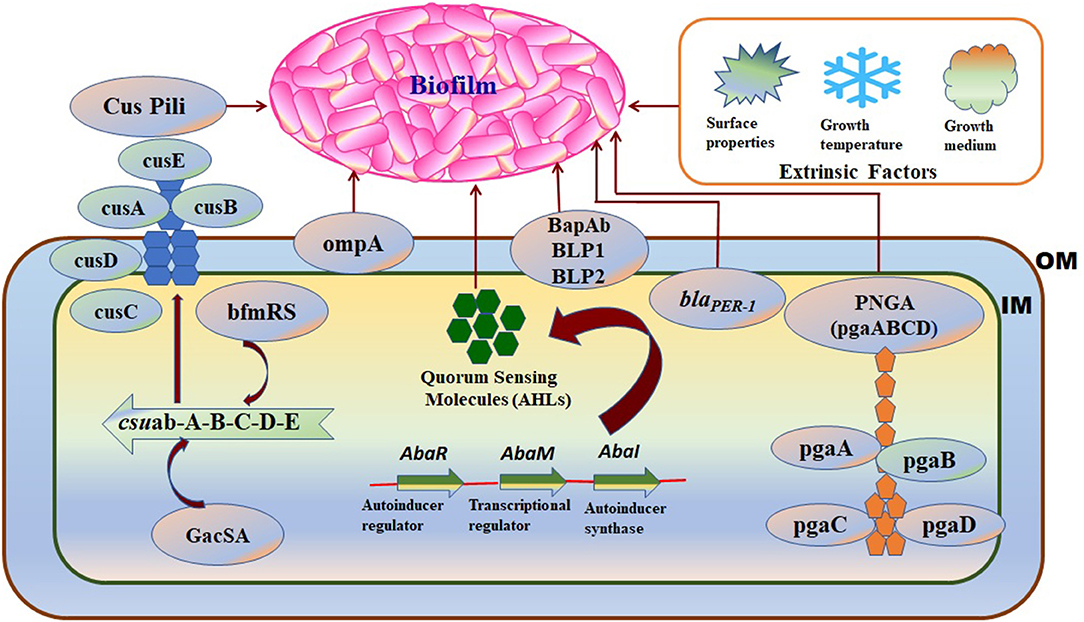
Figure 3. A schematic diagram representing the intrinsic factors (genes) and the extrinsic factors that regulate biofilm formation in A. baumannii: OM, Outer membrane; IM, Inner membrane. Intrinsic factors: PNAG, Poly-(1–6)-N-acetylglucosamine; Csu, Chaperon/usher pilus system; OmpA, Outer membrane protein A; blaPER− 1, Beta-lactamase PER-1; bap-Ab, A. baumannii biofilm-associated protein; AHLs, N-acyl homoserine lactones; Extrinsic factors: surface properties, growth temperature, and growth medium.
Biofilm Associated Protein (Bap)
Biofilm-associated protein (Bap) is a high-molecular-weight protein (8,620 amino acids), essential for cell-cell interactions, biofilm formation, and maturation on various surfaces including polypropylene, polystyrene, and titanium (89). BAP was first identified in A. baumannii by Loehfelm et al. and is identical to the Staphylococcus aureus Bap protein (79). A. baumannii Bap mainly targets carbohydrates in the host cells and is secreted via a type I secretion system. Several studies have documented the presence of Bap in A. baumannii strains and its association with strong biofilm formation (80, 90). Loehfelm et al. showed that Bap protein increases adherence to both normal human neonatal keratinocytes and bronchial epithelial cells (79). Many Bap-like proteins, BLP1 and BLP2 are also harbored by A. baumannii which coordinately contribute toward mature biofilm formation and adhesiveness to epithelial cells in a similar fashion as Bap (80).
Poly-β-(1, 6)-N-Acetlyglucosamine (PNAG)
Poly-β-(1, 6)-N-acetlyglucosamine (PNAG) is one of the major components of polysaccharides and is important for the development of biofilm in both GPB and GNB (81). PNAG is encoded by a cluster of four genes (pgaA, pgaB, pgaC, and pgaD) (82) which share similarities with E. coli and Y. pestis (91). pgaA plays an important role in protein-protein interaction and contains a porin domain which facilitates PNAG translocation through the outer membrane, pgaB involves in PNAG exportation via the outer membrane lipoprotein, pgaC helps in the synthesis of PNAG and pgaD restricts the cytoplasm and promotes pgaC in the synthesis of PNAG (91). Numerous studies showed PNAG is essential for maintaining the integrity of A. baumannii biofilms in a more dynamic and stressful environment (81, 91).
PER-1 β-lactamase
Acinetobacter baumannii adhesion and biofilm formation in both biotic and abiotic surfaces are enhanced by the presence and the expression of the blaPER−1 gene which is a class A extended-spectrum β-lactamase (92). Several studies found that increased cell adhesiveness and biofilm formation was higher in strains harboring the blaPER−1 gene than in those that do not harbor this genetic trait (83, 92, 93). However, Bardbari et al. reported no relationship between biofilm formation and production of PER-1 β-lactamase (94). Therefore, the presence of blaPER−1 probably increases the adhesion property of cells carrying this gene but does not necessarily contribute to biofilm formation.
Csuab-A-B-C-D-E Chaperone-Usher Pilli Assembly System
Chaperon–Usher secretion (CUS) system is required for bacterial attachment on abiotic surfaces that results in microcolony formation and development of biofilm (95). Csu pili are poly-cistronic in nature, adhesive surface organelles that consist of a tip fibrillum and adhesion protein (84). A. baumannii strains produce type I Csu pili that is encoded by an operon: Csuab-A-B-C-D-E. Furthermore, the expression of the Csuab-A-B-C-D-E operon in A. baumannii is regulated by a two-component system BfmRS where BfmR acts as a response regulator and BfmS acts as a sensor kinase (85, 96). Moreover, a second two-component system GacSA controls the Csu pilli gene expression and is indirectly involved in biofilm formation in A. baumannii (97). Other putative chaperone usher pili systems and Pap pili systems, which are homologous to the P pili of E. coli, have also been implicated in A. baumannii biofilm formation and maintenance (98).
Outer Membrane Proteins
Porins are the outer membrane proteins (Omps) that modulate cellular permeability, have an essential role in adaptation, environmental communication, and also play an important role in microbial virulence through drug exclusion mechanisms across the outer membrane channels (86). The outer membrane protein A (OmpA) is a well-recognized and well-characterized virulence factor of A. baumannii and is necessary for the development of robust biofilms on abiotic surfaces (86). The two-component system BfmS/R regulates biofilm formation, pilus, and OmpA expression, along with serum sensitivity (99). Another outer membrane protein of A. baumannii is the Omp 33–36 kDa protein which acts as a channel for water and is associated with resistance to carbapenem antibiotics. Moreover, knockout of omp 33–36 gene in A. baumannii strains had defective growth rate and significantly reduced capability of adherence, invasion, biofilm formation, and cytotoxicity in biotic surfaces (100).
Quorum Sensing (QS)
Quorum sensing (QS) is a cell-to-cell communication process that depends on the bacterial population density. Several small diffusible signaling molecules are involved in QS which activate the expression of genes that control a variety of functions such as virulence, motility, biofilm formation, bioluminescence, and sporulation (87, 101). These diffusible signaling molecules termed autoinducers include oligopeptides in GPB and N acyl-homoserine lactones (AHLs) in GNB (102). The predominant AHL produced by A. baumannii is 3-hydroxy-C12- homoserine lactone. The QS system in A. baumannii is regulated by a two-component system, AbaI/AbaR which is homologous to the typical LuxI/LuxR system found in GNB. abaI encodes the autoinducer synthases which catalyze the synthesis of AHL and AbaR that functions as receptor proteins for AHLs (103). A previous study had shown that mutation of the AHL synthase AbaI affects the surface-associated motility and biofilm formation in A. baumannii. A functional QS system is required for surface-associated motility and robust biofilm formation in A. baumannii ATCC®17978 (104). In addition, another gene named abaM is an uncharacterized member of the RsaM protein family located between abaR and abaI, has been found to play a key role in regulating A. baumannii QS, virulence, surface motility, and biofilm formation (88). The expression and upregulation of another two-component system BfmS/R has also been linked to the QS molecules that enhance the ability of A. baumannii to form biofilm on abiotic surfaces (105). Quorum sensing deficiency causes thinner biofilm formation and lower EPS production, thereby increasing the susceptibility to antibiotics.
Efflux Pumps
Efflux pumps are membrane proteins, that can extrude a wide group of substrates, including antibiotics, detergents, dyes, toxins, and waste metabolites. Several studies suggest that efflux pumps play major roles in biofilm formation and maturation by different mechanisms: efflux of EPSs and quorum quenching (QQ) molecules to facilitate biofilm matrix formation; indirect regulation of genes involved in biofilm formation and efflux of antibiotics or metabolic intermediates (106, 107). There are three types of RND efflux pumps associated with A. baumannii: AdeABC, AdeFGH, and AdeIJK. Yoon et al. reported that mutant strains of AdeABC, AdeFGH, and AdeIJK efflux pumps have significantly reduced biofilm formation in comparison with the wild-type strain. Therefore, biofilm formation in A. baumannii requires expression of efflux pump genes to initiate and maintain biofilm. Another study reported that the mutation of AdeABC and AdeIJK efflux genes were associated with lower expression of several pilus system-encoding proteins CsuA/B, CsuC, and FimA. These proteins play a key role in the initial stages of adhesion, surface colonization, and biofilm formation in A. baumannii (108). Richmond et al. presented that knockout of AdeAB efflux pumps in A. baumannii mutant strain caused significant reduction in biofilm formation on mucosal tissue compared with wild type strain. Therefore, the over-expression of AdeABC and AdeIJK efflux pump regulate the expression of pilus genes and biofilm production, and altered membrane composition in A. baumannii (108, 109). The third RND-type efflux pump AdeFGH is regulated by a LysR-type AdeL transcriptional regulator system. The over-expression of this efflux pump confers multidrug resistance and is linked to the synthesis and transport of autoinducer molecules during biofilm formation in A. baumannii.
Extrinsic Factors Associated With A. baumannii Biofilm Formation: the External Influences
Certain environmental factors that affect A. baumannii biofilm formation are summarized in Table 1 and shown in Figure 3.
Surface Property
Several factors such as roughness, physicochemical properties of a surface, and the presence of biological materials influence the attachment of A. baumannii to abiotic surfaces and the formation of biofilm (110). The ability of A. baumannii to form mature biofilms on polypropylene, polystyrene, titanium, and other medical-associated devices has been associated with several factors including pH, ionic composition, and biomaterial of protein adsorption (111). The presence of biomaterial such as blood, tears, urine, saliva, interstitial fluid, wound cultures and respiratory secretions influence the attachment of bacteria to its surface and promote the formation of biofilm (112). Polycarbonate surfaces are known to develop statistically more biofilm mass than glass, rubber, porcelain, and polypropylene (110). Latex catheters are low-priced and have more elasticity but are prone to bacterial adhesion and biofilm formation. Hence, silicone catheters are preferred over latex catheters (113).
Growth Temperature
Temperature also has an effect on biofilm formation. A. baumannii successfully survived at −20 to 44°C (114). However, different studies have been reported different optimum temperatures for biofilm formation in A. baumannii including 30°C at pH 7 in a medium containing sodium chloride or 25°C (115, 116). Another study showed that biofilm formation in A. baumannii on plastic surfaces was high at 28°C due to the upregulation of certain biofilm-associated proteins (BAPs), Csu pili, and iron-uptake proteins (111).
Growth Medium
The growth medium is also a factor that affects biofilm formation. It has been reported that a static environment with high nutrient containing medium (Tryptic Soy Broth or Brain Heart Infusion Broth) and supplemented with glucose, carbon, and cation sources (Na + sodium, Ca2+ calcium, Fe3+ ferric ion) influences the formation of A. baumannii biofilm than in hydrodynamic environment (117). These modulatory properties of medium and supplemented sources also influence the structural and mechanical properties of A. baumannii biofilms by lowering stiffness and increasing adhesiveness (117). However, clinical isolates of A. baumannii showed a significant reduction in adhesiveness and biofilm formation in the presence of an iron-chelating agent and ethanol on abiotic surfaces (83, 118).
A. baumannii Biofilms and Antibiotic Resistance: A Dangerous Liaison
Antimicrobial Resistance by Biofilm Cells: Understanding the Mechanisms
The term “biofilm resistance” signifies the survival of cells embedded within biofilms for long periods of time in presence of antimicrobials. Biofilm resistance does not indicate that biofilm cells show increased MIC compared to antibiotic-resistant planktonic cells (119). Biofilms are better equipped to evade antimicrobials than planktonic cells because biofilms are not easily destroyed by antimicrobials. Factors that are responsible for biofilm resistance and explain better survival of biofilm cells compared to planktonic cells in presence of antimicrobials are described below (Figure 4).
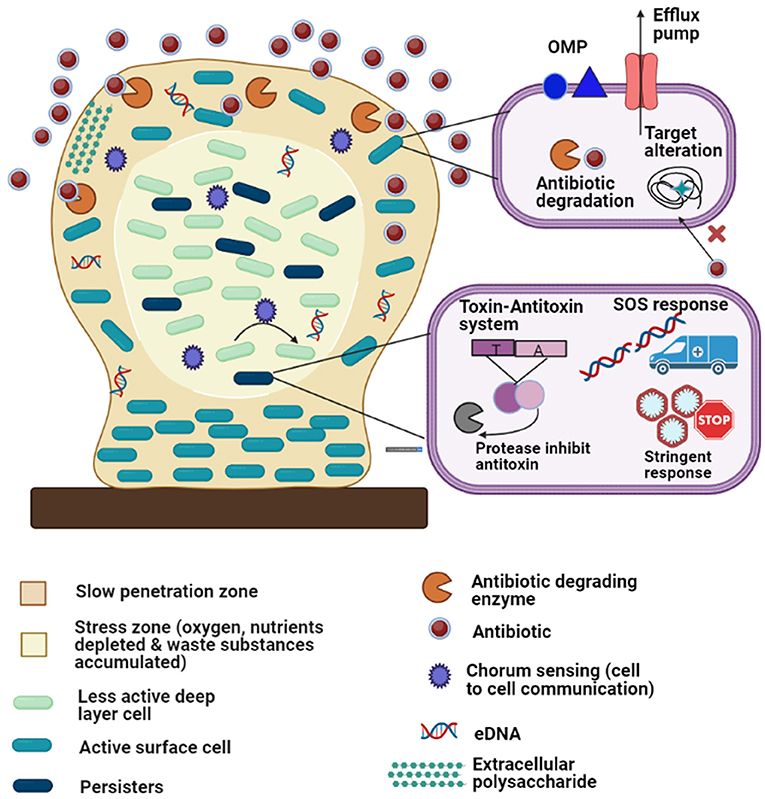
Figure 4. Diagrammatic representation of the antibiotic resistance mechanisms of biofilm-embedded bacterial cells: The biofilm is attached to a biotic or abiotic surface (brown rectangle). Development of persister cells (dark green) and less active deep layer cells (light green) in the stress zone (the core of the biofilm, light cream color) where fewer nutrients are available. The various resistant mechanisms depicted in the figure are as follows: (1) matrix exopolysaccharides cause slow penetration of antibiotics; (2) extracellular DNA (eDNA); (3) multidrug efflux pumps; (4) outer membrane protein; (5) antibiotic degrading enzymes and target modifications (6) quorum sensing; (7) stress responses (oxidative stress response, stringent response, etc.); (8) toxin-antitoxin system and (9) SOS responses.
Diffusion Inhibition of Antibiotics: Restricted Entry
Inhibition of antibiotic diffusion within biofilms can be attained via several mechanisms such as the presence of EPS matrix that serves as the diffusion barrier for antibiotic entry, antibiotic degradation with the help of production of exoenzymes (β-lactamases, β-galactosidase), metal immobilization or chelation, extracellular signaling, a mutation in the antibiotic target site and oxidation-mediated inactivation of antibiotics (120). These processes can lower antibiotic concentration to sublethal levels, leading to the selection of antibiotic-resistant cells within a biofilm. In addition, negatively charged eDNA facilitates the lowering of antibiotic concentrations within biofilms by binding to positively charged antibiotics such as aminoglycosides and gathers antibiotics up to 25% of its weight (121). eDNA can also enhance antibiotic resistance within biofilms by chelating cations such as Mg2+ and creating a cation-depleted environment leading to activation of two-component systems which are linked to AMR (121). It facilitates the transfer of antibiotic resistance genes within biofilms.
eDNA not only comes from lyzed cells but is also actively secreted which indicates that eDNA has an important role in biofilm formation. Its negative charge works as a repulsive force in the initial attachment, but when the distance between cell and surface becomes a few nanometers, eDNA interacts with receptors on the substratum surface to facilitate adhesion (122). Sahu et al. characterized eDNA from a multidrug-resistant clinical strain of A. baumannii and demonstrated its role in in vitro biofilm formation on abiotic surfaces. They supplemented biofilms with eDNA in various preparations, for example, whole-cell lysate, cell-free supernatant, MV suspension, and purified eDNA to mimic its natural availability during growth. Their experiment showed that eDNA supplemented in any of the given forms was able to augment the biofilms on polystyrene microtiter surface significantly (224.64%), whereas biofilm inhibition was 59.41% after DNase I treatment: confirming that eDNA facilitates biofilm formation in A. baumannii (123).
Heterogeneity and Decreased Growth Rate: The Environment Within Matters
The growth rate of organisms embedded in biofilms varies due to oxygen and nutrient depletion especially inside the microcolonies and in the deep cell layer, leading to gradients of nutrients that result in different growth rates such as fast/slow-growing cells, dormant cells, persister cells. These differences in growth rates cause population heterogeneity within the biofilms (124). The slow growth of the bacteria has been observed in mature biofilms (125). Generally, antimicrobials kill rapidly growing cells. Therefore, slow growth undoubtedly contributes to biofilm resistance (126). The phase of the cell division cycle is also known to influence antibiotic resistance within biofilms (127). Apart from heterogeneity observed within biofilms, population heterogeneity has been noted in A. baumannii which exists as opaque-virulent (VIR-O) and translucent-avirulent (AV-T) colonies. The AV-T cells produce denser biofilms than VIR-O cells, thereby showing an antibiotic resistance phenotype (128).
Development of Persisters Within Biofilm: Temporary Resistance
The capacity of microorganisms to grow and survive at higher antibiotic concentrations than their MIC is called antibiotic tolerance. In contrast to resistance, tolerance is basically a transient phase and after exposure to antibiotics for a long time, antibiotics kill the bacteria. Bacterial populations in biofilms that exhibit increased antimicrobial tolerance are called “persister cells” (126). Persister cells are actually responsible for biofilm-associated infections (126, 129). The formation of biofilms and antibiotic-tolerant persisters contributes to the heterogeneity of A. baumannii populations, facilitating their adaptation to fluctuating environments. It was proposed that environmental stress (such as desiccation) causes the death of the main stressed population within the biofilm, where few viable surviving bacteria (persister cells) can resume growth and restore the original population, once the environmental conditions are suitable. This strategy is called the “bust-and-boom” strategy and A. baumannii follows this strategy (130, 131). Multiple mechanisms trigger antibiotic tolerance and development of persisters in biofilm such as stress triggered by antibiotics, the host immune responses, high osmolarity, ROS, changes in pH, efflux pumps, quorum signaling, oxidative stress responses, desiccation, or nutrient starvation. In addition, stringent response (SR), SOS response, and toxin-antitoxin (TA) modules can be activated during persister formation. These pathways result in decreased metabolism, protein aggregation, depletion of ATP, and inhibition of translation (132, 133). In the stringent response, the production of (p)ppGpp (also known as alarmone) is activated by the (p)ppGpp synthetases such as RelA and SpoT. (p)ppGpp regulates various transcriptional and metabolic pathways, such as phosphate, lipid, and amino-acid metabolism (134). Ultimately, the SR shuts down almost all metabolic processes leading to increase tolerance to antibiotics. The formation of A. baumannii persister cells which showed tolerance to rifampicin and colistin due to the deficiency of ppGpp has also been reported (135). DNA damage leading to activation of the SOS response which is also associated with antibiotic tolerance has been noted (136). Together, SR and SOS response molecules activate the TA system in which one gene encodes toxin that shows activities against DNA, RNA, membrane, cell wall synthesis, ATP, and the other encodes antitoxin that binds and inhibits the toxin. Alkasir et al. (137) reported that up-regulation of two toxin-antitoxin systems HigB/HigA, and DUF1044/RelB resulted in high ceftazidime tolerance among A. baumannii persister cells.
Change in Bacterial Morphology due to Environmental Stress: Cause of Resistance
Stress responses result in physiological changes that control the composition and arrangement of the cellular envelope. Such changes in cell morphology can protect cells from nutrition deficiency, cold shock, heat shock, pH change, and also increase their non-susceptibility to several antimicrobial agents (138). A. baumannii embedded within biofilms also face stress due to high osmolarity that causes induction of porins such as OMP33–36 and CarO, leading to carbapenem resistance (30).
Is There an Association Between Biofilm Formation and Antimicrobial Resistance in A. baumannii?
The type of correlation that exists between antibiotic resistance phenotypes and biofilm formation among A. baumannii is controversial.
Several studies have shown that antibiotic-resistant Acinetobacter spp. form strong biofilms compared to susceptible bacteria, indicating a positive correlation between antibiotic resistance and biofilm formation (83, 92–94, 115, 139–143) (Table 2). In the above studies, a statistically significant difference was found between strains with the high biofilm-forming ability and those with low/no biofilm-forming ability with resistance to several categories of antimicrobial agents (β-lactam group, cephalosporin group, aminoglycosides, quinolones, tetracycline, oxytetracycline, aztreonam, etc.). A particular study revealed high biofilm-forming ability among MDR Acinetobacter strains isolated from ICU patients compared to Acinetobacter strains isolated from non-ICU patients which showed resistance to fewer antibiotics (144). Similarly, another study reported high biofilm production among A. baumannii isolated from burn units. These isolates showed high resistance to antibiotics including carbapenems and also showed co-production of AmpC and ESBLs (145). Bardbari et al. compared biofilm-production ability between clinical and environmental A. baumannii. Clinical strains showed strong biofilm production ability compared to environmental strains (58.7 vs. 31.2%). However, a significant correlation was observed between the MDR phenotype and biofilm formation ability in both groups (P = 0.008) (94). Few of the above-mentioned studies also showed a high prevalence of biofilm-related genes including ompA, bfmS, bap, csuE, blaPER−1, and epsA in MDR A. baumannii with a high biofilm-forming ability (83, 92, 93, 139–141).
The diversity and abundance of antibiotic resistance genes (ARGs) in biofilms of Acinetobacter spp. have been investigated by several authors to highlight the fact that the probability of accumulation of ARGs (blaOXA−51, OXA−23, OXA−58, OXA−72, OXA−24/40-like genes, blaTEM, blaVIM, blaNDM, blaSIM, ompA, xerC, and gyrA) or IS elements (ISAba1, ISAba3) in biofilms is higher rather than in the planktonic cells (93, 94, 141).
Though most studies have shown a positive association between biofilm formation and antibiotic resistance in Acinetobacter spp., some studies have documented an inverse relationship between the biofilm formation capacity of clinical strains of A. baumannii and MDR/XDR phenotype (146–152). These studies are comparatively fewer than the studies that show a positive correlation. These studies have been described in detail in Table 2.
HGT Within Biofilms of Acinetobacter spp.: Exchange Matters
Antibiotic resistance genes (ARGs) are often encoded in mobile genetic elements (MGEs) such as conjugative and non-conjugative plasmids, integrative and conjugative elements, transposons, and bacteriophages (153–156). Plasmids and MGEs can easily be transferred to closely related or distantly related bacteria via HGT. HGT also occurs in biofilms and certain factors that enhance the HGT within biofilms are the EPS matrices of biofilm that limit bacterial motility, increased cell-to-cell contact, quorum sensing, high cell density that helps in bacterial interactions. Apart from these, the presence of eDNA, oxygen availability, the SOS response, extracytoplasmic stress, and biomass surface increase the efficiency of plasmid transfer (157).
During conjugation, transfer of plasmid occurs through conjugation pilus which is proteinaceous in nature and serves as a link between donor and recipient cells (155). Transfer of ARGs within a biofilm is mainly associated with conjugation because biofilm cells are attached to a matrix, located close together that provides direct cell to cell contact. Cells remain metabolically active and are embedded within the EPS matrix in which the cells are protected against the harsh environment. These factors enhance HGT within biofilms via conjugation and thereby are considered as HGT hotspots (158–161). Apart from conjugation, transformation is another important mechanism that transfers naked genes within biofilms (162). Free DNA which is released by cell lysis can serve as the donor for transformation.
All AMR genes can be transferred via HGT within the biofilm. However, there are limited studies that show the transmission of specific genes/resistance plasmids within A. baumannii biofilm via conjugation or transformation. Willium et al. provided the first evidence of the natural transformation of Acinetobacter BD413 cells in river biofilms with a mercury resistance plasmid pQM17 (163). Hendrics et al. showed effective natural transformation in biofilms of Acinetobacter spp. strain BD413. The nature of transformants changed with the change in the amount of exogenous DNA. When the amount of DNA was low, transformants formed at the biofilm attachment surface while with an increasing amount of DNA, the accumulation of transformants was observed at the bottom of the biofilm (23). Another study showed both conjugal transfer and natural transformation of plasmids from A. baumannii to E. coli HB 101 and A. baylyi, respectively (115). The spread of a highly promiscuous carbapenem hydrolyzing gene blaNDM−1 was noted from E. coli J53-blaNDM−1 transconjugant to A. baumannii biofilms via conjugation (24).
Apart from conjugation and transformation, the transfer of plasmid DNA is also mediated via the formation of nanotubes (elongated extracellular structure employed cell to cell contact and composed of proteins), outer membrane vesicles, and bacteriophages in Acinetobacter spp. (25–29) but their role in the transfer of ARGs among biofilm-embedded Acinetobacter has not been elucidated yet. HGT also occurs in the microbial ecosystem in the human intestinal tract. HGT in the human gut microbiome can occur via different mechanisms like transduction or conjugation. Different bioinformatics tools and experimental approaches have been developed to determine the association and transfer of antibiotic resistance genes in the gut microbiome (164). The HGT in the gut microbiome may lead to the development and spread of antibiotic resistance genes among commensals and opportunistic pathogens (165).
Resistance of A. baumannii Biofilm-Associated Cells Toward Disinfectants
Disinfectants are chemical agents used to play a key role in the prevention of nosocomial transmission of infectious pathogens (166). The commonly used disinfectants against infectious pathogens in the hospital or industrial environments are 70% ethanol, chlorhexidine, sodium hypochlorite, quaternary ammonium compounds, benzalkonium chloride (BZK), benzethonium chloride (BZT), phenolic disinfectants, hydrogen peroxide, and silver ions (167). Several factors lead to the enhanced resistance toward disinfectants in A. baumannii, e.g., reduced diffusion or reaction limitations of disinfectants in biofilms, overexpression of the EPS matrix, biofilm-specific efflux pumps, phenotypic adaptations of biofilm cells to sublethal concentrations of disinfectants, alterations in genotypic features like gene transfers and mutations due to stress responses, and specific microenvironment conditions that inactivate biocides (168–170). A study has reported that resistance to antiseptics and disinfectants in A. baumannii is largely mediated by efflux proteins encoded by qac genes. The qacA/B genes encode proteins of the MFS-family whereas qacC, qacE, and qacF encode efflux proteins of the SMR-family which are located on mobile elements, thus facilitating their spread and resistance to disinfectants (171). Indeed, inappropriate use of disinfectant solutions with an adequate concentration leads to the selection and emergence of microorganisms resistant to disinfectant in the hospital setting (172).
A. baumannii Sneaks Into Hospitals: Adult and Paediatric Patients
Modern medicine and improved healthcare systems save numerous lives. However, these medical interventions have also provided conditions for microbial growth, entry into human bodies, and infections. Several indwelling devices in the hospital create breaches in the body's defense mechanisms allowing easy access for microorganisms to enter the body. The patients in ICUs already have underlying conditions and are vulnerable to infections; indwelling devices offer more opportunities for such infections. A. baumannii can survive for long periods in the hospital environment, particularly on inanimate surfaces, which may act as a reservoir for cross-colonization and infection outbreaks. Moreover, a previous study showed that A. baumannii can retain its virulence under stress (desiccation and/or starvation in hospital settings) which could facilitate infections (22). In healthcare setups, biofilms pose a serious problem due to the increased antimicrobial tolerance and the potential of biofilm-associated organisms to cause infections in patients with indwelling medical devices (173). A. baumannii easily acquires resistance and the biofilm formation rate in A. baumannii is higher than other species, making this organism a major cause of concern in ICUs. In most cases, indwelling medical device-related infections including bloodstream infections and urinary tract infections are biofilm-associated infections (173). Central venous catheters (CVCs) are the most common medical devices, followed by endotracheal tubes (ETT), ventilators, medical implants that pose a risk of device-related infection. Several in vitro and in vivo studies reported biofilm-formation of A. baumannii on several abiotic surfaces including hospital equipment and indwelling medical devices, such as catheters, endotracheal tubes etc (174–176). Carbapenem-resistant A. baumannii were also found to form biofilm on extracorporeal membrane oxygenation catheters (177). Several reports have also indicated the presence of A. baumannii biofilm on different hospital material, such as latex, anodized aluminum, stainless steel, and polycarbonate surfaces (86, 110, 174). Development of biofilm on medical devices depends on several factors including adherence of microorganisms for prolonged periods of time that results in irreversible attachment of organisms, physicochemical characteristics of the surface, cell density and types of the adherent cells, nutrient composition of the medium, flow rate of liquid through the device, drug concentration, ambient temperature and most importantly hydrophilicity and surface charge of the material of the medical devices (178). The surface proteins of microorganisms that act as virulence factors can specifically bind to host extracellular matrix proteins, such as fibrinogen, fibronectin, and collagen by van der Waals forces and H-bonds (179). These proteins have a high affinity for implants and become easily attached to the implant surface and develop as microcolonies over the entire surface of the host (179). The infections associated with colonization of A. baumannii on medical devices are discussed below. Each important device-related infection is dealt with separately and both adult and pediatric studies have been categorized (178).
Ventilator-Associated Pneumonia (VAP)
Ventilators are devices that support breathing in seriously ill patients by forcing oxygen into the lungs. Some ventilators have tubes that are inserted into the bronchus bypassing an important defense of the lungs, the ciliated cells, introducing microbes easily into the lungs. This entry is facilitated in organisms that form biofilms. Biofilm formation in the oropharynx, tracheostomy, and endotracheal tubes of ventilated patients has been suggested to play a role in the development of ventilator-associated pneumonia (VAP) (180). Sometimes, microorganisms may directly reach the lower airways by inhalation as a result of contamination of medical equipment, and they may reach the lungs. These tubes are indwelling prostheses and are typically made from polyvinyl chloride, latex rubber, or silicone materials, providing a potential surface for the growth of bacteria, especially A. baumannii. A very recent study detected A. baumnnii as one of the most common organisms capable of forming a biofilm on tracheostomy tubes among critically ill patients (181). A large surveillance study from the United States showed the association of A. baumannii with ~ 5 and 10% of ICU-acquired pneumonia (182). Malacarne et al. (183) reported 28.6% of the cases of late-onset VAP due to A. baumannii which were preceded by tracheobronchial colonization with A. baumannii. The formation of biofilm on ETT and its association with VAP was analyzed in several studies where the most frequent bacteria were A. baumannii which lead to high mortality of patients (175, 184–189).
Ventilator-associated pneumonia (VAP) is the second most frequent cause of nosocomial infection in children in ICUs in developing countries (190). Children who develop VAP also have an increased risk of mortality and morbidity (191, 192). A. baumannii is increasingly recognized as an important pathogen causing VAP in neonatal and children ICUs with a trend of high resistance to broad-spectrum antibiotics including carbapenems and colistin (176, 193–196). These studies clearly indicate the association of A. baumannii and VAP.
Bloodstream Infections (BSIs)
Bloodstream infections (BSIs) are primarily associated with the presence of the CVC or as a consequence of extensive hospital-acquired pneumonia (197). Catheters introduce microorganisms into the body as they are directly inserted in the vein to inject antibiotics or other medicines in hospitalized patients. The CVC-related infections are dependent on the patients' age and insertion procedure of CVC. The degree of severity of catheter-related bloodstream infections (CRBSI) is increased when microorganisms form biofilms. The best way to avoid CRBSI is to reduce the unnecessary catheterization, reduce the indwelling duration of CVC, use antibiotic-impregnated (like minocycline/rifampin) catheters, and use preventive locks (197). CRBSI among ICU patients due to A. baumannii biofilm formation on CVC has been noted (198, 199). Recent studies also showed BSI with highly virulent A. baumannii ST2 and ST191 belonging to International Clonal Lineage II that showed strong biofilm-forming ability (200, 201).
CVCs are also used in modern pediatric medication for various purposes including hemodynamic monitoring, infusion of vasoactive medication, hemodialysis, long-term use for chemotherapy, antibiotic treatment, or immunological diseases. CRBSI and central line-associated bloodstream infection (CLABSI) are also reported among children (202–204).
Urinary Tract Infection (UTI)
Among adults, urinary tract infection (UTI) is mostly associated with urinary catheters. Catheter-associated UTIs (CAUTIs) represent about 40% of all nosocomial infections as most of the hospitalized patients need an indwelling urinary catheter throughout their hospital stay (205). The placement of the catheter leaves the sphincter open allowing unbridled access to pathogens present in the hospital environment, the washing action of the urine is also absent in catheter-fitted patients. The catheter also presents a perfect surface for micro-organisms to adhere to and start biofilm formation (206). Given these multiple factors, UTIs are common. Biofilm formation depends on the duration of catheterization as 10–50% of short-term catheterized patients (≤7 days) experience biofilm formation meanwhile biofilm is formed inevitably in all long-term catheterized patients (>28 days) (173, 178). A. baumannii-associated UTI causes serious medical problems because of treatment difficulties due to their resistance to carbapenems and third-generation cephalosporins. Various studies showed Acinetobacter as one of the biofilm-producing organisms associated with CAUTI (5, 207–212).
Urinary tract infections (UTIs) are common infections among children in the first 2 years of life and are considered a common disease in school and pre-school children. Most A. baumannii strains are capable of producing biofilm in percutaneous nephrostomy tubes or urinary catheters, therefore A. baumannii may contribute significantly to UTIs in hospital admitted children and also cause community-acquired UTIs (213, 214).
Traumatic Battlefield, Wound, Burn, Skin, and Soft Tissue Infections
Biofilm formation on medical devices draw significant attention in healthcare settings, but the biofilm formation ability of A. baumannii on biotic surfaces such as on wound, burn, skin and soft tissue have also been noted. Such tissue-related infections in immunocompromised, cancer, and diabetic patients have raised serious concerns (215). Bacterial colonization in open wounds damages the healing process. It has been reported that biofilms are causative factors for many chronic non-healing ulcers (216). Nosocomial A. baumannii deep wound and burn wound infections have been reported in natural or man-made disasters (earthquakes, bombing, military operations) (217–219). Evidence of A. baumannii biofilm in wounds has also been provided by several other studies (220–224). Skin and Soft Tissue Infections (SSTIs) are often accompanied by A. baumannii bacteremia (225–228). The spectrum of infection can extend from cellulitis to necrotizing fasciitis.
Orthopedic Implant-Related Infections
Orthopedic devices are commonly used worldwide for a wide number of procedures including hip or knee replacements, fracture treatment, joint, ligament, and tendon replacements, and other surgical processes. These procedures have become extremely common to restore the function of affected joints, bones, or limbs. Implant-associated infections remain a major problem in orthopedic procedures and is caused by surface-adhering bacteria that form biofilm. The reported rate of implant-related infection is about 5% (229). Implant-associated infection can either be early (within the first 2 months of surgery) or delayed (between the third and the 24th month). Among different microorganisms that cause implant-associated infections, A. baumannii has been diagnosed in the case of periprosthetic joint infections (230, 231).
Neonatal Intensive Care Units
Acinetobacter baumannii has been a major cause of neonatal sepsis and several studies have reported outbreaks in neonatal units (232–234). Neonatal sepsis with drug-resistant and even carbapenem-resistant A. baumanii has also been reported (235, 236). Sepsis in neonates can also lead to meningitis causing high mortality rates. Several neonatal meningitis cases have also been reported due to MDR A. baumannii (237–241).
As with adult ICUs, neonatal ICUs also provide the same set of conditions and devices for A. baumannii to flourish. Most neonates in the ICUs are premature or of low birth weight and require prolonged hospitalization. As they are vulnerable and already fighting for life, they also require life-support systems such as ventilators. Prolonged stay at the hospital on life-support systems always increases the chances of infection particularly in neonates who are on antibiotics and have an immature immune system (242). Apart from infection, colonization of the gut with A. baumannii has also been reported in hospitalized neonates, increasing the possibility of subsequent sepsis due to translocation of the gut bacteria (243). A comparison of bacterial etiology of neonatal sepsis reveals that higher the level of care (Level III against Level II/I) greater the rate of A. baumannii sepsis. A recent study noted A. baumannii as the predominant cause of neonatal sepsis; this study was carried out in 3 tertiary care hospitals in New Delhi, all with level III care (244). Level III units are equipped with devices, which facilitate the formation of biofilms clearly indicating the link. Sources of infection could be varied and unexpected, the devices, the hospital staff, or even mothers who handle the neonate for breastfeeding or kangaroo care (193, 194, 245). Vigilance and infection control are of utmost importance particularly in units that care for neonates.
Strategies of Preventing A. baumannii Biofilms: Fighting Back
With the aim to limit Acinetobacter adhesion to biotic or abiotic surfaces and to inhibit biofilm growth, numerous effective novel anti-biofilm remedies have been developed, few of which have been discussed below (Figure 5).
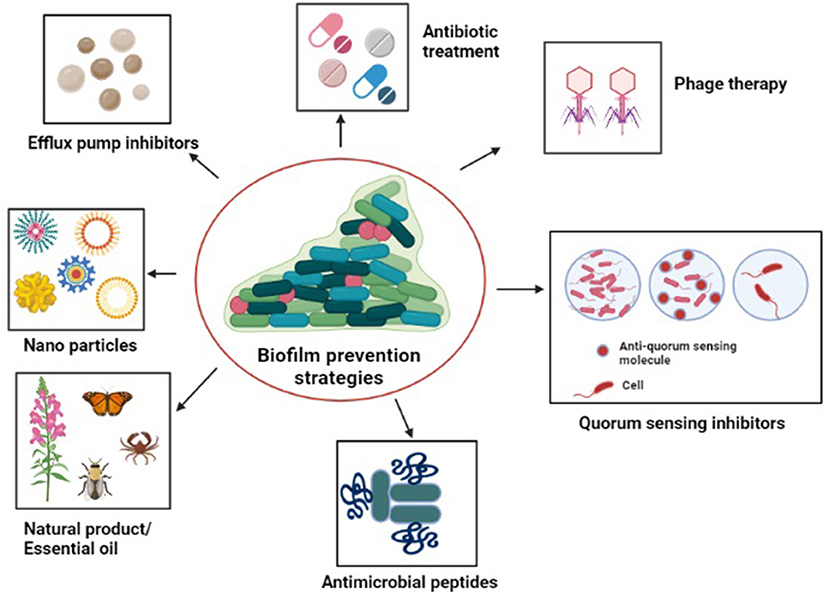
Figure 5. Diagrammatic representation of the strategies to tackle antibiotic-resistant biofilm communities: antibiotic treatment, quorum sensing inhibitors, natural products/essential oils, antimicrobials peptides, efflux pump inhibitors, nanoparticles, and phage therapy.
Antibiotic Treatment: Active Combinations
Due to the high antibiotic tolerance of biofilm cells and restricted penetration of antibiotics through the matrix, it is difficult to treat biofilm-associated infections. Few antibiotics including quinolones, rifampicin, tetracycline, and macrolides show higher penetration ability. Monotherapy is generally inappropriate because of the high antibiotic tolerance of biofilm-embedded cells. With no new antibiotics in the pipeline, antibiotic combinations are the most logical option for the treatment of biofilm infections (246). Combination therapies such as imipenem-rifampicin, colistin-rifampicin, imipenem-colistin-rifampicin, meropenem-sulbactam, and tigecycline-sulbactam have shown significant inhibition of A. baumannii biofilms (247–249). Rifampicin generates hydroxyl radicals, which are highly reactive oxygen forms and the major components of bactericidal agents. A particular study investigated the biofilm inhibitory effect of five biofilm inhibitors (zinc lactate, stannous fluoride, furanone, azithromycin, and rifampicin) either alone or in combination with one of the four antibiotics (imipenem, meropenem, tigecycline, and polymyxin B). These biofilm inhibitors in combination with antibiotics showed different degrees of in vitro synergistic and additive antibacterial effects against XDR A. baumannii under sub-minimal inhibitory concentrations (250). Different studies showed that antimicrobial combinations such as colistin-levofloxacin, colistin-tigecycline- and tigecycline-levofloxacin or these combinations with clarithromycin were used as catheter lock solutions, therefore, effective in the treatment of A. baumannii catheter-related infections (251). Use of minocycline-rifampicin impregnated bladder catheters, gentamicin-releasing urethral catheters, and norfloxacin along with blends of a copolymer of ethylene-vinyl acetate and polyethylene oxide as catheter coatings showed a reduction of CAUTIs (252–254). Clinical trials also showed that the use of medicated CVCs that contain antimicrobial solutions (minocycline-rifampicin, chlorhexidine/silver sulphadiazine, rifampicin-miconazole) on both the external and the luminal surfaces of catheters, exhibited significant inhibition of bacterial attachment but only the minocycline-rifampicin impregnated CVCs were able to reduce CRBSIs (255–257). TRL1068 (a human monoclonal antibody active against an epitope of DNABII proteins that stabilizes biofilm eDNA in both GPB and GNB) in combination with imipenem showed a significant reduction of A. baumannii adherence to catheters (258).
Quorum Sensing Quenchers: Stopping the Communication
Since QS contributes to biofilm production, inhibition of QS signaling pathways result in a reduction of biofilm formation and can be used as a novel therapeutic strategy (259). Biofilm formation in A. baumannii is dependent on the activation of a LuxI/LuxR-type QS network involving AbaI synthase, AbaR receptor, and various AHLs. QS inhibition could be achieved by targeting the synthesis of AHLs. For example, mutation of the AHL synthase AbaI affects the surface-associated motility and robust biofilm formation in A. baumannii ATCC®17978 (104). A low concentration of triclosan (chemical with antibacterial properties) could inhibit the enoyl-acyl carrier protein reductase (FabI), which is important for AHL acyl chain formation (260). The use of natural compounds such as allin, patulin/clavacin, and vanillin were found to interfere with AHL receptors, leading to the prevention of QS signals (261–263). Moreover, AHL analogs, AbaR antagonists (such as streptomycin), anoR antagonist (virstain), and antagonists for di-guanylate cyclase enzyme that synthesize cyclic di-GMP were found to inhibit QS and subsequently biofilm formation in A. baumannii and A. noscomialis (264–268). A marine steroid (Siphonocholin) which has anti-QS activity was found to inhibit the EPS production, swarming motility, and biofilm formation in A. baumannii (269). Certain genetically engineered quorum quenching enzymes such as AHL lactonase and MomL could also successfully inhibit QS signal, leading to disruption of biofilm structure (270–272).
Natural Products/Essential Oils: Nature to the Rescue
Natural products such as microbial, plant, and animal derivatives can effectively reduce A. baumannii infections. Metabolites produced by bacteria have been proved to be effective against A. baumannii biofilm (273). A study showed that A. calcoaceticus could be strongly inhibited by other bacterial (Burkholderia cepacia, Methylo bacterium spp., Mycobacterium mucogenicum, Sphingomonas capsulata, and Staphylococcus spp.) crude cell-free supernatants (274). Maipomycin A which is isolated from the metabolites of the marine actinomycete and acts as an iron chelator inhibits A. baumannii biofilm formation on medical materials including silicone catheters and endotracheal tubes (polyvinyl chloride) (275). Several sets of compounds derived from marine sponges showed inhibition of different bacterial biofilm including A. baumannii specifically through non-microbicidal mechanisms (276). 5-episinuleptolide isolated from Sinularia leptoclados (a genus of soft coral in the family Alcyoniidae), showed anti-biofilm activity against A. baumannii ATCC®19606TM and MDR A. baumannii strains by decreasing the expression of the pgaABCD locus, which encodes the extracellular polysaccharide (poly-PNAG) of the biofilm structure (277). A secreted serine protease, termed “PKF,” was identified in A. baumannii that suppressed A. baumannii biofilm formation (278). Myrtenol which is a bicyclic monoterpene isolated from various plants showed strong anti-biofilm properties against clinical strains of A. baumannii at a concentration of 200 μg/ml. Myrtenol specifically inhibited the mature biofilm, reduced the biofilm thickness, inhibited the biofilm-associated virulence factors, and improved the susceptibility of strains toward conventional antibiotics. Upon myrtenol treatment, suppression of the biofilm-associated genes such as bfmR, csuA/B, bap, ompA, pgaA, pgaC, and katE was found (279). Natural products such as essential oils (EO), plant secondary metabolites could exert broad-spectrum antibacterial activity by disrupting bacterial membrane integrity and also by inhibiting ATP synthesis that result in leakage of metabolites/ions (280). Different EOs with MBIC between 0.3–1.25 μl/ml derived from flowery plants such as Mentha pulegieum L. and Ziziphora tenuior L., could damage the A. baumannii biofilm structure to a great extent. D-isomenthone, pulegone, isopulegone, menthol, and piperitenone were found to be the major components of the plant extract (281, 282). Anti-biofilm properties of four essential oil components (carvacrol, eugenol, thymol, and vanillin) were assessed against the organisms adhering to food surfaces in the meat industry. Carvacrol and thymol showed the most remarkable antimicrobial effect against A. baumannii strains (283). Similarly, other studies also showed that different EOs derived from plants and rich with these essential oil components (Oregano oil rich in carvacrol and thymol contents; cinnamon oil rich in eugenol; Eucalyptus camaldulens oil rich in thymol content) were active against A. baumannii wound infections (284, 285). EO-based nanoemulsions, prepared from Thymus daenensis (plant), showed potent antibiofilm activity against A. baumannii on sublethal dose (56.43% inhibition in 1/2 MIC concentration) after 24 h of incubation (286). Shivaprasad et al. (287) reported the activity of different antibiotics such as imipenem, cefipime, cotrimoxazole, amikacin, ciprofloxacin, piperacillin/tazobactum, cefoperazone, and gentamicin was enhanced against MDR/XDR A. baumannii complex when used in combination with lemongrass EO which showed 65–79% of anti-biofilm activity at a dilution between 0.625–0.156 μl/ml. Most of the essential oils have been tested for in vitro activity against bacteria, only few were subsequently tested in cellular or animal models. Ismail et al. (288) reported that anti-biofilm activity of Pimenta dioica leaf EOs (85% inhibit) was higher than Pimenta racemosa leaf EOs (34%) against A. baumannii and both P. diocia and P. racemosa leaf EOs showed a bactericidal action against A. baumannii within 6 h at 2.08 μg·ml−1. In addition, a significant reduction of A. baumannii microbial load in the mice wound infection model was also found (288). Similarly, oregano oil showed significant antibacterial activity against 11 MDR clinical isolates including A. baumannii with a MIC ranging from 0.08 mg/ml to 0.64 mg/ml. An in vivo study demonstrated that oregano oil topically applied 24 h after bacterial inoculation, sufficiently reduced the bacterial load in the wounds by 3 log10 in 1 h (289).
Antimicrobials Peptides: An Alternative to Antibiotics
Antimicrobial peptides (AMPs) are cationic peptides (15–30 amino acids long) produced by the innate immune response and target the negatively charged cell membrane of bacteria (290). Different AMPs of biological origins have been reported to inhibit A. baumannii biofilm. Human AMP LL37 is one such AMP that inhibits A. baumannii biofilm (291). Human milk lactoferrin which is an iron-chelating AMP also showed slightly more potent antibacterial activity than bovine milk lactoferrin against A. baumannii biofilm (292). Derivative (D-RR4) of a small synthetic peptide, RR (12 amino acids) exhibited potent antibacterial and anti-biofilm activity against P. aeruginosa and A. baumannii in macrophage cells as well as in a Caenorhabditis elegans model (293). Magainin 2 (an antimicrobial peptide consisting of 23 amino acids isolated from the skin of the African clawed frog Xenopus laevis), showed the ability to inhibit A. baumannii biofilm at a very low concentration (294). Potent activity against A. baumannii biofilm has also been observed with several AMPs derived from flies, such as cecropin AMP identified from Musca domestica and another complex of AMPs (Fly larvae immune peptides) from Calliphora vicina (295, 296). Jakiewicz et al. (297) investigated the antimicrobial activity of eight peptides (aurein 1.2, CAMEL, citropin 1.1., LL-37, omiganan, r-omiganan, pexiganan, and temporin A) of different biological origins against A. baumannii biofilm on tracheal tube fragments. Among these peptides, strong anti-biofilm activity was shown by CAMEL and pexiganan with MIC values of 2 μg/ml (297). More recently, four synthetically produced chimeric AMPs have been proven to show anti-biofilm activities against MDR A. baumannii. These AMPs worked synergistically with ciprofloxacin, cefotaxime, or erythromycin to inhibit A. baumannii biofilm (298). The activity of the cationic antimicrobial peptide WLBU2 (24-residue engineered cationic amphipathic peptide) showed promising activity in combination with imipenem and tobramycin against planktonic cells and biofilm of MDR A. baumannii (299). A synthetically produced cyclic peptide ZY4 (17 amino acid long) exhibited biofilm eradication activity by killing the persister cells of A. baumannii and P. aeruginosa within the biofilm. Moreover, the therapeutic potential of ZY4 was also determined in an A. baumannii-induced bacteremia mice model (300). Some AMPs are used as ointments for medical device surfaces or for the reduction of superficial tissue infections (301). In an in vitro experiment, Temporin-L showed an anti-biofilm effect without cell damage, representing the great potential for clinical application (302). AMPs have great potential in clinical treatment by effective removal of biofilms.
Efflux Pump Inhibitors: Blocking an Important Machinery
Studies had shown that efflux pumps play various roles in biofilm formation in ESKAPE pathogens; hence, inhibiting their function by efflux pump inhibitors (EPI) could also inhibit biofilm formation. A compound can be considered as a potent EPI when it has a broad substrate specificity and low off-target toxicity (303). One of the most common EPIs that is usually used in A. baumannii is phenylalanine-arginine β-naphthylamide (PAβN) which was reported to inhibit A. baumannii biofilm formation (304). Two novel serum-associated EPIs known as ABEPI1 and ABEPI2 were identified that potentiated the activities of antibiotics against A. baumannii grown in human serum. Both compounds exhibited similar antibiotic potentiation profiles toward minocycline and ciprofloxacin and inhibited pump activity (305). Another study also generated a set of compounds (pharmacophores) consisting of 2-substituted benzothiazoles which showed significant inhibition of AdeABC efflux pumps in combination with ciprofloxacin (306). Krishnamoorthy et al. assessed the efficacy of two microbicides such as cetrimide and chlorhexidine which adversely modified the expression and function of AdeABC efflux pump in biofilm-associated A. baumannii. Furthermore, they established that these microbicides decreased the negative charges on A. baumannii cell membranes, causing dysregulation of the efflux pump, leading to cell death (307).
Nanoparticles: Small Is Beautiful
Nanoparticles (NPs) are very small in size (<100 nm) with a large surface area and extremely reactive nature. They show broad-spectrum activities against both GPB and GNB and sometimes they have been preferred over antibacterial agents. NP can disrupt biofilm integrity by penetrating bacterial cell membrane, generating ROS, causing ATP depletion, and interacting with polysaccharides, eDNA, proteins, and lipids (308). Different studies have been carried out to understand the role of NPs to inhibit A. baumannii biofilm. A study showed the use of nitric oxide (NO) releasing NPs to treat A. baumannii biofilm-related wound infections in vivo in murine models (309). Another study showed the disruption of A. baumannii biofilm on exposure to nanoemulsion of cetylpyridinium chloride, a quaternary ammonium salt (310). NPs coupled with metals or natural product extracts have been shown to possess inhibitory activity against both planktonic and biofilm-associated cells. A particular study showed the action of silver NPs, gold NPs and silver-gold bimetallic NPs against A. baumannii biofilms. These NPs showed 88% of A. baumannii biofilm inhibition (311). Several other studies also showed the efficiency of silver NPs (AgNPs) in inhibition of Acinetobacter biofilms as these NPs easily penetrate the thick EPS in biofilms. Positively charged AgNPs interact with negatively charged eDNA that plays a major role in the inhibition of biofilms (312, 313). In addition, A. baumannii biofilm inhibition was also observed by selinium NPs, curcumin NPs, aluminum oxide NPs, etc. (314–316). NPs in combination with antibiotics have also been reported to possess substantial antibiofilm activity. AgNPs were found to act synergistically with imipenem, as imipenem lyses the cell wall of bacteria leading to increased penetration of AgNPs into the cells (317). NPs can also be used as drug delivery carriers or as catalysts to promote the penetration of drugs into biofilms; improve the solubility, stability, and biocompatibility of drugs (318).
Phage Therapy: Cocktails That Work
The use of bacteriophages is another approach to control and remove biofilms. Different lytic bacteriophages such as AB7-IBB2 (family of Podoviridae), AB7-IBB1 (Siphoviridae), and vB_AbaMIME-AB2 were found to inhibit A. baumannii biofilms (108 CFU/well) on abiotic and/or biotic surfaces (60–>80%) (319–321). Lood et al. identified 21 distinct lysins (prophages) induced from 13 diverse A. baumannii. Among these lysins, PlyF307 showed the greatest activity, and treatment with PlyF307 was able to significantly reduce planktonic and A. baumannii biofilm both in vitro and in vivo (322). Thandar et al. (323) showed that the C-terminal amino acids (15, 108–115, 117–138) of a phage lysin named P307, alone could efficiently kill A. baumannii (>3 logs) while its engineered derivative (P307SQ-8C) showed improved activity (>5-log kill) along with polymyxin B. Two different phages (B_AbaM_ISTD, and vB_AbaM_NOVI), isolated from Belgrade wastewaters, were found to inhibit A. baumannii biofilms (324). A. baumannii biofilm biomass was inhibited when an environmental phage cocktail was used in combination with antibiotics such as ciprofloxacin, trimethoprim/sulfamethoxazole, gentamicin, tobramycin, imipenem, and meropenem which are generally used in the treatment of UTI. While phage cocktail combined with levofloxacin and amikacin, did not act synergistically (325). An excellent method of inhibiting A. baumannii biofilm was devised by Ran et al. (326) by combining photodynamic bacteriophages (ABP) and Nile blue photosensitizers (NB). NB photosensitizer possessing sulfur atom displayed ROS production ability. Both in vitro and in vivo experiments proved that NB-phage bioconjugate (APNB) could bind to the main components of biofilms and reduce drug resistance caused by biofilms (326).
Other Biofilm Inhibitors
Some other chemicals or compounds that do not belong specifically to the above-mentioned groups have also been reported to inhibit A. baumannii biofilms. In order to determine the effectiveness of biocides for the eradication of MDR A. baumanni biofilms, hydrogen peroxide, and hydrogen peroxide-based formulations were tested. Mixed-culture biofilm cells were found to be more resistant to some biocides, such as hydrogen peroxide and sulfathiazole, than the single-species Acinetobacter biofilm cells. Higher potential for biofilm removal and killing was found among oxidizing biocides, such as sodium hypochlorite and hydrogen peroxide, compared to non-oxidizing biocides (sulfathiazole and glutaraldehyde) (327, 328). Reverse-amide class of 2-aminoimidazole compounds were also found to inhibit A. baumannii biofilms by >95% at 100 μM and had the potential to readily increase the permeation of many conventional antibiotics into the bacterial cell membrane, therefore can be used as a new “drug delivery” mechanism in a variety of systems (329). The efficacy of octenidine dihydrochloride (OH) (disinfectant) was tested to reduce A. baumannii biofilms on polystyrene, stainless steel, catheters and was found to be effective in inhibiting A. baumannii biofilms at a concentration of 5, 10, and 15 mM (330). Biocidal activity of commonly used antiseptics and disinfectants [sodiumhypochlorite, chlorhexidine, orthophthalaldehyde (OPA), peracetic acid (PA), and peracetic acid] were tested against A. baumanni. About 78% of biofilm-producing A. baumannii became susceptible to all disinfectants and antiseptics tested (331). A. baumannii growth and biofilm formation in human serum was reduced by 16 μM of gallium nitrate (hydrated nitrate salt), whereas a higher concentration (64 μM) caused huge disruption of the preformed A. baumannii biofilms (332). N2, N4-disubstituted quinazoline-2,4-diamines which are dihydrofolate reductase inhibitors exhibited anti-biofilm activity (90%) against A. baumannii when the 6-position is replaced with a halide or an alkyl substituent (333). A clinical trial showed that the use of silver alloy on catheter material reduced bacterial colonization, thereby reducing CAUTIs (334). Different clinical trials showed that in addition to silver alloy-coated latex catheters, nitrofurazone-coated silicon catheters were also found to reduce CAUTI during short-term use (<30 days) (335).
Surface Modification and Physical Therapy
Surface modification and physical therapy are the most successful treatment options for eradication or prevention of microbial adhesion on medical devices when antibiotic treatment fails to effectively eliminate medical device-related biofilm infections. An effective physical method is photodynamic therapy (PDT) for removing microbial adhesion and biofilm formation. PDT inhibits biofilm formation and also fights against biofilm infections by producing Reactive Oxygen Species (ROS) and inhibiting the production of some toxic factors that affect bacterial adhesion and biofilm matrix formation (336, 337). PDT technique is also used to combat other implant-related biofilm infections, such as prosthetic joint infections and infections caused by ventilator-associated pneumonia biofilms (338). Low-intensity ultrasound at the physiotherapy level showed effective removal of biofilm by enhancing the activity of antibacterial agents (339). Another effective physical method is the use of water jets for removing biofilms on the surface of implants through the mechanical action of pulse and pressure (340).
Advances in surface engineering have led to the development of antibacterial agents or antiadhesion agents to coat the surface of medical devices that can effectively inhibit the growth of microorganisms. The use of Sharklet™ surface made by shark skin and microscale ribs of various lengths are combined into a repeating diamond micropattern, preventing bacterial colonization and biofilm formation when incorporated into the surfaces of medical devices (341). Several antibacterial coatings, such as chlorhexidine, rifampicin, gentamicin, minocycline, silver sulfadiazine, amikacin, and vancomycin, have been widely used in clinical practice, showing the efficacy of preventing catheter-related and other implant-related infections (342). Hydrophilic polymers such as hyaluronic acid have been used to coat different medically relevant materials like polyurethane catheters and silicon shunts and have been shown to be effective in preventing biofilm formation on polystyrene surfaces. An isoeugenol coating has been shown to prevent the adhesion and formation of biofilms on stainless steel and polyethylene surfaces due to its good antibacterial activity (343). Various hydrogel coatings, heparin coatings, or bindings have been used in medical devices to reduce fibronectin deposition on vascular catheter surfaces due to high antiadhesive activity (344). In addition, inhibition of microbial growth on catheters also depends on the catheter constitutive polymers which are able to absorb large amounts of antibiotics. These polymers can be designed by introducing acidic or basic groups into the polymer side chains that are able to interact with different classes of drugs (345). Polyethylene glycol and bovine albumin could be incorporated into the polymer bulk together with antibiotic or antifungal molecules to increase and control drug release from the polymer matrices.
Discussion
The genus Acinetobacter is not easy to deal with, both for researchers and clinicians alike, starting with the identification of the multiple species to its propensity to form biofilms and acquire antibiotic resistance genes readily (13, 30). A. baumannii has been particularly difficult in ICUs and notorious global clones ST1 and ST2 have also been reported from cases of neonatal sepsis (346, 347). Of the different species “baumannii” is most frequently reported; however, other species of Acinetobacter are also being reported to cause infections (348). The role of “non-baumannii” in human infection has been perceived recently because of the technological advances that allow correct identification of the bacteria at the species level. Identification of the bacteria at the species level heavily depends on the molecular methods or MALDI-TOF. The identification of the species is particularly challenging in low middle-income countries which lack the requisite infrastructure to differentiate the several species. Furthermore, A. baumannii and non-baumannii have distinct resistance mechanisms for antimicrobial agents though phenotypically they seem to be undifferentiated. Though this review primarily focused on baumannii, non-baumannii isolates could be lurching behind and due attention should be paid to them.
Despite all the effort that mankind has made to improve and save lives, it has been seen that microbes have found new strategies every time to evade technological or medical advancements. With the additional challenge of antibiotic resistance, new approaches need to be utilized to restrict the usage of antibiotics. Some remedies (such as antibiotics, nano antimicrobial compounds, natural products, bacteriophages) that directly act on pathogens have already been discussed in this review but antimicrobial remedies that act on the host (several innate immune-enhancing peptides) to potentiate antibiotic action should be explored (349). Similarly, probiotics also confer a health benefit on the host against MDR A. baumannii when administered in adequate amounts combined with immunomodulators, such as lysophosphatidylcholine and antibiotics such as clarithromycin, colistin, tigecycline, or imipenem by stimulating the immune response (350–352). Though advances in medical science are appreciated, there is no underestimating the power of prevention. Hospital infection control is as relevant as in earlier times and can be important in reducing nosocomial infections and interrupting the transmission chain of pathogens such as Acinetobacter.
The ability of Acinetobacter to attach to biotic and abiotic surfaces is useful for the organism, particularly in hospitals. It can colonize the patients (sometimes without any symptoms), caregivers, and hospital surfaces or devices that increase the chances of infection. This realization has prompted several studies on approaches to prevent the formation of biofilms in hospital devices. The approaches are diverse ranging from antibiotic-coated devices, quorum sensing quenchers, natural products, phage cocktails and nanoparticles. However, very few of these approaches have been tested in in vivo models (288, 289) and reached the patient; most are in vitro studies, which have not been carried forward. In vivo studies address many of the shortcomings of in vitro studies and one can better evaluate the safety, toxicity, and efficacy of a drug candidate in in vivo models. Thereby, emphasis needs to be on in vivo models and further clinical trials.
Development of persister cells, antibiotic tolerance, population heterogeneity, and biofilm-related infections should be considered as significant risk factors in the course of choosing an appropriate therapy particularly in the case of A. baumannii. The possible role of the diversity of its reservoirs, its resistance to drying, its capacity to accumulate resistance genes especially plasmid-mediated resistance genes are challenges associated with this organism. Plasmids carrying carbapenem-resistant genes such as blaNDM also carry other resistant genes, which make a panel of antibiotics ineffective. The evolution of new variants of antibiotic-hydrolyzing enzymes, which have better efficiency and better stability, also has made it difficult to treat MDR strains, especially A. baumannii.
As the authors reviewed the aspect of biofilm formation alongside antibiotic resistance in Acinetobacter, it was realized that there is still a lack of clarity regarding the association. It is presumed that this controversy prevails due to the methodology used to test the association. Most studies have used clinical Acinetobacter isolates, carried out in vitro biofilm assays, and tested the antibiotic susceptibility separately. As most clinical isolates are also antibiotic-resistant (independent of their biofilm-forming capability) such studies may not be appropriate in understanding the association. The difference in the susceptibility of the isolates to antibiotics, when studied as planktonic growth as well as biofilms, will probably give a better understanding. As the entry of antibiotics is restricted within biofilms due to several mechanisms such as the presence of EPS matrix, antibiotic degradation, Acinetobacter as biofilms is resistant to the action of antibiotics but this phenomenon is irrespective of the presence of resistance determinants present.
Whole-genome sequencing has generated a vast amount of data that has enriched our understanding of pathogens. Analysis of such data can build an understanding of the epidemic clones, the ARGs or MGEs that are prevalent, their associations with particular clones, etc. The genome data can also be utilized to understand the strengths and weaknesses of antimicrobial agents. However, sequences are mere blueprints and different in vivo and in vitro laboratory assays need to be carried out along with genome sequences to understand potential therapeutic options. The focus should be on robust assays from the leads that are already identified.
Conclusion
Most GNB have an underlying similarity, yet there are several features unique to each, making some of these bacteria such as A. baumannii more challenging than others. The determinants that drive any organism to be a successful pathogen are a consequence of several diverse factors. These factors include antibiotic use, infection control practices, climate change, human behavior, deforestation, availability of resources, and several others, that can over the years determine how these pathogens evolve. Future research will increase our understanding of this pathogen. The clinicians' experience of treating patients with Acinetobacter infection can further strengthen our future approaches to treatment. Harnessing the “clinical eye” with the “scientific fervor” may help devise new strategies to deal with a pathogen that has various tools at its disposal. The use of antimicrobials in hospitals dealing with COVID-19 patients has increased. However, the attention that antibiotic resistance has been drawing for the past few years has waned off, at least temporarily. The strides that we have taken in controlling resistance need to be fostered to effectively control A. baumannii infections.
Author Contributions
SR and GC performed literature search, wrote the manuscript, and prepared all the figures and tables. SB conception, design, wrote the introduction, and discussion along with a critical revision of the manuscript. AM and SD critically revised the manuscript. All authors read and approved the final manuscript.
Funding
This study was supported by the Indian Council of Medical Research (ICMR) intramural funding. SR was supported by a fellowship (Senior Research Associateship) from the Council of Scientific and Industrial Research (CSIR). GC was supported by a fellowship (Scientist C) from Okayama University, Okayama.
Conflict of Interest
The authors declare that the research was conducted in the absence of any commercial or financial relationships that could be construed as a potential conflict of interest.
Publisher's Note
All claims expressed in this article are solely those of the authors and do not necessarily represent those of their affiliated organizations, or those of the publisher, the editors and the reviewers. Any product that may be evaluated in this article, or claim that may be made by its manufacturer, is not guaranteed or endorsed by the publisher.
Acknowledgments
Figures were created with BioRender.com.
References
1. WHO. Global Antimicrobial Resistance Surveillance System (GLASS) Report: Early Implementation 2016–2017. Geneva: World Health Organization (2017).
2. Asokan GV, Ramadhan T, Ahmed E, Sanad H. WHO global priority pathogens list: a bibliometric analysis of medline-pubmed for knowledge mobilization to infection prevention and control practices in Bahrain. Oman Med J. (2019) 34:184–93. doi: 10.5001/omj.2019.37
3. Centers for Disease Control and Prevention. Antibiotic resistant threats in the United States 2019. (2019). Available online at: https://www.cdc.gov/drugresistance/pdf/threats-report/2019-ar-threats-report-508.pdf (accessed January 15, 2020).
4. Peleg AY, Seifert H, Paterson DL. Acinetobacter baumannii: emergence of a successful pathogen. Clin Microbiol Rev. (2008) 21:538–82. doi: 10.1128/CMR.00058-07
5. Xiao D, Wang L, Zhang D, Xiang D, Liu Q, Xing X. Prognosis of patients with Acinetobacter baumannii infection in the intensive care unit: a retrospective analysis. Exp Ther Med. (2017) 13:1630–3. doi: 10.3892/etm.2017.4137
6. Weinberg SE, Villedieu A, Bagdasarian N, Karah N, Teare L, Elamin WF. Control and management of multidrug resistant Acinetobacter baumannii: a review of the evidence and proposal of novel approaches. Infect Prev Pract. (2020) 2:100077. doi: 10.1016/j.infpip.2020.100077
7. Dijkshoorn L, Nemec A, Seifert H. An increasing threat in hospitals: multidrug-resistant Acinetobacter baumannii. Nat Rev Microbiol. (2007) 5:939–51. doi: 10.1038/nrmicro1789
8. Eveillard M, Kempf M, Belmonte O, Pailhories H, Joly-Guillou ML. Reservoirs of Acinetobacter baumannii outside the hospital and potentialinvolvement in emerging human community-acquired infections. Int J Infect Dis. (2013) 17:e802–5. doi: 10.1016/j.ijid.2013.03.021
9. Howard A, O'Donoghue M, Feeney A, Sleator RD. Acinetobacter baumannii: an emerging opportunistic pathogen. Virulence. (2012) 3:243–50. doi: 10.4161/viru.19700
10. Lin MF, Lan CY. Antimicrobial resistance in Acinetobacter baumannii: from bench to bedside. World J Clin Cases. (2014) 16:787–814. doi: 10.12998/wjcc.v2.i12.787
11. Kurcik-Trajkovska B. Acinetobacter spp. - a serious enemy threatening hospitals worldwide. Maced J Med Sci. (2009) 2:157–62. doi: 10.3889/MJMS.1857-5773.2009.0043
12. Litzler PY, Benard L, Barbier-Frebourg N, Vilain S, Jouenne T, Beucher E, et al. Biofilm formation on pyrolytic carbon heart valves: influence of surface free energy, roughness, and bacterial species. J Thorac Cardiovasc Surg. (2007) 134:1025–32. doi: 10.1016/j.jtcvs.2007.06.013
13. Eze EC, Chenia HY, El Zowalaty ME. Acinetobacter baumannii biofilms: effects of physicochemical factors, virulence, antibiotic resistance determinants, gene regulation, and future antimicrobial treatments. Infect Drug Resist. (2018) 11:2277–99. doi: 10.2147/IDR.S169894
14. Youn Sung J. Molecular characterization and antimicrobial susceptibility of biofilm-forming Acinetobacter baumannii clinical isolates from Daejeon, Korea. Korean J Clin Lab Sci. (2018) 50:100–9. doi: 10.15324/kjcls.2018.50.2.100
15. Martí S, Rodríguez-Baño J, Catel-Ferreira M, Jouenne T, Vila J, Seifert H, et al. Biofilm formation at the solid-liquid and air-liquid interfaces by Acinetobacter species. BMC Res Notes. (2011) 4:5. doi: 10.1186/1756-0500-4-5
16. Zeighami H, Valadkhani F, Shapouri R, Samadi E, Haghi F. Virulence characteristics of multidrug resistant biofilm forming Acinetobacter baumannii isolated from intensive care unit patients. BMC Infect Dis. (2019) 19:629. doi: 10.1186/s12879-019-4272-0
17. Giles SK, Stroeher UH, Eijkelkamp BA, Brown MH. Identification of genes essential for pellicle formation in Acinetobacter baumannii. BMC Microbiol. (2015) 15:116. doi: 10.1186/s12866-015-0440-6
18. Pompilio A, Scribano D, Sarshar M, Di Bonaventura G, Palamara AT, Ambrosi C. Gram-negative bacteria holding together in a biofilm: the Acinetobacter baumannii way. Microorganisms. (2021) 9:1353. doi: 10.3390/microorganisms9071353
19. Jacobs AC, Blanchard CE, Catherman SC, Dunman PM, Murata Y. An ribonuclease T2 family protein modulates Acinetobacter baumannii abiotic surface colonization. PLoS ONE. (2014) 9:e85729. doi: 10.1371/journal.pone.0085729
20. Dallo SF, Weitao T. Insights into acinetobacter war-wound infections, biofilms, and control. Adv Skin Wound Care. (2010) 23:169–74. doi: 10.1097/01.ASW.0000363527.08501.a3
21. Gedefie A, Demsis W, Ashagrie M, Kassa Y, Tesfaye M, Tilahun M, et al. Acinetobacter baumannii biofilm formation and its role in disease pathogenesis: a review. Infect Drug Resist. (2021) 14:3711–9. doi: 10.2147/IDR.S332051
22. Chapartegui-González I, Lázaro-Díez M, Bravo Z, Navas J, Icardo JM, Ramos-Vivas J. Acinetobacter baumannii maintains its virulence after long-time starvation. PLoS ONE. (2018) 13:e0201961. doi: 10.1371/journal.pone.0201961
23. Hendrickx L, Hausner M, Wuertz S. Natural genetic transformation in monoculture Acinetobacter sp. strain BD413 biofilms. Appl Environ Microbiol. (2003) 69:1721–7. doi: 10.1128/AEM.69.3.1721-1727.2003
24. Tanner WD, Atkinson RM, Goel RK, Toleman MA, Benson LS, Porucznik CA, et al. Horizontal transfer of the blaNDM-1 gene to Pseudomonas aeruginosa and Acinetobacter baumannii in biofilms. FEMS Microbiol Lett. (2017) 364:fnx048. doi: 10.1093/femsle/fnx048
25. Pande S, Shitut S, Freund L, Westermann M, Bertels F, Colesie C, et al. Metabolic cross-feeding via intercellular nanotubes among bacteria. Nat Commun. (2015) 6:6238. doi: 10.1038/ncomms7238
26. Krahn T, Wibberg D, Maus I, Winkler A, Bontron S, Sczyrba A, et al. Intraspecies transfer of the chromosomal Acinetobacter baumannii blaNDM-1 carbapenemase gene. Antimicrob Agents Chemother. (2016) 60:3032–40. doi: 10.1128/AAC.00124-16
27. Wachino JI, Jin W, Kimura K, Arakawa Y. Intercellular transfer of chromosomal antimicrobial resistance genes between Acinetobacter baumannii strains mediated by prophages. Antimicrob Agents Chemother. (2019) 63:e00334–19. doi: 10.1128/AAC.00334-19
28. Rumbo C, Fernández-Moreira E, Merino M, Poza M, Mendez JA, Soares NC, et al. Horizontal transfer of the OXA-24 carbapenemase gene via outer membrane vesicles: a new mechanism of dissemination of carbapenem resistance genes in Acinetobacter baumannii. Antimicrob Agents Chemother. (2011) 55:3084–90. doi: 10.1128/AAC.00929-10
29. Chatterjee S, Mondal A, Mitra S, Basu S. Acinetobacter baumannii transfers the blaNDM-1 gene via outer membrane vesicles. J Antimicrob Chemother. (2017) 72:2201–7. doi: 10.1093/jac/dkx131
30. Kyriakidis I, Vasileiou E, Pana ZD, Tragiannidis A. Acinetobacter baumannii antibiotic resistance mechanisms. Pathogens. (2021) 10:373. doi: 10.3390/pathogens10030373
31. Qi L, Li H, Zhang C, Liang B, Li J, Wang L, et al. Relationship between antibiotic resistance, biofilm formation, and biofilm-specific resistance in Acinetobacter baumannii. Front Microbiol. (2016) 7:483. doi: 10.3389/fmicb.2016.00483
32. Colquhoun JM, Rather PN. Insights into mechanisms of biofilm formation in Acinetobacter baumannii and implications for uropathogenesis. Front Cell Infect Microbiol. (2020) 10:253. doi: 10.3389/fcimb.2020.00253
33. Dijkshoorn L. The diversity of the genus Acinetobacter. In: Gerischer U, editor. Acinetobacter Molecular Biology. CaisterAcademic Press (2008).
34. Munoz-Price LS, Weinstein RA. Acinetobacter infection. N Engl J Med. (2008) 358:1271–81. doi: 10.1056/NEJMra070741
35. Brisou J, Prevot A. Studies on bacterial taxonomy. X. The revision of species under Acromobacter group. Ann Inst Pasteur. (1954) 86:722–8.
36. Chuang YC, Sheng WH, Li SY, Lin YC, Wang JT, Chen YC, et al. Influence of genospecies of Acinetobacter baumannii complex on clinical outcomes of patients with acinetobacter bacteremia. Clin Infect Dis. (2011) 52:352–60. doi: 10.1093/cid/ciq154
37. Bergogne-Bérézin E, Towner KJ. Acinetobacter spp. as nosocomial pathogens: microbiological, clinical, and epidemiological features. Clin Microbiol Rev. (1996) 9:148–65. doi: 10.1128/CMR.9.2.148
38. Morohoshi T, Saito T. beta-Lactamase and beta-lactam antibiotics resistance in acinetobacter anitratum (syn: A. calcoaceticus). J Antibiot. (1977) 30:969–73. doi: 10.7164/antibiotics.30.969
39. Paton R, Miles RS, Hood J, Amyes SG, Miles RS, Amyes SG. ARI 1: beta-lactamase-mediated imipenem resistance in Acinetobacter baumannii. Int J Antimicrob Agents. (1993) 2:81–7. doi: 10.1016/0924-8579(93)90045-7
40. Higgins PG, Dammhayn C, Hackel M, Seifert H. Global spread of carbapenem-resistant Acinetobacter baumannii. J Antimicrob Chemother. (2010) 65:233–8. doi: 10.1093/jac/dkp428
41. Hamidian M, Nigro SJ. Emergence, molecular mechanisms and global spread of carbapenem-resistant Acinetobacter baumannii. Microb Genom. (2019) 5:e000306. doi: 10.1099/mgen.0.000306
42. Hejnar P, Kolár M, Hájek V. Characteristics of Acinetobacter strains (phenotype classification, antibiotic susceptibility and production ofbeta-lactamases) isolated from haemocultures from patients at theTeaching Hospital in Olomouc. Acta Univ Palacki Olomuc Fac Med. (1999) 142:73–77.
43. Cai Y, Chai D, Wang R, Liang B, Bai N. Colistin resistance of Acinetobacter baumannii: clinical reports, mechanisms and antimicrobial strategies. J Antimicrob Chemother. (2012) 67:1607–15. doi: 10.1093/jac/dks084
44. Sader HS, Jones RN, Stilwell MG, Dowzicky MJ, Fritsche TR. Tigecycline activity tested against 26,474 bloodstream infection isolates: a collection from 6 continents. Diagn Microbiol Infect Dis. (2005) 52:181–6. doi: 10.1016/j.diagmicrobio.2005.05.005
45. Stein GE, Babinchak T. Tigecycline: an update. Diagn Microbiol Infect Dis. (2013) 75:331–6. doi: 10.1016/j.diagmicrobio.2012.12.004
46. Navon-Venezia S, Leavitt A, Carmeli Y. High tigecycline resistance in multidrug-resistant Acinetobacter baumannii. J Antimicrob Chemother. (2007) 59:772–4. doi: 10.1093/jac/dkm018
47. Gonzalez-Villoria AM, Valverde-Garduno V. Antibiotic-resistant Acinetobacter baumannii increasing success remains a challenge as a nosocomial pathogen. J Pathog. (2016) 2016:7318075. doi: 10.1155/2016/7318075
48. Kisil OV, Efimenko TA, Gabrielyan NI, Efremenkova OV. Development of antimicrobial therapy methods to overcome the antibiotic resistance of Acinetobacter baumannii. Acta Naturae. (2020) 12:34–45. doi: 10.32607/actanaturae.10955
49. Clatworthy AE, Pierson E, Hung DT. Targeting virulence: a new paradigm for antimicrobial therapy. Nat Chem Biol. (2007) 3:541–8. doi: 10.1038/nchembio.2007.24
50. Ramirez MS, Don M, Merkier AK, Bistué AJ, Zorreguieta A, Centrón D, et al. Naturally competent Acinetobacter baumannii clinical isolate as a convenient model for genetic studies. J Clin Microbiol. (2010) 48:1488–90. doi: 10.1128/JCM.01264-09
51. Touchon M, Cury J, Yoon EJ, Krizova L, Cerqueira GC, Murphy C, et al. The genomic diversification of the whole Acinetobacter genus: origins, mechanisms, and consequences. Genome Biol Evol. (2014) 6:2866–82. doi: 10.1093/gbe/evu225
52. Traglia GM, Chua K, Centron D, Tolmasky ME, Ramirez MS. Whole-genome sequence analysis of the naturally competent Acinetobacter baumannii clinical isolate A118. Genome Biol Evol. (2014) 6:2235–9. doi: 10.1093/gbe/evu176
53. Perez F, Hujer AM, Hujer KM, Decker BK, Rather PN, Bonomo RA. Global challenge of multidrug-resistant Acinetobacter baumannii. Antimicrob Agents Chemother. (2007) 51:3471–84. doi: 10.1128/AAC.01464-06
54. Munita JM, Arias CA. Mechanisms of antibiotic resistance. Microbiol Spectr. (2016) 4:2015. doi: 10.1128/microbiolspec.VMBF-0016-2015
55. Ambler RP. The structure of beta-lactamases. Philos Trans R Soc Lond B Biol Sci. (1980) 289:321–31. doi: 10.1098/rstb.1980.0049
56. Naas T, Dortet L, Iorga BI. Structural and functional aspects of class a carbapenemases. Curr Drug Targets. (2016) 17:1006–28. doi: 10.2174/1389450117666160310144501
57. Alyamani EJ, Khiyami MA, Booq RY, Alnafjan BM, Altammami MA, Bahwerth FS. Molecular characterization of extended-spectrum beta-lactamases (ESBLs) produced by clinical isolates of Acinetobacter baumannii in Saudi Arabia. Ann Clin Microbiol Antimicrob. (2015) 14:38. doi: 10.1186/s12941-015-0098-9
58. Abdar MH, Taheri-Kalani M, Taheri K, Emadi B, Hasanzadeh A, Sedighi A, et al. Prevalence of extended-spectrum beta-lactamase genes in Acinetobacter baumannii strains isolated from nosocomial infections in Tehran, Iran. GMS Hyg Infect Control. (2019) 14:318. doi: 10.3205/dgkh000318
59. Hamidian M, Hall RM. Resistance to third-generation cephalosporins in Acinetobacter baumannii due to horizontal transfer of a chromosomal segment containing ISAba1-ampC. J Antimicrob Chemother. (2014) 69:2865–6. doi: 10.1093/jac/dku202
60. Lee CR, Lee JH, Park M, Park KS, Bae IK, Kim YB, et al. Biology of Acinetobacter baumannii: pathogenesis, antibiotic resistance mechanisms, and prospective treatment options. Front Cell Infect Microbiol. (2017) 7:55. doi: 10.3389/fcimb.2017.00055
61. Jeon JH, Lee JH, Lee JJ, Park KS, Karim AM, Lee CR, et al. Structural basis for carbapenem-hydrolyzing mechanisms of carbapenemases conferring antibiotic resistance. Int J Mol Sci. (2015) 16:9654–92. doi: 10.3390/ijms16059654
62. Aksoy MD, Çavuşlu S, Tugrul HM. Investigation of metallo beta lactamases and oxacilinases in carbapenem resistant Acinetobacter baumannii strains isolated from inpatients. Balkan Med J. (2015) 32:79–83. doi: 10.5152/balkanmedj.2015.15302
63. Périchon B, Goussard S, Walewski V, Krizova L, Cerqueira G, Murphy C, et al. Identification of 50 class D β-lactamases and 65 Acinetobacter-derived cephalosporinases in Acinetobacter spp. Antimicrob Agents Chemother. (2014) 58:936–49. doi: 10.1128/AAC.01261-13
64. Brown S, Amyes S. OXA (beta)-lactamases in Acinetobacter: the story so far. J Antimicrob Chemother. (2006) 57:1–3. doi: 10.1093/jac/dki425
65. Turton JF, Woodford N, Glover J, Yarde S, Kaufmann ME, Pitt TL. Identification of Acinetobacter baumannii by detection of the blaOXA-51-like carbapenemase gene intrinsic to this species. J Clin Microbiol. (2006) 44:2974–6. doi: 10.1128/JCM.01021-06
66. Vijayakumar S, Gopi R, Gunasekaran P, Bharathy M, Walia K, Anandan S, et al. Molecular characterization of invasive carbapenem-resistant Acinetobacter baumannii from a Tertiary Care Hospital in South India. Infect Dis Ther. (2016) 5:379–87. doi: 10.1007/s40121-016-0125-y
67. Vrancianu CO, Gheorghe I, Czobor IB, Chifiriuc MC. Antibiotic resistance profiles, molecular mechanisms and innovative treatment strategies of Acinetobacter baumannii. Microorganisms. (2020) 8:935. doi: 10.3390/microorganisms8060935
68. Bonnin RA, Poirel L, Naas T, Pirs M, Seme K, Schrenzel J, et al. Dissemination of New Delhi metallo-β-lactamase-1-producing Acinetobacter baumannii in Europe. Clin Microbiol Infect. (2012) 18:E362–5. doi: 10.1111/j.1469-0691.2012.03928.x
69. Poirel L, Nordmann P. Carbapenem resistance in Acinetobacter baumannii: mechanisms and epidemiology. Clin Microbiol Infect. (2006) 12:826–36. doi: 10.1111/j.1469-0691.2006.01456.x
70. Nikibakhsh M, Firoozeh F, Badmasti F, Kabir K, Zibaei M. Molecular study of metallo-β-lactamases and integrons in Acinetobacter baumannii isolates from burn patients. BMC Infect Dis. (2021) 21:782. doi: 10.1186/s12879-021-06513-w
71. Rahman M, Prasad KN, Gupta S, Singh S, Singh A, Pathak A, et al. Prevalence and molecular characterization of New Delhi metallo-beta-lactamases in multidrug-resistant Pseudomonas aeruginosa and Acinetobacter baumannii from India. Microb Drug Resist. (2018) 24:792–98. doi: 10.1089/mdr.2017.0078
72. Coyne S, Courvalin P, Périchon B. Efflux-mediated antibiotic resistance in Acinetobacter spp. Antimicrob Agents Chemother. (2011) 55:947–53. doi: 10.1128/AAC.01388-10
73. Ribera A, Roca I, Ruiz J, Gibert I, Vila J. Partial characterization of a transposon containing the tet(A) determinant in a clinical isolate of Acinetobacter baumannii. J Antimicrob Chemother. (2003) 52:477–80. doi: 10.1093/jac/dkg344
74. ELsheredy A, Yousif Z, Elghazzawi E, Elmenshawy A, Ghazal A. Prevalence of genes encoding aminoglycoside-modifying enzymes and armA among Acinetobacter baumannii clinical isolates in alexandria, Egypt. Infect Disord Drug Targets. (2021) 24:3041. doi: 10.2174/1871526521666210225113041
75. Vashist J, Tiwari V, Das R, Kapil A, Rajeswari MR. Analysis of penicillin-binding proteins (PBPs) in carbapenem resistant Acinetobacter baumannii. Indian J Med Res. (2011) 133:332–8.
76. Vila J, Martí S, Sánchez-Céspedes J. Porins, efflux pumps and multidrug resistance in Acinetobacter baumannii. J Antimicrob Chemother. (2007) 59:1210–5. doi: 10.1093/jac/dkl509
77. Costerton JW, Stewart PS, Greenberg EP. Bacterial biofilms: a common cause of persistent infections. Science. (1999) 284:1318–22. doi: 10.1126/science.284.5418.1318
78. Stoodley P, Hall-Stoodley L, Lappin-Scott HM. Detachment, surface migration, and other dynamic behavior in bacterial biofilms revealed by digital time-lapse imaging. Methods Enzymol. (2001) 337:306–19. doi: 10.1016/s0076-6879(01)37023-4
79. Loehfelm TW, Luke NR, Campagnari AA. Identification and characterization of an Acinetobacter baumannii biofilm-associated protein. J Bacteriol. (2008) 190:1036–44. doi: 10.1128/JB.01416-07
80. De Gregorio E, Del Franco M, Martinucci M, Roscetto E, Zarrilli R, Di Nocera PP. Biofilm-associated proteins: news from Acinetobacter. BMC Genomics. (2015) 16:933. doi: 10.1186/s12864-015-2136-6
81. Itoh Y, Rice JD, Goller C, Pannuri A, Taylor J, Meisner J, et al. Roles of pgaABCD genes in synthesis, modification, and export of the Escherichia coli biofilm adhesin poly-beta-1,6-N-acetyl-D-glucosamine. J Bacteriol. (2008) 190:3670–80. doi: 10.1128/JB.01920-07
82. Flannery A, Le Berre M, Pier GB, O'Gara JP, Kilcoyne M. Glycomics microarrays reveal differential in situ presentation of the biofilm polysaccharide poly-N-acetylglucosamine on Acinetobacter baumannii and Staphylococcus aureus cell surfaces. Int J Mol Sci. (2020) 21:2465. doi: 10.3390/ijms21072465
83. Lee HW, Koh YM, Kim J, Lee JC, Lee YC, Seol SY, et al. Capacity of multidrug-resistant clinical isolates of Acinetobacter baumannii to form biofilm and adhere to epithelial cell surfaces. Clin Microbiol Infect. (2008) 14:49–54. doi: 10.1111/j.1469-0691.2007.01842.x
84. Pakharukova N, Tuittila M, Paavilainen S, Malmi H, Parilova O, Teneberg S, et al. Structural basis for Acinetobacter baumannii biofilm formation. Proc Natl Acad Sci USA. (2018) 115:5558–63. doi: 10.1073/pnas.1800961115
85. Gaddy JA, Actis LA. Regulation of Acinetobacter baumannii biofilm formation. Future Microbiol. (2009) 4:273–8. doi: 10.2217/fmb.09.5
86. Gaddy JA, Tomaras AP, Actis LA. The Acinetobacter baumannii 19606 OmpA protein plays a role in biofilm formation on abiotic surfaces and in the interaction of this pathogen with eukaryotic cells. Infect Immun. (2009) 77:3150–60. doi: 10.1128/IAI.00096-09
87. Bhargava N, Sharma P, Capalash N. Quorum sensing in Acinetobacter: an emerging pathogen. Crit Rev Microbiol. (2010) 36:349–60. doi: 10.3109/1040841X.2010.512269
88. López-Martín M, Dubern JF, Alexander MR, Williams P. AbaM regulates quorum sensing, biofilm formation, and virulence in Acinetobacter baumannii. J Bacteriol. (2021) 203:e00635–20. doi: 10.1128/JB.00635-20
89. Lasa I, Penadés JR. Bap: a family of surface proteins involved in biofilm formation. Res Microbiol. (2006) 157:99–107. doi: 10.1016/j.resmic.2005.11.003
90. Azizi O, Shahcheraghi F, Salimizand H, Modarresi F, Shakibaie MR, Mansouri Sh, et al. Molecular analysis and expression of bap gene in biofilm-forming multi-drug-resistant Acinetobacter baumannii. Rep Biochem Mol Biol. (2016) 5:62–72.
91. Choi AH, Slamti L, Avci FY, Pier GB, Maira-Litrán T. The pgaABCD locus of Acinetobacter baumannii encodes the production of poly-beta-1-6-N-acetylglucosamine, which is critical for biofilm formation. J Bacteriol. (2009) 191:5953–63. doi: 10.1128/JB.00647-09
92. Rao RS, Karthika RU, Singh SP, Shashikala P, Kanungo R, Jayachandran S, et al. Correlation between biofilm production and multiple drug resistance in imipenem resistant clinical isolates of Acinetobacter baumannii. Indian J Med Microbiol. (2008) 26:333–7. doi: 10.4103/0255-0857.43566
93. Khamari B, Lama M, Pachi Pulusu C, Biswal AP, Lingamallu SM, Mukkirla BS, et al. Molecular analyses of biofilm-producing clinical Acinetobacter baumannii isolates from a South Indian Tertiary Care Hospital. Med Princ Pract. (2020) 29:580–7. doi: 10.1159/000508461
94. Bardbari AM, Arabestani MR, Karami M, Keramat F, Alikhani MY, Bagheri KP. Correlation between ability of biofilm formation with their responsible genes and MDR patterns in clinical and environmental Acinetobacter baumannii isolates. Microb Pathog. (2017) 108:122–8. doi: 10.1016/j.micpath.2017.04.039
95. Tomaras AP, Dorsey CW, Edelmann RE, Actis LA. Attachment to and biofilm formation on abiotic surfaces by Acinetobacter baumannii: involvement of a novel chaperone-usher pili assembly system. Microbiology. (2003) 149:3473–84. doi: 10.1099/mic.0.26541-0
96. Tomaras AP, Flagler MJ, Dorsey CW, Gaddy JA, Actis LA. Characterization of a two-component regulatory system from Acinetobacter baumannii that controls biofilm formation and cellular morphology. Microbiology. (2008) 154:3398–409. doi: 10.1099/mic.0.2008/019471-0
97. Harding CM, Hennon SW, Feldman MF. Uncovering the mechanisms of Acinetobacter baumannii virulence. Nat Rev Microbiol. (2018) 16:91–102. doi: 10.1038/nrmicro.2017.148
98. Selasi GN, Nicholas A, Jeon H, Na SH, Kwon HI, Kim YJ, et al. Differences in biofilm mass, expression of biofilm-associated genes, and resistance to desiccation between epidemic and sporadic clones of carbapenem-resistant Acinetobacter baumannii sequence type 191. PLoS ONE. (2016) 11:e0162576. doi: 10.1371/journal.pone.0162576
99. McConnell MJ, Actis L, Pachón J. Acinetobacter baumannii: human infections, factors contributing to pathogenesis and animal models. FEMS Microbiol Rev. (2013) 37:130–55. doi: 10.1111/j.1574-6976.2012.00344.x
100. Rumbo C, Tomás M, Fernández ME, Soares NC, Carvajal M, Santillana E, et al. The Acinetobacter baumannii Omp33-36 porin is a virulence factor that induces apoptosis and modulates autophagy in human cells. Infect Immun. (2014) 82:4666–80. doi: 10.1128/IAI.02034-14
101. Ng WL, Bassler BL. Bacterial quorum-sensing network architectures. Annu Rev Genet. (2009) 43:197–222. doi: 10.1146/annurev-genet-102108-134304
102. Dou Y, Song F, Guo F, Zhou Z, Zhu C, Xiang J, et al. Acinetobacter baumannii quorum-sensing signalling molecule induces the expression of drug-resistance genes. Mol Med Rep. (2017) 15:4061–8. doi: 10.3892/mmr.2017.6528
103. Oh MH, Han K. AbaR is a LuxR type regulator essential for motility and the formation of biofilm and pellicle in Acinetobacter baumannii. Genes Genomics. (2020) 42:1339–46. doi: 10.1007/s13258-020-01005-8
104. Mayer C, Muras A, Parga A, Romero M, Rumbo-Feal S, Poza M, et al. Quorum sensing as a target for controlling surface associated motility and biofilm formation in Acinetobacter baumannii ATCC® 17978TM. Front Microbiol. (2020) 11:565548. doi: 10.3389/fmicb.2020.565548
105. Luo LM, Wu LJ, Xiao YL, Zhao D, Chen ZX, Kang M, et al. Enhancing pili assembly and biofilm formation in Acinetobacter baumannii ATCC19606 using non-native acyl-homoserine lactones. BMC Microbiol. (2015) 15:62. doi: 10.1186/s12866-015-0397-5
106. Rumbo-Feal S, Gomez MJ, Gayoso C, Álvarez-Fraga L, Cabral MP, Aransay AM, et al. Whole transcriptome analysis of Acinetobacter baumannii assessed by RNA-sequencing reveals different mRNA expression profiles in biofilm compared to planktonic cells. PLoS ONE. (2013) 8:e72968. doi: 10.1371/journal.pone.0072968
107. Alav I, Sutton JM, Rahman KM. Role of bacterial efflux pumps in biofilm formation. J Antimicrob Chemother. (2018) 73:2003–20. doi: 10.1093/jac/dky042
108. He X, Lu F, Yuan F, Jiang D, Zhao P, Zhu J, et al. Biofilm formation caused by clinical Acinetobacter baumannii isolates is associated with overexpression of the AdeFGH efflux pump. Antimicrob Agents Chemother. (2015) 59:4817–25. doi: 10.1128/AAC.00877-15
109. Roca I, Espinal P, Martí S, Vila J. First identification and characterization of an AdeABC-like efflux pump in Acinetobacter genomospecies 13TU. Antimicrob Agents Chemother. (2011) 55:1285–6. doi: 10.1128/AAC.01142-10
110. Greene C, Wu J, Rickard AH, Xi C. Evaluation of the ability of Acinetobacter baumannii to form biofilms on six different biomedical relevant surfaces. Lett Appl Microbiol. (2016) 63:233–9. doi: 10.1111/lam.12627
111. Eze EC, El Zowalaty ME. Combined effects of low incubation temperature, minimal growth medium, and low hydrodynamics optimize Acinetobacter baumannii biofilm formation. Infect Drug Resist. (2019) 12:3523–36. doi: 10.2147/IDR.S203919
112. Khatoon Z, McTiernan CD, Suuronen EJ, Mah TF, Alarcon EI. Bacterial biofilm formation on implantable devices and approaches to its treatment and prevention. Heliyon. (2018) 4:e01067. doi: 10.1016/j.heliyon.2018.e01067
113. Jones DS, Gorman SP. Medical device composition and biological secretion influences on biofilm formation. In: Biofilms, Infection, and Antimicrobial Therapy. CRC Press (2005). p. 62–81.
114. Dekic S, Hrenovic J, Ivankovic T, van Wilpe E. Survival of ESKAPE pathogen Acinetobacter baumannii in water of different temperatures and pH. Water Sci Technol. (2018) 78:1370–6. doi: 10.2166/wst.2018.409
115. Pour NK, Dusane DH, Dhakephalkar PK, Zamin FR, Zinjarde SS, Chopade BA. Biofilm formation by Acinetobacter baumannii strains isolated from urinary tract infection and urinary catheters. FEMS Immunol Med Microbiol. (2011) 62:328–38. doi: 10.1111/j.1574-695X.2011.00818.x
116. De Silva PM, Chong P, Fernando DM, Westmacott G, Kumar A. Effect of incubation temperature on antibiotic resistance and virulence factors of acinetobacter baumannii ATCC 17978. Antimicrob Agents Chemother. (2017) 62:e01514-17. doi: 10.1128/AAC.01514-17
117. Martinez LR, Casadevall A. Cryptococcus neoformans biofilm formation depends on surface support and carbon source and reduces fungal cell susceptibility to heat, cold, and UV light. Appl Environ Microbiol. (2007) 73:4592–601. doi: 10.1128/AEM.02506-06
118. Nwugo CC, Arivett BA, Zimbler DL, Gaddy JA, Richards AM, Actis LA. Effect of ethanol on differential protein production and expression of potential virulence functions in the opportunistic pathogen Acinetobacter baumannii. PLoS ONE. (2012) 7:e51936. doi: 10.1371/journal.pone.0051936
119. Gilbert P, Das J, Foley I. Biofilm susceptibility to antimicrobials. Adv Dent Res. (1997) 11:160–7. doi: 10.1177/08959374970110010701
120. Harrison JJ, Ceri H, Turner RJ. Multimetal resistance and tolerance in microbial biofilms. Nat Rev Microbiol. (2007) 5:928–38. doi: 10.1038/nrmicro1774
121. Montanaro L, Poggi A, Visai L, Ravaioli S, Campoccia D, Speziale P, et al. Extracellular DNA in biofilms. Int J Artif Organs. (2011) 34:824–31. doi: 10.5301/ijao.5000051
122. Das T, Sharma PK, Busscher HJ, van der Mei HC, Krom BP. Role of extracellular DNA in initial bacterial adhesion and surface aggregation. Appl Environ Microbiol. (2010) 76:3405–8. doi: 10.1128/AEM.03119-09
123. Sahu PK, Iyer PS, Oak AM, Pardesi KR, Chopade BA. Characterization of eDNA from the clinical strain Acinetobacter baumannii AIIMS 7 and its role in biofilm formation. Sci World J. (2012) 973436. doi: 10.1100/2012/973436
124. Stewart PS, Franklin MJ. Physiological heterogeneity in biofilms. Nat Rev Microbiol. (2008) 6:199–210. doi: 10.1038/nrmicro1838
125. Brown MR, Allison DG, Gilbert P. Resistance of bacterial biofilms to antibiotics: a growth-rate related effect? J Antimicrob Chemother. (1988) 22:777–80. doi: 10.1093/jac/22.6.777
126. Conlon BP, Rowe SE, Lewis K. Persister cells in biofilm associated infections. Adv Exp Med Biol. (2015) 831:1–9. doi: 10.1007/978-3-319-09782-4_1
127. Desai M, Bühler T, Weller PH, Brown MR. Increasing resistance of planktonic and biofilm cultures of Burkholderia cepacia to ciprofloxacin and ceftazidime during exponential growth. J Antimicrob Chemother. (1998) 42:153–60. doi: 10.1093/jac/42.2.153
128. Tipton KA, Dimitrova D, Rather PN. Phase-variable control of multiple phenotypes in Acinetobacter baumannii strain AB5075. J Bacteriol. (2015) 197:2593–9. doi: 10.1128/JB.00188-15
129. Fauvart M, De Groote VN, Michiels J. Role of persister cells in chronic infections: clinical relevance and perspectives on anti-persister therapies. J Med Microbiol. (2011) 60:699–709. doi: 10.1099/jmm.0.030932-0
130. Gayoso CM, Mateos J, Méndez JA, Fernández-Puente P, Rumbo C, Tomás M, et al. Molecular mechanisms involved in the response to desiccation stress and persistence in Acinetobacter baumannii. J Proteome Res. (2014) 13:460–76. doi: 10.1021/pr400603f
131. Rittershaus ES, Baek SH, Sassetti CM. The normalcy of dormancy: common themes in microbial quiescence. Cell Host Microbe. (2013) 13:643–51. doi: 10.1016/j.chom.2013.05.012
132. Harding CM, Hennon SW, Feldman MF. Uncovering the mechanisms of Acinetobacter baumannii virulence. Nat Rev Microbiol. (2018) 16:91–102. doi: 10.1038/nrmicro.2017
133. Barth VC Jr, Rodrigues BÁ, Bonatto GD, Gallo SW, Pagnussatti VE, Ferreira CA, et al. Heterogeneous persister cells formation in Acinetobacter baumannii. PLoS ONE. (2013) 8:e84361. doi: 10.1371/journal.pone.0084361
134. Kushwaha GS, Oyeyemi BF, Bhavesh NS. Stringent response protein as a potential target to intervene persistent bacterial infection. Biochimie. (2019) 165:67–75. doi: 10.1016/j.biochi.2019.07.006
135. Pérez-Varela M, Tierney ARP, Kim JS, Vázquez-Torres A, Rather P. Characterization of RelA in Acinetobacter baumannii. J Bacteriol. (2020) 202:e00045–20. doi: 10.1128/JB.00045-20
136. Dörr T, Lewis K, Vulić M. SOS response induces persistence to fluoroquinolones in Escherichia coli. PLoS Genet. (2009) 5:e1000760. doi: 10.1371/journal.pgen.1000760
137. Alkasir R, Ma Y, Liu F, Li J, Lv N, Xue Y, et al. Characterization and transcriptome analysis of Acinetobacter baumannii persister cells. Microb Drug Resist. (2018) 24:1466–74. doi: 10.1089/mdr.2017.0341
138. Hengge-Aronis R. Regulation of gene expression during entry into stationary phase. In: Neidhardt FC, Curtiss R III, Ingraham JL, Lin ECC, Low KB, Magasanik B, Reznikoff WS, Riley M, Schaechter M, and Umbarger HE. Escherichia coli Salmonella: Cellular Molecular Biology, 2nd Edn. Washington, DC: ASM Press (1996). p. 1497–512.
139. Thummeepak R, Kongthai P, Leungtongkam U, Sitthisak S. Distribution of virulence genes involved in biofilm formation in multi-drug resistant Acinetobacter baumannii clinical isolates. Int Microbiol. (2016) 19:121–9. doi: 10.2436/20.1501.01.270
140. Yang CH, Su PW, Moi SH, Chuang LY. Biofilm formation in Acinetobacter Baumannii: genotype-phenotype correlation. Molecules. (2019) 24:1849. doi: 10.3390/molecules24101849
141. Ranjbar R, Farahani A. Study of genetic diversity, biofilm formation, and detection of Carbapenemase, MBL, ESBL, and tetracycline resistance genes in multidrug-resistant Acinetobacter baumannii isolated from burn wound infections in Iran. Antimicrob Resist Infect Control. (2019) 8:172. doi: 10.1186/s13756-019-0612-5
142. Celik B. Evaluation of the correlation between biofilm formation and drug resistance in clinical isolates of Acinetobacter baumannii. Int J Pathog Res. (2020) 5:16–27. doi: 10.9734/ijpr/2020/v5i130124
143. Asaad AM, Ansari S, Ajlan SE, Awad SM. Epidemiology of biofilm producing Acinetobacter baumannii nosocomial isolates from a tertiary care hospital in Egypt: a cross-sectional study. Infect Drug Resist. (2021) 14:709–17. doi: 10.2147/IDR.S261939
144. Nahar A, Anwar S, Miah MR. Association of biofilm formation with antimicrobial resistance among the acinetobacter species in a tertiary care hospital in Bangladesh. J Med. (2013) 14:28–32. doi: 10.3329/jom.v14i1.14533
145. Emami S, Eftekhar F. The correlation between biofilm formation and drug resistance in nosocomial isolates of Acinetobacter baumannii. Avicenna J Clin Microbiol Infect. (2015) 2:e23954. doi: 10.17795/ajcmi-23954
146. Rodríguez-Baño J, Martí S, Soto S, Fernández-Cuenca F, Cisneros JM, Pachón J, et al. Biofilm formation in Acinetobacter baumannii: associated features and clinical implications. Clin Microbiol Infect. (2008) 14:276–8. doi: 10.1111/j.1469-0691.2007.01916.x
147. Han X, Li Q, Shen L, Hu D, Qu Y. Correlation between the biofilm-forming ability, biofilm-related genes and antimicrobial resistance of Acinetobacter baumannii. Zhonghua Wei Zhong Bing Ji Jiu Yi Xue. (2014) 26:639–43. doi: 10.3760/cma.j.issn.2095-4352.2014.09.007
148. Zhang D, Xia J, Xu Y, Gong M, Zhou Y, Xie L, et al. Biological features of biofilm-forming ability of Acinetobacter baumannii strains derived from 121 elderly patients with hospital-acquired pneumonia. Clin Exp Med. (2016) 16:73–80. doi: 10.1007/s10238-014-0333-2
149. Krzyściak P, Chmielarczyk A, Pobiega M, Romaniszyn D, Wójkowska-Mach J. Acinetobacter baumannii isolated from hospital-acquired infection: biofilm production and drug susceptibility. APMIS. (2017) 125:1017–26. doi: 10.1111/apm.12739
150. Wang YC, Huang TW, Yang YS, Kuo SC, Chen CT, Liu CP, et al. Biofilm formation is not associated with worse outcome in Acinetobacter baumannii bacteraemic pneumonia. Sci Rep. (2018) 8:7289. doi: 10.1038/s41598-018-25661-9
151. Shenkutie AM, Yao MZ, Siu GK, Wong BKC, Leung PH. Biofilm-induced antibiotic resistance in clinical Acinetobacter baumannii isolates. Antibiotics. (2020) 9:817. doi: 10.3390/antibiotics9110817
152. Donadu MG, Mazzarello V, Cappuccinelli P, Zanetti S, Madléna M, Nagy ÁL, et al. Relationship between the biofilm-forming capacity and antimicrobial resistance in clinical Acinetobacter baumannii isolates: results from a laboratory-based in vitro study. Microorganisms. (2021) 9:2384. doi: 10.3390/microorganisms9112384
153. Carattoli A. Plasmids and the spread of resistance. Int J Med Microbiol. (2013) 303:298–304. doi: 10.1016/j.ijmm.2013.02.001
154. Wozniak RA, Waldor MK. Integrative and conjugative elements: mosaic mobile genetic elements enabling dynamic lateral gene flow. Nat Rev Microbiol. (2010) 8:552–63. doi: 10.1038/nrmicro2382
155. Partridge SR, Kwong SM, Firth N, Jensen SO. Mobile genetic elements associated with antimicrobial resistance. Clin Microbiol Rev. (2018) 31:e00088–17. doi: 10.1128/CMR.00088-17
156. Calero-Cáceres W, Ye M, Balcázar JL. Bacteriophages as environmental reservoirs of antibiotic resistance. Trends Microbiol. (2019) 27:570–7. doi: 10.1016/j.tim.2019.02.008
157. Hathroubi S, Mekni MA, Domenico P, Nguyen D, Jacques M. Biofilms: microbial shelters against antibiotics. Microb Drug Resist. (2017) 23:147–56. doi: 10.1089/mdr.2016.0087
158. Hausner M, Wuertz S. High rates of conjugation in bacterial biofilms as determined by quantitative in situ analysis. Appl Environ Microbiol. (1999) 65:3710–3. doi: 10.1128/AEM.65.8.3710-3713.1999
159. Molin S, Tolker-Nielsen T. Gene transfer occurs with enhanced efficiency in biofilms and induces enhanced stabilisation of the biofilm structure. Curr Opin Biotechnol. (2003) 14:255–61. doi: 10.1016/s0958-1669(03)00036-3
160. Aminov RI. Horizontal gene exchange in environmental microbiota. Front Microbiol. (2011) 2:158. doi: 10.3389/fmicb.2011.00158
161. Madsen JS, Burmølle M, Hansen LH, Sørensen SJ. The interconnection between biofilm formation and horizontal gene transfer. FEMS Immunol Med Microbiol. (2012) 65:183–95. doi: 10.1111/j.1574-695X.2012.00960.x
162. Davey ME, O'toole GA. Microbial biofilms: from ecologyto molecular genetics. Microbiol Mol Biol R. (2000) 67:847–67. doi: 10.1128/MMBR.64.4.847-867.2000
163. Williams HG, Day MJ, Fry JC, Stewart GJ. Natural transformation in river epilithon. Appl Environ Microbiol. (1996) 62:2994–8. doi: 10.1128/aem.62.8.2994-2998.1996
164. McInnes RS, McCallum GE, Lamberte LE, van Schaik W. Horizontal transfer of antibiotic resistance genes in the human gut microbiome. Curr Opin Microbiol. (2020) 53:35–43. doi: 10.1016/j.mib.2020.02.002
165. Maeusli M, Lee B, Miller S, Reyna Z, Lu P, Yan J, et al. Horizontal gene transfer of antibiotic resistance from Acinetobacter baylyi to Escherichia coli on lettuce and subsequent antibiotic resistance transmission to the gut microbiome. mSphere. (2020) 5:e00329–20. doi: 10.1128/mSphere.00329-20
166. Bridier A, Briandet R, Thomas V, Dubois-Brissonnet F. Resistance of bacterial biofilms to disinfectants: a review. Biofouling. (2011) 27:1017–32. doi: 10.1080/08927014.2011.626899
167. Hayashi M, Kawamura K, Matsui M, Suzuki M, Suzuki S, Shibayama K, et al. Reduction in chlorhexidine efficacy against multi-drug-resistant Acinetobacter baumannii international clone II. J Hosp Infect. (2017) 95:318–23. doi: 10.1016/j.jhin.2016.12.004
168. Ivanković T, Goić-Barišić I, Hrenović J. Reduced susceptibility to disinfectants of Acinetobacter baumannii biofilms on glass and ceramic. Arh Hig Rada Toksikol. (2017) 68:99–108. doi: 10.1515/aiht-2017-68-2946
169. Tipton KA, Chin CY, Farokhyfar M, Weiss DS, Rather PN. Role of capsule in resistance to disinfectants, host antimicrobials, and desiccation in Acinetobacter baumannii. Antimicrob Agents Chemother. (2018) 62:e01188–18. doi: 10.1128/AAC.01188-18
170. Nor A'shimi MH, Alattraqchi AG, Mohd Rani F, A Rahman NI, Ismail S, Abdullah FH, et al. Biocide susceptibilities and biofilm-forming capacities of Acinetobacter baumannii clinical isolates from Malaysia. J Infect Dev Ctries. (2019) 13:626–33. doi: 10.3855/jidc.11455
171. Wassenaar TM, Ussery D, Nielsen LN, Ingmer H. Review and phylogenetic analysis of qac genes that reduce susceptibility to quaternary ammonium compounds in Staphylococcus species. Eur J Microbiol Immunol. (2015) 5:44–61. doi: 10.1556/EUJMI-D-14-00038
172. Zarrilli R. Acinetobacter baumannii virulence determinants involved in biofilm growth and adherence to host epithelial cells. Virulence. (2016) 7:367–8. doi: 10.1080/21505594.2016.1150405
173. Donlan RM. Biofilm formation: a clinically relevant microbiological process. Clin Infect Dis. (2001) 33:1387–92. doi: 10.1086/322972
174. Djeribi R, Bouchloukh W, Jouenne T, Menaa B. Characterization of bacterial biofilms formed on urinary catheters. Am J Infect Control. (2012) 40:854–9. doi: 10.1016/j.ajic.2011.10.009
175. Gil-Perotin S, Ramirez P, Marti V, Sahuquillo JM, Gonzalez E, Calleja I, et al. Implications of endotracheal tube biofilm in ventilator-associated pneumonia response: a state of concept. Crit Care. (2012) 16:R93. doi: 10.1186/cc11357
176. Ferreira TO, Koto RY, Leite GF, Klautau GB, Nigro S, Silva CB, et al. Microbial investigation of biofilms recovered from endotracheal tubes using sonication in intensive care unit pediatric patients. Braz J Infect Dis. (2016) 20:468–75. doi: 10.1016/j.bjid.2016.07.003
177. Yeo HJ, Yoon SH, Lee SE, Cho WH, Kim D, Jeon D, et al. Bacterial biofilms on extracorporeal membrane oxygenation catheters. ASAIO J. (2018) 64:e48–54. doi: 10.1097/MAT.0000000000000750
178. Donlan RM. Biofilms and device-associated infections. Emerg Infect Dis. (2001) 7:277–81. doi: 10.3201/eid0702.010226
179. Francolini I, Donelli G. Prevention and control of biofilm-based medical-device-related infections. FEMS Immunol Med Microbiol. (2010) 59:227–38. doi: 10.1111/j.1574-695X.2010.00665.x
180. Rello J, Soñora R, Jubert P, Artigas A, Rué M, Vallés J. Pneumonia in intubated patients: role of respiratory airway care. Am J Respir Crit Care Med. (1996) 154:111–5. doi: 10.1164/ajrccm.154.1.8680665
181. Raveendra N, Rathnakara SH, Haswani N, Subramaniam V. Bacterial biofilms on tracheostomy tubes. Indian J Otolaryngol Head Neck Surg. (2021) 6:1–5. doi: 10.1007/s12070-021-02598-6
182. Gaynes R, Edwards JR, National Nosocomial Infections Surveillance System. Overview of nosocomial infections caused by gram-negative bacilli. Clin Infect Dis. (2005) 41:848–54. doi: 10.1086/432803
183. Malacarne P, Corini M, Maremmani P, Viaggi B, Verdigi S. Diagnostic characteristics of routine surveillance cultures of endotracheal aspirate samples in cases of late-onset ventilator-associated pneumonia due to Acinetobacter baumannii. Infect Control Hosp Epidemiol. (2007) 28:867–9. doi: 10.1086/518728
184. Bouza E, Pérez A, Muñoz P, Jesús Pérez M, Rincón C, Sánchez C, et al. Ventilator-associated pneumonia after heart surgery: a prospective analysis and the value of surveillance. Crit Care Med. (2003) 31:1964–70. doi: 10.1097/01.ccm.0000084807.15352.93
185. Hayon J, Figliolini C, Combes A, Trouillet JL, Kassis N, Dombret MC, et al. Role of serial routine microbiologic culture results in the initial management of ventilator-associated pneumonia. Am J Respir Crit Care Med. (2002) 165:41–6. doi: 10.1164/ajrccm.165.1.2105077
186. Depuydt P, Benoit D, Vogelaers D, Decruyenaere J, Vandijck D, Claeys G, et al. Systematic surveillance cultures as a tool to predict involvement of multidrug antibiotic resistant bacteria in ventilator-associated pneumonia. Intensive Care Med. (2008) 34:675–82. doi: 10.1007/s00134-007-0953-z
187. Tsakiridou E, Makris D, Daniil Z, Manoulakas E, Chatzipantazi V, Vlachos O, et al. Acinetobacter baumannii infection in prior ICU bed occupants is an independent risk factor for subsequent cases of ventilator-associated pneumonia. Biomed Res Int. (2014) 2014:193516. doi: 10.1155/2014/193516
188. Souza LCD, Mota VBRD, Carvalho AVDSZ, Corrêa RDGCF, Libério SA, Lopes FF. Association between pathogens from tracheal aspirate and oral biofilm of patients on mechanical ventilation. Braz Oral Res. (2017) 31:e38. doi: 10.1590/1807-3107BOR-2017.vol31.0038
189. Natham H, Kondagadapu S, Kadiyala V, Mohan A, Chaudhury A, Samantaray A. Bacterial colonisation and antibiotic sensitivity profile of endotracheal tubes in mechanically ventilated patients. J Clin Diagn Res. (2019) 13:UC01–6. doi: 10.7860/JCDR/2019/37402.12457
190. Centers for Disease Control and Prevention. Pneumonia (Ventilator-Associated [VAP] and Nonventilator-Associated Pneumonia [PNEU]) Event. Available online at: https://www.cdc.gov/nhsn/pdfs/pscmanual/6pscvapcurrent.pdf (accessed January 2021).
191. Ebenezer K, James EJ, Michael JS, Kang G, Verghese VP. Ventilator-associated Acinetobacter baumannii pneumonia. Indian Pediatr. (2011) 48:964–6. doi: 10.1007/s13312-011-0152-4
192. Chen J, Yang Y, Xiang K, Li D, Liu H. Combined rifampin and sulbactam therapy for multidrug-resistant acinetobacter baumannii ventilator-associated pneumonia in pediatric patients. J AnesthPerioper Med. (2018) 5:176–85. doi: 10.24015/JAPM.2018.0072
193. Thatrimontrichai A, Techato C, Dissaneevate S, Janjindamai W, Maneenil G, Kritsaneepaiboon S, et al. Risk factors and outcomes of carbapenem-resistant Acinetobacter baumannii ventilator-associated pneumonia in the neonate: a case-case-control study. J Infect Chemother. (2016) 22:444–9. doi: 10.1016/j.jiac.2016.03.013
194. Iosifidis E, Pitsava G, Roilides E. Ventilator-associated pneumonia in neonates and children: a systematic analysis of diagnostic methods and prevention. Future Microbiol. (2018) 13:1431–46. doi: 10.2217/fmb-2018-0108
195. Asadian M, Azimi L, Alinejad F, Ostadi Y, Lari AR. Molecular characterization of Acinetobacter baumannii isolated from ventilator-associated pneumonia and burn wound colonization by random amplified polymorphic DNA polymerase chain reaction and the relationship between antibiotic susceptibility and biofilm production. Adv Biomed Res. (2019) 8:58. doi: 10.4103/abr.abr_256_18
196. Kara SS, Polat M, Tapisiz A, Kalkan G, Simsek H, Tezer H. Ventilator associated pneumonia due to carbapenem resistant microorganisms in children. Minerva Pediatr. (2019) 71:349–57. doi: 10.23736/S0026-4946.17.04284-0
197. Gominet M, Compain F, Beloin C, Lebeaux D. Central venous catheters and biofilms: where do we stand in 2017? APMIS. (2017) 125:365–75. doi: 10.1111/apm.12665
198. Storti A, Pizzolitto AC, Pizzolitto EL. Detection of mixed microbial biofilms on central venous catheters removed from intensive care unit patients. Braz J Microbiol. (2005) 36:275–80. doi: 10.1590/S1517-83822005000300013
199. Basher H, Farhana A, Alim S, Khan S. Detection of catheter related blood stream infections in an ICU of a tertiary care center in Northern India. IJMR. (2021) 8:102–7. doi: 10.18231/j.ijmr.2021.020
200. Yoon EJ, Kim D, Lee H, Lee HS, Shin JH, Uh Y, et al. Counter clinical prognoses of patients with bloodstream infections between causative Acinetobacter baumannii clones ST191 and ST451 belonging to the international clonal lineage II. Front Public Health. (2019) 7:233. doi: 10.3389/fpubh.2019.00233
201. Yu K, Zeng W, Xu Y, Liao W, Xu W, Zhou T, et al. Bloodstream infections caused by ST2 Acinetobacter baumannii: risk factors, antibiotic regimens, and virulence over 6 years period in China. Antimicrob Resist Infect Control. (2021) 10:16. doi: 10.1186/s13756-020-00876-6
202. Downes KJ, Metlay JP, Bell LM, McGowan KL, Elliott MR, Shah SS. Polymicrobial bloodstream infections among children and adolescents with central venous catheters evaluated in ambulatory care. Clin Infect Dis. (2008) 46:387–94. doi: 10.1086/525265
203. Ozdemir H, Kendirli T, Ergün H, Ciftçi E, Tapisiz A, Güriz H, et al. Nosocomial infections due to Acinetobacter baumannii in a pediatric intensive care unit in Turkey. Turk J Pediatr. (2011) 53:255–60.
204. Thomas D, Parameswaran N, Harish BN. Catheter related blood stream infections in the paediatric intensive care unit: a descriptive study. Indian J Crit Care Med. (2013) 17:135–9. doi: 10.4103/0972-5229.117038
205. Nicolle LE. Catheter-related urinary tract infection. Drugs Aging. (2005) 22:627–39. doi: 10.2165/00002512-200522080-00001
206. Gilmore BF, Carson L. Bioactive biomaterials for controlling biofilms. In: Barnes L, Cooper I, editors. Biomaterials and Medical Device-Associated Infections. 1st ed., Chapter 8. Woodhead (2014). p. 281.
207. Morris FC, Dexter C, Kostoulias X, Uddin MI, Peleg AY. The mechanisms of disease caused by Acinetobacter baumannii. Front Microbiol. (2019) 10:1601. doi: 10.3389/fmicb.2019.01601
208. De Francesco MA, Ravizzola G, Peroni L, Negrini R, Manca N. Urinary tract infections in Brescia, Italy: etiology of uropathogens and antimicrobial resistance of common uropathogens. Med Sci Monit. (2007) 13:BR136–44.
209. Sievert DM, Ricks P, Edwards JR, Schneider A, Patel J, Srinivasan A, et al. Antimicrobial-resistant pathogens associated with healthcare-associated infections: summary of data reported to the National Healthcare Safety Network at the Centers for Disease Control and Prevention, 2009-2010. Infect Control Hosp Epidemiol. (2013) 34:1–14. doi: 10.1086/668770
210. Alves MJ, Barreira JCM, Carvalho I, Trinta L, Perreira L, Ferreira ICFR, et al. Propensity for biofilm formation by clinical isolates from urinary tract infections: developing a multifactorial predictive model to improve antibiotherapy. J Med Microbiol. (2014) 63:471–7. doi: 10.1099/jmm.0.071746-0
211. Maharjan G, Khadka P, Siddhi SG, Chapagain G, Dhungana GR. Catheter-associated urinary tract infection and obstinate biofilm producers. Can J Infect Dis Med Microbiol. (2018) 2018:7624857. doi: 10.1155/2018/7624857
212. Bagińska N, Cieślik M, Górski A, Jończyk-Matysiak E. The role of antibiotic resistant A. baumannii in the pathogenesis of urinary tract infection and the potential of its treatment with the use of bacteriophage therapy. Antibiotics. (2021) 10:281. doi: 10.3390/antibiotics10030281
213. Mashouf RY, Babalhavaeji H, Yousef J. Urinary tract infections: bacteriology and antibiotic resistance patterns. Indian Pediatr. (2009) 46:617–20.
214. Madhu GN, AnjumAara CA, Mahamud S. Prevalence and antibiotic susceptibility pattern of pathogens in children with urinary tract infection in a tertiary care hospital. Int J Contemporary Pediatrics. (2020) 7:1513–8. doi: 10.18203/2349-3291.ijcp20202607
215. Srinivasan R, Santhakumari S, Poonguzhali P, Geetha M, Dyavaiah M, Xiangmin L. Bacterial biofilm inhibition: a focused review on recent therapeutic strategies for combating the biofilm mediated infections. Front Microbiol. (2021) 12:676458. doi: 10.3389/fmicb.2021.676458
216. Bjarnsholt T, Kirketerp-Møller K, Jensen PØ, Madsen KG, Phipps R, Krogfelt K, et al. Why chronic wounds will not heal: a novel hypothesis. Wound Repair Regen. (2008) 16:2–10. doi: 10.1111/j.1524-475X.2007.00283.x
217. Davis KA, Moran KA, McAllister CK, Gray PJ. Multidrug-resistant Acinetobacter extremity infections in soldiers. Emerg Infect Dis. (2005) 11:1218–24. doi: 10.3201/1108.050103
218. Oncül O, Keskin O, Acar HV, Küçükardali Y, Evrenkaya R, Atasoyu EM, et al. Hospital-acquired infections following the 1999 Marmara earthquake. J Hosp Infect. (2002) 51:47–51. doi: 10.1053/jhin.2002.1205
219. Kennedy PJ, Haertsch PA, Maitz PK. The Bali burn disaster: implications and lessons learned. J Burn Care Rehabil. (2005) 26:125–31. doi: 10.1097/01.bcr.0000155532.31639.0d
220. James GA, Swogger E, Wolcott R, Pulcini Ed, Secor P, Sestrich J, et al. Biofilms in chronic wounds. Wound Repair Regen. (2008) 16:37–44. doi: 10.1111/j.1524-475X.2007.00321.x
221. Sheppard FR, Keiser P, Craft DW, Gage F, Robson M, Brown TS, et al. The majority of US combat casualty soft-tissue wounds are not infected or colonized upon arrival or during treatment at a continental US military medical facility. Am J Surg. (2010) 200:489–95. doi: 10.1016/j.amjsurg.2010.03.001
222. Nair MS, Lau P, Liu Y, Venkitanarayanan K. Efficacy of selenium in controlling Acinetobacter baumannii associated wound infections. Wound Med. (2019) 26:100165. doi: 10.1016/j.wndm.2019.100165
223. Williams DL, Kawaguchi B, Taylor NB, Allyn G, Badham MA, Rogers JC, et al. In vivo efficacy of a unique first-in-class antibiofilm antibiotic for biofilm-related wound infections caused by Acinetobacter baumannii. Biofilm. (2020) 2:100032. doi: 10.1016/j.bioflm.2020.100032
224. Fekrirad Z, Darabpour E, Kashef N. Eradication of Acinetobacter baumannii planktonic and biofilm cells through erythrosine-mediated photodynamic inactivation augmented by acetic acid and chitosan. Curr Microbiol. (2021) 78:879–86. doi: 10.1007/s00284-021-02350-x
225. Sebeny PJ, Riddle MS, Petersen K. Acinetobacter baumannii skin and soft-tissue infection associated with war trauma. Clin Infect Dis. (2008) 47:444–9. doi: 10.1086/590568
226. Guerrero DM, Perez F, Conger NG, Solomkin JS, Adams MD, Rather PN, et al. Acinetobacter baumannii -associated skin and soft tissue infections: recognizing a broadening spectrum of disease. Surg Infect. (2010) 11:49–57. doi: 10.1089/sur.2009.022
227. Sinha N, Niazi M, Lvovsky D. A fatal case of multidrug resistant acinetobacter necrotizing fasciitis: the changing scary face of nosocomial infection. Case Rep Infect Dis. (2014) 2014:705279. doi: 10.1155/2014/705279
228. Ali A, Botha J, Tiruvoipati R. Fatal skin and soft tissue infection of multidrug resistant Acinetobacter baumannii: a case report. Int J Surg Case Rep. (2014) 5:532–6. doi: 10.1016/j.ijscr.2014.04.019
229. Campoccia D, Montanaro L, Arciola CR. The significance of infection related to orthopedic devices and issues of antibiotic resistance. Biomaterials. (2006) 27:2331–9. doi: 10.1016/j.biomaterials.2005.11.044
230. Zimmerli W, Moser C. Pathogenesis and treatment concepts of orthopaedic biofilm infections. FEMS Immunol Med Microbiol. (2012) 65:158–68. doi: 10.1111/j.1574-695X.2012.00938.x
231. Staats A, Li D, Sullivan AC, Stoodley P. Biofilm formation in periprosthetic joint infections. Ann Joint. (2020) 6:43. doi: 10.21037/aoj-20-85
232. Gramatniece A, Silamikelis I, Zahare I, Urtans V, Zahare I, Dimina E, et al. Control of Acinetobacter baumannii outbreak in the neonatal intensive care unit in Latvia: whole-genome sequencing powered investigation and closure of the ward. Antimicrob Resist Infect Control. (2019) 22:8:84. doi: 10.1186/s13756-019-0537-z
233. Duenas M. Outbreak of multidrug-resistant Acinetobacter baumannii in neonatal intensive care unit in El salvador. Open Forum Infectious Diseases. (2015) 2:1778. doi: 10.1093/ofid/ofv133.1328
234. Dalili H, Nayeri F, Shariat M, Farrokhzad N, Sahebi L. The outbreak of Acinetobacter baumannii over a 19-month period in a teaching hospital Neonatal intensive care unit. Clin Pediatr OA. (2019) 4:150. doi: 10.35248/2572-0775.19.4.150
235. Chatterjee S, Datta S, Roy S, Ramanan L, Saha A, Viswanathan R, et al. Carbapenem resistance in Acinetobacter baumannii and other Acinetobacter spp. causing neonatal sepsis: focus on NDM-1 and its linkage to ISAba125. Front Microbiol. (2016) 7:1126. doi: 10.3389/fmicb.2016.01126
236. Roy S, Basu S, Dasgupta S, Singh AK, Viswanathan R. Carbapenem resistance in Acinetobacter baumannii isolated from blood of neonates with sepsis. Indian J Med Microbiol. (2010) 28:416–7. doi: 10.4103/0255-0857.71814
237. Lee SY, Lee JW, Jeong DC, Chung SY, Chung DS, Kang JH. Multidrug-resistant Acinetobacter meningitis in a 3-year-old boy treated with i.v. colistin. Pediatr Int. (2008) 50:584–5. doi: 10.1111/j.1442-200X.2008.02677.x
238. Ozaki T, Nishimura N, Arakawa Y, Suzuki M, Narita A, Yamamoto Y, et al. Community-acquired Acinetobacter baumannii meningitis in a previously healthy 14-month-old boy. J Infect Chemother. (2009) 15:322–4. doi: 10.1007/s10156-009-0704-x
239. Shah I, Kapdi M. Multidrug-resistant Acinetobacter meningitis in children. J Family Med Prim Care. (2016) 5:858–9. doi: 10.4103/2249-4863.201169
240. Xiao J, Zhang C, Ye S. Acinetobacter baumannii meningitis in children: a case series and literature review. Infection. (2019) 47:643–9. doi: 10.1007/s15010-018-1234-1
241. Ozdemir H, Tapisiz A, Ciftçi E, Ince E, Mokhtari H, Güriz H. Successful treatment of three children with post-neurosurgical multidrug-resistant Acinetobacter baumannii meningitis. Infection. (2010) 38:241–4. doi: 10.1007/s15010-010-0018-z
242. Clark R, Powers R, White R, Bloom B, Sanchez P, Benjamin DK Jr. Nosocomial infection in the NICU: a medical complication or unavoidable problem? J Perinatol. (2004) 24:382–8. doi: 10.1038/sj.jp.7211120
243. Roy S, Viswanathan R, Singh A, Das P, Basu S. Gut colonization by multidrug-resistant and carbapenem-resistant Acinetobacter baumannii in neonates. Eur J Clin Microbiol Infect Dis. (2010) 29:1495–500. doi: 10.1007/s10096-010-1030-z
244. Investigators of the Delhi Neonatal Infection Study (DeNIS) collaboration. Characterisation and antimicrobial resistance of sepsis pathogens in neonates born in tertiary care centres in Delhi, India: a cohort study. Lancet Glob Health. (2016) 4:e752–60. doi: 10.1016/S2214-109X(16)30148-6
245. Lawrence RM. Transmission of infectious diseases through breast milk and breastfeeding. Breastfeeding. (2011) 406–73. doi: 10.1016/B978-1-4377-0788-5.10013-6
246. Ciofu O, Rojo-Molinero E, Macià MD, Oliver A. Antibiotic treatment of biofilm infections. APMIS. (2017) 125:304–19. doi: 10.1111/apm.12673
247. Wang Y, Bao W, Guo N, Chen H, Cheng W, Jin K, et al. Antimicrobial activity of the imipenem/rifampicin combination against clinical isolates of Acinetobacter baumannii grown in planktonic and biofilm cultures. World J Microbiol Biotechnol. (2014) 30:3015–25. doi: 10.1007/s11274-014-1728-7
248. Song JY, Cheong HJ, Noh JY, Kim WJ. In vitro comparison of anti-biofilm effects against carbapenem-resistant Acinetobacter baumannii: imipenem, colistin, tigecycline, rifampicin and combinations. Infect Chemother. (2015) 47:27–32. doi: 10.3947/ic.2015.47.1.27
249. Wang YC, Kuo SC, Yang YS, Lee YT, Chiu CH, Chuang MF, et al. Individual or combined effects of meropenem, imipenem, sulbactam, colistin, and tigecycline on biofilm-embedded Acinetobacter baumannii and biofilm architecture. Antimicrob Agents Chemother. (2016) 60:4670–6. doi: 10.1128/AAC.00551-16
250. Peng Q, Lin F, Ling B. In vitro activity of biofilm inhibitors in combination with antibacterial drugs against extensively drug-resistant Acinetobacter baumannii. Sci Rep. (2020) 10:18097. doi: 10.1038/s41598-020-75218-y
251. Ozbek B, Mataraci E. In vitro effectiveness of colistin, tigecycline and levofloxacin alone and combined with clarithromycin and/or heparin as lock solutions against embedded Acinetobacter baumannii strains. J Antimicrob Chemother. (2013) 68:827–30. doi: 10.1093/jac/dks472
252. Cho YW, Park JH, Kim SH, Cho YH, Choi JM, Shin HJ, et al. Gentamicin-releasing urethral catheter for short-term catheterization. J Biomater Sci Polym Ed. (2003) 14:963–72. doi: 10.1163/156856203322381447
253. Darouiche RO, Smith JA Jr, Hanna H, Dhabuwala CB, Steiner MS, Babaian RJ, et al. Efficacy of antimicrobial-impregnated bladder catheters in reducing catheter-associated bacteriuria: a prospective, randomized, multicenter clinical trial. Urology. (1999) 54:976–81. doi: 10.1016/s0090-4295(99)00288-5
254. Park JH, Cho YW, Cho YH, Choi JM, Shin HJ, Bae YH, et al. Norfloxacin-releasing urethral catheter for long-term catheterization. J Biomater Sci Polym Ed. (2003) 14:951–62. doi: 10.1163/156856203322381438
255. León C, Ruiz-Santana S, Rello J, de la Torre MV, Vallés J, Alvarez-Lerma F, et al. Benefits of minocycline and rifampin-impregnated central venous catheters. A prospective, randomized, double-blind, controlled, multicenter trial. Intensive Care Med. (2004) 30:1891–9. doi: 10.1007/s00134-004-2378-2
256. Rupp ME, Lisco SJ, Lipsett PA, Perl TM, Keating K, Civetta JM, et al. Effect of a second-generation venous catheter impregnated with chlorhexidine and silver sulfadiazine on central catheter-related infections: a randomized, controlled trial. Ann Intern Med. (2005) 143:570–80. doi: 10.7326/0003-4819-143-8-200510180-00007
257. Yücel N, Lefering R, Maegele M, Max M, Rossaint R, Koch A, et al. Reduced colonization and infection with miconazole-rifampicin modified central venous catheters: a randomized controlled clinical trial. J Antimicrob Chemother. (2004) 54:1109–15. doi: 10.1093/jac/dkh483
258. Xiong YQ, Estellés A, Li L, Abdelhady W, Gonzales R, Bayer AS, et al. A human biofilm-disrupting monoclonal antibody potentiates antibiotic efficacy in rodent models of both Staphylococcus aureus and Acinetobacter baumannii infections. Antimicrob Agents Chemother. (2017) 61:e00904–17. doi: 10.1128/AAC.00904-17
259. Sperandio V. Novel approaches to bacterial infection therapy by interfering with bacteria-to-bacteria signaling. Expert Rev Anti Infect Ther. (2007) 5:271–6. doi: 10.1586/14787210.5.2.271
260. Hoang TT, Schweizer HP. Characterization of Pseudomonas aeruginosa enoyl-acyl carrier protein reductase (FabI): a target for the antimicrobial triclosan and its role in acylated homoserine lactone synthesis. J Bacteriol. (1999) 181:5489–97. doi: 10.1128/JB.181.17.5489-5497.1999
261. Choo JH, Rukayadi Y, Hwang JK. Inhibition of bacterial quorum sensing by vanilla extract. Lett Appl Microbiol. (2006) 42:637–41. doi: 10.1111/j.1472-765X.2006.01928.x
262. Rasmussen TB, Bjarnsholt T, Skindersoe ME, Hentzer M, Kristoffersen P, Köte M, et al. Screening for quorum-sensing inhibitors (QSI) by use of a novel genetic system, the QSI selector. J Bacteriol. (2005) 187:1799–814. doi: 10.1128/JB.187.5.1799-1814.2005
263. Cady NC, McKean KA, Behnke J, Kubec R, Mosier AP, Kasper SH, et al. Inhibition of biofilm formation, quorum sensing and infection in Pseudomonas aeruginosa by natural products-inspired organosulfur compounds. PLoS ONE. (2012) 7:e38492. doi: 10.1371/journal.pone.0038492
264. Stacy DM, Welsh MA, Rather PN, Blackwell HE. Attenuation of quorum sensing in the pathogen Acinetobacter baumannii using non-native N-Acyl homoserine lactones. ACS Chem Biol. (2012) 7:1719–28. doi: 10.1021/cb300351x
265. Sambanthamoorthy K, Luo C, Pattabiraman N, Feng X, Koestler B, Waters CM, et al. Identification of small molecules inhibiting diguanylate cyclases to control bacterial biofilm development. Biofouling. (2014) 30:17–28. doi: 10.1080/08927014.2013.832224
266. Young DM, Parke D, Ornston LN. Opportunities for genetic investigation afforded by Acinetobacter baylyi, a nutritionally versatile bacterial species that is highly competent for natural transformation. Annu Rev Microbiol. (2005) 59:519–51. doi: 10.1146/annurev.micro.59.051905.105823
267. Nait Chabane Y, Mlouka MB, Alexandre S, Nicol M, Marti S, Pestel-Caron M, et al. Virstatin inhibits biofilm formation and motility of Acinetobacter baumannii. BMC Microbiol. (2014) 14:62. doi: 10.1186/1471-2180-14-62
268. Oh MH, Choi CH. Role of LuxIR homologue AnoIR in acinetobacter nosocomialis and the effect of virstatin on the expression of anoR gene. J Microbiol Biotechnol. (2015) 25:1390–400. doi: 10.4014/jmb.1504.04069
269. Alam P, Alqahtani AS, Mabood HF, Tabish RM, Alajmi MF, Noman OM, et al. Siphonocholin isolated from red sea sponge Siphonochalina siphonella attenuates quorum sensing controlled virulence and biofilm formation. Saudi Pharm J. (2020) 28:1383–91. doi: 10.1016/j.jsps.2020.09.002
270. Chow JY, Yang Y, Tay SB, Chua KL, Yew WS. Disruption of biofilm formation by the human pathogen Acinetobacter baumannii using engineered quorum-quenching lactonases. Antimicrob Agents Chemother. (2014) 58:1802–5. doi: 10.1128/AAC.02410-13
271. Zhang Y, Brackman G, Coenye T. Pitfalls associated with evaluating enzymatic quorum quenching activity: the case of MomL and its effect on Pseudomonas aeruginosa and Acinetobacter baumannii biofilms. PeerJ. (2017) 5:e3251. doi: 10.7717/peerj.3251
272. Paluch E, Rewak-Soroczyńska J, Jedrusik I, Mazurkiewicz E, Jermakow K. Prevention of biofilm formation by quorum quenching. Appl Microbiol Biotechnol. (2020) 104:1871–81. doi: 10.1007/s00253-020-10349-w
273. Seo H, Kim J, Jung J, Jin HM, Jeon CO, Park W. Complexity of cell-cell interactions between Pseudomonas sp. AS1 and Acinetobacter oleivorans DR1: metabolic commensalism, biofilm formation and quorum quenching. Res Microbiol. (2012) 163:173–81. doi: 10.1016/j.resmic.2011.12.003
274. Simões LC, Simões M, Vieira MJ. Intergeneric coaggregation among drinking water bacteria: evidence of a role for Acinetobacter calcoaceticus as a bridging bacterium. Appl Environ Microbiol. (2008) 74:1259–63. doi: 10.1128/AEM.01747-07
275. Zhang J, Liang X, Zhang S, Song Z, Wang C, Xu Y. Maipomycin A, a novel natural compound with promising anti-biofilm activity against gram-negative pathogenic bacteria. Front Microbiol. (2021) 11:598024. doi: 10.3389/fmicb.2020.598024
276. Stowe SD, Richards JJ, Tucker AT, Thompson R, Melander C, Cavanagh J. Anti-biofilm compounds derived from marine sponges. Mar Drugs. (2011) 9:2010–35. doi: 10.3390/md9102010
277. Tseng SP, Hung WC, Huang CY, Lin YS, Chan MY, Lu PL, et al. 5-Episinuleptolide decreases the expression of the extracellular matrix in early biofilm formation of multi-drug resistant Acinetobacter baumannii. Mar Drugs. (2016) 14:143. doi: 10.3390/md14080143
278. King LB, Pangburn MK, McDaniel LS. Serine protease PKF of Acinetobacter baumannii results in serum resistance and suppression of biofilm formation. J Infect Dis. (2013) 207:1128–34. doi: 10.1093/infdis/jis939
279. Selvaraj A, Valliammai A, Sivasankar C, Suba M, Sakthivel G, Pandian SK. Antibiofilm and antivirulence efficacy of myrtenol enhances the antibiotic susceptibility of Acinetobacter baumannii. Sci Rep. (2020) 10:21975. doi: 10.1038/s41598-020-79128-x
280. Nazzaro F, Fratianni F, De Martino L, Coppola R, De Feo V. Effect of essential oils on pathogenic bacteria. Pharmaceuticals. (2013) 6:1451–74. doi: 10.3390/ph6121451
281. Ugur T, Cem C, Isa K, Ataş Ceylan MH. Anti-biofilm and antimicrobial activity of Mentha pulegium L essential oil against multidrug-resistant Acinetobacter baumannii. Trop J Pharm Res. (2016) 5:1039–47. doi: 10.4314/tjpr.v15i5.20
282. Celik C, Tutar U, Karaman I, Hepokur C, Atas M. Evaluation of the antibiofilm and antimicrobial properties of Ziziphora tenuior L. essential oil against multidrug-resistant Acinetobacter baumannii. Int J Pharm. (2016) 12:28–35. doi: 10.3923/ijp.2016.28.35
283. Orhan-Yanikan E, da Silva-Janeiro S, Ruiz-Rico M, Jimenez-Belenguer A, Ayhan K, Bharat JM. Essential oils compounds as antimicrobial and antibiofilm agents against strains present in the meat industry. Food Control. (2019) 101:29–38. doi: 10.1016/j.foodcont.2019.02.035
284. Mayaud L, Carricajo A, Zhiri A, Aubert G. Comparison of bacteriostatic and bactericidal activity of 13 essential oils against strains with varying sensitivity to antibiotics. Lett Appl Microbiol. (2008) 47:167–73. doi: 10.1111/j.1472-765X.2008.02406.x
285. Knezevic P, Aleksic V, Simin N, Svircev E, Petrovic A, Mimica-Dukic N. Antimicrobial activity of Eucalyptus camaldulensis essential oils and their interactions with conventional antimicrobial agents against multi-drug resistant Acinetobacter baumannii. J Ethnopharmacol. (2016) 178:125–36. doi: 10.1016/j.jep.2015.12.008
286. Moghimi R, Aliahmadi A, Rafati H, Abtahi HR, Amini S, Feizabadi MM. Antibacterialand anti-biofilm activity of nanoemulsion of Thymus daenensis oil against multi-drug resistant Acinetobacter baumannii. J Mol Liq. (2018) 265:765–70. doi: 10.1016/j.molliq.2018.07.023
287. Shivaprasad A, Antony B, Rekha B. Antibiofilm activity and the synergistic effect of lemon grass essential oil with antibiotics against A. baumannii complex – a preliminary report. EJPMR. (2019) 6:505–10.
288. Ismail MM, Samir R, Saber FR, Ahmed SR, Farag MA. Pimenta oil as a potential treatment for Acinetobacter Baumannii wound infection: in vitro and in vivo bioassays in relation to its chemical composition. Antibiotics. (2020) 9:679. doi: 10.3390/antibiotics9100679
289. Lu M, Dai T, Murray CK, Wu MX. Bactericidal property of oregano oil against multidrug-resistant clinical isolates. Front Microbiol. (2018) 9:2329. doi: 10.3389/fmicb.2018.02329 Erratum in: Front Microbiol. (2021) 12:713573.
290. Fjell CD, Hiss JA, Hancock RE, Schneider G. Designing antimicrobial peptides: form follows function. Nat Rev Drug Discov. (2011) 11:37–51. doi: 10.1038/nrd3591
291. Feng X, Sambanthamoorthy K, Palys T, Paranavitana C. The human antimicrobial peptide LL-37 and its fragments possess both antimicrobial and antibiofilm activities against multidrug-resistant Acinetobacter baumannii. Peptides. (2013) 49:131–7. doi: 10.1016/j.peptides.2013.09.007
292. Avery TM, Boone RL, Lu J, Spicer SK, Guevara MA, Moore RE, et al. Analysis of antimicrobial and antibiofilm activity of human milk lactoferrin compared to bovine lactoferrin against multidrug resistant and susceptible Acinetobacter baumannii clinical isolates. ACS Infect Dis. (2021) 7:2116–26. doi: 10.1021/acsinfecdis.1c00087
293. Mohamed MF, Brezden A, Mohammad H, Chmielewski J, Seleem MN. A short D-enantiomeric antimicrobial peptide with potent immunomodulatory and antibiofilm activity against multidrug-resistant Pseudomonas aeruginosa and Acinetobacter baumannii. Sci Rep. (2017) 7:6953. doi: 10.1038/s41598-017-07440-0
294. Kim MK, Kang N, Ko SJ, Park J, Park E, Shin DW, et al. Antibacterial and antibiofilm activity and mode of action of magainin 2 against drug-resistant Acinetobacter baumannii. Int J Mol Sci. (2018) 19:3041. doi: 10.3390/ijms19103041
295. Peng J, Long H, Liu W, Wu Z, Wang T, Zeng Z, et al. Antibacterial mechanism of peptide Cec4 against Acinetobacter baumannii. Infect Drug Resist. (2019) 12:2417–28. doi: 10.2147/IDR.S214057
296. Gordya N, Yakovlev A, Kruglikova A, Tulin D, Potolitsina E, Suborova T, et al. Natural antimicrobial peptide complexes in the fighting of antibiotic resistant biofilms: Calliphora vicina medicinal maggots. PLoS ONE. (2017) 12:e0173559. doi: 10.1371/journal.pone.0173559
297. Jaśkiewicz M, Neubauer D, Kazor K, Bartoszewska S, Kamysz W. Antimicrobial activity of selected antimicrobial peptides against planktonic culture and biofilm of Acinetobacter baumannii. Probiotics Antimicrob Proteins. (2019) 11:317–24. doi: 10.1007/s12602-018-9444-5
298. Gopal R, Kim YG, Lee JH, Lee SK, Chae JD, Son BK, et al. Synergistic effects and antibiofilm properties of chimeric peptides against multidrug-resistant Acinetobacter baumannii strains. Antimicrob Agents Chemother. (2014) 58:1622–9. doi: 10.1128/AAC.02473-13
299. Swedan S, Shubair Z, Almaaytah A. Synergism of cationic antimicrobial peptide WLBU2 with antibacterial agents against biofilms of multi-drug resistant Acinetobacter baumannii and Klebsiella pneumoniae. Infect Drug Resist. (2019) 12:2019–30. doi: 10.2147/IDR.S215084
300. Mwangi J, Yin Y, Wang G, Yang M, Li Y, Zhang Z, et al. The antimicrobial peptide ZY4 combats multidrug-resistant Pseudomonas aeruginosa and Acinetobacter baumannii infection. Proc Natl Acad Sci USA. (2019) 116:26516–22. doi: 10.1073/pnas.1909585117
301. de Breij A, Riool M, Cordfunke RA, Malanovic N, de Boer L, Koning RI, et al. The antimicrobial peptide SAAP-148 combats drug-resistant bacteria and biofilms. Sci Transl Med. (2018) 10:eaan4044. doi: 10.1126/scitranslmed.aan4044
302. Di Somma A, Recupido F, Cirillo A, Romano A, Romanelli A, Caserta S, et al. Antibiofilm properties of temporin-L on Pseudomonas fluorescens in static and in-flow conditions. Int J Mol Sci. (2020) 21:8526. doi: 10.3390/ijms21228526
303. Reza A, Sutton JM, Rahman KM. Effectiveness of efflux pump inhibitors as biofilm disruptors and resistance breakers in gram-negative (ESKAPEE) bacteria. Antibiotics. (2019) 8:229. doi: 10.3390/antibiotics8040229
304. Chen L, Li H, Wen H, Zhao B, Niu Y, Mo Q, et al. Biofilm formation in Acinetobacter baumannii was inhibited by PAβN while it had no association with antibiotic resistance. Microbiologyopen. (2020) 9:e1063. doi: 10.1002/mbo3.1063
305. Blanchard C, Barnett P, Perlmutter J, Dunman PM. Identification of Acinetobacter baumannii serum-associated antibiotic efflux pump inhibitors. Antimicrob Agents Chemother. (2014) 58:6360–70. doi: 10.1128/AAC.03535-14
306. Yilmaz S, Altinkanat-Gelmez G, Bolelli K, Guneser-Merdan D, Over-Hasdemir MU, Yildiz I, et al. Pharmacophore generation of 2-substituted benzothiazoles as AdeABC efflux pump inhibitors in A. baumannii. SAR QSAR Environ Res. (2014) 25:551–63. doi: 10.1080/1062936X.2014.919357
307. Krishnamoorthy S, Shah BP, Lee HH, Martinez LR. Microbicides alter the expression and function of RND-type efflux pump AdeABC in biofilm-associated cells of Acinetobacter baumannii clinical isolates. Antimicrob Agents Chemother. (2015) 60:57–63. doi: 10.1128/AAC.01045-15
308. Wang L, Hu C, Shao L. The antimicrobial activity of nanoparticles: present situation and prospects for the future. Int J Nanomedicine. (2017) 12:1227–49. doi: 10.2147/IJN.S121956
309. Mihu MR, Sandkovsky U, Han G, Friedman JM, Nosanchuk JD, Martinez LR. The use of nitric oxide releasing nanoparticles as a treatment against Acinetobacter baumannii in wound infections. Virulence. (2010) 1:62–7. doi: 10.4161/viru.1.2.10038
310. Hwang YY, Ramalingam K, Bienek DR, Lee V, You T, Alvarez R. Antimicrobial activity of nanoemulsion in combination with cetylpyridinium chloride in multidrug-resistant Acinetobacter baumannii. Antimicrob Agents Chemother. (2013) 57:3568–75. doi: 10.1128/AAC.02109-12
311. Salunke GR, Ghosh S, Santosh Kumar RJ, Khade S, Vashisth P, Kale T, et al. Rapid efficient synthesis and characterization of silver, gold, and bimetallic nanoparticles from the medicinal plant Plumbago zeylanica and their application in biofilm control. Int J Nanomedicine. (2014) 9:2635–53. doi: 10.2147/IJN.S59834
312. Gaidhani S, Singh R, Singh D, Patel U, Shevade K, Yeshvekar R, et al. Biofilm disruptionactivity of silver nanoparticles synthesized by Acinetobacter calcoaceticus PUCM 1005. Mater Lett. (2013) 108:324–7. doi: 10.1016/j.matlet.2013.07.023
313. Martinez-Gutierrez F, Boegli L, Agostinho A, Sánchez EM, Bach H, Ruiz F, et al. Anti-biofilm activity of silver nanoparticles against different microorganisms. Biofouling. (2013) 29:651–60. doi: 10.1080/08927014.2013.794225
314. Ramya S, Shanmugasundaram T, Balagurunathan R. Biomedical potential of actinobacterially synthesized selenium nanoparticles with special reference to anti-biofilm, anti-oxidant, wound healing, cytotoxic and anti-viral activities. J Trace Elem Med Biol. (2015) 32:30–9. doi: 10.1016/j.jtemb.2015.05.005
315. Hosseini A, Nejadsattari T, Zargar M. In vitro anti-biofilm activity of curcumin nanoparticles in Acinetobacter baumannii: a culture-based and molecular approach. Arch Clin Infect Dis. (2019) 14:e83263. doi: 10.5812/archcid.83263
316. Muzammil S, Khurshid M, Nawaz I, Siddique MH, Zubair M, Nisar MA, et al. Aluminium oxide nanoparticles inhibit EPS production, adhesion and biofilm formation by multidrug resistant Acinetobacter baumannii. Biofouling. (2020) 36:492–504. doi: 10.1080/08927014.2020.1776856
317. Hendiani S, Abdi AA, Mohammadi P, Kharrazi Sh. Synthesis of silver nanoparticles and its synergistic effects in combination with imipenem and two biocides against biofilm producing Acinetobacter baumannii. Nanomed J. (2015) 2:291–8. doi: 10.7508/NMJ.2015.04.007
318. Tran HM, Tran H, Booth MA, Fox KE, Nguyen TH, Tran N, et al. Nanomaterials for treating bacterial biofilms on implantable medical devices. Nanomaterials. (2020) 10:2253. doi: 10.3390/nano10112253
319. Thawal ND, Yele AB, Sahu PK, Chopade BA. Effect of a novel podophage AB7-IBB2 on Acinetobacter baumannii biofilm. Curr Microbiol. (2012) 65:66–72. doi: 10.1007/s00284-012-0127-2
320. Yele AB, Thawal ND, Sahu PK, Chopade BA. Novel lytic bacteriophage AB7-IBB1 of Acinetobacter baumannii: isolation, characterization and its effect on biofilm. Arch Virol. (2012) 157:1441–50. doi: 10.1007/s00705-012-1320-0
321. Liu Y, Mi Z, Niu W, An X, Yuan X, Liu H, et al. Potential of a lytic bacteriophage to disrupt Acinetobacter baumannii biofilms in vitro. Future Microbiol. (2016) 11:1383–93. doi: 10.2217/fmb-2016-0104
322. Lood R, Winer BY, Pelzek AJ, Diez-Martinez R, Thandar M, Euler CW, et al. Novel phage lysin capable of killing the multidrug-resistant gram-negative bacterium Acinetobacter baumannii in a mouse bacteremia model. Antimicrob Agents Chemother. (2015) 59:1983–91. doi: 10.1128/AAC.04641-14
323. Thandar M, Lood R, Winer BY, Deutsch DR, Euler CW, Fischetti VA. Novel engineered peptides of a phage lysin as effective antimicrobials against multidrug-resistant Acinetobacter baumannii. Antimicrob Agents Chemother. (2016) 60:2671–9. doi: 10.1128/AAC.02972-15
324. Vukotic G, Obradovic M, Novovic K, Di LM, Jovcic B, Fira D, et al. Characterization, antibiofilm, and depolymerizing activity of two phages active on carbapenem-resistant Acinetobacter baumannii. Front Med. (2020) 7:426. doi: 10.3389/fmed.2020.00426
325. Grygorcewicz B, Wojciuk B, Roszak M, Łubowska N, Błazejczak P, Jursa-Kulesza J, et al. Environmental phage-based cocktail and antibiotic combination effects on Acinetobacter baumannii biofilm in a human urine model. Microb Drug Resist. (2021) 27:25–35. doi: 10.1089/mdr.2020.0083
326. Ran B, Yuan Y, Xia W, Li M, Yao Q, Wang Z, et al. A photo-sensitizable phage for multidrug-resistant Acinetobacter baumannii therapy and biofilm ablation. Chem Sci. (2020) 12:1054–61. doi: 10.1039/d0sc04889e
327. Shakeri S, Kermanshahi RK, Moghaddam MM, Emtiazi G. Assessment of biofilm cell removal and killing and biocide efficacy using the microtiter plate test. Biofouling. (2007) 23:79–86. doi: 10.1080/08927010701190011
328. Perumal PK, Wand ME, Sutton JM, Bock LJ. Evaluation of the effectiveness of hydrogen-peroxide-based disinfectants on biofilms formed by gram-negative pathogens. J Hosp Infect. (2014) 87:227–33. doi: 10.1016/j.jhin.2014.05.004
329. Stowe SD, Thompson RJ, Peng L, Su Z, Blackledge MS, Draughn GL, et al. Membrane-permeabilizing activity of reverse-amide 2-aminoimidazole antibiofilm agents against Acinetobacter baumannii. Curr Drug Deliv. (2015) 12:223–30. doi: 10.2174/1567201811666140924125740
330. Narayanan A, Nair MS, Karumathil DP, Baskaran SA, Venkitanarayanan K, Amalaradjou MA. Inactivation of Acinetobacter baumannii biofilms on polystyrene, stainless steel, and urinary catheters by octenidine dihydrochloride. Front Microbiol. (2016) 7:847. doi: 10.3389/fmicb.2016.00847
331. Burcu S, Burak A, Yagci AK. Biofilm production and biocidal efficacy in multidrug-resistant Pseudomonas aeruginosa and Acinetobacter baumannii isolates. Int J Antisepsis Disinfection Sterilization. (2016) 1:7–12. doi: 10.14744/ijads.2016.08208
332. Runci F, Bonchi C, Frangipani E, Visaggio D, Visca P. Acinetobacter baumannii biofilm formation in human serum and disruption by gallium. Antimicrob Agents Chemother. (2016) 61:e01563–16. doi: 10.1128/AAC.01563-16
333. Fleeman R, Van Horn KS, Barber MM, Burda WN, Flanigan DL, Manetsch R, et al. Characterizing the antimicrobial activity of N2, N4-Disubstituted quinazoline-2,4-diamines toward multidrug-resistant Acinetobacter baumannii. Antimicrob Agents Chemother. (2017) 61:e00059–17. doi: 10.1128/AAC.00059-17
334. Schumm K, Lam TB. Types of urethral catheters for management of short-term voiding problems in hospitalized adults: a short version Cochrane review. Neurourol Urodyn. (2008) 27:738–46. doi: 10.1002/nau.20645
335. Johnson JR, Kuskowski MA, Wilt TJ. Systematic review: antimicrobial urinary catheters to prevent catheter-associated urinary tract infection in hospitalized patients. Ann Intern Med. (2006) 144:116–26. doi: 10.7326/0003-4819-144-2-200601170-00009
336. Hu X, Huang YY, Wang Y, Wang X, Hamblin MR. Antimicrobial photodynamic therapy to control clinically relevant biofilm infections. Front Microbiol. (2018) 9:1299. doi: 10.3389/fmicb.2018.01299
337. Ghorbanzadeh R, Assadian H, Chiniforush N, Parker S, Pourakbari B, Ehsani B, et al. Modulation of virulence in Enterococcus faecalis cells surviving antimicrobial photodynamic inactivation with reduced graphene oxide-curcumin: an ex vivo biofilm model. Photodiagnosis Photodyn Ther. (2020) 29:101643. doi: 10.1016/j.pdpdt.2019.101643
338. Geralde MC, Leite IS, Inada NM, Salina AC, Medeiros AI, Kuebler WM, et al. Pneumonia treatment by photodynamic therapy with extracorporeal illumination - an experimental model. Physiol Rep. (2017) 5:e13190. doi: 10.14814/phy2.13190
339. Granick MS, Paribathan C, Shanmugam M, Ramasubbu N. Direct-contact low-frequency ultrasound clearance of biofilm from metallic implant materials. Eplasty. (2017) 17:e13.
340. Ng E, Lim LP. An overview of different interdental cleaning aids and their effectiveness. Dent J. (2019) 7:56. doi: 10.3390/dj7020056
341. Li X, Sun L, Zhang P, Wang Y. Novel approaches to combat medical device-associated biofilms. Coatings. (2021) 1:294. doi: 10.3390/coatings11030294
342. Li H, Fairfax MR, Dubocq F, Darouiche RO, Rajpurkar A, Thompson M, et al. Antibacterial activity of antibiotic coated silicone grafts. J Urol. (1998) 160:1910–3.
343. Nielsen CK, Subbiahdoss G, Zeng G, Salmi Z, Kjems J, Mygind T, et al. Antibacterial isoeugenol coating on stainless steel and polyethylene surfaces prevents biofilm growth. J Appl Microbiol. (2018) 124:179–87. doi: 10.1111/jam.13634
344. John T, Rajpurkar A, Smith G, Fairfax M, Triest J. Antibiotic pretreatment of hydrogel ureteral stent. J Endourol. (2007) 21:1211–6. doi: 10.1089/end.2007.9904
345. Piozzi A, Francolini I, Occhiaperti L, Di Rosa R, Ruggeri V, Donelli G. Polyurethanes loaded with antibiotics: influence of polymer-antibiotic interactions on in vitro activity against Staphylococcus epidermidis. J Chemother. (2004) 16:446–52. doi: 10.1179/joc.2004.16.5.446
346. Roy S, Chatterjee S, Bhattacharjee A, Chattopadhyay P, Saha B, Dutta S, et al. Overexpression of efflux pumps, mutations in the pumps' regulators, chromosomal mutations, and AAC(6')-Ib-cr are associated with fluoroquinolone resistance in diverse sequence types of neonatal septicaemic Acinetobacter baumannii: a 7-year single center study. Front Microbiol. (2021) 12:602724. doi: 10.3389/fmicb.2021.602724
347. Sands K, Carvalho MJ, Portal E, Thomson K, Dyer C, Akpulu C, et al. Characterization of antimicrobial-resistant gram-negative bacteria that cause neonatal sepsis in seven low- and middle-income countries. Nat Microbiol. (2021) 6:512–23. doi: 10.1038/s41564-021-00870-7
348. Al Atrouni A, Joly-Guillou ML, Hamze M, Kempf M. Reservoirs of non-baumannii acinetobacter species. Front Microbiol. (2016) 7:49. doi: 10.3389/fmicb.2016.00049
349. Tyers M, Wright GD. Drug combinations: a strategy to extend the life of antibiotics in the 21st century. Nat Rev Microbiol. (2019) 17:141–55. doi: 10.1038/s41579-018-0141-x
350. Asahara T, Takahashi A, Yuki N, Kaji R, Takahashi T, Nomoto K. Protective effect of a synbiotic against multidrug-resistant Acinetobacter baumannii in a murine infection model. Antimicrob Agents Chemother. (2016) 60:3041–50. doi: 10.1128/AAC.02928-15
351. Parra Millán R, Jiménez Mejías ME, Sánchez Encinales V, Ayerbe Algaba R, Gutiérrez Valencia A, Pachón Ibáñez ME, et al. Efficacy of lysophosphatidylcholine in combination with antimicrobial agents against Acinetobacter baumannii in experimental murine peritoneal sepsis and pneumonia models. Antimicrob Agents Chemother. (2016) 60:4464–70. doi: 10.1128/AAC.02708-15
Keywords: Acinetobacter baumannii, antimicrobial resistance, biofilm regulation, biofilm-associated infections, adult, paediatric, biofilm prevention
Citation: Roy S, Chowdhury G, Mukhopadhyay AK, Dutta S and Basu S (2022) Convergence of Biofilm Formation and Antibiotic Resistance in Acinetobacter baumannii Infection. Front. Med. 9:793615. doi: 10.3389/fmed.2022.793615
Received: 12 October 2021; Accepted: 31 January 2022;
Published: 24 March 2022.
Edited by:
Naveen Kumar Devanga Ragupathi, The University of Sheffield, United KingdomReviewed by:
Jose Ramos-Vivas, Universidad Europea del Atlántico, SpainM. S. Dhiviya Prabaa, Christian Medical College & Hospital, India
Copyright © 2022 Roy, Chowdhury, Mukhopadhyay, Dutta and Basu. This is an open-access article distributed under the terms of the Creative Commons Attribution License (CC BY). The use, distribution or reproduction in other forums is permitted, provided the original author(s) and the copyright owner(s) are credited and that the original publication in this journal is cited, in accordance with accepted academic practice. No use, distribution or reproduction is permitted which does not comply with these terms.
*Correspondence: Sulagna Basu, YmFzdXMubmljZWQmI3gwMDA0MDtnb3YuaW4=; c3VwYWJhc3UmI3gwMDA0MDt5YWhvby5jby5pbg==
†These authors have contributed equally to this work